- 1Laboratorio de Eco-Epidemiología, Departamento de Ecología, Genética y Evolución, Universidad de Buenos Aires, Ciudad Autónoma de Buenos Aires, Argentina
- 2Instituto de Ecología, Genética y Evolución (CONICET – IEGEBA), CONICET – Universidad de Buenos Aires, Buenos Aires, Argentina
- 3Cátedras de Morfología Animal y de Introducción a la Biología, Instituto de Investigaciones Biológicas y Tecnológicas (IIByT), Facultad de Ciencias Exactas Físicas y Naturales, CONICET/Universidad Nacional de Córdoba, Córdoba, Argentina
- 4Ministerio de Salud Pública de San Juan, Programa Control de Vectores, San Juan, Argentina
- 5Centro Nacional de Diagnóstico e Investigación en Endemo-Epidemias (CeNDIE) – Administración Nacional de Laboratorios e Institutos de Salud "Dr. Carlos Malbrán" (ANLIS), Buenos Aires, Argentina
- 6Consejo Nacional de Investigaciones Científicas y Técnicas, Buenos Aires, Argentina
Triatoma infestans, the primary vector of Chagas disease in southern South America, is reported as a dimorphic species for flight muscle development and presents a marked phenotypic plasticity in traits related to flight. In triatomines, studying the relationship between flight muscle development and wing variation is important, since it involves flight capacity and dispersion. Therefore, we examined the association between flight muscle dimorphism and morphological wing variation in T. infestans individuals. We hypothesized that if the underdeveloped flight muscle were associated with a decrease in wing developmental stability, canalization and morphological aspect of wings, and with a loss of wing modularity, then wing variation would be associated with flight muscle dimorphism. Wing size and shape were characterized using geometric morphometrics. Patterns of canalization, developmental instability, and modularity were inferred from fluctuating asymmetry analysis of wing shape. Morphological aspects of wings were estimated using maximum wing length and wing area. The results showed that there are differences in wing shape but not in wing size among groups. Canalization and developmental stability were positively associated in individuals with muscle development and negatively associated in individuals with underdeveloped flight muscles. The covariance structure of variation among individuals was different between groups but not for fluctuating asymmetry, both for females and males. Modularity hypothesis related to the wing subdivision into two parts, a stiff part and a membranous one, showed significant association for the variation among individuals and fluctuating asymmetry variation in females with flight muscles. Wing aspect showed that females with flight muscles exhibited more elongated and thinner wings than those without flight muscles. The results obtained in this study reveal an association between flight muscle development and forewing variation in T. infestans. Females with flight muscles have certain differential characteristics in their wings that would be related to an enhanced flight performance.
1. Introduction
Phenotypic variation is influenced by environmental conditions experienced during development (Debat and David, 2001). Among the developmental organismal properties, canalization and developmental stability are ultimately involved in buffering the degree of phenotypic variation (Willmore et al., 2007). Canalization refers to the ability to produce a consistent phenotype despite genetic and environmental perturbations (Zakharov, 1992). As such, canalization buffers phenotypic variation and limits variation between individuals (Willmore et al., 2007). Developmental stability refers to the tendency to follow the same predetermined developmental pathway, under the same conditions, limiting variation within individuals (Willmore et al., 2007). Fluctuating asymmetry (FA) is the most frequently used measure of developmental stability (Klingenberg and McIntyre, 1998; Debat and David, 2001). Phenotypic variation within and among individuals may be increased under stressful situations, i.e., environmental conditions under which homeostasis may be disrupted, and that may reduce the efficacy of the mechanisms underlying canalization and developmental stability (Hoffmann and Hercus, 2000; Lazić et al., 2015).
Integration is another morphological property related to how phenotypic variation is structured (Cheverud, 1996). The coordination into subunits of major morphological units has long been known as modularity (von Dassow and Munro, 2002; Klingenberg, 2008; Klingenberg et al., 2010). Morphological structures are composed of multiple parts that are more or less distinct from one another in terms of function, anatomical structure, and embryological origins. Modularity is present if the integration within a morphological structure is concentrated within a part or region of a structure, the module, but is relatively weak between different modules (Klingenberg, 2014). Modularity concerns the degree of covariation between parts of a structure and determines whether a structure is a single integrated unit or consists of several distinct modules; in the latter case, identifying modules has become an important task in morphometric research (Klingenberg, 2014). Development integration is related to the interactions between the developmental processes that give rise to different traits. Functional integration refers to the relationship between parts involved in a certain biomechanical or physiological process (Klingenberg, 2014). The evolution of morphological structures might be influenced by both the processes involved in the development of the structure and the function of the structures in the interactions of the organism with the environment (Laubichler and Maienschein, 2009).
Wings and flight muscles are crucial for insect flight. They are developmentally complex, functionally integrated systems that are involved in a key ecological and evolutionary life history trait: dispersion (Roff and Fairbairn, 2007; Lu et al., 2020). Flight muscles are the power engine of flying insects, and wings generate the aerodynamic forces required for flight. Insect flight muscle mass must be at least 12 to 16% of body mass for weight-supported flight (Marden, 1987). Thus, insects either make a substantial investment in flight muscles or do not disperse by flight. In nearly every order of insects there are species with flightless individuals or polymorphism in developed muscles (Marden, 1987). In many cases, the development of flight muscle size is related to environmental factors, such as local population density and food availability (Roff, 1986); in other cases, it is due to a genetic polymorphism (Tada et al., 1994). Flight muscle dimorphism can lead to a reduction in wing stability. In insects, it has long been assumed that the costs of building and maintaining flight muscles limit reproductive output (Renault, 2020). For some insects, there is a trade-off between flight capacity and reproduction (Roff and Fairbairn, 2007). In this sense, the relationship between shape and function in insect wings is expected to be under strong selective pressures, considering the behavioral activities such as location of oviposition and foraging sites, courtship, and predator avoidance (Dudley, 2000). Among other parameters, the aspect of the wing (wing length / wing area) indicates flight capacity, with individuals with high values of this parameter being able to fly faster and more extensively than individuals with low wing aspect values (Betts and Wootton, 1988). For example, in butterflies it has been shown that flight performance depends on factors such as flight muscle, wing asymmetry, and wing morphology (Breuker et al., 2010). In insects, wings are considered modular structures that interact during development and coordinately cooperate with a particular function (Klingenberg and Zaklan, 2000; von Dassow and Munro, 2002). In the holometabolous insects Apis mellifera Linnaeus, 1758 (Hymenoptera, Apidae) and Drosophila melanogaster Meigen, 1830 (Diptera, Drosophilidae), wings are known to behave as functionally integrated units; however in the case of Drosophila Fallén, 1823, there are clear delimitations between the anterior and posterior compartments of the wing in terms of cell lineages and gene expression (e.g., Klingenberg and Zaklan, 2000). In the hemimetabolous insect Oncopeltus Stål, 1868 (Heteroptera: Lygaeidae), forewings are composed of a stiff proximal region and a more flexible membranous apex (hemelytra) (Medved et al., 2015). The developmental ontogenetic pattern of wings involves a pool of genes. The first module, under the control of the nub gene, determines the structuring of the stiff proximal region. The second module, corresponding to the flexible membranous apex of the wing, is under the control of the vg gene (Medved et al., 2015). For Triatoma infestans (Klug, 1839) (Hemiptera, Reduviidae, Triatominae, no studies on the modularity and phenotypic integration have been conducted to define the functional modules into which the hemelytra wing can be divided.
Triatoma infestans is the main vector of Trypanosoma cruzi (Chagas 1909) (Kinetoplastida, Trypanosomatidae), the agent of Chagas disease, in the Southern Cone of South America (World Health Organization, 2017) and expresses a strong trend toward domiciliation (Waleckx et al., 2015). In triatomines, flight is the most important dispersal mechanism (Galvão et al., 2001). It is the main colonization and/or recolonization strategy used by T. infestans after vector control interventions (Schofield, 1985; Vazquez-Prokopek et al., 2006). Indeed, flight distances of between 200 m (Schofield et al., 1992) and 1,500 m (Schweigmann et al., 1988) were reported for the species. In triatomines, flight is modulated by various factors such as nutritional status (Lehane et al., 1992; McEwen and Lehane, 1993; Ceballos et al., 2005), reproductive status (McEwen and Lehane, 1994), population density (McEwen et al., 1993) and environmental conditions (Williams and Schofield, 1985; Lehane et al., 1992; Schofield et al., 1992; Schmidt et al., 2022). The species exhibits differences in dispersal behavior between males and females. Males were found to outnumber females in probability of flight initiation (Ceballos et al., 2005; Vazquez-Prokopek et al., 2006; Gürtler et al., 2014). However, female-biased flight dispersal has been revealed using genetic and morphological markers (Marcet et al., 2008; Gaspe et al., 2012; Pérez de Rosas et al., 2013); in addition, flight initiation probability was found to be higher in females than in males (Gurevitz et al., 2006). Triatoma infestans has been reported as dimorphic for flight muscles in natural populations (Gurevitz et al., 2007). The determinants of the lack of muscle development in adults of T. infestans are unknown. Gurevitz et al. (2009) suggested a combination of genetic and environmental factors. For T. infestans, increased levels of FA were found in populations exposed to the environmental stressors pyrethroid and a combination of different host-feeding sources, habitats and/or season/years (Nattero et al., 2015a, 2017, 2021), suggesting that development stability may be unsettled under certain conditions for this species. Similarly, under pyrethroid exposure, canalization becomes less stringent, allowing the expression of genetic variation (Nattero et al., 2021).
Here, we examine if flight muscle development has any effect on wing centroide size (CS) and shape in adult individuals of T. infestans. Wing morphometry might be highly variable within and across populations and a potential target of selection (Schachter-Broide et al., 2004; Gaspe et al., 2012; Nattero et al., 2015b). Based on the quantification of wing CS and shape in female and male of T. infestans through geometric morphometric techniques, we compared individuals with and without developed flight muscles in search of signs of impaired canalization and developmental instability. If the underdeveloped flight muscle is associated with a decrease in wing developmental stability, canalization, and morphological aspect of wings, and a loss of wing modularity, then wing variation will be associated with flight muscle dimorphism. Specifically, we integrated information across hierarchical levels of variation by assessing the following indicators: (1) variation in wing CS and shape among individuals; (2) variation in wing size and shape within individuals, as described by the degree and type of FA, (3) variation across developmental and functional modules, as measured by the level of wing shape integration, and (4) variation in morphological aspect of wings.
2. Materials and methods
2.1. Insects
Adults of T. infestans included in this study were collected from domestic and peridomestic habitats in an urban area contiguous to San Juan city (Rawson department) (31° 39′ 48” S; 68° 27′ 11” W) and from rural areas in Sarmiento department (32° 08′ 46” S, 68° 42′ 56” W), San Juan province, Argentina, during 2018 and 2019. In each habitat, 20 to 30 females and males were collected and taken to the laboratory in Buenos Aires, Argentina, where they were stored in 70% ethyl alcohol.
2.2. Data collection
At the laboratory, adults were dissected by removing the head and pronotum. The thoracic cavity where flight muscles are located was observed under a stereomicroscope (Zeiss SV11, Germany), and flight muscles were classified into three different categories: Type I: developed flight muscles (11–21% of total body mass), completely filled thoracic cavity, and firm consistency and clear development of longitudinal and transverse muscles (Figure 1A). Type II: almost empty thoracic cavities (3–9% of total body mass), except for the small whitish thoracic contents of almost liquid consistency (Figure 1B). Type III: thoracic cavity completely empty, dark brown and without any liquid content (not shown). According to our previous observations, in T. infestans adults collected in the field or under experimentation, type III corresponds to insects that died of starvation and presumably consumed their muscles during the starved period. This situation was described for other insect species (Marden, 1987). Hence, adults that present Type III flight muscles were not included in this study. After the classification of insects according to flight muscles, a total of 78 females (55 and 23 with and without flight muscles, respectively) and 83 males (56 and 27 with and without flight muscles, respectively) were included in this study. Insects, both with and without developed flight muscle, exhibited macropterous forewings. Left and right forewings of the 161 insects were mounted between microscope slides and cover slips, and photographed with a digital camera (Nikon S9900) connected to the stereomicroscope using a 6× magnification. All images included a reference scale.
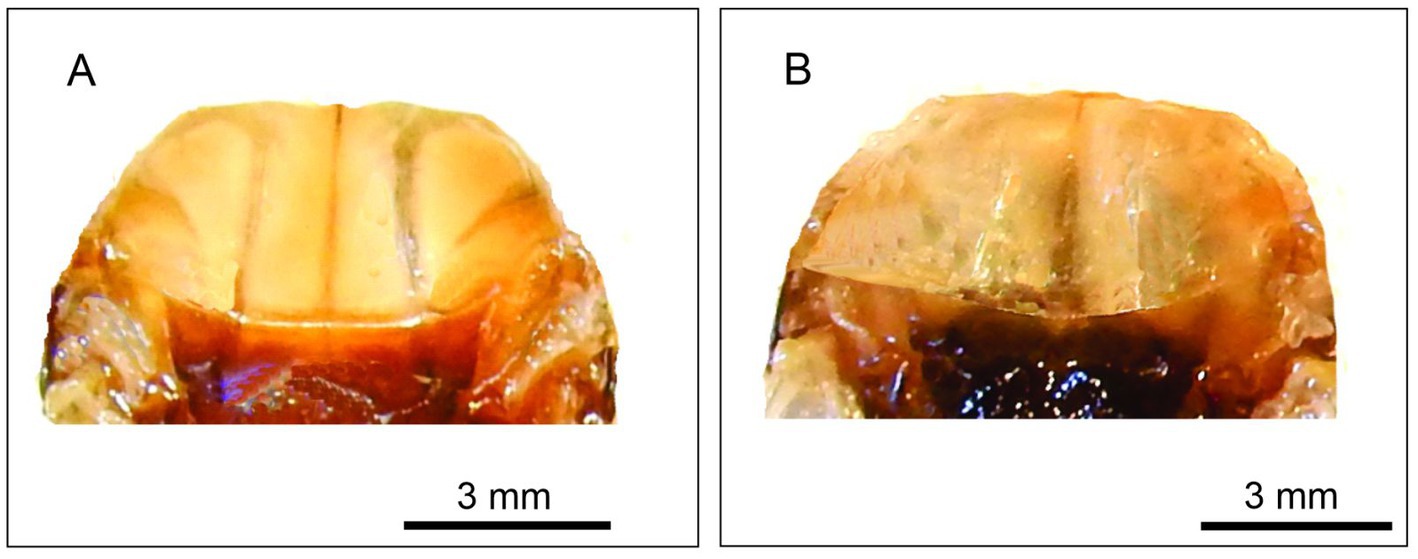
Figure 1. Macroscopic appearance of the thoracic contents of T. infestans individuals: one with flight muscles (A) and the other without flight muscles (B). For the obsevation of flight muscles, head and pronotum were removed.
To quantify variation in wing CS and shape, we used landmark-based geometric morphometrics (Bookstein, 1997). We collected 10 type-I landmarks positioned at vein intersections as described by Schachter-Broide et al. (2004) using the free software tpsDig2 (version 2.31) (Rohlf, 2017). Both right and left wings were digitized twice to estimate measurement error (ME), which is of critical importance in the analysis of FA (Palmer, 1994). We used a generalized full Procrustes fit to superimpose all configurations and remove the effects of position, orientation, and size (Rohlf and Slice, 1990). To analyze matching symmetry, this procedure superimposes the left and right wings, thus allowing the separation of symmetric and asymmetric variation components (Mardia et al., 2000; Klingenberg et al., 2002). We computed CS (i.e., the square root of the sum of squared distances from each landmark to the centroid of the configuration) as a measure of wing size (Dryden and Mardia, 1998). Allometric relationships between wing shape and CS for females and males with and without developed flight muscles were checked with a multivariate regression of Procrustes coordinates on CS. Regression results confirmed significant allometric effects for all groups (p < 0.001 in all cases), but with relatively small allometric effects (less than 7% in all cases).
In addition, the maximum wing length and the total wing area were measured for all sampled individuals. These measurements were taken using the ImageJ software, version 1.52 (https://imagej.nih.gov/ij/).
2.3. Comparisons between individuals with and without flight muscles
A principal component analysis (PCA), based on the covariance matrix of landmark coordinates after the Procrustes fit, was performed to examine dimensionality of shape variation and the global shape data for the complete data set. We compared CS between females and males with and without developed flight muscles using ANOVA tests.
Canalization and developmental stability, as estimators of developmental buffering, were examined in each of the four groups (females and males with and without flight muscles) considering the amount of individual and FA variation in wing CS and shape. We used two-way ANOVAs for size and Procrustes ANOVAs for shape (Klingenberg and McIntyre, 1998), considering individual variation, side, interaction between individual and side as effects, and the ME. The effect of individuals represents the variation among individuals corrected for any asymmetry effects and is considered the symmetric component of shape variation. The side represents the variation due to differences between the right and left wing and is useful to evaluate directional asymmetry. Finally, the interaction between individual and side captures the variability of right–left differences among individuals (FA) and is interpreted as the asymmetric component of shape variation (Klingenberg et al., 2002). The mean square (MS) related to the interaction (individual × side) was used to compute the FA10 index as FA10 = (MS interaction–MSME)/2, following Palmer (1994). This index accounts for measurement error and provides a reliable estimation of FA. Variance estimates were then compared among groups using standard F-tests. To determine the relationship between canalization and developmental stability, the correlation between symmetric and asymmetric variance covariance (VCV) matrices was evaluated within each group for both females and males. Furthermore, we tested whether symmetric and asymmetric wing shape variation components were differently structured in the two groups for each sex. For this purpose, we examined the correlation between VCV matrices examining matrix association across groups. Afterwards, we examined if these differences were reflected in wing shape. We first examined whether individuals without flight muscles exhibited a higher total wing shape variance than individuals with flight muscles. We calculated shape variance as the trace of the within-population-type VCV matrix (Zelditch et al., 2004) and then compared variances across groups within each sex with F-tests.
To examine whether different areas of the landmark configuration correspond to different developmental and/or functional modules, we conducted a modularity analysis of wing shape configuration. We first formulated a developmental hypothesis (hypothesis 1) stating that the landmarks of the stiff proximal region and the membranous apex are two separate modules. This hypothesis was formulated according to the patterns of expression of the genes nub and vg, which specify these two parts of the wing in Hemiptera (Medved et al., 2015; Figure 2A). For Hemiptera, no previous studies have defined the functional modules into which the hemelytra wing can be divided. Therefore, the different modularity hypotheses proposed here are exploratory hypotheses to test functional modularity. We formulated three additional hypotheses of modularity (hypotheses 2, 3 and 4): (1) proximal, central and distal wing compartments are distinct modules (Figure 2B), (2) the anterior and posterior compartments of the wing are distinct modules (Figure 2C), and (3) the anterior, central and posterior compartments of the wing form separate modules (Figure 2D). The modularity hypotheses were evaluated by computing the strength of covariation between the hypothetical modules with alternative partitions of landmarks into subsets of the corresponding sizes (Klingenberg, 2009). If a hypothesis of modularity were correct, the covariation between those subsets of landmarks should be weaker than the covariation between different partitions of the landmarks into subsets (Klingenberg, 2009). We computed the RV coefficient (Escoufier, 1973), which is a multivariate measure of covariation between two sets of landmarks (hypotheses 1 and 3) and the multiset RV coefficient (Klingenberg, 2009) when three compartments were proposed (hypotheses 2 and 4). To test the modularity hypotheses, we used a single Procrustes fit for all landmarks jointly and then examined the covariance of subsets of landmarks within the overall configuration (simultaneous-fit). Spatially contiguous partitions were considered and defined with an adjacency graph (Supplementary material 1): a partition of landmarks is spatially contiguous if all resulting subsets of landmarks are connected through the edges of the adjacency graph (Klingenberg, 2009). All morphometric analyses were performed with the free software MorphoJ (version 1.07a) (Klingenberg, 2011).
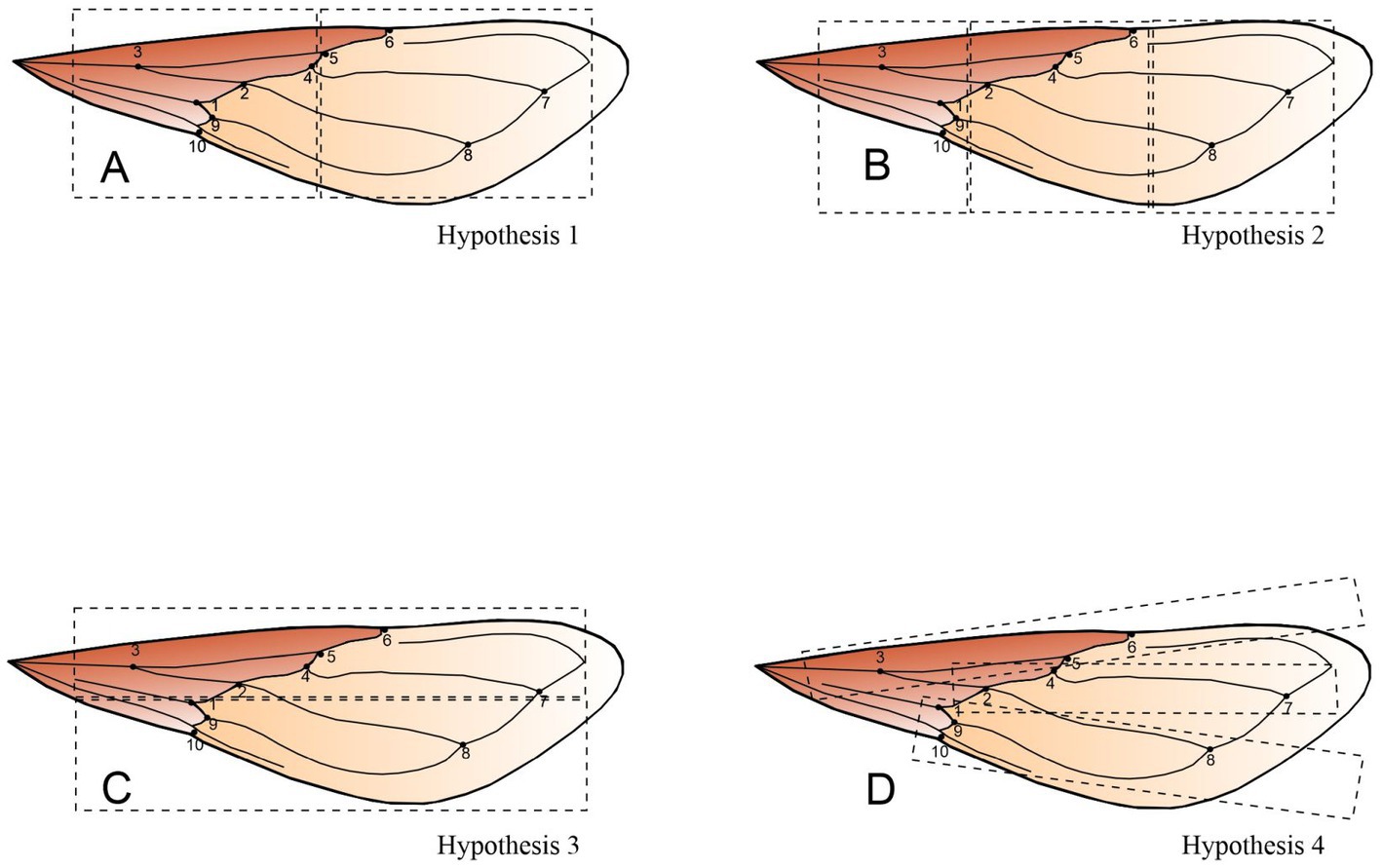
Figure 2. Hypotheses of modularity in T. infestans wing. (A) Hypothesis 1 contrasts the landmarks of the stiff proximal region and the membranous distal region as two separate modules. (B) In hypothesis 2, the proximal, central and distal compartments are distinct modules. (C) In hypothesis 3, the anterior and posterior compartments of the wing are contrasted. (D) Hypothesis 4 contrasts an anterior, central and posterior compartment as three different modules. The dark brown and light brown portions correspond to the stiff and the membranous regions of the wing, respectively.
Finally, we compared the morphological aspect of wings among females and males with and without developed flight muscles. For this, the estimator known as wing aspect ratio: 4 × (wing length)2/wing area (Betts and Wootton, 1988) was compared with one-way ANOVAs tests among groups.
3. Results
PCA on wing shape showed that individuals clustered according to the group to which they belonged (Figure 3). PC2 exhibited a common variation of wing shape in the four groups. ANOVA results performed for wing CS showed that for both females and males, wing size did not vary significantly between individuals with and without developed flight muscles (F(77, 1) = 0.73; p = 0.3944; F(82, 1) = 1.22; p = 0.2735) for females and males, respectively.
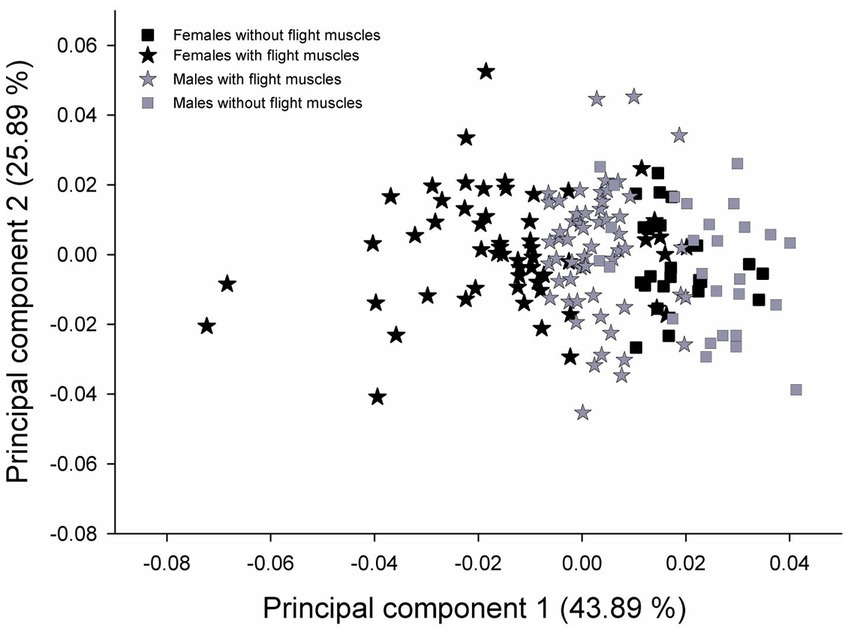
Figure 3. Wing shape variation associated with the two first axes of a PCA performed on the whole dataset for female and male T. infestans. Values in parenthesis represent the percentage of explanation of the variation for each axis. Black symbols: females. Gray symbols: males.
Both females and males with flight muscles showed a significant occurrence of individual and FA variation in wing CS and shape (Table 1). The results of the two-way and Procrustes ANOVAs for females and males without developed flight muscles showed a significant occurrence of wing CS and shape FA; however, individual variation was not significant (Table 1). Mean FA of both wing CS and shape was about four times greater in females and males without developed flight muscles than in those with flight muscles (F-test, p = 0.0014; Figures 4A,B). Symmetric size variation accounted for 85.51 and 89.58% of total size variation for females and males with flight muscles, respectively. The asymmetric size component accounted for 14.49 and 10.42% for females and males, respectively. For individuals without developed flight muscles, of the total size variation, symmetric variation accounted for only 20.05 and 20.80% for females and males, respectively, whereas the asymmetric component accounted for 79.95 and 79.20% for females and males, respectively. Shape variation between females with and without flight muscles (symmetric and asymmetric shape components), as visualized by the PCA corresponding to the individual and individual x side effects, revealed differences among groups. Variability along PC1 for females without flight muscles was more expanded along the whole wing compared with the variability that exhibited the individuals with flight muscles, which was concentrated mainly in the stiff proximal part of the wing (Figure 5). For females without flight muscles, variability was explained by changes in multiple regions of the wing rather than changes in a single part (Figure 5). For the asymmetric shape component, variability of PC1 for females without flight muscles was more expanded in multiple regions of the wing compared with females with flight muscles; for the latter, variability was concentrated mainly in the stiff proximal part of the wing (Figure 5). For males, individuals without flight muscles exhibited changes in multiple regions of the wing compared to males with flight muscles for the PC1 corresponding to the symmetric shape components (Figure 6). For asymmetric components, the shape changes for the first three PCs, for both groups, consisted of shifts and deformations of the wing in multiple regions (Figure 6).
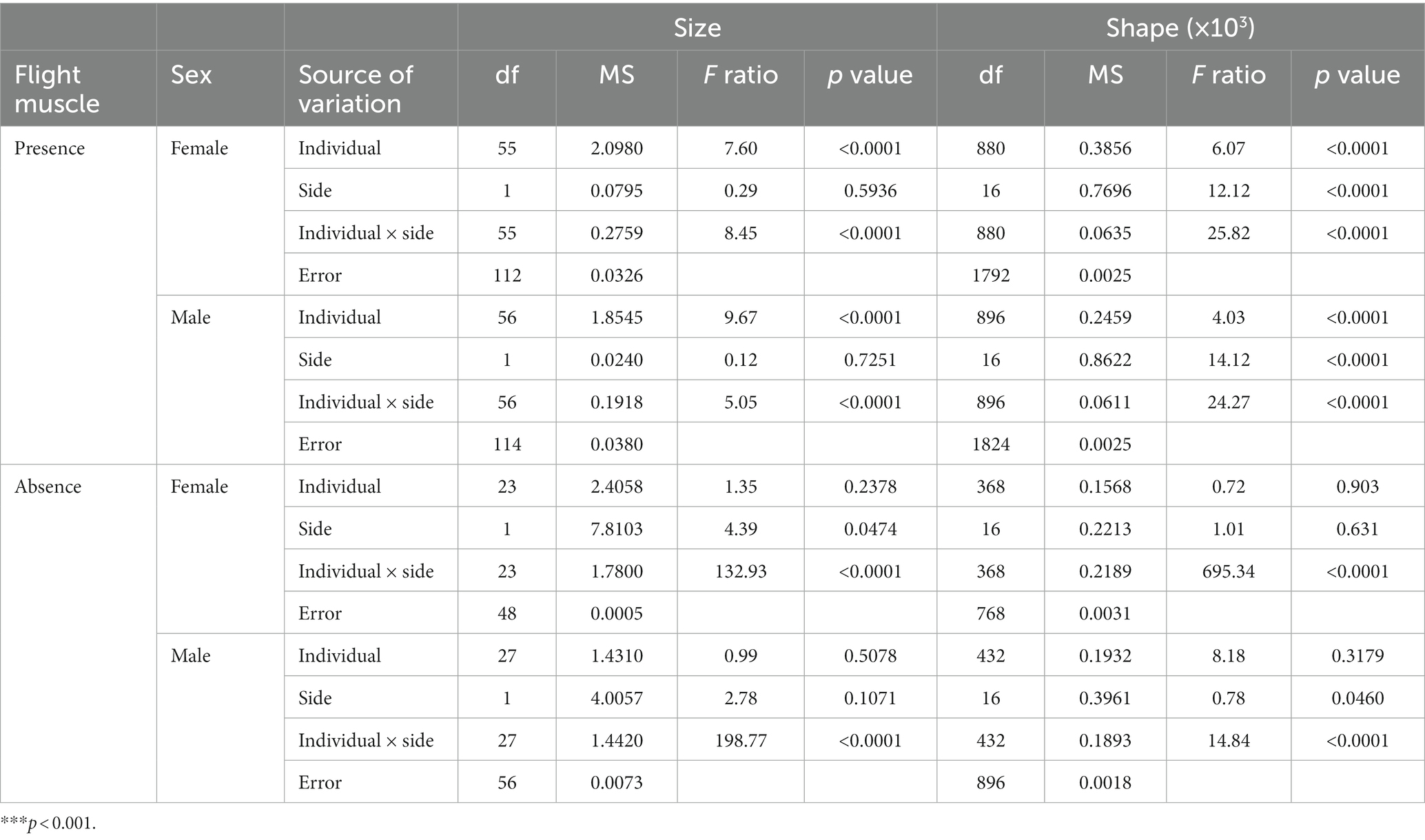
Table 1. Two-way mixed and Procrustes ANOVA for the partitioning of directional mean square (MS) (MS side) (×103 for shape) and non-directional MS (MS side × individual) (×103 for shape) on the deviations from the mean landmark configuration of females and males of T. infestans wings.
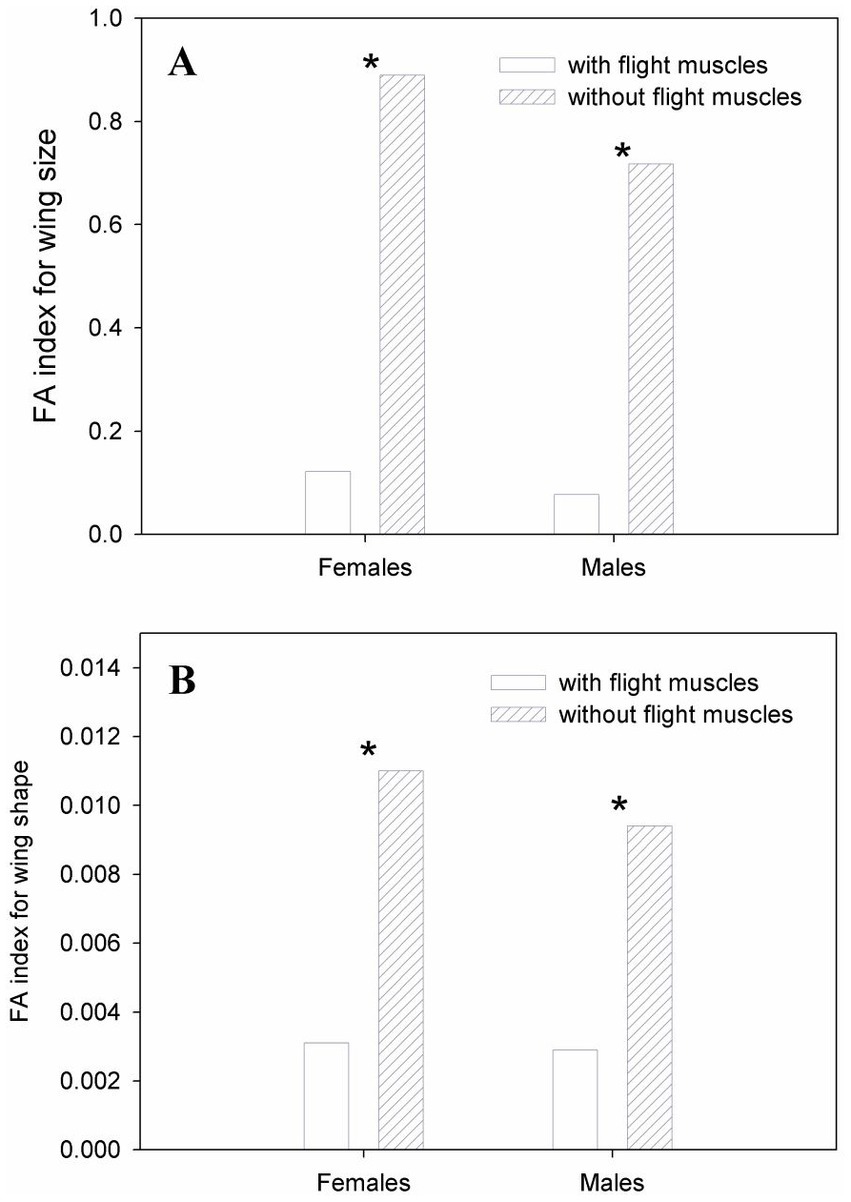
Figure 4. Fluctuating asymmetry variation in wing size (A) and shape (B) in T. infestans females and males. Stars indicate significant differences between individuals with developed flight muscles and without developed flight muscles for females or males at *p < 0.001 (F-test).
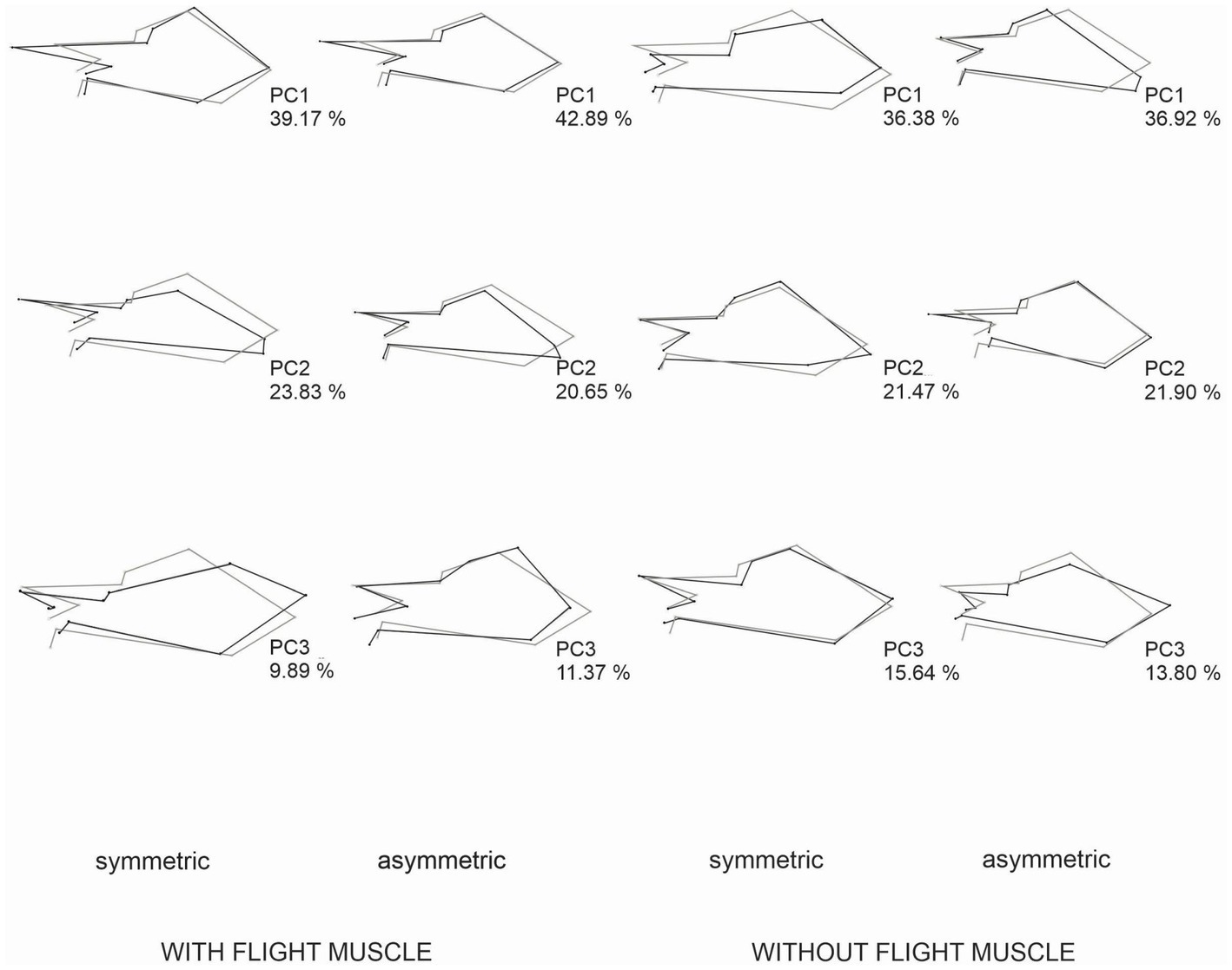
Figure 5. Patterns of shape changes in females with developed flight muscles and without developed flight muscles associated with a PCA of the Procrustes ANOVA effects for individual (symmetric shape component) and individual × side (fluctuating asymmetry). The figure shows shape changes associated with the first three PCs from an average shape (gray outlines) to a target configuration (black outline) along the respective PC. The percentages indicate the portion of shape variation explained by each PC. The scale factor in these graphs is the magnitude of the shape change and corresponds to 0.15 units of the Procrustes distance. For PC1, for both symmetric and asymmetric patterns of variation, shape changes were greater in females without muscle flight. For PC2 and PC3, for both symmetric and asymmetric components, shape change variation was greater in females with flight muscles.
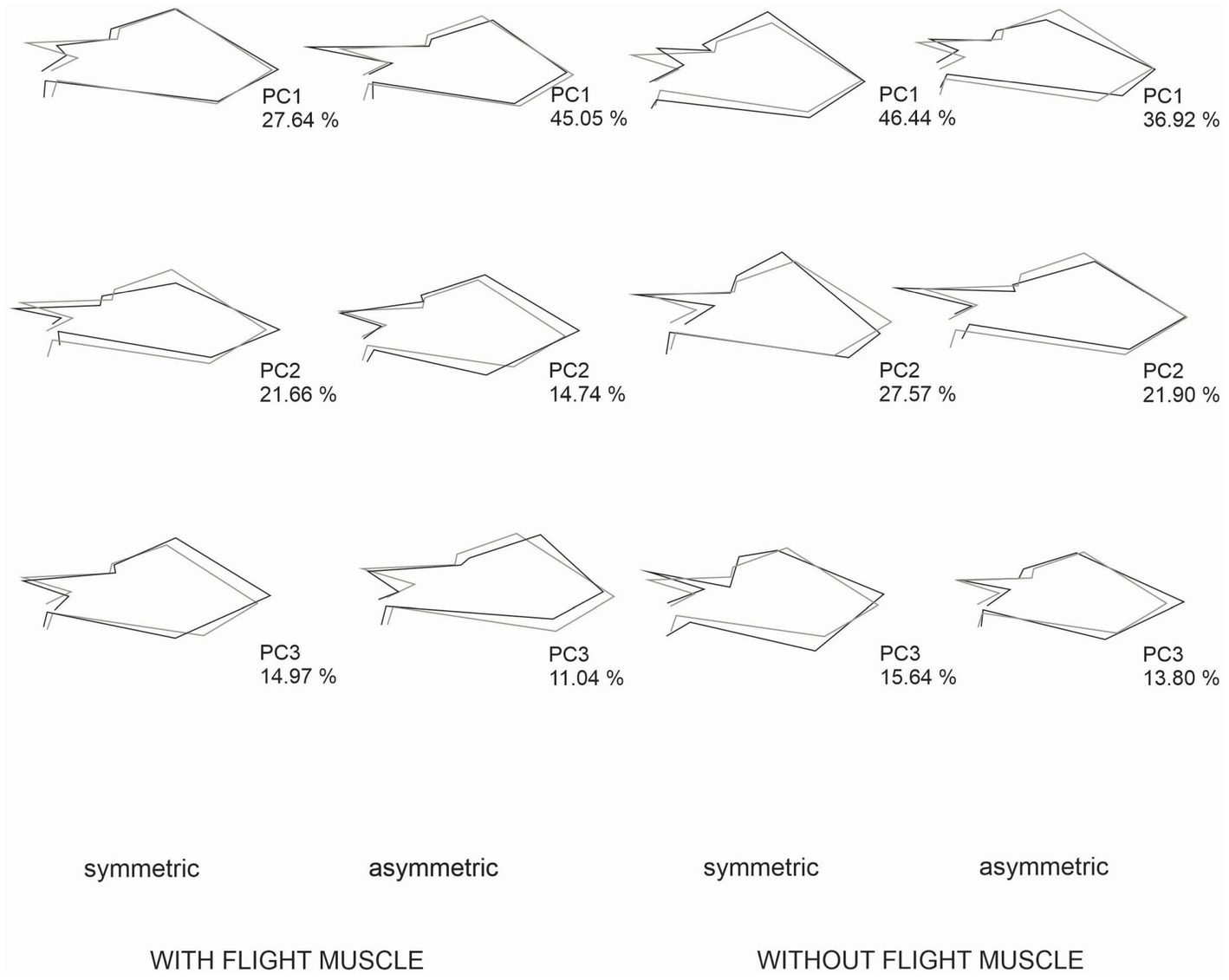
Figure 6. Patterns of shape changes for males with developed flight muscles and without developed flight muscles associated with a PCA of the Procrustes ANOVA effects for individual (symmetric shape component) and individual × side (fluctuating asymmetry). The figure shows shape changes associated with the first three PCs from an average shape (gray outlines) to a target configuration (black outline) along the respective PC. The percentages indicate the portion of shape variation explained by each PC. The scale factor in these graphs is the magnitude of the shape change as 0.15 units of the Procrustes distance. Both symmetric and asymmetric pattern of variation for the three axes involved multiple regions of the wing, both from the stiff proximal and the membranous part or the Hemelytron wing. For PC1, for both symmetric and asymmetric components, shape changes were greater in males without flight muscles. For PC2 and PC3, for both symmetric and asymmetric components, shape change variation involved both males with and without flight muscles.
The correlation matrix between symmetric and asymmetric components showed a positive and significant association for individuals with developed flight muscles (r = 0.935, p = 0.0001; r = 0.825, p = 0.0009 for females and males, respectively) and a negative and non-significant association for individuals without flight muscles (r = −0.803, p = 0.9805; r = −0.255, p = 0.999 for females and males, respectively). Patterns of symmetric shape variation for females were not similar between groups, as indicated by the correlation between the corresponding VCV matrices (r = −0.518, p = 0.983), whereas the pattern of asymmetric shape variation showed statistical significance of the correlation matrix (r = 0.725, p = 0.005). For males, shape variation showed the same tendency as for females, i.e., a non-significant correlation matrix for symmetric components (r = 0.102, p = 0.146) and a significant correlation matrix for asymmetric components (r = 0.708, p = 0.001).
Females without developed flight muscles exhibited significantly higher total wing shape variance than individuals with developed flight muscles (Vwithout FM/Vwith FM = 1.2354, p = 0.021). For males, individuals without developed flight muscles exhibited similar total wing shape variance to that of individuals with developed flight muscles (Vwithout FM/Vwith FM = 1.2071, p = 0.683).
The RV or RVM coefficients obtained in the modularity analysis of wing shape indicated a low-to-high degree of covariation between the different modules of the wing in the subsets of landmarks proposed for the different hypotheses (Table 2). Considering the different modularity hypotheses, covariance for FA was weaker than covariance among individuals (Table 2). The only hypotheses that showed significant results were those that defined modules tangentially to the major axis of the wing (i.e., hypotheses 1 and 2). Only females exhibited significant RV or RVM coefficients (Table 2). Females with developed flight muscles exhibited significant coefficients for hypotheses 1 and 2, both for variation among individuals and FA (Table 2). Females without developed flight muscles exhibited significant coefficients only for hypothesis 1 and for variation among individuals (Table 2).
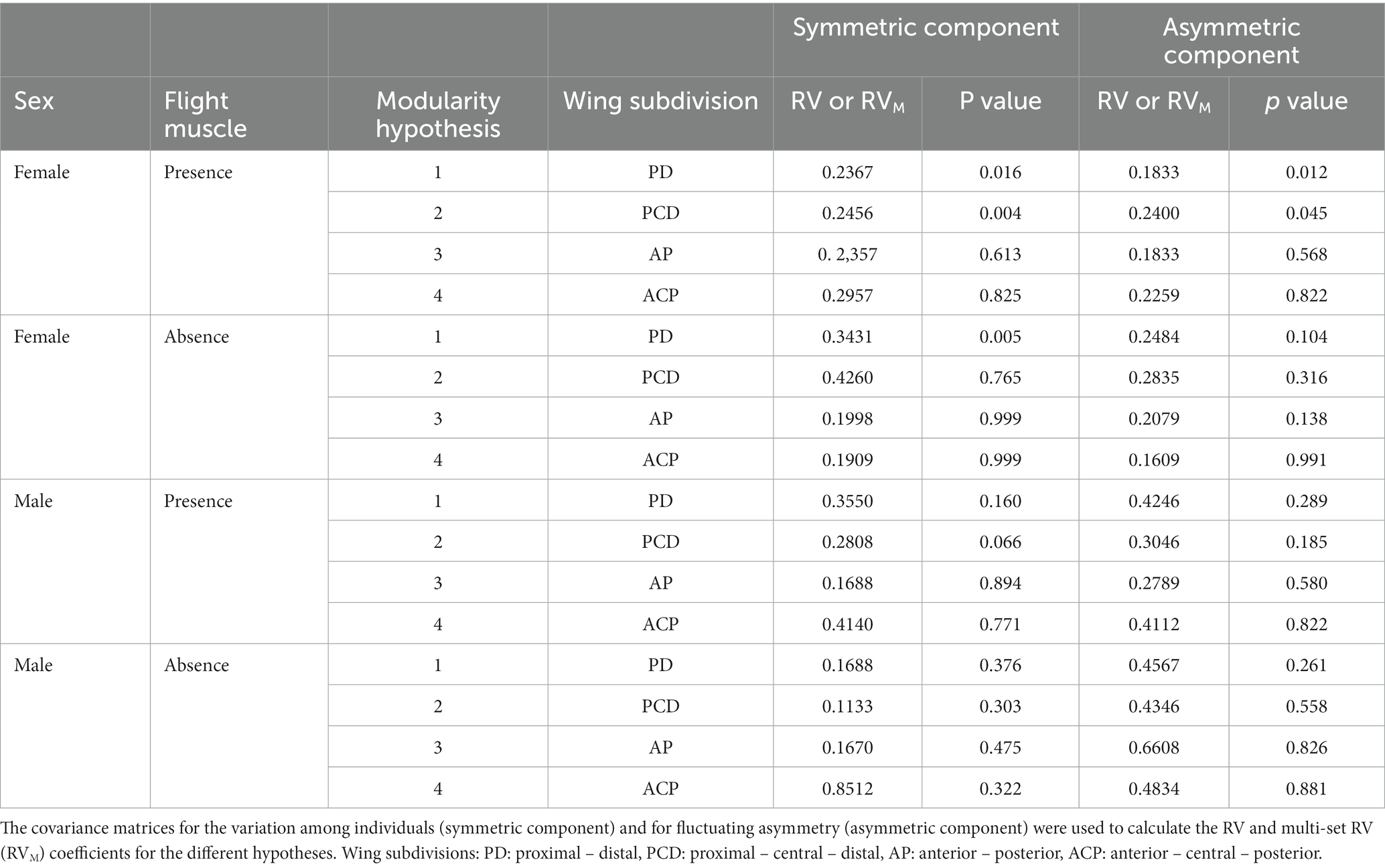
Table 2. Evaluation of the hypotheses of modularity in different subdivisions of the wings (see Figure 2) of T. infestans females and males with and without developed flight muscles.
The comparison of the wing aspect ratio between females with and without developed flight muscles showed a significant difference (F(77, 1) = 3.81; p = 0.001), with females with developed flight muscles exhibiting a bigger wing aspect ratio. For males, no significant differences were observed (F(82, 1) = 0.81; p = 0.428).
4. Discussion
The results obtained in this study reveal an association between flight muscle development and forewing variation in T. infestans. Although there was variation in wing shape, no differences were observed in wing CS among females and males with and without developed flight muscles, implying that flight muscle development is not accompanied with wing CS variation. Moreover, hindwing size did not vary among females and males with and without developed flight muscles (Nattero et al., unpublished data). In some insects, flight muscle dimorphism is due to environmental factors such as food availability and population density (Johnson, 1969; Roff, 1986; Roff, 1990). In other cases, flight polymorphisms are caused by simple Mendelian genetic differences, as in the beetle Heptophylla picea Motschulsky, 1857 (Coleoptera: Scarabaeidae) (Tada et al., 1994). For T. infestans, differences in temperature, photoperiod, and feeding frequency in fourth or fifth nymphs, reared to adults under different laboratory conditions, were not crucial for flight muscle morph development (Gurevitz et al., 2009). These observations do not exclude the possibility that sustained extreme conditions of temperature, photoperiod, and feeding frequency during nymph ontogeny may be determinants of the lack of muscles in adults. Moreover, other environmental factors, such as population density, could be considered determinants of this phenomenon for this species. In triatomines, increased population density implies concomitant decreases in density-dependent blood-feeding success and a higher nutritional stress. Gurevitz et al. (2009) reported that flight muscles in T. infestans were detectable at 4 days after emergence and were completely developed within 4–15 days after emergence. Moreover, the development of flight muscles is an irreversible process determined before or during final ecdysis (Gurevitz et al., 2009). Flight muscle dimorphism in T. infestans was first reported in four neighboring villages in the department of Figueroa, Santiago del Estero province, Argentina (Gurevitz et al., 2007). Later, another study found flight muscle dimorphism in Pampa del Indio, department of San Martín, Chaco province, Argentina (Gurevitz et al., 2009). All these findings suggested a regional phenomenon. Beyond the determinants of flight muscle dimorphism for T. infestans, our analyses showed that wing shape is a highly variable structure, with variation within and among individuals.
4.1. Pattern of variation of developmental instability and canalization
The integrated assessment of various approaches that gaged the contribution of the two measured components of developmental homeostasis, i.e., canalization and developmental stability, revealed that adults without developed flight muscles suffer impaired wing development. The common mechanism underlying the variation of canalization and developmental stability was reduced in males and females without flight muscles. These results evidence that a decrease in developmental buffering can be expected in the lack of muscle development, probably associated with reduced wing stability. In flight muscle polymorphic species, female flightless morphs reach sexual maturity more rapidly and attain higher fecundity compared with females with flight muscles (Johnson, 1969; Roff, 1986; Roff, 1990). For males, reproduction was also found to be enhanced in flightless morphs (Crnokrak and Roff, 1995). These evidences suggest that individuals investing more in reproduction would have experienced reduction in wing stability and individuals that migrate by flight would have invested in a flight migratory syndrome that involved traits, such as morphology, hormone titers, development time and growth rate, distribution of energy stores, flight propensity, and age-specific reproduction (Roff and Fairbairn, 2007).
Our findings indicate that the developmental stability process was affected in individuals without developed flight muscles, with very high increases in fluctuating asymmetry both in shape and size being recorded in comparison with individuals with developed flight muscles. However, for individuals without flight muscles, the canalization process remained active, preventing the final morphology of the wings from being highly polymorphic and maintaining ontogenetic patterns similar to those of individuals that developed under more favorable conditions. For T. infestans, wing FA associated with different host-feeding sources and habitats determines different patterns and amount of FA (Nattero et al., 2015a,b). Another study, in which T. infestans females and males were collected before and 4 months after a community-wide pyrethroid spraying campaign, showed consistently small levels of FA for those individuals collected 4 months after spraying (Nattero et al., 2019). This pattern was suggested to be related, among other possible causes, to the lower triatomine densities after insecticide spraying and the concomitant increase in feeding success of these insects.
4.2. Relationship between canalization and developmental stability
We found a reduction of a common mechanism underlying the variation of canalization and developmental stability, which was confirmed by the lack of significant correlations between the VCV matrices corresponding to FA and among-individual variation for females and males without developed flight muscles. For T. infestans, the study of canalization and developmental stability related to the effects of a sublethal application of a pyrethroid insecticide suggested that treatment affected only individual variation matrices, i.e., insecticide treatment impaired environmental canalization. These results suggest differences in the processes involved in the buffering of variation within and between individuals (Nattero et al., 2021). Breno et al. (2011) compared different growth trajectories and reported a similar result, i.e., no common mechanism between canalization and developmental stability of the skull of Mastomys natalensis Smith, 1834 (Rodentia, Muridae). Moreover, other studies suggest the existence of different molecular mechanisms acting on canalization and developmental stability for different traits and influenced by different stressors (e.g., Debat et al., 2006; Takahashi et al., 2010).
4.3. Covariation of symmetric and asymmetric components between females and males
Our results showed an alteration of the patterns of developmental regulators, since covariation of symmetric and asymmetric components between females and males with and without developed flight muscles revealed incongruent symmetric patterns for wing shape variation but a congruent pattern for asymmetric components. This finding may support the hypothesis that canalization and developmental stability are not necessarily related and that variation among individuals is not necessarily regulated by the same mechanisms. Debat et al. (2009) reported a similar result for D. melanogaster, and showed that the effect of temperature affected among-individual wing variation but not FA. The amount of symmetric and asymmetric variation in individuals with and without developed muscle flight varied greatly. These results suggest that variation spontaneously produced by the developmental system of the wing is higher than the variation among individuals that lack flight muscles. Moreover, shape variance was higher for females and males that lack flight muscles than for those that have flight muscles, although variance was statistically significant only for females. These results might support the idea that those individuals that lack developed flight muscles exhibited a disruption in homeostasis and an increase in wing shape variation. When environmental conditions are suboptimal, buffering mechanisms can be challenged, increasing phenotypic variance (Willmore et al., 2007). All this evidence contributes to the hypothesis that there are multiple signs of increased phenotypic variation related to the lack of flight muscle. T. infestans individuals exposed to stressful environments during developmental ontogeny showed higher phenotypic variation than those developed under more stable conditions (Nattero et al., 2021).
4.4. Pattern of wing shape variation
Visualization of wing shape patterns of variation in the comparisons of groups reveals that there is no consistency in shape variation across groups or between the patterns for symmetric and asymmetric components. Both stiff proximal and membranous distal regions of the hemelytra wing exhibited high variation. Indeed, both intra- and inter-individual shape differences include deformations of the wing areas corresponding to elongated and short wings and to thin and wide wings. To our knowledge, this is the first study that used these approaches to explore the variation of hemelytra wing in a Hemiptera. However, it is difficult to assess if this is a common feature of the wings of Hemiptera, Triatominae or T. infestans.
4.5. Modularity and morphological aspect of the wings
The lack of association between symmetric and asymmetric components, at least for those individuals without flight muscle, suggests a different pattern of morphological integration throughout the wing. This result is consistent with what is expected under the developmental hypothesis proposed, i.e., wing subdivision in proximal and distal portions, and a functional hypothesis, i.e., proximal, central and distal portions; both hypotheses are in agreement with the stiff and membranous part of the hemelytra wing. Interestingly, these hypotheses are significant only for females with and without developed flight muscles considering the variation among individuals and only for females with developed flight muscles. These modularity hypotheses were significant for FA, which implies that the pattern of covariation considering the wing subdivision for FA might derive from spontaneous variation in developmental processes (Klingenberg, 2008). The wing subdivision that showed significant covariation was tissue-bound, and coincides with two different genes that structure these portions of the wing in Hemiptera (Medved et al., 2015). The evolution of morphological structures is influenced by both the processes involved in the development of the structure and its function in the organism’s interactions with the environment (Breuker et al., 2006; Laubichler and Maienschein, 2009). The genetic and developmental structure would evolve to match functional modules (Cheverud, 1984; Wagner and Altenberg, 1996). Recent results considering the functional roles of wing structural components in dragonflies, which belong to the order Odonata, suggest the need for a balance between flexibility and stiffness in the wings (Rajabi and Gorb, 2020). The flexibility of wings should be kept within a suitable range. Our results of the morphological aspect of wings showed that females with developed flight muscles exhibited bigger wings, with an aspect more elongated and thin than that of females without flight muscles. For Triatoma guasayana Wygodzinsky and Abalos, 1949, depending on the period of the year, the individuals present elongated and thin wings, which were suggested to allow sustained long-duration flights, and short and wide wings, which would be related to short flights (Fiad et al., 2022). The study of wing modularity and morphological aspect provides coincident results in that females with developed flight muscles have certain differential characteristics in their wings that suggest an enhanced flight performance.
4.6. Origin of the analyzed insects
The origin of the insects studied in this work is heterogeneous, with adults collected in different habitats from urban and rural contexts. In the rural context, peridomestic habitats such as goat corrals, pig corrals and chicken coops usually displayed extreme and widely variable conditions of temperature, relative humidity, and insolation, which may act as environmental stressors for the developing bugs (Vázquez-Prokopec et al., 2002). Furthermore, domestic habitats had near-optimum temperatures for T. infestans and more capacity to damp variations in external conditions than other frequently infested peridomestic ecotopes (Vázquez-Prokopec et al., 2002). For T. dimidiata (Latreille, 1811), another triatomine species, the comparison of urban and rural insects showed evidence of adaptation to urban areas for morpho-functional related traits (Montes de Oca-Aguilar et al., 2022). For T. infestans the effect of habitat had been demonstrated for different traits such us wing, head and antennal phenotype (Schachter-Broide et al., 2004; Hernández et al., 2011). Body size and sexual dimorphism had also been demonstrated to decrease in domestic populations of this species (Dujardin et al., 1997, 1999); these observations suggest that peridomestic triatomine populations can be differentiated from domestic ones. Also, adults collected in distinct ecotopes differed in the amount and pattern of FA (Nattero et al., 2015). The proportion of the individuals without developed flight muscle collected in the different habitats of urban and rural context varied greatly across habitats, with goat corral and chicken coops being those that exhibited the greatest proportion of these insects (Nattero et al., unpublished data). However, despite the heterogeneity of the insect collection sites, we observed the same expression of the pattern of developmental regulators and morphological wing aspect between individuals with and without flight muscles in T. infestans.
Data availability statement
The raw data supporting the conclusions of this article will be made available by the authors, without undue reservation.
Author contributions
JN, RP, and ALC-d-l-F conceived and designed the experiments. FC collected the insects. JN and FF analyzed the data. JN, RP, FF, FC, and ALC-d-l-F wrote the manuscript. All authors contributed to the article and approved the submitted version.
Funding
This study was supported by grants from CONICET (PIP N°11220110101102) and Agencia Nacional de Promoción Científica y Tecnológica (PICT N° 2018–3200, PICT N° 2019–1884 and PICT-2020-SERIE A-1975).
Acknowledgments
The authors especially thank the members of the Programa Control de Vectores de San Juan for their collaboration and interest in this work. The authors thank Vincent Debat for his valuable comments and suggestions on the manuscript. JN, RP, and ALC-d-l-F are members of the CONICET Researcher’s Career. FF is a doctoral fellow of CONICET.
Conflict of interest
The authors declare that the research was conducted in the absence of any commercial or financial relationships that could be construed as a potential conflict of interest.
Publisher’s note
All claims expressed in this article are solely those of the authors and do not necessarily represent those of their affiliated organizations, or those of the publisher, the editors and the reviewers. Any product that may be evaluated in this article, or claim that may be made by its manufacturer, is not guaranteed or endorsed by the publisher.
Supplementary material
The Supplementary material for this article can be found online at: https://www.frontiersin.org/articles/10.3389/fevo.2023.1211219/full#supplementary-material
References
Betts, C. R., and Wootton, R. J. (1988). Wing shape and flight behaviour in butterflies (Lepidoptera: Papilionoidea and Hesperioidea): a preliminary analysis. J. Exp. Biol. 138, 271–288. doi: 10.1242/jeb.138.1.271
Bookstein, F. L. (1997). Landmark methods for forms without landmarks: localizing group differences in outline shape. Med. Image Anal. 1, 225–243. doi: 10.1016/s1361-8415(97)85012-8
Breno, M., Leirs, H., and Van Dongen, S. (2011). No relationship between canalization and developmental stability of the skull in a natural population of Mastomys natalensis (Rodentia: Muridae). Biol. J. Linnean Soc. 104, 207–216. doi: 10.1111/j.1095-8312.2011.01702.x
Breuker, C. J., Gibbs, M., Van Dongen, S., Merckx, T., and Van Dyck, H. (2010). “The use of geometric morphometrics in studying butterfly wings in an evolutionary ecological context” in Morphometrics for Nonmorphometricians. ed. A. Elewa (Berlin: Springer), 271–287.
Breuker, C. J., Patterson, J. S., and Klingenberg, C. P. (2006). A single basis for developmental buffering of Drosophila wing shape. PLoS One 1:e7. doi: 10.1371/journal.pone.0000007
Ceballos, L. A., Vázquez-Prokopec, G. M., Cecere, M. C., Marcet, P. L., and Gürtler, R. E. (2005). Feeding rates, nutritional status and flight dispersal potential of peridomestic populations of Triatoma infestans in rural northwestern Argentina. Acta Trop. 95, 149–159. doi: 10.1016/j.actatropica.2005.05.010
Cheverud, J. M. (1984). Quantitative genetics and developmental constraints on evolution by selection. J. Theor. Biol. 110, 155–171. doi: 10.1016/S0022-5193(84)80050-8
Cheverud, J. M. (1996). Developmental integration and the evolution of pleiotropy. Am. Zool. 36, 44–50. doi: 10.1093/icb/36.1.44
Crnokrak, P., and Roff, D. A. (1995). The genetic basis of the trade-off between calling and wing morph in males of the cricket Gryllus firmusdoi. Evolution 52, 1111–1118. doi: 10.1111/j.1558-5646.1998.tb01838.x
Debat, V., and David, P. (2001). Mapping phenotypes: canalization, plasticity and developmental stability. Trends Ecol. Evol. 16, 555–561. doi: 10.1016/S0169-5347(01)02266-2
Debat, V., Debelle, A., and Dworkin, I. (2009). Plasticity, canalization and developmental stability of the Drosophila wing: joint effects of mutations and developmental temperature. Evolution 63, 2864–2876. doi: 10.1111/j.1558-5646.2009.00774.x
Debat, V., Milton, C. C., Rutherford, S. L., Klingenberg, C. P., and Hoffmann, A. A. (2006). Hsp90 and the quantitative variation of the shape of Drosophila wing. Evolution 60, 2529–2538. doi: 10.1111/j.0014-3820.2006.tb01887.x
Dudley, R. (2000). The biomechanics of insect flight: Form, function, evolution. Princeton: Princeton University Press.
Dujardin, J. P., Bermudez, H., Casini, C., Schofield, C. J., and Tibayrenc, M. (1997). Metric differences between sylvatic and domestic Triatoma infestans (Heteroptera: Reduviidae) in Bolivia. J. Med. Entomol. 34, 544–551. doi: 10.1093/jmedent/34.5.544
Dujardin, J. P., Panzera, P., and Schofield, C. J. (1999). Triatominae as a model of morphological plasticity under ecological pressure. Mem. Inst. Oswaldo Cruz 94, 223–228. doi: 10.1590/S0074-02761999000700036
Escoufier, Y. (1973). Le traitement des variables vectorielles. Biometrics 29, 751–760. doi: 10.2307/2529140
Fiad, F. G., Cardozo, M., Rodríguez, C. S., Hernández, M. L., Crocco, L. B., and Gorla, D. E. (2022). Ecomorphological variation of the Triatoma guasayana wing shape in semi-arid Chaco region. Acta Trop. 232:106488. doi: 10.1016/j.actatropica.2022.106488
Galvão, C., Da Silva Rocha, D., Jurberg, J., and Carcavallo, R. (2001). Início da atividade de vôo en Triatoma infestans (Klug 1834) e T. melanosoma Martínez, Olmedo and Carcavallo, 1987 (Hemiptera, Reduviidae). Mem. Inst. Oswaldo Cruz 96, 137–140. doi: 10.1590/S0074-02762001000100017
Gaspe, M. S., Schachter-Brodie, J., Gurevitz, J. M., Kitron, U., Gürtler, R. E., and Dujardin, J.-P. (2012). Microgeographic spatial structuring of Triatoma infestans (Hemiptera: Reduviidae) populations using wing geometric morphometry in the argentine Chaco. J. Med. Entomol. 49, 504–514. doi: 10.1603/ME11176
Gurevitz, J. M., Ceballos, L. A., Kitron, U., and Gürtler, R. E. (2006). Flight initiation of Triatoma infestans (Hemiptera: Reduviidae) under natural climatic conditions. J. Med. Entomol. 43, 143–150. doi: 10.1603/0022-2585(2006)043[0143:fiotih]2.0.co;2
Gurevitz, J. M., Kitron, U., and Gürtler, R. E. (2007). Flight muscle dimorphism and heterogeneity in flight initiation of field-collected Triatoma infestans (Hemiptera: Reduviidae). J. Med. Entomol. 44, 186–191. doi: 10.1603/0022-2585(2007)44[186:fmdahi]2.0.co;2
Gurevitz, J. M., Kitron, U., and Gürtler, R. E. (2009). Temporal dynamics of flight muscle development in Triatoma infestans (Hemiptera: Reduviidae). J. Med. Entomol. 46, 1021–1024. doi: 10.1603/033.046.0507
Gürtler, R. E., Cecere, M. C., Fernández, M. P., Vázquez-Prokopec, G. M., Ceballos, L. A., Gurevitz, J. M., et al. (2014). Key source habitats and potential dispersal of Triatoma infestans populations in northwestern Argentina: implications for vector control. PLoS Negl. Trop. Dis. 8:e3238. doi: 10.1371/journal.pntd.0003238
Hernández, M. L., Abrahan, L. B., Dujardin, J.-P., Gorla, D. E., and Catalá, S. S. (2011). Phenotypic variability and population structure of peridomestic Triatoma infestans in rural areas of the arid Chaco (Western Argentina): spatial influence of macro- and microhabitats. Vector-Borne Zoo. Dis. 11, 503–513. doi: 10.1089/vbz.2009.0253
Hoffmann, A. A., and Hercus, M. J. (2000). Environmental stress as an evolutionary force. Bioscience 50, 217–226. doi: 10.1641/0006-3568(2000)050[0217:ESAAEF]2.3.CO;2
Klingenberg, C. P. (2008). Morphological integration and developmental modularity. Annu. Rev. Ecol. Evol. Syst. 39, 115–132. doi: 10.1146/annurev.ecolsys.37.091305.110054
Klingenberg, C. P. (2009). Morphometric integration and modularity in configurations of landmarks: tools for evaluating a-priori hypotheses. Evol. Dev. 11, 405–421. doi: 10.1111/j.1525-142X.2009.00347.x
Klingenberg, C. P. (2011). MorphoJ: an integrated software package for geometric morphometrics. Mol. Ecol. Resour. 11, 353–357. doi: 10.1111/j.1755-0998.2010.02924.x
Klingenberg, C. P. (2014). Studying morphological integration and modularity at multiple levels: concepts and analysis. Philos. Trans. R. Soc. Lond. Ser. B Biol. Sci. 369:20130249. doi: 10.1098/rstb.2013.0249
Klingenberg, C. P., Barluenga, M., and Meyer, A. (2002). Shape analysis of symmetric structures: quantifying variation among individuals and asymmetry. Evolution 56, 1909–1920. doi: 10.1111/j.1558-5646.1998.tb02018.x
Klingenberg, C. P., Debat, V., and Roff, D. A. (2010). Quantitative genetics of shape in cricket wings: developmental integration in a functional structure. Evolution 64, 2935–2951. doi: 10.1111/j.1558-5646.2010.01030.x
Klingenberg, C. P., and McIntyre, G. S. (1998). Geometric morphometrics of developmental instability: analyzing patterns of fluctuating asymmetry with Procrustes methods. Evolution 52, 1363–1375. doi: 10.1126/science.166.3905.592.a
Klingenberg, C. P., and Zaklan, S. D. (2000). Morphological integration between developmental compartments in the Drosophila wing. Evolution 54, 1273–1285. doi: 10.1554/0014-3820(2000)054[1273:MIBDCI]2.0.CO;2
Laubichler, M. D., and Maienschein, J. (2009). Form and function in developmental evolution. Cambridge: Cambridge University Press.
Lazić, M. M., Carretero, M. A., Crnobrnja-Isailović, J., and Kaliontzopoulou, A. (2015). An integrated assessment of canalization, developmental stability, modularity, and allometry in lizard head shape am. Nat. 185, 44–58. doi: 10.1086/679011
Lehane, M. J., McEwen, P. K., Whitaker, C. J., and Schofield, C. J. (1992). The role of temperature and nutritional status in flight initiation by Triatoma infestans. Acta Trop. 52, 27–38. doi: 10.1016/0001-706X(92)90004-H
Lu, K., Liang, S., Han, M., Wu, C., Song, J., Li, C., et al. (2020). Flight muscle and wing mechanical properties are involved in flightlessness of the domestic silkmoth. Bombyx Mori. Insect. 11:220. doi: 10.3390/insects11040220
Marcet, P. L., Mora, M. S., Cutrera, A. P., Jones, L., Gürtler, R. E., Kitron, U., et al. (2008). Genetic structure of Triatoma infestans populations in rural villages of Santiago del Estero, northern Argentina. Infect. Genet. Evol. 8, 835–846. doi: 10.1016/j.meegid.2008.08.002
Marden, J. H. (1987). Maximum lift production during takeoff in flying animals. J. Exp. Biol. 130, 235–258. doi: 10.1242/jeb.130.1.235
Mardia, K. V., Bookstein, F. L., and Moreton, I. N. (2000). Statistical assessment of bilateral symmetry of shapes. Biometrika 87, 285–300. doi: 10.1093/biomet/87.2.285
McEwen, P. K., and Lehane, M. J. (1993). Factors influencing flight initiation in the triatomine bug Triatoma sordida (Hemiptera: Reduviidae). Insect Sci. Appl. 14, 461–464. doi: 10.1017/S1742758400014132
McEwen, P. K., and Lehane, M. J. (1994). Relationship between flight initiation and oviposition in Triatoma infestans (Klug, 1834) (Hemiptera: Reduviidae). J. Appl. Entomol. 117, 217–223. doi: 10.1111/j.1439-0418.1994.tb00728.x
McEwen, P. K., Lehane, M. J., and Whitaker, C. J. (1993). The effect of adult population density on flight initiation in Triatoma infestans (Klug) (hem., Reduviidae). J. Appl. Entomol. 116, 321–325. doi: 10.1111/j.1439-0418.1993.tb01203.x
Medved, V., Marden, J. H., Fescemyerb, H. W., Der, J. P., Liu, J., Mahfooz, N., et al. (2015). Origin and diversification of wings: insights from a neopteran insect. Proc. Natl. Acad. Sci. U. S. A. 112, 15946–15951. doi: 10.1073/pnas.1509517112
Montes de Oca-Aguilar, A. C., González-Martínez, A., Chan-González, R., Ibarra-López, P., Smith-Ávila, S., Córdoba-Aguilar, A., et al. (2022). Signs of urban evolution? Morpho-functional traits co-variation along a nature-urban gradient in a Chagas disease vector. Front. Ecol. Evol. 10:805040. doi: 10.3389/fevo.2022.805040
Nattero, J., Cecere, M. C., and Gürtler, R. E. (2017). Temporal variations of fluctuating asymmetry in wing size and shape of Triatoma infestans populations from Northwest Argentina. Infect. Genet. Evol. 56, 133–142. doi: 10.1016/j.meegid.2017.11.012
Nattero, J., Dujardin, J. -P., Fernández, M. P., and Gürtler, R. E. (2015a). Host-feeding sources and habitats jointly affect wing developmental stability depending on sex in the major Chagas disease vector Triatoma infestans. Infect. Genet. Evol. 36, 539–546. doi: 10.1016/j.meegid.2015.08.032
Nattero, J., Leonhard, G., Gürtler, R. E., and Crocco, L. B. (2015b). Evidence of selection on phenotypic plasticity and cost of plasticity in response to host-feeding sources in the major Chagas disease vector Triatoma infestans. Acta Trop. 152, 237–244. doi: 10.1016/j.actatropica.2015.09.022
Nattero, J., Mougabure-Cueto, G., Debat, V., and Gürtler, R. E. (2021). Phenotypic plasticity, canalisation and developmental stability of Triatoma infestans wings: effects of a sublethal application of a pyrethroid insecticide. Parasit. Vectors 14:355. doi: 10.1186/s13071-021-04857-5
Nattero, J., Piccinali, R. V., Gaspe, M. S., and Gürtler, R. E. (2019). Fluctuating asymmetry and exposure to pyrethroid insecticides in Triatoma infestans populations in northeastern Argentina. Infect. Genet. Evol. 74:103925. doi: 10.1016/j.meegid.2019.103925
Palmer, A. R. (1994). “Fluctuating asymmetry analyses: a primer” in Developmental instability: its origins and evolutionary implications. ed. T. A. Markow (Dordrecht: Kluwer Academic Publishers)
Pérez de Rosas, A. R., Segura, E. L., Fusco, O., Bareiro Guiñazú, A. L., and García, B. A. (2013). Fine-scale genetic structure in populations of the Chagas' disease vector Triatoma insfestans (Hemiptera, Reduviidae). Genetica 141, 107–117. doi: 10.1007/s10709-013-9710-0
Rajabi, H., and Gorb, S. N. (2020). How do dragonfly wings work? A brief guide to functional roles of wing structural components. Int. J. Odonatol. 23, 23–30. doi: 10.1080/13887890.2019.1677515
Renault, D. A. (2020). Review of the phenotypic traits associated with insect dispersal polymorphism, and experimental designs for sorting out resident and disperser phenotypes. Insects 11:214. doi: 10.3390/insects11040214
Roff, D. A. (1986). The evolution of wing dimorphism in insects. Evolution 40, 1009–1020. doi: 10.1111/j.1558-5646.1986.tb00568.x
Roff, D. A. (1990). The evolution of flightlessness in insects. Ecol. Monogr. 60, 389–421. doi: 10.2307/1943013
Roff, D. A., and Fairbairn, D. J. (2007). The evolution and genetics of migration in insects. Bioscience 57, 155–164. doi: 10.2307/1943013
Rohlf, F. J., and Slice, D. E. (1990). Extensions of the Procrustes method for the optimal superimposition of landmarks. Syst. Zool. 39, 40–59. doi: 10.2307/2992207
Schachter-Broide, J., Dujardin, J. -P., Kitron, U., and Gürtler, R. E. (2004). Spatial structuring of Triatoma infestans (Hemiptera, Reduviidae) populations from northwestern Argentina using wing geometric morphometry. J. Med. Entomol. 41, 643–649. doi: 10.1603/0022-2585-41.4.643
Schmidt, J. O., Miller, M. L., and Klotz, S. N. A. (2022). Seasonal flight pattern of the kissing bugs Triatoma rubida and T. protracta (Hemiptera: Reduviidae: Triatominae) in southern Arizona, United States. Insects 13:265. doi: 10.3390/insects13030265
Schofield, C. J. (1985). Population dynamics and control of Triatoma infestans. Ann. Soc. Belge Med. Trop. 65, 149–164.
Schofield, C. J., Lehane, M. J., McEwen, P., Catala, S. S., and Gorla, D. E. (1992). Dispersive flight by Triatoma infestans under natural climatic conditions in Argentina. Med. Vet. Entomol. 6, 51–56. doi: 10.1111/j.1365-2915.1992.tb00035.x
Schweigmann, N., Vallve, S., Muscio, O., Ghillini, N., Alberti, A., and Wisnivesky-Colli, C. (1988). Dispersal flight by Triatoma infestans in an arid area of Argentina. Med. Vet. Entomol. 2, 401–404. doi: 10.1111/j.1365-2915.1988.tb00215.x
Tada, S., Honma, K., Kakizaki, M., and Fujisaki, K. (1994). Genetic mode of flight muscle dimorphism in a Scarabaeid, Heptophylla picea Motschulsky. Appl. Entomol. Zool. 29, 303–305. doi: 10.1303/AEZ.29.303
Takahashi, K. H., Rako, L., Takano-Shimizu, T., Hoffmann, A. A., and Lee, S. F. (2010). Effects of small Hsp genes on developmental stability and microenvironmental canalization. BMC Evol. Biol. 10:284. doi: 10.1186/1471-2148-10-284
Vázquez-Prokopec, G. M., Ceballos, L. A., Cecere, M. C., and Gürtler, R. E. (2002). Seasonal variations of microclimatic conditions in domestic and peridomestic habitats of Triatoma infestans in rural Northwest Argentina. Acta Trop. 84, 229–238. doi: 10.1016/S0001-706X(02)00204-8
Vazquez-Prokopek, G. M., Ceballos, L. A., Marcet, P. L., Cecere, M. C., Cardinal, M. V., Kitron, U., et al. (2006). Seasonal variations in active dispersal of natural populations of Triatoma infestans in rural North-Western Argentina. Med. Vet. Entomol. 20, 273–279. doi: 10.1111/j.1365-2915.2006.00637.x
von Dassow, G., and Munro, E. (2002). Modularity in animal development and evolution: elements of a conceptual framework for EvoDevo. J. Exp. Zool. 285, 307–325. doi: 10.1002/(SICI)1097-010X(19991215)285:4<307::AID-JEZ2>3.0.CO;2-V
Wagner, G. P., and Altenberg, L. (1996). Perspective: complex adaptations and the evolution of evolvability. Evolution 50, 967–976. doi: 10.1111/j.1558-5646.1996.tb02339.x
Waleckx, E., Gourbière, S., and Dumonteil, E. (2015). Intrusive versus domiciliated triatomines and the challenge of adapting vector control practices against Chagas disease. Mem. Inst. Oswaldo Cruz 110, 324–338. doi: 10.1590/0074-02760140409
Williams, N. G., and Schofield, C. J. (1985). The role of temperature in flight initiation of triatomine bugs. Trans. R. Soc. Trop. Med. Hyg. 79:282.
Willmore, K., Young, N. M., and Richtsmeier, J. T. (2007). Phenotypic variability: its components, measurement and underlying developmental processes. Evol. Biol. 34, 99–120. doi: 10.1007/s11692-007-9008-1
World Health Organization. Chagas disease (American trypanosomiasis). (2017). Geneva: World Health Organization.
Zakharov, V. M. (1992). Population phenogenetics: analysis of developmental stability in natural populations. Acta Zool. Fenn. 191, 7–30. doi: 10.1007/BF02424502
Keywords: developmental buffering, flight muscles, integration, Triatominae, wing morphometry, wing aspect ratio
Citation: Nattero J, Piccinali RV, Fiad FG, Cano F and Carbajal-de-la-Fuente AL (2023) Relationship between flight muscle dimorphism and wing morphometry in Triatoma infestans (Klug, 1834) (Hemiptera, Reduviidae, Triatominae). Front. Ecol. Evol. 11:1211219. doi: 10.3389/fevo.2023.1211219
Edited by:
Cleber Galvão, Oswaldo Cruz Foundation (Fiocruz), BrazilReviewed by:
Rodrigo Gurgel-Gonçalves, University of Brasilia, BrazilKaio Cesar Chaboli Alevi, São Paulo State University, Brazil
Copyright © 2023 Nattero, Piccinali, Fiad, Cano and Carbajal-de-la-Fuente. This is an open-access article distributed under the terms of the Creative Commons Attribution License (CC BY). The use, distribution or reproduction in other forums is permitted, provided the original author(s) and the copyright owner(s) are credited and that the original publication in this journal is cited, in accordance with accepted academic practice. No use, distribution or reproduction is permitted which does not comply with these terms.
*Correspondence: Julieta Nattero, anVsaWV0YW5hdHRlcm9AZ21haWwuY29t