- 1Washington State Department of Ecology, Water Quality Program, Bellingham, WA, United States
- 2Western Washington University, College of the Environment, Bellingham, WA, United States
- 3Olympic College, Department of Biology, Bremerton, WA, United States
Introduction: Until recently, much of the Elwha River was inaccessible to anadromous fish species due to the Elwha and Glines Canyon dams. Dam deconstruction resulted in approximately 325 ha of formerly inundated lake beds devoid of natural riparian corridors or adjacent forests. Efforts to restore the vegetation have had varying success. Areas where fine sediments settled along the valley walls quickly regenerated, while coarse terraces were slower to revegetate from plantings and directed seeding. One seeded species, riverbank lupine (Lupinus rivularis), quickly established on the coarse-textured terraces. Riverbank lupine is a pioneering species that assimilates nitrogen (N) through N-fixing bacteria; thus, it enriches the soil with bioavailable N and organic matter upon decomposition.
Methods: The goal of this study was to investigate lupine’s influence on conifer establishment in the coarse sediments of the former Lake Mills basin along the Elwha River. Conifers planted 3 years prior to the study in plots with different levels of lupine cover (sparse, medium, or dense) were measured for growth, foliar total nitrogen, and ectomycorrhizal (ECM) root colonization. Soil N, organic matter (OM), and surrounding plant community composition were also evaluated at this time.
Results: After 3 years, conifers did not statistically differ in height or basal diameter among differing levels of lupine cover. However, conifers in the medium- and dense-cover plots had significantly greater foliar N concentrations (β = 0.25, SE = 0.08, p < 0.001). The roots of conifers in the dense- and medium-cover plots had significantly less ECM colonization than those in the sparse-cover plots (β = 0.14, SE = 0.03, p = 0.03). No differences existed regarding soil total N or OM. Plant community composition differed among lupine cover classes (permutational multivariate analysis of variance (PERMANOVA), (F(2,21) = 2.02, p = 0.01). Higher lupine cover resulted in lower species richness (β = 25.9, SE = 0.61, p = 0.04).
Discussion: Based on our findings, the inclusion of native lupine in coarse, N-limiting soils contributes to the N pools without reducing the growth of planted conifers, which may accelerate forest succession leading to closed canopies much faster than passive recovery.
Introduction
The environmental impacts of dams on forest-river processes have been well documented (Hall et al., 2011; Reidy Liermann et al., 2012) and include alterations to aquatic animal populations, riparian vegetation, hydrology, and river deltas (Li et al., 1987; Jansson et al., 2000; Sharma, 2001; Perry et al., 2016). Damming rivers in the Pacific Northwest (PNW) obstructs many historic anadromous fish migration routes, leading to major species declines (Duda et al., 2008). Riparian and upland forests are inundated through the creation of reservoirs that impede downstream flow and sediment exchange and cause deeply channelized riverbeds that become disconnected from floodplains (Shafroth, 1999; Rood and Mahoney, 2000). Further, dams decrease the delivery of large woody debris from forests to riverine systems, which negatively affects floodplain, delta, and instream habitat complexity, and the nearshore environment (Andersson et al., 2000; Gregory et al., 2002). Inhibiting the passage of anadromous fish affects the overall health of riparian ecosystems by limiting the transfer of marine-derived nutrients to adjacent forests (Helfield and Naiman, 2001; Drake et al., 2002).
Until recently, much of the Elwha River Basin was inaccessible to sea-run fish due to the Elwha and Glines Canyon dams. These dams blocked access to 90% of the spawning habitat, impeded sediment transfer to the nearshore, and degraded stream and riparian habitat (Pess et al., 2008; Shaffer et al., 2008; Duda et al., 2011). Despite the environmental impact, the two dams operated with little regulation for over 50 years until the Federal Power Act (late 1960s–1970s) required the owner to license the dams with the Federal Energy Regulatory Commission (Sadin and Vogel, 2011). This required maintenance for structural integrity and construction of fish passageways (Service, 2011). The Elwha River Ecosystem and Fisheries Restoration Act was enacted in 1992, requiring dam removal to restore riverine habitats, native salmon runs, and other ecosystem processes (DOI (U.S. Department of Interior) et al., 1996; Winter and Crain, 2008). The costs/benefit analysis, combined with the potential to restore the Lower Elwha Klallam Tribe’s salmon runs, began to sway public and political opinion in favor of dam removal (Gregory et al., 2002; Duda et al., 2008).
The deconstruction of both dams and lake drawdown was completed by 2014, restoring access to the upper river and marking the largest dam removal project to date in the United States. Given the unprecedented nature of the large-scale dam removal, there was considerable uncertainty about how the biota would respond to the dewatering of the two reservoirs, which were estimated to store ~21,000,000 m3 of sediment prior to removal (Warrick et al., 2015; Major et al., 2017). A major concern was the impact on terrestrial and aquatic habitats of this increased sediment load (Pizzuto, 2002; Stanley and Doyle, 2003). During drawdown, coarse-grained terraces approximately 3.3 to 7 m thick formed along the Lake Mills bed above the Glines Canyon dam (East et al., 2015). These terraces, composed of unconsolidated sand, gravel, and cobble, resulted in novel landforms perched above the hyporheic zone and situated between the riparian and forest corridors along the Elwha River (Bauman and Kardouni, 2018).
Revegetating these landforms, a fundamental goal of the Elwha River and Ecosystem Restoration Project, required the restoration of river-forest processes and native communities while limiting the establishment of non-native species (DOI National Park Service, 2015). Revegetation plans were designed to promote rapid succession to native forests, thus limiting erosion, regulating water temperature, and providing critical habitats (Chenoweth et al., 2011). In the Lake Mills Basin of the Elwha River, 44.0 ha of newly exposed sediments were actively revegetated from 2013 to 2015. This included broadcasting 2,800 kg of native seed and planting 205,000 herbaceous and woody plants representing 64 species (Chenoweth et al., 2022). Conifers such as Douglas fir (Pseudotsuga menziesii Mirb Franco), grand fir (Abies grandis [Douglas ex D. Don] Lindl.), and western white pine (Pinus monticola Douglas ex D. Don) were incorporated into the planting plan and are considered important indicators of forest recovery, as well as iconic species to the PNW forests (Franklin, 1988; Shafroth et al., 2002).
One seeded forb species, native riverbank lupine (Lupinus rivularis Douglas ex Lindl.), was included in the seed mix and thrived in the coarse sediments along the Lake Mills terraces (Chenoweth et al., 2022). As a legume, it assimilates nitrogen (N) through the endosymbiotic association with Rhizobium, a N-fixing bacterium (Staniewski, 1970). Myrold and Huss-Danell (2003) demonstrated that N-fixing species such as lupine can enhance N availability and organic matter in nutrient-limited soils (Halvorson et al., 1991). Riverbank lupine is an annual, biennial, or short-lived perennial that can grow up to 150 cm in height and acts as a pioneer species adapted to sandy or gravelly soils (Darris and Young-Mathews, 2012). The 2-to-3-year life cycle of riverbank lupine starts with a vegetative phase followed by seeding and senescence with subsequent grow-back periods by seeded progeny.
One of the most well-studied examples of lupine colonization was in plant successional studies conducted after the eruption of Mount Saint Helens in the Pacific Northwest region of the United States. Lupinus lepidus Douglas ex Lindl. was the first colonizing herbaceous plant and formed dense to patchy colonies within a decade after the eruption (del Moral et al., 1995). Its presence was shown to have both facilitative and inhibitory effects on invading plant species, where living colonies inhibited ruderal species, while dead lupine patches facilitated recruitment (Morris and Wood, 1989). Elsewhere at Mount Saint Helens, del Moral and Rozzell (2005) demonstrated that L. lepidus inhibited colonization of forbs in mature lupine colonies. However, older colonies demonstrated a subsequent increase in plant richness and diversity. Further, varying levels of lupine allowed different sets of species to be established, suggesting that variation in lupine abundance may lead to different successional trajectories (del Moral and Rozzell, 2005; del Moral, 2007). As lupine densities gradually decrease, it is hypothesized that their legacy will contribute to soil development and ultimately accelerate plant succession (Vitousek et al., 1987; del Moral, 2007). Therefore, developing our understanding of lupine during primary succession may be an important component of forest restoration projects given its adaptability, ability to facilitate the establishment of neighboring plants, and N-fixing capability (Bishop, 2002).
The planting of native tree seedlings with and into herbaceous cover is common in restoration; however, not all N-fixing herbaceous species facilitate the establishment of neighboring trees (Weidlich et al., 2020). Previous studies utilizing various Lupinus species have illustrated positive tree growth response and increased N acquisition (Prietzel et al., 2008; Mauer et al., 2013; Oldřich et al., 2013). Other projects have also shown that neighboring vegetation acts as “nurse plants” that moderate soil temperatures, increase water and nutrient availability, and promote microbial interactions (Bertness and Callaway, 1994; Raffaele and Veblen, 1998; Flores and Jurado, 2003). L. lepidus has also been reported to stimulate microbial activity in primary successional soils at Mount Saint Helens (Halvorson et al., 1991). Ectomycorrhizal fungi are key components of PNW forest soils, forming symbioses with conifers (Kranabetter et al., 2015). ECM fungi perform a variety of functions that contribute to the establishment, survivability, and resilience of planted seedlings (Massicotte et al., 1999; Smith and Read, 2008). Mature soils typically support diverse and abundant fungal communities essential to forest health (Balestrini et al., 2016). However, dam removal and deposition of coarser sediments may not be conducive to the formation of these symbioses, thus limiting tree establishment after dam removal (Cortese and Bunn, 2017). Therefore, lupine’s presence may facilitate soil development to promote ECM colonization of conifer roots, thereby supporting plant establishment and native plant community recovery.
In this study, we explored how varying densities of riverbank lupine affected the performance of planted conifers (Douglas fir, grand fir, and western white pine), ECM root colonization, soil N and organic matter (OM), and neighboring plant communities in coarse substrates on the exposed terraces of Lake Mills along the Elwha River. We hypothesized that greater lupine densities would result in greater conifer growth, foliar N concentrations, and greater ECM taxonomic richness and colonization. We also hypothesized that greater lupine densities would yield greater C:N, OM, and N content in coarse sediment. Finally, we hypothesized that plant species richness would be lower and community composition would differ in areas of greater vs. lesser lupine density. Documenting species interactions that accelerate succession by aiding the growth of conifers and the development of native plant communities and soils may help future projects that will have to manage the revegetation of coarse sediments left behind after dam removal.
Materials and methods
Study area
The field study was located in Olympic National Park (ONP) along the Elwha River at the location of the former Lake Mills reservoir behind the Glines Canyon dam (Figure 1). The dam was removed over the course of 3 years, and work was completed in 2014. Biologists at ONP and Lower Elwha Klallam Tribe implemented the Elwha River and Ecosystem Restoration Project, which had multiple restoration goals: establishing native vegetation to accelerate forest succession, controlling erosion, regulating water temperature, creating habitat, and limiting exotic plant invasion (Chenoweth et al., 2011).
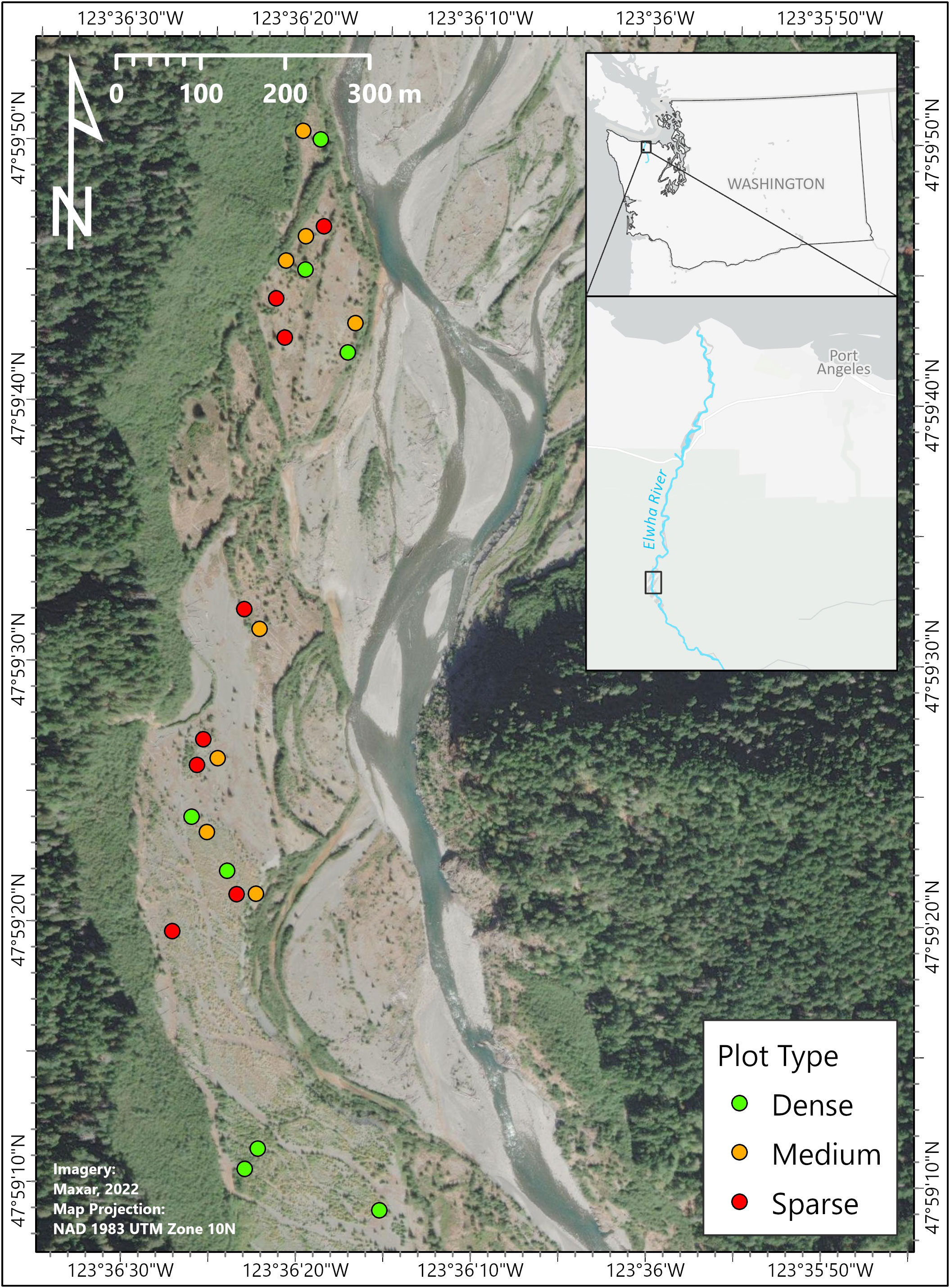
Figure 1 Western terraces formed after the drawdown of Lake Mills along the Elwha River located 22 river km from the mouth. This area was dewatered between 2012 and 2014 via the removal of the Glines Canyon dam. The multiple southwestern terraces were comprised of coarse sediment, sand, and cobble and were actively planted and seeded between 2013 and 2014 with study plots established in 2017. Twenty-four study plots represent three distinct densities of lupine: green = dense (76% lupine cover), orange = medium (58% lupine cover), and red = sparse (15% lupine cover). Map provided by Alex Harnick.
The study area occupied multiple southwestern terraces approximately 1.6 km from the former dam (Figure 1). Conifers were grown in the greenhouse for 3 to 4 years, planted on the restoration site, and grew for approximately 3 years prior to our study in 2017. Areas where trees and shrubs were planted received 430 seeds per square meter from the ONP revegetation crew between 2013 and 2014. Seed mixtures contained a combination of 10 locally harvested and produced grass and forb species at the following quantities: common yarrow (Achillea millifolium; 44.2 kg), spiked bentgrass (Agrostis exarata; 4.0 kg), Suksdorf’s sagewort (Artemisia suksdorfii; 69.2 kg), Pacific brome (Bromus pacificus; 933.5 kg), sedges (Carex pachystachya and Carex deweyana; 202.4 kg combined), slender hairgrass (Deschampsia elongata; 75.1 kg), blue wild-rye (Elymus glaucus; 1,367.0 kg), Oregon sunshine (Eriophyllum lanatum; 70.1 kg), and riverbank lupine (L. rivularis; 59.5 kg; Chenoweth et al., 2022). Lupine was one of the few species that grew vigorously and spread to dominate many restoration areas. However, species were not seeded uniformly, and lupine was established at varying densities along the terraces (J. Chenoweth, per. comm).
Twenty-four plots (8 m × 8 m) were established to capture the range of lupine cover using the transitional zones where lupine is abundant in high to low densities along the western terraces of the Lake Mills reservoir bed (Figure 1). Plots were assigned by visual assessment based on relative cover and assigned one of three density classes: sparse, medium, or dense (eight plots each). Cover-class estimates of lupine abundance confirmed significant differences in the mean cover of lupine among density classes in 8-m2 plots: dense = 76% ( ± 0.04%, medium = 58% ( ± 0.04%), and sparse (15% ± 0.02%) (F(2,21) = 72.94, p< 0.001).
Each plot contained approximately 8–10 living conifers consisting of Douglas fir (P. menziesii), grand fir (A. grandis), and western white pine (P. monticola). After each plot was established, all living conifers were inventoried and numbered. From among the trees in each plot, three were randomly selected, yielding a total of 36 Douglas fir, 21 grand fir, and 15 western white pine. Each tree was assessed for its growth (height and basal diameter), foliar N, and ECM colonization. Soil N and OM and neighboring vegetation were also assessed (described below).
Conifer height, diameter, foliar N, and ECM colonization
Conifer height and basal diameter were measured in October 2018. Height (nearest cm) was measured from the ground surface to the top of the apical bud. Basal diameter (nearest mm) was measured approximately 2 cm above the soil surface using a mechanical caliper. At the beginning of the dormant season (October 2018) when foliar N concentrations had stabilized (Harrison et al., 2011), a small foliar sample (3–5 cm length) of the current year’s growth was taken from each tree by clipping a branch tip. Samples were oven-dried for 1 week at approximately 45°C, ground to a fine powder using a mortar and pestle, and placed in a desiccant chamber for sample preservation. Foliar N concentrations were measured at the Western Washington University, Bellingham campus, using the Thermo Scientific Flash EA1112 Elemental Analyzer (Thermo Electron Corporation, Milan, Italy).
During growth and foliar tissue collection, root tips were excavated from each tree for ECM analysis. These sites were extremely rocky and difficult to sample with cores or probes. Therefore, sediments and rocks were removed with a spade to expose the conifer root system by carefully trenching approximately 50 cm from the base of each seedling to a depth of 25 cm and a width of 45 cm. Approximately three to five root segments that were 10–12 cm long and 1–3 mm in diameter were removed, placed in a plastic bag within a cooler, returned to the laboratory, and stored at 4°C until further processing (described below). At the same time, soil samples for N analysis were extracted (approximately 0.50 L) using a spade to a depth of roughly 18 cm adjacent to the roots of each tree.
Vegetation richness and community composition assessment
Vegetation composition was recorded in a circular, 2-m2 quadrat centered on selected trees. All plant taxa were identified to species, if possible, and plant abundance was visually estimated by cover class: 1 (<1%), 2 (1% to 10%), 3 (11% to 25%), 4 (26% to 50%), 5 (51% to 75%), 6 (76% to 90%), and 7 (>90%). Species richness was expressed as the number of species accumulated across the three quadrats. All plants were identified to species using Hitchcock and Cronquist (1973) and Pojar and MacKinnon (1994). Species were classified as native or introduced (whether naturalized or not). Botanical nomenclature and nativity followed the plant profile descriptions outlined by the United States Department of Agriculture, PLANTS Database (USDA and NRCS, 2023). Species richness included all planted, seeded, or naturally recruited species within each quadrat. L. rivularis and any planted trees were excluded from the community-level analyses (described below).
Soil texture/particle size distribution
To account for the heterogeneity of the sediment, the soil-particle size distribution in each quadrat was assessed. Particle size-class data were collected at 10-cm intervals along two 1.6-m perpendicular transects per quadrat (36 counts per quadrat). The Wentworth scale was used to classify each randomly selected particle by length along the shorter axis (Wentworth, 1922; Supplementary Table 1). The median particle size in each quadrat was determined and assigned as a categorical variable from the Wentworth scale. The average length of each Wentworth size class was determined and assigned to the median of the particle count as a numeric value. Small particle sizes such as sands (0.13 to 1 mm) and silt (<0.13 mm) were not measured directly but were determined by rubbing the selected particles between finger and thumb, with silts being smoother to the touch than sands.
Soil organic matter and nitrogen
Soil collection for OM content was performed by taking the first 3 cm of sediment in four locations per quadrat and compositing them (n = 24; 250 g per composite sample). At the time of sampling, soils were placed in sealed bags after collection, and mass was determined pre- and post-drying. Samples were placed in a drying oven at 45°C for 1 week. Soil OM was measured using weight-loss-on ignition (Wang et al., 2001). Dried samples were weighed, then heated at 540°C for 5 hours in a Nanbei muffle furnace, and then weighed again to calculate OM content (%). After ashing, each sample was weighed to calculate the difference between dry and burned composites, indicating the OM (g) content of the soil sample. A portion of each composite sample was used to determine total N (%). Subsamples were ground to a powder with a Spex Mill grinder, and N content was determined with a Thermo Scientific Flash EA1112 Elemental Analyzer (Thermo Electron Corporation, Milan, Italy).
Ectomycorrhizal colonization and identification
In the laboratory, root tips were washed and placed in a Petri dish in autoclaved, distilled water. Roots were cut into 3-cm segments, and 100 randomly selected root tips per tree were scored for presence/absence of ECM (i.e., presence/absence of a fungal sheath; Simard et al., 1997; Massicotte et al., 1999). ECM colonization was expressed as the percentage of root tips with a fungal sheath. A 3-mm segment of ECM root tip was homogenized using a mortar and pestle, and DNA was extracted using appropriate buffers and filter columns provided by QIAGEN® DNeasy Plant Pro Kit per manufacturer’s protocol (QIAGEN, Germantown, MD, USA). Approximately 10 ng of this DNA was used for PCR amplification using primers ITS1-F (5′ cttggtcatttagaggaagtaa 3′) and ITS4 (5′ tcctccgcttattgatatgc 3′; Gardes and Bruns, 1993). PCRs were based on the following concentrations for a 25-μl reaction: 12.5 μl of GoTaq® Green Master Mix (Promega, Madison, WI, USA), 0.25 μl of 25 μM of each primer, 11 μl of molecular grade water, and 1 μl of DNA template. Temperature cycling was accomplished using GeneAmp PCR System 9700, which allowed for a programmable Thermal Cycler Heating regimen (described in Bauman et al., 2022).
Positive PCR products were confirmed using gel electrophoresis and purified using a Wizard® SV 96 Genomic DNA Purification System (Promega, Madison, WI, USA). DNA concentration was quantified using a Thermo Scientific 2000 1-position Spectrophotometer (Thermo Fisher Scientific, Pittsburg, PA, USA) prior to sequencing. Sanger sequencing was performed using the Applied Biosystems ABI Prism 3730 DNA Analyzer (Retrogen Inc., San Diego, CA, USA). The DNA sequences were analyzed and edited using Retrogen Inc. software. To identify the fungus found on roots, internal transcribed spacer (ITS) sequences from samples were compared with those in the GenBank using the BLAST search (Altschul et al., 1997). The genus of each fungus reported in this study was based on the best matches of those in the GenBank with a >97% ITS sequence similarity as a threshold.
Statistical approach
Linear mixed-effects models (Zuur et al., 2007) were used to assess the relationships between relative abundance of lupine (cover class) and tree height (cm), tree basal diameter (mm), ECM root colonization (%), foliar and soil C:N, foliar and soil N (%), soil OM (%), species richness, and number of non-native species. Lupine cover class was the fixed factor in the model. Tree species and median soil particle size class comprised the random factors to account for varying genotypes and heterogeneity of substrate, respectively. In its simplest form, the linear mixed-effects model can be written as follows:
where Y is the response variable, Li is the fixed effect of lupine cover class (dense, medium, or sparse), S is the random effect of median soil particle size class using the Wentworth scale, T is the random effect of tree species nested within the lupine cover class, μ is the true mean response among all experimental units, and ϵ is residual error.
Evaluation of mixed-effects model terms was performed using ANOVA tests and posterior predictive simulation to make an informed judgment on model fit using lme4: mixed-effects modeling with R (Gelman and Hill, 2006; Bates et al., 2015). The ANOVA tested for the significance of the fixed and random effects on each response variable (α = 0.05). The Welch–Satterthwaite equation was used to calculate the approximate degrees of freedom. Significant ANOVA products were evaluated for differences among lupine cover classes using Fisher’s least significant difference (LSD) pairwise comparisons. Fisher’s LSD procedure reduces the risk of false-positive judgments when the number of planned comparisons is small, as in this study (Marcus et al., 1976; Keppel, 1991; Meier, 2006; Gamst et al., 2008). The posterior predictive simulation (predictive power %) of the model was examined by generating an ensemble of simulations (n = 1,000) and observing how often the model predictions fell within the inner quartile range of the observed data to examine model fitness (Gelman and Hill, 2006).
To assess parametric test assumptions, all data were examined for normality and homoscedasticity using the Shapiro–Wilks and Levene’s tests, respectively. To achieve equal variance, transformations were performed on soil particle size (ψ; numeric class value squared) and ECM abundance (square root). Particle size gradation has a logarithmic scale (log2) since it doubles between each size class when expressed metrically as a length. Therefore, log2 was applied to the mean value for each particle bin level to produce ψ (Bunte and Abt, 2001). All statistical analyses were performed using the R Core Team (2013).
Permutational multivariate analysis of variance (PERMANOVA) was used to compare community composition among lupine cover classes. Planted trees and rare species (those with<1% cover) were excluded from the analysis. PERMANOVA was performed using the adonis function in the vegan package in R (Oksanen et al., 2010). The compositional variation among lupine cover classes was illustrated graphically with a non-metric multidimensional scaling (NMDS) ordination of a plot × species-abundance matrix using cover-class midpoints. The analysis was run with the metaMDS function in R using Bray–Curtis as the measure of dissimilarity (Oksanen et al., 2010). Plant species cover values were standardized via Wisconsin double standardization to improve results by equalizing emphasis among sample units and species. With the use of the indicspecies package, a multipattern analysis was used to identify the set of species with the highest association value with the perspective lupine cover classes using the multipatt function (De Cáceres and Legendre, 2009). A multi-level pattern analysis allowed species to be indicators of the three lupine cover classes by calculating an indicator value based on the product of the species’ relative abundance and relative frequency within each plot. The significance was determined by α = 0.05 for all analyses performed for this study.
Results
Conifer growth, foliar N concentrations, soils, and ECM colonization
Tree height was statistically similar among the three lupine cover classes: dense (35.7 ± 3.4 cm), medium (39.0 ± 2.4 cm), and sparse (33.0 ± 2.0 cm). Basal diameter followed the same non-significant trend when compared: dense cover (10.2 ± 0.6 mm), medium cover (9.7 ± 0.5 mm), and sparse cover (11.0 ± 0.6 mm). Foliar N concentrations did differ; conifers in dense- (1.8% ± 0.12%) and medium-cover (1.6% ± 0.09%) classes had higher concentrations of foliar N than those in sparse cover (1.3% ± 0.08%; β = 0.25, SE = 0.08, p< 0.001; Figure 2A).
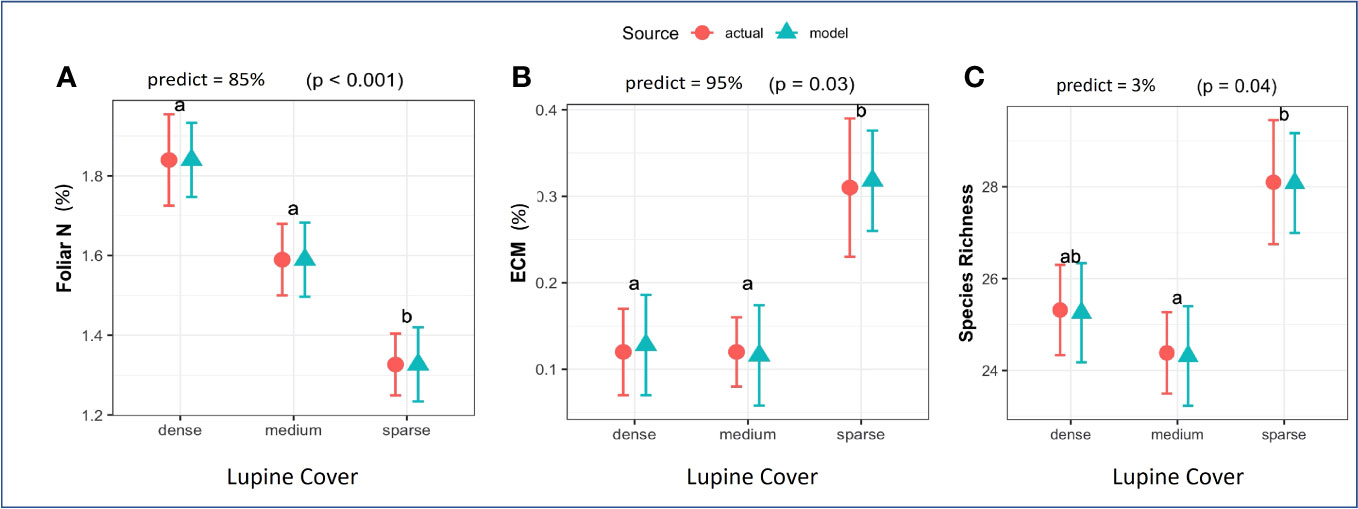
Figure 2 Response variables for which lupine cover class was a significant predictor in the mixed-effects models: (A) foliar N, (B) percent ectomycorrhizal (ECM) root colonization, and (C) species richness (mean number of species per 2-m2 quadrat). The modeled intercept and standard error were included to offer a visual comparison of measured (actual) vs. predicted (model). At the top of each plot, the “predict” value (%) quantifies the predictive power of the model, and the p-value indicates model significance. Treatments that do not share the same letter are significantly different (α = 0.05).
Soil OM did not differ among the cover classes and averaged 0.02% ± 0.001%, regardless of the density. Similarly, total soil N did not differ statistically: dense cover (1.8% ± 0.11%), medium cover (1.6% ± 0.09%), and sparse cover (1.3% ± 0.08%). None of the response variables showed significant variation related to median soil particle size or tree species (random effects in the mixed models).
ECM root colonization was greater in plots with sparse lupine cover (0.31% ± 0.08%) than in plots with dense-cover (0.12% ± 0.05%) and medium-cover plots (0.12% ± 0.04%; β = 0.14, SE = 0.03, p = 0.03; Figure 2B). Nine fungal taxa were detected on conifer roots (Table 1). The most frequent were Thelephora terrestris and Wilcoxina mikolae. Suillus luteus, Tuber pacificum, Hebeloma velutipes, and Rhizopogon were also present, but less common. Non-ECM taxon included a pathogen (Phialocephala sp.) and two endophytes, one in the order Helotiales and the second a species of Leptosphaeria (Table 1).
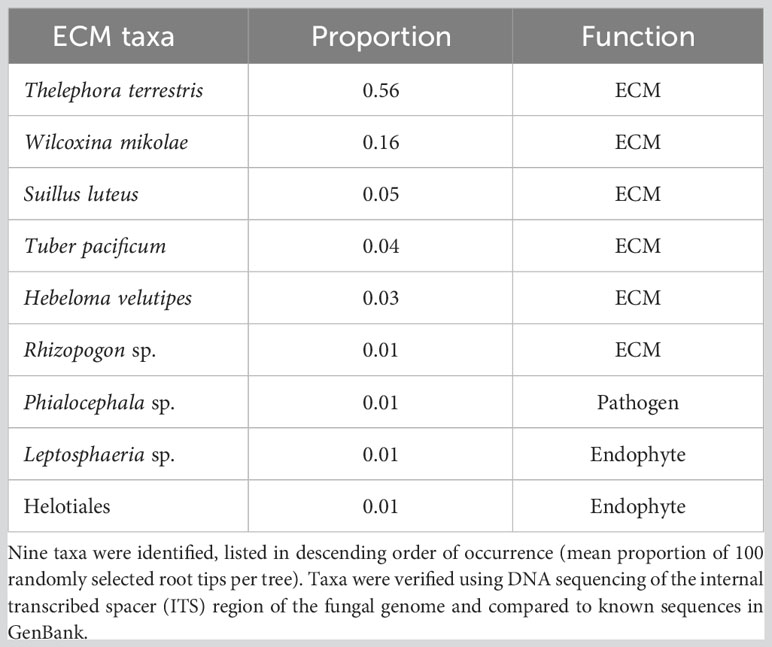
Table 1 List of ectomycorrhizal (ECM) fungi sampled from the roots of three conifer species planted on the dewatered terraces of Lake Mills in the Elwha River Valley.
Vegetation community composition
In total, we observed 49 plant taxa in plots, which included planted, seeded, and naturally recruiting species (Supplementary Table 2). Of these, 11 were grasses, 16 were forbs, and 22 were trees or shrubs. Plant species richness was greater in plots with sparse cover (28.5 ± 1.37) than in plots with medium cover (24.3 ± 0.89), while richness in plots with dense cover (25.2 ± 0.99) did not differ significantly from that of either sparse- or medium-cover plots (β = 25.9, SE = 0.61, p = 0.04; Figure 2C). The number of non-native plant species did not differ among the lupine cover classes.
An NMDS ordination illustrates a greater degree of compositional similarity between dense- and medium-cover plots than between medium- and sparse-cover plots (stress = 0.12, k = 2; Figure 3). The separation of dense- and sparse-cover plots along NMDS1 is consistent with their compositional difference, as determined by PERMANOVA (F(2,21) = 2.02, p = 0.01). Vulpia (VUPR) appeared associated with the sparse-cover plots, whereas Aira species (AICA and AIPR), hairy cat’s ear (HYRA), Oregon sunshine (ERLA), and blue wild rye (ELGL) were associated with the dense-cover plots (Figure 3). Results of a multipattern analysis indicated that no species were indicators of the sparse lupine-cover plots, but wood groundsel (SESY) and black cottonwood (POBA) were indicators of the medium- and dense-cover plots (all p < 0.05).
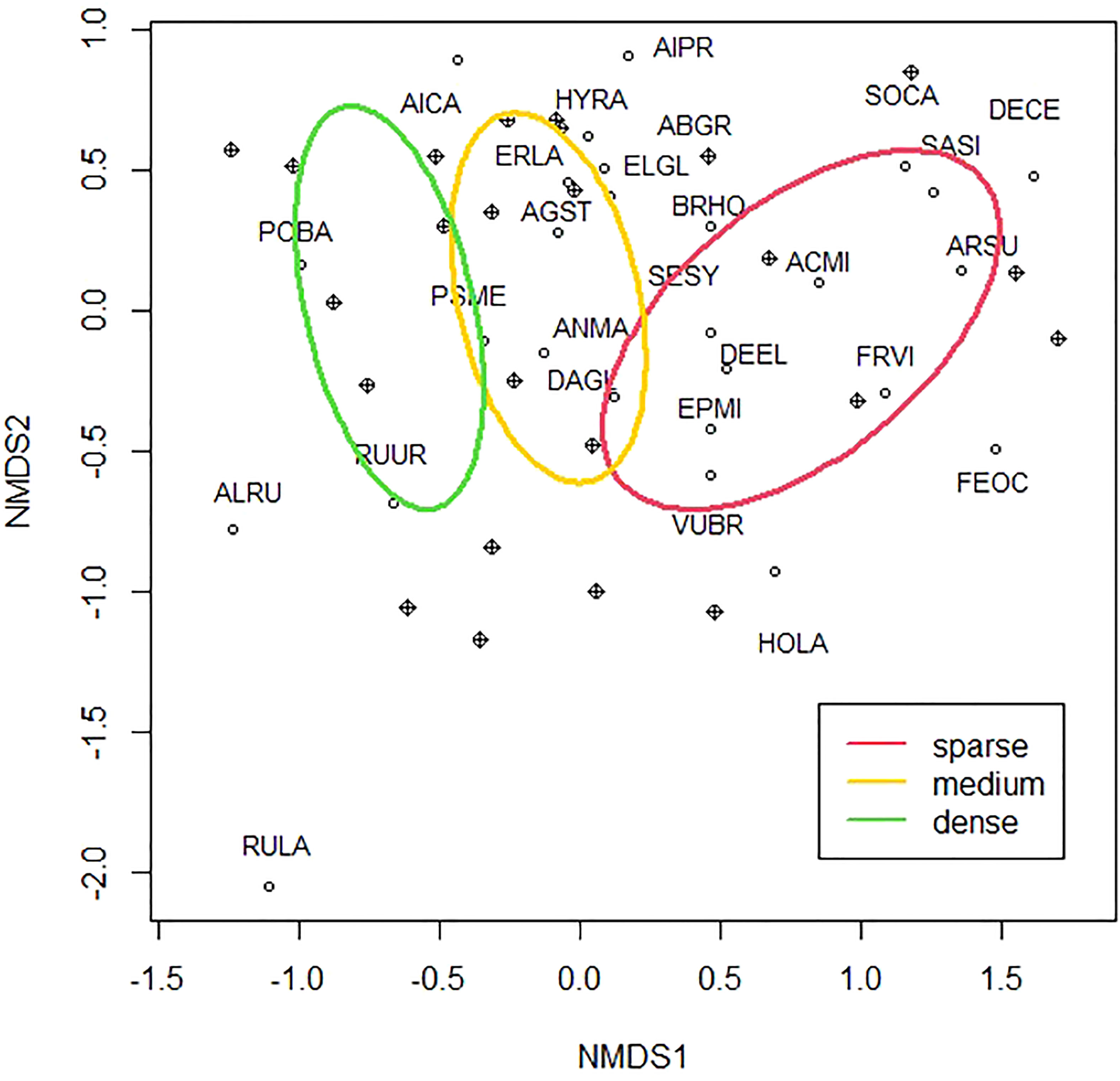
Figure 3 Non-metric multidimensional scaling (NMDS) ordination of plots representing the three lupine cover classes. Plant species centroids are represented by four-letter codes (see Supplementary Table 2 for the full names of species), and plots are represented by circles with crosses. Ellipses represent the 95% confidence intervals of plots within each lupine cover class.
Discussion
We documented lupine’s ability to thrive in highly disturbed landscapes without reducing the growth of planted conifers. A direct benefit included an increase in foliar N concentrations among seedlings growing in the medium and dense lupine cover classes. An unexpected finding was the reduction of ECM on conifer roots in medium and dense lupine plots. In addition, areas of dense lupine cover had fewer, and a different composition of, species than did areas of sparse lupine cover.
There was no difference in tree height or diameter among the three lupine cover classes. This differed from Mauer et al. (2013), who demonstrated Norway spruce (Picea abies), European beech (Fagus sylvatica), and Scots pine (Pinus sylvestris) to have an increased height and collar diameter when sowed among three species of lupine (Lupinus angustifolius, Lupinus albus, and Lupinus polyphyllus) after a 5-year growing period. In our study, greater foliar N was correlated with greater cover of riverbank lupine—at concentrations well above those deemed adequate for conifers in the PNW (N > 1.25%; Radwan and Brix, 1986; Walker and Gessel, 1990). Scots pines also showed an increase in foliar N levels during the first decade of growth when lupine was present (Prietzel et al., 2008). N-fixation by both Alnus incana and Lupinus nootkatensis was incorporated into the foliage of birch trees after 20 years on degraded forest soils (Myrold and Huss-Danell, 2003).
We also demonstrated a significant reduction of ECM colonization on conifer roots in the medium- and dense-cover plots. One possible explanation for the decrease in ECM colonization is that conifers may reduce carbon allocation to their ECM symbiont when N is not limiting (Corrêa et al., 2008). Our results support other research illustrating that carbon transfer from the host plant to the fungal symbiont depends on the N status of the tree (Nilsson et al., 2005). Field studies have documented that nitrogen fertilization can directly reduce ECM growth and colonization rates on longleaf pine (Pinus palustris; Sims et al., 2007). Ostonen et al. (2011) demonstrated that as N deposition loads increased, ECM biomass decreased in stands of Norwegian spruce (P. abies). This phenomenon was also observed by Nilsson and Wallander (2003) where Norwegian spruce under N additions resulted in a 50% ECM reduction when compared to trees within non-fertilized plots. This finding supports hypotheses that speculate mycorrhizal relationships range from mutualistic to parasitic, depending on soil nutrient status (Johnson, 1993; Johnson et al., 1997). Therefore, we propose a new hypothesis that investing carbon in non-photosynthetic symbionts is more costly than acquiring N from neighboring N-fixers.
We found less ECM species richness on novel terraces. Of the taxa identified, T. terrestris and W. mikolae were the most abundant. Both of these species are noted as among the most ubiquitous greenhouse and field ectomycorrhizas symbiotic with grand fir, Douglas fir, and western white pine (Massicotte et al., 1999; Hilszczańska and Sierota, 2013). Rare species included S. luteus, T. pacificum, and a Rhizopogon sp., all considered prevalent in PNW forests (Dahlberg, 2001). In addition, the average root colonization was rather low (averaged 30% ECM root tips). Soil organic matter is important for mycorrhizal symbioses, and studies have shown that mycorrhizal infection increases with organic matter amendments (Baar and DeVries, 1995; Lunt and Hedger, 2003). This low rate of colonization and small species list likely reflect a soil environment insufficient to support functional mycorrhizas (Cortese and Bunn, 2017).
As nitrogen fixers, Lupinus species develop N pools via atmospheric N fixation, which become available to plants via the mineralization of organic N into ammonium (Vitousek et al., 2002; Robertson and Groffman, 2007). While this study illustrated lupine’s ability to influence bioavailable N to neighboring conifers, we did not see differences in total soil N. In addition, we observed no differences when C:N and OM were compared among the lupine cover classes. The inability to detect differences in soil N may have been due to immediate bio-assimilation by conifers and neighboring vegetation coupled with N leaching in sediments with low water/nutrient holding capacity. We acknowledge that the measure of soil N was a single point-in-time measurement of total N, which could explain the lack of difference in soil N among lupine cover classes. Future work would benefit from the use of ion exchange resins, which integrate N availability over time (Qian and Schoenau, 2002). We noted that lupine litter-fall was abundant on the soil surface 4 years after seeding/planting; additional time may be needed to observe changes in the soil profile (Vitousek et al., 2002). In comparison, Halvorson et al. (1992) and Halvorson and Smith (1995) measured significant organic matter production and total increased N near the lupine’s (L. lepidus and Lupinus latifolius) rhizosphere, 7 years after the Mount Saint Helens eruption.
With regard to the vegetation community, we found that species richness decreased in plots with abundant lupine. This was also documented following the Mount Saint Helens eruption; Morris and Wood (1989) demonstrated that Pacific lupine (L. lepidus) inhibited immediate colonization of forbs in the early years, while later studies demonstrated a subsequent increase in plant community species richness that was accelerated by the presence of lupine (del Moral, 2007). We also found compositional differences in plots with dense vs. sparse lupine cover, suggesting that lupine may influence the trajectory of community development during primary succession (del Moral and Rozzell, 2005). The increase observed in plant species in the sparse lupine plots included a few rare native and non-native plants; however, the non-native species count was not different among the cover classes, and neither species was in high abundance or a significant indicator of sparse cover class. Chenoweth et al. (2022) found that seeding herbaceous species (including lupine) on these coarse terraces deterred non-native species establishment. This is of particular importance in coarse, nutrient-poor substrates with the lack of canopy closure that creates an opportunity for the invasion of exotic ruderal (Shafroth et al., 2002; Tabacchi et al., 2005; Funk and Vitousek, 2007; Michel et al., 2011). Important to the overarching goal of accelerating forest succession, lupine’s potential role as a beneficial cover crop may aid in the establishment of planted conifers while deterring non-native plant communities (Fierke and Kauffman, 2006; Peltzer et al., 2009; Urgenson et al., 2009).
Conclusion
The damming and the subsequent dewatering of Lake Mills created a phenomenal disturbance, leaving novel terraces void of ecological legacies. Similar to the post-eruption landforms colonized by lupine at Mount Saint Helens, seeded lupine in the Elwha River Valley was able to establish on the barren substrates. Important to restoration projects, lupine’s cover did not impede conifer growth in the early years of planting. We acknowledge that we used natural variation in lupine density as a predictor of conifer performance and soil development; therefore, lupine density itself could be a response to variation in soils, vegetation, or other environmental features. Spatial variation in lupine abundance could be caused by or otherwise confounded with other environmental conditions that were not measured. Regardless, when comparing conifer growth within dense lupine cover, lupine canopies were not found to be detrimental.
Further, lupine’s contribution to bio-available N promoted adequate foliar nitrogen concentrations that may contribute to greater growth in the subsequent years. It is not clear whether this increase in available N was the mechanism behind the inverse relationship with lupine and ECM root colonization; however, current research is further investigating this. This may be an explanation of tradeoffs: ECM fungi aid in nutrient uptake/growth in low lupine density cover, but N-fixation by lupine serves this function at higher densities.
This study also illustrates the challenges for revegetation in coarse sediments and the important function of a cover crop on sediment surfaces. Lupine OM contributions are an important attribute for the successional trajectory, given its biennial life cycle and ability to tolerate resource-limited soils. Although lupine did not contribute to an increase in soil carbon or OM, we hypothesize that lupine will contribute important carbon pools to the forest soil-building process after a decade of recovery, which may also lead to an increase in ECM fungal colonization and native plant recruitment. Importantly, this study also documented the benefits of including a locally native N-fixing species (riverbank lupine) in the Elwha River Restoration project, which can inform future practitioners when considering strategies of revegetation after dam removal. Based on our findings and corroborative literature, we recommend the inclusion of native lupine in highly disturbed, N-limiting soils to contribute to the N pools for native tree establishment. These attributes may accelerate forest succession leading to closed canopies much faster than passive recovery on coarse soils.
Data availability statement
The datasets presented in this study can be found in online repositories. The names of the repository/repositories and accession number(s) can be found in the article/Supplementary Material.
Author contributions
JK: literature review, study design, data collection and analysis, and synthesis. MDL: DNA extraction and PCR. AL: DNA extraction and PCR, and literature review. JB: literature review, study design, data collection and analysis, synthesis, and project oversite. All authors contributed to the article and approved the submitted version.
Funding
Research funding was provided by the Brooks Family Research Fund, Western Washington University’s Research and Sponsored Programs, the Stuntz Mycology Fund, and the Ross Travel Grant.
Acknowledgments
Dr. Jim Helfield, Amy Lawrence, and Dr. Robin Matthews provided feedback on the study design, statistical analysis, and draft revisions. Joshua Chenoweth, who led the forest restoration project in the Elwha River basin, provided intimate knowledge of the system, restoration methods, and efficient field sampling methods.
Conflict of interest
The authors declare that the research was conducted in the absence of any commercial or financial relationships that could be construed as a potential conflict of interest.
Publisher’s note
All claims expressed in this article are solely those of the authors and do not necessarily represent those of their affiliated organizations, or those of the publisher, the editors and the reviewers. Any product that may be evaluated in this article, or claim that may be made by its manufacturer, is not guaranteed or endorsed by the publisher.
Supplementary material
The Supplementary Material for this article can be found online at: https://www.frontiersin.org/articles/10.3389/fevo.2023.1214117/full#supplementary-material
References
Altschul S. F., Madden T. L., Schaffer A. A., Zhang J., Zhang Z., Miller W., et al. (1997). Gapped BLAST and PSIBLAST: a new generation of protein database search programs. Nucleic Acids Res. 25, 3389–3402. doi: 10.1093/nar/25.17.3389
Andersson E., Nilsson C., Johansson M. E. (2000). Effects of river fragmentation on plant dispersal and riparian flora. Regul. Rivers.: Res. Manage. 16, 83–89. doi: 10.1002/(SICI)1099-1646(200001/02)16:1<83::AID-RRR567>3.0.CO;2-T
Baar J., DeVries F. (1995). Effects of manipulation of litter and humus layers on ectomycorrhizal colonization potential in Scots pine stands of different age. Mycorrhiza 5, 267–272. doi: 10.1007/BF00204960
Balestrini R., Kottke I., Martin. F. (2016). “Structure and development of ectomycorrhizal roots,” in Molecular mycorrhizal symbiosis (Hoboken, NJ: John Wiley & Sons, Inc).
Bates D., Mächler M., Bolker B., Walker S. (2015). Fitting linear mixed-effects models using lme4. J. Stat. Softw. 67 (1), 1–48. doi: 10.18637/jss.v067.i01
Bauman J. M., Franklin J., Santas A. (2022). Castanea dentata interactions and ectomycorrhizal colonization in novel ecosystems. Ecol. Restor. 40, 179–190. doi: 10.3368/er.40.3.179
Bauman J. M., Kardouni J. D. (2018). Elwha River restoration: Tribal voices matter in the restoration of natural resources. Reclamation. Matters. Fall. 2), 23–29.
Bertness M. D., Calaway R. M. (1994). Positive interactions in communities. Trends Ecol. Evol. 9, 191–193. doi: 10.1016/0169-5347(94)90088-4
Bishop J. (2002). Early primary succession on Mount St. Helens: impact of insect herbivores on colonizing lupines. Ecology 83, 191–202. doi: 10.1890/0012-9658(2002)083[0191:EPSOMS]2.0.CO;2
Bunte K., Abt S. R. (2001). Sampling surface and subsurface particle-size distributions in wadable gravel- and cobble-bed streams for analyses in sediment transport, hydraulics, and streambed monitoring. (Fort Collins, CO: U.S. Department of Agriculture, Forest Service, Rocky Mountain Research Station). doi: 10.2737/RMRS-GTR-74
Chenoweth J., Acker S. A., McHenry M. L. (2011). Revegetation and restoration plan for Lake Mills and Lake Aldwell (Port Angeles, WA: Olympic National Park and the Lower Elwha Klallam Tribe).
Chenoweth J., Bakker J. D., Acker S. A. (2022). Planting, seeding, and sediment impact restoration success following dam removal. Restor. Ecol. 30, 1–13. doi: 10.1111/rec.13506
Corrêa A., Strasser R. J., Martins-Loução M. A. (2008). Response of plants to ectomycorrhizae in N-limited conditions: which factors determine its variation? Mycorrhiza 18, 413–427. doi: 10.1007/s00572-008-0195-0
Cortese A. M., Bunn R. A. (2017). Availability and function of arbuscular mycorrhizal and ectomycorrhizal fungi during revegetation of dewatered reservoirs left after dam removal. Restor. Ecol. 25, 63–71. doi: 10.1111/rec.12406
Dahlberg A. (2001). Community ecology of ectomycorrhizal fungi: an advancing interdisciplinary field. New Phytol. 150, 555–562. doi: 10.1046/j.1469-8137.2001.00142.x
Darris D., Young-Mathews A. (2012). Plant fact sheet for riverbank lupine (Lupinus rivularis) (Corvallis, OR: USDA-Natural Resources Conservation Service, Corvallis Plant Materials Center).
De Cáceres M., Legendre P. (2009). Associations between species and groups of sites: Indices and statistical inference. Ecology 90, 3566–3574. doi: 10.1890/08-1823.1
del Moral R., Titus J. H., Cook A. M. (1995). Early primary succession on Mount St. Helens, USA. J. Veg. Sci. 6, 107–120.
del Moral R. (2007). Limits to convergence of vegetation during early primary succession. J. Vegetation. Sci. 18, 479–488. doi: 10.1111/j.1654-1103.2007.tb02562.x
del Moral R., Rozzell R. L. (2005). Long-term effects of Lupinus lepidus on vegetation dynamics at Mount St. Helens. Plant Ecol. 181, 203–215. doi: 10.1007/s11258-005-6627-4
DOI (U.S. Department of Interior), NMFS (National Marine Fisheries Service), Lower Elwha S’Klallam Tribe (1996). “Elwha River restoration implementation,” in Draft environmental impact statement, April 1996 (Port Angeles, WA: Olympic National Park).
DOI National Park Service (2015) Elwha revegetation (Port Angeles, WA: Olympic National Park). Available at: https://www.nps.gov/olym/learn/nature/elwha-revegetation.htm (Accessed 17 October 2015).
Drake D. C., Naiman R. J., Helfield J. M. (2002). Reconstructing salmon abundance for rivers: an initial dendrochronological evaluation. Ecology 83, 2971–2977. doi: 10.1890/0012-9658(2002)083[2971:RSAIRA]2.0.CO;2
Duda J. J., Freilich J. E., Schreiner E. G. (2008). Baseline studies in the elwha river ecosystem prior to dam removal: introduction to the special issue. Northwest. Sci. 82, 1–12. doi: 10.3955/0029-344X-82.S.I.1
Duda J. J., Warrick J. A., Magirl C. S. (2011). “Coastal and lower Elwha River, Washington, prior to dam removal - history, status, and defining characteristics,” in Coastal habitats of the Elwha River: biological and physical patterns and processes prior to dam removal. U.S. Geological Survey scientific investigations report 2011-5120. Eds. Duda J. J., Warrick J. A., Magirl C. S. (Reston, VA: U.S. Geological Survey), 1–26.
East A. E., Pess G. R., Bountry J. A., Magirl C. S., Ritchie A. C., Logan J. B., et al. (2015). Large-scale dam removal on the Elwha River, Washington, USA: River channel and floodplain geomorphic change. Geomorphology 228, 765–786. doi: 10.1016/j.geomorph.2014.08.028
Fierke M. K., Kauffman J. B. (2006). Invasive species influence riparian plant diversity along a successional gradient, Willamette River, Oregon. Natural Areas. J. 26, 376–382. doi: 10.3375/0885-8608(2006)26[376:ISIRPD]2.0.CO;2
Flores J., Jurado E. (2003). Are nurse-protégé interaction more common among plants from arid environments? J. Vegetation. Sci. 14, 911–916. doi: 10.1111/j.1654-1103.2003.tb02225.x
Franklin J. F. (1988). “Pacific northwest forests,” in North American terrestrial vegetation. Eds. Barbour M. G., Billings W. D. (London: Cambridge University Press), 103–130.
Funk J., Vitousek P. (2007). Resource-use efficiency and plant invasion in low-resource systems. Nature 446, 1079–1081. doi: 10.1038/nature05719
Gamst G., Meyers L., Guarino A. (2008). “Frontmatter,” in Analysis of variance designs: A conceptual and computational approach with SPSS and SAS (pp. I-iv) (Cambridge: Cambridge University Press).
Gardes M., Bruns T. D. (1993). ITS primers with enhanced specificity for basidiomycetes – application to the identification of mycorrhizae and rusts. Mol. Ecol. 2, 113–118. doi: 10.1111/j.1365-294X.1993.tb00005.x
Gelman A., Hill J. (2006). Data analysis using regression and multilevel/hierarchical models (Cambridge, England: Cambridge University Press).
Gregory S., Hiram L., Judy L. (2002). The conceptual basis for ecological responses to dam removal: resource managers face enormous challenges in assessing the consequences of removing large dams from rivers and evaluating management options. BioScience 52, 713–723. doi: 10.1641/0006-3568(2002)052[0713:TCBFER]2.0.CO;2
Hall C. J., Jordaan A., Frisk M. G. (2011). The historic influence of dams on diadromous fish habitat with a focus on river herring and hydrologic longitudinal connectivity. Landscape Ecol. 26, 95–107. doi: 10.1007/s10980-010-9539-1
Halvorson J. J., Franz E. H., Smith J. L., Black R. A. (1992). Nitrogenase activity, nitrogen fixation, and nitrogen inputs by lupines at Mount St. Helens. Ecology 73, 87–98. doi: 10.2307/1938723
Halvorson J. J., Smith J. L. (1995). Decomposition of lupine biomass by soil microorganisms in developing Mound St. Helens pyroclastic soils. Soil Biol. Biochem. 27, 983–992. doi: 10.1016/0038-0717(95)00026-B
Halvorson J. J., Smith J. L., Franz E. H. (1991). Lupine influence on soil carbon, nitrogen and microbial activity in developing ecosystems at Mount St. Helens. Oecologia 87, 162–170. doi: 10.1007/BF00325253
Harrison R. B., Maguire D. A., Page-Dumroese D. (2011). Best management practices for maintaining soil productivity in the Douglas-fir region: Chapter 6 Maintaining adequate nitrogen supply - principles, decision-support tools, and best management practices (Corvallis: Oregon State University Press).
Helfield J. M., Naiman R. J. (2001). Effects of salmon-derived nitrogen on riparian forest growth and implications for stream productivity. Ecology 82, 2403–2409. doi: 10.1890/0012-9658(2001)082[2403:EOSDNO]2.0.CO;2
Hilszczańska D., Sierota Z. (2013). Persistence of ectomycorrhizas by Thelephora terrestris on outplanted Scots pine seedlings. Acta Mycol. 41 (2), 313–318. doi: 10.5586/am.2006.032
Hitchcock C. L., Cronquist A. (1973). Flora of the pacific northwest: an illustrated manual. 5th edn (Seattle and London: University of Washington Press).
Jansson R., Nilson C., Dynesius M., Andersson E. (2000). Effects of river regulation on river-margin vegetation: A comparison of eight boreal rivers. Ecol. Appl. 10, 203–224. doi: 10.1890/1051-0761(2000)010[0203:EORROR]2.0.CO;2
Johnson N. C. (1993). Can fertilization of soil select less mutualistic mycorrhizae? Ecol. Appl. 3, 749–757. doi: 10.2307/1942106
Johnson N. C., Graham J. H., Smith F. A. (1997). Functioning of mycorrhizal associations along the mutualism-parasitism continuum. New Phytol. 135, 575–585. doi: 10.1046/j.1469-8137.1997.00729.x
Keppel G. (1991). Design and analysis: A researcher’s handbook (Englewood Cliffs, N.J: Prentice Hall).
Kranabetter J. M., Stoehr M., O’Neill G. A. (2015). Ectomycorrhizal fungal maladaptation and growth reductions associated with assisted migration of Douglas-fir. New Phytol. 206, 1135–1144. doi: 10.1111/nph.13287
Li H. W., Schreck C. B., Bond C. E., Rexstad E. (1987). “Factors influencing changes in fish assemblages of Pacific Northwest streams,” in Community and evolutionary ecology of north american stream fishes (Norman: University of Oklahoma Press), 193–202.
Lunt P. H., Hedger J. N. (2003). Effects of organic enrichment of mine spoil on growth and nutrient uptake in oak seedlings inoculated with selected ectomycorrhizal fungi. Restor. Ecol. 11, 125–130. doi: 10.1046/j.1526-100X.2003.09968.x
Major J. J., East A. E., O’Connor J. E., Grant G. E., Wilcox A. C., Magirl C. S., et al. (2017). “Geomorphic responses to U.S. dam removals–a two-decade perspective,” in Gravel bed rivers and disasters. Eds. Tsutsumi D., Laronne J. (Hoboken, New Jersey, USA: Wiley and Sons), 355–383.
Marcus R., Peritz E., Gabriel K. R. (1976). On closed testing procedures with special reference to ordered analysis of variance. Biometrika 63, 655–660. doi: 10.1093/biomet/63.3.655
Massicotte H. B., Molina R., Tackaberry L. E., Smith J. E., Amaranthus M. P. (1999). Diversity and host specificity of ectomycorrhizal fungi retrieved from three adjacent forest sites by five host species. Can. J. Bot. 77, 1053–1076. doi: 10.1139/b99-115
Mauer O., Vavříček D., Palátová E. (2013). Assessing the influence of the Lupinus genus in the biological reclamation of sites degraded by whole-area dozer soil treatment. Acta Univ. Agricult. Silvicult. Mendelianae. Brunensis. 61 (3), 711–720. doi: 10.11118/actaun201361030711
Meier U. (2006). A note on the power of fisher’s least significant difference procedure. Pharm. Stat 5, 253–263. doi: 10.1002/pst.210
Michel J. T., Helfield J. M., Hooper D. U. (2011). Seed rain and revegetation of exposed substrates following dam removal on the Elwha River. Northwest. Sci. 85, 15–29. doi: 10.3955/046.085.0102
Morris W. F., Wood D. M. (1989). The role of Lupinus lepidus in succession on Mount St. Helens: facilitation or inhibition? Ecology 70, 697–703. doi: 10.2307/1940220
Myrold D., Huss-Danell K. (2003). Alder and lupine enhance nitrogen cycling in a degraded forest soil in Northern Sweden. Plant Soil 254, 47–56. doi: 10.1023/A:1024951115548
Nilsson L. O., Giesler R., Bååth E., Wallander H. (2005). Growth and biomass of mycorrhizal mycelia in coniferous forests along short natural nutrient gradients. New Phytol. 165, 613–622. doi: 10.1111/j.1469-8137.2004.01223.x
Nilsson L. O., Wallander H. (2003). Production of external mycelium by ectomycorrhizal fungi in a Norway spruce forest was reduced in response to nitrogen fertilization. New Phytol. 158, 409–416. doi: 10.1046/j.1469-8137.2003.00728.x
Oksanen J., Blanchet F. G., Kindt R., Legendre P., Ohara R. G., Simpson G. L., et al. (2010) Vegan: Community ecology package. R package version 1.17–0. Available at: http://CRAN.Rproject.org/package=vegan (Accessed October 11, 2019).
Oldřich M., Vavříček D., Palátová E. (2013). Assessing the influence of the Lupinus genus in the biological reclamation of sites degraded by whole area dozer soil treatment. Acta Univ. Agric. Silvic. Mendel. 61, 711–720. doi: 10.11118/actaun201361030711
Ostonen I., Helmisaari H.-S., Borken W., Tedersoo L., Kukumägi M., Bahram M., et al. (2011). Fine root foraging strategies in Norway spruce forests across a European climate gradient. Global Change Biol. 17 (12), 3620–3632. doi: 10.1111/j.1365-2486.2011.02501.x
Peltzer D. A., Bellingham P. J., Kurokawa H., Walker L. R., Wardle D. A., Yeates G. W. (2009). Punching above their weight: low-biomass non-native plant species alter soil properties during primary succession. Oiko 118, 1001–1014. doi: 10.1111/j.1600-0706.2009.17244.x
Perry L. G., Shafroth P. B., Perakis S. S. (2016). Riparian soil development linked to forest succession above and below dams along the Elwha River, Washington, USA. Ecosystems 20, 104–129. doi: 10.1007/s10021-016-0080-1
Pess G. R., McHenry M. L., Beechie T. J., Davies J. (2008). Biological impacts of the Elwha River dams and potential salmonid responses to dam removal. Northwest. Sci. 82, 72–90. doi: 10.3955/0029-344X-82.S.I.72
Pizzuto J. (2002). Effects of Dam Removal on River Form and Process Although many well-established concepts of fluvial geomorphology are relevant for evaluating the effects of dam removal, geomorphologists remain unable to forecast stream channel changes caused by the removal of specific dams. BioScience 52, 683–691. doi: 10.1641/0006-3568(2002)052[0683:EODROR]2.0.CO;2
Pojar J., MacKinnon A. (1994). Plants of the pacific northwest coast: washington, oregon, british columbia & Alaska. Eds. Alaback P., Antos J., Goward T., Lertzman K., Pojar R., Reed A., Turner N., Vitt D. (Vancouver, British Columbia: Lone Pine Publishing).
Prietzel J., Rehfuess K. E., Stetter U., Pretzsch H. (2008). Changes of soil chemistry, stand nutrition, and stand growth at two Scots pine (Pinus sylvestris L.) sites in Central Europe during 40 years after fertilization, liming, and lupine introduction. Eur. J. For. Res. 127, 43–61. doi: 10.1007/s10342-007-0181-7
Qian P., Schoenau J. J. (2002). Practical applications of ion exchange resins in agricultural and environmental soil research. Can. J. Soil Sci. 82, 9–21. doi: 10.4141/S00-091
Radwan M. A., Brix H. (1986). Nutrition of Douglas-fir. Douglas-fir: stand management for the future. Eds. Oliver C. D., Hanley D. P., Johnson J. A. (Seattle: Institute of Forest Resources, University of Washington), 177–188.
Raffaele E., Veblen T. T. (1998). Facilitation by nurse shrubs of resprouting behavior in a post-fire shrubland in northern Patagonia, Argentina. J. Vegetation. Sci. 9, 693–698. doi: 10.2307/3237287
R Core Team (2013). R: A language and environment for statistical computing (Vienna, Austria: R Foundation for Statistical Computing). Available at: http://www.R-project.org/.
Reidy Liermann C., Nilsson C., Robertson J., Ng R. Y. (2012). Implications of dam obstruction for global freshwater fish diversity. Bioscience 62, 539–548. doi: 10.1525/bio.2012.62.6.5
Robertson G. P., Groffman P. M. (2007). Nitrogen transformations. In soil microbiology, ecology and biochemistry (Burlington, Massachusetts, USA: Elsevier), 341–364.
Rood S. B., Mahoney J. M. (2000). Revised instream flow regulation enables cottonwood recruitment along the St. Mary river, Alberta, Canada. Rivers 7, 109–125.
Sadin P., Vogel D. (2011) An interpretive history of the elwha river valley and the legacy of hydropower on washington’s olympic peninsula. Available at: https://www.nps.gov/olym/learn/nature/elwha-river-restoration-research-publications.htm (Accessed 16 July 2018).
Shaffer J. A., Crain P., Winter B., McHenry M., Lear C., Randle T. (2008). Nearshore restoration of the Elwha River through removal of the Elwha and Glines Canyon dams: an overview. Northwest. Sci. 82, 48–58. doi: 10.3955/0029-344X-82.S.I.48
Shafroth P. (1999). Downstream effects of dams on riparian vegetation: Bill Williams river, Arizona. Dissertation. Abstracts. International. Part B.: Sci. Eng. 60, 928. doi: 10.1641/0006-3568(2002)052[0703:PRORVT]2.0.CO;2
Shafroth P. B., Friedman J. M., Auble G. T., Scott M. L., Braatne J. H. (2002). Potential response of riparian vegetation to dam removal. Bioscience 52, 703–712.
Sharma V. K. (2001). Post impoundment changes in river Beas after Pong Dam emergence. Appl. Fisheries. Aquacult. 1, 9–12.
Simard S., Perry D., Smith J., Molina R. (1997). Effects of soil trenching on occurrence of ectomycorrhizas on Pseudotsuga menziesii seedlings grown in mature forests of Betula papyrifera and Pseudotsuga menziesii. New Phytol. 136, 327–340. doi: 10.1046/j.1469-8137.1997.00731.x
Sims S. E., Hendricks J. J., Mitchell R. J., Kuehn K. A., Pecot S. D. (2007). Nitrogen decreases and precipitation increases ectomycorrhizal extramatrical mycelia production in a longleaf pine forest. Mycorrhiza 17 (4), 299–309. doi: 10.1007/s00572-007-0105-x
Staniewski R. (1970). Typing of rhizobium by phages. Can. J. Microbiol. 16, 1003–1009. doi: 10.1139/m70-170
Stanley E. H., Doyle M. W. (2003). Trading off: the ecological effects of dam removal. Front. Ecol. Environ. 1, 15–22. doi: 10.1890/1540-9295(2003)001[0015:TOTEEO]2.0.CO;2
Tabacchi E., Planty-Tabacchi A. M., Roques L., Nadal E. (2005). Seed inputs in riparian zones: Implications for plant invasion. River. Res. Appl. 21, 299–313. doi: 10.1002/rra.848
Urgenson L. S., Reichard S. H., Halpern C. B. (2009). Community and ecosystem consequences of giant knotweed (Polygonum sachalinense) invasion into riparian forests of western Washington, USA. Biol. Conserv. 142, 1536–1541. doi: 10.1016/j.biocon.2009.02.023
USDA, NRCS (2023). The PLANTS database (Greensboro, NC USA: National Plant Data Team). Available at: http://plants.usda.gov.
Vitousek P. M., Cassman K., Cleveland C., Crews T., Field C. B., Grimm N. B., et al. (2002). “Towards an ecological understanding of biological nitrogen fixation,” in The nitrogen cycle at regional to global scales. Eds. Boyer E. W., Howarth R. W. (Dordrecht/Boston/London: Springer Netherlands), 1–45.
Vitousek P. M., Walker L. R., Whiteaker L. D., Mueller Dombois D., Matson P. A. (1987). Biological invasion by Myrica faya alters ecosystem development in Hawai’i. Science 238, 802–804. doi: 10.1126/science.238.4828.802
Walker R. B., Gessel S. P. (1990). Technical note: mineral deficiency symptoms of Pacific Northwest conifers. Western. J. Appl. Forestry. 5, 96–98. doi: 10.1093/wjaf/5.3.96
Wang Q., Li Y., Wang Y. (2001). Optimizing the weight loss-on-ignition methodology to quantify organic and carbonate carbon of sediments from diverse sources. Environ. Monit. Assess. 174, 241–257. doi: 10.1007/s10661-010-1454-z
Warrick J. A., Bountry J. A., East A. E., Magirl C. S., Randle T. J., Gelfenbaum G., et al. (2015). Large-scale dam removal on the Elwha River, Washington, USA: Source-to-sink sediment budget and synthesis. Geomorphology 246, 729–750. doi: 10.1016/j.geomorph.2015.01.010
Weidlich E. W. A., Florido F. G., Sorriniand T. B., Brancalion P. H. S. (2020). Controlling invasive plant species in ecological restoration: A global review. J. Appl. Ecol. 9, 1806–1817. doi: 10.1111/1365-2664.13656
Wentworth C. K. (1922). A scale of grade and class terms for clastic sediments. J. Geol. 30, 377–392. doi: 10.1086/622910
Winter B. D., Crain P. (2008). Making the case for ecosystem restoration by dam removal in the elwha river. Washington. Northwest. Sci. 82, 13–28. doi: 10.3955/0029-344X-82.S.I.13
Keywords: companion planting, restoration, soil development, vegetation, cover crop
Citation: Kardouni J, Danilchik Lindsay M, Labay A and Bauman JM (2023) Riverbank lupine’s (Lupinus rivularis) influence on conifer growth, ectomycorrhizal colonization, and neighboring vegetation in coarse sediments left behind after dam removal. Front. Ecol. Evol. 11:1214117. doi: 10.3389/fevo.2023.1214117
Received: 28 April 2023; Accepted: 18 September 2023;
Published: 13 October 2023.
Edited by:
Rebecca McCaffery, United States Department of the Interior, United StatesReviewed by:
Charles Halpern, University of Washington, United StatesLaura Perry, Colorado State University, United States
Copyright © 2023 Kardouni, Danilchik Lindsay, Labay and Bauman. This is an open-access article distributed under the terms of the Creative Commons Attribution License (CC BY). The use, distribution or reproduction in other forums is permitted, provided the original author(s) and the copyright owner(s) are credited and that the original publication in this journal is cited, in accordance with accepted academic practice. No use, distribution or reproduction is permitted which does not comply with these terms.
*Correspondence: Jenise M. Bauman, SmVuaXNlLkJhdW1hbkB3d3UuZWR1