- 1Environmental Studies Program, The Evergreen State College, Olympia, WA, United States
- 2Fish Ecology Division, National Marine Fisheries Service, National Oceanic and Atmospheric Association, Seattle, WA, United States
- 3U.S. Geological Survey, Western Fisheries Research Center, Seattle, WA, United States
- 4U.S. Fish and Wildlife Service, Western Washington Fish and Wildlife Conservation Office, Lacey, WA, United States
- 5Clallam County Department of Community Development, Port Angeles, WA, United States
- 6Tribal Environmental Quality Program, Lower Elwha Klallam Tribe, Port Angeles, WA, United States
- 7U.S. Geological Survey, Pacific Coastal and Marine Science Center, Santa Cruz, CA, United States
- 8Resilient Landscapes Program, San Francisco Estuary Institute, Richmond, CA, United States
Large-scale dam removals provide opportunities to restore river function in the long-term and are massive disturbances to riverine ecosystems in the short-term. The removal of two dams on the Elwha River (WA, USA) between 2011 and 2014 was the largest dam removal project to be completed by that time and has since resulted in major changes to channel dynamics, river substrates, in-stream communities, and the size and shape of the river delta. To assess ecosystem function across the restored Elwha watershed, we compared leaf litter decomposition at twenty sites: 1) four tributary sites not influenced by restoration activities; 2) four river sites downstream of the upper dam (Glines Canyon Dam); 3) four river sites within the footprint of the former Aldwell Reservoir upstream of the lower dam (Elwha Dam); 4) four river sites downstream of the lower dam; and 5) four lentic sites in the newly developing Elwha delta. Three major findings emerged: 1) decomposition rates differed among sections of the Elwha watershed, with slowest decomposition rates at the delta sites and fastest decomposition rates just downstream of the upper dam; 2) aquatic macroinvertebrate communities establishing in leaf litterbags differed significantly among sections of the Elwha watershed; and 3) aquatic fungal communities growing on leaf litter differed significantly among sections. Aquatic macroinvertebrate and fungal diversity were sensitive to differences in canopy cover, water chemistry, and river bottom sediments across sites, with a stronger relationship to elevation for aquatic macroinvertebrates. As the Elwha River undergoes recovery following the massive sediment flows associated with dam removal, we expect to see changes in leaf litter processing dynamics and shifts in litter-dependent decomposer communities (both fungal and invertebrate) involved in this key ecosystem process.
1 Introduction
Dams have long been known to negatively influence river systems (Stanford and Ward, 2001; Morley et al., 2008; Pess et al., 2008; Colas et al., 2016), but in recent decades, as dams are decommissioned and removed, there are both short-term disturbances caused by dam removal, as well as long-term benefits of reconnected watersheds (Bednarek, 2001; Stanley and Doyle, 2003; Duda et al., 2016; Bellmore et al., 2019; Ding et al., 2019; Morley et al., 2020; Atristain et al., 2023). Dams impede the flow of water, sediment, and organic matter from upstream to downstream reaches (Minear and Kondolf, 2009; Atristain et al., 2023), causing changes upstream of dams to flow velocity, stream water temperature, and the deposition of sediment and organic material (Warrick et al., 2015; Warrick et al., 2019). Downstream, dams often create sediment starvation conditions, reduce discharge, increase velocity, reduce allochthonous material transport (Salomão et al., 2019; Tabucanon et al., 2019; Li et al., 2020; Kasahara et al., 2022), scour the riverbed, and alter nutrient cycling (Maavara et al., 2020). During and following dam removals, watersheds can experience significantly greater sediment and organic matter loads as material deposited in reservoirs is mobilized and transported downstream (Bednarek, 2001; Foley et al., 2015; Foley et al., 2017a; Peters et al., 2017; Bellmore et al., 2019), contributing a greater proportion of finer-grained sediments than before dam removal (Kibler et al., 2011; Tullos et al., 2014; East et al., 2018). Dam removal can flood sediment- and resource-starved reaches with excessive sediment and potentially either overwhelm decomposer communities with too much organic material or create anoxic conditions throughout watersheds, both of which could alter rates of organic matter processing (Muehlbauer et al., 2009).
Organic matter processing is a key ecosystem function that links terrestrial and aquatic communities in riverine habitats (Cummins et al., 1973; Benfield, 1996; Wallace et al., 1997) and has been predicted to show longitudinal patterns in riverine systems according to the River Continuum Concept (RCC; Vannote et al., 1980), with modifications predicted by the Riverine Productivity Model (RPM; Thorp and Delong, 1994). Rivers and streams partially depend on allochthonous inputs of organic matter from riparian forests, especially in forested headwaters, along tributaries, and when in-stream autochthonous (algal) resources are diminished. However, inputs of organic matter from the floodplain, side channels, and tributaries can also provide valuable resources for larger rivers (Junk et al., 1989; Thorp and Delong, 1994). Inputs of woody debris (Flory and Milner, 1999; Milner and Gloyne-Phillips, 2005; Wootton, 2012), leaf litter (Cummins et al., 1973; Petersen and Cummins, 1974; Webster and Benfield, 1986), and plant reproductive structures (Flory and Milner, 1999; Garthwaite et al., 2021) are all important resources for a variety of in-stream decomposers (microbial and invertebrate) and support stream food webs (Cummins and Klug, 1979; Cummins et al., 1989; Graça, 2001; Hayer et al., 2022). The establishment of microbial communities on organic matter conditions tissues and alters stoichiometry, making the organic matter more nutritious for macroinvertebrate shredders, grazers, and collectors that feed on biofilms on leaf surfaces (Arias-Real et al., 2018). Finally, the processing of organic matter and breakdown of material from coarse particulate organic matter (CPOM; leaves, flowers, twigs, wood) to fine particulate organic matter (FPOM; leaf and wood fragments, feces) feeds a diverse downstream community of filtering and collecting organisms (Kaushik and Hynes, 1971; Cummins and Klug, 1979; Vannote et al., 1980; Petersen et al., 1989). Organic matter decomposition rates are predicted to be fastest in shady headwaters with macroinvertebrate communities dominated by shredders, and slower in larger reaches of rivers dominated by collectors and grazers (Vannote et al., 1980). However, decomposition rates may still be relatively fast along the edges of large rivers where woody debris entrains litter, benthic macroinvertebrates have peak densities (Thorp and Delong, 1994), and some studies have reported high percentages of shredders (Chauvet, 1997). Processing of organic matter is predicted to be slow in large order rivers where sediment deposition leads to anoxic and unstable conditions (Thorp and Delong, 1994) and physical fragmentation rates may be lower (Chauvet, 1997).
Dam removals can be large but temporary disturbances, and the release of sediment and organic matter may alter environmental conditions for decomposers as well as the available stocks of organic matter for processing (Muehlbauer et al., 2009; Atristain et al., 2023). After 100 years of impoundment, two dams on the Elwha River (WA, USA) accumulated an estimated 21 million m3 of sediment, of which ~50% was fine organic and inorganic particles (Warrick et al., 2019). The removal of these dams was the largest controlled-sediment release in history, and it occurred in concurrent increments between 2011 to 2014 to balance the severity and duration of sediment pulses during key life stages for salmon. Throughout 2011–2016, large sediment pulses altered substrate and organic matter stocks throughout the Elwha watershed (Warrick et al., 2015; Ritchie et al., 2018; Morley et al., 2020), and shifting water quality parameters showed greater turbidity, nitrate, ammonium, and phosphate (Foley et al., 2017b). At the same time, anadromous fish were able to access upstream spawning areas for the first time in a century.
In this study, we examined how leaf litter decomposition varied across different sections of the Elwha River following the sediment-pulse stage of dam removal. Our primary response variables were litter decomposition rate and associated macroinvertebrate and fungal community composition. Due to the mosaic of effects during and following dam removal throughout the watershed, we hypothesized that: 1) decomposition rates would differ throughout the watershed, with fastest rates in low-order tributaries and slowest rates at the new Elwha delta (as predicted by the RCC and RPM and based on expected high rates of sediment deposition at downstream locations); 2) decomposition rates would be influenced by environmental variables across sites (for example, decomposition rates may be negatively influenced by fine sediment deposition and positively influenced by numbers of shredders and temperature); 3) decomposer communities (fungal and invertebrate) would differ among sections of the watershed, with highest diversity at tributary sites and lowest diversity in the new Elwha delta (as predicted by the RCC and RPM and based on expected high rates of sediment deposition at downstream locations); and 4) decomposer community metrics would be influenced by environmental variables across sites, but the variables influencing fungi and macroinvertebrates may vary.
2 Materials and methods
2.1 Site description
The Elwha River basin ranges in elevation from approximately 1372 m at the headwaters inside Olympic National Park to sea level at the delta, where it drains into the Strait of Juan de Fuca (Figure 1). The Elwha drains a large, mostly protected watershed of over 833 km2, 83% of which resides inside Olympic National Park. The geology of the watershed consists of sandstone/shale bedrock in the upper basin and alluvial deposits/glacial till in the lower basin (Duda et al., 2008). Riparian forest vegetation includes bigleaf maple (Acer macrophyllum Pursh), red alder (Alnus rubra Bong.), Douglas fir (Pseudotsuga menziesii [Mirb.] Franco), western redcedar (Thuja plicata Donn ex D. Don), western hemlock (Tsuga heterophylla [Raf.] Sarg.), and various shrub understory assemblages.
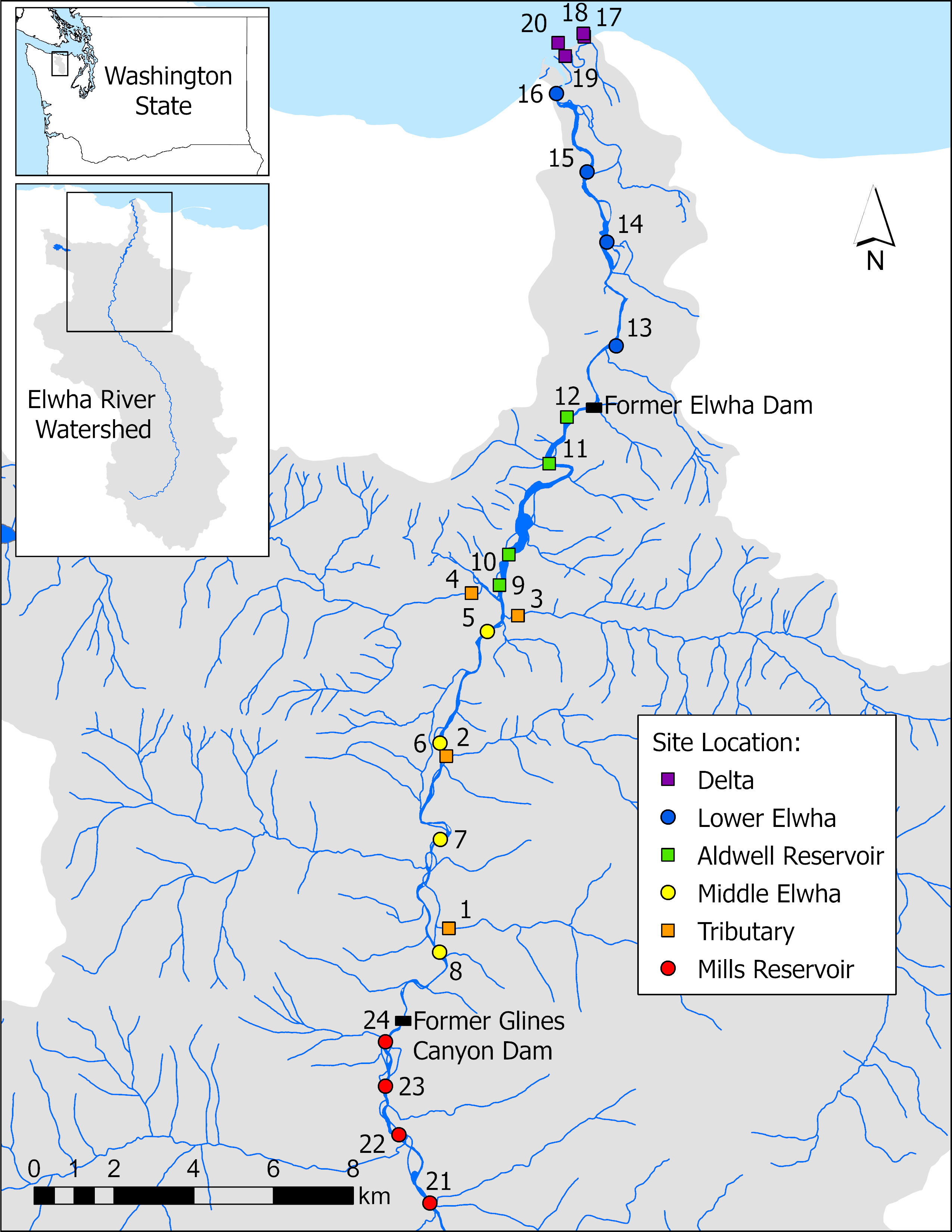
Figure 1 Map of all 24 study locations across six sections of the Elwha River watershed following the removal of two major dams: 1) Orange squares: four tributary sites not physically influenced by restoration activities (Griff Creek, Lower Little River, Indian Creek, and Madison Creek); 2) Yellow circles: four river sites downstream of the upper Glines Canyon Dam; 3) Green squares: four river sites within the footprint of the former Aldwell Reservoir upstream of the lower Elwha Dam; 4) Blue circles: four river sites downstream of the lower Elwha Dam; and 5) Purple squares: four lentic sites in the newly developing Elwha delta (freshwater sites, not brackish). An additional four sites (Red circles) were located in the Elwha River upstream of the Glines Canyon Dam in the former Mills reservoir footprint and were used for only a subset of this study (see Section 2.2).
This study took place at 20 locations throughout the Elwha watershed (Figure 1; four additional sites were used for just a subset of the study) distributed across five distinct river sections: 1) four tributary sites not physically influenced by restoration activities (Griff Creek, Lower Little River, Indian Creek, and Madison Creek); 2) four river sites downstream of the upper Glines Canyon Dam; 3) four river sites within the footprint of the former Aldwell Reservoir upstream of the lower Elwha Dam; 4) four river sites downstream of the lower Elwha Dam; and 5) four lentic sites in the newly developing Elwha delta (freshwater sites, not brackish). An additional four sites were located in the Elwha River upstream of the Glines Canyon Dam in the former Mills reservoir footprint and were used for only a subset of this study due to issues with access (see Section 2.2). Leaf litter experiments were carried out in the autumn of 2016 and 2017, with decomposition rates calculated in 2016 and fungal litter colonization assessed in 2017. The study was designed with both decomposition rates and fungal colonization to take place in 2016, but a freezer failure destroyed fungal samples from 2016 and new samples were incubated for fungal establishment in 2017.
2.2 Leaf litter decomposition study
In fall of 2016, we examined bigleaf maple (A. macrophyllum) litter decomposition rates at the 20 primary sites within the Elwha River watershed post-dam removal. Bigleaf maple was chosen as a litter source because it is common throughout the Elwha watershed and it has moderate decomposition rates (not as fast as alder, not as slow as conifer needles, both of which are also common throughout the watershed). Naturally abscised leaves were collected from bigleaf maple stands at 5 source locations within 5 km of the Elwha River. Leaves were air-dried, petioles were removed, and 4.00 g +/− 0.10 g quantities were placed into coarse-mesh (6.4-mm openings) litterbags to allow invertebrate access (n = 60 bags per maple source; N = 300). During litterbag preparation, we counted the number of maple tar spots (Rhytisma punctatum, a common fungal endophyte) on leaves in each bag because this fungal infection has been shown to influence decomposition rates in other studies (LeRoy et al., 2011; Wolfe et al., 2019). Litterbags were then randomly assigned both a harvest date and one of 20 locations within the five sections in the Elwha watershed. Fifteen litterbags were placed at each site in pools near monitored riffles, attached to 2-m rings of steel cable, and anchored into place using sandbags. Litterbags were placed in late August 2016, and five replicate litter bags were harvested from each location after 2, 5, and 6 weeks (specific dates varied slightly depending on sample location and retrieval logistics; final harvest date was determined by the onset of high flows). Harvested litterbags were placed into individual polyethylene zipper bags and transported on ice to the laboratory for processing.
Litterbags were processed within 12 hours of harvesting. Sediment and macroinvertebrates were rinsed from leaf fragments and sieved through 250-μm nets for preservation in 70% ethanol. At the week 5 harvest, leaf punches were collected for microbial analysis (but those samples were lost in a freezer accident, so we repeated this aspect of the study in 2017 for fungal amplicon sequencing, see below). Remaining leaf material was oven-dried at 70°C for 72 hours then ground, and 0.25 g subsamples were combusted at 500°C for one hour to determine ash-free dry mass (AFDM). A follow-up incubation of additional litterbags (n = 4) at each of the full 24 locations (including the former Lake Mills Reservoir) was used to evaluate fungal colonization of leaf litter in August–September 2017 (with 1-week and 3-week incubations, see Section 2.4).
2.3 Aquatic macroinvertebrates
Preserved macroinvertebrate samples from the first harvest date were sorted into three major categories for further identification: EPT taxa (Ephemeroptera, Plecoptera, Trichoptera), other insects (members of families other than EPT), and non-insects (i.e., Arachnida, Oligochaeta, Mollusca, and other non-insect invertebrates). Functional feeding groups were determined for each taxon. Litterbags at all locations retained sufficient leaf litter at the first harvest date to provide substrate, habitat, and food resources for invertebrates. All macroinvertebrate identifications were made using a dissecting microscope, to the lowest taxonomic level possible (typically genus to family for insects and class to order for non-insects) using Merritt et al. (2019) and Thorp and Covich (2009). Samples from locations 9 and 10 were accidentally combined during processing and samples from locations 14 and 15 were so large they were subsampled (25% was sorted and identified and then estimated to the whole sample size). Prior to analysis, rare taxa were consolidated at the family level. Reference specimens are stored in the LeRoy Aquatic Ecology Lab at The Evergreen State College.
2.4 Fungal amplicon sequencing
Subsamples (25 mg) of lyophilized leaf litter from litterbags collected on two harvest dates (1‐ and 3‐week 2017 litterbag incubations at 24 locations) were weighed into vials, and microbial DNA was extracted using a DNeasy PowerSoil Pro kit (Qiagen) following the manufacturer’s instructions. Subsamples were transferred to 96-well plates along with controls to detect contamination during bacterial and fungal library preparation.
Genomic DNA was amplified using an ITS barcoded primer set, adapted for the HiSeq2000 and MiSeq systems (Illumina). These primers were designed by Kabir Peay’s laboratory at Stanford University (Smith and Peay, 2014). The reverse amplification primer also contained a twelve base barcode sequence that supports pooling of up to 2,167 different samples in each lane (Caporaso et al., 2011; Caporaso et al., 2012). Each 25 µL PCR reaction contained 9.5 µL of PCR Water (MO BIO, certified DNA-Free), 12.5 µL of AccuStart II PCR ToughMix (QuantaBio, 2× concentration, 1× final), 1 µL Golay barcode tagged Forward Primer (5 µM concentration, 200 pM final), 1 µL Reverse Primer (5 µM concentration, 200 pM final), and 1 µL of template DNA. The conditions for PCR were as follows: 94°C for 3 min to denature the DNA, with 35 cycles at 94 °C for 45 s, 50 °C for 60 s, and 72 °C for 90 s; with a final extension of 10 min at 72 °C to ensure complete amplification. Amplicons were quantified using PicoGreen (Invitrogen) and a plate reader. Once quantified, different volumes of each of the products were pooled into a single tube so that each amplicon was represented equally. This pool was then cleaned up using AMPure XP Beads (Beckman Coulter), and then quantified using a fluorometer (Qubit, Invitrogen). After quantification, the molarity of the pool was determined and diluted down to 2 nM, denatured, and then diluted to a final concentration of 6.75 pM with a 10% PhiX spike for 2 × 251 bp sequencing on the Illumina MiSeq (Argonne National Laboratories).
Sequences were demultiplexed at Argonne National Laboratories using QIIME (Bolyen et al., 2019). Similar sequences were assigned to operational taxonomic units (OTUs) by clustering sequences at a 97% similarity threshold with reference to the UNITE full fungal database (Nilsson et al., 2019). OTUs were filtered to remove singletons and summarized to list taxonomic levels down to species. Unassigned taxa were filtered from the OTU table in QIIME. A phyloseq object containing the OTU-by-sample matrix, sample metadata, and taxonomic information was combined for downstream analyses (McMurdie and Holmes, 2013). We removed OTUs that were present in less than 1% of samples and normalized for variable sequencing depth by calculating the proportional abundance of OTUs within each sample (McMurdie and Holmes, 2014) prior to community analysis.
2.5 Environmental covariates
Study sites were measured in the summer/fall of 2016 and 2017 for a variety of in-stream habitat, water quality, and biological variables (Table S1) following the methods of Morley et al. (2008; 2020) and Duda et al. (2011); for more details, please see these previous studies. Briefly we measured wetted width (m using a laser range finder, Impulse), depth at sample location (cm), canopy cover (measured using a modified convex spherical densiometer, %), benthic chlorophyll-a (for algae scrubbed and rinsed from five cobbles per site, filtered onto 47 mm glass fiber filters [1 µm pore size] and measured using fluorometry, μg cm−2), water temperature and specific conductivity (measured using a model 85 multiprobe, YSI, °C and μS cm−2, respectively), total N, total P, NO3−-N, NH4+-N, NO2− N, PO43−-P, and SiO44−-Si (μg L−1; measured using a continuous flow RFA/2 system, Alpkem), fine sediments and substrate diameters (measured using pebble counts, % and D50 [median sediment size], respectively), benthic macroinvertebrate density (measured using a slack sampler [500 μm mesh, 0.25 m2 frame; Moulton et al., 2002], number m−2), shredders (% of total invertebrates in the benthos), and organic and inorganic matter density in both rock cobbles and in seston (μg cm−2 and μg L−1, respectively). For all measurements, values were averaged across five locations at each site. We determined elevation (m above sea level) and river distance (km) using ArcInfo 9.1 (Earth Systems Research Institute Redlands, CA). In addition, these sites were assessed for fine sediment (<3.35 mm diameter) and salmonid spawning gravels (3.35–75 mm diameter) from a population of riffle crests available in 2016 and 2017 to coincide with biological sampling (see methods in Peters et al., 2017). Briefly, three subsamples were collected from each riffle crest, dried, sieved (mesh openings of 75, 26.5, 13.2, 9.5, 3.35, 2.0, 0.85, and 0.106 mm), and weighed to the nearest 0.001 kg. Fine sediments (< 0.0106 mm) were determined from water column collections before and after sediment collection using gravimetric methods (Peters et al., 2017). The data from the three subsamples were combined to produce a summary of the riffle conditions.
2.6 Statistical analysis
We used permutational (Monte Carlo) statistical tests for all analyses in R (R version 4.3.1; R Core Team, 2021) due to unequal variances and non-normality for some variables. Analysis of leaf litter decomposition rates (k day−1) required a natural log-transformation of percent AFDM remaining to determine exponential decay rates by regressing ln % AFDM remaining by harvest day (Olson, 1963; Petersen and Cummins, 1974; Benfield, 1996). Decay constants (−k) were compared using permutational analysis of covariance (ANCOVA) where significant interactions with time reflected significant differences in decomposition rates (slopes). We used a permutational three-way ANCOVA to compare maple litter sources, river sections, days in stream, and all possible interactions. Litter sources did not differ and so all litter sources were pooled and a follow-up two-way ANCOVA was used to determine significant differences in decomposition rates (section*day interaction) among river sections. We used Tukey’s Honest Significant Difference (HSD) test to make pairwise comparisons of decomposition rates among river sections. We used permutational linear regressions to test for linear relationships between k values and a large suite of environmental variables (physical, chemical, biological, sedimentary variables, and individual decomposer taxa; see Table S1), and the total number of Rhytisma punctatum stromatal infections in each litterbag. We used Principal Components Analysis (PCA) to combine environmental factors into six principal components that we used to explain variation in k, but none of the principal components explained significant fractions of the variation in k, so were excluded from analysis. Statistical analyses were conducted using the package lmPerm (aovp, lmp; Wheeler and Torchiano, 2016), and figures were produced using the R-package ggplot2 (Wickham et al., 2023).
We calculated simple diversity metrics for decomposer communities in each litterbag (macroinvertebrates after 2 weeks of incubation and fungi after 1 and 3 weeks of incubation). For benthic macroinvertebrates, we calculated total abundance (total number of individuals per litterbag), taxa richness (number of unique taxa per litterbag), % EPT taxa, % shredders, and Shannon’s diversity index (H′ per litterbag) using the vegan package, but for microbial communities we used the iNext package (Hsieh et al., 2016; Chao et al., 2022; Hsieh and Chao, 2022), which uses rarefaction to account for unequal sequencing depth for fungal OTUs to estimate fungal richness (counts of OTUs per litterbag) and Shannon’s diversity index (H′ per litterbag). To examine relationships between fungal OTU richness and diversity among harvest dates (1-week and 3-week) and river sections, we first used permutational two-way ANOVAs with harvest*section interactions. For fungal communities, harvest was not a significant effect in any model, so we ran follow-up permutational one-way ANOVAs by river section with Tukey HSD post hoc tests. For macroinvertebrates from the 2-week harvest, we ran permutational one-way ANOVAs by river section with Tukey HSD post hoc tests. We examined linear relationships among invertebrate and fungal community metrics, decomposition rates, and environmental variables (listed above) using permutational simple linear regressions. We used Principal Components Analysis (PCA) to combine environmental factors into six principal components that we used to explain variation in community metrics, but none of the principal components explained significant fractions of the variation, so were excluded from analysis.
To examine broader patterns in decomposer community composition, we used two-way permutational multivariate analyses of variance (PerMANOVAs) and we visualized differences among assemblages using non-metric multidimensional scaling (NMDS) ordinations with Bray-Curtis distance measures using the package vegan in R (Oksanen et al., 2022). For fungal OTUs, we used a 2-way PerMANOVA with harvest, section, and the harvest*section interaction. Harvest was not a significant effect in this model, so we ran a follow-up one-way PerMANOVA to determine the influence of river section on fungal community composition. Similarly, we ran a one-way PerMANOVA to determine the influence of river section on macroinvertebrate community composition. We used Bonferroni-corrected pairwise comparisons to determine significant differences among sections. We ran follow-up permutational tests for homogeneity of multivariate dispersion (PermDisp) to examine whether communities in each section of the Elwha were equally dispersed. Fungal OTU counts were converted to proportional abundances using the R-package phyloseq (McMurdie and Holmes, 2013; Morgan and Ramos, 2023) and macroinvertebrate abundances were log(x+1) transformed to preserve zeros (McCune et al., 2002). Correlations between NMDS ordination axes and environmental variables (listed above) were conducted using the R-package vegan. Indicator Species Analysis (ISA) determined which members of the macroinvertebrate community were significantly associated with a particular river section, which we performed with the R-package vegan. A slightly different approach was used to determine which fungal community members were associated with a particular river section. We used the R-package ALDEx2 (Fernandes et al., 2013) to perform differential abundance tests following central log ratio (CLR) transformations to lower false positive discovery rates (McMurdie and Holmes, 2014). OTUs were considered indicators when mean proportions were significantly different between one section and all others based on Benjamini-Hochberg corrected p-values from Wilcoxon rank sum tests, and comparing ALDEx2 effect sizes (Gloor et al., 2017).
3 Results
3.1 Leaf litter decomposition
Maple leaf litter decomposition rates differed significantly among sections of the Elwha watershed (Figure 2; section*days: F(4,384) = 8.87, p < 0.0001). In particular, litterbags placed in the newly formed Elwha Delta decomposed slowest, and litterbags placed in the middle reaches of the Elwha, just downstream of the upper dam, decomposed fastest. Decomposition rates for specific locations (n = 4 per section) across the watershed ranged almost two orders of magnitude from −0.00631 at one of the Delta sites to −0.09521 for one of the Aldwell Reservoir sites (Table 1). Decomposition rates were not significantly influenced by differences in litter quality among the five maple sources used to create litterbags (source*days: F(4,384) = 0.19, p = 0.999), but decomposition rates were negatively influenced by the number of endophyte-infected stromatal patches of Rhytisma punctatum on the initial leaf litter (F(1,18) = 7.92, p = 0.0115). Decomposition rates across the twenty locations were not significantly related to any physical, chemical, or substrate variables across the watershed, but were positively related to %EPT taxa (F(1,18) = 4.85, p = 0.0409; Table 2). When we statistically account for the number of Rhytisma patches, there are negative linear relationships between decomposition rates and both the smallest sediment size (<0.0106 mm [Sed1]; F(1,14) = 8.96, p = 0.0096) and the inorganic material in the sediment (F(1,14) = 5.35, p = 0.0365; Table S2), but no other environmental variables were significantly related to k. However, although the mechanisms for relationships with decomposition rates may not always be clear, a suite of invertebrates and fungi were significantly related to k (Table S2).
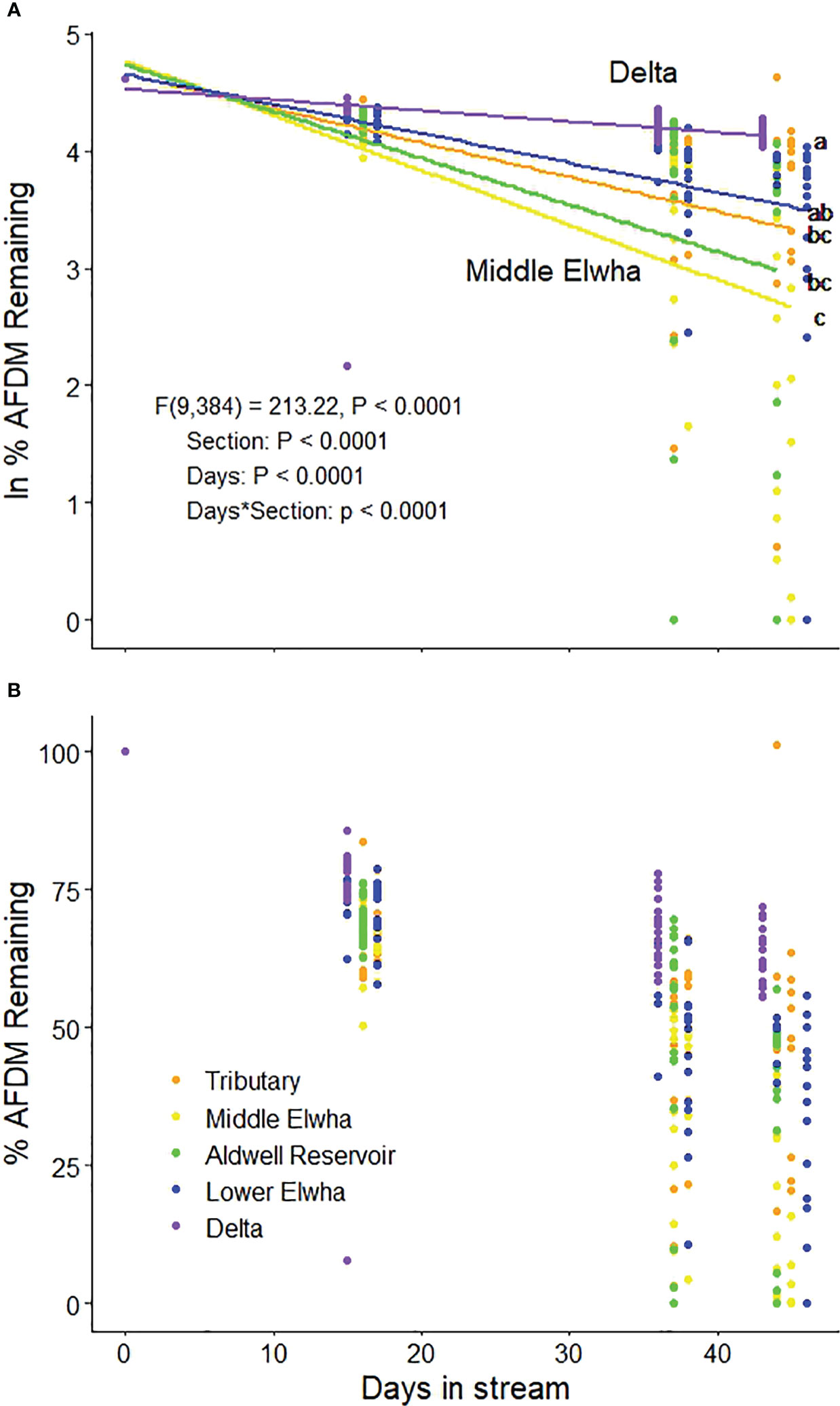
Figure 2 (A) Regression lines showing ln percent ash free dry mass (AFDM) remaining through time showing decomposition rates (slopes of exponential regression lines) for bigleaf maple leaf litter, and (B) percent ash free dry mass remaining through time for bigleaf maple litter decomposing in five sections of the Elwha River watershed following the removal of two major dams in 2014: 1) Orange circles: four tributary sites not physically influenced by restoration activities (Griff Creek, Lower Little River, Indian Creek, and Madison Creek); 2) Yellow circles: four river sites downstream of the upper Glines Canyon Dam; 3) Green circles: four river sites within the footprint of the former Aldwell Reservoir upstream of the lower Elwha Dam; 4) Blue circles: four river sites downstream of the lower Elwha Dam; and 5) Purple circles: four lentic sites in the newly developing Elwha delta (freshwater sites, not brackish). Lower case letters represent decomposition rates that differ significantly (p < 0.05).
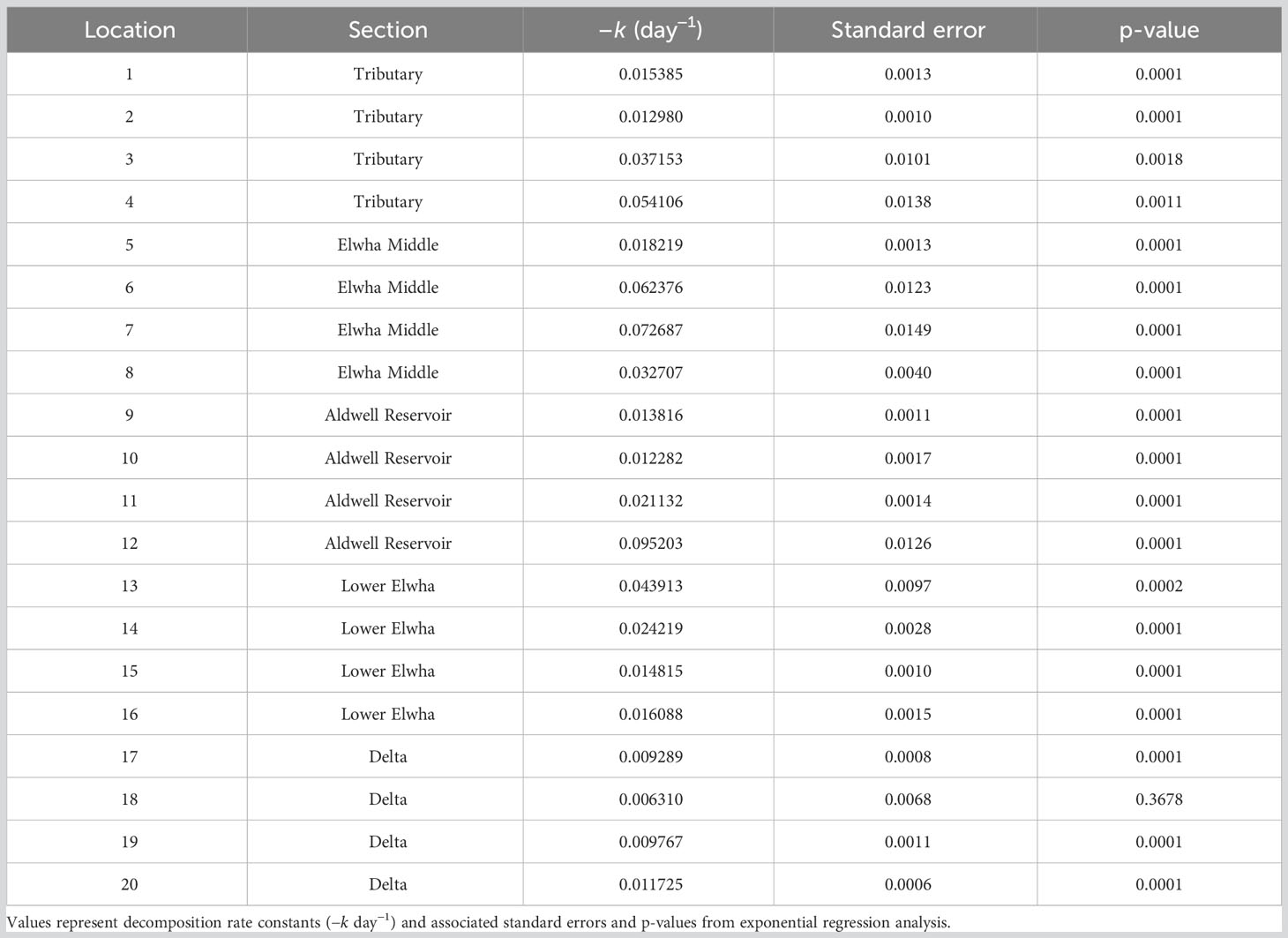
Table 1 Decomposition rates at each of twenty locations across five sections of the Elwha watershed post-dam removal.
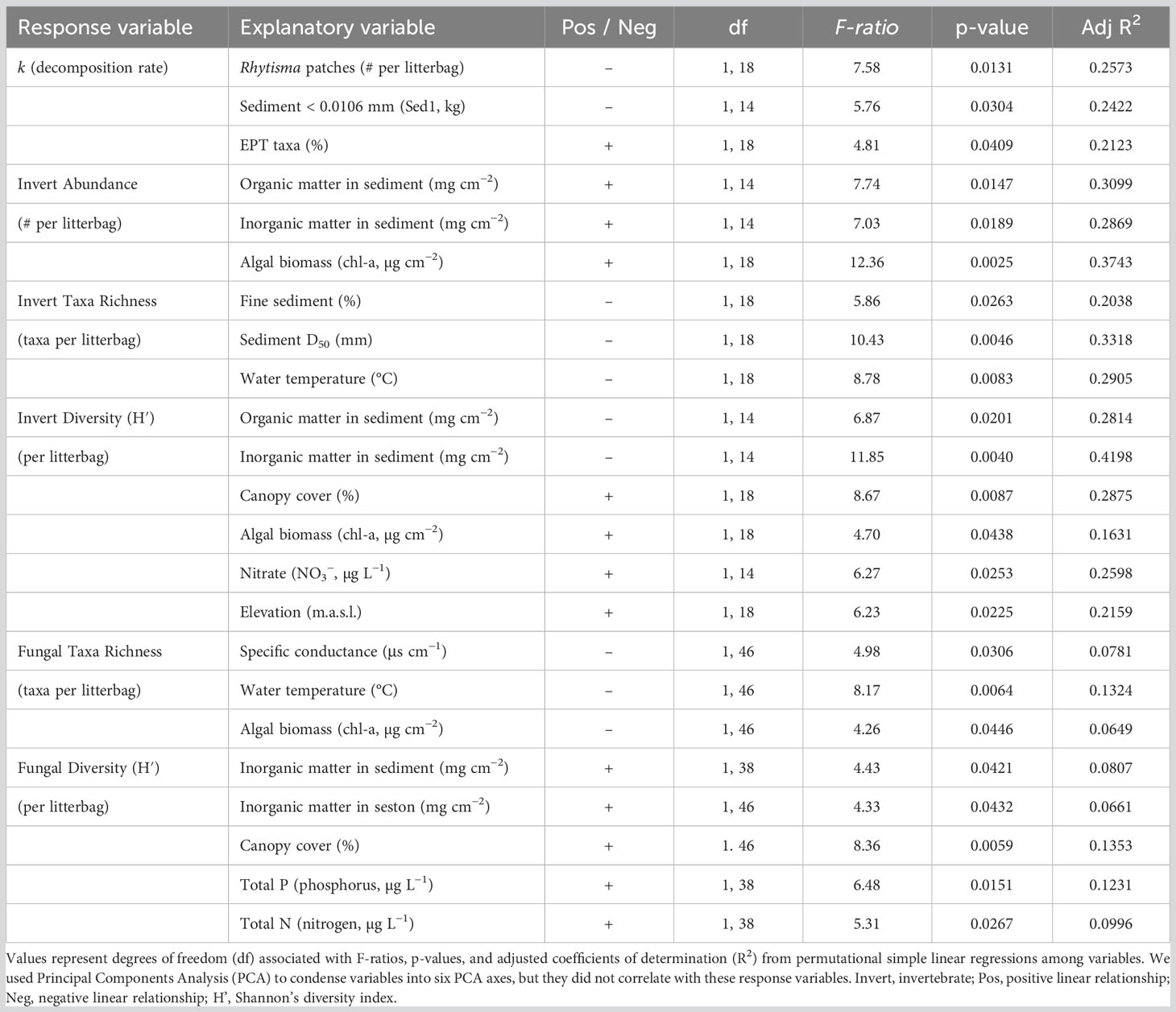
Table 2 Significant linear relationships among community and environmental variables across 20–24 locations in the Elwha watershed.
3.2 Macroinvertebrates
We identified 37 macroinvertebrate taxa in leaf litter bags, from a total of 32 families and 11 orders. The abundance, richness, and diversity of macroinvertebrates in leaf litterbags varied among river sections and were related to several environmental variables across the watershed. The abundance of macroinvertebrates was significantly different across sections (F(4,15) = 2.97, p = 0.001), with highest abundances at Lower Elwha sites and lowest abundances at Tributary sites (Figure 3A). The richness of macroinvertebrate taxa was significantly different among sections (F(4,15) = 4.77, p = 0.0114), with highest richness at sites in the former Aldwell Reservoir and Lower Elwha, compared to lowest taxa richness at the Elwha Delta (Figure 3B). Shannon’s Diversity Index values for litter bag macroinvertebrates were significantly different among sections (Figure 3C; F(4,15) = 8.38, p = 0.0026), with highest diversity at the Tributary sites, followed by the Middle Elwha and Delta, and lowest diversity at the Aldwell Reservoir and Lower Elwha sites. The percentage of EPT taxa differed significantly among river sections (F(4,15) = 22.89, p < 0.0001) with highest values at the Tributary and Middle Elwha sites, with increasingly lower values at Aldwell Reservoir, Lower Elwha, and Delta sites. The percentage of shredders did not differ among sections (F(4,15) = 1.95, p = 0.1205).
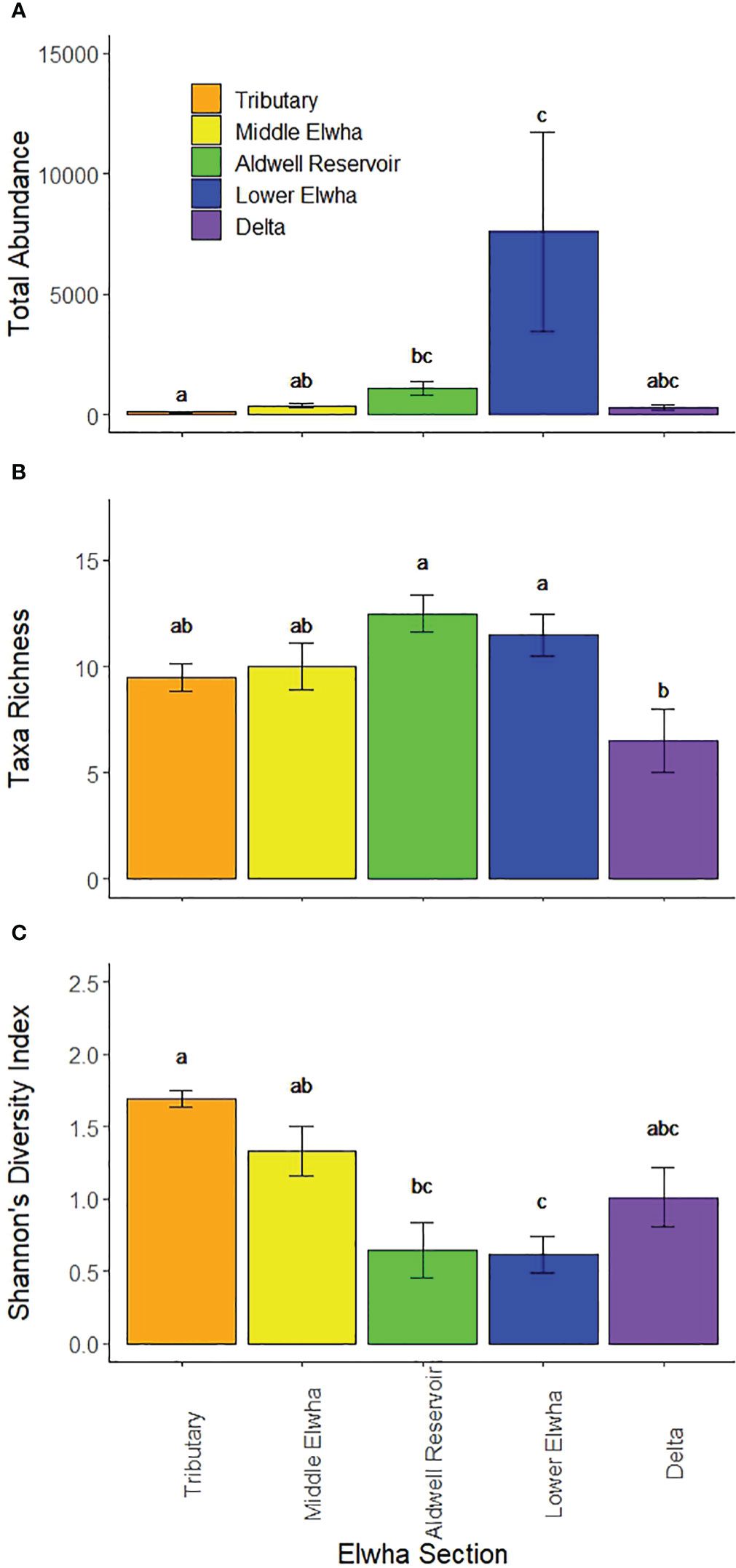
Figure 3 Aquatic macroinvertebrate patterns showing: (A) total invertebrate abundance (mean number of individuals per litterbag), (B) invertebrate taxa richness (mean number of species per litterbag), and (C) invertebrate taxa diversity (mean Shannon’s H’ per litterbag) across five Elwha watershed sections: 1) Orange bars: four tributary sites not physically influenced by restoration activities (Griff Creek, Lower Little River, Indian Creek, and Madison Creek); 2) Yellow bars: four river sites downstream of the upper Glines Canyon Dam; 3) Green bars: four river sites within the footprint of the former Aldwell Reservoir upstream of the lower Elwha Dam; 4) Blue bars: four river sites downstream of the lower Elwha Dam; and 5) Purple bars: four lentic sites in the newly developing Elwha delta (freshwater sites, not brackish). Bars represent means +/− 1 standard error and lower-case letters represent diversity values that differ significantly.
The abundance of macroinvertebrates was positively related to algal biomass (chl-a: F(1,18) = 4.98, p = 0.0386; Table 2), and both organic (F(1,14) = 7.74, p = 0.0147) and inorganic material in the sediment (F(1,14) = 7.03, p = 0.0189). The richness of macroinvertebrate taxa was positively related to sediment size (D50; F(1,18) = 10.43, p = 0.0046) and negatively related to the proportion of fine sediments (F(1,18) = 5.86, p = 0.0263) and water temperature (F(1,18) = 8.78, p = 0.0083). The diversity of macroinvertebrate taxa was positively related to the elevation (F(1,18) = 6.23, p = 0.0225) and river distance of the site (F(1,18) = 5.19, p = 0.0352), canopy cover (F(1,18) = 8.67, p = 0.0087), and nitrate (F(1,14) = 6.27, p = 0.0253), while being negatively related to both latitude (F(1,18) = 5.55, p = 0.0300) and longitude (F(1,18) = 8.15, p = 0.0150), both the organic (F(1,14) = 6.87, p = 0.0201) and inorganic material in the sediment (F(1,14) = 11.85, p = 0.0040), and algal biomass on rock surfaces (F(1,18) = 4.70, p = 0.0438).
The structure of macroinvertebrate communities establishing in leaf litterbags was also significantly different across river sections (Figure 4; F(4,15) = 5.21, p < 0.0001, stress = 0.0547), with Delta communities clearly separated from other sections of the Elwha watershed. Macroinvertebrate communities did not differ in terms of multivariate dispersion (F(4,15) = 1.47, p = 0.2690). Environmental variables such as elevation, canopy cover, specific conductance, nitrate, and increased macroinvertebrate diversity were correlated with macroinvertebrate communities found at Tributary sites. Variables like organic matter in seston and increasing taxa richness and abundance were correlated with macroinvertebrate communities found at the Aldwell Reservoir and Lower Elwha sites, with only temperature correlated with macroinvertebrate communities found at Delta sites (Figure 4). There were several significant indicator taxa, with the mayfly Baetis sp. associated with the Middle Elwha, while Oligochaeta, Chironomidae, and the stonefly Zapada sp. were associated with the Lower Elwha, and only a Physidae gastropod was associated with the Elwha Delta (Table 3).
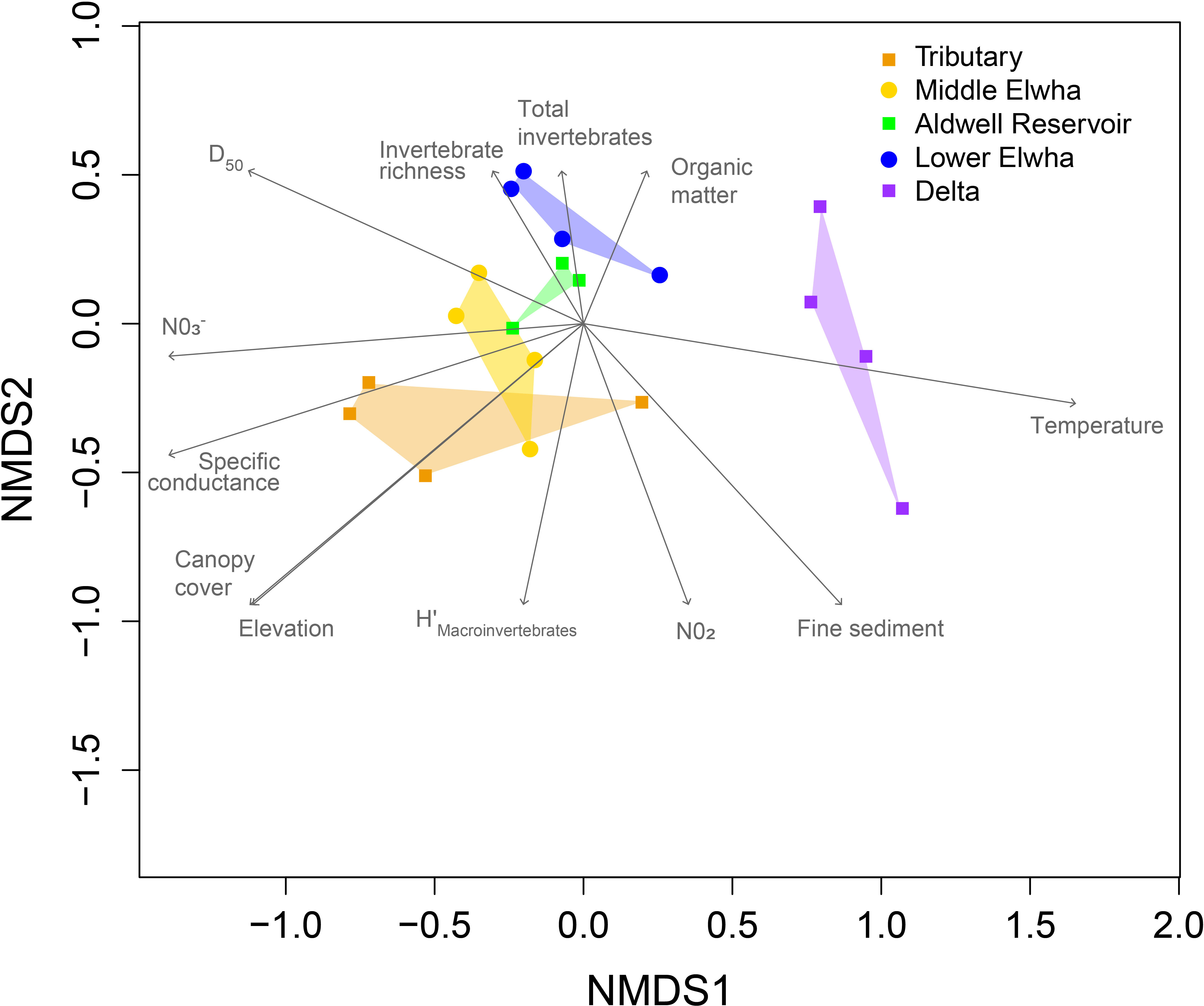
Figure 4 Non-metric multidimensional scaling (NMDS) ordination of aquatic macroinvertebrate communities. Symbols represent macroinvertebrate communities colonizing leaf litterbags in the Elwha River watershed following the removal of two major dams in 2014 placed at 20 study locations across five sections: 1) Orange squares: four tributary sites not physically influenced by restoration activities (Griff Creek, Lower Little River, Indian Creek, and Madison Creek); 2) Yellow circles: four river sites downstream of the upper Glines Canyon Dam; 3) Green squares: four river sites within the footprint of the former Aldwell Reservoir upstream of the lower Elwha Dam; 4) Blue circles: four river sites downstream of the lower Elwha Dam; and 5) Purple squares: four lentic sites in the newly developing Elwha delta (freshwater sites, not brackish). Vectors represent significant correlations through ordination space with environmental and biological factors. H’, Shannon’s diversity index; NO3−, nitrate concentration; NO2, nitrite concentration; D50, median sediment size.
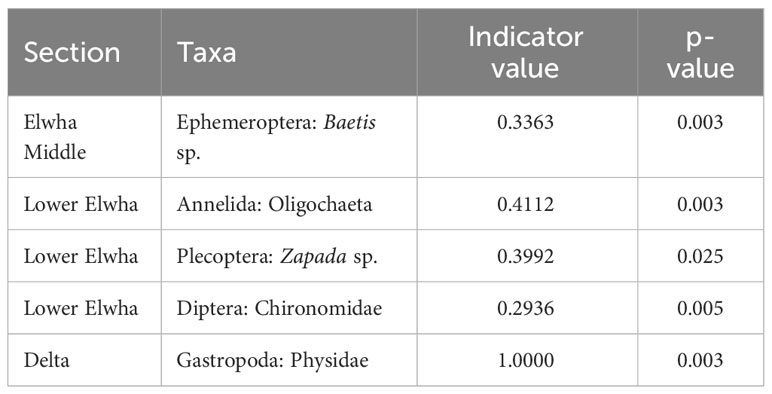
Table 3 Macroinvertebrate taxa identified from leaf litter bags that indicate for specific sections of the Elwha watershed.
3.3 Fungal decomposers
Leaf litter from across the Elwha watershed was colonized by 326 aquatic fungal taxa (OTUs). There were no significant differences in fungal taxa richness (F(1,36) = 2.95, p = 0.0708) or Shannon’s Diversity Index (F(1,36) = 0.27, p = 0.7843) by harvest date (1 versus 3 weeks), nor significant interactions between harvest date and river section (richness: F(1,36) = 1.16, p = 0.3383; diversity: F(5,36) = 0.72, p = 0.6186), so both harvests were pooled for subsequent one-way ANOVA models. Fungal taxa richness was not significantly different across sections of the Elwha watershed (F(5,42) = 1.35, p = 0.2679; Figure 5A), but fungal diversity (Shannon’s Diversity Index) differed significantly across sections (F(5,42) = 2.87, p = 0.0204). Litter incubated at the site of the former Mills Reservoir had the lowest fungal diversity and litter incubated in the Lower Elwha had the highest fungal diversity (Figure 5B).
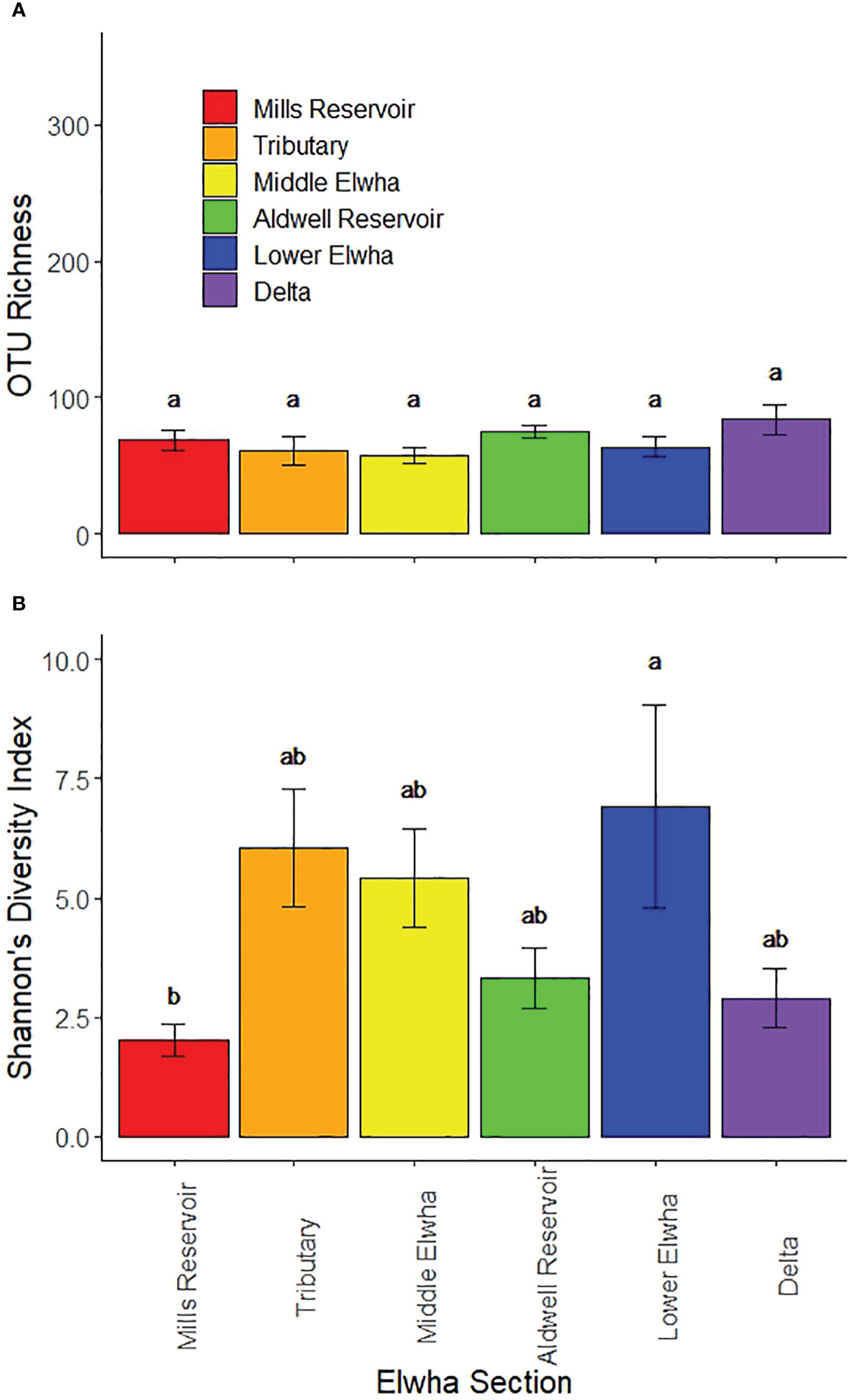
Figure 5 Aquatic fungal patterns showing: (A) fungal taxa richness (mean number of OTUs per litterbag), and (B) fungal taxa diversity (mean Shannon’s H’ per litterbag) across six different Elwha watershed sections: 1) Red bars: four rivers sites upstream of the Glines Canyon Dam in the former Mills reservoir footprint 2) Orange bars: four tributary sites not physically influenced by restoration activities (Griff Creek, Lower Little River, Indian Creek, and Madison Creek); 3) Yellow bars: four river sites downstream of the upper Glines Canyon Dam; 4) Green bars: four river sites within the footprint of the former Aldwell Reservoir upstream of the lower Elwha Dam; 5) Blue bars: four river sites downstream of the lower Elwha Dam; and 6) Purple bars: four lentic sites in the newly developing Elwha delta (freshwater sites, not brackish). Bars represent means +/− 1 standard error and lower-case letters represent diversity values that differ significantly (p < 0.05).
Fungal taxa richness was negatively related to water temperature (Table 2; F(1,46) = 8.17, p = 0.0064), specific conductance (F(1,46) = 4.98, p = 0.0306), and algal biomass (chl-a: F(1,46) = 4.26, p = 0.0446) across the watershed. Fungal diversity was positively related to canopy cover (F(1,46) = 8.36, p = 0.0059), total P (F(1,38) = 6.48, p = 0.0151), total N (F(1,38) = 5.31, p = 0.0267), inorganic material at the site, both in the sediment (F(1,38) = 4.43, p = 0.0421) and in the seston (F(1,46) = 4.33, p = 0.0432). Several fungal taxa were differentially abundant in this study. Members of the order Helotiales (OTU 96) were significantly less abundant at the Aldwell Reservoir site than all other sites (ALDEx2 effect size = −1.21, p = 0.0159), and three OTUs, including members of the genus Cladosporium sp. (OTU 6; ALDEx2 effect size = 1.37, p = 0.0021) and two unidentified fungi (OTU 326; ALDEx2 effect size = 1.42, p = 0.0038; OTU 2; ALDEx2 effect size = 1.06, p = 0.0045) were significantly more abundant in Tributary sites.
Aquatic fungal communities that established on leaf litter were also significantly different across river sections (Figure 6; F(5,36) = 3.47, p = 0.0001, stress = 0.0914), but they did not differ between harvests (F(1,36) = 2.10, p = 0.0600) and were not influenced by the harvest*section interaction (F(5,36) = 1.49, p = 0.0709). Fungal taxa communities on leaf litter were similar to each other for Mills Reservoir, and those communities differed significantly from many other communities, except the Tributary and Delta sites. Surprisingly, the fungal communities at the Delta sites did not differ significantly from any other sites. These differences could have been driven by large differences in multivariate dispersion across sections of the Elwha (F(5,42) = 7.73, p = 0.001). Environmental variables such as specific conductance, canopy cover, algal biomass (chl-a), total P, and larger sediment sizes (P7: proportion 9.5–13.2 mm; and P8: proportion 13.2–26.5 mm) were correlated with fungal communities found at Tributary sites (Table 2). Variables like SiO4 and the diversity of macroinvertebrate communities were correlated with fungal communities at some Middle Elwha sites, while fungal richness was correlated with fungal communities at the former Aldwell Reservoir, and smaller sediment sizes (P2: proportion 0.0106–0.106 mm) was correlated with fungal community structure at the former Mills Reservoir sites (Figure 6).
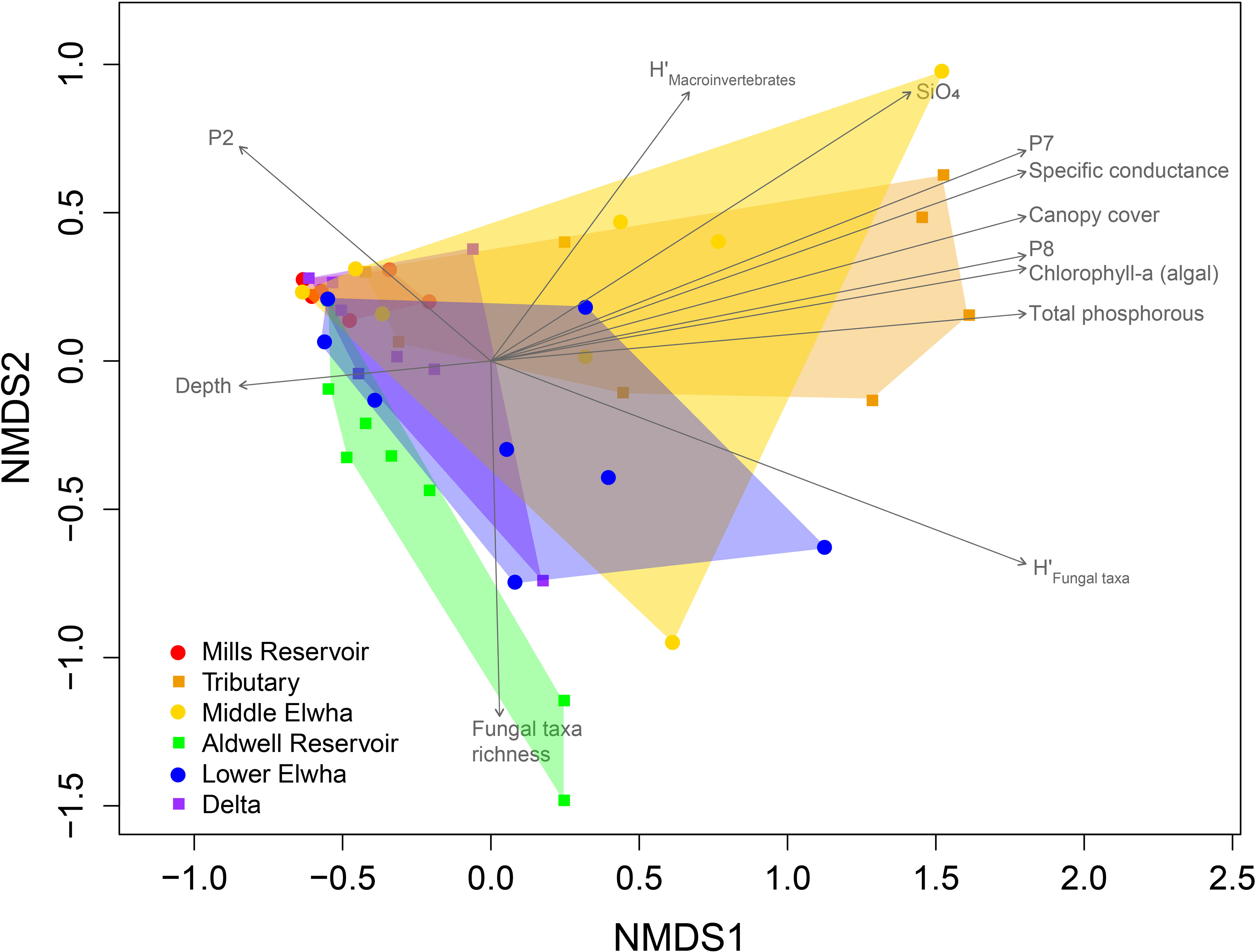
Figure 6 Non-metric multidimensional scaling (NMDS) ordination of aquatic fungal communities. Symbols represent fungal OTU (operational taxonomic unit) communities colonizing leaf litterbags in the Elwha River watershed following the removal of two major dams in 2014 and placed at 24 study locations across six sections: 1) Red circles: four rivers sites upstream of the Glines Canyon Dam in the former Mills reservoir footprint 2) Orange squares: four tributary sites not physically influenced by restoration activities (Griff Creek, Lower Little River, Indian Creek, and Madison Creek); 3) Yellow circles: four river sites downstream of the upper Glines Canyon Dam; 4) Green squares: four river sites within the footprint of the former Aldwell Reservoir upstream of the lower Elwha Dam; 5) Blue circles: four river sites downstream of the lower Elwha Dam; and 6) Purple squares: four lentic sites in the newly developing Elwha delta (freshwater sites, not brackish). Vectors represent significant correlations through ordination space with environmental and biological factors. H’, Shannon’s diversity index; SiO4, silicate concentration; P8, P7, and P2 represent proportions of sediment of various sizes (P2 = proportion of sediment between 0.0106–0.106 mm; P7 = proportion of sediment between 9.5–13.2 mm; P8 = proportion of sediment between 13.2–26.5 mm).
4 Discussion
This is the first study to examine leaf litter decomposition rates following the removal of large dams in a restored landscape. A few related studies have examined changes in leaf litter decomposition following small dam removal. One of these studies showed slower decomposition rates and much lower fungal biomass and macroinvertebrate diversity in leaf litter bags downstream of a diversion dam (Muehlbauer et al., 2009). Following six months of flow restoration, there was still slower decomposition at the site downstream of the dam, but both fungi and macroinvertebrates had recovered to upstream levels (Muehlbauer et al., 2009). In addition, aquatic macroinvertebrate communities began to converge following flow restoration, providing evidence that communities can recover within several years of flow restoration (Muehlbauer et al., 2009). One additional study examined reservoir drawdown during dam removal and found that organic matter decomposition was slower downstream of a dam before and during drawdown compared to nearby undammed reaches, but that all drawdown effects disappeared quickly (within one year), likely due to a long and slow drawdown process (Atristain et al., 2023). This study did not examine leaf litter decomposition, but instead used thin sheets of wood to examine organic matter processing. Reservoir drawdown negatively influenced biofilm metabolism and reduced autotrophic biofilm biomass (chlorophyll-a) on woody substrates, but both recovered quickly following drawdown (Atristain et al., 2023). In the present study, we found the slowest rates of decomposition in the new Elwha delta (as hypothesized), but contrary to our hypotheses the fastest decomposition rates were not in the tributaries, but in the Middle Elwha, possibly due to low deposition of fine sediment materials in this section (Peters et al., 2017). Decomposition rates did not decline longitudinally from upstream to downstream as has been hypothesized (Naiman et al., 1987; Sedell et al., 1989), but we may have seen more of a longitudinal pattern had we extended the study further upstream.
Although there are very few studies examining leaf litter decomposition following dam removals, there are several additional studies that have examined leaf litter decomposition above and below dams. In general, the type of dam can partially determine the influence of the dam on leaf litter decomposition because diversion dams result in downstream dewatered areas while hydropower dams result in high velocity, cold water releases below dams. Several studies report slower leaf litter decomposition rates below dams (Nelson and Roline, 2000; Tornwall, 2011; Mendoza–Lera et al., 2012; González et al., 2013), sometimes with significant reductions in shredders (Short and Ward, 1980; Tornwall, 2011; Mendoza–Lera et al., 2012) or fungal biomass below dams (Muehlbauer et al., 2009; Colas et al., 2016). However, at dam sites with high nutrient concentrations in tailwaters, the biomass of fungi and macroinvertebrates can be higher at downstream sites (Casas et al., 2000; Menéndez et al., 2012) and counteract the hydrological effects of dams, resulting in no difference in decomposition rates upstream and downstream of dams (Casas et al., 2000; Tabucanon et al., 2019), or occasionally, higher rates of leaf litter decomposition downstream of dams (Short and Ward, 1980; Menéndez et al., 2012; Russing, 2015).
The River Continuum Concept (RCC; Vannote et al., 1980) suggests that the importance of leaf litter inputs as carbon and energy sources to streams and rivers will decline in a downstream direction, with the greatest reliance on leaf litter in the headwaters and the least reliance in large-order rivers (Naiman et al., 1987; Sedell et al., 1989). The Riverine Productivity Model (RPM) modifies these predictions to argue that large rivers receive organic matter inputs laterally and from floodplains, and that decomposer communities can be important in large rivers, especially in littoral habitats (Thorp and Delong, 1994). Litter inputs to large rivers can still be important, as shown by one study which compared leaf decomposition in a low-order stream to a high-order river, and found slower decomposition rates in the high-order river, likely due to sediment deposition, but similar biological activity by invertebrates and fungi at both locations (Chauvet, 1997). Another study compared four locations within two river systems and found highest decomposition rates in headwaters with variation among lower reaches, but generally slower rates in high-order rivers (Minshall et al., 1983). Although not from a direct leaf litter decomposition study, one previous microbial study from throughout a river continuum found that microbial communities in sediments at headwater sites relied on allochthonous dissolved organic matter, with a shift to autochthonous dissolved organic matter downstream (Freixa et al., 2016). Newer visions of the River Continuum incorporate a more patchy dendritic network, and a less strict longitudinal system, incorporating ideas from landscape ecology and metacommunity ecology (Doretto et al., 2020). The newer RPM makes note of the importance of near-continuous inputs of coarse particulate organic matter in larger rivers (Thorp and Delong, 1994). There have been very few studies of leaf litter decomposition throughout watersheds to directly compare low-order and higher-order reaches of the same system, despite the ages of models like the RCC and RPM as well as more recent calls to do this research (Doretto et al., 2020).
Some of the patterns we observed in our data are similar to findings from an earlier study comparing benthic invertebrate and periphyton in dammed and undammed sections of the Elwha (Morley et al., 2008). In that study, the highest densities of periphyton were found in the lower river. Invertebrate communities in these areas of high autochthonous organic matter production may be less efficient at processing leaf litter, as reflected in the lower decomposition rates we observed in Lower Elwha and Delta. Morley et al. (2008) also found that benthic macroinvertebrate community structure differed between Upper, Middle, and Lower sections of the Elwha, with a higher proportion of non-insect taxa in the Lower Elwha compared to a dominance by mayfly in reaches between and above the dams. These pre-dam removal patterns still existed when this study was conducted after dam removal in 2016, with litterbag dwelling baetid mayflies associated with the Middle Elwha and chironomids and oligochaetes associated with the Lower Elwha. Other studies also show baetid mayflies to be abundant at sites just downstream of dams, especially those with high nutrient concentrations in the tailwaters (Brittain and Saltveit, 1989; Casas et al., 2000). In our study, we found that a Nemouridae stonefly (Zapada sp.) was also an indicator species for the Lower Elwha sites. Given that previous studies have shown Nemouridae stoneflies to be significantly less abundant downstream of dam sites (Mendoza–Lera et al., 2012), this finding could indicate potential post-dam recovery of in-stream litter dwelling macroinvertebrates.
Some of the most dramatic hydrological and habitat changes in the Elwha watershed after dam removal occurred downstream of both dams in the Lower Elwha (Locations 13, 14, 15, and 16) and the Delta (Locations 17, 18, 19, and 20). Predictive modeling expected that approximately 50–60% of the estimated 21 +/− 3 × 106 m3 of fine and coarse-grained accumulated sediment behind the dams would erode from the reservoirs, and within the first two years after dam removal, the steep, high-energy Elwha River had transported a large fraction of sediments (mainly fine sediments; Warrick et al., 2015; Peters et al., 2017; Ritchie et al., 2018; Warrick et al., 2019), and water turbidity increased by three orders of magnitude (Foley et al., 2015). This study took place just after the period of greatest geomorphic change in the Elwha watershed, 5–6 years after the start of the dam removal process, and about two years after the removal process had been completed (East et al., 2018). The extreme redistribution and deposition of sediment pushed the river delta approximately 200 m offshore, reducing tidal influence in the pre-dam removal Elwha delta complex and transforming it into a river-driven freshwater system (Foley et al., 2015). This loss of estuarine habitat due to altered water flow, decreased salinity, and elevated turbidity is strongly predicted to alter Elwha food web structure and benthic nutrient cycling in the delta, and likely contributed to the slowest decomposition rates, low fungal diversity, and relatively low macroinvertebrate abundance and diversity at the Delta sites. Seaward of the Delta sites, in tidally regulated wetlands (Foley et al., 2017b), newly formed habitats are emerging where future studies could examine how primary and secondary succession proceed for decomposers in dynamic environments.
The macroinvertebrate and fungal decomposer communities we examined responded differently to the dam removal landscape of the Elwha River. Aquatic macroinvertebrate communities inhabiting leaf litter were structured differently at the Delta sites compared to the rest of the river. In contrast, fungal communities at the Delta sites were not different from any other communities in the river and the communities upstream of both dams (Mills Reservoir sites) were the most different from other sections of the river. Community structure within a section of the river was also more consistent for invertebrate communities, which did not differ in terms of multivariate dispersion among sections. In contrast, fungal communities were most similar to one another upstream of both dams (Mills Reservoir sites), and fungal community dispersion was much higher downstream in the watershed. Invertebrate diversity was highest at Tributary sites and decreased downstream (with a slight uptick at Delta sites), while fungal taxa diversity was actually highest at the Lower Elwha sites and lowest at the most upstream site (Mills Reservoir). Despite these community differences for invertebrates and fungi, two environmental variables, specific conductance and canopy cover influenced the community structure of both groups, with strong positive relationships with Tributary sites. The environmental variables that influenced macroinvertebrate diversity tended to be those associated with tributary conditions: greater canopy cover, higher elevations, and more upstream river distances. These patterns support the results shown by a large review of decomposer communities (Cummins and Klug, 1979; Cummins et al., 1989; Graça, 2001; Hayer et al., 2022). The environmental variables that influenced fungal diversity included greater canopy cover, but also higher nutrient contents (N and P) and greater proportions of inorganic material in the seston and sediment. There are fewer studies examining fungal communities across longitudinal gradients in river systems, but previous research in large rivers argues that fungal decomposers are major players even in high order river reaches (Baldy et al., 1995).
The Glines Canyon and Elwha dams were constructed without fish passage facilities, which prevented upstream migration of anadromous salmonids for over 90 years, as well as prevented extant resident salmonids above the dams from migrating downstream (Brenkman et al., 2008; Pess et al., 2008). The regulation of the river severely disrupted sediment transport and deposition, as well as the movement of woody debris, resulting in a loss of suitable spawning habitat in the reaches of the Lower Elwha (Pess et al., 2008). Pre-dam removal salmonid population declines likely contributed to decreased primary productivity in the Elwha River due to nutrient limitation, as marine-derived nutrient inputs from salmonids are important for freshwater food webs across trophic levels (Duda et al., 2011; Tonra et al., 2015; Kane et al., 2020). As anadromous salmonids return to the Elwha (Kane et al., 2020; Quinn et al., 2021), we expect increases in productivity at all trophic levels and continued alterations to other ecosystem processes like organic matter processing. For example, we found highest levels of fungal diversity at Lower Elwha sites, which could be related to greatest spawner densities.
Dam decommissioning and removal in the United States has increased in the last several decades as social and ecological risks have begun to outweigh benefits generated by dams (Duda et al., 2016). At the time of this study, the simultaneous removal of the Elwha and Glines Canyon dams on the Elwha River in northwestern Washington State was the largest dam removal in the world (Warrick et al., 2019). The two dam removals on the Elwha River offered a unique opportunity to evaluate the impact of watershed-wide ecological restoration within a relatively short timeframe on organic matter processing and decomposer communities. Information on fundamental ecological processes such as organic matter decomposition are essential to better understand the mechanisms underpinning restoration response.
Data availability statement
The datasets presented in this study can be found in online repositories. The names of the repository/repositories and accession number(s) can be found below: https://github.com/carrileroy/Elwha2016.
Ethics statement
The manuscript presents research on animals that do not require ethical approval for their study.
Author contributions
CL, SM, and JD conceived of this study. CL supervised all research and students, coordinated field trips, ensured quality control, created figures, analyzed data, coordinated microbial sequencing and processed sequencing data, and wrote major components of the manuscript. SM coordinated field trips, collected and summarized field data on environmental variables, supervised NOAA staff and field collections, and provided expert opinion on macroinvertebrate identification. JD coordinated field trips, collected and summarized field data on environmental variables, and supervised USGS staff and field work. MS sorted macroinvertebrate samples and provided preliminary identifications. AZ, PL, CP, AB, BK, SH, and CL updated macroinvertebrate identifications and organized final data. PL created the GIS map in ArcGIS Pro. RP provided sediment analysis at all study sites. RM and MF coordinated the placement and removal of litterbags and fungal samples at the Elwha Delta sites. RJ coordinated the placement of fungal samples across all river sections. JV prepared and processed fungal samples for DNA extraction. All authors contributed to the article and approved the submitted version.
Funding
This research was completed with minimal funding. The U.S. Geological Survey Ecosystems Mission Area and National Oceanographic and Atmospheric Administration provided in-kind support to JD and SM, respectively. The Lower Elwha Klallam Tribe provided in-kind support to RM and JS. The U.S. Fish and Wildlife Service (USFWS) provided in-kind support to RP for sediment data collection and analysis. Funding for two undergraduate summer salaries was provided by the Evergreen Summer Undergraduate Research Fellowship (SURF) Program (E. Wolfe in 2015 and M. Taylor in 2016).
Acknowledgments
The U.S. Geological Survey (USGS) and National Oceanographic and Atmospheric Administration (NOAA) were issued permits for Elwha River research in Olympic National Park. James Starr coordinated the collection of leaf litter material from five different Acer macrophyllum sources. The Evergreen State College and itsScience Support Center provided support for field and laboratory work. Undergraduate students at The Evergreen State College contributed to both field and laboratory work: M. Taylor, E. Wolfe, A. Pratt, M. Chen, E. Potter, and N. Criss. We thank internal reviewers from both NOAA and USGS for helpful revisions to this manuscript. We also thank the handling editor and two reviewers for their contributions to improving this manuscript.
Conflict of interest
The authors declare that the research was conducted in the absence of any commercial or financial relationships that could be construed as a potential conflict of interest.
Publisher’s note
All claims expressed in this article are solely those of the authors and do not necessarily represent those of their affiliated organizations, or those of the publisher, the editors and the reviewers. Any product that may be evaluated in this article, or claim that may be made by its manufacturer, is not guaranteed or endorsed by the publisher.
Author disclaimer
Any use of trade, firm, or product names is for descriptive purposes only and does not imply endorsement by the U.S. Government.
Supplementary material
The Supplementary Material for this article can be found online at: https://www.frontiersin.org/articles/10.3389/fevo.2023.1231689/full#supplementary-material
References
Arias-Real R., Menéndez M., Abril M., Oliva F., Muñoz I. (2018). Quality and quantity of leaf litter: Both are important for feeding preferences and growth of an aquatic shredder. PloS One 13, e0208272. doi: 10.1371/journal.pone.0208272
Atristain M., von Schiller D., Larrañaga A., Elosegi A. (2023). Short-term effects of a large dam decommissioning on biofilm structure and functioning. Restor. Ecol. 31, e13779. doi: 10.1111/rec.13779
Baldy V., Gessner M. O., Chauvet E. (1995). Bacteria, fungi and the breakdown of leaf litter in a large river. Oikos 74, 93–102. doi: 10.2307/3545678
Bednarek A. T. (2001). Undamming rivers: A review of the ecological impacts of dam removal. Environ. Manage. 27, 803–814. doi: 10.1007/s002670010189
Bellmore J. R., Pess G. R., Duda J. J., O’Connor J. E., East A. E., Foley M. M., et al. (2019). Conceptualizing ecological responses to dam removal: If you remove it, what’s to come? BioScience 69, 26–39. doi: 10.1093/biosci/biy152
Benfield E. F. (1996). “Leaf breakdown in stream ecosystems,” in Methods in Stream Ecology. Eds. Hauer F. R., Lamberti G. A. (San Diego, CA:Academic Press), 579–589.
Bolyen E., Rideout J. R., Dillon M. R., Bokulich N. A., Abnet C. C., Al-Ghalith G. A., et al. (2019). Reproducible, interactive, scalable and extensible microbiome data science using QIIME 2. Nat. Biotechnol. 37, 852–857. doi: 10.1038/s41587-019-0209-9
Brenkman S. J., Pess G. R., Torgersen C. E., Kloehn K. K., Duda J. J., Corbett S. C. (2008). Predicting recolonization patterns and interactions between potamodromous and anadromous salmonids in response to dam removal in the Elwha River, Washington State, USA. nwsc 82, 91–106. doi: 10.3955/0029-344X-82.S.I.91
Brittain J. E., Saltveit S. J. (1989). A review of the effect of river regulation on mayflies (Ephemeroptera). Regulated Rivers: Res. Manage. 3, 191–204. doi: 10.1002/rrr.3450030119
Caporaso J. G., Lauber C. L., Walters W. A., Berg-Lyons D., Huntley J., Fierer N., et al. (2012). Ultra-high-throughput microbial community analysis on the Illumina HiSeq and MiSeq platforms. ISME J. 6, 1621–1624. doi: 10.1038/ismej.2012.8
Caporaso J. G., Lauber C. L., Walters W. A., Berg-Lyons D., Lozupone C. A., Turnbaugh P. J., et al. (2011). Global patterns of 16S rRNA diversity at a depth of millions of sequences per sample. Proc. Natl. Acad. Sci. 108, 4516–4522. doi: 10.1073/pnas.1000080107
Casas J., Zamora-Muñoz C., Archila F., Alba-Tercedor J. (2000). The effect of a headwater dam on the use of leaf bags by invertebrate communities. Regulated Rivers: Res. Manage. 16, 577–591. doi: 10.1002/1099-1646(200011/12)16:6<577::AID-RRR587>3.0.CO;2-P
Chao A., Ma K. H., Hsieh T. C. (2022). User’s guide for iNEXT online: Software for interpolation and extrapolation of species diversity (Hsin-Chu, Taiwan: Institute of Statistics, National Tsing Hua University). Available at: http://chao.stat.nthu.edu.tw/wordpress/wp-content/uploads/software/iNEXTOnline_UserGuide.pdf.
Chauvet E. (1997). Leaf litter decomposition in large rivers: the case of the River Garonne. Limnetica 13, 65–70. doi: 10.23818/limn.13.17
Colas F., Baudoin J.-M., Chauvet E., Clivot H., Danger M., Guérold F., et al. (2016). Dam-associated multiple-stressor impacts on fungal biomass and richness reveal the initial signs of ecosystem functioning impairment. Ecol. Indic. 60, 1077–1090. doi: 10.1016/j.ecolind.2015.08.027
Cummins K. W., Klug M. J. (1979). Feeding ecology of stream invertebrates. Annu. Rev. Ecol. Syst. 10, 147–172. doi: 10.1146/annurev.es.10.110179.001051
Cummins K. W., Petersen R. C., Howard F. O., Wuycheck J. C., Holt V. I. (1973). The utilization of leaf litter by stream detritivores. Ecology 54, 336–345. doi: 10.2307/1934341
Cummins K. W., Wilzbach M. A., Gates D. M., Perry J. B., Taliaferro W. B. (1989). Shredders and riparian vegetation. BioScience 39, 24–30. doi: 10.2307/1310804
Ding L., Chen L., Ding C., Tao J. (2019). Global trends in dam removal and related research: A systematic review based on associated datasets and bibliometric analysis. Chin. Geogr. Sci. 29, 1–12. doi: 10.1007/s11769-018-1009-8
Doretto A., Piano E., Larson C. E. (2020). The River Continuum Concept: lessons from the past and perspectives for the future. Can. J. Fish. Aquat. Sci. 77, 1853–1864. doi: 10.1139/cjfas-2020-0039
Duda J. J., Coe H. J., Morley S. A., Kloehn K. K. (2011). Establishing spatial trends in water chemistry and stable isotopes (δ15N and δ13C) in the Elwha River prior to dam removal and salmon recolonization. River Res. Appl. 27, 1169–1181. doi: 10.1002/rra.1413
Duda J. J., Freilich J. E., Schreiner E. G. (2008). Baseline studies in the Elwha River ecosystem prior to dam removal: Introduction to the Special Issue. nwsc 82, 1–12. doi: 10.3955/0029-344X-82.S.I.1
Duda J. J., Wieferich D. J., Bristol R. S., Bellmore J. R., Hutchison V. B., Vittum K. M., et al. (2016). Dam Removal Information Portal (DRIP)—A map-based resource linking scientific studies and associated geospatial information about dam removals (Reston, VA:U.S. Geological Survey) Open File Report 2016 - 1132. doi: 10.3133/ofr20161132
East A. E., Logan J. B., Mastin M. C., Ritchie A. C., Bountry J. A., Magirl C. S., et al. (2018). Geomorphic evolution of a gravel-bed river under sediment-starved versus sediment-rich conditions: River response to the world’s largest dam removal. J. Geophys. Res.: Earth Surface 123, 3338–3369. doi: 10.1029/2018JF004703
Fernandes A. D., Macklaim J. M., Linn T. G., Reid G., Gloor G. B. (2013). ANOVA-like differential expression (ALDEx) analysis for mixed population RNA-seq. PloS One 8, e67019. doi: 10.1371/journal.pone.0067019
Flory E. A., Milner A. M. (1999). Influence of riparian vegetation on invertebrate assemblages in a recently formed stream in Glacier Bay National Park, Alaska. J. North Am. Benthol. Soc. 18, 261–273. doi: 10.2307/1468464
Foley M. M., Bellmore J. R., O’Connor J. E., Duda J. J., East A. E., Grant G. E., et al. (2017a). Dam removal: Listening in. Water Resour. Res. 53, 5229–5246. doi: 10.1002/2017WR020457
Foley M. M., Duda J. J., Beirne M. M., Paradis R., Ritchie A., Warrick J. A. (2015). Rapid water quality change in the Elwha River estuary complex during dam removal. Limnol. Oceanogr. 60, 1719–1732. doi: 10.1002/lno.10129
Foley M. M., Warrick J. A., Ritchie A., Stevens A. W., Shafroth P. B., Duda J. J., et al. (2017b). Coastal habitat and biological community response to dam removal on the Elwha River. Ecol. Monogr. 87, 552–577. doi: 10.1002/ecm.1268
Freixa A., Ejarque E., Crognale S., Amalfitano S., Fazi S., Butturini A., et al. (2016). Sediment microbial communities rely on different dissolved organic matter sources along a Mediterranean river continuum. Limnol. Oceanogr. 61, 1389–1405. doi: 10.1002/lno.10308
Garthwaite I. J., Froedin-Morgensen A., Hartford S. H., Claeson S. M., Hobbs J. M. R., LeRoy C. J. (2021). Summer flower pulses: Catkin litter processing in headwater streams. Fundam. Appl. Limnol. 195, 243–254. doi: 10.1127/fal/2021/1384
Gloor G. B., Macklaim J. M., Pawlowsky-Glahn V., Egozcue J. J. (2017)Microbiome datasets are compositional: And this is not optional (Accessed November 6, 2022).
González J. M., Mollá S., Roblas N., Descals E., Moya Ó., Casado C. (2013). Small dams decrease leaf litter breakdown rates in Mediterranean mountain streams. Hydrobiologia 712, 117–128. doi: 10.1007/s10750-012-1144-7
Graça M. A. S. (2001). The role of invertebrates on leaf litter decomposition in streams – A Review. Int. Rev. Hydrobiol. 86, 383–393. doi: 10.1002/1522-2632(200107)86:4/5<383::AID-IROH383>3.0.CO;2-D
Hayer M., Wymore A. S., Hungate B. A., Schwartz E., Koch B. J., Marks J. C. (2022). Microbes on decomposing litter in streams: Entering on the leaf or colonizing in the water? ISME J. 16, 717–725. doi: 10.1038/s41396-021-01114-6
Hsieh T. C., Chao K. H. M. (2022) iNEXT: Interpolation and Extrapolation for Species Diversity. Available at: https://cran.r-project.org/web/packages/iNEXT/index.html (Accessed September 8, 2023).
Hsieh T. C., Ma K. H., Chao A. (2016). iNEXT: An R package for rarefaction and extrapolation of species diversity (Hill numbers). Methods Ecol. Evol. 7, 1451–1456. doi: 10.1111/2041-210X.12613
Junk W. J., Bayley P. B., Sparks R. E. (1989). “The flood pulse concept in river-floodplain systems,” in Proceedings of the International Large River Symposium. Ed. Dodge D. P. (Canadian Special Publications of Fisheries and Aquatic Sciences.), 110–127.
Kane W., Brown R., Bastow J. (2020). Monitoring the return of marine-derived nitrogen to riparian areas in response to dam removal on the Elwha River, Washington. nwsc 94, 118–128. doi: 10.3955/046.094.0203
Kasahara T., Li Y., Tanaka A. (2022). Effects of dams and reservoirs on organic matter decomposition in the hyporheic zone in forest mountain streams. Hydrobiologia 849, 2949–2965. doi: 10.1007/s10750-022-04905-w
Kaushik N. K. K., Hynes H. B. N. (1971). The fate of the dead leaves that fall into streams. Archiv fur Hydrobiol. 68, 465–515.
Kibler K., Tullos D., Kondolf M. (2011). Evolving expectations of dam removal outcomes: Downstream geomorphic effects following removal of a small, gravel-filled dam. JAWRA J. Am. Water Resour. Assoc. 47, 408–423. doi: 10.1111/j.1752-1688.2011.00523.x
LeRoy C. J., Fischer D. G., Halstead K., Pryor M., Bailey J. K., Schweitzer J. A. (2011). A fungal endophyte slows litter decomposition in streams: Endophyte slows in-stream decomposition. Freshw. Biol. 56, 1426–1433. doi: 10.1111/j.1365-2427.2011.02581.x
Li Y., Kasahara T., Chiwa M., Fujimoto N. (2020). Effects of dams and reservoirs on organic matter decomposition in forested mountain streams in western Japan. River Res. Appl. 36, 1257–1266. doi: 10.1002/rra.3640
Maavara T., Chen Q., Van Meter K., Brown L. E., Zhang J., Ni J., et al. (2020). River dam impacts on biogeochemical cycling. Nat. Rev. Earth Environ. 1, 103–116. doi: 10.1038/s43017-019-0019-0
McCune B., Grace J. B., Urban D. L. (2002) Analysis of ecological communities (Gleneden Beach, USA). Available at: https://www.vliz.be/en/imis?refid=259383 (Accessed November 6, 2022).
McMurdie P. J., Holmes S. (2013). Phyloseq: An R package for reproducible interactive analysis and graphics of microbiome census data. PloS One 8, e61217. doi: 10.1371/journal.pone.0061217
McMurdie P. J., Holmes S. (2014). Waste not, want not: Why rarefying microbiome data is inadmissible. PloS Comput. Biol. 10, e1003531. doi: 10.1371/journal.pcbi.1003531
Mendoza–Lera C., Larrañaga A., Pérez J., Descals E., Martínez A., Moya O., et al. (2012). Headwater reservoirs weaken terrestrial-aquatic linkage by slowing leaf-litter processing in downstream regulated reaches. River Res. Appl. 28, 13–22. doi: 10.1002/rra.1434
Menéndez M., Descals E., Riera T., Moya O. (2012). Effect of small reservoirs on leaf litter decomposition in Mediterranean headwater streams. Hydrobiologia 691, 135–146. doi: 10.1007/s10750-012-1064-6
Merritt R. W., Cummins K. W., Berg M. B. (2019). An Introduction to the Aquatic Insects of North America. 4th edition (Dubuque, Iowa: Kendall Hunt Publishing).
Milner A. M., Gloyne-Phillips I. T. (2005). The role of riparian vegetation and woody debris in the development of macroinvertebrate assemblages in streams. River Res. Appl. 21, 403–420. doi: 10.1002/rra.815
Minear J. T., Kondolf G. M. (2009). Estimating reservoir sedimentation rates at large spatial and temporal scales: A case study of California. Water Resour. Res. 45, W12502. doi: 10.1029/2007WR006703
Minshall G. W., Petersen R. C., Cummins K. W., Bott T. L., Sedell J. R., Cushing C. E., et al. (1983). Interbiome Comparison of Stream Ecosystem Dynamics. Ecol. Monogr. 53, 1–25. doi: 10.2307/1942585
Morgan M., Ramos M. (2023) BiocManager: Access the Bioconductor Project Package Repository. Available at: https://cran.r-project.org/web/packages/BiocManager/index.html (Accessed September 8, 2023).
Morley S. A., Duda J. J., Coe H. J., Kloehn K. K., McHenry M. L. (2008). Benthic invertebrates and periphyton in the Elwha River basin: Current conditions and predicted response to dam removal. Northwest Sci. 82, 179–196. doi: 10.3955/0029-344X-82.S.I.179
Morley S. A., Foley M. M., Duda J. J., Beirne M. M., Paradis R. L., Johnson R. C., et al. (2020). Shifting food web structure during dam removal—Disturbance and recovery during a major restoration action. PloS One 15, e0239198. doi: 10.1371/journal.pone.0239198
Moulton S. R., Kennen J. G., Goldstein R. M., Hambrook J. A. (2002). Revised Protocols for Sampling Algal, Invertebrate, and Fish Communities as Part of the National Water-Quality Assessment Program (Reston, VA. U.S. Geological Survey) Open-File Report 02-150.
Muehlbauer J. D., LeRoy C. J., Lovett J. M., Flaccus K. K., Vlieg J. K., Marks J. C. (2009). Short-term responses of decomposers to flow restoration in Fossil Creek, Arizona, USA. Hydrobiologia 618, 35–45. doi: 10.1007/s10750-008-9545-3
Naiman R. J., Melillo J. M., Lock M. A., Ford T. E., Reice S. R. (1987). Longitudinal patterns of ecosystem processes and community structure in a subarctic river continuum. Ecology 68, 1139–1156. doi: 10.2307/1939199
Nelson S. M., Roline R. A. (2000). Leaf litter breakdown in a mountain stream impacted by a hypolimnetic release reservoir. J. Freshw. Ecol. 15, 479–490. doi: 10.1080/02705060.2000.9663770
Nilsson R. H., Anslan S., Bahram M., Wurzbacher C., Baldrian P., Tedersoo L. (2019). Mycobiome diversity: High-throughput sequencing and identification of fungi. Nat. Rev. Microbiol. 17, 95–109. doi: 10.1038/s41579-018-0116-y
Oksanen J., Simpson G. L., Blanchet F. G., Kindt R., Legendre P., Minchin P. R., et al. (2022) vegan: Community Ecology Package. Available at: https://cran.r-project.org/web/packages/vegan/index.html (Accessed September 8, 2023).
Olson J. S. (1963). Energy storage and the balance of producers and decomposers in ecological systems. Ecology 44, 322–331. doi: 10.2307/1932179
Pess G. R., McHenry M. L., Beechie T. J., Davies J. (2008). Biological impacts of the Elwha River dams and potential salmonid responses to dam removal. Northwest Sci. 82, 72–90. doi: 10.3955/0029-344X-82.S.I.72
Peters R. J., Liermann M., McHenry M. L., Bakke P., Pess G. R. (2017). Changes in streambed composition in salmonid spawning habitat of the Elwha River during dam removal. JAWRA J. Am. Water Resour. Assoc. 53, 871–885. doi: 10.1111/1752-1688.12536
Petersen R. C., Cummins K. W. (1974). Leaf processing in a woodland stream. Freshw. Biol. 4, 343–368. doi: 10.1111/j.1365-2427.1974.tb00103.x
Petersen R. C., Cummins K. W., Ward G. M. (1989). Microbial and animal processing of detritus in a woodland stream. Ecol. Monogr. 59, 21–39. doi: 10.2307/2937290
Quinn T. P., Pess G. R., Sutherland B. J. G., Brenkman S. J., Withler R. E., Flynn K., et al. (2021). Resumption of anadromy or straying? Origins of sockeye salmon in the Elwha River. Trans. Am. Fish. Soc. 150, 452–464. doi: 10.1002/tafs.10294
R Core Team (2021). R: A language and environment for statistical computing. (Vienna, Austria: R Foundation for Statistical Computing).
Ritchie A. C., Warrick J. A., East A. E., Magirl C. S., Stevens A. W., Bountry J. A., et al. (2018). Morphodynamic evolution following sediment release from the world’s largest dam removal. Sci. Rep. 8, 13279. doi: 10.1038/s41598-018-30817-8
Russing B. E. (2015). Low-head dams alter stream physicochemical conditions and leaf litter decomposition. Masters Thesis (Boone, NC: Appalachian State University).
Salomão V. P., Tonin A. M., de Souza Rezende R., Leite G. F. M., Alvim E. A. C. C., Quintão J. M. B., et al. (2019). Small dam impairs invertebrate and microbial assemblages as well as leaf breakdown: a study case from a tropical savanna stream. Limnologica 77, 125685. doi: 10.1016/j.limno.2019.125685
Sedell J., Richey J., Swanson F. (1989). The river continuum concept: A basis for the expected ecosystem behavior of very large rivers? Proc. Int. Large River Symposium 106, 49–55.
Short R. A., Ward J. V. (1980). Leaf litter processing in a regulated Rocky Mountain stream. Can. J. Fish. Aquat. Sci. 37, 123–127. doi: 10.1139/f80-015
Smith D. P., Peay K. G. (2014). Sequence depth, not PCR replication, improves ecological inference from next generation DNA sequencing. PLoS ONE 9, e90234. doi: 10.1371/journal.pone.0090234
Stanford J. A., Ward J. V. (2001). Revisiting the serial discontinuity concept. Regulated Rivers: Res. Manage. 17, 303–310. doi: 10.1002/rrr.659
Stanley E. H., Doyle M. W. (2003). Trading off: The ecological effects of dam removal. Front. Ecol. Environ. 1, 15–22. doi: 10.1890/1540-9295(2003)001[0015:TOTEEO]2.0.CO;2
Tabucanon A. S., Xue W., Fujino T. (2019). Assessing alteration of leaf litter breakdown rate influenced by dam operation in Nakatsugawa River and Arakawa River, Central Japan. Watershed Ecol. Environ. 1, 1–9. doi: 10.1016/j.wsee.2018.12.001
Thorp J. H., Covich A. P. (2009). Ecology and Classification of North American Freshwater Invertebrates. 3rd edition (Amsterdam; Boston: Academic Press).
Thorp J. H., Delong M. D. (1994). The Riverine Productivity Model: An heuristic view of carbon sources and organic processing in large river ecosystems. Oikos 70, 305–308. doi: 10.2307/3545642
Tonra C. M., Sager-Fradkin K., Morley S. A., Duda J. J., Marra P. P. (2015). The rapid return of marine-derived nutrients to a freshwater food web following dam removal. Biol. Conserv. 192, 130–134. doi: 10.1016/j.biocon.2015.09.009
Tornwall B. M. (2011). Effects of a low head dam on a dominant detritivore and detrital processing in a headwater stream. Masters Thesis (Boone, NC: Appalachian State University).
Tullos D. D., Finn D. S., Walter C. (2014). Geomorphic and ecological disturbance and recovery from two small dams and their removal. PloS One 9, e108091. doi: 10.1371/journal.pone.0108091
Vannote R. L., Minshall G. W., Cummins K. W., Sedell J. R., Cushing C. E. (1980). The river continuum concept. CJFAS 37, 130–137. doi: 10.1139/f80–017
Wallace J. B., Eggert S. L., Meyer J. L., Webster J. R. (1997). Multiple trophic levels of a forest stream linked to terrestrial litter inputs. Science 277, 102–104. doi: 10.1126/science.277.5322.102
Warrick J. A., Bountry J. A., East A. E., Magirl C. S., Randle T. J., Gelfenbaum G., et al. (2015). Large-scale dam removal on the Elwha River, Washington, USA: Source-to-sink sediment budget and synthesis. Geomorphology 246, 729–750. doi: 10.1016/j.geomorph.2015.01.010
Warrick J. A., Stevens A. W., Miller I. M., Harrison S. R., Ritchie A. C., Gelfenbaum G. (2019). World’s largest dam removal reverses coastal erosion. Sci. Rep. 9, 13968. doi: 10.1038/s41598-019-50387-7
Webster J. R., Benfield E. F. (1986). Vascular plant breakdown in freshwater ecosystems. Annu. Rev. Ecol. Syst. 17, 567–594. doi: 10.1146/annurev.es.17.110186.003031
Wheeler B., Torchiano M. (2016) lmPerm: Permutation Tests for Linear Models. Available at: https://cran.r-project.org/web/packages/lmPerm/index.html (Accessed September 8, 2023).
Wickham H., Chang W., Henry L., Pedersen T. L., Takahashi K., Wilke C., et al. (2023) ggplot2: Create Elegant Data Visualisations Using the Grammar of Graphics. Available at: https://cran.r-project.org/web/packages/ggplot2/index.html (Accessed September 8, 2023).
Wolfe E. R., Younginger B. S., LeRoy C. J. (2019). Fungal endophyte-infected leaf litter alters in-stream microbial communities and negatively influences aquatic fungal sporulation. Oikos 128, 405–415. doi: 10.1111/oik.05619
Keywords: dam removal, leaf litter decomposition, aquatic–terrestrial interaction, macroinvertebrate communities, fungal communities, aquatic decomposition, ecological restoration
Citation: LeRoy CJ, Morley SA, Duda JJ, Zinck AA, Lamoureux PJ, Pennell C, Bailey A, Oswell C, Silva M, Kamakawiwo’ole BK, Hartford S, Van Der Hout J, Peters R, Mahan R, Stapleton J, Johnson RC and Foley MM (2023) Leaf litter decomposition and detrital communities following the removal of two large dams on the Elwha River (Washington, USA). Front. Ecol. Evol. 11:1231689. doi: 10.3389/fevo.2023.1231689
Received: 30 May 2023; Accepted: 03 October 2023;
Published: 19 October 2023.
Edited by:
Marco Girardello, Joint Research Centre (Italy), ItalyReviewed by:
Tim Wootton, The University of Chicago, United StatesManuela Abelho, Instituto Politécnico de Coimbra, Portugal
Copyright © 2023 LeRoy, Morley, Duda, Zinck, Lamoureux, Pennell, Bailey, Oswell, Silva, Kamakawiwo’ole, Hartford, Van Der Hout, Peters, Mahan, Stapleton, Johnson and Foley. This is an open-access article distributed under the terms of the Creative Commons Attribution License (CC BY). The use, distribution or reproduction in other forums is permitted, provided the original author(s) and the copyright owner(s) are credited and that the original publication in this journal is cited, in accordance with accepted academic practice. No use, distribution or reproduction is permitted which does not comply with these terms.
*Correspondence: Carri J. LeRoy, LeRoyC@evergreen.edu
†Present addresses: Brandy K. Kamakawiwo’ole, Washington Department of Agriculture, Pest Program, Olympia, WA, United States
Sorrel Hartford, Department of Ecology and Evolutionary Biology, University of Michigan, Ann Arbor, MI, United States
Jacqueline Van Der Hout, Nicolas School of the Environment, Duke University, Durham, NC, United States