- 1Department of Evolutionary Genetics, Max Planck Institute for Evolutionary Biology, Plön, Germany
- 2Department of Evolutionary Biology, Faculty of Biology, Adam Mickiewicz University, Poznań, Poland
- 3Department of Biological Sciences, Indian Institute of Science Education and Research (IISER) Bhopal, Bhopal, India
- 4Faculty of Biology, Institute of Organismic and Molecular Evolution (iomE), Johannes Gutenberg Universität, Mainz, Germany
Diet may have an important influence on life history and behavioral traits involved in sexual selection. Males of high condition should be better able to bear the costs of high trait expression and hence express more elaborate sexual signals. Here, we follow Mus musculus domesticus raised in semi-natural conditions across four generations of standard (SQ) versus high quality (HQ) food, containing a higher energy level and manufactured to boost body condition during reproduction. We investigate multivariate effects on life history, pre- and post-copulatory traits of males. In addition, we investigate the effects of a food switch in the third generation on trait expression. From the F3 generation on, HQ males were more dominant. This resulted in HQ males being preferred in mate choice even though no post-copulatory traits were affected, suggesting a condition-dependent allocation to different traits. Sons of food switched males showed reduced pre- but not post-copulatory trait expression, irrespective of the direction of the food switch, indicating a mismatch rather than adaptive plastic adjustments in the first generation. We conclude that males balance investment into different traits based on the diet to achieve similar fitness. Furthermore, we show that adaptive shifts in male reproductive strategies need several generations to emerge while first responses to changing environments reflect a constrained trait expression.
1 Introduction
Intra-sexual selection is one of the key forces of evolution that contributes to reproductive fitness by increasing competitiveness for acquiring mates and successful fertilizations (Andersson and Iwasa, 1996). In many species, it is not limited to the classic morphological sexually selected traits such as male ornaments but also shapes developmental, physiological, behavioral and life history characters (Lailvaux and Irschick, 2006; Cally et al., 2019; Garlovsky et al., 2022). Many studies have shown variation in sexually selected traits due to environmental heterogeneity (Cornwallis and Uller, 2010; Ingleby et al., 2010). Changing environmental conditions have been shown to influence sex-specific traits such as courtship activity, mate choice, mating behavior, parental care and ultimately, population dynamics on ecological and evolutionary time scales (Maan and Seehausen, 2011).
One of the most widespread and vital environmental factors known to influence sexually selected traits relates to the availability and quality of the diet (Morehouse et al., 2020). Most sexually selected traits show condition-dependence, i.e., being smaller or expressed to a lesser extent in lower quality individuals (Rowe and Houle, 1996). For instance, in the two-spot ladybird beetle, Adalia bipunctata, males kept on high quality food had larger ejaculate size with increased non-sperm ejaculates. In comparison, males from low quality food allocated more resources to higher sperm production despite a lower ejaculate size (Perry and Rowe, 2010). Since organisms face trade-offs while allocating metabolic resources, they may adopt different life history strategies by making adjustments in growth rate, longevity and fecundity in response to the quality of the available diet. However, studies investigating sexually and non-sexually selected traits within a broader life history framework are only starting to accumulate recently (Fox et al., 2019; Morehouse et al., 2020).
Adjustments of trait expression within and between individuals in response to changing environmental conditions often arise due to phenotypic plasticity as a fast line of adaptive response (West-Eberhard, 1989). Phenotypic plasticity profoundly influences variation in the expression of sexually selected traits, thereby influencing their adaptive value and the overall process of sexual selection (Fox et al., 2019). Adaptive plasticity of sexually selected traits can be irreversible at sexual maturation due to early environmental conditions encountered during ontogeny or can be highly flexible in adulthood and change when environmental conditions fluctuate (Piersma and Drent, 2003; Price, 2006). Limiting resources during ontogeny versus after sexual maturation have been shown to influence trait expression differently (Katsuki et al., 2012; Macartney et al., 2019). In their meta-analysis, Macartney et al. (2019) showed a generally stronger and long-lasting response of traits when dietary restrictions occurred before sexual maturation. As an example for flexible changes during adulthood, male collared flycatchers, Ficedula albicollis, were found to change the sexually selected white forehead patch width according to the breeding season within individuals (Griffith and Sheldon, 2001).
Phenotypic trait adjustments can either arise within the lifetime of individuals or across one or few generations due to parental or trans-generational effects (Bonduriansky and Day, 2009). In sticklebacks for example, male mating success depends on the rearing environment of both, the male itself but also its father (Fuxjäger et al., 2019). Such inter-generational effects are adaptive when the parents’ predictions of their offspring’s environment shape the phenotypes of their offspring and potentially those of later descendants to match the environmental conditions optimally (Mousseau and Fox, 1998; Bateson et al., 2014). Thus, under matching environmental conditions across generations, plasticity can act as a first line of response towards environmental change, buying time for the slower genetic adaptations to catch up (Bonduriansky and Day, 2009; Chevin et al., 2010). If environmental conditions mismatch, plasticity often results in suboptimal, reduced trait expressions (Cushing, 1990). Evidence for plastic, intergenerational effects on sexually selected traits arising as consequences of parental diets come for example from studies like Krause and Naguib (2015), which have shown that male zebra finches (Taeniopygia guttata) had smaller cheek patches when raised under poor nutritional conditions. Another study showed that developmental speed and exploratory behavior were affected trans-generationally by the interaction of the grandparental and parental diet in zebra finches (Krause and Naguib, 2014). Furthermore, recent evidence suggests that inter-generational plasticity could impact not only mate choice or display traits of offspring but also the magnitude and target of sexual selection (Qvarnström and Price, 2001). To date, most studies investigating the condition-dependent expression of sexually selected traits stem from lab-based studies manipulating diet and measuring its effects in animals whose behavioral repertoire is limited by the experimental conditions, which may hamper a complete understanding of allocation processes to naturally and sexually selected traits (Morehouse et al., 2020).
In the present study, we provided four semi-natural colonies of wild house mice (Mus musculus domesticus) either with standard (SQ) or high quality (HQ) food, containing ~12% more energy across four generations. House mice are known to be ecological opportunists, thriving in a wide range of habitats and occupying a large nutritional niche (Bronson, 1979). This enables the species to successfully invade even harsh environments and adjust in a short time (Mathias et al., 2004). The caloric capacity of the diet is one of the most important factors influencing body condition and reproductive capacity (Bronson, 1979). Using the same food treatments as reported here, we previously observed a direct effect on female reproductive performance, enabling females to produce larger litters that are weaned with higher body mass and in shorter time compared to females fed with SQ food. In addition, we observed several behavioral, physiological and life history adaptations in wild house mice within three generations of adjusting to the respective food type (SQ or HQ food). In essence, high quality populations developed increasingly faster generation times, i.e., starting to reproduce at earlier ages and higher fecundity rates, i.e., producing more offspring with faster growth in a shorter time across the generations while simultaneously becoming more and more risk-averse (Prabh et al., 2023). House mice are regarded as polygynous but females have also been shown to be actively polyandrous both in the wild (Dean et al., 2006), as well as under controlled conditions (Manser et al., 2011). Polyandry suggests an option for postcopulatory selection processes such as sperm competition. It has been shown to increase offspring survival (Firman and Simmons, 2008a) and to facilitate inbreeding avoidance (Firman and Simmons, 2008b). Here, we now ask the following questions:
1) Do adjustments to food quality across generations include sexually selected male traits such as body mass development, testes mass, occurrence of injuries from fights with competitors or sperm quantity?
2) We investigate how these traits affect male fitness by testing likelihood to reproduce and mass at first reproduction.
3) We ask if inter-generational plastic adjustments to male sexually selected traits and reproductive success occur. Therefore, we conduct a food switch in two of the four treatments in the third generation and investigate phenotypic effects, including growth, sperm quantity and quality, and mate choice in the F4 generation.
According to the silver spoon hypothesis (Lindström, 1999; Monaghan, 2008) and the condition-dependence hypothesis (Morehouse, 2014), we expect males of HQ food to invest more resources/energy into the development of sexually-selected traits, i.e., showing faster growth (Vincenzi et al., 2013) or larger testes relative to their body mass. Such elaborate traits should increase individual fitness and/or male competition for females. Higher levels of competition between males are then expected to be reflected in higher rates of injuries in HQ males (for example, because of fights for territories and mate guarding). On a physiological level, testes mass, sperm quantity and quality are often reduced under nutritional limitations (Macartney et al., 2019) and may conversely show an increased expression in HQ compared to SQ males.
Based on the observed life history and behavioral adjustments that occurred within one to two generations (Prabh et al., 2023), we expect an equally fast response of sexually selected traits following a parental food switch. According to the match-mismatch hypothesis (Nederhof and Schmidt, 2012), we expect males whose parents experienced a food switch to show a general reduction in sexually selected traits and ultimately suffer fitness loss. Alternatively, we expect males that switched from SQ to HQ food to increase expression of costly traits because they have more resources available than anticipated. To study the effects of diet and diet switch, we measured body mass development, testes mass, sperm quantity and quality, and male fecundity. We also measured the consequences of these trait expressions on behavioral responses in aggressive dominance relationships and performance in mate choice tests. Previous studies investigating the effects of nutrition on male dominance are primarily available for invertebrates and have shown mixed results (South et al., 2011; Han and Dingemanse, 2017).
2 Materials and methods
2.1 Animals and housing
Mice (Mus musculus domesticus) originally descended from wild populations sampled in the Cologne/Bonn region and were maintained under laboratory conditions for several generations. Founder mice (F0) were born and raised in cages and fed with standard quality food. In total, 160 animals (80 males, 80 females) were distributed to four semi-natural enclosures by placing 20 unrelated males and females into each enclosure. For obtaining generations F1–F3, the populations could reproduce until at least 60 offspring per enclosure were reached, which, depending on the season, needed between three to seven months per generation. After obtaining the desired number of offspring for a generation, the previous generation was removed. Two enclosures received standard quality food (SQ) Altromin© 1324 and two high quality food (HQ) Altromin© 1414 throughout the experiment. The SQ diet contained 3227 kcal/kg of metabolizable energy (24% from protein, 11% from fat, and 65% from carbohydrate), and the HQ diet contained 3680 kcal/kg (28% from protein, 22% from fat, and 50% from carbohydrate), thus, ~12% more kcal/kg metabolizable energy than the SQ diet. The HQ diet is a specifically developed food mix to enhance body condition of mice during the energetically demanding breeding and results in higher reproductive output, both, in terms of number of offspring produced, offspring growth and shorter inter-birth intervals (Börsch-Haubold et al., 2014). In the third generation, the food type was switched in one enclosure per treatment from HQ to SQ, and alternatively from SQ to HQ prior to the start of offspring production (electronic supplementary material, Figure S1). Hence, during the fourth generation, two enclosures received a mismatching food type (referred to as HQ/SQ and SQ/HQ) compared to their parents but all enclosures received either only SQ or only HQ food. This also means that for the fourth generation, no replicate on the population level is available and thus patterns found here regarding sexually selected traits of males, should be considered carefully. In total, the experiment ran for 22 months.
For house mice, the natural environment is a barn or another human shelter (König and Lindholm, 2012). To mimic such an environment as closely as possible, each semi-natural enclosure (19.6 m2) was equipped with wood chip bedding (©Rettenmaier), various nesting materials and 12 houses, providing nesting space. Food and water were provided ad libitum and distributed uniformly across the room at nine feeding stations. Such conditions are generally similar to those found in natural house mouse populations across Europe since house mice live commensally with humans and typically occur where food availability is unlimited such as in granaries or barns (Berry, 1981). The rooms followed natural temperature and light conditions but freezing was prevented by an underfloor heating system. In addition to natural light, artificial lights were automatically turned on from 8:00 to 16:00. The enclosures were monitored at an interval of one month to collect data on individual body mass, reproduction and fresh bite marks. Therefore, all the animals were caught and weighed, new individuals weighing more than 10 g were tagged with RFID transponders for individual recognition (ISO Transponders, Planet ID), and ear clips were sampled for parentage assignment.
Population development and population densities in our semi-natural enclosures correspond to house mouse populations that are under long-term observation with free access and the possibility for dispersal (König and Lindholm, 2012). After several years of establishment, a free-ranging study population reached population densities of ~70 males and ~70 females each, distributed across 72 m2, i.e., on average 2 adult animals per m2. Each generation of our populations ran with an average of 3 adult animals per m2. In general, house mice across Europe live in loosely connected sub-populations, so-called demes (Duncan et al., 1979). Recent studies showed that such demes primarily consist of extended families with an average relatedness of 0.3–0.5 but less than expected levels of inbreeding (Linnenbrink et al., 2018). In our populations, we have recently shown similar levels of genetic population structure, level of heterozygosity and inbreeding (Prabh et al., 2023). In addition, we also see clustering into three to four, largely disconnected demes within each of the semi-natural enclosures (unpublished data), comparable to what has been observed in other study populations (König et al., 2015).
Males were investigated for ten traits. Part of them were sampled directly under semi-natural conditions (A). In addition, we also caught several males and tested them for specific traits after transferring them into cages (B).
A) Experiments conducted under semi-natural conditions:
For all four generations, male body mass development, fresh bite marks, and reproductive fitness, i.e., number of surviving offspring to weaning per month, were measured, and males were later dissected to investigate the testes/body mass percentage and average sperm concentrations. On a population level, we recorded for each generation and treatment the level of polyandry, i.e., the number of litters sired by multiple males.
B) Experiments conducted in cage housing:
Random adult males (> 20g) of the F4 generation were transferred to Type III standard cages at least 24 h before the experiment with an enrichment, a shelter, bedding material, water and respective food ad libitum. They were then tested for mate choice, male dominance, fecundity, testes/body mass percentage and sperm motility and concentration, as described in detail below.
2.1.1 Experiments conducted under semi-natural conditions
2.1.1.1 Male body mass development and fitness
Every month during the monitoring, all live individuals were taken out of the enclosures and weighed. Dead animals were mostly assigned by being missing although we frequently found the bodies of dead animals.
2.1.1.1.1 Parentage analysis
Seventeen microsatellite markers were used for parentage analysis, and parentage assignment was done using the procedure adapted from Linnenbrink et al. (2013). Briefly, DNA extracted from the ear clips was amplified using a Multiplex PCR kit (QIAGEN). Samples were then run on an ABI 3730 Sequencer (Applied Biosystems). GeneMarker (V2.6.4) was used to call alleles, and Colony [©COLONY | Zoological Society of London (ZSL)] was used to assign the parentages. The assignment was based on the maximum likelihood of each potential parental pair, assuming that reproduction was sexual, with a polygamous mating system, possible inbreeding and all animals being present in the semi-natural enclosure being possible parents.
2.1.1.1.2 Reproductive fitness
Only males sighted in > 3 monitorings, i.e., fully adult, were used to compare the treatments’ reproductive fitness. The individual reproductive success was calculated by dividing the total number of offspring (as assigned by the parentage analysis) by the total number of monitorings the male was sighted for (i.e., its maximum age). This was done to adjust for the fact that some males had less time to sire offspring than others because we removed each generation when enough offspring of the next generation were present. Running a similar analysis based on the total number of offspring produced yielded similar results (see electronic supplementary material). We also compared the body mass at first successful reproduction, i.e., the monitoring at which a male first sired offspring (that survived until weaning) across treatments.
2.1.1.2 Male reproductive traits
2.1.1.2.1 Testes weight
When enough offspring of the following generation were born, fifteen randomly chosen adult males out of all males present in the enclosure were dissected to assess testes’ mass and sperm concentration. We later removed all males from the analysis which were sired by parents belonging to different generations according to parentage assignments, resulting in 7–15 adult males per enclosure and generation. Males were killed using carbon dioxide, followed by cervical dislocation. The whole body and testes were weighed, and the testes/body mass percentage was calculated.
2.1.1.2.2 Sperm quantity
Sperm quantity was assessed using a modified version of the procedure described in Wang (2002). The epididymis was sliced in a Phosphate buffer solution (PBS). The sperm suspension was incubated in a thermomixer for 10 min at 40°C to kill the sperms. In a Burker chamber, 10 μl of 1:20 or 1:40 diluted sperm suspension was used accordingly to get a sperm count of around 3–10 per square. Sperms were counted twice under a microscope at 40× with a PH2 filter in 25 squares.
2.1.2 Experiments in cage housing
2.1.2.1 Behavioral tests
2.1.2.1.1 Mate choice
The experimental setup for mate choice analysis was adapted from Linnenbrink and von Merten (2017). One male per food treatment (HQ, SQ and HQ/SQ, SQ/HQ) from F4 generation was placed in one of four satellite cages. In total, we ran 22 sets of mate choice tests, and twelve males per treatment were used. Naive focal females previously fed with SQ food were placed in a central cage with access to all four satellite cages via Plexi tubes. The female could communicate with males but not mate, as the male cages were divided using metal meshes with only one side accessible to the female. All the animals were provided with bedding, respective food, and water ad libitum during the experiment. The female was provided with a mixture of HQ and SQ food (electronic supplementary material, Figure S2).
Four experimental setups were used simultaneously, with each setup visually separated. A Panasonic Full HD 10MP HC-V180 video camera was used for recording. Experiments ran for 24 hours, and three one-hour videos were recorded at the interval of every 12 hours; at the start of experiments (17:30–18:30), the following day (7:30–8:30), and before the experiment was stopped (17:30–18:30). After giving the males a rest for 4–5 days, the same satellite male quad with a new naive female were then placed in different positions of satellite cages. The total time spent by the female and the number of female visits to a satellite cage in each recording of the setup were scored.
2.1.2.1.2 Male aggression and dominance
To test male aggression, adult males of all generations (i.e., males that had been sighted > 3 monitorings) were checked for fresh bite marks during the monthly population monitorings. In addition, male dominance was tested in F4 generation by placing 35 randomly chosen males (17 SQ, 18 HQ) in cages with holes at least 48 hours prior to the start of experiments. Pairs of one SQ and one HQ male with approximately similar body mass and age were established. On the day of testing, the cages of the two opponents were connected by a tube made of red Plexiglas (i.e., being transparent for humans but appearing dark and opaque to the mice). Males were prevented from having direct access to each other by a metal grid in the middle of the tube to prevent any injuries. Interactions of opponents were then video recorded for 15 minutes (electronic supplementary material, Figure S3). During that time, we recorded how often the focal male initiated attacks (i.e., jumping at the metal grid when the opponent was visible on the other side), lost a fight independent of who initiated it (i.e., retreated from the metal grid while the opponent stayed), won a fight (i.e., the opponent retreated first) and initiated threats such as tail whipping or taking a position on its hind legs (often a posture from which an attack is launched). In total, each male was tested against three opponents to average out the potential influences of the opponent on the expressed behavior. Between encounters, a male was given at least 24 hours break.
2.1.2.2 Male fecundity
Random adult males (F4 generation, N = 12 per treatment) were chosen from HQ and SQ treatments to test for male fecundity. A naive female previously fed on SQ food was later transferred to the male cage and allowed to interact for the next ten days before being removed. The male was then transferred to a clean cage and was paired in the same way with another naive female for ten days. Hence, every male was given two chances to reproduce. During this time, both males and females were simultaneously provided with both types of foods. This was done to avoid the possible direct influence of different diets on females. The females were immediately dissected upon removal to check for pregnancy status and to assess the number of fetuses.
2.1.2.3 Sperm motility
Ten random F4 generation adult males from each treatment, including the food switch, were tested for sperm motility after the mate choice experiment using a modified protocol from Goodson et al. (2011). The mT-B25 buffer, overlaid with mineral oil, was left undisturbed overnight at 37°C and 5% CO2 in an incubator. The following day, one cauda epididymis was transferred to the emulsion at 40°C. It was then sliced with an 18-gauge needle. The suspension with the epididymis was incubated at the same settings for 20 minutes to allow the sperms to swim out. After ten minutes, the residual large piece of the epididymis was removed, and the suspension was further incubated for another 70 mins. After 90 minutes of the capacitation period, the sperm suspension was diluted nine folds using the same buffer. In a Leja2 slide, 30 μl of diluted sperm suspension was added, and the motility data in terms of the percentage of motile sperms were gained using CEROS sperm analyzer by Hamilton Thorne Biosciences. Four frames were recorded for each individual.
2.2 Statistical analysis
For the experiments conducted within the semi-natural enclosures, HQ and SQ treatments were compared across all four generations. To check for potential parental effects, all treatments (HQ, HQ/SQ, SQ, SQ/HQ) of the F4 generation were also analyzed separately. For statistical analysis, R Studio version 4.1.1 was used.
2.2.1 Life history traits
To analyze body mass development for all generations, we considered body masses until the sixth monitoring (6 months of age) and analyzed the generations separately. We used linear mixed-effects models (LMER) (Bates et al., 2014) with treatment, monitoring, and treatment × monitoring as fixed effects and individual ID as a random effect.
To analyze male reproduction, we first compared the mass of males at first successful reproduction (i.e., the monitoring at which a male first sired offspring) using two LMs with treatment, generation, treatment × generation, and reproductive success as fixed effects across all generations (using SQ and HQ treatments) and F4 generation (without generation and treatment × generation as effects but having four treatments) separately. We assigned binary variables for the males successful (1) and unsuccessful (0) in siring offspring. We used GLMs with binomial distribution with treatment, generation, treatment x generation, and monitoring as fixed effects across all generations and separately for the F4 generation (without generation and treatment × generation as effects). We also used Spearman’s correlation test to check the correlation between the mass of males at first successful reproduction and their reproductive success.
2.2.2 Behavioral traits (pre-copulatory traits)
We constructed a series of three models to analyze the time a female spent in each male’s cage during the mate choice experiment. First, we compared the time spent by the female in each male cage for the first hour of recording, i.e., immediately after the test had started. During this time, we did not expect a female to make a choice already since she first had to sample her options. In a second model, we analyzed the time spent by the female in each male’s cage during the second and third recording, i.e., when we expected them to have made a choice. Additionally, we analyzed the data from the last hour of recording also separately in case that females had become even more strict in their choice of male after the night. For the second model (i.e., combined recordings 2 and 3), we only considered setups in which the females spent more than 30% (second recording) and 50% (third recording) of the time in a specific male cage, i.e., showed considerable interest in a specific male. The time spent inside the male cage followed Poisson distribution after square root transformation, so we rounded it to the closest full number and used a GLMER model. Male treatment and mass were coded as fixed effects, and female ID as a random effect for all three models except the second model, where we also used recording number as a fixed effect.
We used a GLMER assuming a binomial distribution to analyze fresh bite occurrences for all generations. For this model, treatment, generation, treatment × generation and mass at every monitoring were used as fixed effects and the individual ID as a random effect. A similar model was built for the F4 generation (where we had four instead of only two treatments) but without the generation as an effect. For the tube dominance tests, we used GLMs for the total number of attacks initiated and attacks won by the focal male in all three trials assuming a Poisson distribution and coded male treatment and male body mass as fixed effects. We converted the data for overall attacks lost and threat displays in all three trials into a binary variable because of heavy zero inflation and used GLMs with binomial distribution with male treatment and body mass as fixed effects.
2.2.3 Physiology and reproduction (post-copulatory traits)
Two different LMs with treatment, generation, treatment × generation and body mass as fixed effects were used to analyze sperm concentration for treatments without a food switch for all generations and the generations with a food switch, i.e., F3 and F4 separately. Similar models were used for testes/body mass percentage but with the individual’s age when dissected instead of body mass. To find correlations between average sperm concentration and testes/body mass percentage, body mass and age of the individuals at the time of dissection, Pearson’s correlation tests were used. For F4 testes/body mass percentage, we used an LM with body mass as a fixed effect and performed the pairwise t-test to compare sperm motility between the four treatments. Proportions of pregnant females were compared using a Chi2-proportion test. Owing to the smaller sample size, we checked if the data was normally distributed using the Shapiro-Wilks normality test for the total number of fetuses implanted by the males, and then we performed the Wilcoxon signed-rank test for the same.
For all analyses, model assumptions were checked visually by checking qq-plots and plotting residuals against fitted values.
3 Results
For the full statistical output of all models, refer to the electronic supplementary material. On average, 21% of litters that have surviving offspring until weaning were sired by more than one male (Supplementary Table S3). The proportion of multi-sire litters did not differ between treatments in any generation.
3.1 Life history traits
SQ males grew faster in F1 (E=0.412, SE=0.165, p=0.013) conversely, HQ males grew faster in F2 (E=−0.914, SE=0.168, p<0.001). On the other hand, males of both the treatments grew similarly in F3 but for F4, both SQ and HQ/SQ had a faster body mass development than HQ (E=1.106, SE=0.219, p<0.001 and E=0.642, SE=0.258, p=0.013) (electronic supplementary material, Figure S4).
We found no significant treatment or generation effect for the mass of males at first successful reproduction (electronic supplementary material, Figure S5). Likewise, no significant treatment difference was seen in the percentage of males siring offspring in all generations. No correlation was observed between the reproductive success and the mass at which the mice had their first offspring. There was no significant treatment or generation effect on the likelihood of reproducing, their number of offspring or their reproductive fitness (electronic supplementary material, Figure S6).
3.2 Behavioral traits (pre-copulatory traits)
We found no significant treatment effect during the analysis of the first hour of the mate choice experiment. We found a significant treatment effect for the combined recordings of 12 and 24 hours (2 = 17.473, df = 3, p < 0.001, see Figure 1). Post-hoc analysis showed that the preference for HQ was significantly higher than for the other treatments (HQ-HQ/SQ: E= 0.514, SE= 0.137, p= 0.001; HQ-SQ: E= 0.350, SE= 0.132, p= 0.016; HQ-SQ/HQ: E= 0.396, SE= 0.136, p= 0.010). Analyzing the third recording separately, revealed a similar effect showing a significant treatment effect (Chi2 = 14.95, df = 3, p= 0.002) with a significantly higher preference for pure HQ over other HQ/SQ and SQ/HQ (E= 0.510, SE= 0.174, p= 0.01 and E= 0.578, SE= 0.181, p= 0.008).
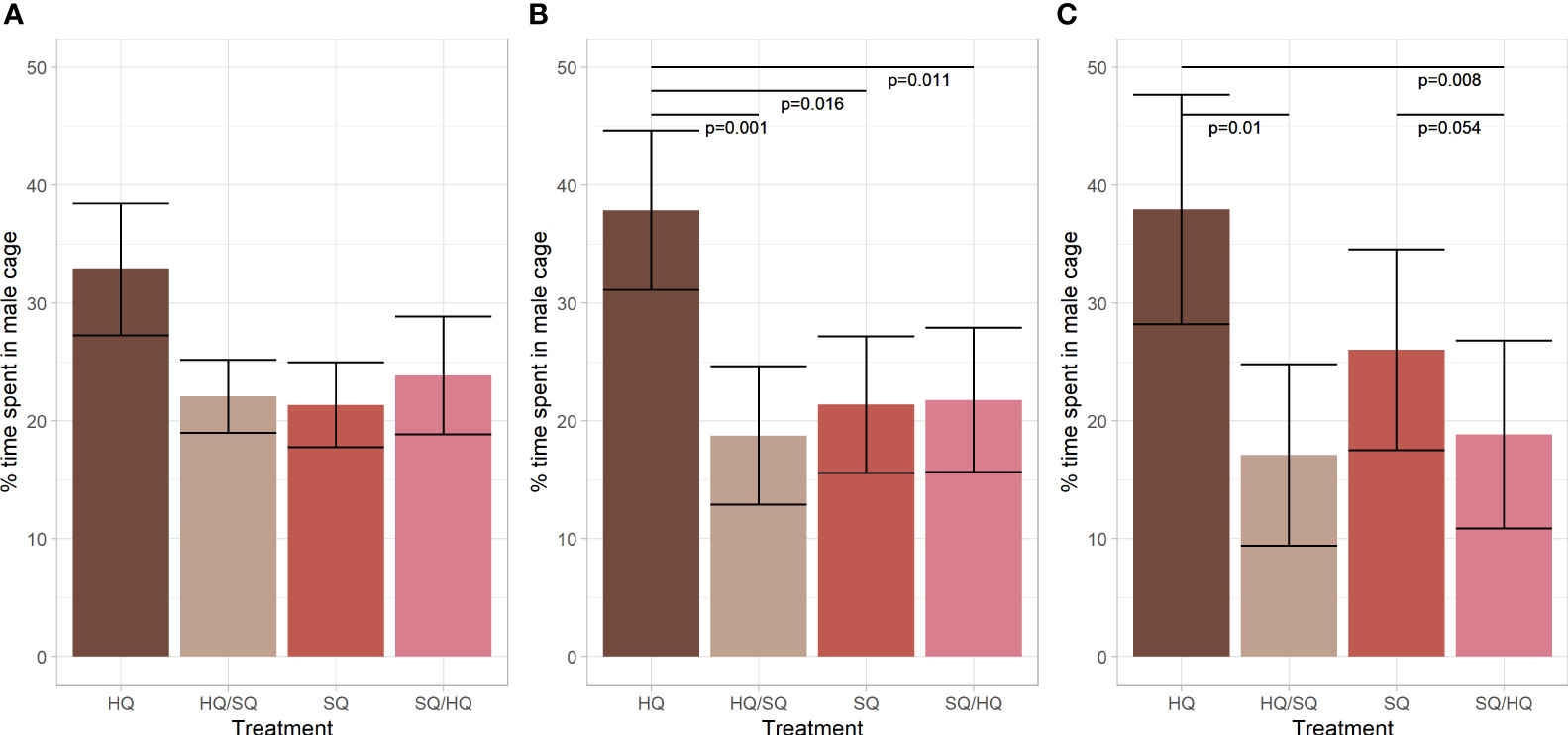
Figure 1 Mate choice experiment males of the F4 generation. (A) Time spent by females in each male cage during the first hour of the experiment. (B) Time spent by females in each male cage during the 12–24 hours of the experiment. (C) Time spent by females in each male cage during the last hour of the experiment after 24 hours. Error bars represent the standard error of the mean.
There was no overall treatment difference for the likelihood of showing bite marks, but the generations differed from each other (Chi2 = 28.783, df=3, p < 0.001) and larger males were more likely to have fresh bites (Chi2 = 27.592, df=1, p < 0.001). There was also an overall treatment by generation effect (Chi2 = 9.515, df=3, p=0.023). In F3 and F4, SQ males had more bites than HQ males (F3: E=−0.815, SE=0.343, p=0.037 and F4: E=−1.034, SE= 0.411, p=0.028, see Figure 2). In addition, for F4, the model showed that pure HQ males had a lower bite occurence compared to pure SQ males (E = −1.088, SE = 0.443, p = 0.021). The males switching from HQ to SQ had fewer bites than pure HQ males (E=1.182, SE=0.468, p=0.021) and fewer bites than pure SQ males (E=−2.270, SE=0.539, p=<0.001) while SQ/HQ males had more bites than pure HQ/SQ males (E=−1.846, SE=0.517, p = 0.001).
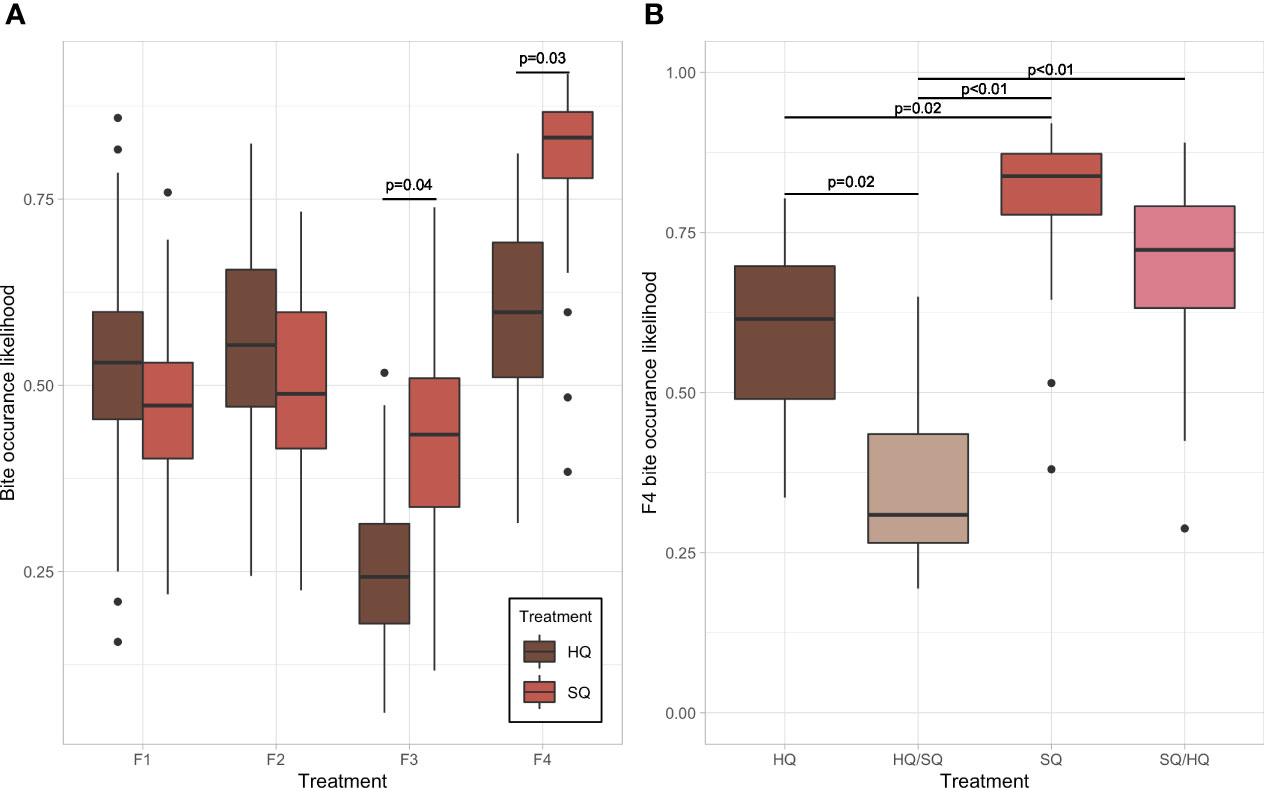
Figure 2 Bite occurrence likelihood in males from different food quality treatments. (A) Generation-wise differences in the likelihood of bite marks on HQ and SQ males. (B) Treatment-wise difference in the likelihood of bite marks on males from different food quality treatments.
HQ males initiated more (E=−0.463, SE= 0.116, p<0.001) and won more (E=−1.233, SE=0.203, p<0.001) attacks than SQ males (Figure 3). Heavier males were more likely to initiate (E=−0.089, SE=0.021, p<0.001) and win attacks (E=−0.105, SE=0.032, p=0.001). There was no treatment difference for the likelihood of losing a fight (although SQ>HQ being close to significance with p = 0.052) or for the likelihood to threaten.
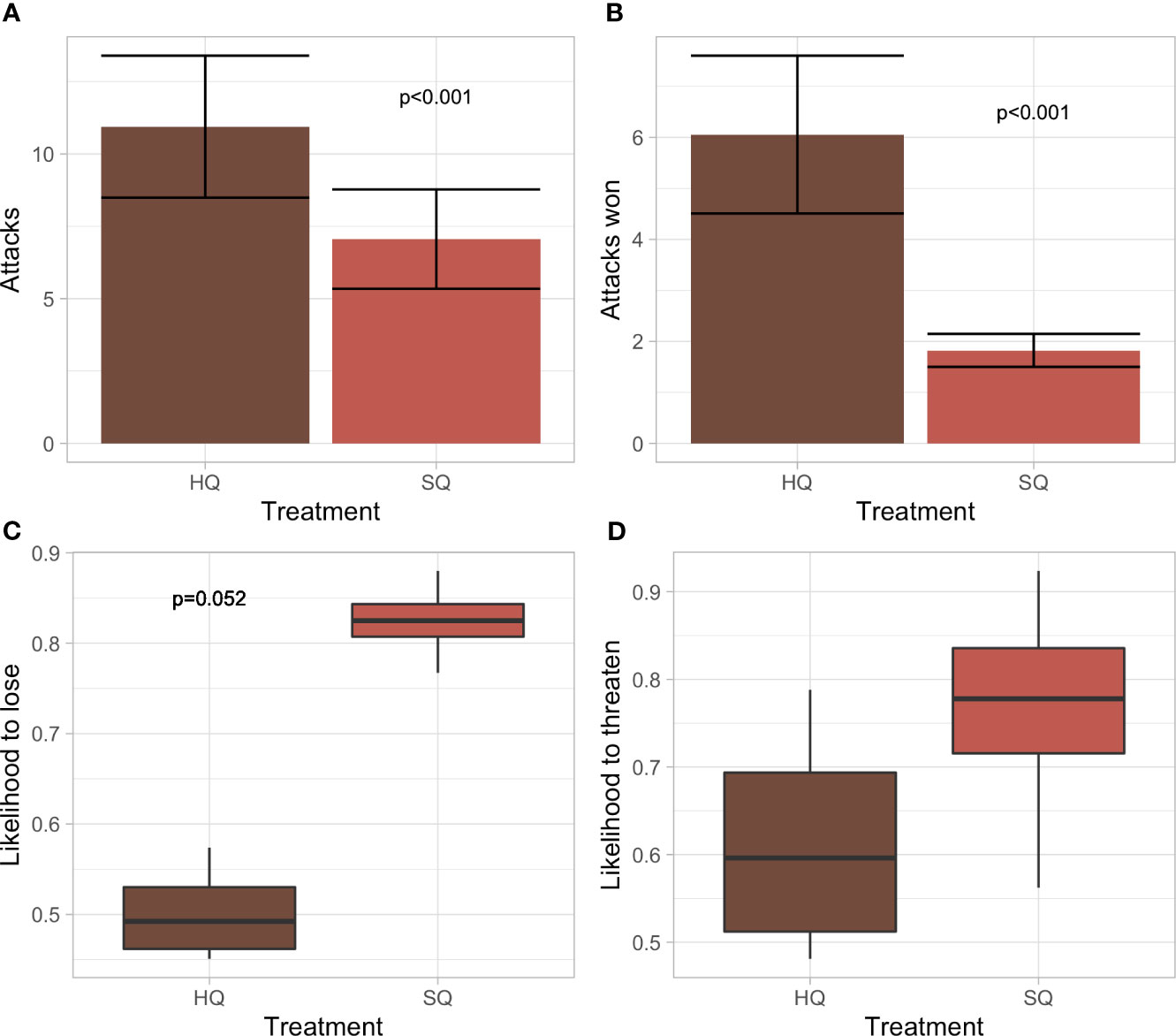
Figure 3 Tube dominance test for HQ and SQ males from F4-generation. (A) Number of attacks initiated by HQ and SQ males. (B) Number of attacks won by HQ and SQ males. (C) Likelihood of losing a fight between HQ and SQ males. (D) Likelihood of threats initiated by HQ and SQ males.
3.3 Physiology and reproduction (post-copulatory traits)
We saw no differences within generations between treatments for sperm concentrations and testes/body mass percentage for all generations, including the generations with food switch treatments i.e., F3 and F4 (electronic supplementary material, Figure S8). However, when not considering generations separately (i.e., averaging across F3 and F4), we saw that a food switch from HQ to SQ did not affect testes/body mass percentage and sperm concentration but a switch from SQ to HQ increased sperm concentration (SQ/HQ – SQ: E=−3756412, SE= 1397046, p=0.029; SQ/HQ – HQ/SQ: E= −3459488, SE=1317780, p=0.029).
No significant difference in the percentage of pregnant females occurred between the two treatments. Likewise, the number of implanted fetuses did not differ between treatments (electronic supplementary material, Figure S9).
We saw no treatment effect for testes to body mass percentage. However, individuals with higher body mass had a higher testes/body mass percentage (df=1, Chi2 = 0.491, p<0.001). There was no difference in sperm motility across treatments in F4 (electronic supplementary material, Figure S10).
4 Discussion
We did not observe any direct effects on male fitness traits such as the mass at first reproduction, number of offspring produced, likelihood to reproduce or fecundity between males of the different diets. Male growth was variable across generations and thus probably influenced by spatial and/or temporal environmental heterogeneity. However, we found diet consistently affecting pre-copulatory behavioral traits, suggesting that males follow different strategies on different food qualities to achieve similar fitness. One likely mediator of different strategies is territorial aggression in males. Starting in the F3 generation, SQ males had a higher occurrence of bites. When tested against each other, HQ males initiated and won more fights than SQ males. Consequently, females generally preferred HQ males despite no noticeable physiological differences between SQ and HQ males in sperm quality, quantity or testes size. Switching food types did not affect offspring growth, but we found effects on behavioral traits. The occurrence of bite marks decreased in the offspring generation regardless of the direction of the switch, and sons of food switched animals were the least preferred in mate choice.
4.1 Effects of high-quality diet on male fitness and sexually-selected traits
We did not observe any consistent effects of the diet on reproduction (onset of reproduction, likelihood to reproduce, reproductive fitness or total number of offspring produced) across the generations. Low quality nutrition is well-described to negatively affect female reproductive traits across taxa, i.e., later onset of reproduction or lower reproductive success (California voles (Batzli, 1986); house mice (Bomford, 1987); sparrows (Arcese and Smith, 1988); Daphnia (Vanni and Lampert, 1992)). However, less attention has been given to the effects of nutrition on male fitness traits. In the European grapevine moth, the male larval diet strongly influenced mating success and fecundity (Moreau et al., 2007). Our data, on the other hand, suggest no effect of diet on male reproductive characteristics in house mice. Known differences in reproduction between HQ and SQ, for example, in fecundity rates or generation times of house mice, are more pronounced in females than in males (Prabh et al., 2023). Taken together, these results imply that observed effects of food quality on population development (Bomford, 1987; Eccard and Ylönen, 2001) are mainly mediated by females. Conversely, males may allocate available resources to different traits based on diet and use alternative reproductive tactics to achieve similar fitness (Mehlis et al., 2015; Morimoto and Wigby, 2016; Morehouse et al., 2020). Drosophila males fed with different diets experimentally manipulating the ratio of protein to carbohydrates for example, invested differentially into pre- or post-copulatory traits. Depending on the specific diet, males either allocated resources into pre-copulatory trait expression, aiming to attract mating partners, or into post-copulatory traits aiming to maximize offspring production but no diet allowed optimization of both types of traits (Morimoto and Wigby, 2016). Another example for diet-based allocation into pre- versus post-copulatory traits comes from sticklebacks. Food-restricted males were found to prioritize current over future reproductive success by intense allocation into breeding coloration and sperm production (Mehlis et al., 2015).
Generally, male aggressive behavior is a strong predictor of reproductive success because it influences the likelihood of establishing and maintaining a territory as well as preventing other males from mating (Oakeshott, 1974; Dewsbury, 1982), and usually, about 90% of all offspring in house mice are sired by territory-holders (Mackintosh, 1970). Hence, dominance in aggressive encounters is one way to monopolize access over females (Harvey et al., 1989). We found that males fed with high-quality food express higher pre-copulatory trait values, i.e., being dominant in aggressive encounters and eventually being preferred as a mate. However, whether these results translate to a more natural situation, i.e., if HQ males are really able to outcompete SQ males under (semi)-natural conditions, remains to be tested. Contrary to our prediction that bite marks indicate overt aggression, SQ males of the third and fourth generations were more likely to show bite marks in the semi-natural enclosures compared to HQ males, while, in the tube dominance tests, HQ individuals initiated and won more attacks than SQ individuals (Figure 3), indicating that HQ males were more aggressive and dominant and therefore, less likely to suffer injuries. This is in line with results showing high quality food raising aggression levels, especially in competitive environments such as territories or those with scarce resources (Wallner and Machatschke, 2010). Alternatively, males may resort to different behavioral strategies to ensure mating success. For example, males use fighting strategies that rely more on threats than physical engagement, as observed in fruit flies (Legros et al., 2021). Although not statistically significant, we found SQ males to threaten more often during tube dominance encounters. Low quality males of some species develop a “sneaker” strategy, thereby avoiding aggressive encounters with potential rivals (Moczek and Emlen, 2000). In the mate choice tests, HQ males were preferred by females. The costs of elaborated male signaling to attract females are linked to body condition because the proportional costs should be higher for males of low condition (Grafen, 1990). This has been verified numerous times and across multiple species (Harrison et al., 2017). Condition-dependence of male displays plays a fundamental role in ensuring fitness benefits of mate choice because females may use the expression of displays as honest signals for direct fitness benefits such as disease avoidance, territory quality and parental contribution (Hegyi et al., 2019), but also to assess genetic benefits (Møller and Jennions, 2001).
While we saw a higher investment into pre-copulatory behavioral traits by HQ males, we did not observe effects in post-copulatory traits such as the testes/body mass percentage or sperm quantity. Given that mice from both food treatments showed similar levels of polyandry, i.e., litters sired by more than one male across all generations, finding no differences in investment into post-copulatory traits, appear reasonable. Likewise, zebra finches raised on low- or high-quality food did not differ in relative testes mass, sperm counts or sperm motility (Birkhead et al., 1999). Both examples illustrate that males fed on HQ may generally be more likely to invest in traits which allow them to compete with other males to maximize fitness rather than relying on post-copulatory, physiological and/or reproductive traits. Accordingly, we did not find an effect of male food quality on fecundity, i.e., the likelihood to successfully fertilize a female or the number of fetuses implanted by the female.
We also expected HQ males to have a faster body mass development or higher adult body mass, because both often show strong links to fitness (Ricklefs, 1984; Boag, 1987; Stamps, 2007). For example, zebra finches raised on lower quality food showed reduced growth and lower adult body mass in both sexes (Boag, 1987; Martins, 2004). Other studies found mixed results, reporting reduced adult mass in SQ-raised animals, as well as no difference or sex-specific effects (Birkhead et al., 1999; Martins, 2004; Arnold et al., 2007). Here, we find similarly fluctuating effects across four generations, with males in F1 and F3 showing similar growth and adult mass, while HQ and SQ males grew faster and larger in F2 and F4, respectively. Hence, our data indicate that growth and adult mass are highly variable and may not consistently contribute to alternative male tactics in house mice. Males may instead allocate excess energy reserves into the expression of traits such as aggressive dominance and mate attraction signaling.
4.2 Effects of a parental diet switch on sexually-selected traits
Phenotypic plasticity of male sexually selected traits might influence how sexual selection affects a population’s reproductive output. Recent theory calls for broader incorporation of sexually-selected traits into studies of plastic adjustments to fast-changing environments (Head et al., 2016). Here, we conducted a food switch in the third generation to study fast occurring adjustments of sexually-selected traits. Until this switch, a population genetics analysis gave no indication for a genetic differentiation between the food treatments or for inbreeding within enclosures (Prabh et al., 2023). Thus, all observed effects are most likely due to plastic, epigenetic adjustments. In general, we found a food switch in the F3 generation to affect the expression of pre-copulatory, behavioral traits of sons, while we did not find convincing evidence for effects on post-copulatory effects or growth. Dietary shifts have been shown to influence both pre- and post-copulatory traits in a wide range of taxa in the animal kingdom. Diet restriction in the viviparous fish, Dermogenys collettei caused a reduction in body size, length of beaks, testis size, and courtship behavior such as male–male competition compared to males maintained on high diet. On the contrary, the diet restricted males showed a higher red beak and fin coloration relative to high conditioned males which is an important cue for the females during mate choice decisions (Fernlund Isaksson et al., 2022).
Sons of males that experienced a switch in food quality generally showed a decreased occurrence of bite marks compared to their respective control treatments (Figure 2). Interestingly, this decrease occurred irrespective of the direction of the food switch, i.e., from lower to higher quality or vice versa, suggesting a general mismatch of aggressive phenotypes rather than a “silver-spoon” for males receiving a better-than-expected food quality (Frankenhuis and Del Giudice, 2012). Likewise, we observed that females showed a significantly lower preference for sons of food switched males (Figure 1). A switch from HQ to SQ treatment resulted in significantly lower preference than pure HQ treatment, while a switch from SQ to HQ still reduced females’ preference though not statistically significant.
While measuring the post-copulatory traits, we did not find a significant difference between the individuals when we compared the testes/body mass percentage and sperm motility. However, we did see that SQ/HQ males had an overall higher testes/body mass percentage than HQ/SQ and SQ. Growth was enhanced in one but unaffected in the other food switch treatment. Sons of males from SQ and HQ/SQ treatments grew faster than HQ males. According to Tatar and Carey (1995), food quality can affect the trade-off between reproductive effort and other life history traits such as longevity. In milkweed bugs, males with a new diet showed a longer lifespan but invested less in mating effort and fertility than the males with an ancestral diet (Attisano et al., 2012). Similarly, there may be trade-offs between survival and the expression of male sexually selected traits as shown in neriid flies, Telostylinus angusticollis (Sentinella et al., 2013). Although we did not measure survival here, males may have invested in traits which conferred survival advantages following a food switch in the parental generation.
Taken together, our data show that an unexpected shift of diet, irrespective of shifting to better or worse, induces reduced allocation to costly sexually selected traits in sons, which presumably go hand in hand with increased investment into self-maintenance and an emphasis on survival. These findings align with expectations of the match-mismatch hypothesis, stating that individuals are more likely to show maladaptive traits or suffer from diseases when a mismatch occurs between the early programming environment and the later (adult) environment (Nederhof and Schmidt, 2012). The effects we found here show that the expression of sexually selected male traits in house mice and maybe also in other small mammals is shaped already during early life stages, either pre-natally by maternal effects or soon after birth, resulting in a constrained trait expression in adult sons living under mismatching conditions. This was an unexpected outcome since we expected a shift from lower to a high quality to yield a silver spoon effect (Lindström, 1999; Monaghan, 2008). To our knowledge, this study is the first to conduct such a match-mismatch experiment under semi-natural conditions in a mammal species. Our partially unexpected findings may reflect mixed results regarding condition-dependent trait expressions in the literature. In any way, our findings argue for a careful choice of experimental design, including several traits that may compete in allocation processes and involve more than one generation to test the effects of potentially adaptive plastic changes in trait expression. We saw that changes in pre-copulatory behavioral traits occur fast, as hypothesized (e.g., Fox et al., 2019), but initial changes in the first generation, after an environmental shift (SQ to HQ) reflect a mismatch rather than an adaptive adjustment. Potentially adaptive shifts in male reproductive strategies emerged only from the third generation onwards. Hence, researchers aiming to incorporate sexually selected traits to answer questions about the role of sexual selection in facilitating population persistence in the face of rapid environmental change need to conduct experimental studies very carefully.
Data availability statement
The original contributions presented in the study are included in the article/Supplementary Material. Further inquiries can be directed to the corresponding author.
Ethics statement
The animal study was reviewed and approved by Ministerium für Energiewende, Landwirtschaftliche Räume und Umwelt, Kiel.
Author contributions
NP and AG conceived the study design, NP collected and analyzed data, except for mate choice tests, which were done by SG and fitness data which HA collected and analyzed. All authors contributed to data analysis and writing.
Conflict of interest
The authors declare that the research was conducted in the absence of any commercial or financial relationships that could be construed as a potential conflict of interest.
Publisher’s note
All claims expressed in this article are solely those of the authors and do not necessarily represent those of their affiliated organizations, or those of the publisher, the editors and the reviewers. Any product that may be evaluated in this article, or claim that may be made by its manufacturer, is not guaranteed or endorsed by the publisher.
Supplementary material
The Supplementary Material for this article can be found online at: https://www.frontiersin.org/articles/10.3389/fevo.2023.1207480/full#supplementary-material
References
Andersson M., Iwasa Y. (1996). Sexual selection. Trends Ecol. Evol. 11 (2), 53–58. doi: 10.1016/0169-5347(96)81042-1
Arcese P., Smith J. N. (1988). Effects of population density and supplemental food on reproduction in song sparrows. J. Anim. Ecol. 1, 119–136. doi: 10.2307/4768
Arnold K. E., Blount J. D., Metcalfe N. B., Orr K. J., Adam A., Houston D., et al. (2007). Sex-specific differences in compensation for poor neonatal nutrition in the zebra finch Taeniopygia guttata. J. Avian Biol. 38 (3), 356–366. doi: 10.1111/j.0908-8857.2007.03818.x
Attisano A., Moore A. J., Moore P. J. (2012). Reproduction-longevity trade-offs reflect diet, not adaptation. J. Evolutionary Biol. 25 (5), 873–880. doi: 10.1111/j.1420-9101.2012.02476.x
Bates D., Mächler M., Bolker B., Walker S. (2014). Fitting linear mixed-effects models using lme4. arXiv preprint arXiv:1406.5823.
Bateson P., Gluckman P., Hanson M. (2014). The biology of developmental plasticity and the Predictive Adaptive Response hypothesis. J. Physiol. 592 (11), 2357–2368. doi: 10.1113/jphysiol.2014.271460
Batzli G. O. (1986). Nutritional ecology of the California vole: effects of food quality on reproduction. Ecology 67 (2), 406–412. doi: 10.2307/1938583
Birkhead T. R., Fletcher F., Pellatt E. J. (1999). Nestling diet, secondary sexual traits and fitness in the zebra finch. Proc. R. Soc. London. Ser. B: Biol. Sci. 266 (1417), 385–390. doi: 10.1098/rspb.1999.0649
Boag P. T. (1987). Effects of nestling diet on growth and adult size of zebra finches (Poephila guttata). Auk 104 (2), 155–166. doi: 10.1093/auk/104.2.155
Bomford M. (1987). Food and reproduction of wild house mice. 2. A field experiment to examine the effect of food availability and food quality on breeding in spring. Wildlife Res. 14 (2), 197–206. doi: 10.1071/WR9870197
Bonduriansky R., Day T. (2009). Nongenetic inheritance and its evolutionary implications. Annu. Rev. Ecology Evolution Systematics 40, 103–125. doi: 10.1146/annurev.ecolsys.39.110707.173441
Börsch-Haubold A. G., Montero I., Konrad K., Haubold B. (2014). Genome-wide quantitative analysis of histone H3 lysine 4 trimethylation in wild house mouse liver: environmental change causes epigenetic plasticity. PloS One 9 (5), e97568. doi: 10.1371/journal.pone.0097568
Bronson F. H. (1979). The reproductive ecology of the house mouse. Q. Rev. Biol. 54 (3), 265–299. doi: 10.1086/411295
Cally J. G., Stuart-Fox D., Holman L. (2019). Meta-analytic evidence that sexual selection improves population fitness. Nat. Commun. 10 (1), 2017. doi: 10.1038/s41467-019-10074-7
Chevin L. M., Lande R., Mace G. M. (2010). Adaptation, plasticity, and extinction in a changing environment: towards a predictive theory. PloS Biol. 8 (4), e1000357. doi: 10.1371/journal.pbio.1000357
Cornwallis C. K., Uller T. (2010). Towards an evolutionary ecology of sexual traits. Trends Ecol. Evol. 25 (3), 145–152. doi: 10.1016/j.tree.2009.09.008
Cushing D. H. (1990). Plankton production and year-class strength in fish populations: an update of the match/mismatch hypothesis. Adv. Mar. Biol. 26, 249–293. doi: 10.1016/S0065-2881(08)60202-3
Dean M. D., Ardlie K. G., Nachman M. W. (2006). The frequency of multiple paternity suggests that sperm competition is common in house mice (Mus domesticus). Mol. Ecol. 15 (13), 4141–4151. doi: 10.1111/j.1365-294X.2006.03068.x
Dewsbury D. A. (1982). Pregnancy blockage following multiple-male copulation or exposure at the time of mating in deer mice, Peromyscus maniculatus. Behav. Ecol. Sociobiology 11, 37–42. doi: 10.1007/BF00297664
Duncan W. R., Wakeland E. K., Klein J. (1979). Heterozygosity of H–2 loci in wild mice. Nature 281 (5732), 603–605. doi: 10.1038/281603a0
Eccard J. A., Ylönen H. (2001). Initiation of breeding after winter in bank voles: effects of food and population density. Can. J. Zoology 79 (10), 1743–1753. doi: 10.1139/z01-133
Fernlund Isaksson E., Reuland C., Kahrl A. F., Devigili A., Fitzpatrick J. L. (2022). Resource-dependent investment in male sexual traits in a viviparous fish. Behav. Ecol. 33 (5), 954–966. doi: 10.1093/beheco/arac060
Firman R. C., Simmons L. W. (2008a). Polyandry facilitates postcopulatory inbreeding avoidance in house mice. Evolution 62 (3), 603–611. doi: 10.1111/j.1558-5646.2007.00307.x
Firman R. C., Simmons L. W. (2008b). Polyandry, sperm competition, and reproductive success in mice. Behav. Ecol. 19 (4), 695–702. doi: 10.1093/beheco/arm158
Fox R. J., Fromhage L., Jennions M. D. (2019). Sexual selection, phenotypic plasticity and female reproductive output. Philos. Trans. R. Soc. B 374 (1768), 20180184. doi: 10.1098/rstb.2018.0184
Frankenhuis W. E., Del Giudice M. (2012). When do adaptive developmental mechanisms yield maladaptive outcomes? Dev. Psychol. 48 (3), 628. doi: 10.1037/a0025629
Fuxjäger L., Wanzenböck S., Ringler E., Wegner K. M., Ahnelt H., Shama L. N. (2019). Within-generation and transgenerational plasticity of mate choice in oceanic stickleback under climate change. Philos. Trans. R. Soc. B 374 (1768), 20180183. doi: 10.1098/rstb.2018.0183
Garlovsky M. D., Holman L., Brooks A. L., Novicic Z. K., Snook R. R. (2022). Experimental sexual selection affects the evolution of physiological and life-history traits. J. Evolutionary Biol. 35 (5), 742–751. doi: 10.1111/jeb.14003
Goodson S. G., Zhang Z., Tsuruta J. K., Wang W., O'Brien D. A. (2011). Classification of mouse sperm motility patterns using an automated multiclass support vector machines model. Biol. Reprod. 84 (6), 1207–1215. doi: 10.1095/biolreprod.110.088989
Grafen A. (1990). Sexual selection unhandicapped by the Fisher process. J. Theor. Biol. 144 (4), 473–516. doi: 10.1016/S0022-5193(05)80087-6
Griffith S. C., Sheldon B. C. (2001). Phenotypic plasticity in the expression of sexually selected traits: neglected components of variation. Anim. Behav. 61 (5), 987–993. doi: 10.1006/anbe.2000.1666
Han C. S., Dingemanse N. J. (2017). You are what you eat: diet shapes body composition, personality and behavioural stability. BMC Evolutionary Biol. 17 (1), 1–6. doi: 10.1186/s12862-016-0852-4
Harrison S. J., Godin J. G., Bertram S. M. (2017). Influence of dietary nutrient balance on aggression and signalling in male field crickets. Anim. Behav. 134, 123–134. doi: 10.1016/j.anbehav.2017.10.006
Harvey S., Jemiolo B., Novotny M. (1989). Pattern of volatile compounds in dominant and subordinate male mouse urine. J. Chem. Ecol. 15, 2061–2072. doi: 10.1007/BF01207438
Head M. L., Jennions M. D., Zajitschek S. R. (2016). Sexual selection: incorporating non-genetic inheritance. Curr. Opin. Behav. Sci. 12, 129–137. doi: 10.1016/j.cobeha.2016.10.005
Hegyi G., Jenni-Eiermann S., Boross N., Garamszegi L. Z., Laczi M., Kötél D., et al. (2019). Ornaments and condition: plumage patch sizes, nutritional reserve state, reserve accumulation, and reserve depletion. Behav. Ecol. Sociobiology 73, 1–10. doi: 10.1007/s00265-019-2701-0
Ingleby F. C., Hunt J., Hosken D. J. (2010). The role of genotype-by-environment interactions in sexual selection. J. Evolutionary Biol. 23 (10), 2031–2045. doi: 10.1111/j.1420-9101.2010.02080.x
Katsuki M., Okada Y., Okada K. (2012). Impacts of diet quality on life-history and reproductive traits in male and female armed beetle, Gnatocerus cornutus. Ecol. Entomology 37, 463–470. doi: 10.1111/j.1365-2311.2012.01390.x
König B., Lindholm A. K., Lopes P. C., Dobay A., Steinert S., Buschmann F. J. U. (2015). A system for automatic recording of social behavior in a free-living wild house mouse population. Anim. Biotelemetry 3 (1), 1–15. doi: 10.1186/s40317-015-0069-0
König B., Lindholm A. K. (2012). The complex social environment of female house mice (Mus domesticus). In: Macholan M., Baird S. J., Munclinger P., Pialek J. eds. Evolution of the House Mouse. Cambridge: Cambridge University Press, 114–134. doi: 10.1017/CBO9781139044547.007
Krause E. T., Naguib M. (2014). Effects of parental and own early developmental conditions on the phenotype in zebra finches (Taeniopygia guttata). Evolutionary Ecol. 28, 263–275. doi: 10.1007/s10682-013-9674-7
Krause E. T., Naguib M. (2015). Zebra finch males compensate in plumage ornaments at sexual maturation for a bad start in life. Front. Zoology 12 (1), 1–7. doi: 10.1186/1742-9994-12-S1-S11
Lailvaux S. P., Irschick D. J. (2006). A functional perspective on sexual selection: insights and future prospects. Anim. Behav. 72 (2), 263–273. doi: 10.1016/j.anbehav.2006.02.003
Legros J., Tang G., Gautrais J., Fernandez M. P., Trannoy S. (2021). Long-term dietary restriction leads to development of alternative fighting strategies. Front. Behav. Neurosci. 14, 599676. doi: 10.3389/fnbeh.2020.599676
Lindström J. (1999). Early development and fitness in birds and mammals. Trends Ecol. Evol. 14 (9), 343–348. doi: 10.1016/S0169-5347(99)01639-0
Linnenbrink M., Teschke M., Montero I., Vallier M., Tautz D. (2018). Meta-populational demes constitute a reservoir for large MHC allele diversity in wild house mice (Mus musculus). Front. Zoology 15, 1–14. doi: 10.1186/s12983-018-0266-9
Linnenbrink M., von Merten S. (2017). No speed dating please! Patterns of social preference in male and female house mice. Front. Zoology 14, 1–4. doi: 10.1186/s12983-017-0224-y
Linnenbrink M., Wang J., Hardouin E. A., Künzel S., Metzler D., Baines J. F. (2013). The role of biogeography in shaping diversity of the intestinal microbiota in house mice. Mol. Ecol. 22 (7), 1904–1916. doi: 10.1111/mec.12206
Maan M. E., Seehausen O. (2011). Ecology, sexual selection and speciation. Ecol. Lett. 14 (6), 591–602. doi: 10.1111/j.1461-0248.2011.01606.x
Macartney E. L., Crean A. J., Nakagawa S., Bonduriansky R. (2019). Effects of nutrient limitation on sperm and seminal fluid: a systematic review and meta-analysis. Biol. Rev. 94 (5), 1722–1739. doi: 10.1111/brv.12524
Mackintosh J. H. (1970). Territory formation by laboratory mice. Anim. Behav. 18, 177–183. doi: 10.1016/0003-3472(70)90088-6
Manser A., Lindholm A. K., König B., Bagheri H. C. (2011). Polyandry and the decrease of a selfish genetic element in a wild house mouse population. Evolution 65 (9), 2435–2447. doi: 10.1111/j.1558-5646.2011.01336.x
Martins T. L. (2004). Sex-specific growth rates in zebra finch nestlings: a possible mechanism for sex ratio adjustment. Behav. Ecol. 15 (1), 174–180. doi: 10.1093/beheco/arg094
Mathias M. L., Nunes A. C., Marques C. C., Sousa I., Ramalhinho M. G., Auffray J. C., et al. (2004). Adaptive energetics in house mice, Mus musculus domesticus, from the island of Porto Santo (Madeira archipelago, North Atlantic). Comp. Biochem. Physiol. Part A: Mol. Integr. Physiol. 137 (4), 703–709. doi: 10.1016/j.cbpb.2004.02.003
Mehlis M., Rick I. P., Bakker T. C. (2015). Dynamic resource allocation between pre-and postcopulatory episodes of sexual selection determines competitive fertilization success. Proc. R. Soc. B: Biol. Sci. 282 (1817), 20151279. doi: 10.1098/rspb.2015.1279
Moczek A. P., Emlen D. J. (2000). Male horn dimorphism in the scarab beetle, Onthophagus taurus: do alternative reproductive tactics favour alternative phenotypes? Anim. Behav. 59 (2), 459–466. doi: 10.1006/anbe.1999.1342
Møller A., Jennions M. (2001). How important are direct fitness benefits of sexual selection? Naturwissenschaften 88, 401–415. doi: 10.1007/s001140100255
Monaghan P. (2008). Early growth conditions, phenotypic development and environmental change. Philos. Trans. R. Soc. B: Biol. Sci. 363 (1497), 1635–1645. doi: 10.1098/rstb.2007.0011
Moreau J., Thiéry D., Troussard J. P., Benrey B. (2007). Grape variety affects female but also male reproductive success in wild European grapevine moths. Ecol. Entomology 32 (6), 747–753. doi: 10.1111/j.1365-2311.2007.00930.x
Morehouse N. I. (2014). Condition-dependent ornaments, life histories, and the evolving architecture of resource-use. Am. Zoologist 54 (4), 591–600. doi: 10.1093/icb/icu103
Morehouse N. I., Raubenheimer D., Kay A., Bertram S. M. (2020). Integrating nutritional and behavioral ecology: Mutual benefits and new frontiers. Adv. Study Behav. 52, 29–63. doi: 10.1016/bs.asb.2020.01.002
Morimoto J., Wigby S. (2016). Differential effects of male nutrient balance on pre-and post-copulatory traits, and consequences for female reproduction in Drosophila melanogaster. Sci. Rep. 6 (1), 27673. doi: 10.1038/srep27673
Mousseau T. A., Fox C. W. (1998). The adaptive significance of maternal effects. Trends Ecol. Evol. 13 (10), 403–407. doi: 10.1016/S0169-5347(98)01472-4
Nederhof E., Schmidt M. V. (2012). Mismatch or cumulative stress: toward an integrated hypothesis of programming effects. Physiol. Behav. 106 (5), 691–700. doi: 10.1016/j.physbeh.2011.12.008
Oakeshott J. G. (1974). Social dominance, aggressiveness and mating success among male house mice (Mus musculus). Oecologia 15, 143–158. doi: 10.1007/BF00345742
Perry J. C., Rowe L. (2010). Condition-dependent ejaculate size and composition in a ladybird beetle. Proc. R. Soc. B: Biol. Sci. 277 (1700), 3639–3647. doi: 10.1098/rspb.2010.0810
Piersma T., Drent J. (2003). Phenotypic flexibility and the evolution of organismal design. Trends Ecol. Evol. 18, 228–233. doi: 10.1016/S0169-5347(03)00036-3
Prabh N., Linnenbrink M., Jovicic M., Guenther A. (2023). Fast adjustment of pace-of-life and risk-taking to changes in food quality by altered gene expression in house mice. Ecol. Lett. 26 (1), 99–110. doi: 10.1111/ele.14137
Price T. D. (2006). Phenotypic plasticity, sexual selection and the evolution of colour patterns. J. Exp. Biol. 209, 2368–2376. doi: 10.1242/jeb.02183
Qvarnström A., Price T. D. (2001). Maternal effects, paternal effects and sexual selection. Trends Ecol. Evol. 16 (2), 95–100. doi: 10.1016/S0169-5347(00)02063-2
Ricklefs R. E. (1984). The optimization of growth rate in altricial birds. Ecology 65 (5), 1602–1616. doi: 10.2307/1939139
Rowe L., Houle D. (1996). The lek paradox and the capture of genetic variance by condition dependent traits. Proc. R. Soc. London. Ser. B: Biol. Sci. 263 (1375), 1415–1421. doi: 10.1098/rspb.1996.0207
Sentinella A. T., Crean A. J., Bonduriansky R. (2013). Dietary protein mediates a trade-off between larval survival and the development of male secondary sexual traits. Funct. Ecol. 27 (5), 1134–1144. doi: 10.1111/1365-2435.12104
South S. H., House C. M., Moore A. J., Simpson S. J., Hunt J. (2011). Male cockroaches prefer a high carbohydrate diet that makes them more attractive to females: implications for the study of condition dependence. Evolution 65 (6), 1594–1606. doi: 10.1111/j.1558-5646.2011.01233.x
Stamps J. A. (2007). Growth-mortality tradeoffs and ‘personality traits’ in animals. Ecol. Lett. 10 (5), 355–363. doi: 10.1111/j.1461-0248.2007.01034.x
Tatar M., Carey J. R. (1995). Nutrition mediates reproductive trade-offs with age-specific mortality in the beetle Callosobruchus maculatus. Ecology 76 (7), 2066–2073. doi: 10.2307/1941681
Vanni M. J., Lampert W. (1992). Food quality effects on life history traits and fitness in the generalist herbivore Daphnia. Oecologia 92, 48–57. doi: 10.1007/BF00317261
Vincenzi S., Hatch S., Mangel M., Kitaysky A. (2013). Food availability affects onset of reproduction in a long-lived seabird. Proc. R. Soc. B: Biol. Sci. 280 (1760), 20130554. doi: 10.1098/rspb.2013.0554
Wallner B., Machatschke I. H. (2010). Influence of nutrition on aggression. CABI Rev. 17 (2009), 1–10. doi: 10.1079/PAVSNNR20094075
Wang Y. (2002). Epididymal sperm count. Curr. Protoc. Toxicol. 14 (1), 16–16. doi: 10.1002/0471140856.tx1606s14
Keywords: sexual selection, phenotypic plasticity, trans-generational effects, parental effects, life-history, food quality
Citation: Porwal N, Gangothri S, Agarwal H and Guenther A (2023) Does good food make male mice sexy? The influence of diet on male sexually selected traits. Front. Ecol. Evol. 11:1207480. doi: 10.3389/fevo.2023.1207480
Received: 17 April 2023; Accepted: 18 July 2023;
Published: 02 August 2023.
Edited by:
Ann Valerie Hedrick, University of California, Davis, United StatesReviewed by:
Andrea Pilastro, University of Padua, ItalyÁlvaro Navarro Castilla, Autonomous University of Madrid, Spain
Copyright © 2023 Porwal, Gangothri, Agarwal and Guenther. This is an open-access article distributed under the terms of the Creative Commons Attribution License (CC BY). The use, distribution or reproduction in other forums is permitted, provided the original author(s) and the copyright owner(s) are credited and that the original publication in this journal is cited, in accordance with accepted academic practice. No use, distribution or reproduction is permitted which does not comply with these terms.
*Correspondence: Anja Guenther, Z3VlbnRoZXJAZXZvbGJpby5tcGcuZGU=
†ORCID: Neelam Porwal, orcid.org/0000-0002-3739-124X
Anja Guenther, orcid.org/0000-0002-2350-0185