- 1Department of Forestry, Michigan State University, East Lansing, MI, United States
- 2Program in Ecology, Evolution, and Behavior, Michigan State University, East Lansing, MI, United States
- 3Department of Agricultural and Environmental Sciences, Tennessee State University, Nashville, TN, United States
Shade tolerance is a central concept in forest ecology and strongly influences forest community dynamics. However, the plant traits and conditions conferring shade tolerance are yet to be resolved. We propose that shade tolerance is shaped not only by responses to light but also by a species’ defense and recovery functional traits, soil microbial communities, and interactions of these factors with light availability. We conducted a greenhouse experiment for three temperate species in the genus Acer that vary in shade tolerance. We grew newly germinated seedlings in two light levels (2% and 30% sun) and controlled additions of microbial filtrates using a wet-sieving technique. Microbial filtrate treatments included: <20 µm, likely dominated by pathogenic microbes; 40-250 µm, containing arbuscular mycorrhizal fungi (AMF); combination, including both filtrate sizes; and sterilized combination. We monitored survival for nine weeks and measured fine root AMF colonization, hypocotyl phenolics, stem lignin, and stem+root nonstructural carbohydrates (NSC) at three-week intervals. We found that differences in seedling survival between low and high light only occurred when microbes were present. AMF colonization, phenolics, and NSC generally increased with light. Phenolics were greater with <20 µm microbial filtrate, suggesting that soil-borne pathogens may induce phenolic production; and NSC was greater with 40-250 µm filtrate, suggesting that mycorrhizal fungi may induce NSC production. Across species, microbe treatments, and light availability, survival increased as phenolics and NSC increased. Therefore, shade tolerance may be explained by interactions among soil-borne microbes, seedling traits, and light availability, providing a more mechanistic and trait-based explanation of shade tolerance and thus forest community dynamics.
1 Introduction
Tree seedling mortality responses to understory light availability are an important filter of mature tree species composition and drivers of forest community dynamics (Pacala et al., 1996). Thus, plant survival in low light, or shade tolerance (Shirley, 1943) is a central concept in forest ecology. The seedling establishment phase, a major demographic bottleneck, is also critical for future community dynamics (Gurevitch et al., 2020). However, seedling responses to shade are far more complex than responses to light availability alone (Valladares and Niinemets, 2008; Valladares et al., 2016). Understanding the functional traits and biotic and abiotic conditions that convey shade tolerance are key to a more mechanistic understanding of forest community dynamics.
A plant’s ability to tolerate low light conditions can be moderated by soil-borne microbes, like soil-borne pathogens and mycorrhizal fungi (Jiang et al., 2020). Soil-borne pathogens (including fungi, oomycetes, and bacteria) can cause high seedling mortality (Song and Corlett, 2022), especially in shade (O’Hanlon-Manners and Kotanen, 2004; McCarthy-Neumann and Kobe, 2008; McCarthy-Neumann and Ibáñez, 2013), where wetter conditions enhance microbe reproduction and dispersal (Reinhart et al., 2010; Hersh et al., 2012; Liu and He, 2019). Arbuscular mycorrhizal fungi (AMF) can provide water and nutrients in exchange for sugars (Wipf et al., 2019). However, they may parasitize tree seedlings and increase mortality in low light (Ibáñez and McCarthy-Neumann, 2016; Konvalinková and Jansa, 2016), despite mutualistic tendencies and greater abundance in high light (Bereau et al., 2000; Shi et al., 2014; Koorem et al., 2017).
AMF, pathogens, and light levels can interact to influence seedling mortality. In high light, AMF root colonization is greater (Ibáñez and McCarthy-Neumann, 2016; Konvalinková and Jansa, 2016; Koorem et al., 2017), which can reduce the growth of fungal pathogens, potentially by competing for root space (Borowicz, 2001). AMF may also indirectly ameliorate pathogen effects (Liang et al., 2015), by providing water and nutrients to the host plant (Graham, 2001) and inducing production of defensive traits (Pozo and Azcón-Aguilar, 2007; Zamioudis and Pieterse, 2012) that protect against pathogens (Azcón-Aguilar et al., 2002; Violle et al., 2012). Conversely, in low light, seedling mortality may increase, due to the combined carbon costs of maintaining the AMF mutualism and recovery from pathogen attack.
Seedlings could also experience higher mortality from soil-borne microbes due to shade-induced changes in defensive functional trait values, such as reduced phenolics (Ichihara and Yamaji, 2009) and lignin (Rogers et al., 2005; Falcioni et al., 2018). Additionally, carbon limitation in shade and lower stored nonstructural carbohydrates (NSC) may constrain recovery from disease (Kobe, 1997; Kobe et al., 2010). Functional trait values may not only differ within a species based on light availability, but may also vary among species in relation to shade tolerance (Imaji and Seiwa, 2010). Shade tolerant species are typically less vulnerable to mortality by soil-borne microbes than shade intolerant species (McCarthy-Neumann and Kobe, 2008; McCarthy-Neumann and Kobe, 2010; Cortois et al., 2016) at least partly because shade tolerant species allocate more carbon to chemical and physical defenses (Coley et al., 1985; Coley and Barone, 1996), and recovery (Kitajima, 1994; Myers and Kitajima, 2007; Poorter et al., 2010).
To examine the effects of light availability, soil-borne microbes, tree seedling functional traits, and their interactions on light-dependent seedling survival, we established an experiment to test the following hypotheses: 1) within species, decreased survival under low versus high light only occurs in the presence of soil microbes; 2) mycorrhizal colonization is lower in the <20 µm microbial filtrate where pathogens are likely to be the dominant microbial group; 3) as defensive traits, phenolics and lignin are induced to higher levels in soils where microbes are present (non-sterilized) and in higher light availability; 4) as a recovery trait, NSC is lower in soils where microbes are present and in low light availability; 5) across all species and when microbes are present, survival increases as phenolics, lignin, and NSC increase.
2 Materials and methods
We conducted a fully factorial blocked-design greenhouse experiment at the Michigan State University Tree Research Center in Lansing, Michigan, USA (42.7 °N, 84.5 °W). The experiment consisted of three species (Acer negundo, A. rubrum, and A. saccharum), four microbial communities (<20 µm, representing pathogenic microbes; 40-250 µm, representing AMF; combined filtrate [both <20 µm and 40-250 µm]; and sterilized combined filtrate) and two light levels (2% and 30% full sun, representing shade and light gap environments). Individual pots were set up on six different benches (three per light level), where all treatment combinations were represented. We planted 80 seedlings per treatment combination for a total of 1,920 seedlings. We monitored seedlings every three days for survival, and randomly selected subsets for trait measurements at three, six, and nine weeks.
2.1 Species selection
We selected three biogeographically widespread, co-occurring tree species within the genus Acer: saccharum, rubrum, and negundo. These species have similar seed sizes (Osunkoya et al., 1994), but vary in shade tolerance (Burns and Honkala, 1990; Niinemets and Valladares, 2006).
2.2 Light availability
We grew seedlings at two light levels (2% and 30% full sun). We created light treatments by covering six greenhouse benches (three per treatment) with an inner layer of black shade cloth and an outer layer of reflective knitted poly-aluminum shade cloth (BFG Supply, Burton, Ohio, USA). We confirmed light levels using PAR (photosynthetically active radiation) measurements at each bench with a LI-COR 250A quantum sensor (LI-COR, Lincoln, Nebraska, USA) on a uniformly overcast day.
2.3 Soil collection and preparation of soil inocula
Soils were collected from Alma College’s Ecological Field Station in Vestaburg, Michigan, USA (43.4°N, 84.9 °W), in a 100-ha mixed-hardwood forest stand containing a 3-ha subplot with mapped and tagged trees. In August 2016, we randomly selected three adult trees per species. We selected adults that were at least two crown diameters away from other study species to reduce potential cross-culturing of soil. We collected soil (top 15 cm) within 1 m of each focal tree stem, maintained as separate replicates throughout the remainder of the experiment (as recommended by Rinella and Reinhart, 2018). We prepared soil by dicing roots and sifting soil through a 1 cm mesh sieve, retaining all roots that passed through the 1 cm sieve, as they may harbor host-specific microbial communities. Soil samples were stored at 4 °C for up to two months before preparation of soil inocula filtrates.
We created four microbial communities from sifted field soil using a wet-sieving method (Klironomos, 2002; Callaway et al., 2011; Pizano et al., 2014; Liang et al., 2015; König et al., 2016). For each extraction, we agitated 50 g of soil in a blender with 250 mL of deionized water at high speed for 60 sec, then passed the slurry through three analytical sieves (250-, 40-, and 20 µm) using a high-pressure water hose, for a total volume of 800 mL. To minimize contamination between treatments, we cleaned the sieves ultrasonically for 5 min between each extraction. The 250 µm sieve collected larger roots and coarser soil. We floated material retained by the 40 µm sieve on the surface of a 60% sucrose solution and centrifuged it at 688 g for 20 min. We collected material in the water and at the water-sucrose interface on 47 mm Whatman no.1 filter paper, surface sterilized it with 10% NaOCl for 10 sec, and washed it with distilled water under a filtration vacuum. We divided each filter paper into eight equal pieces. We collected the filtrate that passed through the 20 µm sieve and separated it into eight 100 mL containers. In sum, we created three microbial communities based on filtrate size classes: <20 µm, 40-250 µm, and combined (containing both <20 µm and 40-250 µm filtrates).
To test for abiotic effects of conspecific cultured soils due to nutrients or allelopathy, we combined filtrates (20- and 40-250 µm) and sterilized by steam autoclaves (at 121 °C for two hours). We also quantified and compared AMF colonization in the sterilized and <20 µm filtrates against a control containing only filter paper and deionized water to test the effectiveness of the sterilization for reducing microbes. We found no AMF colonization in soils treated with the sterilized (t = 2.97, df =176, p = 0.003) or <20 µm filtrates (t = 3.79, df = 178, p < 0.001), relative to a distilled water control. Filtrates were kept refrigerated at 4 °C for up to 48 hours, before adding them to the greenhouse pots.
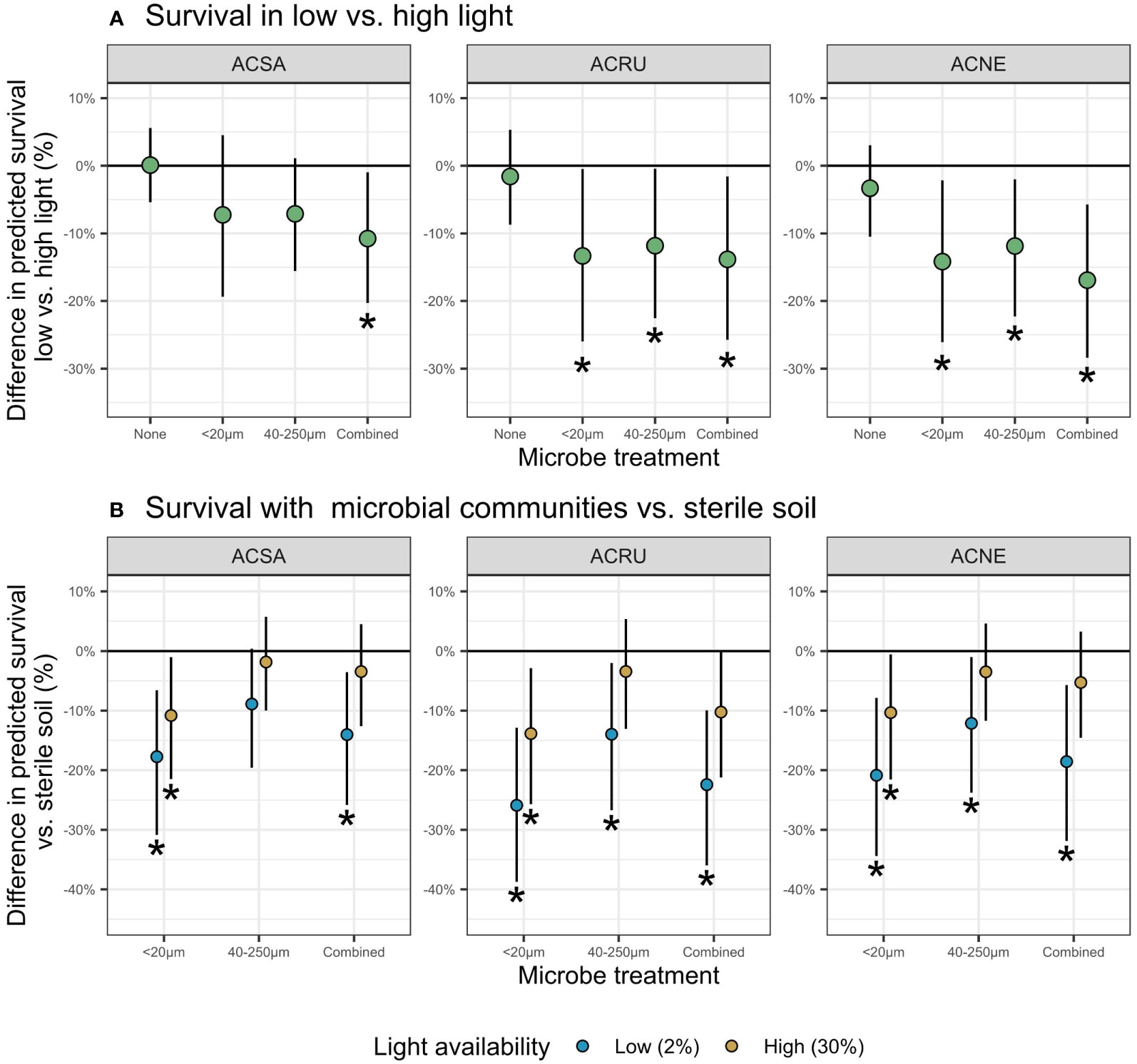
Figure 1 Difference in predicted survival (mean ± 90% credible interval) in (A) low versus high light and (B) relative to sterilized soil. For each species and microbial communities, at the end of 9-weeks. Negative values indicate decreased survival in treatment versus (A) high light or (B) sterilized soil. Statistically significant differences (90% CI do not overlap 0) are indicated by asterisks.
In the greenhouse, we filled 1,920 (655 cm3) deepots (Stuewe and Sons, Tangent, Oregon, USA) with sterilized commercial topsoil (Hammond Farms Landscape Supply, Lansing, Michigan, USA). To aid seedling germination, we also topped each pot with 2 cm of 85% sterilized commercial soil mix, containing peat moss, perlite, and vermiculite (Fafard 4P Mix, Sun Gro Horticulture, Agawan, Massachusetts, USA). In pilot trials of this experiment, we found over 50% seedling mortality in the first two weeks, without the addition of the commercial soil mix (Wood pers. observation). We steam sterilized the topsoil and soil mix by autoclaving twice at 121 °C for 2 hours, with a 48-hour incubation period between cycles.
Within 48 hours of wet sieving, we added microbe treatments to soil, keeping filtrate from each adult and species separate. To enable microbial communities to sporulate and AMF hyphae to establish, we cultured the soil with Allium as bait plants, before planting Acer seedlings (Klironomos et al., 1999; Al-Yahya’ei et al., 2011). In January 2017, each pot was planted with three germinating Allium tuberosum seedlings. After two months, we removed aboveground A. tuberosum seedling biomass.
One week after Allium removal, we planted Acer seedlings whose hypocotyls had emerged within the three previous days. We purchased seeds from Sheffield’s Seed Company (Locke, New York, USA). To minimize microbes from non-experimental soil sources, we surface sterilized the seeds with 0.6% NaOCl both prior to cold stratification and germinating in perlite. We watered the seedlings three times per week with 50 mL deionized water.
2.4 Survival and functional trait measurements
We grew the Acer seedlings for nine weeks. We recorded emergence and survival every three days and assigned date of death as the first census with total leaf and stem tissue necrosis. We harvested a random subset of 20 seedlings for each species and each treatment at three, six, and nine weeks, to quantify AMF colonization, phenolics, lignin, and NSC. We chose these times for our sub-harvests, because in a previous greenhouse experiment, mortality curves for tree seedlings subjected to soil-borne pathogens often increased at week three and peaked between four to six weeks after germination (McCarthy-Neumann and Ibáñez, 2012).
To quantify percent AMF colonization, we stained seedling roots with a 5% Shaeffer black ink in vinegar solution (Vierheilig et al., 1998) and counted fungal structures (e.g., arbuscules, coils, vesicles, hyphae) along 100 intersections under the microscope (McGonigle et al., 1990). To quantify phenolics, we analyzed hypocotyl samples, using a microplate-adapted colorimetric total phenolics assay with Folin-Ciocalteu reagent (Ainsworth and Gillespie, 2007). To quantify lignin, we analyzed root and stem samples with an Ankom 200 fiber analyzer (ANKOM Technologies, Macedon, NY, USA), using the acid-fiber detergent fiber filter technique. To quantify NSC, we analyzed stem samples, using a standardized enzyme method for sugar and starch extraction and quantification (Landhäusser et al., 2018).
2.5 Statistical analyses
To test the influence of light availability and microbial community on tree seedling survival, we used an individual based counting process in a Cox survival model (Burnham and Anderson, 2002; McCarthy-Neumann and Ibáñez, 2012). Data for each seedling and each time, were coded as 0 until the seedling was found dead. We used a count process to model the number of events (mortality, ) until the experiment ended at nine weeks. We modeled the likelihood as:
and the process as:
where parameters were estimated as a function of the hazard (h), which is the intrinsic rate of mortality due to individual age or time within the experiment), and of risk (μ), which is the extrinsic rate of mortality due to light availability and microbial community. Simulations (3 chains) were run until convergence of the parameters was ensured (25,000 iterations) and then run for another 50,000 iterations, from which the posterior parameter values (Supplementary Figures 1, 2) and predicted survival (Supplementary Figure 3) were estimated. Predicted survival values were used to assess whether there were differences in how species responded to microbe treatments and light. We then used predicted survival values and their associated uncertainty to test if there were differences in how species responded to low versus high light and in different microbe treatments. Differences that did not include zero in their 90% credible intervals were considered statistically significant (Kruschke, 2014).
We used linear mixed effects models to evaluate the effects of light availability and microbial community on seedling traits. We ran individual models for each species and trait, where light level and microbial community were treated as fixed effects, and harvest time, bench (nested within light level), and adult tree were treated as random effects.
We then used linear mixed effects models to assess the effects of traits on seedling survival in the three weeks following trait measurement (i.e., traits collected at three weeks were used to predict seedling survival from three to six weeks, and traits at six weeks were used to predict seedling survival from six to nine weeks). We treated AMF colonization, phenolics, lignin, and NSC as fixed effects, and included light level as a covariate. Species was treated as a random effect.
We evaluated relative support for each of our linear mixed effects models using multi-modal inference with corrected Akaike’s Information Criterion (Burnham and Anderson, 2002). Models with ΔAICc < 6 of the best-approximating model were considered plausible (Richards, 2008).
We performed all analyses in R 3.5.1 (R Core Team, 2020). We used the rjags package (Plummer, 2019) to fit survival models and to run predicted survival and contrast simulations. We used the built-in “lmer” function to fit linear mixed effects models and tested significance of main effects using the “Anova” function in the car package (Fox and Weisberg, 2019). Model selection for linear mixed effects models was determined with the “step” function in the lmerTest package (Kuznetsova et al., 2017). Post-hoc Tukey pairwise comparisons of significant main effects were made using the “emmeans” and “joint_tests” functions in the multcomp package (Hothorn et al., 2008; Lenth, 2020).
3 Results
3.1 Differences in seedling survival in low versus high light occurred only when microbes were present
Differences in seedling survival in low light versus high light, a measure of shade tolerance, appeared only in the presence of soil biota (Figure 1A). In low light, survival decreased up to 14% with the <20 µm filtrate, 12% with the 40-250 µm filtrate, and 17% with the combined filtrate. A. negundo experienced the largest decreases in survival (17% reduction in the combined filtrate), whereas A. saccharum had the lowest (11% reduction in the combined filtrate). Furthermore, A. saccharum survival decreased only in the presence of combined filtrate.
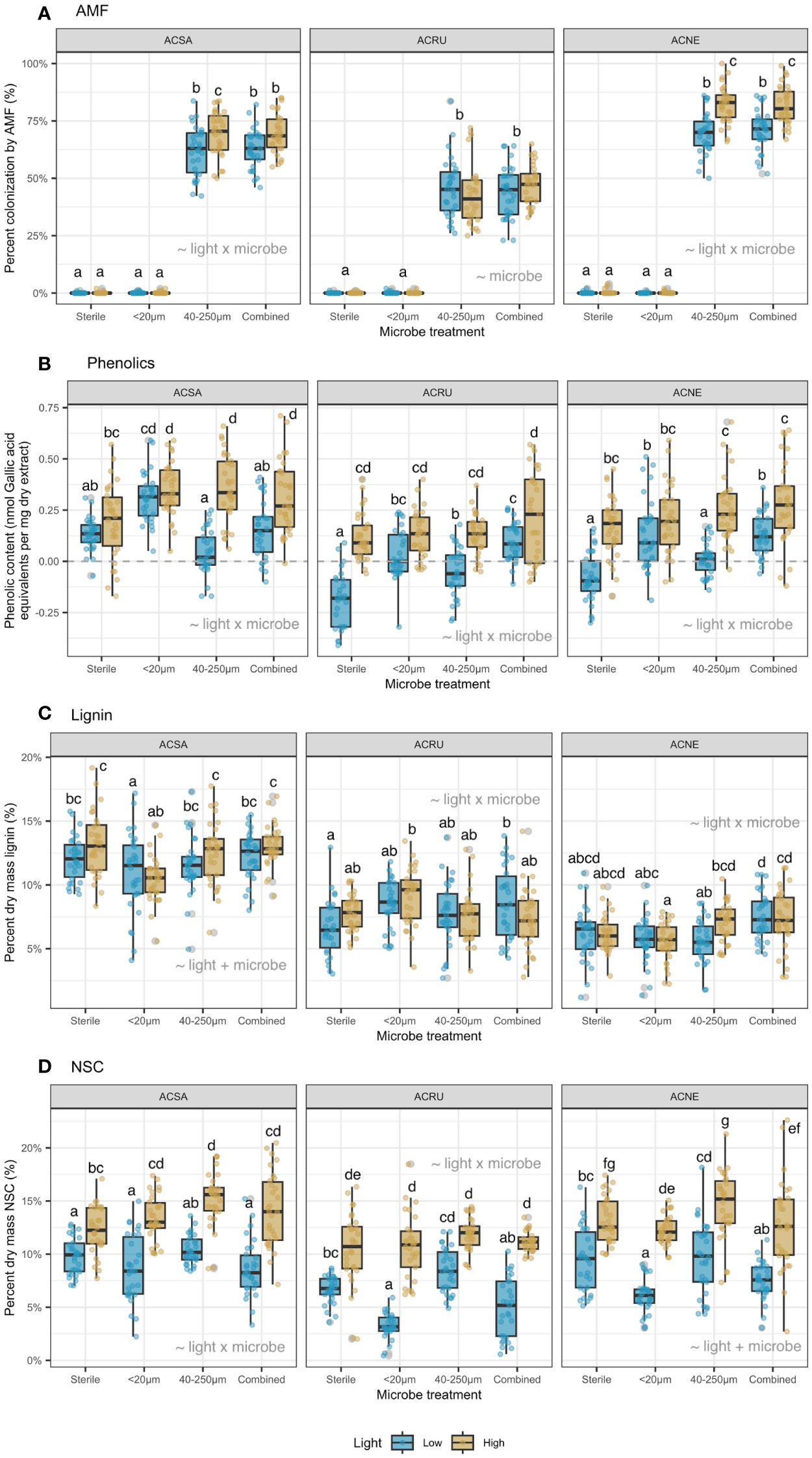
Figure 2 (A) percent colonization AMF (%), (B) phenolic content (nmol Gallic acid equivalents per mg dry extract), (C) percent dry mass lignin (%), and (D) percent dry mass NSC (%). Means within each panel not sharing a letter are statistically different by the Tukey test at alpha = 0.05. Best-fit model terms are overlayed on each panel.
Microbial treatments influenced seedling survival in only three of the nine cases in high light, but in eight of nine in low light (Figure 1B). At low light, the presence of soil biota relative to sterilized soil decreased survival 12-26% for all species and filtrates, except for A. saccharum with 40-250 µm filtrate. Additionally, negative soil biota effects were more common with the <20 µm filtrate, decreasing survival for all three species, regardless of light level.
3.2 Seedling functional trait values varied with light availability and microbial community
Across all treatments, percent root colonization by AMF was 11-18% greater in high than low light for A. saccharum and A. negundo (Figure 2A). There was no AMF colonization in the <20 µm filtrate, and colonization was similar in the 40-250 µm and combined filtrates. Overall, A. negundo (76%) had the highest AMF colonization, compared to A. saccharum (66%) and A. rubrum (45%).
Phenolic content (nmol Gallic acid equivalents per mg dry extract) increased with light availability across almost all microbe filtrate treatments (Figure 2B); the only exception was A. saccharum with the <20 µm filtrate. For A. rubrum and A. negundo, phenolic content was negligible in low light with the sterilized and combined filtrates. Overall, A. saccharum had the highest phenolic content (0.23 nmol/mg), compared to A. negundo (0.14 nmol/mg) and A. rubrum (0.06 nmol/mg).
Percent dry mass lignin was greater in high than low light but depended upon species and microbial filtrate (Figure 2C). For A. saccharum, lignin increased 5% across microbe filtrates, in high versus low light. For A. negundo, lignin increased 27% but only in the 40-250 µm filtrate. Across species, A. saccharum had the highest percent dry mass lignin (12%), compared to A. rubrum (8%) and A. negundo (6%).
Percent dry mass NSC was greater in high than low light for all species and was generally greatest with the 40-250 µm filtrate (Figure 2D). Additionally, for A. rubrum and A. negundo, NSC decreased 40-70% in the <20 µm and combined microbe filtrates, in low versus high light. Across species, A. saccharum had the highest dry mass NSC (12%), compared to A. negundo (11%) and A. rubrum (8%).
3.3 Seedling functional trait values are associated with survival
Survival increased with both phenolics (χ2 = 5.93, df = 1, p = 0.015; Figure 3A) and NSC (χ2 = 7.72, df = 1, p = 0.005; Figure 3B). However, there was no significant relationship between light availability and survival (p > 0.05), either alone or interacting with phenolics and NSC.
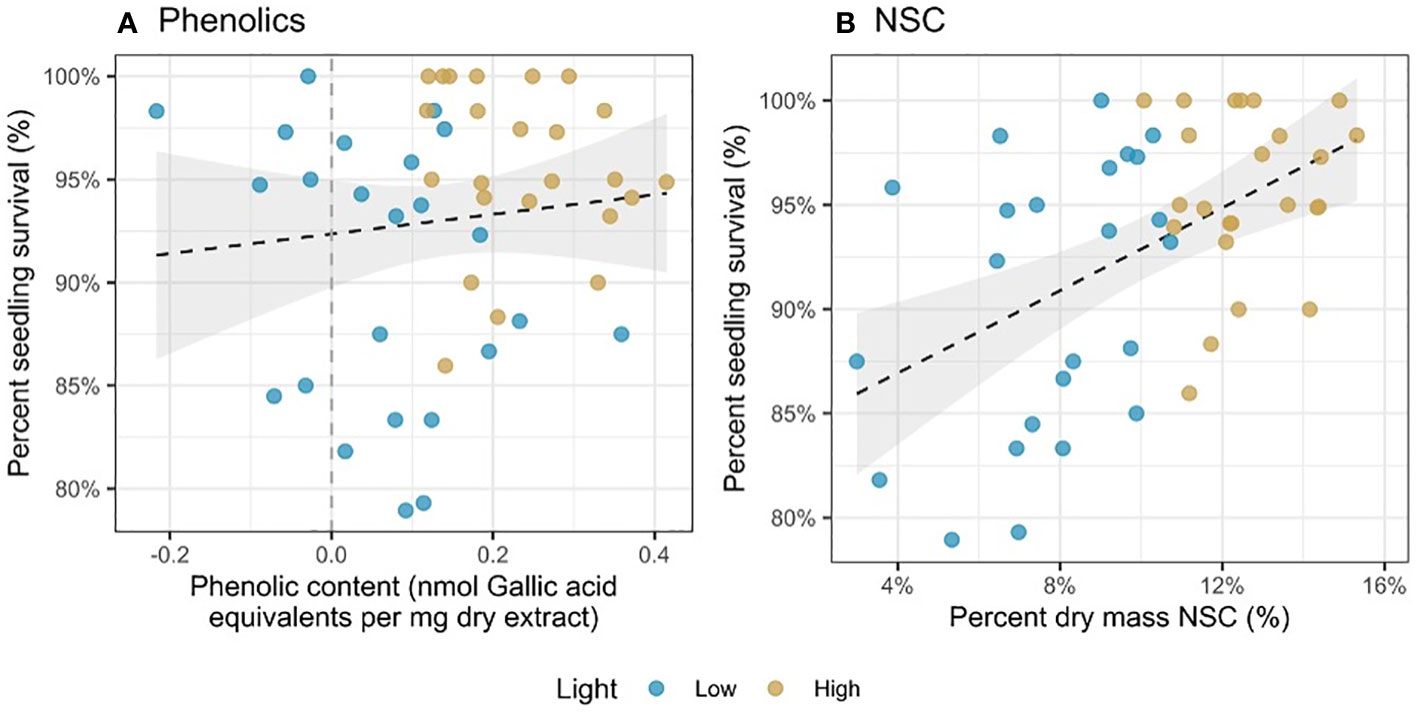
Figure 3 Percent seedling survival as a function of (A) phenolic content (nmol Gallic acid equivalents per mg dry extract) and (B) percent dry mass NSC (%). Each point represents a mean of trait values at a harvest time (3 or 6 weeks) and survival for seedlings in the 3 weeks after harvest.
4 Discussion
Our results support that survival of newly germinated tree seedlings in low versus high light, or “shade tolerance,” may be due to interactions between low light and soil-borne microbes and be mediated by defense and recovery functional traits. Previous studies have demonstrated that seedling functional traits are influenced by light and microbes and that functional traits can influence growth and survival at low light (Falster et al., 2018), but have not linked resources, traits, and survival, as in this study.
Across species, overall seedling survivorship and insensitivity to shading corresponded with shade tolerance categorizations. A. saccharum (shade tolerant) had the highest overall survival and was least sensitive to the microbial filtrates, compared to A. rubrum (intermediate) and A. negundo (intolerant). In our study, A. negundo had the highest AMF colonization of the three study species (Figure 2A) and had the largest decreases in low-light survival with added microbial filtrates (Figure 1B). This aligns with prior studies which have shown that shade intolerant species experience greater mortality from disease in shade (Augspurger, 1984a; Pizano et al., 2014) and higher growth when grown in high light (Xi et al., 2023). Similarly, shade tolerant species showed no significant growth responses to microbial filtrate treatments, in contrast to pioneer species that were more sensitive to the habitat from which soil microbial filtrates were collected (Pizano et al., 2017).
4.1 Soil-borne microbes explain variation in tree seedling survival responses to light
There is good support that the 20 µm filtrate in our study is primarily composed of fungi and bacteria. We found no evidence of mycorrhizal colonization in our <20 µm soil filtrate (Figure 2A), suggesting that this treatment is mainly composed of non-mycorrhizal fungi and bacteria (also consistent with Klironomos, 2002). Additionally, there is support that the 40 µm filtrate is primarily composed of the mycorrhizal community associated with soils cultured by conspecific adults. In a prior study that investigated this methodology, Wagg et al. (2014) found that soil passing through 250 µm sieves contained ~80% of the mycorrhizal community with an additional ~20% of the mycorrhizal community passing through the 50 µm sieves. In the Wagg et al. (2014) study, filtrate ≤25 µm in size, effectively had no nematodes, <10% mycorrhizal fungi, ~70% other fungi and ~90% bacteria of the original soil community. In addition, several other studies have used these filtrate size classes to isolate and investigate the roles of soil-borne pathogens (McCarthy-Neumann and Kobe, 2008; König et al., 2016) and AMF (Klironomos, 2002; Callaway et al., 2011; Liang et al., 2015; Pizano et al., 2017).
Differences in low versus high light survival appeared only when soil-borne microbes were present (Figure 1A), supporting hypothesis 1. With microbes present, survival decreased for all species, with the largest differences occurring in low light (Figure 1B). Our results are consistent with previous research demonstrating that tree seedlings have higher mortality in shade and that the major cause of seedling death arises from disease (Vaartaja, 1962; Augspurger, 1984a; Augspurger, 1984b). Our results are also consistent with Liang et al. (2015), who utilized the wet-sieving method and found that pathogens were associated with decreased biomass and survival, AMF were associated with increased biomass, and combined filtrate treatments canceled each other out for both biomass and survival; however, Liang et al. (2015) did not consider interactions with light availability.
We did not see increases in seedling survival when AMF were present, despite high percent colonization of seedling roots. This is in contrast to the study by Liang et al. (2015), in which they found higher seedling survival with AMF. However, benefits of AMF in our study may have manifested in growth (Gehring, 2003), which we did not measure. Young seedlings still relying on maternal seed reserves and high resource availability in the greenhouse may have diminished the importance of AMF (Kulmatiski and Kardol, 2008; Forero et al., 2019; Heinze et al., 2020). Moreover, AMF may have acted indirectly by enhancing production of phenolics and NSC, which were both positively correlated with AMF colonization (Supplementary Figure 4). We also did not see any negative relationship between AMF colonization and NSC in low light, which could have suggested that AMF act parasitically when photosynthates are limited.
4.2 Amounts of functional traits varied with light availability and soil-borne microbes
For all seedling species examined, phenolic content increased when microbes were present, supporting this aspect of hypothesis 3. Both AMF (Vierheilig, 2004; Whipps, 2004; Pozo and Azcón-Aguilar, 2007) and pathogens (Nicholson and Hammerschmidt, 1992; Witzell and Martín, 2008) can induce phenolics production. Seedling phenolics also consistently increased with light availability (Figure 2B), supporting this aspect of hypothesis 3 and suggesting alleviation of photosynthate constraints on chemical defense production (Ballaré, 2014).
Similarly, percent dry mass lignin increased with light availability and was highest in the 40-250 µm filtrate (Figure 2C), also supporting hypothesis 3. Higher lignin in the 40-250 µm filtrate and lower lignin in the <20 µm and combined filtrates for A. rubrum suggest that AMF have a positive effect while pathogens have a negative effect on lignin production.
In partial support of hypothesis 4, we found that seedling NSC decreased when pathogens were present (Figure 2D), consistent with NSC reserves acting as a carbon buffer after damage (McPherson and Williams, 1998; Gleason and Ares, 2004; Myers and Kitajima, 2007; Kobe et al., 2010). However, contrary to predictions, NSC increased when AMF were present, but only when not combined with the pathogen filtrate. The positive association between AMF colonization and NSC is consistent with Li et al., (2022).
4.2 Functional traits were associated with greater seedling survival
Partly supporting hypothesis 5, we found that both phenolics (chemical defense) and NSC (carbon buffer precluding recovery from damage) had positive associations with tree seedling survival. These results are consistent with previous studies that have speculated higher allocation to defensive traits increases survival of shade tolerant seedlings in low light conditions (Vaartaja, 1962; Augspurger, 1984a; Augspurger and Kelly, 1984; Kitajima, 1994; Alvarez-Clare and Kitajima, 2007).
We found no evidence of an association between lignin and survival. This was in contrast to previous studies that have posited that differences in lignin development impact seedling susceptibility to pathogens (Sattler and Funnell-Harris, 2013; Lee et al., 2019; Zhu et al., 2021). In this study, A. saccharum, the most shade tolerant species, had up to 50% greater lignin than the other two species (Figure 2D), but these differences in lignin values did not manifest in differences in survival.
4.3 Caveats and future research
There are several areas upon which future research could build on this work. We used sucrose-centrifugation to separate AMF spores from most other microbes and debris, and a bleach sterilization step to kill potential pathogens. While we were able to see high AMF colonization in the 40-250 µm and combined filtrates, and no colonization in the <20 µm or sterilized filtrates (Figure 2A), we cannot eliminate the possibility that we excluded some AMF in smaller filtrate sizes and included additional microbes and debris in the 40-250 µm filtrate. A more robust method would include further isolating pure AMF spores, as with Callaway (2011) and Pizano et al. (2017). Alternatively, by adding a genetic analysis of the microbial inoculum, we could have determined more accurately which microbial groups were present, which would enhance our understanding of the results. We recommend that future research utilize the wet-sieving method in conjunction with spore isolation and/or genetic analyses.
Likewise, by culturing the pots with Allium, we may have inadvertently increased AMF presence, disproportionate to pathogens, or increased the relative abundance of microbes that specialize with Allium, rather than Acer species. Although often thought of as generalists, AMF can show some host specificity (Yang et al., 2012; Kajihara et al., 2022), which could influence post-culturing microbial communities. Thus, we recommend that future experiments culture AMF and other microbe communities with the host species of interest (in this case, Acer spp.). In future experiments, we recommend using the host species as a bait plant in the culturing step.
While we investigated three co-occurring species within a single genus to make broader generalizations, subsequent studies trying to generalize these results should include more species across additional levels of shade tolerance. Also, effects of light availability could be caused by changes in microclimate (e.g., soil temperature and moisture) and not directly due to irradiance. Similarly, effects of light may be mediated by photoreceptors and jasmonates, not just assimilate availability through higher photosynthetic rates (Ballaré and Austin, 2019; Pierik and Ballaré, 2021).
Additionally, we utilized shade cloth in the greenhouse to create shaded conditions, not vegetation shade. Future studies should consider teasing apart these mechanisms in the field, rather than the greenhouse, to provide more realistic seedling responses. Furthermore, although we were interested in light availability and shade tolerance, other environmental variables, such as nutrient or water availability, also could influence seedling survivorship (McCarthy-Neumann and Kobe, 2019).
4.4 Implications for forest community dynamics
This study provides a needed first step in developing a mechanistic understanding of how soil-borne microbes impact seedling shade tolerance, explained through functional traits. Although fast-growing shade intolerant species may be expected to outcompete shade tolerant species in high light (Pacala et al., 1996), shade intolerant species can be limited by the negative interactive effects of soil-borne microbes at low light (McCarthy-Neumann and Kobe, 2010; Liu and He, 2019), restricting their recruitment niche to areas with higher light and fewer soil-borne microbes. In this paper, we have demonstrated the importance of interactions between soil-borne microbes and light availability in determining tree seedling survival. Furthermore, we have related both intra- and interspecific differences in survival to functional traits, supporting a more trait-based and mechanistic approach to understanding forest community dynamics.
Data availability statement
The datasets presented in this study can be found in online repositories. The names of the repository/repositories and accession number(s) can be found below: Dryad Repository https://doi.org/10.5061/dryad.7d7wm380b.
Author contributions
All authors conceived the ideas and methodology. KW collected and analyzed the data and lead the writing of the manuscript. RK and SM-N contributed critically to the drafts. All authors contributed to the article and approved the submitted version.
Funding
This research was funded by the National Science Foundation (NSF DEB 145732), Michigan State University, and Alma College.
Acknowledgments
We would like to thank Dr. Inés Ibáñez at University of Michigan for her help with developing the survival models and Dr. Walter Carson for his comments on earlier versions of the manuscript.
Conflict of interest
The authors declare that the research was conducted in the absence of any commercial or financial relationships that could be construed as a potential conflict of interest.
Publisher’s note
All claims expressed in this article are solely those of the authors and do not necessarily represent those of their affiliated organizations, or those of the publisher, the editors and the reviewers. Any product that may be evaluated in this article, or claim that may be made by its manufacturer, is not guaranteed or endorsed by the publisher.
Supplementary material
The Supplementary Material for this article can be found online at: https://www.frontiersin.org/articles/10.3389/fevo.2023.1224540/full#supplementary-material
References
Ainsworth E. A., Gillespie K. M. (2007). Estimation of total phenolic content and other oxidation substrates in plant tissues using Folin–Ciocalteu reagent. Nat. Protoc. 2, 875–877. doi: 10.1038/nprot.2007.102
Alvarez-Clare S., Kitajima K. (2007). Physical defense traits enhance seedling survival of neotropical tree species. Funct. Ecol. 21, 1044–1054. doi: 10.1111/j.1365-2435.2007.01320.x
Al-Yahya’ei M. N., Oehl F., Vallino M., Lumini E., Redecker D., Wiemken A., et al. (2011). Unique arbuscular mycorrhizal fungal communities uncovered in date palm plantations and surrounding desert habitats of Southern Arabia. Mycorrhiza 21, 195–209. doi: 10.1007/s00572-010-0323-5
Augspurger C. K. (1984a). Light requirements of Neotropical tree seedlings: A comparative study of growth and survival. J. Ecol. 72, 777. doi: 10.2307/2259531
Augspurger C. K. (1984b). Seedling survival of tropical tree species: Interactions of dispersal distance, light-gaps, and pathogens. Ecology 65, 1705–1712. doi: 10.2307/1937766
Augspurger C. K., Kelly C. K. (1984). Pathogen mortality of tropical tree seedlings: Experimental studies of the effects of dispersal distance, seedling density, and light conditions. Oecologia 61, 211–217. doi: 10.1007/BF00396763
Azcón-Aguilar C., Jaizme-Vega M. C., Calvet C. (2002). “The contribution of arbuscular mycorrhizal fungi to the control of soil-borne plant pathogens,” in Mycorrhizal technology in Agriculture. Eds. Gianinazzi S., Schüepp H., Barea J. M., Haselwandter K. (Basel: Birkhäuser Basel), 187–197. doi: 10.1007/978-3-0348-8117-3_15
Ballaré C. L. (2014). Light regulation of plant defense. Ann. Rev. Plant Biol. 65, 335–363. doi: 10.1146/annurev-arplant-050213-040145
Ballaré C. L., Austin A. T. (2019). Recalculating growth and defense strategies under competition: Key roles of photoreceptors and jasmonates. J. Exp. Bot. 70, 3425–3434. doi: 10.1093/jxb/erz237
Bereau M., Barigah T. S., Louisanna E., Garbaye J. (2000). Effects of endomycorrhizal development and light regimes on the growth of Dicorynia guianensis Amshoff seedlings. Ann. For. Sci. 57, 725–733. doi: 10.1051/forest:2000153
Borowicz V. A. (2001). Do arbuscular mycorrhizal fungi alter plant-pathogen relations? Ecology 82, 3057–3068. doi: 10.2307/2679834
Burnham K. P., Anderson D. R. (2002). Model selection and multimodel inference. 2nd ed (New York: NY: Springer-Verlag).
Burns R. M., Honkala B. H. (1990). Silvics of North America: 2. Hardwoods (Washington, DC: U.S: Department of Agriculture, Forest Service).
Callaway R. M., Bedmar E. J., Reinhart K. O., Silvan C. G., Klironomos J. (2011). Effects of soil biota from different ranges on “Robinia” invasion: Acquiring mutualists and escaping pathogens. Ecology 92, 1027–1035. Available at: https://cran.r-project.org/web/packages/rjags/rjags.pdf.
Coley P. D., Barone J. A. (1996). Herbivory and plant defenses in tropical forests. Annu. Rev. Ecol. Syst. 27, 305–335. doi: 10.1146/annurev.ecolsys.27.1.305
Coley P. D., Bryant J. P., Chapin F. S. (1985). Resource availability and plant antiherbivore defense. Science 230, 895–899. doi: 10.1126/science.230.4728.895
Core Team R. (2020). R: A language and environment for statistical computing. version 4.0.2 (Vienna, Austria: R Foundation for Statistical Computing).
Cortois R., Schröder-Georgi T., Weigelt A., Putten W. H., De Deyn G. B. (2016). Plant–soil feedbacks: role of plant functional group and plant traits. J. Ecol. 104, 1608–1617. doi: 10.1111/1365-2745.12643
Falcioni R., Moriwaki T., de Oliveira D. M., Andreotti G. C., de Souza L. A., dos Santos W. D., et al. (2018). Increased gibberellins and light levels promotes cell wall thickness and enhance lignin deposition in xylem fibers. Front. Plant Sci. 9. doi: 10.3389/fpls.2018.01391
Falster D. S., Duursma R. A., FitzJohn R. G. (2018). How functional traits influence plant growth and shade tolerance across the life cycle. Proc. Natl. Acad. Sci. U.S.A. 115, E6789–E6798. doi: 10.1073/pnas.1714044115
Forero L. E., Grenzer J., Heinze J., Schittko C., Kulmatiski A. (2019). Greenhouse- and field-measured plant-soil feedbacks are not correlated. Front. Environ. Sci. 7. doi: 10.3389/fenvs.2019.00184
Gehring C. A. (2003). Growth responses to arbuscular mycorrhizal fungi by rain forest seedlings vary with light intensity and tree species. Plant Ecol. 167, 127–139. doi: 10.1023/A:1023989610773
Gleason S. M., Ares A. (2004). Photosynthesis, carbohydrate storage and survival of a native and an introduced tree species in relation to light and defoliation. Tree Physiol. 24, 1087–1097. doi: 10.1093/treephys/24.10.1087
Graham J. H. (2001). What do root pathogens see in mycorrhizas? New Phytol. 149, 357–359. doi: 10.1046/j.1469-8137.2001.00077.x
Gurevitch J. S., Scheiner M., Fox G. A. (2020). “The Ecology of Plants,” in Sunderland, 3rd ed (Massacuhsetts, USA: Sinauer Associates).
Heinze J., Wacker A., Kulmatiski A. (2020). Plant–soil feedback effects altered by aboveground herbivory explain plant species abundance in the landscape. Ecology 00(00), e03023. doi: 10.1002/ecy.3023
Hersh M. H., Vilgalys R., Clark J. S. (2012). Evaluating the impacts of multiple generalist fungal pathogens on temperate tree seedling survival. Ecology 93, 511–520. doi: 10.1890/11-0598.1
Hothorn T., Bretz F., Westfall P. (2008). Simultaneous inference in general parametric models. Biometric J. 50, 346–363. doi: 10.1002/bimj.200810425
Ibáñez I., McCarthy-Neumann S. (2016). Effects of mycorrhizal fungi on tree seedling growth: Quantifying the parasitism–mutualism transition along a light gradient. Can. J. For. Res. 46, 48–57. doi: 10.1139/cjfr-2015-0327
Ichihara Y., Yamaji K. (2009). Effect of light conditions on the resistance of current-year Fagus crenata seedlings against fungal pathogens causing damping-off in a natural Beech forest: Fungus isolation and histological and chemical resistance. J. Chem. Ecol. 35, 1077–1085. doi: 10.1007/s10886-009-9687-4
Imaji A., Seiwa K. (2010). Carbon allocation to defense, storage, and growth in seedlings of two temperate broad-leaved tree species. Oecologia 162, 273–281. doi: 10.1007/s00442-009-1453-3
Jiang J., Abbott K. C., Baudena M., Eppinga M. B., Umbanhowar J. A., Bever J. D. (2020). Pathogens and mutualists as joint drivers of host species coexistence and turnover: implications for plant competition and succession. Am. Nat. 195, 591–602. doi: 10.1086/707355
Kajihara K. T., Egan C. P., Swift S. O. I., Wall C. B., Muir C. D., Hynson N. A. (2022). Core arbuscular mycorrhizal fungi are predicted by their high abundance–occupancy relationship while host-specific taxa are rare and geographically structured. New Phytol. 234, 1464–1476. doi: 10.1111/nph.18058
Kitajima K. (1994). Relative importance of photosynthetic traits and allocation patterns as correlates of seedling shade tolerance of 13 tropical trees. Oecologia 98, 419–428. doi: 10.1007/BF00324232
Klironomos J. N. (2002). Feedback with soil biota contributes to plant rarity and invasiveness in communities. Nature 417, 67–70. doi: 10.1038/417067a
Klironomos J. N., Bednarczuk E. M., Neville J. (1999). Reproductive significance of feeding on saprobic and arbuscular mycorrhizal fungi by the collembolan, Folsomia candida. Funct. Ecol. 13, 756–761. doi: 10.1046/j.1365-2435.1999.00379.x
Kobe R. K. (1997). Carbohydrate allocation to storage as a basis of interspecific variation in sapling survivorship and growth. Oikos 80, 226. doi: 10.2307/3546590
Kobe R. K., Iyer M., Walters M. B. (2010). Optimal partitioning theory revisited: Nonstructural carbohydrates dominate root mass responses to nitrogen. Ecology 91, 166–179. doi: 10.1890/09-0027.1
König J., van Kleunen M., Dawson W. (2016). No consistent legacy effects of invasion by giant goldenrod (Solidago gigantea) via soil biota on native plant growth. J. Plant Ecol. 9, 320–327. doi: 10.1093/jpe/rtv054
Konvalinková T., Jansa J. (2016). Lights off for arbuscular mycorrhiza: On its symbiotic functioning under light deprivation. Front. Plant Sci. 7. doi: 10.3389/fpls.2016.00782
Koorem K., Tulva I., Davison J., Jairus T., Öpik M., Vasar M., et al. (2017). Arbuscular mycorrhizal fungal communities in forest plant roots are simultaneously shaped by host characteristics and canopy-mediated light availability. Plant Soil 410, 259–271. doi: 10.1007/s11104-016-3004-0
Kulmatiski A., Kardol P. (2008). “Getting plant-soil feedbacks out of the greenhouse: Experimental and conceptual approaches,” in Progress in Botany Progress in Botany. Eds. Lüttge U., Beyschlag W., Murata J. (Berlin, Heidelberg: Springer Berlin Heidelberg), 449–472. doi: 10.1007/978-3-540-72954-9_18
Kuznetsova A., Brockhoff P. B., Christensen R. H. B. (2017). lmerTest package: Tests in linear mixed effects models. J. Stat. Software 82, 1–26. doi: 10.18637/jss.v082.i13
Landhäusser S. M., Chow P. S., Dickman L. T., Furze M. E., Kuhlman I., Schmid S., et al. (2018). Standardized protocols and procedures can precisely and accurately quantify non-structural carbohydrates. Tree Physiol. 38, 1764–1778. doi: 10.1093/treephys/tpy118
Lee M.-H., Jeon H. S., Kim S. H., Chung J. H., Roppolo D., Lee H.-J., et al. (2019). Lignin-based barrier restricts pathogens to the infection site and confers resistance in plants. EMBO J. 38, e101948. doi: 10.15252/embj.2019101948
Lenth R. (2020). emmeans: estimated marginal means, aka least-squares means (R package version 1.5.1).
Li Y.-L., Jin Z.-X., Luo G.-Y., Chen C., Sun Z.-S., Wang X.-Y. (2022). Effects of arbuscular mycorrhizal fungi inoculation on non-structural carbohydrate contents and C:N:P stoichiometry of Heptacodium miconioides under drought stress. Ying Yong Sheng Tai Xue Bao 33, 963–971. doi: 10.13287/j.1001-9332.202204.014
Liang M., Liu X., Etienne R. S., Huang F., Wang Y., Yu S. (2015). Arbuscular mycorrhizal fungi counteract the Janzen-Connell effect of soil pathogens. Ecology 96, 562–574. doi: 10.1890/14-0871.1
Liu Y., He F. (2019). Incorporating the disease triangle framework for testing the effect of soil-borne pathogens on tree species diversity. Funct. Ecol. 33, 1211–1222. doi: 10.1111/1365-2435.13345
McCarthy-Neumann S., Ibáñez I. (2012). Tree range expansion may be enhanced by escape from negative plant–soil feedbacks. Ecology 93, 2637–2649. doi: 10.1890/11-2281.1
McCarthy-Neumann S., Ibáñez I. (2013). Plant–soil feedback links negative distance dependence and light gradient partitioning during seedling establishment. Ecology 94, 780–786. doi: 10.1890/12-1338.1
McCarthy-Neumann S., Kobe R. K. (2008). Tolerance of soil pathogens co-varies with shade tolerance across species of tropical tree seedlings. Ecology 89, 1883–1892. doi: 10.1890/07-0211.1
McCarthy-Neumann S., Kobe R. K. (2010). Conspecific and heterospecific plant-soil feedbacks influence survivorship and growth of temperate tree seedlings. J. Ecol. 98, 408–418. doi: 10.1111/j.1365-2745.2009.01620.x
McCarthy-Neumann S., Kobe R. K. (2019). Site soil-fertility and light availability influence plant-soil feedback. Front. Ecol. Evol. 7. doi: 10.3389/fevo.2019.00383
McGonigle T. P., Miller M. H., Evans D. G., Fairchild G. L., Swan J. A. (1990). A new method which gives an objective measure of colonization of roots by vesicular—arbuscular mycorrhizal fungi. New Phytol. 115, 495–501. doi: 10.1111/j.1469-8137.1990.tb00476.x
McPherson K., Williams K. (1998). The role of carbohydrate reserves in the growth, resilience, and persistence of cabbage palm seedlings (Sabal palmetto). Oecologia 117, 460–468. doi: 10.1007/s004420050681
Myers J. A., Kitajima K. (2007). Carbohydrate storage enhances seedling shade and stress tolerance in a neotropical forest. J. Ecol. 95, 383–395. doi: 10.1111/j.1365-2745.2006.01207.x
Nicholson R. L., Hammerschmidt R. (1992). Phenolic compounds and their role in disease resistance. Annu. Rev. Phytopathol. 30, 369–389. doi: 10.1146/annurev.py.30.090192.002101
Niinemets Ü., Valladares F. (2006). Tolerance to shade, drought, and waterlogging of temperate northern hemisphere trees and shrubs. Ecol. Monogr. 76, 521–547. doi: 10.1890/0012-9615(2006)076[0521:TTSDAW]2.0.CO;2
O’Hanlon-Manners D. L., Kotanen P. M. (2004). Evidence that fungal pathogens inhibit recruitment of a shade-intolerant tree, white birch (Betula papyrifera), in understory habitats. Oecologia 140, 650–653. doi: 10.1007/s00442-004-1625-0
Osunkoya O. O., Ash J. E., Hopkins M. S., Graham A. W. (1994). Influence of seed size and seedling ecological attributes on shade-tolerance of rain-forest tree species in Northern Queensland. J. Ecol. 82, 149. doi: 10.2307/2261394
Pacala S. W., Canham C. D., Saponara J., Silander J. A., Kobe R. K., Ribbens E. (1996). Forest models defined by field measurements: Estimation, error analysis and dynamics. Ecol. Monogr. 66, 1–43. doi: 10.2307/2963479
Pierik R., Ballaré C. L. (2021). Control of plant growth and defense by photoreceptors: From mechanisms to opportunities in agriculture. Mol. Plant 14, 61–76. doi: 10.1016/j.molp.2020.11.021
Pizano C., Mangan S. A., Graham J. H., Kitajima K. (2014). Habitat-specific positive and negative effects of soil biota on seedling growth in a fragmented tropical montane landscape. Oikos 123, 846–856. doi: 10.1111/oik.01032
Pizano C., Mangan S. A., Graham J. H., Kitajima K. (2017). Host-specific effects of soil microbial filtrates prevail over those of arbuscular mycorrhizae in a fragmented landscape. Ecol. Appl. 27, 1946–1957. doi: 10.1002/eap.1579
Plummer M. (2019). rjags: Bayesian graphical models using MCMC. Available at: https://cran.r-project.org/web/packages/rjags/rjags.pdf.
Poorter L., Kitajima K., Mercado P., Chubiña J., Melgar I., Prins H. H. T. (2010). Resprouting as a persistence strategy of tropical forest trees: Relations with carbohydrate storage and shade tolerance. Ecology 91, 2613–2627. doi: 10.1890/09-0862.1
Pozo M. J., Azcón-Aguilar C. (2007). Unraveling mycorrhiza-induced resistance. Curr. Opin. Plant Biol. 10, 393–398. doi: 10.1016/j.pbi.2007.05.004
Reinhart K. O., Royo A. A., Kageyama S. A., Clay K. (2010). Canopy gaps decrease microbial densities and disease risk for a shade-intolerant tree species. Acta Oecologica 36, 530–536. doi: 10.1016/j.actao.2010.07.006
Richards S. A. (2008). Dealing with overdispersed count data in applied ecology. J. Appl. Ecol. 45, 218–227. doi: 10.1111/j.1365-2664.2007.01377.x
Rinella M. J., Reinhart K. O. (2018). Toward more robust plant-soil feedback research. Ecology 99, 550–556. doi: 10.1002/ecy.2146
Rogers L. A., Dubos C., Cullis I. F., Surman C., Poole M., Willment J., et al. (2005). Light, the circadian clock, and sugar perception in the control of lignin biosynthesis. J. Exp. Bot. 56, 1651–1663. doi: 10.1093/jxb/eri162
Sattler S., Funnell-Harris D. (2013). Modifying lignin to improve bioenergy feedstocks: Strengthening the barrier against pathogens? Front. Plant Sci. 4. doi: 10.3389/fpls.2013.00070
Shi G., Liu Y., Johnson N. C., Olsson P. A., Mao L., Cheng G., et al. (2014). Interactive influence of light intensity and soil fertility on root-associated arbuscular mycorrhizal fungi. Plant Soil 378, 173–188. doi: 10.1007/s11104-014-2022-z
Song X., Corlett R. T. (2022). Do natural enemies mediate conspecific negative distance- and density-dependence of trees? A meta-analysis of exclusion experiments. Oikos 2022, e08509. doi: 10.1111/oik.08509
Vaartaja O. (1962). The relationship of fungi to survival of shaded tree seedlings. Ecology 43, 547–549. doi: 10.2307/1933389
Valladares F., Laanisto L., Niinemets Ü., Zavala M. A. (2016). Shedding light on shade: Ecological perspectives of understorey plant life. Plant Ecol. Diversity 9, 237–251. doi: 10.1080/17550874.2016.1210262
Valladares F., Niinemets Ü. (2008). Shade tolerance, a key plant feature of complex nature and consequences. Annu. Rev. Ecol. Evol. Syst. 39, 237–257. doi: 10.1146/annurev.ecolsys.39.110707.173506
Vierheilig H. (2004). Further root colonization by arbuscular mycorrhizal fungi in already mycorrhizal plants is suppressed after a critical level of root colonization. J. Plant Physiol. 161, 339–341. doi: 10.1078/0176-1617-01097
Vierheilig H., Coughlan A. P., Wyss U., Piché Y. (1998). Ink and vinegar, a simple staining technique for arbuscular-mycorrhizal fungi. Appl. Environ. Microbiol. 64, 5004–5007. doi: 10.1128/AEM.64.12.5004-5007.1998
Violle C., Enquist B. J., McGill B. J., Jiang L., Albert C. H., Hulshof C., et al. (2012). The return of the variance: Intraspecific variability in community ecology. Trends Ecol. Evol. 27, 244–252. doi: 10.1016/j.tree.2011.11.014
Wagg C., Bender S. F., Widmer F., van der Heijden M. G. A. (2014). Soil biodiversity and soil community composition determine ecosystem multifunctionality. Proc. Natl. Acad. Sci. U.S.A. 111, 5266–5270. doi: 10.1073/pnas.1320054111
Whipps J. M. (2004). Prospects and limitations for mycorrhizas in biocontrol of root pathogens. Can. J. Bot. 82, 1198–1227. doi: 10.1139/b04-082
Wipf D., Krajinski F., Tuinen D., Recorbet G., Courty P.-E. (2019). Trading on the arbuscular mycorrhiza market: from arbuscules to common mycorrhizal networks. New Phytol. 223, 1127–1142. doi: 10.1111/nph.15775
Witzell J., Martín J. A. (2008). Phenolic metabolites in the resistance of northern forest trees to pathogens — past experiences and future prospects. Can. J. For. Res. 38, 2711–2727. doi: 10.1139/X08-112
Xi N., McCarthy-Neumann S., Feng J., Wu H., Wang W., Semchenko M. (2023). Light availability and plant shade tolerance modify plant-microbial interactions and feedbacks in subtropical trees. New Phytologist. 238, 393–404. doi: 10.1111/nph.18737
Yang H., Zang Y., Yuan Y., Tang J., Chen X. (2012). Selectivity by host plants affects the distribution of arbuscular mycorrhizal fungi: evidence from ITS rDNA sequence metadata. BMC Evolutionary Biol. 12, 50. doi: 10.1186/1471-2148-12-50
Zamioudis C., Pieterse C. M. J. (2012). Modulation of host immunity by beneficial microbes. MPMI 25, 139–150. doi: 10.1094/MPMI-06-11-0179
Keywords: arbuscular mycorrhizal fungi, lignin, nonstructural carbohydrates, soil-borne microbes, pathogens, phenolics
Citation: Wood KEA, Kobe RK and McCarthy-Neumann S (2023) Tree seedling shade tolerance arises from interactions with microbes and is mediated by functional traits. Front. Ecol. Evol. 11:1224540. doi: 10.3389/fevo.2023.1224540
Received: 17 May 2023; Accepted: 10 October 2023;
Published: 02 November 2023.
Edited by:
Md. Harun-Or- Rashid, Bangladesh Agricultural Research Institute, BangladeshReviewed by:
Mohammad Tofajjal Hossain, Bangladesh Agricultural Research Institute, BangladeshMd. Muzahid E. Rahman, Bangladesh Agricultural Research Institute, Bangladesh
Copyright © 2023 Wood, Kobe and McCarthy-Neumann. This is an open-access article distributed under the terms of the Creative Commons Attribution License (CC BY). The use, distribution or reproduction in other forums is permitted, provided the original author(s) and the copyright owner(s) are credited and that the original publication in this journal is cited, in accordance with accepted academic practice. No use, distribution or reproduction is permitted which does not comply with these terms.
*Correspondence: Katherine E. A. Wood, d29vZGthdDdAbXN1LmVkdQ==