- 1Canadian Rivers Institute and Department of Biology, University of New Brunswick, Fredericton, NB, Canada
- 2Department of Aquatic Sciences and Assessment, Swedish University of Agricultural Sciences, Uppsala, Sweden
- 3Department of Supervision and Contingency Planning, Norwegian Water Resources and Energy Directorate, Oslo, Norway
- 4Natural History Museum, University of Oslo, Oslo, Norway
- 5Department of Biology and Cold Regions Research Centre, Wilfrid Laurier University, Waterloo, ON, Canada
Introduction: Arctic freshwater ecosystems are undergoing rapid environmental transformation because of climate change, which is predicted to produce fundamental alterations in river community structure and function.
Methods: We explored how climate change affects benthic invertebrate communities of Arctic streams by examining patterns of their biological traits along latitudinal and climatic gradients in eastern North America (Canada) and northwestern Europe (Sweden, Norway).
Results: Despite differences in taxonomic composition between continents, we identified similarities in the functional trait niche (FTN) of predominant macroinvertebrate taxonomic groups. Trait composition differed by latitude in eastern Canada, with a predominance of cold-tolerant taxa, tubular body shape, and cased and attached habits at the highest latitudes. Differences in trait composition were evident among ecoregions in Europe, with trait dominance at the highest latitudes that was comparable to North America. There was a similar increase in the relative abundance of cold tolerance and tubular body shape and a decrease in obligate shredders and trait richness with decreasing temperatures across both continents.
Discussion: These patterns are indicative of FTNs that include physiological traits and habits that are advantageous for the low temperatures, short ice-free period, and low riparian vegetation cover at the highest latitudes. We predict that climate change will lead to an increase in functional diversity at high latitudes, as organisms with trait modalities that are currently only found at lower latitudes move northward. However, this change in trait composition will be mediated by the effect of spatial connectivity on dispersal ability, with slower change occurring on Arctic islands. These findings can support modelling of future change in Arctic freshwater assemblages in response to ongoing climate change.
1 Introduction
Arctic regions are warming at nearly four times the global rate (0.73°C per decade in the Arctic compared to a global rate of 0.19°C per decade; Rantanen et al., 2022), and as a result, freshwater ecosystems are undergoing rapid environmental transformations that will cause fundamental alterations to the structure and function of the biotic communities (Mustonen et al., 2018; Heino et al., 2020; Woolway et al., 2022). Arctic freshwaters are typically species-poor and predominated by cold-tolerant taxa, an ecological niche that reflects the extreme environment and climatic conditions of the region (Goedkoop et al., 2022). While it is difficult to predict specific changes in Arctic freshwater biota distribution and abundance that will be associated with climate change due to limited spatial coverage of baseline data and a lack of long-term monitoring data (Goedkoop et al., 2022), a first approximation for forecasting the hypothesized alterations is to examine changes in community structure along contemporary latitudinal and climatic gradients (i.e., space for time analysis). Such an approach is based upon the concept of “bioclimatic envelopes”, which asserts that climatic differences across regions or latitudes have a predominant role in determining the geographical distribution of species (Pearson and Dawson, 2003). Although biodiversity responses along latitudinal and climatic gradients may vary among organisms and trophic levels in specific Arctic regions (Lau et al., 2022), recent circumpolar freshwater biodiversity analyses show a general decline in richness with increasing latitude, reflecting both the lower temperatures and greater geographic isolation beyond the northern border of the continental Arctic (e.g., Goedkoop et al., 2022; Lento et al., 2022).
The detection of climate-related changes in freshwater biotic communities across continental-scale biogeographic regions (e.g., low to high Arctic) is complicated by smaller-scale taxonomic differences. Statzner and Beche (2010) argued that a potential solution to this problem would be to use general biological traits of organisms, indicative of ecological functions, which are comparable among communities even though taxonomic composition may differ. Such ecological traits can exhibit stability across large spatial areas (e.g., ecoregions; Statzner and Beche, 2010; Martini et al., 2021; Kunz et al., 2022) likely because environmental gradients act as filters that limit the ecological functions that can be expressed within regions (Poff, 1997; Schmera et al., 2017). The combination of trait states expressed by a taxon, its Functional Trait Niche (FTN; sensu Poff et al., 2006), determines a taxon’s occurrence along an environmental gradient and its response to shifts in environmental drivers (Schmera et al., 2022). Thus, how climate change-induced shifts in contemporary environmental drivers, such as temperature, sediments, nutrients, and land cover, will affect freshwater communities and food webs in Arctic ecosystems is likely revealed in part by latitudinal shifts in functional ecological traits (Brown et al., 2018). Moreover, ecological traits information for benthic assemblages of streams in North America and Europe has developed extensively over the past several decades, making it feasible to use trait composition to facilitate inter-continental comparisons (Statzner and Beche, 2010; Culp et al., 2011), as long as inconsistencies in terminology and trait assignment in different trait databases are considered (Martini et al., 2021; Kunz et al., 2022).
With continued warming, losses of stenothermic species and increased competition through range expansion of southern eurythermic species are expected to alter the structural and functional composition of Arctic freshwater ecosystems (Culp et al., 2012; Wrona et al., 2013; Lento et al., 2019). Predicting the magnitude and direction of future changes in functional composition requires an understanding of how trait diversity and the relative abundances of different trait modalities (states) vary in response to climatic drivers. Our objectives were therefore to compare and contrast the distribution of ecological traits in benthic invertebrate assemblages of streams along latitudinal and climatic gradients in the eastern and western Atlantic regions, i.e., eastern North America and northwestern Europe. Although the latitudinal gradients examined in North America and Europe covered similar distances, climatic conditions in Europe are less extreme. Moreover, temperatures in the eastern Canadian Arctic in North America have remained relatively stable compared to other parts of the circumpolar Arctic (Prowse et al., 2006), thereby offering the potential to examine ecological patterns that are relatively unchanged by climatic shifts.
In this paper, we assessed variation in specific traits and trait diversity metrics in relation to both latitude and temperature (climate normals) to examine how latitudinal changes in the abiotic template structure the distribution of ecological traits. This information is vital for our understanding of ecosystem function in Arctic freshwaters and will support the prediction of future shifts in the relative abundance and diversity of traits in response to climate change. We hypothesize that contemporary distributions of functional traits of stream macroinvertebrate communities reflect the shift to colder environments at the highest latitudes, with a greater predominance of adaptations and advantageous physiological conditions and habits. We predict a higher relative abundance of cold tolerance traits at higher latitudes, as well as traits such as case-making and burrower habits that may provide additional protection from the elements (Figure 1), consistent with the adaptations required to survive in arctic conditions (Danks, 2004). We also predict that increasing latitude will be associated with a lower relative abundance of the shredder feeding habit, particularly obligate shredders (Figure 1), due to the latitudinal decline in catchment vegetation and leaf litter input to streams and concurrent shift towards algal-dominated trophic pathways. As mainly cold-adapted species are favored at higher latitudes, we predict an overall decrease in trait richness but an increase in trait evenness with increasing latitude (Figure 1).
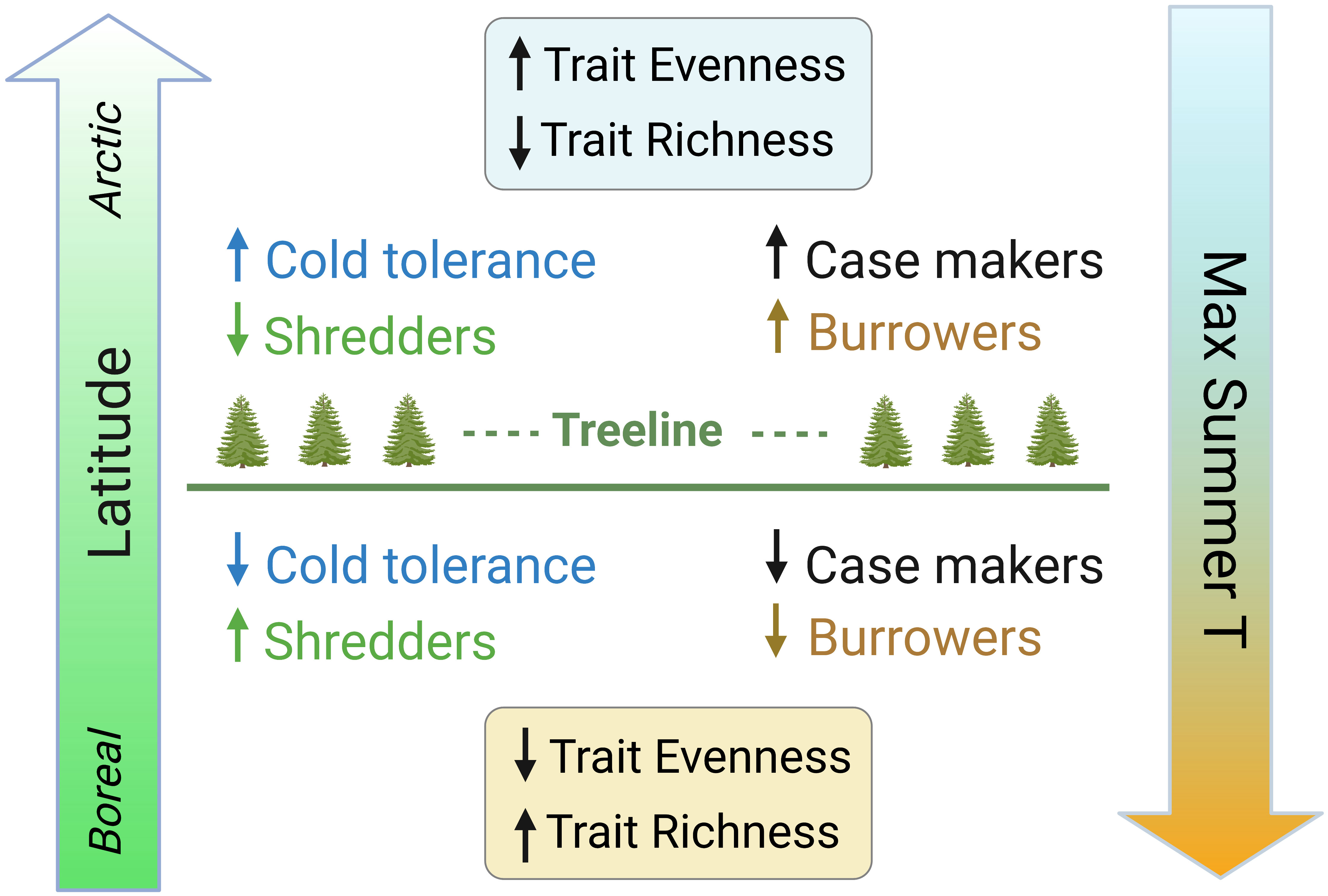
Figure 1 Conceptual diagram of predicted shifts in stream benthic macroinvertebrate trait modalities and metrics along latitudinal and temperature gradients in the Arctic. Low latitude sites below the treeline with higher maximum summer temperatures are predicted to have lower relative abundance of cold tolerance, case makers, and burrowers, higher relative abundance of shredders, and overall lower trait evenness and higher trait richness, whereas high latitude sites above the treeline with lower maximum summer temperatures are predicted to show the opposite patterns. The range of maximum temperatures in this study is from 16.5°C at the lowest latitudes to 1.6°C at the highest latitudes.
2 Material and methods
2.1 Study area
2.1.1 North America
In North America, 86 sites were sampled along a gradient that covered the full latitudinal extent of the eastern Canadian Arctic. Groups of sites were sampled at each of four latitudes along the gradient: 58°N, 63°N, 72°N, and 81°N (Figure 2; Supplementary Table 1). Due to the remoteness of these largely roadless areas, most sites were chosen based on the feasibility of helicopter access. At 58°N, 25 sites were sampled in northeastern Québec and in the vicinity of Torngat Mountains National Park in northern Labrador. At 63°N, 16 sites were sampled near the city of Iqaluit, in southern Baffin Island, Nunavut. There were 27 sites sampled at 72°N in three areas of northern Baffin Island: near the town of Pond Inlet, and on Borden Peninsula and Bylot Island, both part of Sirmilik National Park. At 81°N, 18 sites were sampled on Ellesmere Island, Nunavut, near Lake Hazen in Quttinirpaaq National Park. The eastern Canadian Arctic is extremely sparsely populated (Iqaluit and Pond Inlet, the two population centers located near sampling sites, have populations of less than 7,000 and 2,000, respectively). As a result, human impacts were minimal at all sampling sites (TN = 26 – 823 µg L−1, TP = 0.9 – 8.5 µg L−1). Catchment area varied among sites at each latitude; catchment area was similar at 63°N (285.8 ± 179.9 km2, mean ± SE hereafter) and 72°N (197.1 ± 89.7 km2), and was also similar at 58°N (540.5 ± 178.4 km2) and 81°N (569.9 ± 408.2 km2), though catchment area was much more variable at 81°N. Sampling sites were at a mean altitude of 123 (± 13) m above sea level. Water source differed among sites, with varying influences of glacial, snowmelt, lake, and groundwater sources, though sites at 58°N and 63°N had minimal glacial influence.
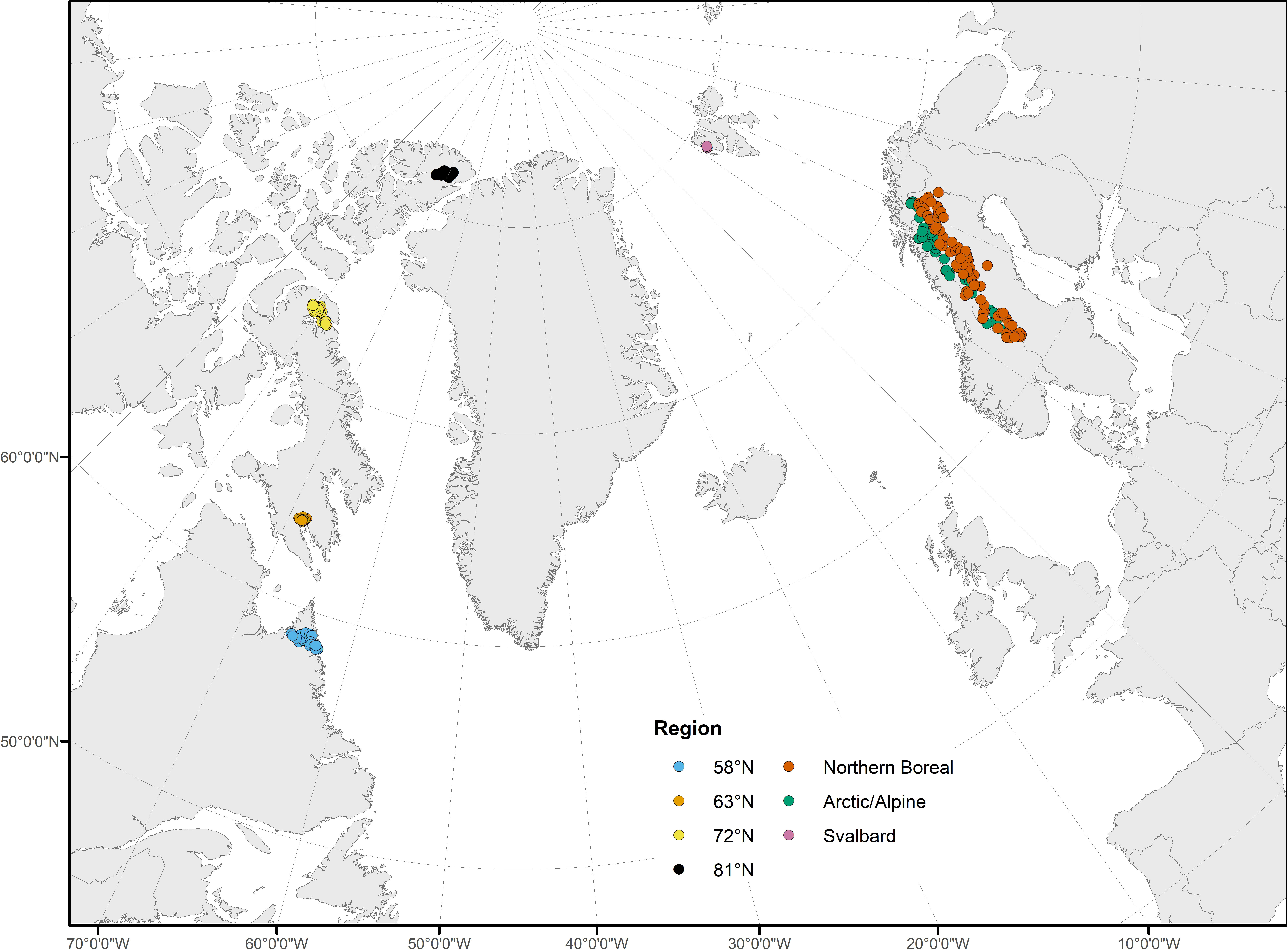
Figure 2 Study area map, including sample sites in North America at 58°N, 63°N, 72°N, and 81°N, and in northwestern Europe in the Northern Boreal and Arctic/Alpine regions and Svalbard. Points correspond to sample locations and are colored based on region.
The areas sampled along the North American latitudinal gradient fall within the Arctic Cordillera ecoregion (sites at 58°N, 72°N, and 81°N) and the Northern Arctic ecoregion (sites at 63°N, 72°N, and 81°N; Commission for Environmental Cooperation Working Group, 1997). The Arctic Cordillera ecoregion is characterized by numerous mountains, deep valleys, and fjords, whereas the Northern Arctic ecoregion landscape is predominated by plains and hills (Wiken et al., 1996). Vegetation is limited or absent in northern regions of both ecozones, but southern regions, particularly along the coast, contain areas of tundra meadow with small shrubs or hummocks with mosses and lichens (Wiken et al., 1996). Both ecoregions experience a cold climate, with a short growing season and significant spring freshets. High-latitude streams and rivers in the sampling area are generally ice-covered from late September through June, whereas the ice-on period may span from November to May in Labrador (58°N) during warmer years (temperature logger data; results not shown).
2.1.2 Northwestern Europe
Samples were collected from 41 streams in the Arctic/Alpine and 79 streams in the Northern Boreal ecoregions of Sweden (Nordic Council of Ministers, 1984; but modified according to Gustafsson and Ahlén, 1996) as part of a Swedish national lake and stream survey (Figure 2; Supplementary Table 1; Wilander et al., 2003). The Arctic/Alpine and Northern Boreal ecoregions in Sweden cover a latitudinal gradient between 61.1–69.2°N and are part of the global subarctic region. The Arctic/Alpine ecoregion is characterized by bare rock and tundra vegetation (dwarf shrubs and willow) above the treeline (ca. 700–1100 m a.s.l.) and mountain birch forest with sparse stands of pine and spruce at lower elevations. The Northern Boreal ecoregion is situated just east of the Arctic/Alpine ecoregion and is characterized by forests (predominantly birch, pine, and spruce) and peat bogs. Both ecoregions are relatively unimpacted, although hydropower dams, local mining, and forestry activities occur (to a limited extent) in the Northern Boreal ecoregion. These ecoregions form the northernmost regions of the Scandinavian Peninsula and are among the most remote, and sparsely populated regions in Scandinavia and Europe.
As a consequence of their remote locations, the vast majority of streams were visited by helicopter. The streams were randomly selected from the National Catchment Register (Swedish Meteorological and Hydrological Institute) among streams with catchment sizes of 15–250 km2 (see Wilander et al., 2003 for a detailed description of the selection procedure). All 120 stream sites were situated between 61–69°N at elevations of 473 ± 10 m (mean ± SE; range = 261–808 m) above sea level. Urban and agricultural (almost exclusively grassland) land use in the catchments was less than 1% for 3 and 36 streams, respectively, and was absent in >80 streams. The streams were not affected by anthropogenic eutrophication (TN = 77–604 µg L−1, TP = 2–20 µg L−1) and only received low levels of long-range pollutants (e.g. metals, sulfate) by atmospheric deposition.
In order to cover a latitudinal gradient similar to that of the North American streams, samples from six sites located at 79°N on the archipelago of Svalbard (Norway) were also included (Figure 2; Supplementary Table 1). These sites were 5–110 m above sea level and belonged to two separate treeless catchments in the Arctic ecozone: Bayelva (30.9 km2; three sites) and Londonelva (0.7 km2; three sites) (Milner et al., 2001; Lods-Crozet et al., 2007). River Bayelva originates from glaciers Austre Brøggerbreen (area 11.7 km2) and Vestre Brøggerbreen (5.3 km2), which have been retreating for >100 years with a retreat rate of approximately 0.4 m year–1 (Tranter et al., 1996). River Londonelva is a non-glacial system fed by snowmelt and rainfall. There is no urbanization or anthropogenic influence within the catchments (the research base of Ny-Ålesund represents the only nearby anthropogenic influence outside of limited mineral exploration that took place > 70 years ago). Nutrient concentrations were low in all streams (TN = 0–55 µg L−1, TP = 2–24 µg L−1).
2.2 Data collection
2.2.1 North America
Sampling in North America took place in August 2007 (58°N), August 2008 (63°N and 81°N), and July 2009 (72°N) (Culp et al., 2019). Benthic macroinvertebrate sampling followed the standard Canadian Aquatic Biomonitoring Network approach (Environment Canada, 2012). A 400-μm-mesh kick net was held downstream while the collector moved through the riffle in a zigzag pattern, disturbing the substrate by kicking and rubbing rocks for a period of 3 minutes (Environment Canada, 2012). Invertebrate samples were preserved in ethanol (80%, final concentration) and transported to the laboratory for sorting and identification to the lowest practical taxonomic level (genus when possible) by a Society of Freshwater Science certified taxonomist. Due to the high abundance of Chironomidae, the total abundance of each chironomid taxon was estimated using sub-sampling procedures.
2.2.2 Northwestern Europe
Macroinvertebrate samples were collected at Swedish sites in September–October 2000 and at Norwegian sites in July–August 1997, using standardized kick sampling (EN 27828 1994) and a kick net (mesh size 500 μm [Sweden] or 250 μm [Norway]). In total, five kick samples (each 1 m × 1 min [Sweden] or 30 s [Norway]) were collected from riffles in each site and preserved in ethanol (70%, final concentration). In the laboratory, macroinvertebrates were sorted and identified to the lowest possible taxonomic level, i.e., mostly genus or species, with Chironomidae identified to subfamily and some non-insect groups such as Oligochaeta identified to higher taxonomic levels. Invertebrate data from the five kick samples were pooled for each site for subsequent analysis.
2.3 Data preparation
North American invertebrate taxonomic data were converted to trait data using the US EPA’s North American trait database (U.S. Environmental Protection Agency (EPA), 2012). European taxonomic data were converted to trait data using the trait databases of Usseglio-Polatera et al. (2000) and Bis and Usseglio-Polatera (2004). Traits that were described in both the North American and the European databases were selected for analysis; these included feeding habits, temperature preference, body shape, and habitat preference (see Table 1 for full list of 14 traits). For each trait, modalities (or states) were combined and renamed as necessary to ensure maximum comparability of the trait data across continents (see Table 1 for full list of 53 trait modalities). Because of the importance of cold tolerance in Arctic invertebrate communities, a special “cold” trait modality was created for the temperature preference trait and assigned to the taxa that were described as tolerant of maximum temperatures ≤ 4°C by Milner et al. (2001): Oligochaeta, Tipulidae, and the Chironomidae subfamilies Diamesinae and Orthocladiinae.
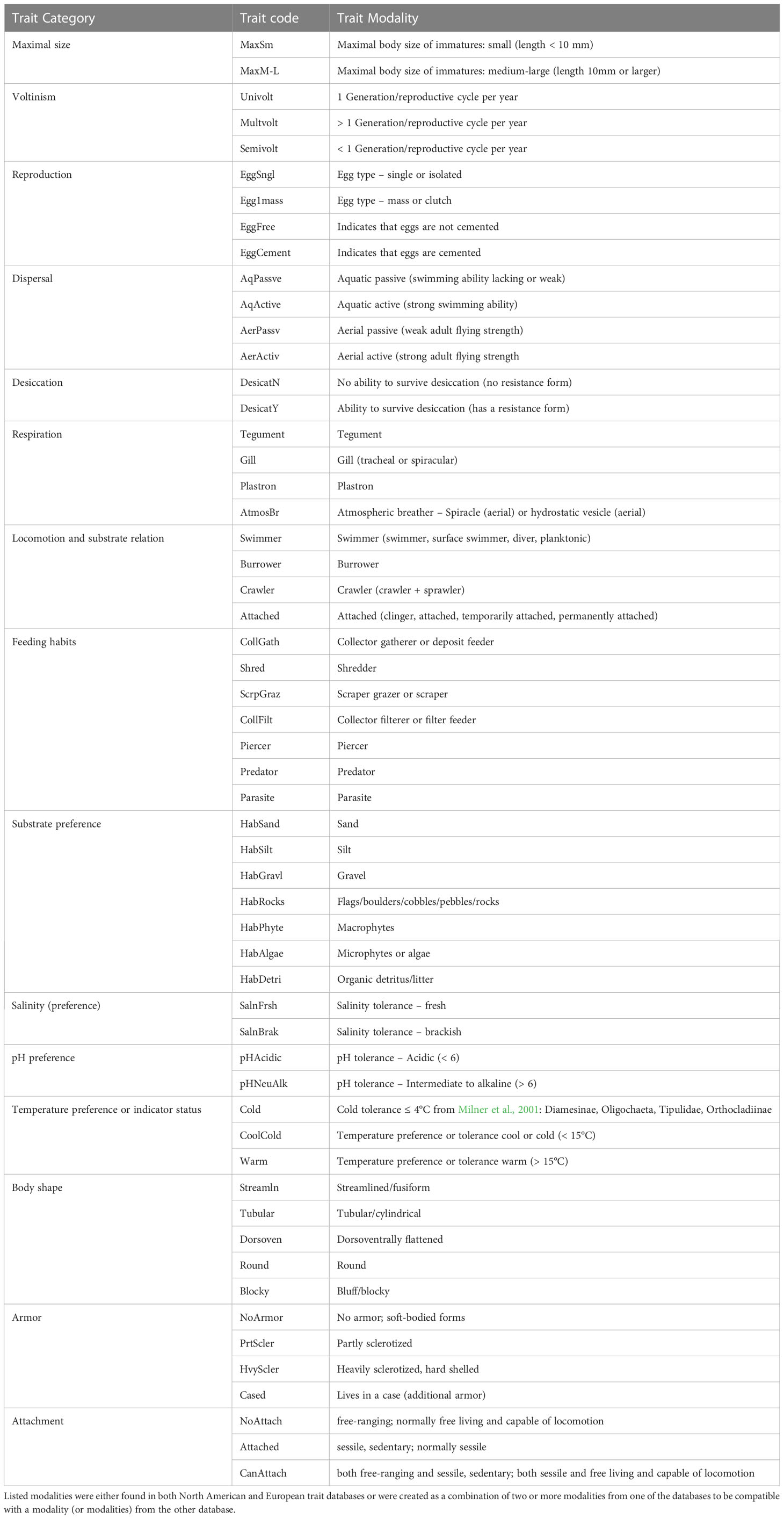
Table 1 Summary of harmonized trait modalities used in analyses, including the trait category, trait code (used in figures) and a description of the trait modality.
Trait modalities were assigned at the level of genus where possible, with the exception of Chironomidae, for which modalities were assigned at the level of subfamily. When a genus was not described in the database, trait modalities for the most closely related taxon were assigned. The European trait databases used fuzzy coding to assign trait modalities to taxa, whereas the North American database assigned trait modalities on a presence/absence basis. In order to make trait data comparable for both continents, European trait data were transformed into a binary structure, with all selected trait modalities categorized as either present (1) or absent (0) for a given taxon. Because trait databases were generally not built based on observations from Arctic systems (and therefore did not capture Arctic-specific adaptations in habit or lifecycle), we did not attempt to choose a single modality for each trait; rather, we used a multistate approach for assigning trait modalities for each taxon. For both European and North American data, trait data were summarized as the relative abundance of organisms in a sample with a given trait modality. To isolate the potential for differences in feeding mode north of the treeline, we separated out taxa that were only assigned as shredders (i.e., not assigned any other feeding mode) in each database, and calculated the relative abundance of these organisms as the obligate shredder trait. In addition, we calculated several diversity metrics based on trait composition, including trait richness and Simpson’s evenness.
2.4 Analytical approach
2.4.1 Functional trait niches
The trait modalities of all taxa collected along the North American and European latitudinal gradients were used to examine the functional trait niche (FTN) overlap of Arctic taxa. For each continent, we used Gower’s similarity coefficient (Gower, 1971) to calculate pairwise similarity matrices among all collected taxa based on each taxon’s trait modalities (i.e., each taxon’s FTN). We ran multivariate Principal Coordinates Analysis (PCoA) separately on the North American and European similarity matrices to evaluate FTN overlap among Arctic taxa on each continent. We also ran Redundancy Analysis (RDA) on the trait similarity matrix, with trait modalities for each taxon used as constraining variables to allow identification of the trait modalities that contributed most to differences among taxa.
2.4.2 Gradients in trait composition
Principal Components Analysis (PCA) was used to examine the spatial trait patterns for each continent based on a matrix of the relative abundance of traits per site, and to determine whether there were any shifts in trait dominance along the latitudinal gradients. The relative abundance of traits was logit-transformed to approximate a normal distribution before PCA (Warton and Hui, 2011). All multivariate analyses were conducted using Canoco for Windows (Version 4.5; Plant Research International).
2.4.3 Spatial patterns in trait abundance and diversity
We used univariate analysis of individual trait modalities and diversity metrics to more closely examine spatial differences in trait composition and to relate those differences to climate gradients. North American samples were naturally grouped by latitude, so we ran a one-way ANOVA for each trait modality or diversity metric to compare mean values among latitudes. When the ANOVA indicated a significant differences among latitudes (at α = 0.05), we used Tukey’s Honestly Significant Difference (HSD) test to identify pairwise differences. When the ANOVA residuals did not meet the assumptions of normality or homoscedasticity, we used a nonparametric Kruskal Wallis test followed by Wilcoxon pairwise tests with a Bonferroni correction. For Europe, we compared trait modalities and diversity metrics among boreal, alpine and Svalbard sites. Because there were so few Svalbard sites compared to the boreal and alpine sites (resulting in heteroscedasticity of residuals), we ran all analyses of European data using a nonparametric Kruskal Wallis test followed by Wilcoxon pairwise tests with a Bonferroni correction when there were significant differences among regions.
2.4.4 Trait patterns in relation to latitude and temperature
We examined broader patterns in traits in relation to both latitude and climate by plotting data from both continents together while comparing separate regression slopes between continents. While we recognize that trait data for each continent came from a different trait database and therefore some variation among continents might be attributable to differences in how the databases have assigned modalities to taxa (even though we have harmonized the modalities used for both North American and northwestern European datasets), the combined plots allowed us to examine whether patterns appeared similar across both continents. We ran separate simple linear regressions for each continent and compared slopes using 95% confidence intervals to confirm the degree of similarity among slopes, as described below.
Trait modalities/diversity metrics were first plotted as a function of latitude and data for North America and Europe were fit with separate simple linear regression lines. To characterize climatic differences among sample regions that varied not only in latitude but also in elevation, we extracted the long-term average (1970–2000) maximum August temperature for each sampling location, as a measure of summer high temperatures, from WorldClim (Version 2; http://worldclim.org/version2; Fick and Hijmans, 2017). Air temperature was used as a proxy for water temperature as recommended by Yang et al. (2021), and was regressed with latitude (separately by continent, because European sites covered a latitudinal and altitudinal gradient) to quantify the amount of variance in temperature that could be explained by latitude. We then tested regressions of trait modalities/diversity metrics as a function of temperature. Data for both continents were plotted together, but separate linear regressions were fit for each continent. Where assumptions of normality and homoscedasticity were not met, we used rank regression analysis and compared different score functions (e.g., Wilcoxon, normal, or bent; Kloke and McKean, 2012) to find the best fit to the data. Rank regression lines were not added to scatterplots, as plots represented actual data rather than ranks. All graphs and univariate analyses were completed in R version 4.2.2 (R Development Core Team, 2022) using the packages ggplot2 (Wickham, 2016), car (Fox and Weisberg, 2019), multcomp (Hothorn et al., 2008), and Rfit (Kloke and McKean, 2012).
3 Results
3.1 Functional trait niches
3.1.1 North America
The PCoA for North America indicated a distinction between the FTN occupied by Diptera and Oligochaeta taxa and the FTN of Ephemeroptera, Plecoptera, and Trichoptera (EPT) taxa (Figure 3A). These taxonomic groups were separated along the first axis of the PCoA, which explained 23.0% of the variance among taxa. Diptera and Oligochaeta FTNs were characterized by a lack of armor, a tubular body shape, burrower habit, and cold tolerance (Figure 3B). The FTNs of EPT genera shared a great deal of overlap, and included the presence of gills, a dorsoventrally-flattened body shape, partial sclerotization, and a wide range of habitat preferences (ranging from silt to rocks), temperature tolerances (warm to cool/cold), and feeding habits (shredders, scraper/grazers, and collector/gatherers) (Figure 3B). The second axis explained 12.6% of the variance in organism traits, and predominantly represented a separation of Arachnida (aquatic mites) from other taxa (Figure 3A). The FTN of Arachnida taxa was characterized by a round body shape, maximum small body size, parasite or predator feeding habits, and cemented eggs or single egg mass (Figure 3B). On the negative end of the second axis gradient, a selection of Trichoptera genera was slightly separated from the majority of EPT on the basis of size (> 10mm), univoltine life cycle, attached habit, presence of a case, and passive aquatic and aerial life stages (Figure 3B).
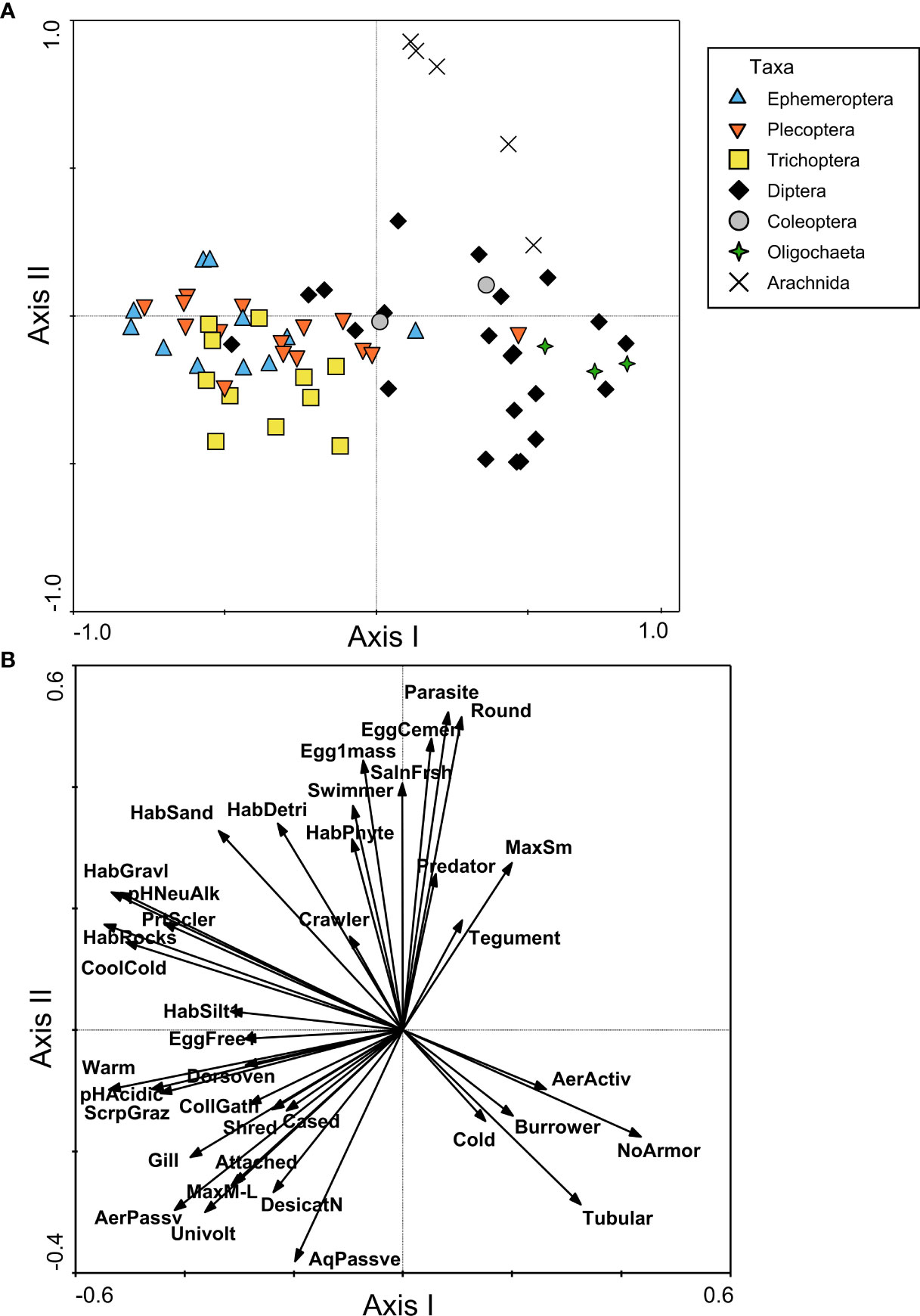
Figure 3 Functional trait niche assessment of benthic macroinvertebrates from North American samples, including (A) PCoA plot based on a similarity matrix of taxa traits, with points representing individual taxa and shapes/colors used to group taxa by order or higher classification level; and (B) RDA plot with trait modalities used as constraining variables (shown as vectors). The plot in (B) only includes vectors for trait modalities that had a correlation with the first or second axis of |r| > 0.25. Trait modality abbreviations can be found in Table 1.
3.1.2 Northwestern Europe
FTNs for northwestern European taxa were evident in the spatial groupings of genera in the PCoA, though there was a great deal of overlap of taxon groups, likely due to the diversity of this region and trait redundancy among taxa. Ephemeroptera, Plecoptera, and Odonata taxa were separated from Diptera and Coleoptera taxa along the first axis of the PCoA, which explained 7.5% of the variation among taxa (Figure 4A). Ephemeroptera, Plecoptera, and Odonata taxa were positively associated with a dorsoventrally flattened shape, shredder habit, partial sclerotization, and single eggs (Figure 4B). Coleoptera taxa were associated with physiological trait modalities such as heavy sclerotization, streamlined, and small size (< 10mm), as well as respiration modes such as atmospheric breather and plastron (Figure 4B). Diptera taxa overlapped with many Trichoptera taxa in the PCoA (Figure 4A) and therefore shared a similar FTN, although most Trichoptera were more closely associated with the second axis of PCoA (explaining 6.6% of the variance). Diptera and Trichoptera were positively associated with a tubular body shape, attached and cased habits, passive aerial life stage, and collector/filterer feeding habit (Figure 4B). Diptera were additionally associated with no armor, gravel habitat, and reproductive trait modalities (multivoltine, egg single mass) on the first axis, whereas Trichoptera were positively associated with medium to large size (> 10 mm), rock habitat, gills, and predator feeding habit on the second axis (Figure 4B). In contrast to the North American FTNs, temperature preference/tolerance trait modalities for European taxa were only weakly correlated with the RDA axes (|r| < 0.25), which indicated that they did not contribute greatly to distinctions among taxa.
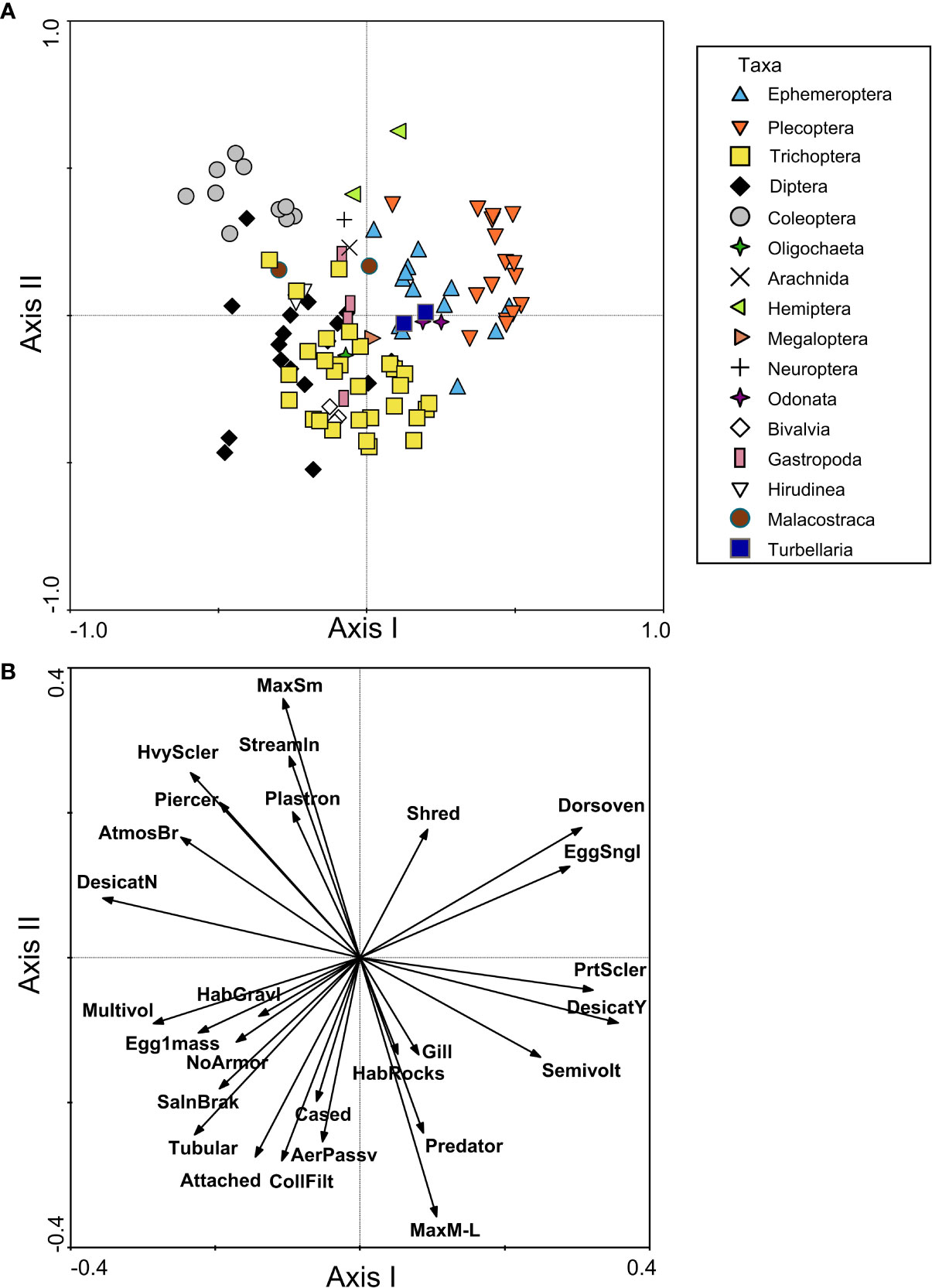
Figure 4 Functional trait niche assessment of benthic macroinvertebrates from northwestern Europe samples, including (A) PCoA plot based on a similarity matrix of taxa traits, with points representing individual taxa and shapes/colors used to group taxa by order or higher classification level; and (B) RDA plot with trait modalities used as constraining variables (shown as vectors). The plot in (B) only includes vectors for trait modalities that had a correlation with the first or second axis of |r| > 0.25. Trait modality abbreviations can be found in Table 1.
3.2 Gradients in trait composition
3.2.1 North America
The first axis of the PCA of North American data, which explained 55.2% of the variance in community traits, reflected a latitudinal gradient from 58°N on the positive end of the axis to 63°N near the origin and 72°N on the negative end of the axis (Figure 5A). Sites from 81°N were spread across the first axis, overlapping with sites from other latitudes. Sites from 58°N were positively associated with trait modalities describing body shape, such as streamlined, dorsoventrally flattened, and blocky, as well as partial sclerotization, gills, swimmer habit, and a variety of reproductive trait modalities (Figure 5A). A large number of trait modalities were found near the origin or positively associated with higher latitude sites, primarily because macroinvertebrates at higher latitudes had a reduced number of trait modalities compared to 58°N, resulting in higher relative abundance of the modalities. However, some trait modalities were clearly associated with higher latitude sites, including cold tolerance, tubular shape, burrower and cased habits, no armor, and tegument respiration, indicative of the FTN occupied by Diptera and Oligochaeta taxa.
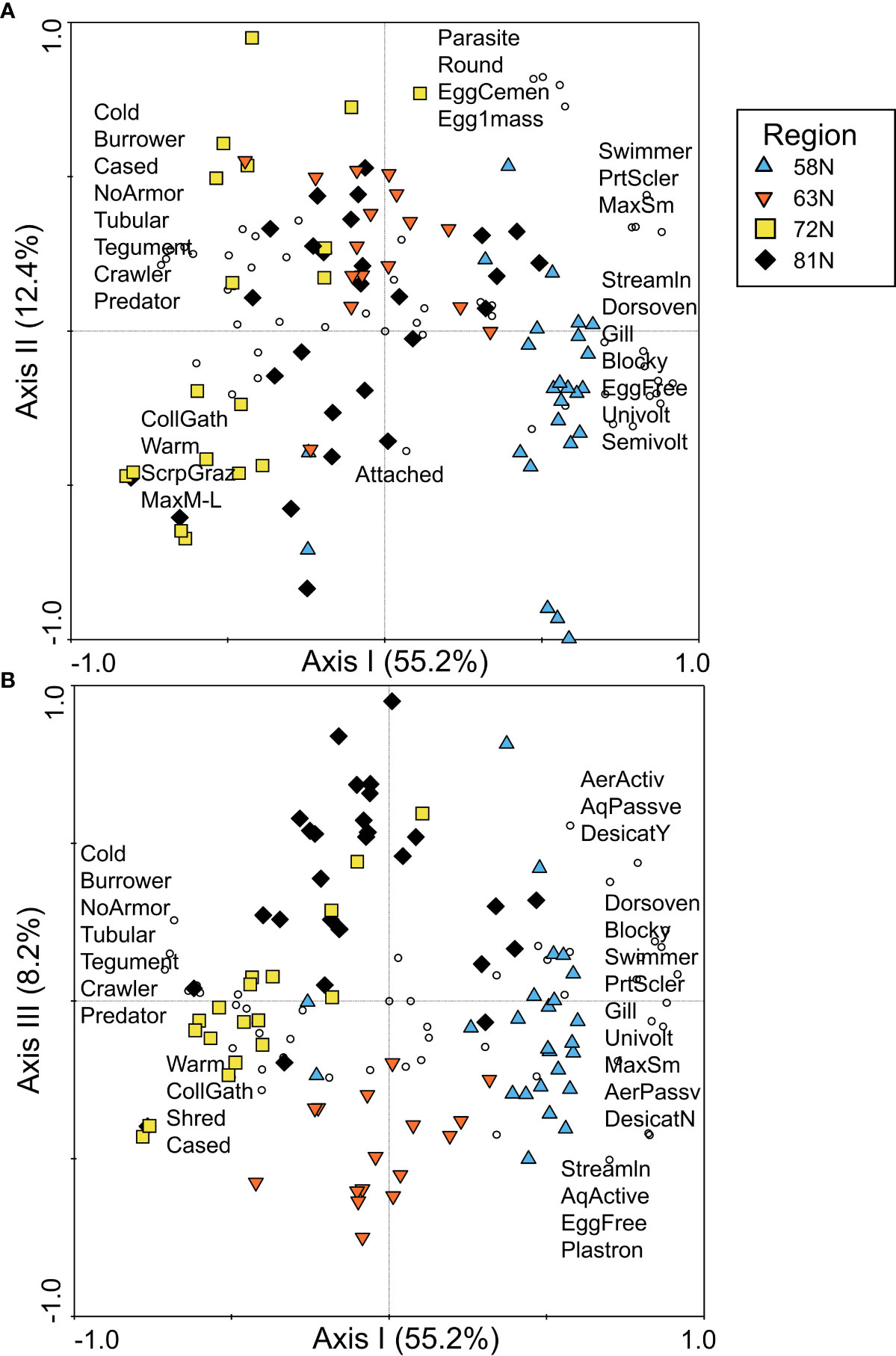
Figure 5 PCA ordination of trait relative abundance data for North America, plotted as (A) PCA axis I and axis II and (B) PCA axis I and axis III, with symbol colors/shapes indicating the four latitude regions sampled in eastern Canada (58°N, 63°N, 72°N, and 81°N) and circles representing trait modalities. A subset of trait modality labels is presented for each figure in the approximate location of the trait modality points to ease interpretation of the figures. Trait modality abbreviations can be found in Table 1.
There was greater distinction among higher latitude sites when the third axis of the PCA was plotted (Figure 5B). The third axis, which explained 8.2% of the variance in trait composition, separated sites at 63°N from sites at 81°N. Neither latitude was strongly associated with any particular trait modalities along the third axis, likely reflecting the fact that the greatest contrast in trait composition was between 58°N and all other latitudes.
3.2.2 Northwestern Europe
The strongest distinction in trait composition among regions in northwestern Europe was a separation of Svalbard sites from boreal and alpine sites along the first axis of the PCA (Figure 6A). Svalbard sites were positively associated with trait modalities that included cold tolerance and cool/cold preference, tubular body shape, no armor, attached and cased habits, and parasite and collector/filterer feeding habits (Figure 6B). On the opposite end of the first axis gradient, boreal and alpine sites were associated with trait modalities such as warm temperature preference, body shape modalities such as dorsoventrally flattened and streamlined, partial sclerotization, small size, rocky habitat, and shredder feeding habit (Figure 6B). Separation between boreal and alpine regions was evident along the second axis of the PCoA, which explained 15% of the variance in trait composition (Figure 6A). Boreal sites were more strongly positively associated with streamlined and shredder trait modalities, as well as heavy sclerotization, respiration via plastron, and crawler and swimmer habits, whereas alpine sites had a stronger positive association with burrower and predator habits and egg clutch (Figure 6B).
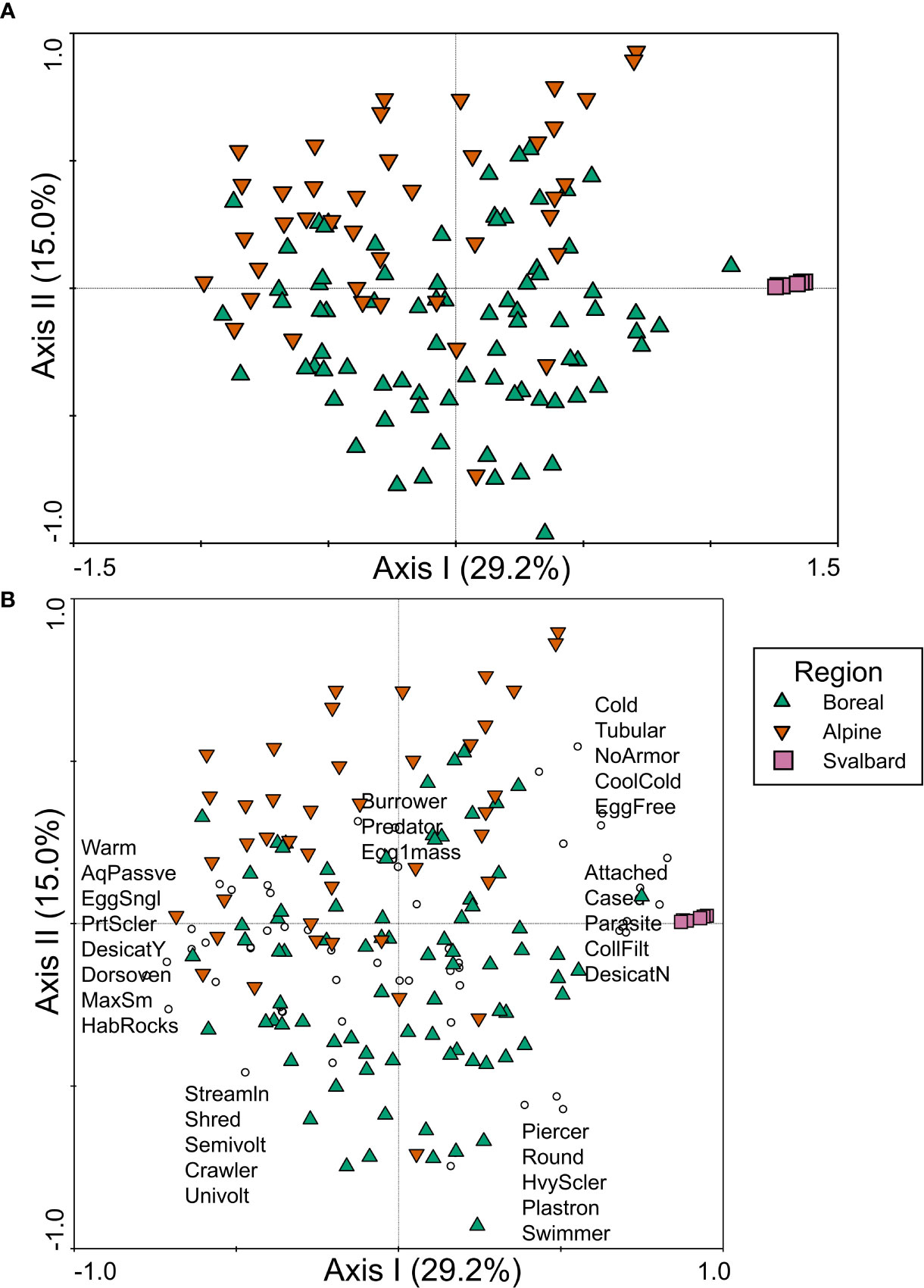
Figure 6 PCA ordination of trait relative abundance data for northwestern Europe, plotted as (A) samples and (B) samples and trait modalities, with symbol colors/shapes indicating the three regions sampled in Sweden and Norway (boreal ecoregion, alpine ecoregion, and Svalbard) and circles representing trait modalities. A subset of trait modality labels is presented in (B) in the approximate location of the trait modality points to ease interpretation of the figure. Trait modality abbreviations can be found in Table 1.
3.3 Spatial patterns in trait abundance and diversity
3.3.1 North America
Assessment of spatial patterns in traits for North America indicated significant differences among latitudes for a selection of trait modalities (Figure 7). The relative abundances of cold tolerance and tubular body shape were both significantly higher at 81°N than they were at 58°N or 63°N (Figures 7A, B). Trait modality relative abundance was also significantly lower at 58°N for tegument respiration, the burrower, crawler, and cased habits, and the no armor trait modality (p < 0.05; Supplementary Figure 1). These results indicated a predominance of cold-tolerant, soft-bodied, tubular, tegument-breathing organisms at higher latitudes, with burrowing and case-making habits as protection. The shredder feeding habit was also significantly lower at 58°N than at the other three latitudes (Figure 7C), a result that was contrary to expectations, given latitudinal shifts in riparian vegetation. However, when obligate shredders (taxa that were classified only as shredders) were isolated, there was a significantly lower relative abundance at 81°N than at other latitudes (Figure 7D), as organisms at the highest latitudes had multiple feeding habits. Other trait modalities were predominantly found at the lowest latitude sites, including streamlined and dorsoventrally flattened body shapes, partial sclerotization, gill respiration, swimmer habit, univoltine reproduction, desiccation intolerance, and passive aquatic and aerial life cycles (all trait modalities significantly higher at 58°N than other latitudes at α = 0.05; Supplementary Figure 2). These trait modalities were indicative of organisms with both armor and delicate respiration organs, a body shape and habits built for fast flows, and a lower tolerance for adverse conditions.
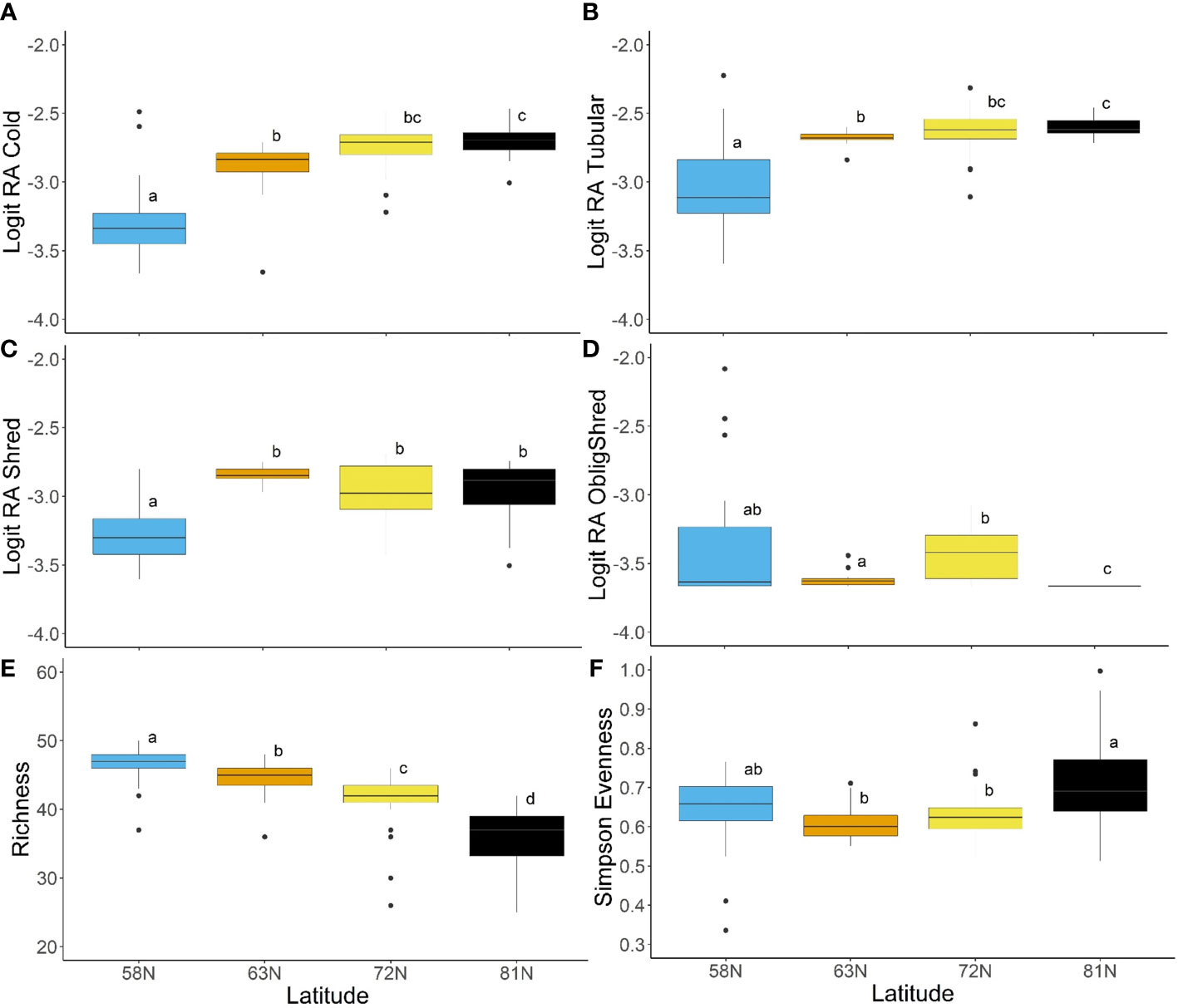
Figure 7 Box plots showing the results of Tukey HSD post hoc analysis (or Wilcoxon pairwise tests with Bonferroni correction for non-parametric analyses) comparing trait modalities and trait diversity metrics among latitude regions for North America, including (A) logit-transformed relative abundance of cold-tolerance, (B) logit-transformed relative abundance of tubular body shape, (C) logit-transformed relative abundance of shredder feeding habit, (D) logit-transformed relative abundance of obligate shredders, (E) trait richness, and (F) Simpson’s evenness index. Letters on plots indicate statistical significance at α = 0.05.
Diversity metrics provided clear evidence of latitudinal patterns in trait composition among North American rivers. In particular, trait richness was significantly different among all latitude groups, and showed a declining pattern towards the north (Figure 7E). Simpson diversity also declined with increasing latitude, though only 58°N and 63°N differed significantly from all other latitude groups (Supplementary Figure 2). Simpson evenness was significantly higher at 81°N than at 63°N or 72°N, but did not differ significantly from the lowest latitude (Figure 7F).
3.3.2 Northwestern Europe
Differences in the relative abundance of individual trait modalities among European sites were most evident when Svalbard was compared with the boreal and alpine regions (Figure 8). Svalbard sites had a significantly higher relative abundance of cold tolerance and tubular shape trait modalities compared to the lower latitude regions (Figures 8A, B). Similar patterns were found for multiple feeding habits (collector/gatherer, collector/filterer, scraper/grazer, and parasite), multivoltine reproduction, attached and cased habits, silt habitat preference, and the no armor trait modality (Supplementary Figure 3). The burrower habit and cool/cold temperature preference trait modalities were also significantly more abundant in Svalbard sites than in boreal sites (not significantly different from alpine sites; Supplementary Figure 3). Together, these traits indicated that Svalbard was dominated by cold-tolerant, tubular-shaped, soft-bodied organisms with burrowing and cased habits for protection, similar to what was found for 81°N in Canada. In contrast to patterns observed in the North American data, there was a significantly higher relative abundance of swimmer and desiccation intolerance trait modalities at the high latitude Svalbard sites (Supplementary Figure 3). Also in contrast to North America, the European data indicated significant differences in the relative abundance of the shredder trait between all regions, with the highest relative abundance in the boreal region and the lowest in Svalbard (Figure 8C). This pattern reflected the expectation that the shredder habit would be less predominant at high latitudes where riparian vegetation is limited or absent. When only obligate shredders were examined, there was a similar pattern of significantly lower relative abundance at Svalbard sites, though boreal and alpine sites were no longer significantly different (Figure 8D). Other trait modalities that were significantly less abundant in Svalbard sites included maximum small size (< 10 mm), streamlined and dorsoventrally flattened body shapes, partial and heavy sclerotization, crawler habit, active aquatic and aerial life stages, univoltine and semivoltine reproduction, single eggs, and atmospheric respiration (Supplementary Figure 4). For many of these traits, there was no significant difference between boreal and alpine sites, which indicated a general similarity of the trait composition of these regions.
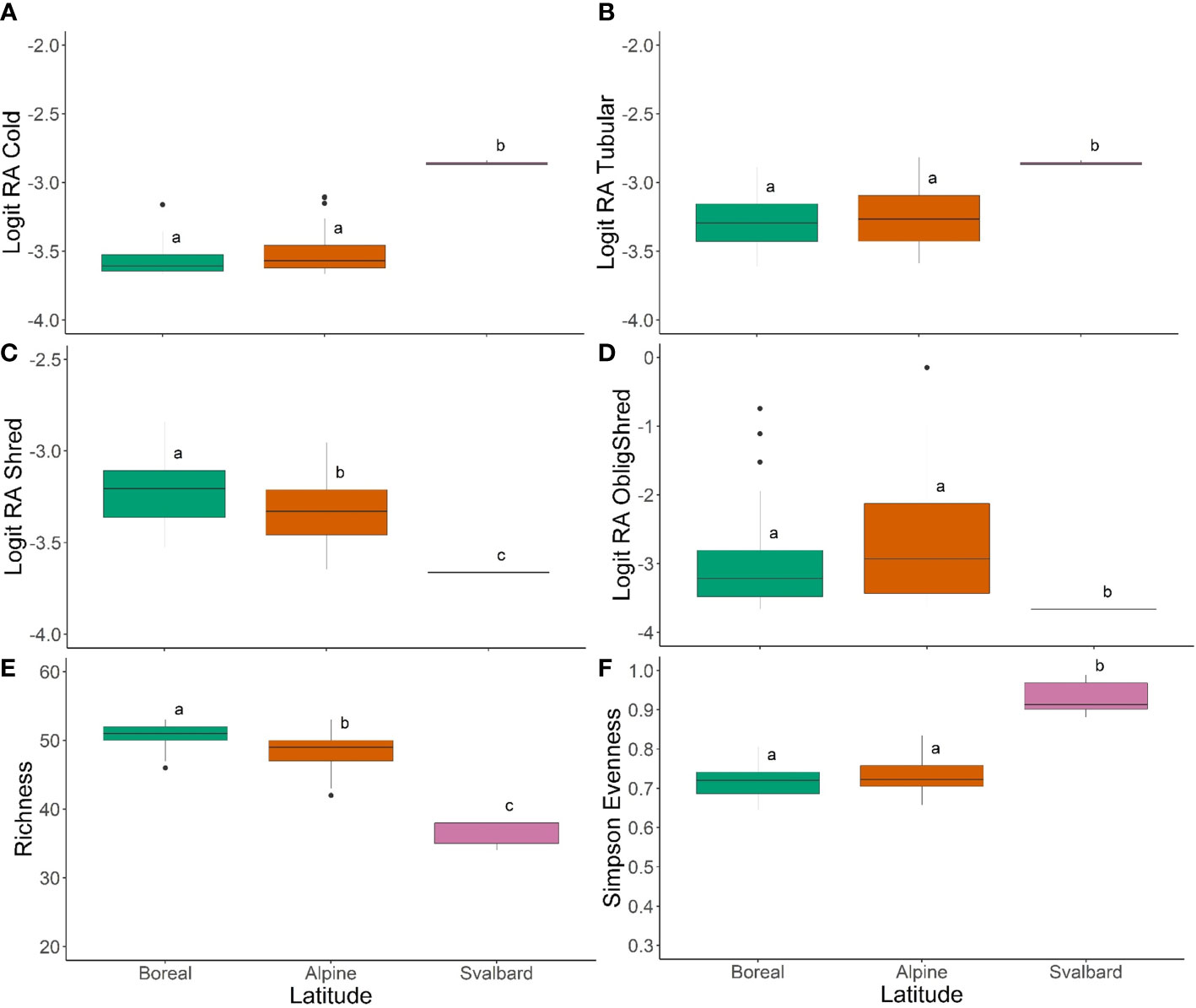
Figure 8 Box plots showing the results of Wilcoxon pairwise tests with Bonferroni correction comparing trait modalities and trait diversity metrics among regions for northwestern Europe, including (A) logit-transformed relative abundance of cold-tolerance, (B) logit-transformed relative abundance of tubular body shape, (C) logit-transformed relative abundance of shredder feeding habit, (D) logit-transformed relative abundance of obligate shredders, (E) trait richness, and (F) Simpson’s evenness index. Letters on plots indicate statistical significance at α = 0.05.
Comparison of trait diversity metrics among regions provided evidence of lower diversity of traits in Svalbard compared to the lower latitude regions. Trait richness was significantly different among all three regions, and was highest on average in boreal sites and lowest in Svalbard sites (Figure 8E). Simpson diversity did not differ between alpine and Svalbard sites, but was significantly higher on average in boreal sites (Supplementary Figure 4). In contrast, trait evenness was significantly higher in Svalbard sites than in boreal or alpine sites (Figure 8F), which likely reflected the low taxonomic diversity of Svalbard streams.
3.4 Trait patterns in relation to latitude and temperature
Trait data from North America and Europe were plotted together to look more broadly at trends in relation to latitude and temperature across both continents, but patterns in relation to latitude were slightly weaker due to the high variability in boreal and alpine sites in Sweden (Supplementary Figure 5). Boreal and alpine sites covered a similar latitudinal range, but elevational differences among sites were instead expected to contribute to variation in trait composition. To better capture this variation while also accounting for differences among latitudinal regions in North America, we examined the relationship of traits with historical maximum summer air temperatures for each site. For North American sites, latitude was strongly related to long-term average maximum August air temperature (linear regression slope = −0.29, R2 = 0.929; Supplementary Figure 6). The relationship was more variable for European sites, but indicated warmer temperatures than North America at the lowest latitudes and a sharper decline in temperature with increasing latitude (slope = −0.45, R2 = 0.677; Supplementary Figure 6).
Several trait modalities and trait diversity metrics were strongly associated with temperature for both North America and Europe, and the slope of the relationship with temperature was similar among continents in several cases. In particular, the relative abundance of cold tolerance decreased significantly with increasing air temperature in North America and Europe (Table 2 and Figure 9A). The 95% confidence intervals for the slopes of the regressions of cold tolerance on temperature overlapped for North America and Europe, which indicated that there was no significant difference in regression slopes between continents (Table 2 and Figure 9B). Similar, albeit weaker, patterns were found with the tubular shape (Table 2 and Figure 9B), burrower habit and no armor trait modalities (Supplementary Figure 7), consistent with the suggestion that these traits were predominant at cold, high latitude sites. The relative abundance of shredders declined significantly with increasing temperature in the European data, but increased significantly with increasing temperature in the North American data (Table 2 and Figure 9C). Inconsistencies in how this trait was assigned by North American and European trait databases likely contributed to differences in this pattern between sites from each continent, and an overall weak relationship (R2Europe = 0.240, R2North America = 0.144). When the relative abundance of obligate shredders was regressed on temperature (using rank regression), there was more consistency among continents (95% confidence intervals indicated no significant difference among continents), although the pattern of increasing abundance with increasing temperature was weak (R2North America = 0.042, R2Europe = 0.038) and the slopes were not significantly different from 0 (Table 2 and Figure 9D). The weak patterns for obligate shredders likely reflected variability at lower latitudes for both continents and the small sample size on Svalbard for Europe.
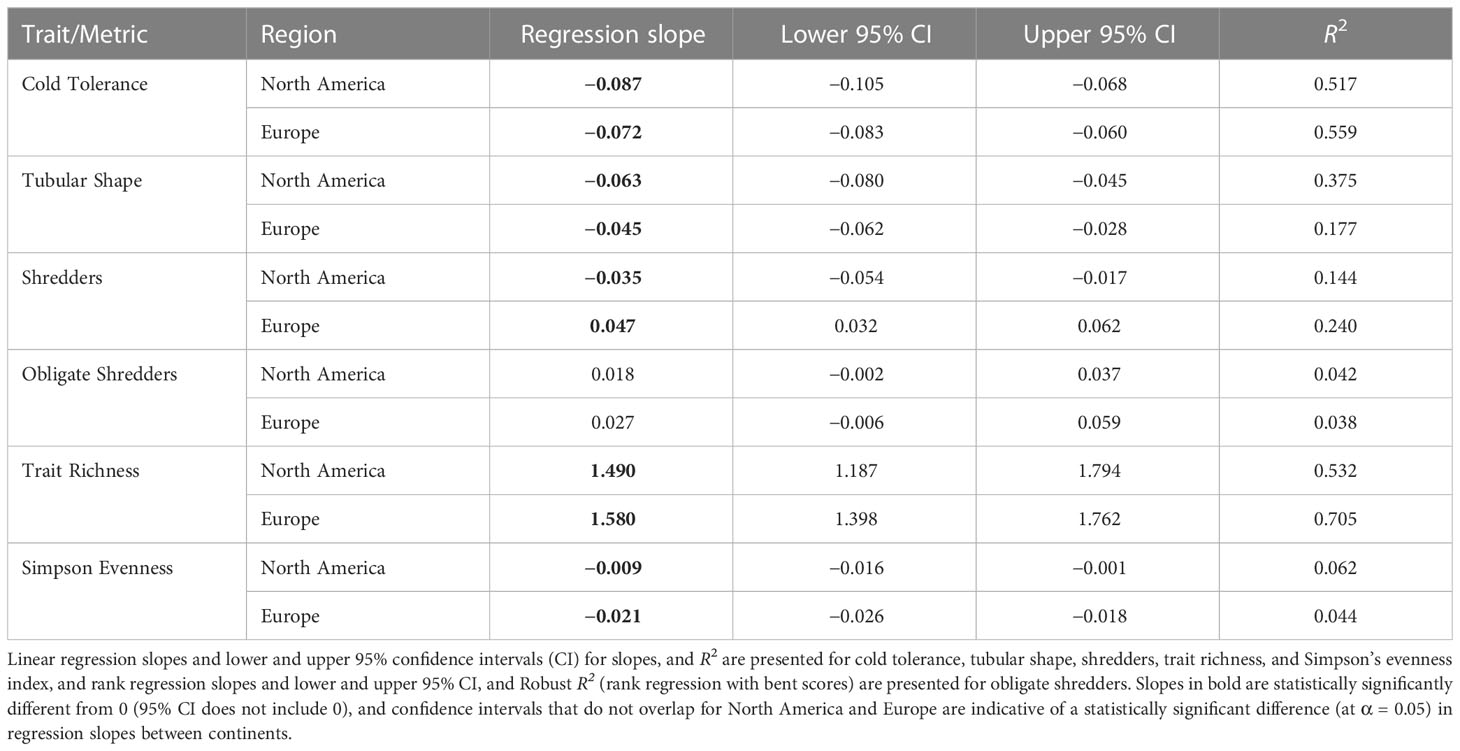
Table 2 Results of regressions of traits/metrics as a function of long-term average maximum August air temperature (a proxy for summer water temperature) using data for North America and Europe.
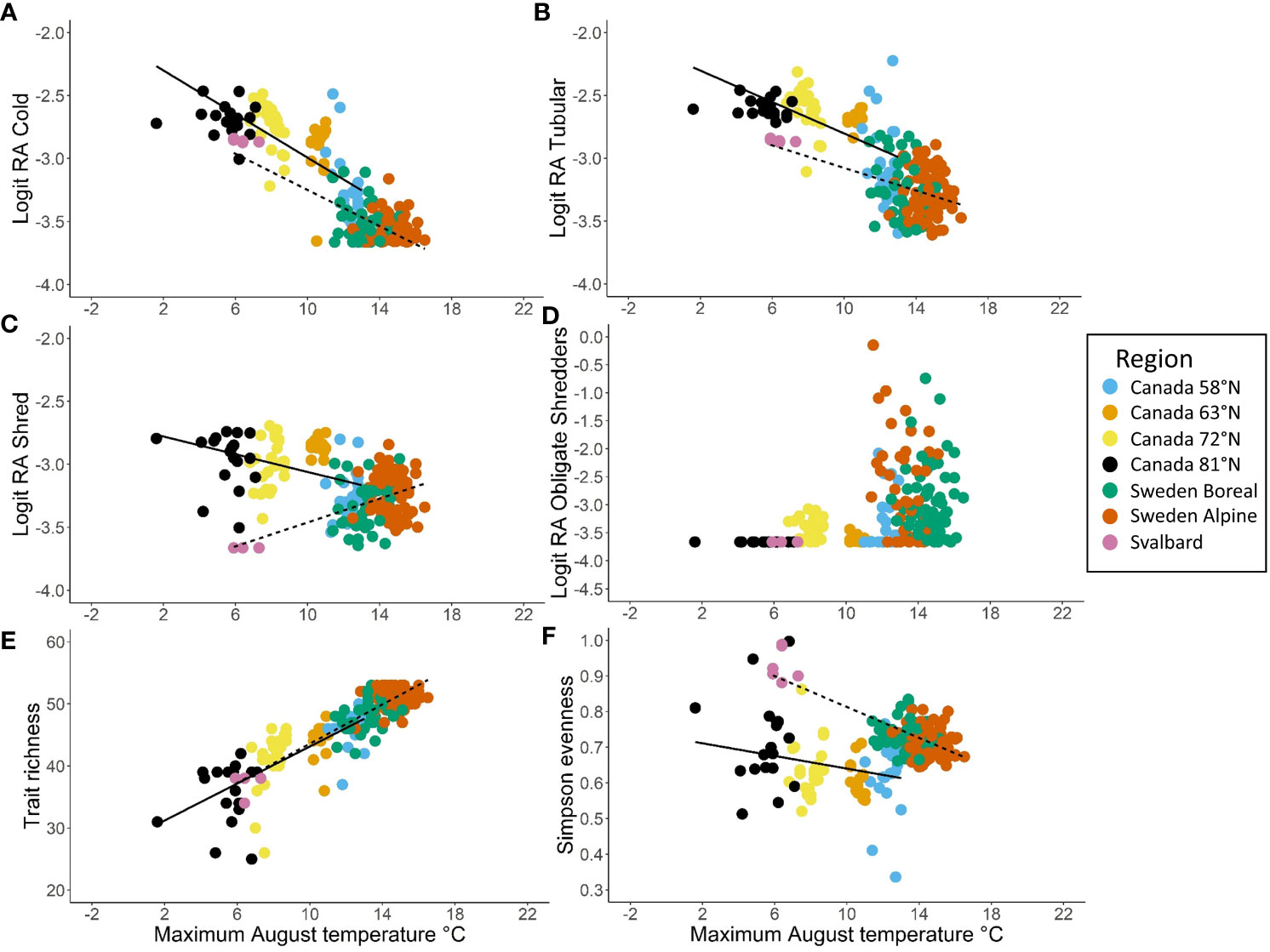
Figure 9 Trait modalities and trait diversity metrics plotted as a function of long-term average (1970–2000) maximum August temperature (°C) with separate simple linear regression lines for North America (solid) and northwestern Europe (dashed), including (A) logit relative abundance of cold tolerance, (B) logit relative abundance of tubular shape, (C) logit relative abundance of shredder feeding habit, (D) logit relative abundance of obligate shredders, (E) trait richness, and (F) Simpson evenness. Regression lines are not plotted in (D) because the obligate shredder trait was fit with rank regressions for both North America and Europe. Regions within North America and northwestern Europe are indicated by the colors of the points.
Trait richness showed the strongest relationship with temperature (R2North America = 0.532, R2Europe = 0.705), increasing significantly along the gradient of increasing temperature with a similar slope in each continent (Table 2 and Figure 9E). A similar relationship was evident when Simpson diversity was regressed on temperature (Supplementary Figure 7). In contrast, differences in Simpson evenness between North America and Europe resulted in a weak, negative relationship with temperature that differed in slope between continents (Table 2; Figure 9F).
4 Discussion
The trait composition of Arctic freshwater macroinvertebrate assemblages in eastern North America and northwestern Europe reflected a greater diversity of trait modalities at low latitudes/warmer sites and a reduced trait pool at high latitudes/colder sites that was characterized by adaptations related to extreme cold temperatures and a short growing season. There was no evidence of trait modalities that were restricted only to high latitudes, but the depauperate trait composition of high latitude assemblages was unique because of the absence of specific trait modalities – including many traits that were predominant at low latitudes. With increasing latitude and decreasing temperature, the relative abundance of the obligate shredder feeding habit decreased, while that of cold tolerance and protection traits such as case-making and burrower habits increased. These findings are consistent with our predictions of temperature-related gradients in trait composition. Moreover, the observed strong relationship of trait modalities with air temperature implies that Arctic warming and landscape greening will shift the contemporary, unique trait composition of the cold sites toward increased dominance of traits more specific to warm and/or forested landscape sites. The magnitude of this shift, however, will depend on the extent to which connectivity allows for northward movement of taxa (Lento et al., 2022).
4.1 FTNs and Arctic trait composition
Our analysis of Arctic taxon trait composition identified distinct FTNs for several different taxonomic groups, consistent with earlier studies that have suggested that different trait niches are filled by different taxa (Usseglio-Polatera et al., 2000; Poff et al., 2006; Schmera et al., 2022). Coarse taxonomic groupings (e.g., order or higher) can be distinguished on the basis of their FTNs in part due to phylogenetic constraints that are represented by particular combinations of trait modalities that are shared by closely-related taxa (Poff et al., 2006; Schmera et al., 2022). This co-occurence of trait modalities helps identify the ecological strategy of the taxon and the habitats in which it can thrive (Poff et al., 2006; Schmera et al., 2017; Schmera et al., 2022). For example, taxa that share adaptations such as freeze tolerance, generalist feeding, and protection from exposure all have a good ability to survive in Arctic freshwater systems (Danks, 2004).
In our study, the FTNs of Ephemeroptera and Plecoptera were distinct from those of Diptera and Oligochaeta, and physiological traits, such as cold tolerance, body shape, respiration mode, protection, and feeding habit (which relates to mouthparts), contributed strongly to those differences. Ephemeroptera and Plecoptera are not generally found at high latitudes (Culp et al., 2019; Lento et al., 2022), which suggests that their FTN (characterized by blocky, streamlined, or dorsoventrally-flattened bodies; gills; and shredder feeding habit) does not align with the trait adaptations required for high latitude freshwater ecosystems. In contrast, the FTN of Diptera and Oligochaeta, which included cold-tolerance, tubular body shape, and cased and burrower habits, appears to have allowed these taxa to dominate high latitude ecosystems (Lento et al., 2022). These traits are consistent with the required adaptations for survival in arctic conditions as outlined by Danks (2004). The FTN of Trichoptera was similar to that of Ephemeroptera and Plecoptera in the PCoA, but also shared a number of trait modalities with Diptera taxa. Although Trichoptera are absent in streams on Svalbard (Milner et al., 2001; Lods-Crozet et al., 2007) and Ellesmere Island (Culp et al., 2019), the trichopteran Apatania zonella is present in deep, permanent lakes on Svalbard (Coulson et al., 2014; Chertoprud et al., 2017). This example of a pioneering trichopteran taxa in high Arctic lakes may relate to the fact that lakes and their outflows are sites where heat is stored, and thus form hotspots for range extension in Arctic landscapes.
Strong similarities in FTNs among taxonomic groups indicated a high degree of functional redundancy for some taxa. Trait composition for Ephemeroptera and Plecoptera taxa showed substantial overlap, largely due to similarities in body shape, respiration mode, and feeding habits. This redundancy in trait composition suggests that Ephemeroptera and Plecoptera fill a similar niche, and that the functional loss of one taxon may be compensated for by functionally redundant taxa within these groups (Schmera et al., 2012; Schmera et al., 2017). Diptera and Oligochaeta taxa also had a number of traits in common, largely due to similarities in physiology and habit. Functional redundancy was also evident among several taxa in the larger taxonomic pool of the European sites. Such functionally redundant taxa are unlikely to exhibit complete overlap with the niche of other taxa due to a less than perfect redundancy (i.e., similarities in many, but not all trait modalities), or differences in food and habitat resource use (Loreau, 2004); however, assemblages with a higher degree of functional redundancy are expected to be more resilient to environmental change (Schmera et al., 2012; Brown et al., 2018). Where environmental changes support the introduction of traits that were previously incompatible with the habitat (e.g., cold intolerance, obligate shredder feeding habit), these may be accompanied by an increase in functional diversity that subsequently may affect functional redundancy (Bruno et al., 2019).
4.2 Spatial variation in traits
The predominant pattern in trait composition in both North America and Europe was a loss of trait modalities at the highest latitudes and coldest sites that reflected the loss of EPT and other taxonomic groups. Taxonomic richness of stream benthic macroinvertebrates is lowest at high latitudes (Lento et al., 2022), and streams on Ellesmere Island in North America and on Svalbard in Europe are known to be devoid of EPT taxa and predominantly or entirely inhabited by Diptera and Oligochaeta taxa (Lods-Crozet et al., 2007; Culp et al., 2019). Lento et al. (2022) emphasized that nestedness was the predominant component of taxonomic beta diversity in comparisons of stream macroinvertebrate assemblages from regions at high and low latitudes in the Arctic, which indicated a loss of taxa at high latitudes rather than a change in taxa. Similarly, our results showed a loss of several trait modalities at high latitudes (e.g., modalities from the FTN occupied by EPT taxa) and a compensatory increase in relative abundance of trait modalities of the remaining taxa (e.g., modalities from the FTN occupied by Diptera and Oligochaeta). These results reflect the strong environmental filters for the traits that are found at high latitudes.
Differences in trait composition among lower latitude sites were more subtle. For example, sites at 63°N and 72°N in North America often had a similar relative abundance of traits to those observed at either 58°N or 81°N, indicating a gradient of change along these latitudes. The small differences between Northern Boreal and Arctic/Alpine mainland sites in Europe were likely due to the fact that latitudinal gradients were obscured by elevational gradients in these regions. However, even when trait patterns were related to temperature instead of latitude, there was a high degree of similarity among European sites that likely reflected the strong connectivity between these regions, as rivers drain west-to-east from the Scandinavian mountains to the Baltic Sea. The strong similarity in trait composition between the Arctic/Alpine and Northern Boreal regions in Europe suggests that range expansion of species with continued warming will occur quickly in these highly connected regions, resulting in rapid changes to trait composition (particularly in the more species-poor Arctic/Alpine region), and potential homogenization of trait composition across regions. Conversely, this suggests that the northward movement of species to Svalbard and in the Canadian Arctic Archipelago will be delayed due to strong spatial discontinuity, and may even be impossible for poor dispersers. These patterns highlight the need to incorporate spatial connectivity when making predictions about how trait composition may change in the future.
4.3 Environmental drivers of trait composition
Spatial patterns in trait composition were clearly associated with temperature gradients across our study area. Long-term average maximum summer air temperature explained a large amount of the variability in trait richness and in the relative abundance of several trait modalities. Cold temperatures were associated with lower trait richness and a higher relative abundance of cold tolerance, tubular body shape, and cased and burrower habits, reflecting the FTN of taxa adapted to arctic conditions. The trait modalities that predominated in our high Arctic stream sites were consistent with Danks (2004) predictions of requirements for Arctic survival, including physiological adaptations to survive cold temperatures and a short growing season, and cocoons and cases to offer protection from freezing and from mechanical damage caused by ice formation and movement. The low trait richness of the coldest sites suggests that low temperatures select for particular FTNs rather than promoting diversity of FTNs, leading to a reduced pool of traits. With continued warming, the relative abundance of Arctic-specific FTNs in cold regions is likely to be reduced as trait diversity increases.
Functional feeding groups, and in particular the shredder feeding habit, were predicted to vary along our latitudinal and climatic gradients due to the low or nonexistent riparian vegetation cover above the treeline. Indeed, Johnson et al. (2004) showed a decline in shredding stream taxa between the Northern Boreal and Arctic/Alpine ecoregions, while that for lakes was similar. However, analysis of North American trait data indicated an increase in the relative abundance of shredders at higher latitudes due to the assignment of Chironomidae as shredders. While this partially reflects the nature in which traits were assigned to taxa (using a multistate approach, and thus assigning Chironomidae to multiple feeding habits as supported by observations in the trait database), it also speaks to the importance of trait plasticity under extreme conditions (Hamilton et al., 2020). Because of the short growing season, organisms at high latitudes and cold regions are generalist feeders with multiple feeding habits, and their food source and mode of feeding may differ depending on local conditions. For example, Chételat et al. (2010) assessed feeding habits of chironomids in high Arctic lakes through gut content analysis and stable isotopes and compared their findings with other chironomid diet studies in the literature. Their results showed that many chironomid taxa were generalist feeders, and that the dominant carbon source for chironomids varied regionally across the Arctic, with a lower contribution of terrestrial carbon in the high Arctic than in Alaska. The generalist feeding adaptation allows for opportunistic feeding in regions such as the high Arctic, where riparian vegetation and detritus inputs are generally lacking. In our study, only a small subset of taxa were classified exclusively as shredders in trait databases, and these obligate shredder taxa were lost at the highest latitudes, a finding that was consistent with our prediction that vegetation would be a main driver of trait composition.
Attributing spatial patterns in trait composition to temperature and vegetation patterns along our latitudinal gradients is complicated by the lack of spatial connectivity on Arctic islands (Lento et al., 2022). While shifts in trait composition in relation to temperature and other drivers are facilitated by strong connectivity between the Arctic/Alpine and Northern Boreal regions in Sweden, dispersal across physical barriers and among islands is more limited. For example, Brittain et al. (2022) noted that taxonomic diversity of stream macroinvertebrates in western Fennoscandia was limited by the mountains bordering Sweden and Norway. Also, Lento et al. (2022) observed lower taxonomic diversity of macroinvertebrates in Arctic lakes on islands regardless of latitude, which suggested a strong effect of spatial discontinuity in those systems. While aerial dispersal of insects over hundreds of kilometers has been noted in the Arctic (see Danks, 2004 and references therein), the isolation of Arctic islands likely plays a large role in the observed low trait diversity at higher latitudes.
4.4 Implications of climate change for trait composition and food webs
Climate change is causing increases in temperatures (IPCC, 2021) and changes to the timing, magnitude, and type of precipitation in the Arctic (Bintanja and Andry, 2017; Hanssen-Bauer et al., 2019; Pedersen et al., 2022), which will have both direct and indirect effects on the structure and function of freshwater communities (Heino et al., 2020; Woolway et al., 2022; Milner et al., 2023). Warming temperatures and changes to the precipitation regime will alter the abiotic template (Huser et al., 2022) and the environmental filters that select for traits in Arctic freshwater food webs. For example, Milner et al. (2023) observed shifts in taxonomic composition of Arctic streams sampled over a period of more than 20 years that were related to changes in air temperature, precipitation, and depth of the snowpack. Changes in trait composition in relation to warming temperatures and increased precipitation could include an increase in the number of organisms with a weaker cold tolerance, and an increase in the abundance of desiccation intolerant organisms (Danks, 2004). Overall, trait diversity may increase, as was observed in alpine systems in the Alps that were sampled over a 40-year period (Bruno et al., 2019). However, the complex and interacting nature of predicted ecosystem changes in the Arctic complicates the prediction of expected functional changes, and the indirect effects of permafrost thaw (Chin et al., 2016), glacial retreat and subsequent changes in water source (Milner et al., 2017), vegetation greening (Jia et al., 2009; Myers-Smith et al., 2011), and browning of freshwaters (Finstad et al., 2016; Hayden et al., 2019) may have variable impacts on trait composition and food web function.
With continued warming and permafrost thaw, ancient particulate carbon is remobilized and can become an additional direct food resource for Arctic stream consumers, including filter feeders and grazers (Hågvar et al., 2016; Kendrick et al., 2018). In addition, microbial processing of ancient dissolved carbon by benthic biofilms can subsequently be assimilated by grazers (Hågvar et al., 2016; Kendrick et al., 2018; Tanentzap et al., 2021). Such carbon remobilization and microbial processing thus implies an additional trophic transfer pathway for high-latitude streams, particularly so for filter-feeders and grazers. This means that changes in benthic macroinvertebrate trait compositions resulting from warming and increases in riparian vegetation will also be accompanied by fundamental changes in the trophic dynamics of the Arctic stream food webs.
Our finding that obligate shredders are largely absent at very high latitudes and that their relative abundances increase along with increased development of riparian vegetation at lower latitudes has important implications for the food webs of streams. These implications originate from the findings that leaf-shredding invertebrates are generally lower in polyunsaturated fatty acids (PUFA) than grazers, which feed on PUFA-rich algae (Lau et al., 2012; Guo et al., 2021), and thus have lower food quality for invertebrate predators and fish than do grazers. Shredding on terrestrial leaves implies a less efficient trophic transfer from basal resources to fish than grazing (Brett et al., 2017; Grieve and Lau, 2018). Although shredders generally contain less PUFA than grazers, shredders are able to assimilate PUFA from the complex biofilms that grow on conditioned plant litter (Guo et al., 2016), which provides an additional trophic pathway for PUFA to predators. Also, increases in riparian vegetation development and shading are expected to reduce the light stress for the benthic algal community and stimulate the production of benthic diatoms that are rich in PUFA (Guo et al., 2015), which promote overall trophic transfer efficiency and consumer production (Lau et al., 2014; Brett et al., 2017). Thus, along with increased vegetation development (Elmendorf et al., 2012) and the continued greening of the Arctic landscapes (Myers-Smith et al., 2011; Jenkins et al., 2020), the northward range expansion of obligate shredders from southern warmer regions will not only enhance the functional diversity but also introduce a new detrital trophic pathway for stream food webs in the Arctic.
4.5 Conclusions and future needs
Our cross-continental study shows that major changes in FTNs occur in a similar way along different latitudinal gradients in the Arctic regions and that these changes are primarily driven by the loss of obligate shredders and the increases of cold-adapted taxa at the very northern latitudes. However, we found no evidence of trait modalities that were restricted only to high latitudes, but instead found that the trait composition of high latitude/cold region assemblages was unique because of the absence of traits that were specific to the warmer southern regions. We identified climate-change driven landscape alterations, such as the development of riparian vegetation and permafrost thaws, as key processes that modify trait compositions of benthic invertebrates and their associated food webs. The finding that trait modalities correlated strongly with air temperature implies that Arctic warming and landscape greening will shift the unique trait composition of the cold Arctic sites toward increased dominance of traits more specific to warmer and/or forested landscapes. These shifts are expected to be facilitated by the increased spatial connectivity of continental landscapes, and hindered by isolated islands and archipelagos.
Large-scale, continental studies of functional traits require harmonized data and a coordinated approach to assigning trait information (Kunz et al., 2022). To date, such a harmonized trait database that can be applied across multiple continents is lacking. Existing trait databases differ in taxa, trait modalities/definitions for the same traits, and methods for assigning traits to taxa (Kunz et al., 2022). As a result, researchers are left with the challenge of combining data across databases or, as in this study, harmonizing trait categories and trait modalities while conducting separate analyses by continent. Even with the applied harmonization of trait modalities in our study, there were inconsistencies in some of the latitudinal trait patterns between North America and Europe that may be a result of how traits were assigned in each trait database. For Arctic studies, a further challenge is the lack of databases that contain observations from Arctic freshwaters. Trait plasticity in extreme environments may not be captured by trait information in existing databases (Hamilton et al., 2020), and previous studies have identified adaptations of organisms living in the Arctic, such as extended life cycles (Butler, 1982), generalist feeding (Chételat et al., 2010), or extreme cold tolerance (Milner et al., 2001; Danks, 2004). We began to address this issue by assigning a cold tolerance trait to our data that was based on observations from glacial systems, but more work is needed to develop a harmonized, circumpolar, Arctic-relevant trait database to facilitate future large-scale assessments.
Data availability statement
The raw data supporting the conclusions of this article will be made available by the authors, without undue reservation.
Author contributions
JL, DL, JC, and WG initiated and designed the study. JB, JC, and WG contributed data. JL and DL harmonized data, conducted data analysis, and created tables and figures. JL, DL, JB, JC, and WG contributed to writing/editing paper. All authors contributed to the article and approved the submitted version.
Funding
The authors acknowledge the EU-BioDiversa/Belmont Forum funded project Arctic-BIODIVER, an interdisciplinary, international research program focused on filling gaps in Arctic freshwater biodiversity knowledge, and Canada’s Arctic BioNet program of the International Polar Year. We also acknowledge national funders in Canada and Sweden, i.e. the Swedish Research Council for the Environment, Agricultural Sciences and Spatial Planning (FORMAS; projects 2018-02432 and 2021-01062), Canada’s Natural Science and Engineering Research Council, and Environment and Climate Change Canada (ECCC). Canada’s Polar Continental Shelf Project provided transportation and logistical support in Ellesmere and Baffin Islands. We also thank the Swedish Environmental Protection Agency for funding national surveys of inland waters in the past. Sampling in Svalbard was supported by funding from the Norwegian Environment Agency and the EU-funded Arctic and Alpine Ecosystem Research project (AASER).
Acknowledgments
We are grateful for the superb field and technical assistance of K. Heard, E. Luiker and D. Hryn (ECCC), D. Cote, A. Simpson, T. Knight and T. Sheldon (Torngat Mountains National Park Reserve); A. Maher and C. Elverum (Sirmilik National Park); R. Glenfield (Quttinirpaaq National Park); J. Sweetman and D. McLennan (Parks Canada); and to northern community members who served as bear monitors for our field crews.
Conflict of interest
The authors declare that the research was conducted in the absence of any commercial or financial relationships that could be construed as a potential conflict of interest.
Publisher’s note
All claims expressed in this article are solely those of the authors and do not necessarily represent those of their affiliated organizations, or those of the publisher, the editors and the reviewers. Any product that may be evaluated in this article, or claim that may be made by its manufacturer, is not guaranteed or endorsed by the publisher.
Supplementary material
The Supplementary Material for this article can be found online at: https://www.frontiersin.org/articles/10.3389/fevo.2023.1209612/full#supplementary-material
References
Bintanja R., Andry O. (2017). Towards a rain-dominated arctic. Nat. Climate Change 7 (4), 263. doi: 10.1038/nclimate3240
Bis B., Usseglio-Polatera P. (2004). Species traits analysis: STAR deliverable N2 to the European Commission, 148 p.
Brett M. T., Bunn S. E., Chandra S., Galloway A. W. E., Guo F., Kainz M. J., et al. (2017). How important are terrestrial organic carbon inputs for secondary production in freshwater ecosystems? Freshw. Biol. 62 (5), 833–853. doi: 10.1111/fwb.12909
Brittain J. E., Heino J., Friberg N., Aroviita J., Kahlert M., Karjalainen S. M., et al. (2022). Ecological correlates of riverine diatom and macroinvertebrate alpha and beta diversity across Arctic Fennoscandia. Freshw. Biol. 67 (1), 49–63. doi: 10.1111/fwb.13616
Brown L. E., Khamis K., Wilkes M., Blaen P., Brittain J. E., Carrivick J. L., et al. (2018). Functional diversity and community assembly of river invertebrates show globally consistent responses to decreasing glacier cover. Nat. Ecol. Evol. 2 (2), 325. doi: 10.1038/s41559-017-0426-x
Bruno D., Belmar O., Maire A., Morel A., Dumont B., Datry T. (2019). Structural and functional responses of invertebrate communities to climate change and flow regulation in alpine catchments. Global Change Biol. 25 (5), 1612–1628. doi: 10.1111/gcb.14581
Butler M. G. (1982). A 7-year life cycle for two Chironomus species in arctic Alaskan tundra ponds (Diptera: Chironomidae). Can. J. Zool. 60 (1), 58–70. doi: 10.1139/z82-008
Chertoprud M. V., Palatov D. M., Dimante-Deimantovica I. (2017). Macrobenthic communities in water bodies and streams of Svalbard, Norway. J. Nat. Hist. 51 (47-48), 2809–2825. doi: 10.1080/00222933.2017.1395092
Chételat J., Cloutier L., Amyot M. (2010). Carbon sources for lake food webs in the Canadian High Arctic and other regions of Arctic North America. Polar Biol. 33 (8), 1111–1123. doi: 10.1007/s00300-010-0797-9
Chin K. S., Lento J., Culp J. M., Lacelle D., Kokelj S. V. (2016). Permafrost thaw and intense thermokarst activity decreases abundance of stream benthic macroinvertebrates. Global Change Biol. 22 (8), 2715–2728. doi: 10.1111/gcb.13225
Commission for Environmental Cooperation Working Group (1997). Ecological regions of North America - toward a common perspective Vol. 71 (Montreal, Canada: Comission for Environmental Cooperation).
Coulson S. J., Convey P., Aakra K., Aarvik L., Ávila-Jiménez M. L., Babenko A., et al. (2014). The terrestrial and freshwater invertebrate biodiversity of the archipelagoes of the Barents Sea; Svalbard, Franz Josef Land and Novaya Zemlya. Soil Biol. Biochem. 68, 440–470. doi: 10.1016/j.soilbio.2013.10.006
Culp J. M., Armanini D. G., Dunbar M. J., Orlofske J. M., Poff N. L., Pollard A. I., et al. (2011). Incorporating traits in aquatic biomonitoring to enhance causal diagnosis and prediction. Integr. Environ. Assess. Manage. 7 (2), 187–197. doi: 10.1002/ieam.128
Culp J. M., Lento J., Curry R. A., Luiker E., Halliwell D. (2019). Arctic biodiversity of stream macroinvertebrates declines in response to latitudinal change in the abiotic template. Freshw. Sci. 38 (3), 465–479. doi: 10.1086/704887
Culp J. M., Lento J., Goedkoop W., Power M., Rautio M., Christoffersen K. S., et al. (2012). Developing a circumpolar monitoring framework for Arctic freshwater biodiversity. Biodiversity 13 (3-4), 215–227. doi: 10.1080/14888386.2012.717526
Danks H. V. (2004). Seasonal adaptations in arctic insects. Integr. Comp. Biol. 44 (2), 85–94. doi: 10.1093/icb/44.2.85
Elmendorf S. C., Henry G. H., Hollister R. D., Björk R. G., Boulanger-Lapointe N., Cooper E. J., et al. (2012). Plot-scale evidence of tundra vegetation change and links to recent summer warming. Nat. Climate Change 2 (6), 453. doi: 10.1038/nclimate1465
Environment Canada (2012). Canadian aquatic biomonitoring network field manual - wadeable streams Vol. 57 (Dartmouth, NS, Canada: Government of Canada Publications).
Fick S. E., Hijmans R. J. (2017). Worldclim 2: New 1-km spatial resolution climate surfaces for global land areas. Int. J. Climatology (online version) 37 (12), 4302–4315. doi: 10.1002/joc.5086
Finstad A. G., Andersen T., Larsen S., Tominaga K., Blumentrath S., De Wit H. A., et al. (2016). From greening to browning: Catchment vegetation development and reduced S-deposition promote organic carbon load on decadal time scales in Nordic lakes. Sci. Rep. 6, 31944. doi: 10.1038/srep31944
Fox J., Weisberg S. (2019). An {R} Companion to applied regression, third edition (Thousand Oaks, CA: Sage).
Goedkoop W., Culp J. M., Christensen T., Christoffersen K. S., Fefilova E., Guðbergsson G., et al. (2022). Improving the framework for assessment of ecological change in the Arctic: A circumpolar synthesis of freshwater biodiversity. Freshw. Biol. 67 (1), 210–223. doi: 10.1111/fwb.13873
Gower J. C. (1971). A general coefficient of similarity and some of its properties. Biometrics 27, 857–871. doi: 10.2307/2528823
Grieve A., Lau D. C. (2018). Do autochthonous resources enhance trophic transfer of allochthonous organic matter to aquatic consumers, or vice versa? Ecosphere 9 (6), e02307. doi: 10.1002/ecs2.2307
Guo F., Ebm N., Bunn S. E., Brett M. T., Hager H., Kainz M. J. (2021). Longitudinal variation in the nutritional quality of basal food sources and its effect on invertebrates and fish in subalpine rivers. J. Anim. Ecol. 90 (11), 2678–2691. doi: 10.1111/1365-2656.13574
Guo F., Kainz M. J., Sheldon F., Bunn S. E. (2015). Spatial variation in periphyton fatty acid composition in subtropical streams. Freshw. Biol. 60 (7), 1411–1422. doi: 10.1111/fwb.12578
Guo F., Kainz M. J., Valdez D., Sheldon F., Bunn S. E. (2016). High-quality algae attached to leaf litter boost invertebrate shredder growth. Freshw. Sci. 35 (4), 1213–1221. doi: 10.1086/688667
Gustafsson L., Ahlén I. (1996). Geography of plants and animals (Stockholm: Almqvist and Wiksell International).
Hågvar S., Ohlson M., Brittain J. E. (2016). A melting glacier feeds aquatic and terrestrial invertebrates with ancient carbon and supports early succession. Arctic Antarctic Alpine Res. 48 (3), 551–562. doi: 10.1657/AAAR0016-027
Hamilton A. T., Schäfer R. B., Pyne M. I., Chessman B., Kakouei K., Boersma K. S., et al. (2020). Limitations of trait-based approaches for stressor assessment: The case of freshwater invertebrates and climate drivers. Global Change Biol. 26 (2), 364–379. doi: 10.1111/gcb.14846
Hanssen-Bauer I., Førland E., Hisdal H., Mayer S., Sandø A., Sorteberg A. (2019). “Climate in Svalbard 2100. A knowledge base for climate adaptation,” in Norsk klimaservicesenter (NKSS)/norwegian centre for climate services (NCCS), vol. 206. (Oslo, Norway: Norwegian Centre for Climate Services (NCCS).
Hayden B., Harrod C., Thomas S. M., Eloranta A., Myllykangas J. P., Siwertsson A., et al. (2019). From clear lakes to murky waters–tracing the functional response of high-latitude lake communities to concurrent “greening” and “browning”. Ecol. Lett. 22 (5), 807–816. doi: 10.1111/ele.13238
Heino J., Culp J. M., Erkinaro J., Goedkoop W., Lento J., Rühland K. M., et al. (2020). Abruptly and irreversibly changing Arctic freshwaters urgently require standardized monitoring. J. Appl. Ecol. 57, 1192–1198. doi: 10.1111/1365-2664.13645
Hothorn T., Bretz F., Westfall P. (2008). Simultaneous inference in general parametric models. Biometrical J. 50 (3), 346–363. doi: 10.1002/bimj.200810425
Huser B. J., Futter M. N., Bogan D., Brittain J. E., Culp J. M., Goedkoop W., et al. (2022). Spatial and temporal variation in Arctic freshwater chemistry - Reflecting climate-induced landscape alterations and a changing template for biodiversity. Freshw. Biol. 67 (1), 14–29. doi: 10.1111/fwb.13645
IPCC (2021). “Climate change 2021: the physical science basis,” in Contribution of working group I to the sixth assessment report of the intergovernmental panel on climate change. Eds. Masson-Delmotte V., Zhai P., PIrani A., Connors S. L., Péan C., Berger S., Caud N., Chen Y., Goldfarb L., Gomis M. I., Huang M., Leitzell K., Lonnoy E., Matthews J. B. R., Maycock T. K., Waterfield T., Yelekçi O., Yu R., Zhou B. (London: Cambridge University Press).
Jenkins L. K., Barry T., Bosse K. R., Currie W. S., Christensen T., Longan S., et al. (2020). Satellite-based decadal change assessments of pan-Arctic environments. Ambio 49, 820–832. doi: 10.1007/s13280-019-01249-z
Jia G. J., Epstein H. E., Walker D. A. (2009). Vegetation greening in the Canadian Arctic related to decadal warming. J. Environ. Monit. 11 (12), 2231–2238. doi: 10.1039/b911677j
Johnson R. K., Goedkoop W., Sandin L. (2004). Spatial scale and ecological relationships between the macroinvertebrate communities of stony habitats of streams and lakes. Freshw. Biol. 49, 1179–1194. doi: 10.1111/j.1365-2427.2004.01262.x
Kendrick M. R., Huryn A. D., Bowden W. B., Deegan L. A., Findlay R. H., Hershey A. E., et al. (2018). Linking permafrost thaw to shifting biogeochemistry and food web resources in an arctic river. Global Change Biol. 24 (12), 5738–5750. doi: 10.1111/gcb.14448
Kloke J. D., McKean J. W. (2012). Rfit: rank-based estimation for linear models. R J. 4 (2), 57–64. doi: 10.32614/RJ-2012-014
Kunz S., Kefford B. J., Schmidt-Kloiber A., Matthaei C. D., Usseglio-Polatera P., Graf W., et al. (2022). Tackling inconsistencies among freshwater invertebrate trait databases: harmonising across continents and aggregating taxonomic resolution. Freshw. Biol. 67 (2), 275–291. doi: 10.1111/fwb.13840
Lau D. C. P., Christoffersen K. S., Erkinaro J., Hayden B., Heino J., Hellsten S., et al. (2022). Multitrophic biodiversity patterns and environmental descriptors of sub-Arctic lakes in northern Europe. Freshw. Biol. 67 (1), 30–48. doi: 10.1111/fwb.13477
Lau D. C., Sundh I., Vrede T., Pickova J., Goedkoop W. (2014). Autochthonous resources are the main driver of consumer production in dystrophic boreal lakes. Ecology 95 (6), 1506–1519. doi: 10.1890/13-1141.1
Lau D. C., Vrede T., Pickova J., Goedkoop W. (2012). Fatty acid composition of consumers in boreal lakes–variation across species, space and time. Freshw. Biol. 57 (1), 24–38. doi: 10.1111/j.1365-2427.2011.02690.x
Lento J., Culp J. M., Levenstein B., Aroviita J., Baturina M. A., Bogan D., et al. (2022). Temperature and spatial connectivity drive patterns in freshwater macroinvertebrate diversity across the Arctic. Freshw. Biol. 67 (1), 159–175. doi: 10.1111/fwb.13805
Lento J., Goedkoop W., Culp J., Christoffersen K., Fefilova E., Guðbergsson G., et al. (2019). State of the arctic freshwater biodiversity report Vol. 124 (Akureyri, Iceland: Conservation of Arctic Flora and Fauna (CAFF) International Secretariat).
Lods-Crozet B., Lencioni V., Brittain J. E., Marziali L., Rossaro B. (2007). Contrasting chironomid assemblages in two high Arctic streams on Svalbard. Fundam. Appl. Limnolgy Archiv für Hydrobiologie 170 (3), 211–222. doi: 10.1127/1863-9135/2007/0170-0211
Loreau M. (2004). Does functional redundancy exist? Oikos 104 (3), 606–611. doi: 10.1111/j.0030-1299.2004.12685.x
Martini S., Larras F., Boyé A., Faure E., Aberle N., Archambault P., et al. (2021). Functional trait-based approaches as a common framework for aquatic ecologists. Limnol. Oceanogr. 66 (3), 965–994. doi: 10.1002/lno.11655
Milner A. M., Brittain J. E., Castella E., Petts G. E. (2001). Trends of macroinvertebrate community structure in glacier-fed rivers in relation to environmental conditions: a synthesis. Freshw. Biol. 46, 1833–1847. doi: 10.1046/j.1365-2427.2001.00861.x
Milner A. M., Khamis K., Battin T. J., Brittain J. E., Barrand N. E., Füreder L., et al. (2017). Glacier shrinkage driving global changes in downstream systems. Proc. Natl. Acad. Sci. 114 (37), 9770–9778. doi: 10.1073/pnas.1619807114
Milner A. M., Loza Vega E. M., Matthews T. J., Conn S. C., Windsor F. M. (2023). Long-term changes in macroinvertebrate communities across high-latitude streams. Global Change Biol. 00, 1–12. doi: 10.1111/gcb.16648
Mustonen K. R., Mykrä H., Marttila H., Sarremejane R., Veijalainen N., Sippel K., et al. (2018). Thermal and hydrologic responses to climate change predict marked alterations in boreal stream invertebrate assemblages. Global Change Biol. 24 (6), 2434–2446. doi: 10.1111/gcb.14053
Myers-Smith I. H., Forbes B. C., Wilmking M., Hallinger M., Lantz T., Blok D., et al. (2011). Shrub expansion in tundra ecosystems: dynamics, impacts and research priorities. Environ. Res. Lett. 6 (4), 045509. doi: 10.1088/1748-9326/6/4/045509
Nordic Council of Ministers (1984). Geographic regions of the Nordic countries (Oslo, Norway: Nordic Council of Ministers).
Pearson R. G., Dawson T. P. (2003). Predicting the impacts of climate change on the distribution of species: are bioclimate envelope models useful? Global Ecol. Biogeography 12, 361–371. doi: 10.1046/j.1466-822X.2003.00042.x
Pedersen Å. Ø., Convey P., Newsham K., Mosbacher J. B., Fuglei E., Ravolainen V., et al. (2022). Five decades of terrestrial and freshwater research at Ny-Ålesund, Svalbard. Polar Res. 41. doi: 10.33265/polar.v41.6310
Poff N. L. (1997). Landscape filters and species traits: towards mechanistic understanding and prediction in stream ecology. J. North Am. Benthol. Soc. 16 (2), 391–409. doi: 10.2307/1468026
Poff N. L., Olden J. D., Vieira N. K. M., Finn D. S., Simmons M. P., Kiondratieff B. C. (2006). Functional trait niches of North American lotic insects: traits-based ecological applications in light of phylogenetic relationships. J. North Am. Benthol. Soc. 25 (4), 730–755. doi: 10.1899/0887-3593(2006)025[0730:FTNONA]2.0.CO;2
Prowse T. D., Wrona F. J., Reist J. D., Gibson J., Hobbie J. E., Lévesque L. M. J., et al. (2006). Historical changes in Arctic freshwater ecosystems. Ambio 35 (7), 339–346. doi: 10.1579/0044-7447(2006)35[339:HCIAFE]2.0.CO;2
Rantanen M., Karpechko A. Y., Lipponen A., Nordling K., Hyvärinen O., Ruosteenoja K., et al. (2022). The Arctic has warmed nearly four times faster than the globe since 1979. Commun. Earth Environ. 3, 168. doi: 10.1038/s43247-022-00498-3
R Development Core Team (2022). R: A language and environment for statistical computing (Vienna, Austria: R Foundation for Statistical Computing).
Schmera D., Baur B., Erős T. (2012). Does functional redundancy of communities provide insurance against human disturbances? Anal. using regional-scale stream invertebrate data. Hydrobiologia 693 (1), 183–194. doi: 10.1007/s10750-012-1107-z
Schmera D., Heino J., Podani J. (2022). Characterising functional strategies and trait space of freshwater macroinvertebrates. Sci. Rep. 12 (1), 1–9. doi: 10.1038/s41598-022-16472-0
Schmera D., Heino J., Podani J., Erős T., Dolédec S. (2017). Functional diversity: a review of methodology and current knowledge in freshwater macroinvertebrate research. Hydrobiologia 787 (1), 27–44. doi: 10.1007/s10750-016-2974-5
Statzner B., Beche L. A. (2010). Can biological invertebrate traits resolve effects of multiple stressors on running water ecosystems? Freshw. Biol. 55, 80–119. doi: 10.1111/j.1365-2427.2009.02369.x
Tanentzap A. J., Burd K., Kuhn M., Estop-Aragonés C., Tank S. E., Olefeldt D. (2021). Aged soils contribute little to contemporary carbon cycling downstream of thawing permafrost peatlands. Global Change Biol. 27 (20), 5368–5382. doi: 10.1111/gcb.15756
Tranter M., Brown G. H., Hodson A. J., Gurnell A. M. (1996). Hydrochemistry as an indicator of subglacial drainage system structure: A comparison of alpine and sub-polar environments. Hydrol. Process. 10 (4), 541–556. doi: 10.1002/(SICI)1099-1085(199604)10:4<541::AID-HYP391>3.0.CO;2-9
U.S. Environmental Protection Agency (EPA) (2012). Freshwater traits database (Washington, DC: Global Change Research Program, National Center for Environmental Assessment).
Usseglio-Polatera P., Bournaud M., Richoux P., Tachet H. (2000). Biological and ecological traits of benthic freshwater macroinvertebrates: relationships and definition of groups with similar traits. Freshw. Biol. 43, 175–205. doi: 10.1046/j.1365-2427.2000.00535.x
Warton D. I., Hui F. K. C. (2011). The arcsine is asinine: the analysis of proportions in ecology. Ecology 92, 3–10. doi: 10.1890/10-0340.1
Wiken E., Gauthier D., Marshall I., Lawton K., Hirvonen H. (1996). A perspective on Canada's ecosystems: an overview of the terrestrial and marine ecozones (Ottawa: Canadian Council on Ecological Areas).
Wilander A., Johnson R. K., Goedkoop W. (2003). A synoptic study of water chemistry and benthic fauna in Swedish lakes and streams Vol. 117 (Uppsala: Department of Environmental Assessment, Swedish University of Agricultural Sciences).
Woolway R. I., Sharma S., Smol J. P. (2022). Lakes in hot water: the impacts of a changing climate on aquatic ecosystems. BioScience 72 (11), 1050–1061. doi: 10.1093/biosci/biac052
Wrona F. J., Reist J. D., Amundsen P.-A., Chambers P. A., Christoffersen K. S., Culp J. M., et al. (2013). “Arctic biodiversity assessment,” in Status and trends in arctic biodiversity. Ed. Meltofte H. (Akureyri, Iceland: Conservation of Arctic Flora and Fauna (CAFF), 335–377.
Keywords: Arctic, freshwater, functional trait, benthic macroinvertebrate, diversity, climate change, stream, temperature
Citation: Lento J, Lau DCP, Brittain JE, Culp JM and Goedkoop W (2023) Macroinvertebrate traits in Arctic streams reveal latitudinal patterns in physiology and habits that are strongly linked to climate. Front. Ecol. Evol. 11:1209612. doi: 10.3389/fevo.2023.1209612
Received: 21 April 2023; Accepted: 21 July 2023;
Published: 18 August 2023.
Edited by:
Yixin Zhang, Soochow University, ChinaReviewed by:
Tan Lu, Chinese Academy of Sciences (CAS), ChinaJunjiro Negishi, Hokkaido University, Japan
Jan Ciborowski, University of Calgary, Canada
Copyright © 2023 Lento, Lau, Brittain, Culp and Goedkoop. This is an open-access article distributed under the terms of the Creative Commons Attribution License (CC BY). The use, distribution or reproduction in other forums is permitted, provided the original author(s) and the copyright owner(s) are credited and that the original publication in this journal is cited, in accordance with accepted academic practice. No use, distribution or reproduction is permitted which does not comply with these terms.
*Correspondence: Jennifer Lento, amxlbnRvQGdtYWlsLmNvbQ==
†These authors share first authorship
‡These authors share senior authorship