- 1U.S. Geological Survey, Western Ecological Research Center, Davis, CA, United States
- 2Department of Biology, University of North Florida, Jacksonville, FL, United States
- 3Oregon State University, Department of Fisheries, Wildlife and Conservation Sciences, Corvallis, OR, United States
- 4Suisun Resource Conservation District, Suisun City, CA, United States
Understanding the drivers of variability in plant diversity from local to landscape spatial scales is a challenge in ecological systems. Environmental gradients exist at several spatial scales and can be nested hierarchically, influencing patterns of plant diversity in complex ways. As plant community dynamics influence ecosystem function, understanding the drivers of plant community variability across space is paramount for predicting potential shifts in ecosystem function from global change. Determining the scales at which stress gradients influence vegetation composition is crucial to inform management and restoration of tidal marshes for specific functions. Here, we analyzed vegetation community composition in 51 tidal marshes from the San Francisco Bay Estuary, California, USA. We used model-based compositional analysis and rank abundance curves to quantify environmental (elevation/tidal frame position, distance to channel, and channel salinity) and species trait (species form, wetland indicator status, and native status) influences on plant community variability at the marsh site and estuary scales. While environmental impacts on plant diversity varied by species and their relationships to each other, overall impacts increased in strength from marsh to estuary scales. Relative species abundance was important in structuring these tidal marsh communities even with the limited species pools dominated by a few species. Rank abundance curves revealed different community structures by region with higher species evenness at plots higher in the tidal frame and adjacent to freshwater channels. By identifying interactions (species–species, species–environment, and environment–trait) at multiple scales (local, landscape), we begin to understand how variability measurements could be interpreted for conservation and land management decisions.
1 Introduction
Natural and physical processes determine plant community organization along environmental gradients; yet, understanding these relationships is challenging given that numerous processes occur across multiple spatial scales. Vegetation communities are often influenced by biotic and abiotic factors (and their interactions) such as weather (temperature and precipitation); substrate (e.g., moisture, nutrients, microbes); topography; competition and facilitation; and disturbances such as drought, fire, and herbivory (Bridge and Johnson, 2000; Van der Heijden et al., 2008, Brooker et al., 2008; Clarke et al., 2010; Mokany et al., 2022; Xi et al., 2022). Climate-driven vegetation changes are well documented globally over the Holocene (e.g., Neumann et al., 2010; Marquer et al., 2017) and in multiple ecosystems from alpine and tundra to temperate forests (Michelsen et al., 2011; Oakes et al., 2014; Maliniemi et al., 2018; Song et al., 2018). Impacts from disturbances and changes in diversity drivers have also been shown to be scale dependent (Graham et al., 1990; Chaneton and Facelli, 1991; Bernhardt-Römermann et al., 2015). In managed tidal wetlands, plant diversity at local versus landscape scales was dependent on variables such as salinity gradients and water management (Jones et al., 2021). Differences in species rank and relative abundance across temporal and spatial scales can result from changes in community diversity and response to the environment (Jones et al., 2017; Avolio et al., 2019).
Tidal marshes are highly productive, dynamic ecosystems that help support estuarine biodiversity, confer flood protection, and have aesthetic and recreational value (Barbier et al., 2011). Marsh environmental gradients are guided by elevation and tidal range (Rogers and Woodroffe, 2015) as well as past hydrological manipulations (Oosterlee et al., 2018). Tidal marshes are dominated by halophytic plants adapted to natural flooding regimes (Adam, 1993), with flooding controlling local site geomorphology, nutrient availability, soil and water salinity, and soil redox. These flooding regimes influence the zonation and productivity of marsh halophytic plant species (Engels et al., 2011; Janousek et al., 2016). Species zonation is also impacted by species-level interactions (Bertness, 1991; Crain et al., 2004). Human activities and disturbances have had profound influences in tidal marshes, altering environmental conditions such as flooding patterns, sediment availability, nutrient levels, contaminants, water quality, and the introduction of invasive species (Takekawa et al., 2006; Gilby et al., 2021) and affecting species distributions (Gedan et al., 2009).
Vegetation patterns are important indicators of ecosystem transformations from anthropogenic stressors such as land-use change (Tasser and Tappeiner 2002), non-native species invasion (Sundaram and Hiremath, 2012), and water diversion (Elmore et al., 2003). For example, land area may change when freshwater diversion or reconnections occurs (White et al., 2023) and plant communities may change with diking of low tidal lands (Mora and Burdick, 2013) or seasonal impoundment of tidal marshes (Jones et al., 2021). Large scale ecosystem transformations have been documented over the last century primarily due to human disturbance, including modification to hydrologic conditions (Busch et al., 1998). In the Everglades of southeast Florida, USA, the marsh vegetation communities have changed over the last 80 years due to road and levee building and sea-level rise (SLR) (Ross et al., 2000). A similar type of comparison study documented a stable vegetation community in Greenland over 40 years, possibly due to the small magnitude of temperature change (Daniëls et al., 2011).
The stress gradient hypothesis is one framework for understanding plant community organization (Bertness and Callaway, 1994; Maestre et al., 2005; Lortie and Callaway, 2006; Maestre et al., 2009). The hypothesis states that biotic interactions are driven by facilitation (positive interactions) under conditions of high abiotic stresses (e.g., temperature, salinity, inundation) and competition under more benign conditions (Maestre et al., 2006). Considering that tidal marshes are naturally a stressful environment it can be difficult to determine if plant community organization is driven by facilitation or competition as outlined by the stress gradient hypothesis. A conceptual model for tidal marshes states that plant community composition is driven primarily by the abiotic factors of the system (Kirwan and Guntenspergen, 2015). In a mesocosm experiment, Schile et al. (2017) demonstrated that facilitation did not occur between two marsh sedges when increased flooding was simulated. In a field and greenhouse experiment, Morzaria-Luna and Zedler (2014) found that the type of stress gradient, salt marsh species, and experiment duration determined the role of competition and facilitation. The spatial scale of a study could also feasibly impact how environmental gradients shape species interactions and diversity.
Understanding the influence of stress gradients on plant communities is also important in preparing for future climate impacts (Bertness and Ewanchuk, 2002). Climate change and SLR will cause a substantial shift in several fundamental abiotic characteristics of estuaries (Parker and Boyer, 2019) but impacts to vegetation communities may be less clear and scale dependent. Accelerating ocean and atmospheric warming, changing water salinity (Cloern et al., 2011), fog, freshwater flow from tributaries, and SLR (IPCC, 2022) will alter the condition and distribution of marshes. These alterations can become “early indicators” of estuary change (Rogers et al., 2014; Garner et al., 2015).
Given that plant diversity and distribution influence ecosystem function, identifying the drivers of these patterns at multiple scales is paramount for understanding potential shifts in ecosystem function and managing for climate impacts. We conducted an analysis on a large vegetation, elevation, water level, and channel salinity dataset compiled over a decade to examine how gradients of flooding influence tidal marsh vegetation patterns across multiple scales in the San Francisco Bay Estuary, California, USA. Our questions were: (1) Which environmental gradients influence species diversity at the site and estuary scale? (2) Are species correlations impacted by environmental gradients? (3) Do species traits explain environmental drivers of diversity? and (4) Do differences in species rank and relative abundance influence the structure of marsh communities given the limited species pools?
2 Methods
2.1 Study sites
The San Francisco Bay Estuary (SFBE) in California is the second largest estuary in the United States and comprises nine counties with a population of over 8 million people (United States Census Bureau, 2021). The estuary has a Mediterranean climate with mild rainy winters and cool foggy summers. The weather is largely influenced by the cold water of the California Current flowing toward the equator year-round and the Davidson Current bringing warmer water toward the pole during the winter months. The SFBE is made up of multiple regions with different environments – South San Francisco Bay, Central San Francisco Bay, San Pablo Bay, Suisun Bay, and the Delta (Figure 1). In this inverted estuary, the Sacramento and San Joaquin Rivers flow through the Delta into brackish Suisun Bay and through the narrow Carquinez Strait into the greater San Francisco Bay embayment (Cloern and Jassby, 2012). Tidal marine waters are exchanged through the Golden Gate to the Pacific Ocean. The tidal regime is mixed semidiurnal with an average diurnal range of 2.6 m in South San Francisco (SF) Bay, 1.8 m in San Pablo Bay, and 1.2 m in the Delta (NOAA tide stations 9414509, 9415252, 9415316; https://tidesandcurrents.noaa.gov). Despite its highly urbanized landscape (Nichols et al., 1986), the SFBE is home to an array of wildlife that use tidal wetlands, including state and federally listed species such as the Chinook salmon (Oncorhynchus tshawytscha), California Ridgway’s Rail (Rallus longirostris obsoletus), salt marsh harvest mouse (Reithrodontomys raviventris), Delta smelt (Hypomesus transpacificus), and longfin smelt (Spirinchus thaleichthys) (California Endangered Species Act 1970; Federal Endangered Species Act 1973; California Natural Diversity Database (CNDDB), 2023). It is important habitat for migratory and nesting birds, supporting more than 50% of the Pacific flyway waterfowl and millions of shorebirds (Takekawa et al., 2001). Tidal marshes in the more saline parts of the estuary generally lie above mean tide level (MTL) and are characterized by relatively flat elevation platforms occurring around mean high water (MHW) (Takekawa et al., 2013a). Extensive modifications of the estuary have resulted in 80% loss of historic tidal wetlands, but restoration efforts are ongoing (Marcus, 2000).
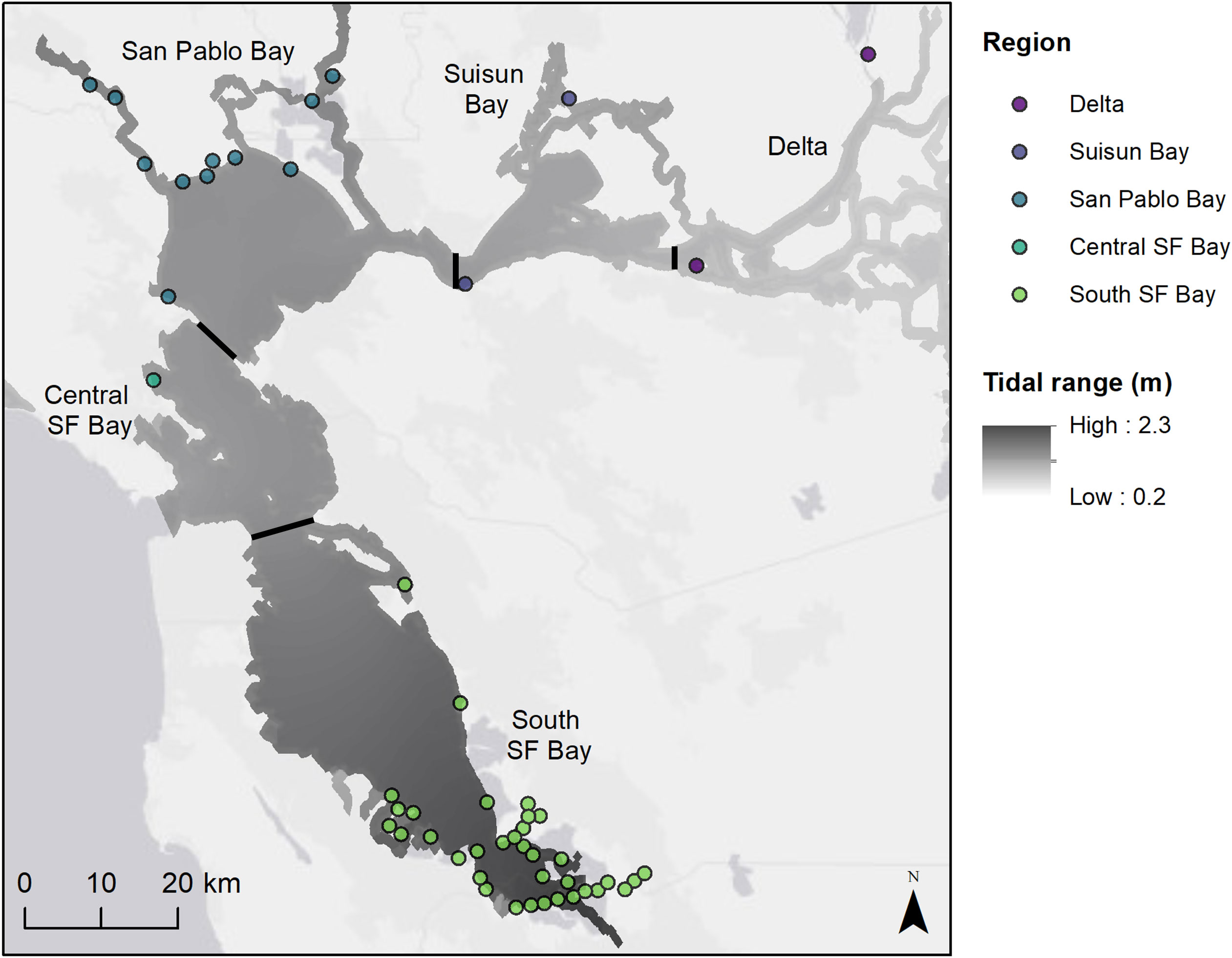
Figure 1 Map of study sites colored and labeled by region (sub-embayments). Tidal range is displayed in greyscale from low to high (NOAA VDATUM model; Parker et al., 2003).
For this effort, fifty-one tidal marsh sites across five regions (sub-embayments) were surveyed in the Delta, Suisun Bay, San Pablo Bay, Central SF Bay, and South SF Bay (Figure 1). These sites varied in geographic size, sample size, geomorphic setting, and tide range (Table S1). Some sites were close in proximity, such as many of the South Bay sites. However, sites were separated by large channels or land features that delineated their physical boundaries.
2.2 Field surveys and environmental data
Vegetation surveys spanned ten years, from July 2008 to October 2018 (Table S1). A total of 5,026 plots were surveyed. Most plots were positioned on transects along an elevation gradient, while non-transect plot placement was used at one site (Arrowhead Marsh) due to its small size and the desire to avoid endangered wildlife species. Most transects were evenly distributed across each site, where possible, to capture spatial variability along elevation and distance gradients. At each plot, percent cover of all plant species, bare ground, and litter was visually assessed within a 0.25 m2 quadrat. Total plant cover in a plot could exceed 100 percent due to vegetation layering. Bare ground and litter cover was estimated as total area visible through the vegetation from above the plot. Vascular plant nomenclature followed Baldwin et al. (2012). Geographic position (in UTM) and elevation (North American Vertical Datum of 1988, NAVD88) were recorded at each plot. Sampling season was not expected to significantly influence species identification and cover estimates. The Mediterranean climate of the SFBE with short mild winters creates fairly unseasonal wetlands. Most of the wetland species are perennial and identifiable throughout the year.
Environmental gradients were selected based on previous studies that identified soil salinity and tidal inundation as the most influential drivers of tidal marsh vegetation structure (Engels and Jensen, 2009; Watson and Byrne, 2009; Janousek and Folger, 2014). Channel salinity was calculated at the site or multi-site scale (in PSU, Table S1) with data from locally deployed water sensors or from San Francisco Bay National Estuarine Research Reserve (NERR) sites (Takekawa et al., 2013a, Thorne et al., 2019; Table S2). Salinity dataloggers (Solinst and Odyssey) were programmed to record specific conductivity (in µS/cm) at 12-minute (Solinst) or 30-minute (Odyssey) intervals and were deployed for a minimum of one year. Annual mean salinity was calculated across the deployment period and converted to PSU. Salinity values were assigned to each marsh based on nearest available measurements, from either a NERR site or logger deployment. All salinity values represent marsh channel or creek conditions, and not soil porewater salinity.
A survey-grade Real Time Kinematic (RTK) global positioning systems (GPS) rover was used to measure location and elevation (± 1 cm horizontal, ± 2 cm vertical manufacturer-stated accuracy; Leica Geosystems Inc., Norcross, Georgia). Rover positions were received in real time from the Leica Smartnet system using a CDMA modem (http://www.leica-geosystems.us/en/index.htm). The WGS 84 ellipsoid model was used for horizontal positioning and NAVD88 for vertical positioning. Rover accuracy and precision were evaluated by measuring positions at local National Geodetic Survey benchmarks; all errors were within the stated rover error. Elevation data was converted to z* (z* = [NAVD88 − MTL]/[MHHW – MTL]), a unitless measure of elevation relative to the local tidal frame which accounts for variation in tidal range and allows for direct comparison across sites (Swanson et al., 2014).
Local tidal datums were calculated from multiple sources including NOAA tide stations (https://tidesandcurrents.noaa.gov/), deployed water level loggers, and NOAA’s VDATUM model (Parker et al., 2003; Table S2). Water loggers (Solinst) were deployed at select sites and programmed to record water level information at 6-minute intervals. Data were corrected for atmospheric pressure with barometric data from barometric dataloggers (Solinst; Takekawa et al., 2013a; Thorne et al., 2019). These water level values were used to calculate mean higher high water (MHHW) while mean tidal level (MTL) was estimated from NOAA’s VDATUM model (Parker et al., 2003). At some sites, we used tidal datums from nearby NOAA tide gages where local loggers were absent (Table S2).
Distance to channel was calculated as the distance (in meters) between each plot and the digitalized boundaries of all nearby channels and bays. Channels were digitized based on 2020 NAIP imagery supplemented with LiDAR when necessary. The centerline was digitized on small channels (~1 – 2.5 m wide), while both edges were digitized on large channels (> 2.5 m), bays, and rivers.
2.3 Statistical analyses: site and estuary abundance models
To understand the relationship between plant communities and environmental gradients at different scales, generalized linear latent variable models (GLLVMs; Skrondal and Rabe-Hesketh, 2004) were analyzed in R version 4.2.2 (R Core Team, 2022; package ‘GLLVM’; Niku et al., 2019; Niku et al., 2021) at the site and estuary levels. This type of model incorporates latent variables that quantify species response correlations and environment-trait interactions, known as fourth-corner terms (Skrondal and Rabe-Hesketh, 2004; Niku et al., 2021). At the site scale, GLLVMs were built with elevation (tidal position) and channel distance as environmental covariates. At the estuary scale, one model was built using all plot data. Site sampling intensity was accounted for by including row effects by site. Salinity was added as an environmental covariate to the estuary model as salinity data were collected at the site/multisite levels. All models were fit using negative binomial distributions. They identified species with strong associations, either negative or positive, between environmental covariates and species presence and abundance. Strong associations were defined by models with confidence intervals that did not overlap with zero. Associations were used to identify how elevation (tidal position), channel distance, and salinity influenced plant presence and abundance at the site and estuary scales. Species that changed associations between the site and estuary models were identified to examine scale-dependency.
Species associations from site and estuary abundance models were combined with percent cover values from field surveys to calculate site-specific cover of species with strong associations to each environmental variable. All species with strong associations (either negative or positive for each environmental covariate) were identified by site, and their mean percent cover values were added together to compute a total percent cover of strongly associated species. Sites with high total cover values indicate marsh communities most influenced by elevation, channel distance, or salinity.
2.4 Species correlations
To investigate the impacts of environment conditions on species interactions, species correlation matrices were compared between models with and without environmental predictors (elevation, channel distance, and salinity). Species positively correlated with each other could indicate facilitation while competition may be identified by negative correlations. Latent variables used in GLLVMs include correlation values across all model response variables used to create correlation matrices. Species relationship differences across environmental gradients were also identified by comparing correlation matrices from data subset along gradient thresholds.
2.5 Environment–trait interactions
To examine why species differ in their response to environmental drivers, species traits were incorporated into a “fourth corner” estuary model. Species traits included: California native/non-native status, wetland indicator status (upland – almost always occurs in upland habitat; facultative upland – usually occurs in upland habitat; facultative – equally likely in upland and wetland habitats; facultative wetland – usually occurs in wetlands; and obligate – almost always occurs in wetlands), and plant form (tree, shrub, forb/herb, and graminoid) (USDA, 2023; calflora.org; Baldwin et al., 2012). Strong environmental–trait interactions, or fourth corner terms, were identified from model coefficient plots.
2.6 Rank abundance curves
Differences in relative species abundance were examined by creating rank abundance curves (RACs) for each site and region as well as environmental covariates. Species richness and species evenness were evaluated by comparing RACs within regions and across the estuary. RAC differences were compared by site and region using R (package ‘CODYN’; Hallett et al., 2020).
3 Results
3.1 Surveys
Eighty-one species were identified across all sites surveyed (Table S3). The number of species per site ranged from 1 to 31 with an average of 8.0 ± 6.5 species per site. Most plots were dominated by one or two plant species.
3.2 Site and estuary abundance models
At the site scale, elevation/tidal position (z*) was strongly associated with plant cover for more than half of the species (58%; Figure 2A). Most of these species (70%) had a positive association with elevation – they were more abundant at higher elevations. Some species that were more abundant at lower elevations included Schoenoplectus americanus and Spartina foliosa. Distance to channel had a weaker relationship with species abundance with an average association of 52%. Most of these species (73%) had a negative association with distance to channel indicating they were more abundant closer to a tidal channel or bay edge, such as Spartina foliosa.
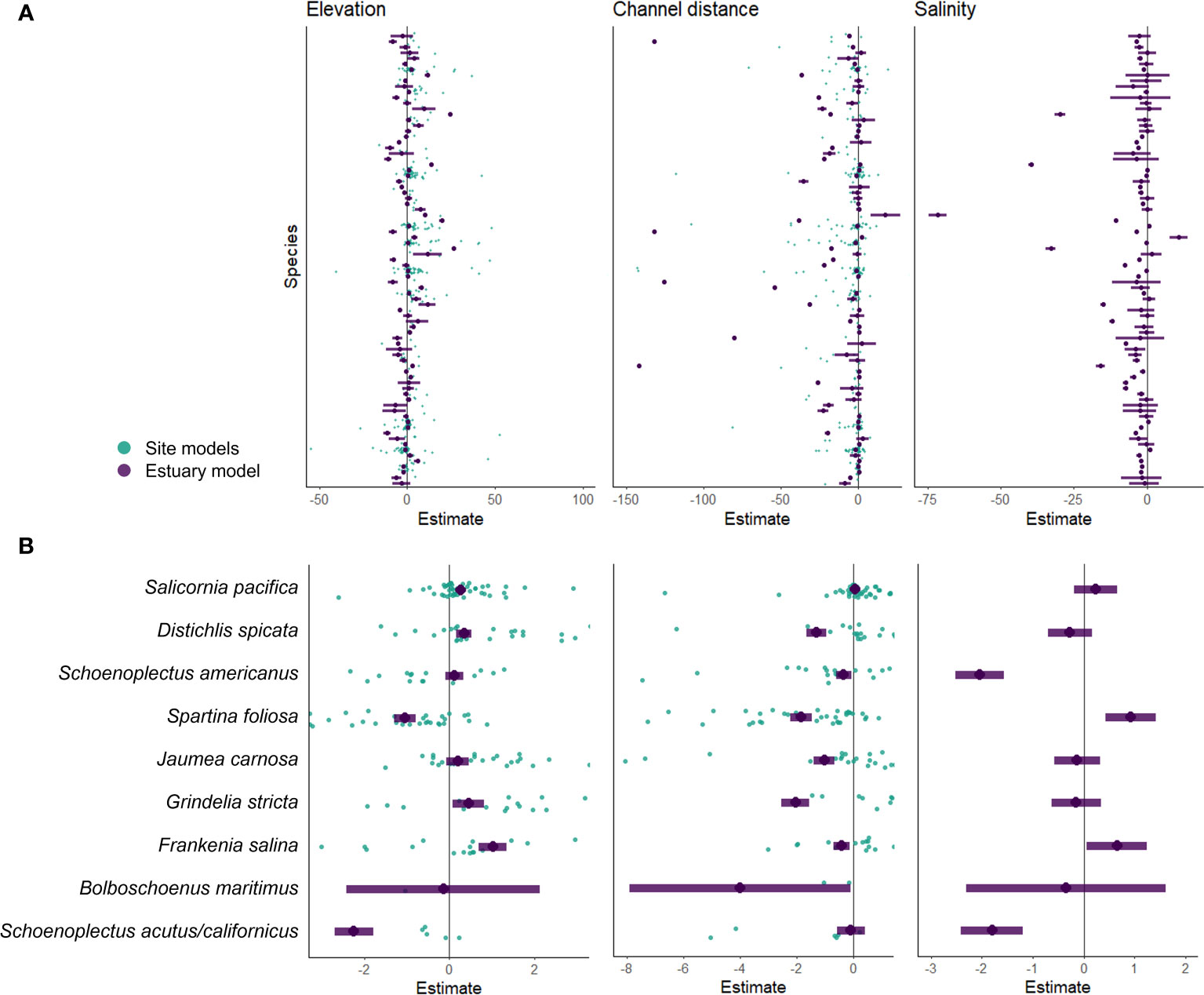
Figure 2 GLLVM species estimates for elevation, channel distance, and salinity covariates for all species (A) and 9 abundant species of interest (B). Teal points are estimated coefficients modeled at the site scale. Purple points and lines are estimated coefficients with 95% confidence intervals modeled at the estuary scale (one value per species).
At the estuary scale, environmental covariates (elevation/tidal position, distance to channel, and salinity) explained about 49% of the total community variation (Figure 2A). After incorporating region as a variable, the covariates explained about 65% of the variation. Of the three environmental covariates we examined, elevation and distance to channel had the strongest association with species composition. Elevation was associated with species abundance for 64% of the species, distance to channel for 57%, and salinity for 51% (Figure 2A). Coefficient estimates varied between site and estuary scale models as seen in 9 abundant species of interest in the estuary (Figure 2B).
Assigning a single environmental association for each species across the estuary, as opposed to each site, resulted in associations that were either confirmed (no association to positive or negative), changed (negative to positive or vice versa), or lost (positive or negative to no association) (Figure 3). With an increase in sample size, the estuary model confirmed existing positive associations to elevation (tidal position) for species such as Atriplex prostrata and Frankenia salina (Figure 3A). At the estuary level, Typha species had a negative association to elevation (tidal position) but at the site level these species had no strong association or even a positive association to tidal position in the Delta (Figure 3A). Salicornia pacifica had a negative association and no association to tidal position at multiple marsh sites (7 and 25 respectively of 49 sites), yet the estuary model identified a strong positive association (Figure 3A).
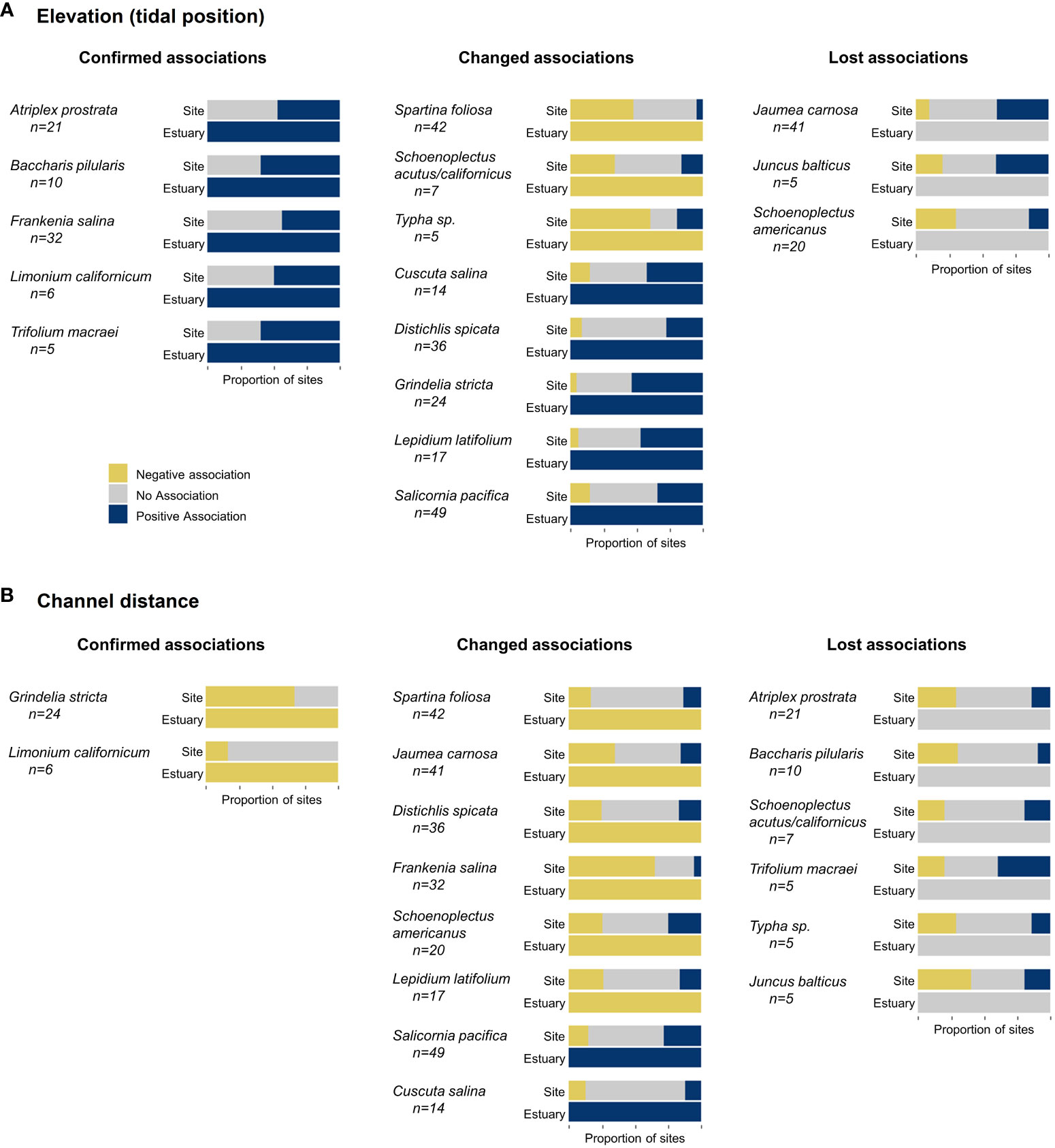
Figure 3 Proportion of marsh sites with negative and positive associations to elevation/tidal position (A) and channel distance (B) from site- and estuary-level modeling. Species are grouped by environmental association differences between scales, including those with confirmed associations (no association to positive or negative), changed relationships (positive to negative or negative to positive), and lost relationships (positive or negative to no association). Species shown here were located at a minimum of 5 marsh sites (total number of sites are listed below each species name).
Schoenoplectus americanus had a strong association to tidal position at most of its marsh sites but did not have a strong association at the estuary scale (Figure 3A).
Similar differences in species association to channel distance occurred between site and estuary models. The increased sample size confirmed negative associations for Grindelia stricta and Limonium californicum, but more species changed or lost associations between scales (Figure 3B). Schoenoplectus americanus had a strong positive association at 5 sites and no association at 10 sites (of 20 sites), yet a negative association at the estuary scale (Figure 3B). Jaumea carnosa had a positive association with channel distance at 7 of 41 sites, including all sites in the Delta and Suisun Bay where it was located, yet a negative association across the estuary. Atriplex prostata had a strong association to channel distance at 9 of 21 marsh sites but did not have a strong association at the estuary scale (Figure 3B).
The total percent cover of species with strong association to these variables differed greatly by site (Figures 4A, C, Table S4). Sites with the highest percent cover of species associated with elevation were in Suisun Bay and the Delta, while the sites with the lowest percent cover were in South SF Bay (Figure 4A). Total percent cover of species associated with channel distance varied across the regions with the highest cover in Suisun Bay (Figure 4C). Given that species associations varied considerably between site models, associations at the site scale did not always correspond to associations at the estuary scale. Spatially, there was a narrower range in percent cover values across sites based on the estuary-wide model (Figures 4B, D, E, Table S4). Most sites had moderate to high cover of species strongly associated with elevation and channel distance (Figures 4B, D). The greatest cover of species associated with salinity occurred at more inland sites associated with freshwater sources in Suisun Bay, the Delta, and the southeastern area of South SF Bay (Figure 4E). More species had strong associations with elevation and channel distance when modeled at the estuary scale compared to the site scale (Figure S5). Species associations were less frequent for channel salinity (Figure S5).
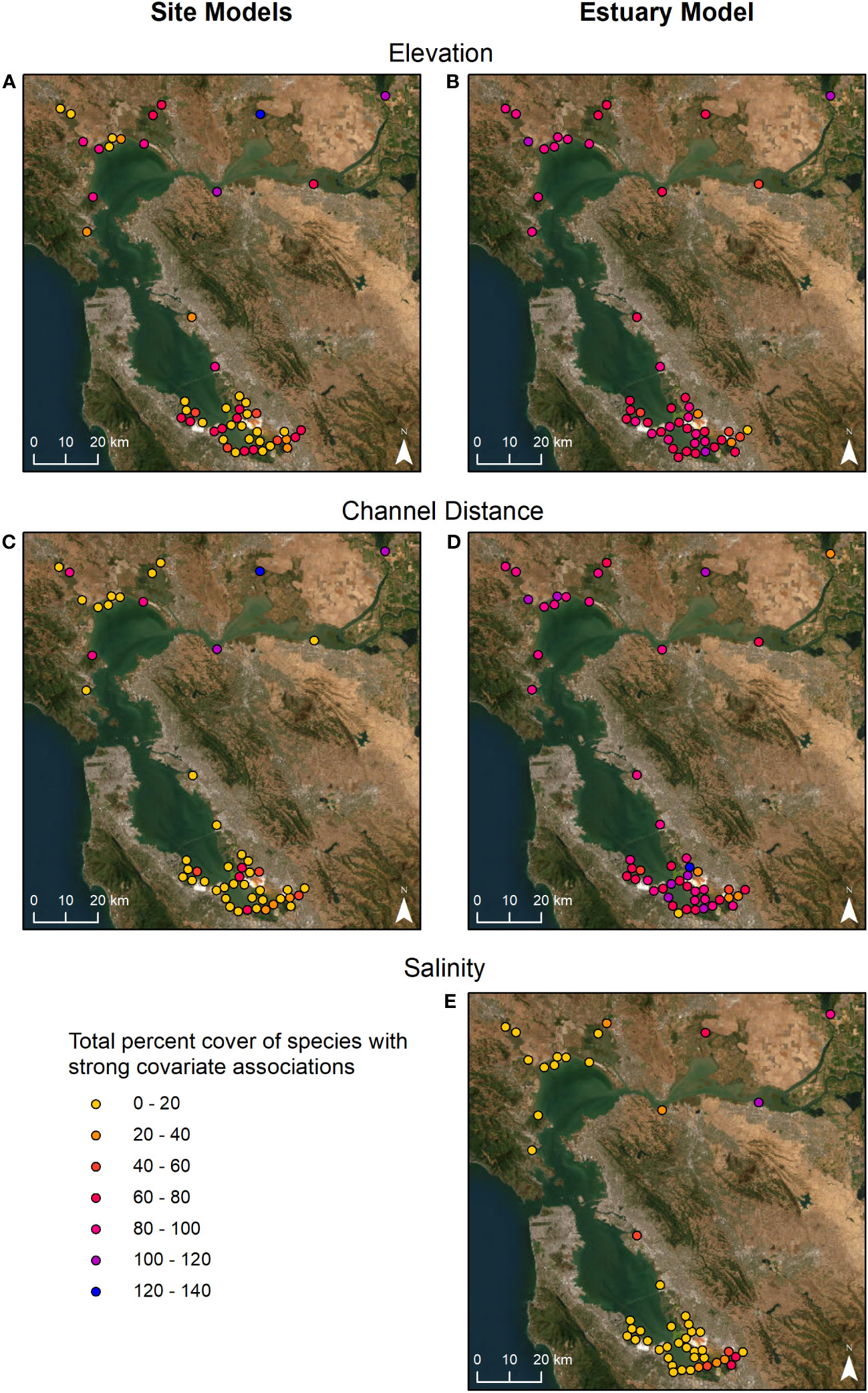
Figure 4 Marsh sites colored by total percent cover of plant species with strong associations to elevation, distance to nearest channel, and salinity modeled at the site (A, C) and estuary (B, D, E) scale.
3.3 Species co-occurrence patterns
Without accounting for environmental conditions, multiple positive species correlations were identified (Figure 5A). When environmental variables were incorporated into the model, almost no species correlations were observed (Figure 5A). Species were most likely found together because they had similar habitat preferences. The environmental gradients explained nearly all the species interactions when modeled across the entire estuary. However, species co-occurrence patterns did vary along the salinity gradient, where both positive and negative correlations were more common adjacent to less saline channels (Figure 5B). Species co-occurrence patterns appeared unaffected by position within the tidal frame and proximity to channel edge (Figure 5B).
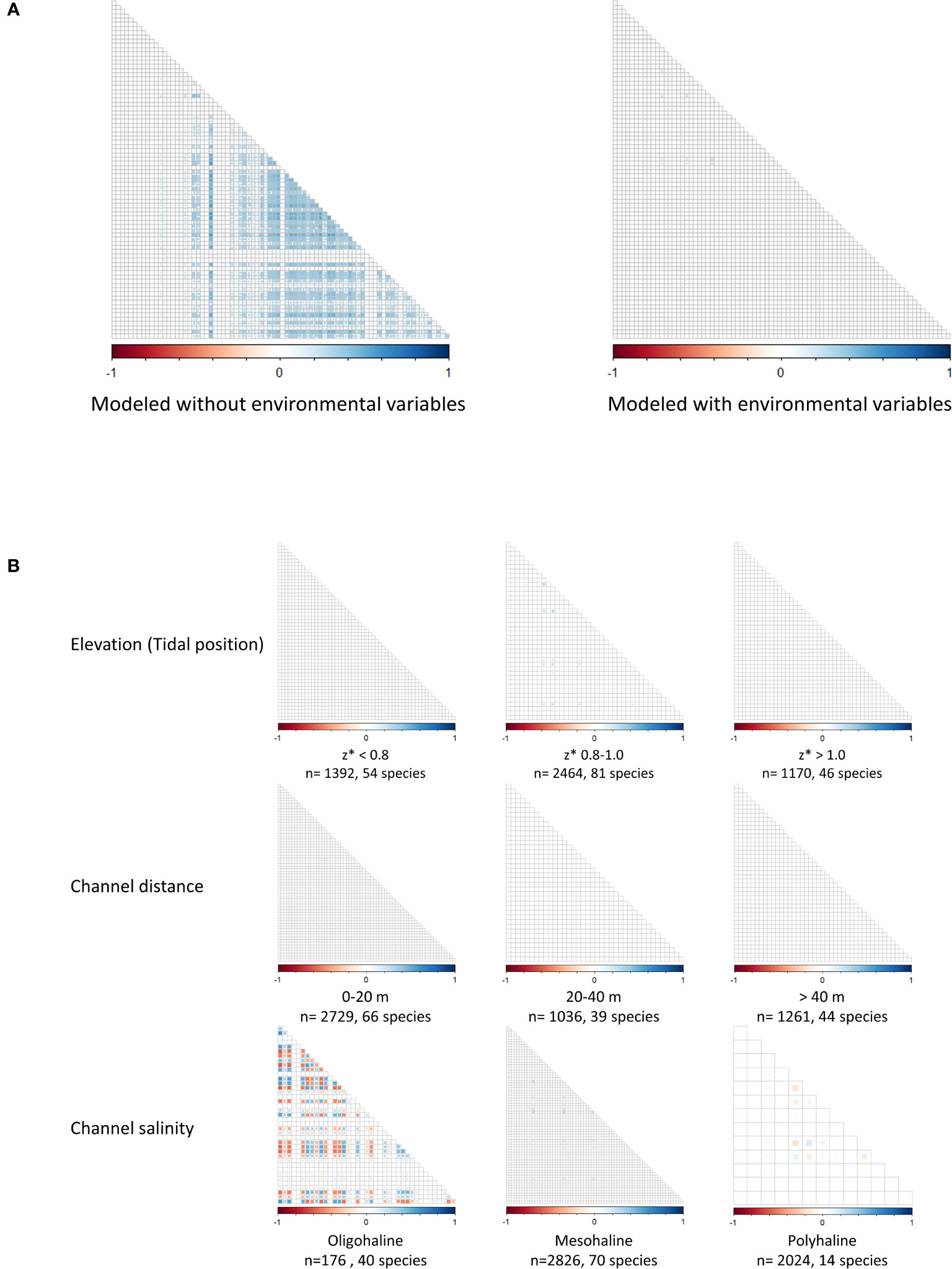
Figure 5 Species correlation matrices modeled with and without environmental variables (A) and along environmental gradients (B) (Elevation/tidal position, Channel distance, Channel salinity). Each gradient dataset includes sample size and number of species. Red squares indicate negatively correlated species, and blue squares indicate positive correlations.
3.4 Environment–trait interactions
Multiple environment–trait interactions were observed between regions and along environmental gradients (Figure 6). As expected, trees were more abundant higher in the tidal frame and farther from channel edges whereas shrubs, forbs/herbs, and graminoids were found closer to channels. Only forbs/herbs showed interactions along the salinity gradient as they were less abundant near saline channels. Species wetland status had no impact on abundance across the elevation gradient. All species had varying degrees of negative association to channel distance regardless of wetland status. Only upland species showed an interaction with salinity as they were more abundant near saline channels.
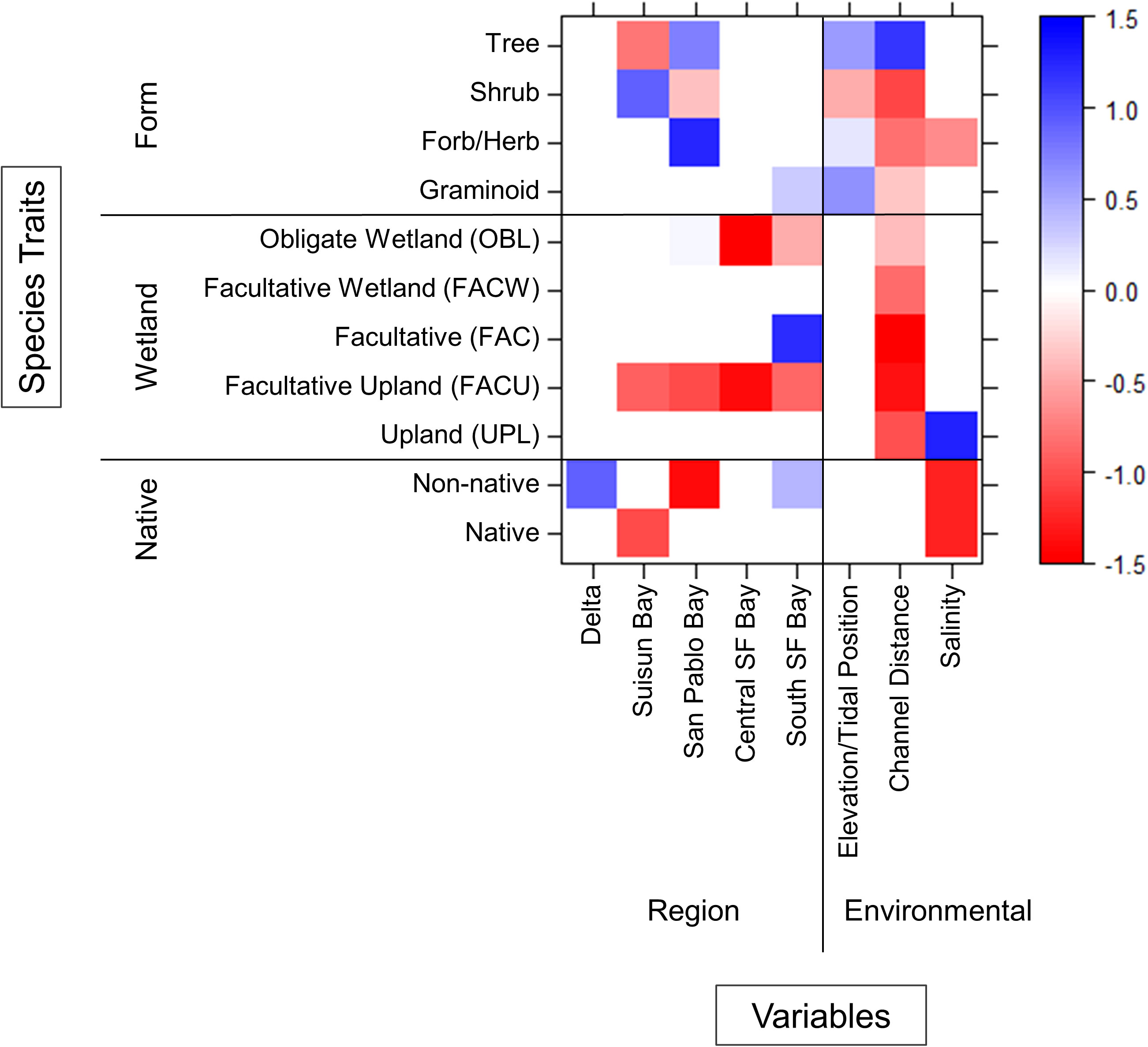
Figure 6 Environment–trait relationships that influence species abundance at the estuary scale. Interactions colored blue indicate positive associations and red indicate negative associations.
The influence of all species traits (form, wetland status, and native status) on abundance varied between regions (Figure 6). Trees were more abundant in San Pablo Bay and less abundant in Suisun Bay whereas shrubs had the opposite relationship. Forbs/herbs were more abundant in San Pablo Bay and graminids more abundant in South SF Bay. Obligate wetland species were less abundant in Central and South SF Bay. Facultative species were more abundant solely in South SF Bay. Facultative upland species were less abundant at all regions except for the Delta. Native plant species were less abundant in Suisun Bay. Non-native species were most abundant in the Delta and South SF Bay and less abundant in San Pablo Bay.
3.5 Rank abundance curves
RACs illustrated different levels of species dominance by site and region (Figure 7). Lower species evenness was observed at sites in San Pablo Bay, Central SF Bay, South SF Bay, and Delta. Most of these sites had similar species diversity, with Salicornia pacifica as the most abundant species (Figures 7B, D–F). The more inland sites at Suisun Bay exhibited higher evenness. Most sites in this fresher part of the estuary (Delta and Suisun Bay) did not have an individual species that accounted for over 50% of the proportional abundance (Figures 7A, B).
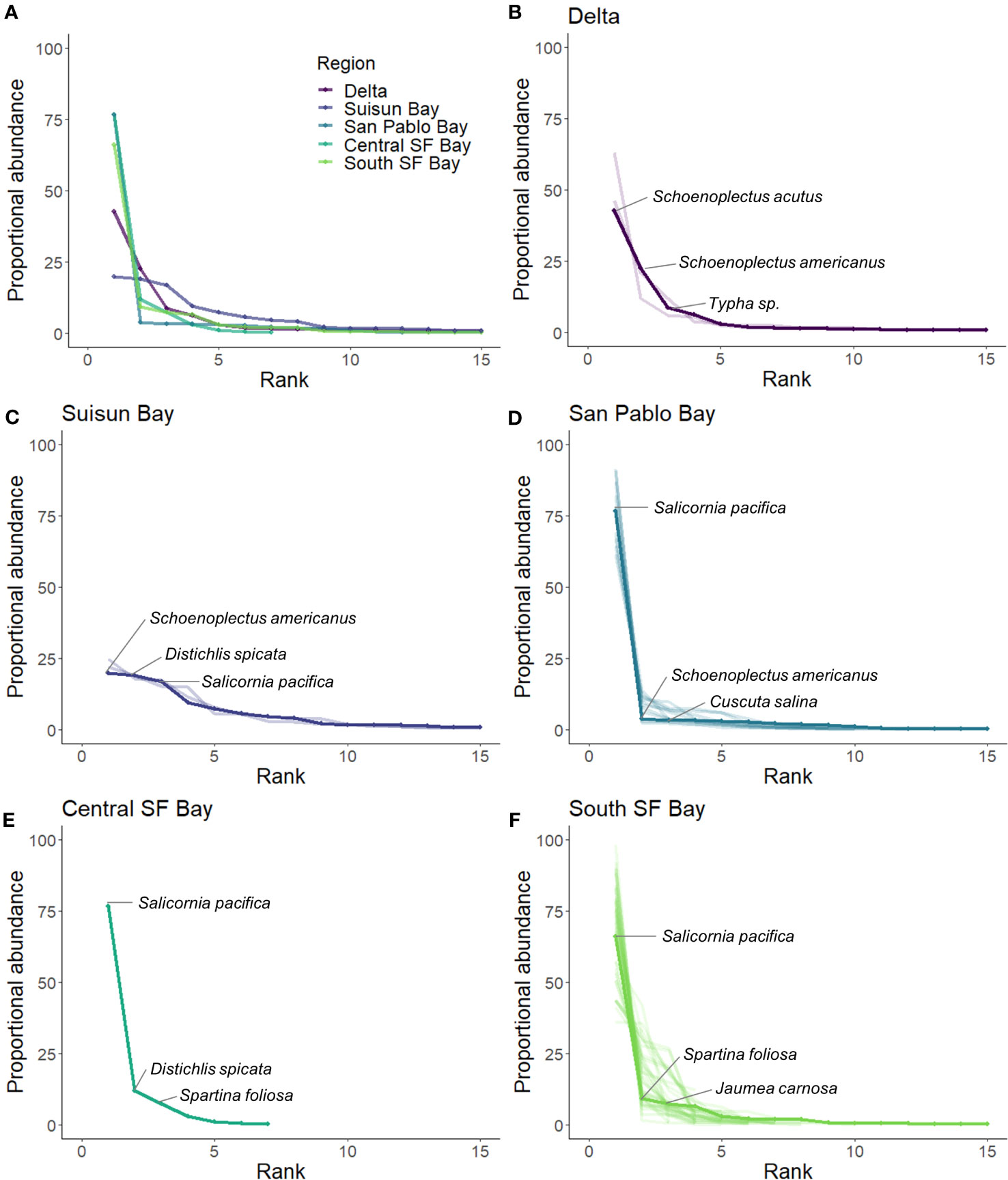
Figure 7 Rank abundance curves (RACs) calculated by region [solid lines; (A–F)] and sites within region [transparent lines; (B–F)]. The three most abundant species are labeled within region.
RACs by environmental gradients demonstrated a clear influence of flooding and salinity on species rank and abundance (Figure 8). Lower species evenness and similar dominant species (Salicornia pacifica and Schoenoplectus acutus) were measured at plots lower in the tidal frame (z* < 1.5; Figure 8A). Plots higher in the tidal frame (z* > 1.5) exhibited higher species evenness with variable dominant species (Festuca microstchys and Baccharis pilularis). There was no measurable difference in species rank or abundance by distance to channel (Figure 8B), Salicornia pacifica was always most abundant. The communities located near oligohaline channels with assumed lower soil salinity had more species evenness, while communities adjacent to more saline channels were dominated by Salicornia pacifica (Figure 8C).
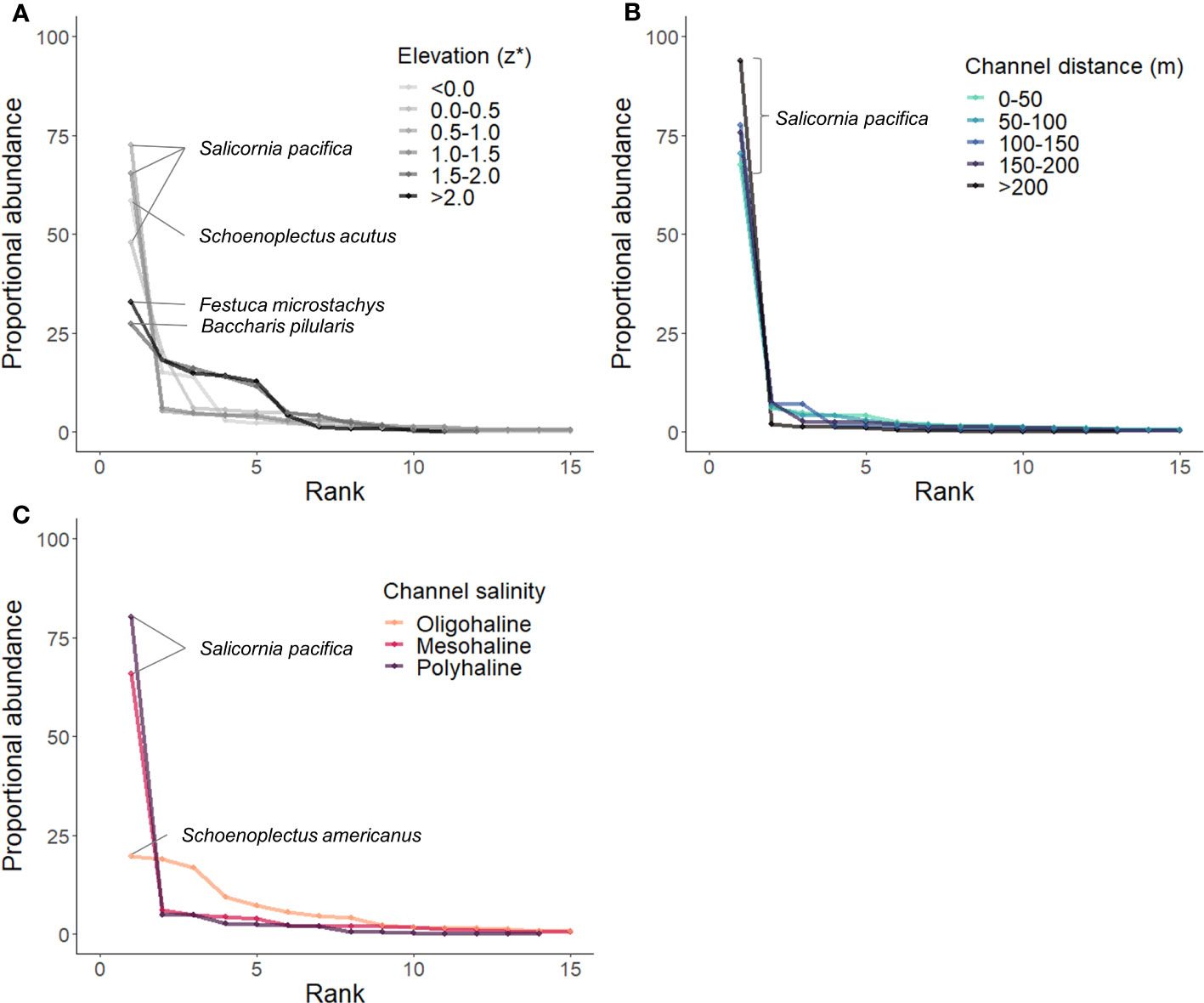
Figure 8 Rank abundance curves (RACs) calculated by stress gradient [(A) Elevation/tidal position, (B) Channel distance, (C) Salinity]. The topmost abundant species are labeled.
Pairwise comparisons of site RACs revealed that sites differed most by species richness, calculated as the difference in richness between samples divided by the number of unique species in both samples (40%; Figure S6A). Species rank was the next most important measure of site difference (25%) followed by species number (22%) and evenness (15%; Figure S6A). Species number is calculated as the difference in species number between samples divided by the total number of species in both samples. While all sites were treated independently in the RAC comparison, sites within the same region would be expected to have similar RACs. The unequal number of sites by region could possibly affect which metric detected the most difference in RACs. However, when comparing differences by regional RACs, the order and magnitude of importance metrics were comparable (species richness – 49%, rank – 27%, species number – 26%, and evenness – 3%) (Figure S6B). Species richness and rank explained most of the variability in the plant communities across the estuary at the site and regional scale.
4 Discussion
Our results showed that vegetation communities were organized within marshes along expected gradients of abiotic drivers (i.e, elevation, flooding). This aligns with other marsh studies that found plant communities organized by climate and human impacts (Ewanchuk and Bertness, 2004), and flooding and salinity tolerances (Muench and Elsey-Quirk, 2019). Multiple manipulation studies have demonstrated the importance of flooding and salinity tolerances to marsh plant distribution and abundance (e.g., Sharpe and Baldwin, 2012; Peng et al., 2018; Buffington et al., 2020). However, plant species differ in their tolerance of abiotic stress or deviation from the optimum conditions, enhancing plant species stratification within a site (e.g., Liancourt et al., 2005). While we found similar drivers of plant diversity at both the site and estuary levels, the magnitude of importance and direction of effects varied by scale, indicating context dependency (Figure 2). Regional or estuary-wide results are useful to identify trends but using them to inform management decisions at specific sites may not be ideal. Given that species associations change from site to estuary scales, local-scale processes and site variables should be investigated.
The stress-gradient hypothesis predicts that relative frequency of plant facilitation and competition will vary inversely with physical stress (Maestre et al., 2005) with the lower limit of marsh plant distribution set by abiotic stress and the upper limit set by biotic competition (Bertness and Ellison, 1987; Menge and Sutherland, 1987; Pennings et al., 2005), similar to our finding of abundant species correlations adjacent to oligohaline channels (Figure 5). Yet, species correlations were not observed higher in the tidal frame or further from channels, at the more stressful end of the inundation gradient (Figure 5). Species richness and evenness was quite high in the oligohaline marshes (Figures 5, 8). Less salt stress, abundant perennial and annual species, and lack of species dominance builds complex community structures in oligohaline marshes (Odum, 1988). This could result in multivariable biotic and abiotic interactions, making it difficult to identify competition and facilitation in these marshes. In the SFBE, plant species diversity decreased with salt tolerant species increase, presumably due to water diversion and dams (Goman et al., 2008). How stress gradients impact plant variability can be unpredictable as species abundance is not solely based on environmental interactions but also species–species interactions and environmental–trait interactions.
4.1 Species relationships are related to wetland structure
Within tidal marshes, plant functional traits have the potential to influence both marsh processes (e.g., accretion, channel formation) and species distributions (Schwarz et al., 2018). In our analysis, there was some variation between the environmental gradients and species trait relationships (Figure 6). Trait relationships varied between regions, possibly signaling additional environmental conditions that were not represented in the models or region-specific species responses. Tidal wetlands differ in channel structure, hydrology, elevation, and salinity which can result in variable relationships with common plant species (Watson and Byrne, 2009; Janousek and Folger, 2014). Understanding linkages between biogeomorphic features in estuaries and plant species composition is key to understanding vegetation community organization at small and large spatial scales (Van de Koppel et al., 2012). While all study sites were tidal with similar biogeomorphic features, the patterns of old dikes, berms, and levees throughout each site could have impacted plant communities at a much finer scale. Individually constructed earthen levees can impact ecosystem processes at coastal marshes long after abandonment (Hall et al., 2022). These “hidden levees” can cause a disconnect between channel distance, elevation, and inundation regime resulting in less flood tolerant vegetation and shallower organic rich soils landward of the abandoned levees (Hall et al., 2022). More research is needed on the impacts of past biogeomorphic features at these sites.
Our analysis showed that distance to channel and elevation relative to tidal flooding were the primary drivers for vegetation community structure and plant variability, but the magnitude of importance of these drivers varied by marsh site and scale. However, other factors related to flooding could be useful in determining stress gradient impacts, such as inundation frequency and duration. Future studies would benefit from more in-depth interaction measurements and species-specific responses to environmental stress gradients. Different halophyte plant species have varying tolerances to flooding; for example Spartina foliosa (California cordgrass) can tolerate extended flooding and Salicornia pacifica (pickleweed) is tolerant of higher salinities (Woo and Takekawa, 2012; Takekawa et al., 2013b; Gallego-Tévar et al., 2020). The spatial variability and distribution of plants by traits can be used to infer multiple habitat characteristics, but recent research suggests that there are limits to the extent to which traits can be related to ecosystem properties (Van der Plas et al., 2020).
4.2 Scale is important in spatial trends
This analysis identified elevation (tidal frame position), channel distance, and channel salinity as variables that strongly influenced abundance for more than half of the species at both the marsh site and estuary scales. Previous research found similarly strong responses of plant diversity to channel distance (Sanderson et al., 2001). The observed difference in magnitude of effect is supported by previous work which found plant diversity was dependent on scale (Jones et al., 2021; Korell et al., 2021), elevation (Janousek et al., 2019), and salinity (Bonin and Zedler, 2008). At the site scale, the highest total cover of species associated with elevation were in the Delta and Suisun Bay, possibly indicating more zonation in areas with lower tidal ranges and an increase in plant cover related to brackish and freshwater conditions; these marshes are dominated by grass and graminoid families such as Poacae (grasses), Cyperaceae (sedges), and Juncaceae (rushes). Sites with high cover associated with channel distance were spread across the estuary at both the marsh site and estuary scales. Many marshes have higher cover near channels due to the presence of Grindelia stricta (gumplant) and Salicornia pacifica (pickleweed). There are other additional factors with site-specific gradients, such as soil salinity, that may contribute to the importance of channel distance in species abundance. Sites with freshwater influence (the Delta, Suisun Bay, inland South SF Bay) had more species with abundance driven by channel salinity. Future studies should include soil salinity, rather than channel salinity, to capture site variability and improve site-level model coefficient strength.
As sea levels rise, marsh plant communities will shift from primarily high marsh species to low marsh species (Donnelly and Bertness, 2001; Wasson et al., 2013), significantly changing habitats. These types of plant species shifts have been projected under accelerating SLR scenarios, demonstrating drastic changes over the coming century with loss of relative elevation (Schile et al., 2014; Thorne et al., 2018; Buffington et al., 2021). At the site level, Miner Slough in the Delta and Coyote Creek in South SF Bay had the highest plant cover negatively associated with salinity. In other words, plant abundance was highest at low salinities throughout these sites, making them particularity vulnerable to saltwater intrusion from SLR. Miner Slough is a freshwater tidal marsh site located off the Sacramento River and the only freshwater Delta site in our study; therefore, a larger sample size of similar marshes is needed to determine if this pattern holds. Coyote Creek marsh is located on a large channel with freshwater input from an upland sewage treatment plant, and therefore, is a unique case study in SFBE.
Species were found to respond differently to environmental factors depending on the scale. For example, Schoenoplectus americanus, a bulrush native to CA, exhibited a strong positive association with elevation in the estuary scale model and in multiple, but not all, marsh site models. Strong negative associations to elevation were documented at the two Suisun Bay sites as well as half of the San Pablo Bay sites. Similar site-specific variations in plant vertical zonation have been previously observed along the Pacific coast (Janousek et al., 2019). As an obligate wetland species, S. americanus is expected to be more abundant closer to channels (as was seen in the estuary model), but some site models documented the opposite relationship (namely both Suisun Bay sites and a few sites in San Pablo Bay and South SF Bay). Species-environment associations that changed between site and estuary models indicate scale dependency for select species and environmental gradients (Figure 3). A combination of multiple environmental factors, rather than a single gradient, is likely responsible for marsh plant zonation (Eleuterius and Eleuterius, 1979; Silvestri et al., 2005). Other factors with the potential to influence plant diversity and composition at different scales include competition (Costa et al., 2003), microtopography (Courtwright and Findlay, 2011), soil salinity (He et al., 2011), herbivory (Rand, 2002; Elschot et al., 2017), geomorphic setting (Shipley, 2010), and local climate (De Leeuw et al., 1991). There could be fewer species capable of withstanding saline conditions, impacting diversity and rank abundance. Saltwater intrusion in some marshes has also been shown to strongly affect species composition rather than factors such as biomass production (Li and Pennings, 2019). More investigation is needed to understand ecosystem wide drivers of plant communities, which could be done with remote imagery (e.g., Li et al., 2005; Rosso et al., 2005; Adam et al., 2010) and long-term monitoring of abiotic drivers (Trowbridge et al., 2016).
The difference in plant associations from the site to estuary scales and overall trait interactions could be influenced by unequal samples within regions. South SF Bay had the most sites (35 sites; 1,567 plots) and San Pablo Bay had the most plots (11 sites; 2,864 plots) compared to Suisun Bay (2 sites; 176 plots), the Delta (2 sites; 88 plots), and Central SF Bay (1 site; 361 plots). The dataset also contained unequal covariate distributions with most plots existing close to channels, near MHHW (z* = 1), and adjacent to mesohaline channels (Figures S1–S4). Given the deficient quantity of freshwater tidal marsh sites in the estuary due to development, there was not enough power to confidently detect the loss of rare plants, something common to freshwater systems since these communities experience drastic changes in response to shifting environmental gradients (Neubauer, 2013; Li et al., 2022).
Species associations with environmental gradients were sometimes stronger (larger coefficients and greater species cover; Figures 2, 4) at the site scale compared to the estuary scale, but when averaged across all sites, the estuary scale model had more species associations (Figure S5). Even though species pools were fairly similar across all sites (10 species made up 90% of occurrences), the relative abundance was determined by prevailing environmental conditions specific to the site. Local changes in environmental drivers influence plant communities within a small regional inference space, as seen in the variable RACs (Figures 7, 8). Understanding how local conditions influence plant variability is crucial in land management and conservation. At landscape scales, changes in vegetation patterns have been documented by large disturbance events (e.g., fire; Taylor et al., 2021) and human impacts (e.g., human pressure; Malavasi et al., 2016). Ecological studies at small scales would require different interpretations than a large-scale study.
4.3 Climate change relevance
There has been a growing interest in how best to track the effects of our changing climate. Increased flooding due to SLR can lead to vegetation loss and conversion of marsh to unvegetated mud flat (Orson et al., 1985; Morris et al., 2002), as well as an increase in erosion, creation of new channels, and the expansion of existing channels (Moffett and Gorelick, 2016). Increasing inundation can affect salinity concentrations leading to changes in plant communities, biomass, and productivity (Engels and Jensen, 2009; Ryan and Boyer, 2012; Janousek and Mayo, 2013; Snedden et al., 2015; Janousek et al., 2016). Previous modeling in California marshes showed drastic changes in marsh vegetation under SLR scenarios (Thorne et al., 2018); however, these models are based on generalizations of plant tolerances to flooding and focus on main functional plant groups, therefore missing plant community or diversity concerns that may manifest prior to state change from vegetated to unvegetated systems.
SLR impacts to marsh plant diversity are dependent on species associations and position along environmental gradients. Species more abundant at higher marsh elevations will be negatively impacted by rising sea levels due to loss of habitat, vegetation vigor, and soil condition from increased flooding time (Reed and Cahoon, 1992). If a physical barrier prevents upland migration, the marsh will experience a phenomenon known as “coastal squeeze” (Pontee, 2013). Species with abundance influenced by channel proximity may be negatively impacted as SLR inundates species near water forcing migration from channel widening (Hartig et al., 2002), which narrows existing habitat for species far from water. SLR will also expose more marsh plants to saline water, with saltwater intrusion predicted to be more common in the future (Cloern et al., 2011).
Future SLR is anticipated to lead to flooding, erosion, and saltwater intrusion (Cloern et al., 2011; Thorne et al., 2018). Sweet et al. (2022) project an increase in sea level between 0.3 m and 2.5 m by 2100 for the SFBE, depending on realized levels of global greenhouse gas emissions. The results from this study can be used when considering an unpredictable future related to SLR. Here we identified species community and abundance relationships across the SFBE and investigated how they relate to environmental variables. With rising sea levels, plant communities will shift lower in the tidal frame and experience more frequent saltwater intrusion. This shift is likely to result in less species evenness as select species become dominant in these lower and saltier environments (Figure 8). This type of information can be used by ecologists and wetland managers to project how vegetation communities will change in response to SLR and develop monitoring strategies that are scale dependent. Slight changes in vegetation composition or associations could be early indicators of change within the abiotic conditions of the estuary (Kearney et al., 2011; Schepers et al., 2017; Kutcher et al., 2022). This approach has been successfully deployed to monitor marsh restoration and holds the same premises (Handa et al., 2002).
Large scale analyses for abiotic drivers may be biased by other marsh associations and mask individual drivers at the local scale. At the local scale, site-specific drivers may dominate the influence on changes for species that were associated with high elevation, low salinity, and interior locations (Figure 2). At the regional or estuary scale, species may re-order to look more like the saline San Francisco Bay, dominated by a few species, and less like Suisun Bay with high evenness and patchiness (Jones et al., 2021). This has strong functional implications for carbon and nitrogen cycling (Knox et al., 2018; Windham-Myers et al., 2018; Baldocchi, 2020; Russell et al., 2023), habitat provisioning for threatened and endangered species (smelt, rails), recreation, and aesthetics.
Data availability statement
The datasets presented in this study can be found in online repositories. The names of the repository/repositories and accession number(s) can be found below: Lyndsay L. Rankin, Scott F. Jones, Christopher N. Janousek, Kevin J. Buffington, John Y. Takekawa, Karen M. Thorne, 2023, Marsh vegetation surveys across the San Francisco Bay Estuary, 2008–2018: U.S. Geological Survey Data Release, https://doi.org/10.5066/P94F802H.
Author contributions
SJ and KT conceived the research. LR analyzed the data. JT, CJ, KB, and KT collected the data. All authors contributed to the article and approved the submitted version.
Funding
The author(s) declare financial support was received for the research, authorship, and/or publication of this article. The project described in this publication was supported by funding from the U.S. Geological Survey National Climate Adaptation Science Centers, the National Oceanic and Atmospheric Administration (grant NA15NOS4780171), and the USGS Western Ecological Research Center.
Acknowledgments
We would like to thank land managers from the U.S. Fish and Wildlife Service, California State Parks, NOAA National Estuarine Research Reserve, California Department of Fish and Wildlife, Solano Land Trust, and East Bay Regional Parks District for granting site access to conduct this study. We express thanks to the countless field technicians who helped with these surveys. Thanks to Audrey Colley for ArcGIS assistance and Chase Freeman for data collection. Thanks to Robert Klinger for statistical advice and guidance. The project described in this publication was supported by funding from the U.S. Geological Survey National Climate Adaptation Science Centers, the National Oceanic and Atmospheric Administration (grant NA15NOS4780171), and the USGS Western Ecological Research Center. Any use of trade, firm, or product names is for descriptive purposes only and does not imply endorsement by the U.S. Government.
Conflict of interest
The authors declare that the research was conducted in the absence of any commercial or financial relationships that could be construed as a potential conflict of interest.
Publisher’s note
All claims expressed in this article are solely those of the authors and do not necessarily represent those of their affiliated organizations, or those of the publisher, the editors and the reviewers. Any product that may be evaluated in this article, or claim that may be made by its manufacturer, is not guaranteed or endorsed by the publisher.
Supplementary material
The Supplementary Material for this article can be found online at: https://www.frontiersin.org/articles/10.3389/fevo.2023.1215964/full#supplementary-material
References
Adam E., Mutanga O., Rugege D. (2010). Multispectral and hyperspectral remote sensing for identification and mapping of wetland vegetation: a review. Wetlands Ecol. Manage. 18, 281–296. doi: 10.1007/s11273-009-9169-z
Avolio M. L., Carroll I. T., Collins S. L., Houseman G. R., Hallett L. M., Isbell F., et al. (2019). A comprehensive approach to analyzing community dynamics using rank abundance curves. Ecosphere 10, e02881. doi: 10.1002/ecs2.2881
Baldocchi D. D. (2020). How eddy covariance flux measurements have contributed to our understanding of Global Change Biology. Global Change Biol. 26, 242–260. doi: 10.1111/gcb.14807
Baldwin B. G., Goldman D. H., Keil D. J., Patterson R., Rosatti T. J. (2012). The Jepson manual: vascular plants of California. (Berkeley: University of California Press).
Barbier E. B., Hacker S. D., Kennedy C., Koch E. W., Stier A. C., Silliman B. R. (2011). The value of estuarine and coastal ecosystem services. Ecol. Monogr. 810, 169–193. doi: 10.1890/10-1510.1
Bernhardt-Römermann M., Baeten L., Craven D., De Frenne P., Hédl R., Lenoir J., et al. (2015). Drivers of temporal changes in temperate forest plant diversity vary across spatial scales. Global Change Biol. 21, 3726–3737. doi: 10.1111/gcb.12993
Bertness M. D. (1991). Interspecific interactions among high marsh perennials in a New England salt marsh. Ecology 72, 125–137. doi: 10.2307/1938908
Bertness M. D., Callaway R. (1994). Positive interactions in communities. Trends Ecol. Evol. 9, 191–193. doi: 10.1016/0169-5347(94)90088-4
Bertness M. D., Ellison A. M. (1987). Determinants of pattern in a New England salt marsh plant community. Ecol. Monogr. 57, 129–147. doi: 10.2307/1942621
Bertness M. D., Ewanchuk P. J. (2002). Latitudinal and climate-driven variation in the strength and nature of biological interactions in New England salt marshes. Oecologia 132, 392–401. doi: 10.1007/s00442-002-0972-y
Bonin C. L., Zedler J. B. (2008). Southern California salt marsh dominance relates to plant traits and plasticity. Estuaries Coasts 31, 682–693. doi: 10.1007/s12237-008-9057-4
Bridge S. R. J., Johnson E. A. (2000). Geomorphic principles of terrain organization and vegetation gradients. J. Vege. Sci. 11, 57–70. doi: 10.2307/3236776
Brooker R. W., Maestre F. T., Callaway R. M., Lortie C. L., Cavieres L. A., Kunstler G., et al. (2008). Facilitation in plant communities: the past, the present, and the future. J. Ecol. 96, 18–34. doi: 10.1111/j.1365-2745.2007.01295.x
Buffington K. J., Goodman A. C., Freeman C. M., Thorne K. M. (2020). Testing the interactive effects of flooding and salinity on tidal marsh plant productivity. Aquat. Bot. 164, 103231. doi: 10.1016/j.aquabot.2020.103231
Buffington K. J., Janousek C. N., Dugger B. D., Callaway J. C., Sloane E., SChile-Beers L., et al. (2021). Incorporation of uncertainty to improve projections of tidal wetland elevation and carbon accumulation with sea-level rise. PloS One 16, e0256707. doi: 10.1371/journal.pone.0256707
Busch D. E., Loftus W. F., Bass O. L. (1998). Long-term hydrologic effects on marsh plant community structure in the southern Everglades. Wetlands 18, 230–241. doi: 10.1007/BF03161658
California Natural Diversity Database (CNDDB). (2023). Special Animals List (Sacramento, CA: California Department of Fish and Wildlife).
Chaneton E. J., Facelli J. M. (1991). Disturbance effects on plant community diversity: spatial scales and dominance hierarchies. Vegetatio 93, 143–155. doi: 10.1007/BF00033208
Clarke P. J., Lawes M. J., Midgley J. J. (2010). Resprouting as a key functional trait in woody plants—challenges to developing new organizing principles. New Phytol. 188, 651–654. doi: 10.1111/j.1469-8137.2010.03508.x
Cloern J. E., Jassby A. D. (2012). Drivers of change in estuarine-coastal ecosystems: Discoveries from four decades of study in San Francisco Bay. Rev. Geophys. 50, RG4001. doi: 10.1029/2012RG000397
Cloern J. E., Knowles N., Brown L. R., Cayan D., Dettinger M. D., Morgan T. L., et al. (2011). Projected evolution of California’s San Francisco Bay-Delta-River system in a century of climate change. PloS One 6, e24465. doi: 10.1371/journal.pone.0024465
Costa C. S., Marangoni J. C., Azevedo A. M. (2003). Plant zonation in irregularly flooded salt marshes: relative importance of stress tolerance and biological interactions. J. Ecol. 91, 951–965. doi: 10.1046/j.1365-2745.2003.00821.x
Courtwright J., Findlay S. E. (2011). Effects of microtopography on hydrology, physicochemistry, and vegetation in a tidal swamp of the Hudson River. Wetlands 31, 239–249. doi: 10.1007/s13157-011-0156-9
Crain C. M., Silliman B. R., Bertness S. L., Bertness M. D. (2004). Physical and biotic drivers of plant distribution across estuarine salinity gradients. Ecology 85, 2539–2549. doi: 10.1890/03-0745
Daniëls F. J., de Molenaar J. G., Chytrý M., Tichý L. (2011). Vegetation change in Southeast Greenland? Tasiilaq revisited after 40 years. Appl. Vege. Sci. 14, 230–241. doi: 10.1111/j.1654-109X.2010.01107.x
De Leeuw J., Van den Dool A., De Munck W., Nieuwenhuize J., Beeftink W. G. (1991). Factors influencing the soil salinity regime along an intertidal gradient. Estuarine Coast. Shelf Sci. 32, 87–97. doi: 10.1016/0272-7714(91)90030-F
Donnelly J. P., Bertness M. D. (2001). Rapid shoreward encroachment of salt marsh cordgrass in response to accelerated sea-level rise. Proc. Natl. Acad. Sci. 98, 14218–14223. doi: 10.1073/pnas.251209298
Eleuterius L. N., Eleuterius C. K. (1979). Tide levels and salt marsh zonation. Bull. Mar. Sci. 29, 394–400.
Elmore A. J., Mustard J. F., Manning S. J. (2003). Regional patterns of plant community response to changes in water: Owens Valley, California. Ecol. Appl. 13, 443–460. doi: 10.1890/1051-0761(2003)013[0443:RPOPCR]2.0.CO;2
Elschot K., Vermeulen A., Vandenbruwaene W., Bakker J. P., Bouma T. J., Stahl J., et al. (2017). Top-down vs. bottom-up control on vegetation composition in a tidal marsh depends on scale. PloS One 12, e0169960. doi: 10.1371/journal.pone.0169960
Engels J. G., Jensen K. (2009). Patterns of wetland plant diversity along estuarine stress gradients of the Elbe (Germany) and Connecticut (USA) rivers. Plant Ecol. Divers. 2, 301–311. doi: 10.1080/17550870903508947
Engels J. G., Rink F., Jensen K. (2011). Stress tolerance and biotic interactions determine plant zonation patterns in estuarine marshes during seedling emergence and early establishment. J. Ecol. 99, 277–287. doi: 10.1111/j.1365-2745.2010.01745.x
Ewanchuk P. J., Bertness M. D. (2004). Structure and organization of a northern New England salt marsh plant community. J. Ecol. 92, 72–85. doi: 10.1111/j.1365-2745.2004.00838.x
Gallego-Tévar B., Grewell B. J., Futrell C. J., Drenovsky R. E., Castillo J. M. (2020). Interactive effects of salinity and inundation on native Spartina foliosa, invasive S. densiflora and their hybrid from San Francisco Estuary, California. Ann. Bot. 125, 377–389. doi: 10.1093/aob/mcz170
Garner K. L., Chang M. Y., Fulda M. T., Berlin J. A., Freed R. E., Soo-Hoo M. M., et al. (2015). Impacts of sea level rise and climate change on coastal plant species in the central California coast. PeerJ 3, e958. doi: 10.7717/peerj.958
Gedan K. B., Silliman B. R., Bertness M. D. (2009). Centuries of human-driven change in salt marsh ecosystems. Annu. Rev. Mar. Sci. 1, 117–141. doi: 10.1146/annurev.marine.010908.163930
Gilby B. L., Weinstein M. P., Baker R., Cebrian J., Alford S. B., Chelsky A., et al. (2021). Human actions alter tidal marsh seascapes and the provision of ecosystem services. Estuaries Coasts 44, 1628–1636. doi: 10.1007/s12237-020-00830-0
Goman M., Malamud-Roam F., Ingram B. L. (2008). Holocene environmental history and evolution of a tidal salt marsh in San Francisco Bay, California. J. Coast. Res. 24, 1126–1137. doi: 10.2112/08A-0005.1
Graham R. L., Turner M. G., Dale V. H. (1990). How increasing CO 2 and climate change affect forests. BioScience 40, 575–587. doi: 10.2307/1311298
Hall E. A., Molino G. D., Messerschmidt T. C., Kirwan M. L. (2022). Hidden levees: Small-scale flood defense on rural coasts. Anthropocene 40, 100350. doi: 10.1016/j.ancene.2022.100350
Hallett L., Avolio M., Carroll I., Jones S., MacDonald A., Flynn D., et al. (2020). Codyn: community dynamics metrics. R package version 2.0.5. doi: 10.5063/F1N877Z6
Handa I. T., Harmsen R., Jefferies R. L. (2002). Patterns of vegetation change and the recovery potential of degraded areas in a coastal marsh system of the Hudson Bay lowlands. J. Ecol. 90, 86–99. doi: 10.1046/j.0022-0477.2001.00635.x
Hartig E. K., Gornitz V., Kolker A., Mushacke F., Fallon D. (2002). Anthropogenic and climate-change impacts on salt marshes of Jamaica Bay, New York City. Wetlands 22, 71–89. doi: 10.1672/0277-5212(2002)022[0071:AACCIO]2.0.CO;2
He Y., Li X., Craft C., Ma Z., Sun Y. (2011). Relationships between vegetation zonation and environmental factors in newly formed tidal marshes of the Yangtze River estuary. Wetlands Ecol. Manage. 19, 341–349. doi: 10.1007/s11273-011-9220-8
IPCC (2022). Climate Change 2022: Impacts, Adaptation and Vulnerability. Contribution of Working Group II to the Sixth Assessment Report of the Intergovernmental Panel on Climate Change. Eds. Pörtner H.-O., Roberts D. C., Tignor M., Poloczanska E. S., Mintenbeck K., Alegría A., Craig M., Langsdorf S., Löschke S., Möller V., Okem A., Rama B. (Cambridge, UK and New York, NY, USA: Cambridge University Press), 3056. doi: 10.1017/9781009325844
Janousek C. N., Buffington K. J., Thorne K. M., Guntenspergen G. R., Takekawa J. Y., Dugger B. D. (2016). Potential effects of sea-level rise on plant productivity: species-specific responses in northeast Pacific tidal marshes. Mar. Ecol. Prog. Ser. 548, 111–125. doi: 10.3354/meps11683
Janousek C. N., Folger C. L. (2014). Variation in tidal wetland plant diversity and composition within and among coastal estuaries: assessing the relative importance of environmental gradients. J. Vege. Sci. 25, 534–545. doi: 10.1111/jvs.12107
Janousek C. N., Mayo C. (2013). Plant responses to increased inundation and salt exposure: interactive effects on tidal marsh productivity. Plant Ecol. 214, 917–928. doi: 10.1007/s11258-013-0218-6
Janousek C. N., Thorne K. M., Takekawa J. Y. (2019). Vertical zonation and niche breadth of tidal marsh plants along the northeast Pacific coast. Estuaries Coasts 42, 85–98. doi: 10.1007/s12237-018-0420-9
Jones S. F., Janousek C. N., Casazza M. L., Takekawa J. Y., Thorne K. M. (2021). Seasonal impoundment alters patterns of tidal wetland plant diversity across spatial scales. Ecosphere 12, e03366. doi: 10.1002/ecs2.3366
Jones S. K., Ripplinger J., Collins S. L. (2017). Species reordering, not changes in richness, drives long-term dynamics in grassland communities. Ecol. Lett. 20, 1556–1565. doi: 10.1111/ele.12864
Kearney M. S., Riter J. A., Turner R. E. (2011). Freshwater river diversions for marsh restoration in Louisiana: Twenty-six years of changing vegetative cover and marsh area. Geophys. Res. Lett. 38 (16), L16405. doi: 10.1029/2011GL047847
Kirwan M. L., Guntenspergen G. R. (2015). Response of plant productivity to experimental flooding in a stable and a submerging marsh. Ecosystems 18, 903–913. doi: 10.1007/s10021-015-9870-0
Knox S. H., Windham-Myers L., Anderson F., Sturtevant C., Bergamaschi B. (2018). Direct and indirect effects of tides on ecosystem-scale CO2 exchange in a brackish tidal marsh in Northern California. J. Geophys. Res.: Biogeosci. 123, 787–806. doi: 10.1002/2017JG004048
Korell L., Auge H., Chase J. M., Harpole W. S., Knight T. M. (2021). Responses of plant diversity to precipitation change are strongest at local spatial scales and in drylands. Nat. Commun. 12, 2489. doi: 10.1038/s41467-021-22766-0
Kutcher T. E., Raposa K. B., Roman C. T. (2022). A rapid method to assess salt marsh condition and guide management decisions. Ecol. Indic. 138, 108841. doi: 10.1016/j.ecolind.2022.108841
Li F., Angelini C., Byers J. E., Craft C., Pennings S. C. (2022). Responses of a tidal freshwater marsh plant community to chronic and pulsed saline intrusion. J. Ecol. 110, 1508–1524. doi: 10.1111/1365-2745.13885
Li F., Pennings S. C. (2019). Response and recovery of low-salinity marsh plant communities to presses and pulses of elevated salinity. Estuaries Coasts 42, 708–718. doi: 10.1007/s12237-018-00490-1
Li L., Ustin S. L., Lay M. (2005). Application of multiple endmember spectral mixture analysis (MESMA) to AVIRIS imagery for coastal salt marsh mapping: a case study in China Camp, CA, USA. Int. J. Remote Sens. 26, 5193–5207. doi: 10.1080/01431160500218911
Liancourt P., Callaway R. M., Michalet R. (2005). Stress tolerance and competitive-response ability determine the outcome of biotic interactions. Ecology 86, 1611–1618. doi: 10.1890/04-1398
Lortie C. J., Callaway R. M. (2006). Re-analysis of meta-analysis: support for the stress-gradient hypothesis. J. Ecol. 94, 7–16. doi: 10.1111/j.1365-2745.2005.01066.x
Maestre F. T., Callaway R. M., Valladares F., Lortie C. J. (2009). Refining the stress-gradient hypothesis for competition and facilitation in plant communities. J. Ecol. 97, 199–205. doi: 10.1111/j.1365-2745.2008.01476.x
Maestre F. T., Valladares F., Reynolds J. F. (2005). Is the change of plant–plant interactions with abiotic stress predictable? A meta-anal. Field results arid environ. J. Ecol. 93, 748–757. doi: 10.1111/j.1365-2745.2005.01017.x
Maestre F. T., Valladares F., Reynolds J. F. (2006). The stress-gradient hypothesis does not fit all relationships between plant–plant interactions and abiotic stress: further insights from arid environments. J. Ecol. 94, 17–22. doi: 10.1111/j.1365-2745.2005.01089.x
Malavasi M., Santoro R., Cutini M., Acosta A. T. R., Carranza M. L. (2016). The impact of human pressure on landscape patterns and plant species richness in Mediterranean coastal dunes. Plant Biosystems-an Int. J. Dealing All Aspects Plant Biol. 150, 73–82. doi: 10.1080/11263504.2014.913730
Maliniemi T., Kapfer J., Saccone P., Skog A., Virtanen R. (2018). Long-term vegetation changes of treeless heath communities in northern Fennoscandia: Links to climate change trends and reindeer grazing. J. Vege. Sci. 29, 469–479. doi: 10.1111/jvs.12630
Marcus L. (2000). Restoring tidal wetlands at Sonoma Baylands, San Francisco Bay, California. Ecol. Eng. 15, 373–383. doi: 10.1016/S0925-8574(00)00087-2
Marquer L., Gaillard M. J., Sugita S., Poska A., Trondman A. K., Mazier F., et al. (2017). Quantifying the effects of land use and climate on Holocene vegetation in Europe. Quaternary Sci. Rev. 171, 20–37. doi: 10.1016/j.quascirev.2017.07.001
Menge B. A., Sutherland J. P. (1987). Community regulation: variation in disturbance, competition, and predation in relation to environmental stress and recruitment. Am. Nat. 130, 730–757. doi: 10.1086/284741
Michelsen O., Syverhuset A. O., Pedersen B., Holten J. I. (2011). The impact of climate change on recent vegetation changes on Dovrefjell, Norway. Diversity 3, 91–111. doi: 10.3390/d3010091
Moffett K. B., Gorelick S. M. (2016). Alternative stable states of tidal marsh vegetation patterns and channel complexity. Ecohydrology 9, 1639–1662. doi: 10.1002/eco.1755
Mokany K., McCarthy J. K., Falster D. S., Gallagher R. V., Harwood T. D., Kooyman R., et al. (2022). Patterns and drivers of plant diversity across Australia. Ecography 2022, e06426. doi: 10.1111/ecog.06426
Mora J. W., Burdick D. M. (2013). Effects of man-made berms upon plant communities in New England salt marshes. Wetlands Ecol. Manage. 21, 131–145. doi: 10.1007/s11273-013-9285-7
Morris J. T., Sundareshwar P. V., Nietch P. T., Kjerfve B., Cahoon D. R. (2002). Responses of coastal wetlands to rising sea levels. Ecology 83, 2869–2877. doi: 10.1890/0012-9658(2002)083[2869:ROCWTR]2.0.CO;2
Morzaria-Luna H. N., Zedler J. B. (2014). Competitive interactions between two salt marsh halophytes across stress gradients. Wetlands 34, 31–42. doi: 10.1007/s13157-013-0479-9
Muench A., Elsey-Quirk T. (2019). Competitive reversal between plant species is driven by species-specific tolerance to flooding stress and nutrient acquisition during early marsh succession. J. Appl. Ecol. 56, 2236–2247. doi: 10.1111/1365-2664.13458
Neubauer S. C. (2013). Ecosystem responses of a tidal freshwater marsh experiencing saltwater intrusion and altered hydrology. Estuaries Coasts 36, 491–507. doi: 10.1007/s12237-011-9455-x
Neumann F. H., Scott L., Bousman C. B., Van As L. (2010). A Holocene sequence of vegetation change at Lake Eteza, coastal KwaZulu-natal, South Africa. Rev. Palaeobotany Palynol. 162, 39–53. doi: 10.1016/j.revpalbo.2010.05.001
Nichols F. H., Cloern J. E., Luoma S. N., Peterson D. H. (1986). The modification of an estuary. Science 231, 567–573. doi: 10.1126/science.231.4738.567
Niku J., Brooks W., Herliansyah R., Hui F. K. C., Korhonen P., Taskinen S., et al. (2023). gllvm: Generalized linear latent variable models. R package version 1.4.3.
Niku J., Hui F. K. C., Taskinen S., Warton D. I. (2019). gllvm - Fast analysis of multivariate abundance data with generalized linear latent variable models in R. Methods Ecol. Evol. 10, 2173–2182. doi: 10.1111/2041-210X.13303
Oakes L. E., Hennon P. E., O’Hara K. L., Dirzo R. (2014). Long-term vegetation changes in a temperate forest impacted by climate change. Ecosphere 5, 1–28. doi: 10.1890/ES14-00225.1
Odum W. E. (1988). Comparative ecology of tidal freshwater and salt marshes. Annu. Rev. Ecol. Evol. Systs. 19 (1), 147–176.
Oosterlee L., Cox T. J., Vandenbruwaene W., Maris T., Temmerman S., Meire P. (2018). Tidal marsh restoration design affects feedbacks between inundation and elevation change. Estuaries coasts 41, 613–625. doi: 10.1007/s12237-017-0314-2
Orson R. A., Panageotou W., Leatherman S. P. (1985). Response of tidal salt marshes of the US Atlantic and Gulf coasts to rising sea levels. J. Coast. Res. 1, 29–37.
Parker B., Milbert D., Hess K., Gill S. (2003). National VDatum–The implementation of a national vertical datum transformation database. Silver Spring: National Oceanic and Atmospheric Administration, National Ocean Service. 9.
Peng D., Chen L., Pennings S. C., Zhang Y. (2018). Using a marsh organ to predict future plant communities in a Chinese estuary invaded by an exotic grass and mangrove. Limnol. Oceanogr. 63, 2595–2605. doi: 10.1002/lno.10962
Pennings S. C., Grant M. B., Bertness M. D. (2005). Plant zonation in low-latitude salt marshes: disentangling the roles of flooding, salinity and competition. J. Ecol. 93, 159–167. doi: 10.1111/j.1365-2745.2004.00959.x
Pontee N. (2013). Defining coastal squeeze: A discussion. Ocean Coast. Manage. 84, 204–207. doi: 10.1016/j.ocecoaman.2013.07.010
Rand T. A. (2002). Variation in insect herbivory across a salt marsh tidal gradient influences plant survival and distribution. Oecologia 132, 549–558. doi: 10.1007/s00442-002-0989-2
R Core Team (2022). R: A language and environment for statistical computing (Vienna, Australia: R Foundation for Statistical Computing). Available at: https://www.R-project.org/.
Reed D. J., Cahoon D. R. (1992). The relationship between marsh surface topography, hydroperiod, and growth of Spartina alterniflora in a deteriorating Louisiana salt marsh. J. Coast. Res. 8, 77–87.
Rogers K., Saintilan N., Woodroffe C. D. (2014). Surface elevation change and vegetation distribution dynamics in a subtropical coastal wetland: implications for coastal wetland response to climate change. Estuarine Coast. Shelf Sci. 149, 46–56. doi: 10.1016/j.ecss.2014.07.009
Rogers K., Woodroffe C. D. (2015). “Tidal flats and salt marshes,” in Coastal environments and global change. ed. Masselink G., Gehrels R. (West Sussex: John Wiley and Sons), 227–250. doi: 10.1002/9781119117261.ch10
Ross M. S., Meeder J. F., Sah J. P., Ruiz P. L., Telesnicki G. J. (2000). The southeast saline Everglades revisited: 50 years of coastal vegetation change. J. Vege. Sci. 11, 101–112. doi: 10.2307/3236781
Rosso P. H., Ustin S. L., Hastings A. (2005). Mapping marshland vegetation of San Francisco Bay, California, using hyperspectral data. Int. J. Remote Sens. 26, 5169–5191. doi: 10.1080/01431160500218770
Russell S. J., Windham-Myers L., Stuart-Haëntjens E. J., Bergamaschi B. A., Anderson F., Oikawa P., et al. (2023). Increased salinity decreases annual gross primary productivity at a Northern California brackish tidal marsh. Environ. Res. Lett. 18, 034045. doi: 10.1088/1748-9326/acbbdf
Ryan A. B., Boyer K. E. (2012). Nitrogen further promotes a dominant salt marsh plant in an increasingly saline environment. J. Plant Ecol. 5, 429–441. doi: 10.1093/jpe/rts001
Sanderson E. W., Foin T. C., Ustin S. L. (2001). A simple empirical model of salt marsh plant spatial distributions with respect to a tidal channel network. Ecol. Model. 139, 293–307. doi: 10.1016/S0304-3800(01)00253-8
Schepers L., Kirwan M., Guntenspergen G., Temmerman S. (2017). Spatio-temporal development of vegetation die-off in a submerging coastal marsh. Limnol. Oceanogr. 62, 137–150. doi: 10.1002/lno.10381
Schile L. M., Callaway J. C., Morris J. T., Stralberg D., Parker V. T., Kelly M. (2014). Modeling tidal marsh distribution with sea-level rise: evaluating the role of vegetation, sediment, and upland habitat in marsh resiliency. PloS One 9, e88760. doi: 10.1371/journal.pone.0088760
Schile L. M., Callaway J. C., Suding K. N., Kelly N. M. (2017). Can community structure track sea-level rise? Stress and competitive controls in tidal wetlands. Ecol. Evol. 7, 1276–1285. doi: 10.1002/ece3.2758
Schwarz C., Gourgue O., Van Belzen J., Zhu Z., Bouma T. J., Van De Koppel J., et al. (2018). Self-organization of a biogeomorphic landscape controlled by plant life-history traits. Nat. Geosci. 11, 672–677. doi: 10.1038/s41561-018-0180-y
Sharpe P. J., Baldwin A. H. (2012). Tidal marsh plant community response to sea-level rise: a mesocosm study. Aquat. Bot. 101, 34–40. doi: 10.1016/j.aquabot.2012.03.015
Shipley B. (2010). From plant traits to vegetation structure: chance and selection in the assembly of ecological communities (Cambridge: Cambridge University Press).
Silvestri S., Defina A., Marani M. (2005). Tidal regime, salinity and salt marsh plant zonation. Estuarine Coast. shelf Sci. 62, 119–130. doi: 10.1016/j.ecss.2004.08.010
Skrondal A., Rabe-Hesketh S. (2004). Generalized latent variable modeling: Multilevel, longitudinal, and structural equation models (Boca Raton: CRC Press).
Snedden G. A., Cretini K., Patton B. (2015). Inundation and salinity impacts to above- and belowground productivity in Spartina patens and Spartina alterniflora in the Mississippi River deltaic plain:implications for using river diversions as restoration tools. Ecol. Eng. 81, 133–139. doi: 10.1016/j.ecoleng.2015.04.035
Song Y., Jin L., Wang H. (2018). Vegetation changes along the Qinghai-Tibet Plateau engineering corridor since 2000 induced by climate change and human activities. Remote Sens. 10, 95. doi: 10.3390/rs10010095
Sundaram B., Hiremath A. J. (2012). Lantana camara invasion in a heterogeneous landscape: patterns of spread and correlation with changes in native vegetation. Biol. Invasions 14, 1127–1141. doi: 10.1007/s10530-011-0144-2
Swanson K. M., Drexler J. Z., Schoellhamer D. H., Thorne K. M., Casazza M. L., Overton C. T., et al. (2014). Wetland accretion rate model of ecosystem resilience (WARMER) and its application to habitat sustainability for endangered species in the San Francisco estuary. Estuaries Coasts 37, 476–492. doi: 10.1007/s12237-013-9694-0
Sweet W. V., Hamlington B. D., Kopp R. E., Weaver C. P., Barnard P. L., Bekaert D., et al. (2022). Global and regional sea level rise scenarios for the United States: Updated mean projections and extreme water level probabilities along U.S. coastlines. NOAA Technical Report NOS 01 (Silver Spring: National Oceanic and Atmospheric Administration, National Ocean Service), 111 pp. https://oceanservice.noaa.gov/hazards/sealevelrise/noaa-nostechrpt01-global-regional-SLR-scenarios-US.pdf.
Takekawa J. Y., Thorne K. M., Buffington K. Y., Spragens K. A., Swanson K. M., Drexler J. Z., et al. (2013b). Final report for sea-level rise response modeling for San Francisco Bay estuary tidal marshes U.S. Geological Survey Open File Report 2013-1081, 161 pp.
Takekawa J. Y., Lu C. T., Pratt R. T. (2001). Avian communities in baylands and artificial salt evaporation ponds of the San Francisco Bay estuary. Hydrobiologia 466, 317–328. doi: 10.1023/A:1014546524957
Takekawa J. Y., Thorne K. M., Buffington K. J., Freeman C. M., Powelson K. W., Block G. (2013a). Assessing marsh response from sea-level rise applying local site conditions: Humboldt Bay National Wildlife Refuge. Unpubl. Data Summary Report (Vallejo, CA: USGS Western Ecological Research Center). 44pp + Appendices.
Takekawa J. Y., Woo I., Spautz H., Nur N., Grenier J. L., Malamud-Roam K., et al. (2006). Environmental threats to tidal-marsh vertebrates of the San Francisco Bay estuary. Stud. Avian Biol. 32, 176.
Tasser E., Tappeiner U. (2002). Impact of land use changes on mountain vegetation. Appl. vegetation Sci. 5, 173–184. doi: 10.1111/j.1654-109X.2002.tb00547.x
Taylor A. H., Poulos H. M., Kluber J., Issacs R., Pawlikowski N., Barton A. M. (2021). Controls on spatial patterns of wildfire severity and early post-fire vegetation development in an Arizona Sky Island, USA. Landscape Ecol. 36, 2637–2656. doi: 10.1007/s10980-021-01260-4
Thorne K. M., Backe K. E., Freeman C. M., Buffington K. J., Forstner T. M., Goodman A. C. (2019). Don Edwards National Wildlife Refuge Vegetation, Elevation, Inundation Inventory to Inform Sea-level Rise Vulnerability. Unpubl. Data Summary Report (Davis, CA: USGS Western Ecological Research Center). 112pp.
Thorne K., MacDonald G., Guntenspergen G., Ambrose R., Buffington K., Dugger B., et al. (2018). U.S. Pacific coastal wetland resilience and vulnerability to sea-level rise. Sci. Adv. 4, eaao3270. doi: 10.1126/sciadv.aao3270
Trowbridge P. R., Davis J. A., Mumley T., Taberski K., Feger N., Valiela L., et al. (2016). The regional monitoring program for water quality in San Francisco Bay, California, USA: science in support of managing water quality. Regional Stud. Mar. Sci. 4, 21–33. doi: 10.1016/j.rsma.2015.10.002
United States Census Bureau (2021). American Community Survey 5-year Data, (2009-2021) (United States government). Available at: https://data.census.gov/cedsci/.
USDA, NRCS (2023). The PLANTS Database (Greensboro, NC USA: National Plant Data Team). Available at: http://plants.usda.gov.
Van de Koppel J., Bouma T. J., Herman P. M. (2012). The influence of local-and landscape-scale processes on spatial self-organization in estuarine ecosystems. J. Exp. Biol. 215, 962–967. doi: 10.1242/jeb.060467
Van Der Heijden M. G., Bardgett R. D., Van Straalen N. M. (2008). The unseen majority: soil microbes as drivers of plant diversity and productivity in terrestrial ecosystems. Ecol. Lett. 11, 296–310. doi: 10.1111/j.1461-0248.2007.01139.x
Van der Plas F., Schröder-Georgi T., Weigelt A., Barry K., Meyer S., Alzate A., et al. (2020). Plant traits alone are poor predictors of ecosystem properties and long-term ecosystem functioning. Nat. Ecol. Evol. 4, 1602–1611. doi: 10.1038/s41559-020-01316-9
Wasson K., Woolfolk A., Fresquez C. (2013). Ecotones as indicators of changing environmental conditions: rapid migration of salt marsh–upland boundaries. Estuaries Coasts 36, 654–664. doi: 10.1007/s12237-013-9601-8
Watson E. B., Byrne R. (2009). Abundance and diversity of tidal marsh plants along the salinity gradient of the San Francisco Estuary: implications for global change ecology. Plant Ecol. 205, 113–128. doi: 10.1007/s11258-009-9602-7
White J. R., Couvillion B., Day J. W. (2023). Coastal wetland area change for two freshwater diversions in the Mississippi River Delta. Ecol. Eng. 186, 106819. doi: 10.1016/j.ecoleng.2022.106819
Windham-Myers L., Bergamaschi B., Anderson F., Knox S., Miller R., Fujii R. (2018). Potential for negative emissions of greenhouse gases (CO2, CH4 and N2O) through coastal peatland re-establishment: Novel insights from high frequency flux data at meter and kilometer scales. Environ. Res. Lett. 13, 045005. doi: 10.1088/1748-9326/aaae74
Woo I., Takekawa J. Y. (2012). Will inundation and salinity levels associated with projected sea level rise reduce the survival, growth, and reproductive capacity of Sarcocornia pacifica (pickleweed)? Aquat. Bot. 102, 8–14. doi: 10.1016/j.aquabot.2012.03.014
Keywords: plant diversity, tidal marsh, environmental gradient, GLLVM, environment–trait association, abundance modeling, rank abundance curve
Citation: Rankin LL, Jones SF, Janousek CN, Buffington KJ, Takekawa JY and Thorne KM (2023) Stress gradients structure spatial variability in coastal tidal marsh plant composition and diversity in a major Pacific coast estuary. Front. Ecol. Evol. 11:1215964. doi: 10.3389/fevo.2023.1215964
Received: 02 May 2023; Accepted: 20 October 2023;
Published: 21 November 2023.
Edited by:
Thomas George Bornman, South African Environmental Observation Network (SAEON), South AfricaReviewed by:
Peter M.J. Herman, Delft University of Technology, NetherlandsDavid Burdick, University of New Hampshire, United States
Copyright © 2023 Rankin, Jones, Janousek, Buffington, Takekawa and Thorne. This is an open-access article distributed under the terms of the Creative Commons Attribution License (CC BY). The use, distribution or reproduction in other forums is permitted, provided the original author(s) and the copyright owner(s) are credited and that the original publication in this journal is cited, in accordance with accepted academic practice. No use, distribution or reproduction is permitted which does not comply with these terms.
*Correspondence: Lyndsay L. Rankin, bHJhbmtpbkB1c2dzLmdvdg==