- 1School of Architecture, Tianjin University, Tianjin, China
- 2Tianjin Key Laboratory of Architecture Physical Environment and Ecological Technology, School of Architecture, Tianjin University, Tianjin, China
- 3School of Architecture, Tianjin Chengjian University, Tianjin, China
The significant ecological risks posed by artificial light at night (ALAN) are rapidly increasing globally. The rapid development of narrow-spectrum light-emitting diodes (LEDs) presents various challenges for reducing fatal attraction to ALAN and associated ecological risks. However, the potential risks and variations in the fatal attraction have not been precisely measured and assessed. Insects are ecological indicator species and photosensitive animals that are often fatally attracted to ALAN. In this paper, we conduct phototaxis experiments in Tianjin, China. We explored insect phototactic behavior and rhythms by comparing the effects of different time periods and spectra on the number of phototactic insects using UV light as a baseline. The spectra include seven narrow-spectrum lights with different peak wavelengths and two broad-spectrum lights with different color temperatures. In general, shorter wavelength light was more attractive: short-wave blue light (447 nm and 478 nm) was the most attractive, followed by medium-wave green light (500 nm and 519 nm) and long-wave red light (627 nm, 660 nm, and 740 nm). Insects were more attracted to 4,500 K LEDs than 3,000 K LEDs, but the difference in attraction between 4,500 K and 3,000 K LEDs was not significant. For eco-risk periods, that is, the peak hours of the fatal attraction of insects to ALAN in the field, LEDs attract insects at the fastest rate from 20:00 to 21:30 (from 1.5 hours to 3 hours after sunset). The phototactic rate curves of insect orders differed among different spectral LEDs. We proposed a method to predict the relative attractiveness of LEDs to insects and orders by calculating the relative attraction coefficient (Pi) of each narrow-spectrum LED and assigning Pi as a coefficient to the spectral irradiance percentage of the corresponding band of the LEDs to be predicted. The model-calculated relative attraction was highly significantly correlated with both the experimentally obtained attraction and the spectral response of insect vision. The results demonstrate that the attractiveness of broad-spectrum LEDs to insects can be altered, evaluated, and predicted through narrow-spectrum LEDs. Our findings will aid the development of ecological light sources, ecological conservation, and improvements in urban light ecology.
1 Introduction
Over the past two decades, light pollution has become one of the fastest-growing forms of environmental pollution, and it has dramatically altered the spatial and temporal extent, light intensity, and spectral properties of the natural light environment at night (Bennie et al., 2015; Falchi et al., 2016). Even at low levels of light pollution, prolonged and sustained exposure to light pollution can affect the behavior and physiology of individuals and have population-level consequences (Hoelker et al., 2010; Davies et al., 2013; Gaston et al., 2015; Bennie et al., 2016). Insects are photosensitive animals and ecological indicator species (Lawes et al., 2005; Ferrer-Paris et al., 2016). The abundance and biomass of insect populations are declining significantly worldwide, and ALAN, which is near ubiquitous in urban habitats and interferes with insect behavior, is thought to be one of the major drivers of declines in insect populations (Owens et al., 2020; Kalinkat et al., 2021). ALAN can induce the fatal attraction of positively phototactic insects (Bates et al., 2014; Plummer et al., 2016), which causes one-third of attracted insects to die before dawn due to exhaustion or predation (van Langevelde et al., 2018). For example, approximately 100 billion insects die each summer in Germany due to fatal attraction (Eisenbeis, 2006).
Nocturnal insects are generally phototactic (positively or negatively phototactic). For positively phototropic insects, most are trichromats, with photoreceptors’ spectral sensitivity concentrated in the UV light (340–380 nm), blue light (440–480 nm), and green light (480–580 nm) (van der Kooi et al., 2021), and are easily attracted to light sources rich in UV and short-wave visible light (van der Kooi et al., 2021). The phototropism of insects might significantly differ among orders. Some insects (such as most Lepidoptera and Hymenoptera) with broad visual sensitivity can be attracted to artificial light at wavelengths of 300–700 nm (Briscoe and Chittka, 2001; van der Kooi et al., 2021), whereas some flying insects are attracted to long-wave visible light, such as amber lights (610.72 ± 47.88 nm) (Owens et al., 2022a). The insect species and artificial light (spectral distribution and light intensity) determine the extent to which insects are attracted to artificial light. In particular, spectral variability can directly affect the attractiveness of artificial light (Owens et al., 2020). Previous studies of the effect of the spectrum on insects have mainly focused on pest control and insect conservation. Most research on pest control has focused on short-wave monochromatic light and used information on short-wave spectral sensitivity to improve pest prevention and control technologies (Shimoda and Honda, 2013; Pan et al., 2021). Research on insect protection has mainly focused on the attraction of insects to urban light sources (mainly street lighting) (Degen et al., 2016; De Medeiros et al., 2017) and photosensitive insects (Owens et al., 2022b). However, few field studies have explored the convergence of multiple insects on narrow-spectrum monochromatic light sequences (with different peak wavelengths in nm) with the aim of conserving insect diversity (Desouhant et al., 2019).
LEDs have become a widely used environmentally friendly light source for their high energy efficiency, spectral tunability, and other characteristics (Welbers et al., 2017; Cao et al., 2022). LEDs have also become the focus of ecological and environmental protection research because they pose novel ecological threats. Researchers believe that the peak of the spectral composition of LEDs is in the blue range, to which many insects appear to be sensitive (Longcore et al., 2015; Donners et al., 2018; Grubisic et al., 2018; Macgregor et al., 2019). Appropriate adjustments to the spectral composition of LEDs can reduce their ecological risks and allow them to become eco-friendly light sources, such as by reducing or eliminating threatening wavelengths in LEDs, which can reduce the attraction of insects to light and their mortality caused by the use of ALAN (Pimputkar et al., 2009; Longcore et al., 2015; Justice and Justice, 2016; Kamei et al., 2021; Martin et al., 2021). In view of the differences in phototaxis between insect species, changes in the spectral composition of nightscape lighting might induce phototactic responses of photosensitive insects with specific wavelengths. However, the precise responses that would be expected remain unclear, and this increases the uncertainty of the ecological risks of light pollution from ALAN.
In addition, the spectral composition of broad-spectrum LED white lights with different color temperatures (in K) can result in differences in insect attraction, such as differences between warm 2,200–2,700 K white light (peaks in the long wavelength light) and cool 5,000–6,500 K white light (peaks in the short wavelength light) (Longcore et al., 2015; Wakefield et al., 2016; Bolliger et al., 2020; Kamei et al., 2021). However, many studies have been conducted to compare insect attraction to LEDs with relatively significant color temperature differences, and differences in insect attraction to LEDs with typical and appropriate color temperatures (3,000–4,500 K) remain unclear (Bolliger et al., 2020; Kamei et al., 2021).
The extensive use of LEDs is rapidly changing the spectral composition of ALAN globally. The spectral energy distribution of LEDs could result in an increase or decrease in the attraction of insects. However, the spectral distribution of LEDs is complex, such as broad-spectrum LEDs that are characterized by the same color but different spectra. The complexity creates both opportunities and challenges for research on the conservation of phototactic insect diversity. The opportunity is to develop insect-friendly eco-light sources using homochromatic low-risk spectra. The challenge is that due to the complexity and diversity of the spectral distributions, substantial experiments are required to determine the attraction of insects to LEDs with different spectral distributions.
Phototactic rhythms are another important characteristic of nocturnal insects (Gaston et al., 2017). Many studies have explored the phototactic rhythms of nocturnal agricultural pests (Steinbauer and Weir, 2007; Qi et al., 2014). Insects have specific patterns of phototactic behavior, but the phototactic rhythms vary among insect species (Gaston et al., 2017). Most current studies have aimed at exploring the phototactic rhythms and peak phototaxis periods of specific pests through light trap experiments to improve the efficiency of light trapping and control agricultural and livestock losses caused by pests (Tu et al., 2016; Mi et al., 2019). Identifying the peak hours of the fatal attraction of insects to ALAN in the field, that is the eco-risk periods, can enhance the accuracy of assessments of the ecological risks of light pollution.
Studies of insect phototaxis have mainly focused on laboratory experiments (Owens and Lewis, 2018; Desouhant et al., 2019). Although laboratory experiments have many advantages, some advantages of field experiments cannot be replaced by laboratory experiments, such as ensuring the complexity and realism of experimental scenarios, as well as maintaining insect diversity and the actual living conditions of insects (Owens and Lewis, 2018).
In this study, we compared the effects of narrow-spectrum monochromatic light and broad-spectrum mixed white light in typical landscape LEDs on the phototropism, phototaxis behavior, and phototactic rhythms of insects in an urban–rural fringe, which is characterized by drastic changes in ALAN and the ecological environment. The research target is to identify the eco-friendly spectrum, eco-risk spectrum, and eco-risk periods, when insects are active at night, for different insect groups and orders, so as to reduce the threat of light pollution to insects. Meanwhile, we try to predict and assess the attraction of insects to LEDS with different spectral distributions by utilizing the typical narrow-spectrum light attraction patterns. We constructed a relative attraction model, which could greatly influence the assessment of the attraction of insects to landscape lighting, as well as the development and application of insect-friendly eco-light sources. The relative attraction model consists of two parts: the first is to determine the relative attraction coefficients for representative bands of the experimental narrow-spectrum light, and the second is to estimate the relative attraction of the predicted light source, which is to calculate the weight of each representative band in the spectral energy distribution of the predicted light source and assign the weight to the relative attractiveness coefficient. We aimed to provide information that will aid future research and the development of eco-friendly LEDs (with spectral optimization and temporal intelligent control). The model we constructed could provide new ideas and methods for rapidly predicting and assessing the attraction of insect groups and orders to LEDs that generally have different spectral distributions.
2 Materials and methods
2.1 Experimental sites
This study was conducted at the Beiyang Yuan Campus of Tianjin University in the urban–rural fringe of Jinnan District, Tianjin, China (Figure 1). Tianjin belongs to the Jing-Jin-Ji urban agglomeration, where urbanization and night lighting are increasing rapidly (Jiang et al., 2017). Tianjin has wetland nature reserves such as Tuanbowa, Beidagang, Qilihai, and Dahuangbao (Figure 1A). The urban–rural fringe, known as the region located between the urban and rural areas (Peng et al., 2016), has extensive ALAN (Cox et al., 2020) and is experiencing ecological instability due to urbanization (Cao et al., 2020). The urban–rural fringe selected for this experiment is a new urban area under continuous construction. The experimental area is located in the newly built campus (39.003°N, 117.317°E) within the new urban area. The campus has 35% green space and 11.9% water areas (Bennie et al., 2018). The proportion of green space of the experimental area is approximately 76% (Figure 1). Three types of green areas were selected as experimental sites: landscape woods (Site A, the average value of ambient light was 0.0005 W/m2), regularly mown lawns (Site B, 0.0007 W/m2), and waterfront areas (Site C, 0.0006 W/m2) at a linear distance of approximately 2 m from the river, which are typical urban green space habitats. The three experimental sites are similar in size and separated from each other by approximately 100 m or more. As these sites are located approximately 15–20 m away from existing light sources and the light sources around the sites were off during the experimental days due to being on holiday, these sites are not exposed to direct artificial lighting from the existing environment.
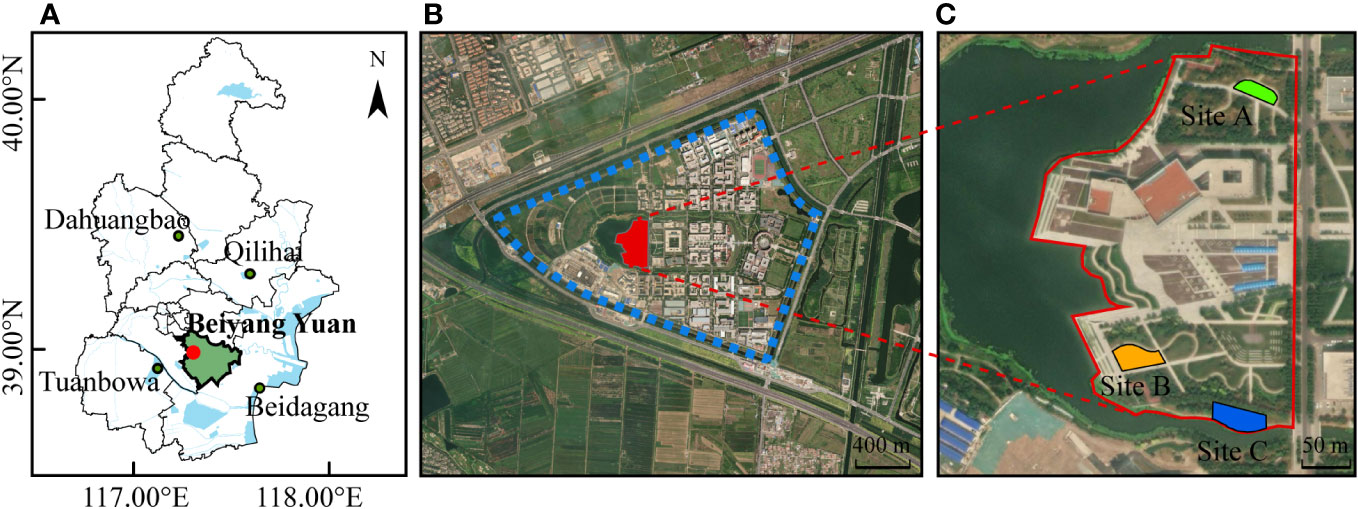
Figure 1 Location of the experimental area. (A) Location of Tianjin University Beiyang Yuan Campus in Jinnan District, Tianjin, China. (B) Beiyang Yuan Campus. (C) Experimental sites: Site (A), Site (B) and Site (C). These maps are provided by Mapbox (http://Mapbox.com).
2.2 Experimental equipment
The experimental setup unit was mainly comprised of an experimental shelf, a lamp, and a sticky board. The experimental shelf was 60 cm × 30 cm × 70 cm, including the upper experimental lamp frame and the lower sticky board frame (Supplementary Figure 1). The experimental lamp emitted light downward and was approximately 50 cm away from the sticky board below it. The spacing was fine-tuned to ensure that the irradiance of the board’s surface satisfied the irradiance required for the experiment (28.60 ± 0.50 W/m2), as described in 2.3. The sticky board was white cardboard evenly coated with sticky glue on one side; the size was 42.0 cm × 29.7 cm. The white sticky boards avoided interference with the spectrum and colour temperature of the experimental treatments. Compared with poison bottles, sticky boards for capture facilitate the identification of small insects, maintain their original form, and reduce errors in insect classification (Pawson and Bader, 2014).
2.3 Spectral and light intensity settings
Our experiment included ten light treatments (seven LED narrow-spectrum monochromatic lights with different wavelength peaks and two LED broad-spectrum mixed white lights with different color temperatures, and a UV light, Figure 2). The seven types of LED monochromatic lights are commonly used for nightscape lighting. The two types of LED white lights are rarely studied and have unclear effects on insect phototaxis. UV was included in this study because many light sources contain UV light, which potentially poses a threat to many animals (Longcore et al., 2018). In addition, the light sources in many previous studies contain UV or violet light; thus, UV can be used to bridge the results between these studies (Barghini and Souza de Medeiros, 2012). In this study, we used UV attractiveness as a baseline and compared narrow- and broad-spectrum light with UV light to determine the eco-friendly advantages and relative attractiveness of different spectral distributions. We divided the 10 experimental LEDs into four groups for comparative analysis according to the spectral distribution of artificial light and the diversity and number of insects: (1) UV, Royal Blue, and Blue; (2) Cyan and Green; (3) Red Orange, Deep Red, and Far Red; and (4) 4,500 K and 3,000 K white light.
Irradiance nicely approximates the brightness as it is perceived by non-human animals (Longcore et al., 2018; Owens and Lewis, 2018). Therefore, we used irradiance as the light parameter. We investigated the landscape lighting of green areas in four typical city parks in Tianjin and measured irradiance at 50 cm directly in front of the light source using a CL-500A spectroradiometer (Konica Minolta Holdings Inc, Tokyo, Japan). A distance of 50 cm from the light source was chosen for two reasons: (1) preliminary trials suggested this was the approximate distance most insects flew around existing light sources, and (2) this made the investigation comparable with existing studies (Pawson and Bader, 2014). We measured the irradiance of five types of landscape light sources (a total of 50 light sources), ranging from 10.59 to 46.67 W/m2, with an average of 28.60 W/m2. Based on this, we used an irradiance of 28.60 ± 0.50 W/m2 as the light source in this field experiment. This was the same level of illumination used in previous studies (Longcore et al. (2015); Farnworth et al. (2018).
To ensure that the irradiance of each experimental light source was consistent with the average irradiance (28.60 ± 0.50 W/m2), first, we adjusted the irradiance of the light source in an optical darkroom. We conducted standardized measurements using a CL-500A to ensure that the irradiance at 50 cm directly below the light source (that is the sticky board frame) was 28.6 ± 0.5 W/m2. Second, before the start of each daily experiment, we slightly moved the height of the sticky board frame up and down in the experimental sites and took measurements using a CL-500A spectroradiometer to make the irradiance 28.60 ± 0.50 W/m2 at the center of the sticky board frame under the light source.
2.4 Experimental process
Preliminary trials and formal experiments were completed from August 10 to September 9, 2020. In the preliminary trials, we used the no-light treatment as a control group. Within the control group, the count of insects captured by the white sticky boards was nearly zero in the original lighting environment of the site. This result of the control group confirmed the correctness of our decision not to establish a control group and instead use UV light as the baseline. Furthermore, the result demonstrated that the white color and the glue of the sticky boards were hardly attractive to insects. In addition, the experimental group treated with artificial light demonstrated the effectiveness of insect capture, affirming the suitability of maintaining a 50 cm distance between the experimental lamp and the sticky board. The formal experiment was conducted in four rounds, each in the order of Site A, Site B, and Site C (Supplementary Table 2). To minimize the potential effect of prior sampling activities, each round was separated by one day; there was thus a three-day ecological recovery period for each site. Nights with temperatures above 15°C and wind speeds less than 19 km/h are considered suitable for insects (van Langevelde et al., 2011; Pawson and Bader, 2014; Wakefield et al., 2016). During the experiment, the temperature ranged from 22°C to 29°C and the maximum wind speed was 13 km/h thereby ensuring that environmental factors, such as weather, did not interfere with the behavior of the insects. Astronomical twilight is the darkest and the last stage of dusk in the evening. At that time of the year, sunset was between 18:30 and 18:50 and the end of astronomical twilight was between 20:00 to 20:30. We measured the light environment of the experimental site at 20:00 on non-experimental days with irradiances ranging from 0.0003–0.0013 W/m2 (average irradiance 0.0007 W/m2). Therefore, the irradiance change of the light environment after 20:00 can be neglected. The weather and meteorological information from 20:00 to 24:00 of the experiment days are shown in Figure 3. The weather information was obtained from the China Meteorological Data Network (2023). And the Sunset Time, Astronomical Twilight, and Moon Phases were obtained from Time and Date (2023).
Based on the spatial scales of the experimental sites and previous studies (Pawson and Bader, 2014; Eccard et al., 2018), we arranged ten experimental spots with 3-m intervals in each site. An experimental setup unit was randomly placed at an experimental spot. The experiments were conducted for 4 h from 20:00 to 24:00 when the natural nighttime light environment was mostly stable, and the ALAN interference was strongest. Insect phototaxis requires a certain amount of dark adaptation time, which generally begins 30–60 min after sunset (Jing and Lei, 2004). Therefore, on each experimental day, we turned on the lighting at 19:50 and measured the irradiance of the sticky boards. From 20:00 to 24:00, with 0.5 hours as the unit time, we changed the sticky boards every half hour to obtain the number of phototactic insects within each time unit. The sticky boards were labeled with information about the date, site, spectrum, and time period.
2.5 Statistical analysis
Insect samples were identified using an Olympus dissecting microscope, and the order and number of phototactic insects were determined for each period under various artificial lights. The K-S normality test of the single-sample non-parametric test was performed for the number of insects in each spectrum and each insect order. The P-values of the significance tests obtained from the K-S test were all less than 0.05, indicating that the data were not normal. Therefore, we used the median to describe the number of insects. We used multiple independent-samples Kruskal-Wallis tests (Bonferroni correction, P<0.05) to analyze whether there were differences in the number of insects captured by the 10 LEDs among the different spectra and eight periods. Descriptive statistical analyses were performed.
We analyzed variation in the attraction of insects to artificial light at different periods using the phototactic rate as a parameter. The phototactic rate was calculated as follows. We analyzed spectral and temporal variation in the attraction of insects to artificial light by characterizing variation in the phototactic rate with time and the decay speeds of the phototactic rates. Moreover, we analyzed the risk spectra and time periods by extracting periods in which phototactic rates were greater than the median and twice the median. We excluded high-risk spectral UV and calculated the median phototactic rate for nine spectra and eight periods for each insect order. We assigned a value of 1 to the periods when the phototactic rate was higher than the median and 2 to the periods when it was two times higher than the median.
Where NP is the number of phototactic insects per period, and NT is the total number of phototactic insects.
We used generalized linear mixed models (GLMMs) to analyze the effects of spectral types and time period on insect orders and numbers (Zuur et al., 2010). First, in the Data Structures of the GLMM analysis interface, we specified structural relationships between records in the dataset to account for correlations among insect captures. We set site, spectral type, and time period as Subjects and set the experimental date as the Repeated Measures in Effects. Second, in the Fields and Effects interface, the Target was related to the Fixed effects via a specified link function. We set the total number of insects and the number of Diptera, Hemipteran, Coleoptera, and Lepidoptera as the Target variables (response variables). We set site, spectral type, and time period as fixed effects (predictor variables) and the date as a Random effect. Given that the number of insects were counted and the transition was discrete, we used the “negative binomial regression” as the link function. We performed 1,000 iterations of the GLMM with a 95% confidence level. We determined whether the model converged by parameter convergence and visual inspection, with the parameter convergence value set to 0.000001; the threshold for statistical significance for all parameters was P<0.05, and the number of samples was >400 (Kamei et al., 2021). In addition, the estimated means of the spectra were analyzed, and deviation in the spectra was determined based on the original values of the target scale. Deviation contrasts compare the number of insects captured in each spectrum to the mean number in all spectra. GLMMs were analyzed for all insects and four dominant insect orders (Diptera, Hemiptera, Coleoptera, and Lepidoptera). To ensure the robustness of the statistical analysis, other insect orders with low numbers were not analyzed. The experimental data were analyzed, and graphs were made using SPSS R 26.0 and Origin 2022, respectively.
2.6 Relative attraction model construction
According to spectral attraction patterns, we quantified the relative attraction of narrow-spectrum LEDs to insects and proposed a method for predicting and evaluating the relative attraction of insects to broad-spectrum LEDs in landscapes, which is described below.
Representative bands of narrow-spectrum light. According to the spectral energy distribution of eight narrow-spectrum lights, we divided the visible light from 360 to 780 nm into eight bands, using the intersection of the spectral energy distribution curves of the adjacent narrow-spectrum light as the boundary (Figure 2).
Relative attraction coefficient (Pi). The relative attraction of insects to different experimental light sources was calculated based on the average number of captured insects (ANCI) per 0.5 h. We set the ANCI per 0.5 h of UV light (369 nm) to 1.000, and the ratios of ANCI/0.5 h of the narrow-spectrum lights to ANCI/0.5 h of the UV light was the relative attraction coefficient of the representative bands of each narrow-spectrum light, which was referred to as the relative attraction coefficient (Pi). Pi was calculated as follows. Where ANCI′ is the ANCI per 0.5 h, the is ANCI′ of the narrow-spectrum lights, and the is ANCI′ of the UV light.
Validation of relative attraction coefficient (Pi) We compared the Pi of the representative bands with the spectral response model proposed by Donners et al. (2018).
Estimation of the relative attraction of the predicted light source (ε). According to the light source’s spectral energy distribution, each wavelength’s spectral irradiance was calculated as the proportion of the total irradiance (i.e., the percentage of spectral irradiance). The Pi of each representative band was then assigned a weight to the spectral irradiance share of that band. The estimated relative attraction of the light source ϵ was calculated as follows.
where i is the i band within the n (n ≤ 8) representative bands, and the wavelength range is ai – bi. Ai,λ is the percentage of spectral irradiance at wavelength λ in the i band. SI is the spectral irradiance (unit W/m2), and SIi,λ is the spectral irradiance of wavelength λ in the range ai – bi of the i-band. Pi is the relative attraction coefficient of the i-band obtained from the previous calculation. ε is the relative attraction of the predicted light source using UV light as a baseline. εUV is the relative attraction of UV light estimated using this method.
In addition, we compared the relative attractions ε with the experimentally obtained relative attraction coefficients Pi for the 4,500 K and 3,000 K LEDs, as a cross-validation of the experimental and predicted models. And we performed median comparisons, paired-sample t-tests, and correlation analyses.
3 Results
During the 12-day field experiment of insect phototaxis, a total of 45,431 insects under 14 insect orders were captured, including 27,728 (61.03%) of Diptera, 8,925 (19.65%) of Hemiptera, 5,028 (11.07%) of Coleoptera, 2,602 (5.73%) of Lepidoptera, 488 (1.07%) of Hymenoptera, 301 (0.66%) of Trichoptera, and 359 (0.79%) of eight other orders. The phototactic insects captured were mainly from the orders Diptera, Hemiptera, Coleoptera, and Lepidoptera, which accounted for 97.47% of all insects. We used these four major phototactic insect orders to study the phototactic behavior of insects.
The Kruskal-Wallis test revealed significant differences in the number of insects among different spectra (P<0.001)and among time periods from 20:00 to 24:00 (P<0.001). The results of the GLMMs analysis showed that “Site” (F=0.420, p=0.657) was not statistically significant, meaning that there was no significant difference in the number of insects between the three sites. Therefore, we excluded the “site” factor and reanalyzed the results to obtain the fixed effects of each factor (Table 1). There were significant differences in the number of insects under different spectral distributions (F=494.214, P<0.001), periods (F=61.741, P<0.001), and insect orders (F=1518.306, P<0.001).
3.1 Spectral effect on insect phototaxis
Overall, the average daily median number of insects captured under each experimental LED was 228, with a range of 2 – 2311. The median, mean, and standard error of the number of insects captured per 0.5 h during the experimental period are shown in Figure 4. These four dominant insect orders exhibited similar patterns of attraction to the spectral distribution, with the strongest attraction to UV, followed by blue, which was approximately 20% of the insects captured by UV. The attraction was moderate in the Royal Blue, Green, Cyan, 4,500 K, and 3,000 K treatments. Attraction was weak in the Far Red, Deep Red, and Red Orange treatments.
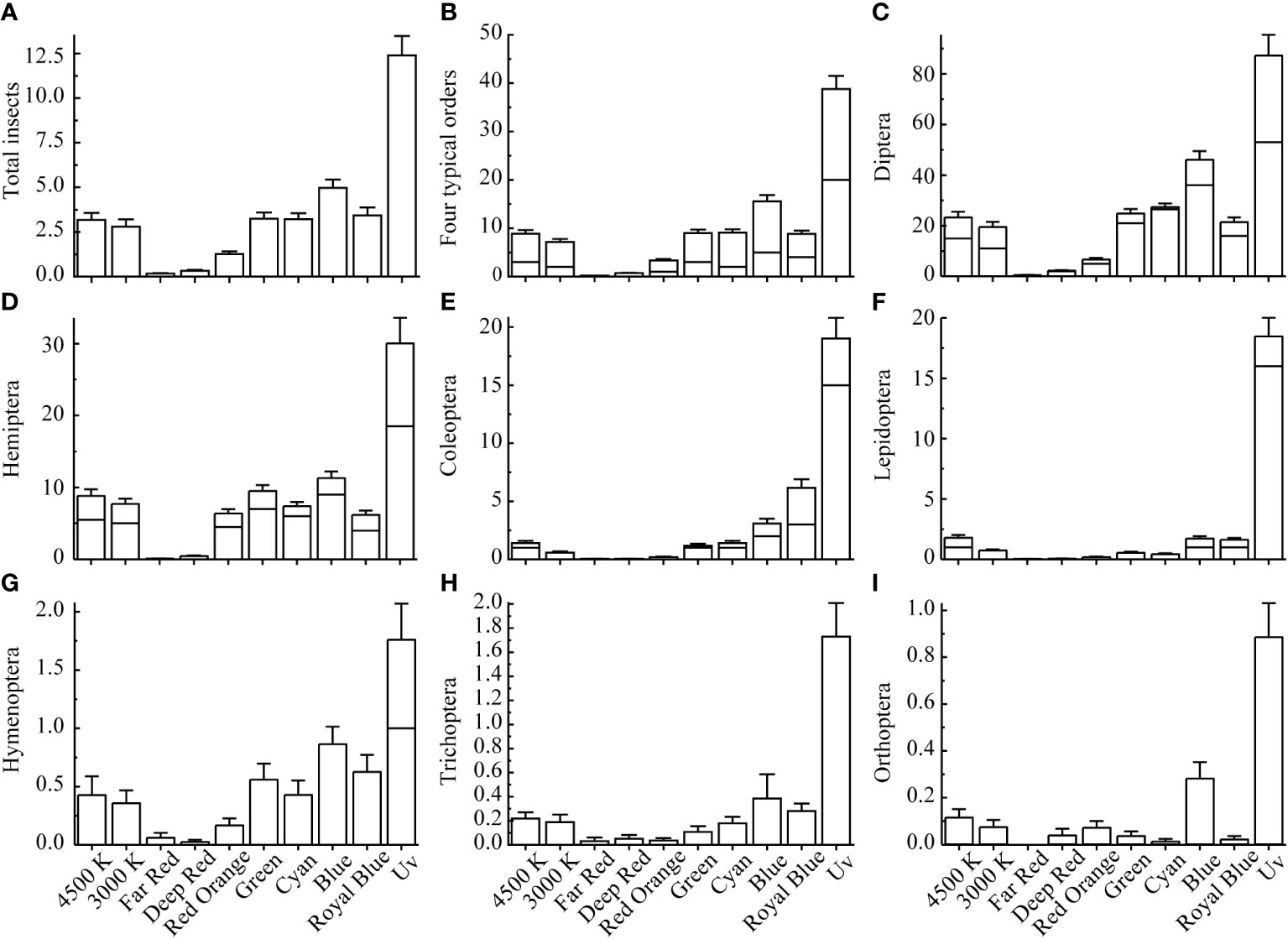
Figure 4 Descriptive statistics of the number of insects captured per unit period. (A) All insects, (B) Four dominant insects, (C) Diptera, (D) Hemiptera, (E) Coleoptera, (F) Lepidoptera, (G) Hymenoptera, (H) Trichoptera, and (I) Orthoptera. The middle of the box is the median line, the upper end of the box is the mean line, and the standard error line indicates SE.
The deviation estimates (Figure 5) revealed that, with the exception of the UV treatment, captures were highest in the Blue and Royal Blue treatments; captures in the 4,500 K, Green, and Cyan treatments were slightly higher than the mean, but captures in these treatments did not significantly differ from the mean (P<0.05) (Figure 5A). The captures in the 3,000 K, Far Red, Deep Red, and Red Orange treatments were lower than the mean value; captures in the 3,000 K treatment did not significantly differ from the mean value. According to the estimated mean values of the GLMM for different spectra (Supplementary Table 1), the number of captures in the 4,500 K LED treatment was estimated to be 1.43 times higher than that in the 3,000 K LED treatment (1.43 times for 4,500 K/3,000 K), 8.40 times for UV/4,500 K, 12.00 times for UV/3,000 K, 1.65 times for Blue/4,500 K, and 2.36 times for Blue/3,000 K. In narrow-spectrum light, the shorter wavelength light is more attractive. Short-wave blue light (Royal Blue, 447 nm; Blue, 478 nm) was the most attractive, followed by medium-wave green light (Cyan, 500 nm; Green, 519 nm) and long-wave red light (Red Orange, 627 nm; Deep Red, 660 nm; Far Red, 740 nm).
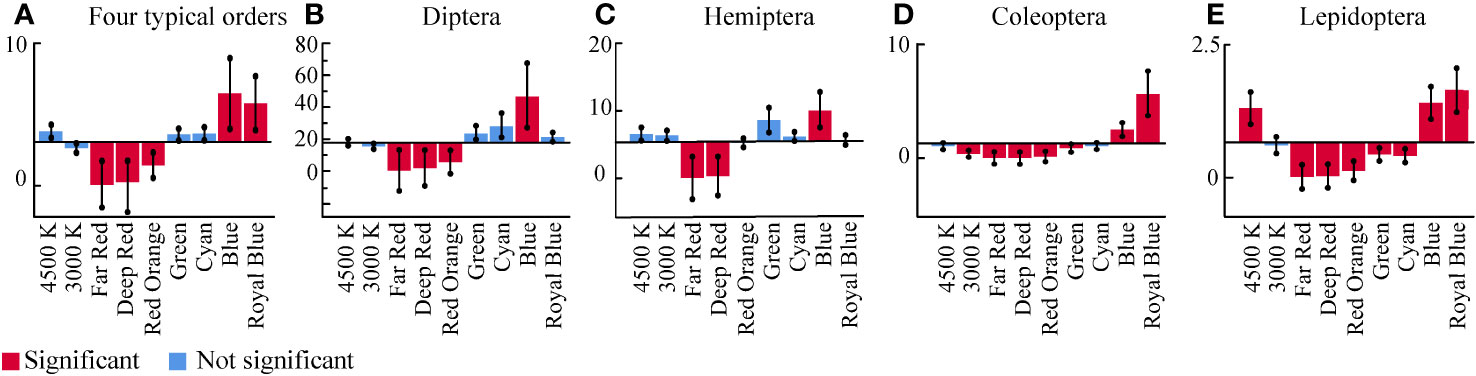
Figure 5 Deviation estimate plotted by SPSS. Deviation between the number of attracted insects and the overall estimated mean value for all experimental spectra. (A) Four insect orders, (B) Diptera, (C) Hemiptera, (D) Coleoptera, (E) Lepidoptera. The horizontal line is the overall estimated mean value for the number of insects. Vertical boxes are the result of deviation comparisons. The significance level was less than 0.05.
We used the deviation estimates to distinguish between eco-risk spectra and eco-friendly spectra of dominant insect orders (Figure 5). The deviation estimate is the comparison between each level of the factor and the overall mean. The spectra in which the number of insects captured was above the mean and significantly different from the mean were defined as High eco-risk spectra. The Blue for Diptera and Hemiptera, the Royal Blue and Blue for Coleoptera, and Royal Blue, Blue and White 4,500 K for Lepidoptera were High eco-risk spectra. The spectra in which the number of insects captured was not significantly different from the mean were defined as Moderate eco-risk spectra. The narrow-spectrum light (Green, Cyan, and Royal Blue) and broad-spectrum light (4,500 K and 3,000 K) were Moderate eco-risk spectra for Diptera and Hemiptera. The Cyan and 4,500 K for Coleoptera and the 3,000 K for Lepidoptera were Moderate eco-risk spectra. The spectra in which the number of insects captured was below the mean and significantly different from the mean were defined as Low eco-risk spectra, which can also be called eco-friendly spectra. The Far Red, Deep Red, and Red Orange for all insect orders, and Green and 3,000 K for Coleoptera and Lepidoptera were Low eco-risk spectra.
3.2 Spectral–temporal effects on the phototactic rhythm of insects
3.2.1 All insect orders
Throughout the experiment, sunset was between 18:30 and 18:50 and the end of astronomical twilight was between 20:00 to 20:30. Diptera captures were the most common across the entire experimental period (20:00–24:00), and the proportion of Diptera captures was significantly higher during the 20:30–21:00 period; Diptera captures after this peak period varied (Figure 6). The proportion of Hemiptera was the second largest; Hemiptera captures varied significantly between 20:00 and 21:30 but were stable in the other periods. The proportion of Coleoptera captures decreased rapidly from 20:00 to 21:00. The proportion of Lepidoptera captures was initially small and gradually increased with time.
During the study period, the overall attraction of the insect community to artificial light was highest between 20:00 and 21:30 (Figure 7). The overall phototaxis of the insect community gradually decreased over time. During 20:00–21:30, the phototactic rate decreased the fastest; after 21:30, the decline in the phototactic rate gradually slowed. The phototactic rate of these four orders of insects decreased gradually, but the attraction to the artificial light of different orders varied over time. The attraction of Diptera to artificial light was highest between 20:00 and 21:00, decreased significantly during 21:01–21:30, and then decreased. The phototactic rate curves of Hemiptera, Coleoptera, and Lepidoptera to artificial light were similar; the attraction was highest in 20:00–20:30. The attraction of Hemiptera and Coleoptera decreased significantly in 20:31–22:30, and the attraction of Lepidoptera decreased steadily from 20:31 to 22:00.
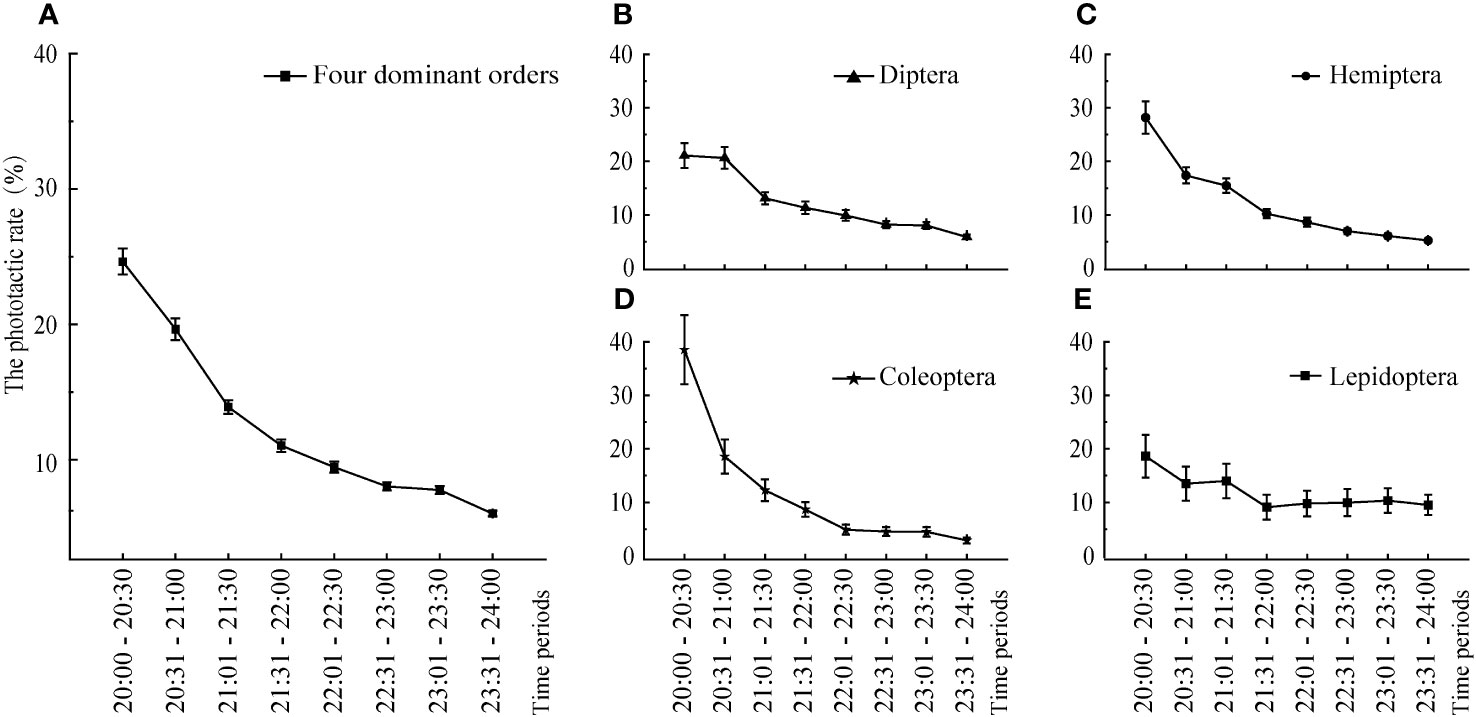
Figure 7 The phototactic rates of the dominant orders over time. Data are median ± SE. (A) Four dominant orders, (B) Diptera, (C) Hemiptera, (D) Coleoptera, (E) Lepidoptera.
3.2.2 Four dominant insect orders
The phototactic rate with time and the decay speeds of the phototactic rates are shown in Figures 8, 9, respectively. In Figure 9, P1–P8 corresponds to the eight periods from 20:00–20:30 to 20:30–24:00, respectively. Decay speed is equivalent to the change in phototactic rates. Red indicates decay speeds<0, meaning that the phototactic rate in the following period was lower than that in the previous period; blue indicates decay speeds >0, meaning that the phototactic rate in the following period was higher than that in the previous period. In Figure 9, the sum of the absolute values of the minimum and maximum phototactic rates is 1 for each insect order and spectrum.
Consistent variation was observed in changes in phototactic rates with time for each insect order under experimental LEDs with different spectral distributions, and phototactic rates generally decreased steadily or in a fluctuating manner. The phototactic rates of Diptera did not vary significantly at 20:00–20:30 and 20:30–21:30 and only slightly decreased or increased; however, after 21:30, the phototactic rates rapidly decreased. The phototactic rates of Hemiptera decreased significantly at 20:30–21:30, especially in the UV, Royal Blue, and Blue treatments. In the Cyan, Green, Red Orange, 4,500 K, and 3,000 K white light treatments, the phototactic rates of Hemiptera consistently decreased. The decrease in the phototactic rates of Coleoptera under narrow-spectrum lights was most pronounced during 20:30–21:30. Under broad-spectrum white light, the phototactic rates of Coleoptera decreased gradually. Under narrow-spectrum lights, the phototactic rates of Lepidoptera were highest in the first four periods. Under white lights, the phototactic rates decreased significantly at 20:30–21:30. When the number of phototactic insects was relatively small, changes in phototactic rates and the rate of decrease over time were variable.
The risk spectra and time periods in which phototactic rates were higher than the median and two times the median, as seen in Table 2. The periods and spectra in the table are indicated by the abbreviations mentioned above. The peak phototactic rate of Diptera and Coleoptera was concentrated at 20:00–22:00, and that of Hemiptera and Lepidoptera was concentrated at 20:00–21:30. The risk spectra were Blue and Cyan for Diptera; Blue and Green for Hemiptera; Royal Blue, Blue, and Cyan for Coleoptera; and Royal Blue, and Blue for Lepidoptera. Diptera and Hemiptera were consistently affected by the two broad-spectrum LED white lights. The risk of 4,500 K white light was significantly higher than that of 3,000 K white light for Coleoptera and Lepidoptera. In each time period, spectra with a value of 0 can be selected for spectral combinations of eco-friendly lighting, which is expected to reduce the attractiveness of ALAN to insects.
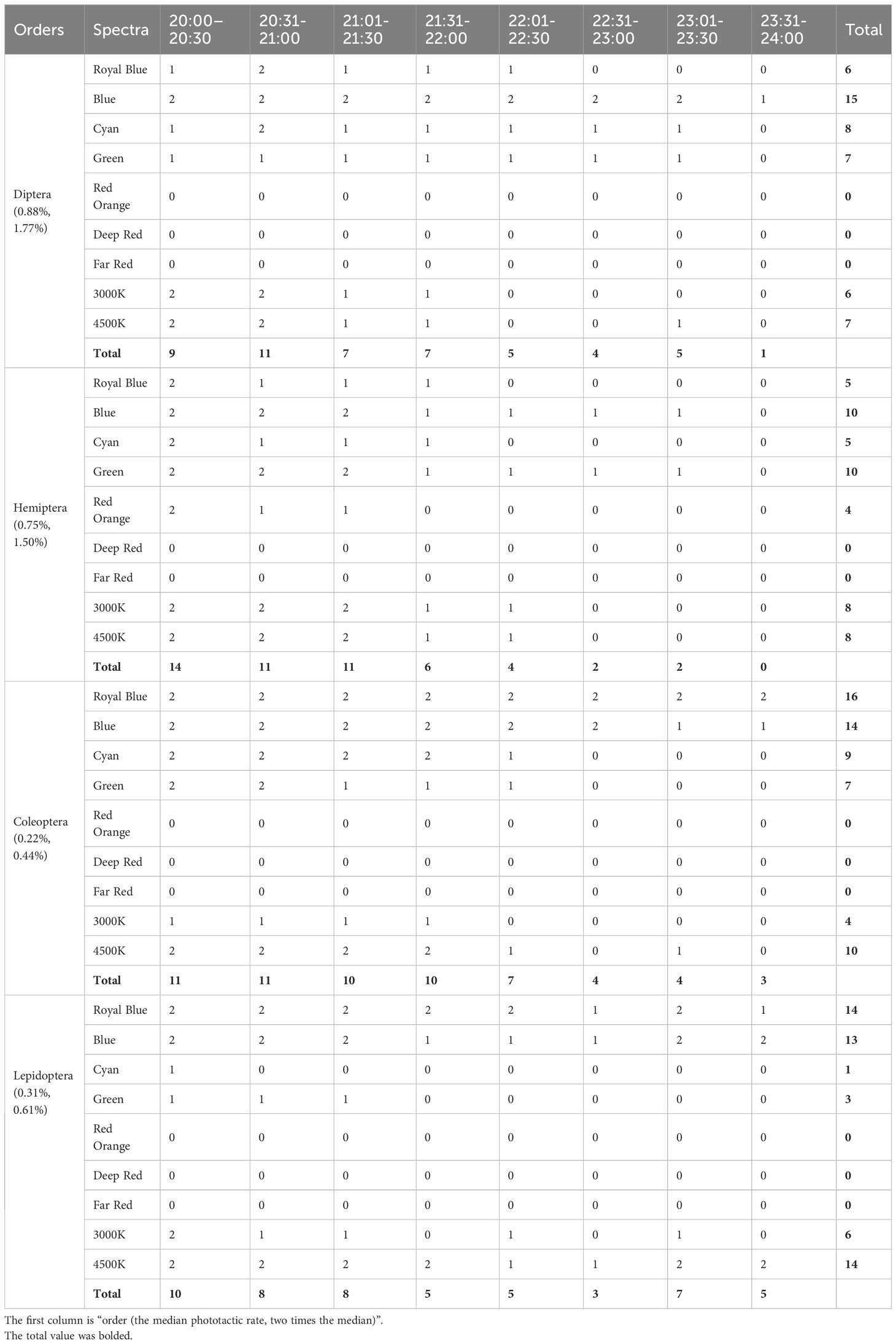
Table 2 The spectra and time periods in which phototactic rates were higher than the median and two times the median.
3.3 Relative attraction model of artificial light
3.3.1 Model calculation
Based on the experimental data, the relative attraction coefficient Pi of each narrow-spectrum light representative band for Lepidoptera, Hemiptera, Diptera, Coleoptera, and all insects was calculated (Table 3).
3.3.2 Model validation
Correlations of the relative attraction coefficients of all insect orders, Diptera, Hemiptera, Coleoptera, and Lepidoptera to the eight narrow-spectrum light bands with the spectral responses were determined as proposed by Donners et al. (2018). The Spearman correlation coefficients between Pi and the spectral response were 0.941, 0.871, 0.874, 0.972, and 0.941 for all insect orders, Diptera, Hemiptera, Coleoptera, and Lepidoptera, respectively, and these correlations were significant at the 0.01 level (two-tailed). There was thus a high degree of confidence in the relative attraction coefficient obtained using this method.
In addition, we predicted the relative attraction ϵ for the broad-spectrum experimental light sources 4,500 K and 3,000 K LEDs (Figure 10A) using the equations. We compared ϵ with the experimentally obtained Pi (Table 4). For 3,000 K LEDs, the median values of Pi and ϵ were 0.050 and 0.053, respectively, with P=0.174 (P>0.05) for the paired-sample t-test and Spearman correlation coefficient of 0.980 (P=0.003). For the 4,500 K LEDs, the median values of Pi and ϵ were 0.100 and 0.095, respectively, with P=0.09 for the paired-sample t-tests and Spearman correlation coefficient of 0.964 (P=0.008). There was no significant difference in the relative attractiveness between the predicted values using the model and the values obtained from the experiments in the 3,000 K and 4,500 K LED treatments.
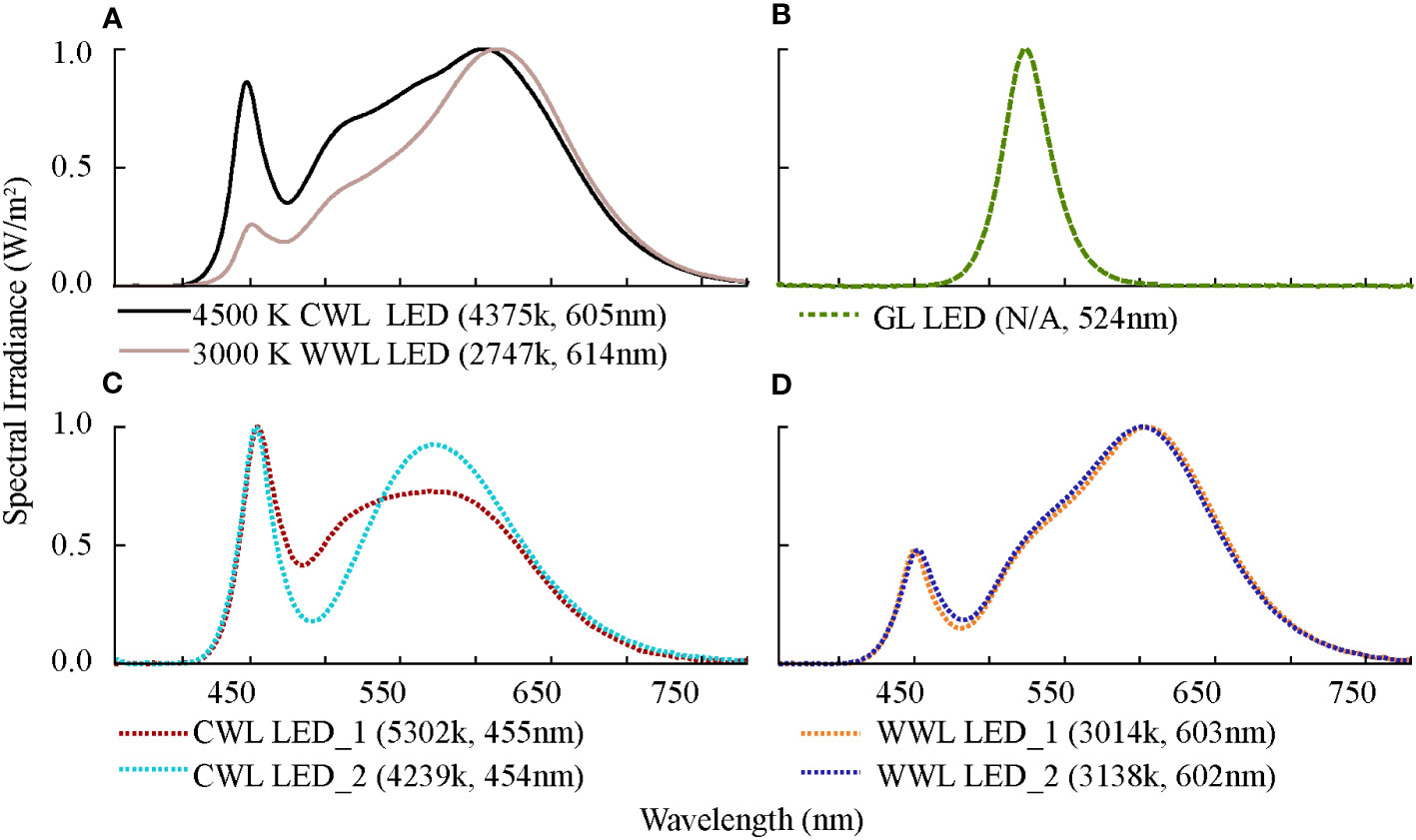
Figure 10 Spectral distribution and basic information of seven typical landscape LEDs. GL LED refers to green-light LED, CWL LED_1 and CWL LED_2 represent two types of cool white-light LEDs, and WWL LED_1 and WWL LED_2 represent two types of warm white-light LEDs. (A) 4500 K CWL LED and 3000 K CWL LED, (B) GL LED, (C) CWL LED, (D) WWL LED.
3.3.3 Model application
We used the relative attraction model to quantify the relative attraction of insects to typical landscape lighting sources. We investigated three city parks in Tianjin (Olympic Sports Center, People’s Park, and South Cui Ping Park) and collected information on five typical landscape LEDs. Their spectral distribution and basic information are shown in Figure 10, including one green-light LED (Figure 10B), two cool white-light LEDs with peak wavelengths in the blue band (Figure 10C), and two warm white-light LEDs in the orange band (Figure 10D). The relative attraction of the five typical landscape LEDs is shown in Table 5. The relative attraction was highest for green-light LEDs, followed by cool white-light LEDs and warm white-light LEDs. The attraction risks were highest for Hemiptera, followed by Coleoptera, Lepidoptera, and Diptera.
4 Discussion
We explored spectral and spectral–temporal variation in the attraction of insects to narrow-spectrum monochromatic light and broad-spectrum mixed white light, quantified the relative attraction of insects to narrow-spectrum light, and proposed a model to predict the relative attraction of insects to landscape light sources. Insect orders were ranked according to the number of attracted insects from largest to smallest (Diptera, followed by Hemiptera, Coleoptera, and Lepidoptera), and these findings are consistent with the results of van Grunsven et al. (2014); Wakefield et al. (2016). Diptera was the most abundant insect order, accounting for 61.03% of the captured insects.
We found significant differences in the attraction of insects to different wavelengths of monochromatic light. In general, shorter wavelengths resulted in greater attraction. Short-wave blue light, such as Royal Blue (447 nm) and Blue (478 nm), were the most attractive, followed by medium-wave green light, such as Cyan (500 nm) and Green (519 nm), and long-wave red light, such as Red Orange (627 nm), Deep Red (660 nm), and Far Red (740 nm). Generally, insects have three photosensitivity peaks, UV (≈350 nm), blue (≈440 nm), and green (≈530 nm) (Sun et al., 2014; Park and Lee, 2017; Kamei et al., 2021). However, the attraction of insects to narrow-spectrum light varied among orders; for example, the attraction of Coleoptera and Lepidoptera to Royal Blue (447 nm) was significantly higher than that of Diptera and Hemiptera, which is similar to the results of previous studies. Coleoptera and Lepidoptera clearly and consistently attracted to violet light at 395 nm and 418 nm (Pan et al., 2021). Given that insects are fatally attracted to UV, light sources that contain UV, such as mercury vapor and metal halide lamps, should be avoided (Owens and Lewis, 2018).
There may be differences in spectral sensitivity between different species of the same insect order. Sun et al. (2014) showed that Rapholitha molesta (Lepidoptera: Olethreutidae) is sensitive to short-wavelength and medium-wavelength artificial light; it is strongly attracted to green light (peak wavelength: 520; wavelength range: 500–570 nm), followed by violet (421; 400–450 nm) and blue (470; 450–500 nm). Such variation might stem from differences in spectral sensitivity between orders, regional variation, or the relatively low abundance of insects (Briscoe and Chittka, 2001).
In addition, the spectral composition of broad-spectrum LEDs can affect the attraction of insects. Captures of four dominant insect orders were 1.43 times higher in the 4,500 K LED treatment than in the 3,000 K LED treatment. The number of insects captured by the 4,500 K LED was more similar to the number of insects captured in the Cyan (500 nm) and Green (519 nm) treatments compared with the 3,000 K LED. This can be attributed to the fact that the 4,500 K LED contains more energy in the blue wavelength band than the 3,000 K LED. In a previous study, 3,500 K LEDs were shown to be more attractive to arthropods than 2,700 K LEDs (Longcore et al., 2015). However, no significant differences in the attraction of Diptera and Hemiptera to the 4,500 K and 3,000 K LEDs were detected according to a GLMM analysis; this is consistent with the results of a previous study showing that the color temperature of LEDs did not affect the attraction of insects in the orders Diptera and Lepidoptera (Pawson and Bader, 2014; Wakefield et al., 2016). Although differences caused by color temperature were not significant, cool color temperature LEDs were more attractive than warm color temperature LEDs. Therefore, a high amount of short-wave light in the LED spectrum might have stronger deleterious ecological effects (Kamei et al., 2021). Attraction to nocturnal arthropods can be minimized by adjusting the spectral composition of white-light LEDs (Longcore et al., 2015). The high efficiency, low energy requirements, and low cost of LEDs have contributed to the large-scale use of broad-spectrum and narrow-spectrum LEDs in municipal and landscape lighting. In China, the market share of LED lighting products grew from 65% in 2017 to 80% in 2021 and is expected to reach 83% in 2022 (AskCI, 2022). Therefore, efforts to reduce ecological stress should focus on spectral modulation and controlling the quantity and brightness level of lighting (van Grunsven et al., 2014).
According to Owens and Lewis (2018); Owens et al. (2020), some positive phototactic insects hover around light traps until they are injured, exhausted, or dead. We assume that insects attracted by artificial light cannot escape the attraction until the light source is turned off; that is, the number of attracted insects continues to increase. Under this assumption, insects are continuously attracted to artificial light throughout the night when artificial light is present. At the time when ALAN and the ecosystem were in strongest conflict (20:00-24:00), the nocturnal attraction was strongest during 20:00–21:30, with sunset between 18:30 and 18:50. Peak phototactic periods were identified in previous studies: from 19:45 to 21:30 for Heteronyx chlorotica (summer; with sunset between 18:06 and 19:32; latitude 35°09’55.7″ S, longitude 149°02’49.9″ E; altitude 615 m above sea level) (Steinbauer and Weir, 2007); from 20:00 to 21:30 for Hemiptera pests, such as rice planthoppers and Cyrtorhinus lividipenni (summer; with sunset between 18:50 and 19:12; latitude 22°38′17.54″ N, longitude 114°05′52.35″ E) (Yang et al., 2012; Qi et al., 2014); from 22:00 to 2:00 the following day for some Lepidoptera insects (summer; with sunset between 21.00 and 22.00; latitude 46°30′0.00″ N, longitude 10°0′0.00″ E) (Knop et al., 2018). Peak phototactic periods varied among different species of insects, but they were primarily concentrated from 1 to 3 hours after sunset. Some species of insects had multiple peak phototactic periods. Heliothis nubigera had two peak periods, with the first peak occurring around 20:00 (~3 hours after sunset) and the second one around midnight (at temperatures ranging from 22 °C–32 °C; sunset at 18:20; latitude 32°6′49.068″ N, longitude 34°48′15.732″ E)(Kravchenko et al., 2021). For Heteronyx praecox, peak phototactic periods were observed at 21:30 and 23:15 during summer (with sunset between 18:06 and 19:32; latitude 35°09’55.7″ S, longitude 149°02’49.9″ E; altitude 615 m above sea level) (Steinbauer and Weir, 2007). These results demonstrated the consistency and difference of phototactic rhythms in different insect species and the relationship between phototactic rhythms and sunset time. In addition, the attraction of most insects gradually decreased after 21:30. (Qi et al., 2014; Cai et al., 2016). Similar to the results of Qi et al. (2014), the peak phototactic behavior of some insect orders was maintained for a few minutes to a few ten minutes before the number of phototactic insects rapidly diminished and remained at a low level until dawn the following day. The daily appearance times and activity rhythms of nocturnal insects are determined by an internal clock affected by ambient light or temperature (David, 2009; Tataroglu and Emery, 2014). If ALAN is sufficiently intense and temporally persistent and has a specific spectral composition, long-term exposure to ALAN may alter the internal biological clock of insects, which can alter their activity patterns synchronously with the photoperiod (Owens and Lewis, 2018). In this study, we observed differences in insect phototactic behavior and rhythms under different artificial light spectra and used this information to optimize the spectral and temporal combinations of ecological lighting to minimize the adverse effects of ALAN. For example, time controllers and motion sensors can be customized for specific species and environments to modulate the spectral composition of lighting, such as by dimming, shielding, and controlling lighting during different periods. Insect phototactic rhythms are affected by various factors such as temperature, humidity, light, rainfall, moonlight, and wind (Steinbauer et al., 2012; Qi et al., 2014). Field experiments should be optimized in the future. For example, the effects of broad-spectrum LEDs on the phototaxis of insects require further study, and field experiments should be conducted in different zoogeographic regions.
We proposed a model for predicting the relative attraction of narrow-spectrum and broad-spectrum LEDs to four insect orders based on the patterns of attraction observed in experiments. Longcore et al. (2018) predicted the response of insects and exemplar insect species to light sources with different spectral distributions based on their behavior or visual characteristics and indices of the action spectrum of the spectral irradiance of artificial light without field studies. Donners et al. (2018) constructed attraction models based on spectral response curves of insect eyes and field experimental data to predict the total number of attracted flying insects rather than the number of insects from different taxonomic levels (e.g., orders). However, differences in behavioral responses may exist between populations of the same species (Briscoe and Chittka, 2001; van der Kooi et al., 2021). There might also be differences in the attraction of insects to artificial light in different habitats and insect communities (Wakefield et al., 2018). These differences indicate the need for more studies to characterize the fine spectral effects of phototaxis for different animals and insects in different geographical regions and target populations. Our study was focused on the urban–rural fringe of Tianjin, which is located in the Huang-Huai Plain Subregion, the North China region, the East Asian sub-region, and the Palearctic region of the Arctogaea Arctogacan realm. We conducted field experiments to predict the relative attraction of all insects and four insect orders to artificial light with different spectral distributions and validated the model using visual response models of insects and experimental data.
The limitation of the observed spectral attraction pattern was manifested in its limited range of applicability, which was constrained to common park lighting levels (ranging from 10.59 to 46.67 W/m², with an average of 28.6 W/m²). In addition to the spectrum, the attraction of insects to ALAN is also affected by other factors, including light intensity (Reber et al., 2015; Liao et al., 2020), duration of light exposure (Chen et al., 2016), thermal radiation (Wakefield et al., 2016), polarized light (Turcsanyi et al., 2009), flicker rate (Inger et al., 2014), the distribution of luminescent intensity (Wakefield et al., 2018), and light source height (Silva et al., 2017).
From an ecological perspective, turning off the lighting, turning on the lighting after 21:30, or utilizing red and orange lights is favorable for insects, while being unfriendly to humans. Our study was focused on the lighting of urban parks or green space areas where human activities make nighttime lighting essential. However, there is a clear conflict between nighttime artificial light and ecological conservation in these areas. The aim of our study was to determine whether ecological risks could be reduced by avoiding light sources with risk spectra and reducing the amount of lighting during risk periods while ensuring that human needs are met. Furthermore, in areas with little or no human activity, a minimum level of light should be maintained, or light should be avoided altogether. The reality is that there are no easy win-wins here. The pursuit of harmonious approaches that meet human requirements while ensuring ecological friendliness is an area where future research and development should be concentrated.
Decision-making authority for lighting planning and updates to current lighting systems is often given to relevant government construction and management departments. These institutions focus on controlling lighting to reduce the effects on residents in compliance with relevant laws and standards, which consider the needs for economic development, public aesthetics, cultural demands, functional requirements, and ecological impacts. However, few standards exist for controlling lighting to reduce ecological risks to susceptible species; most standards are focused on the direction of light and cut-off of light. In addition, few laws and regulations focus on spectral changes that are based on the results of scientific research and light source technology. The findings of this study highlight the need for collaborative research among ecologists, electrical engineers, and lighting engineers and the incorporation of spectral limits into lighting regulations to facilitate the development of LED eco-light sources and eco-friendly lighting technology (Pawson and Bader, 2014).
5 Conclusion
Our results revealed significant differences in the attraction of insect communities and orders to light sources with different spectral compositions. In general, the shorter wavelength light was more attractive. Among the narrow-spectrum LEDs, insect captures were highest in the short-wave blue light (Royal Blue, 447 nm; Blue, 478 nm) treatments; these wavelengths comprise the high ecological risk spectrum. This was followed by mid-wave green light (Cyan, 500 nm; Green, 519 nm), which comprises the moderate ecological risk spectrum, and long-wave red light (Red Orange, 627 nm; Deep Red, 660 nm; Far Red, 740 nm), which comprises the low-ecological risk spectrum (i.e., the eco-friendly spectrum). Diptera and Hemiptera showed higher attraction to Blue (478 nm) than to Royal Blue (447 nm); by contrast, Coleoptera and Lepidoptera showed higher attraction to Royal Blue (447 nm). The broad-spectrum 4,500 K LED lights attracted 1.43 times the number of insects than the 3,000 K LED lights. The number of insects captured by the 4,500 K LED was more similar to the number of insects captured in the Cyan (500 nm) and Green (519 nm) treatments compared with the 3,000 K LEDs. However, there was no significant difference in insect attraction between 3,000 K and 4,500 K LEDs. The overall pattern in the phototactic rate curves of different insect orders to different spectral LEDs was consistent, with the peak phototaxis period being 20:00–21:30, with sunset between 18:30 and 18:50. After this period, the phototactic rate decreased and remained low until the end of the experiment. The spectral distribution may regulate the phototactic rhythms of insects, alter the decay speeds of the phototactic rates and peak phototactic periods, and lead to differences between insect orders.
Additional studies are needed to precisely assess the ecological risks of light pollution. The spectral and temporal properties of ecological lighting can be optimized based on the spectral and temporal variation in the attraction of insects to artificial light to minimize the adverse effects of ALAN. We proposed a quantitative model for determining the relative attraction of insects to outdoor landscape LEDs and validated the model. Before the model can be widely used for light source evaluations and eco-light source development, a more refined comparative analysis of narrow-spectrum and broad-spectrum artificial light is needed; the effects of different light intensities and durations also merit exploration. The results of this research will aid the development of eco-light sources, ecological protection, and improvements to urban light ecology.
Data availability statement
The original contributions presented in the study are included in the article/Supplementary Material. Further inquiries can be directed to the corresponding author.
Ethics statement
We declare that the animal use protocol in our study has been reviewed and approved by the Animal Ethical and Welfare Committee (AEWC) of Tianjin University.
Author contributions
QH, GL, LW, JY, and ZY designed the experimental set up and research question. QH, ZY, XC, and PX conducted the experiments, data collection and database creation. QH, GL, LW, JY, and PX performed statistical analyses, and contributed to discussions, manuscript writing and revision, and approved the submitted version.
Funding
This work was supported by grants from the National Natural Science Foundation of China (No. 51978453) and the Science and Technology Commission of Shanghai Municipality (22DZ1202400, 22DZ1202404).
Acknowledgments
We like to express our gratitude to Mujie Qi and his team, the School of Life Sciences, Nankai University, China, for their insect expertise and specialized instruments.
Conflict of interest
The authors declare that the research was conducted in the absence of any commercial or financial relationships that could be construed as a potential conflict of interest.
Publisher’s note
All claims expressed in this article are solely those of the authors and do not necessarily represent those of their affiliated organizations, or those of the publisher, the editors and the reviewers. Any product that may be evaluated in this article, or claim that may be made by its manufacturer, is not guaranteed or endorsed by the publisher.
Supplementary material
The Supplementary Material for this article can be found online at: https://www.frontiersin.org/articles/10.3389/fevo.2023.1206404/full#supplementary-material
References
AskCI (2022) China led lighting industry 2022 market outlook and investment research forecast report (short version). Available at: https://www.askci.com/news/chanye/20220609/1637151884848_3.shtml (Accessed February 11, 2023).
Barghini A., Souza de Medeiros B. A. (2012). Uv radiation as an attractor for insects. Leukos 9, 47–56. doi: 10.1582/leukos.2012.09.01.003
Bates A. J., Sadler J. P., Grundy D., Lowe N., Davis G., Baker D., et al. (2014). Garden and landscape-scale correlates of moths of differing conservation status: Significant effects of urbanization and habitat diversity. PloS One 9, 11. doi: 10.1371/journal.pone.0086925
Bennie J., Davies T. W., Cruse D., Gaston K. J. (2016). Ecological effects of artificial light at night on wild plants. J. Ecol. 104, 611–620. doi: 10.1111/1365-2745.12551
Bennie J., Davies T. W., Cruse D., Inger R., Gaston K. J. (2018). Artificial light at night causes top-down and bottom-up trophic effects on invertebrate populations. J. Appl. Ecol. 55, 2698–2706. doi: 10.1111/1365-2664.13240
Bennie J., Duffy J. P., Davies T. W., Correa-Cano M. E., Gaston K. J. (2015). Global trends in exposure to light pollution in natural terrestrial ecosystems. Remote Sens. 7, 2715–2730. doi: 10.3390/rs70302715
Bolliger J., Hennet T., Wermelinger B., Blum S., Haller J., Obrist M. K. (2020). Low impact of two led colors on nocturnal insect abundance and bat activity in a peri-urban environment. J. Insect Conserv. 24, 625–635. doi: 10.1007/s10841-020-00235-1
Briscoe A. D., Chittka L. (2001). The evolution of color vision in insects. Annu. Rev. Entomol. 46, 471–510. doi: 10.1146/ANNUREV.ENTO.46.1.471
Cai L., Jia Y., Wen Y., Zhang X., Wang R., Li T., et al. (2016). Study on the behavioral rhythms of migratory population of rice planthoppers, nilaparvata lugens, sogatella furcifera and laodelphax striatellus, light-trapped by blacklamp. Chin. J. Appl. Entomol. 53, 604–611. (Chinese).
Cao L. N., Li W., Devakumar B., Ma N., Huang X. Y., Lee A. F. (2022). Full-spectrum white light-emitting diodes enabled by an efficient broadband green-emitting cay2zrscal3o12:Ce3+ garnet phosphor. ACS Appl. Mater. Interfaces 14, 5643–5652. doi: 10.1021/acsami.1c23286
Cao Y. M., Langdon P., Chen X., Huang C. L., Yan Y., Yang J., et al. (2020). Regime shifts in shallow lake ecosystems along an urban -rural gradient in central China. Sci. Total Environ. 733, 14. doi: 10.1016/j.scitotenv.2020.139309
Chen F., Shi J., Keena M. (2016). Evaluation of the effects of light intensity and time interval after the start of scotophase on the female flight propensity of asian gypsy moth (lepidoptera: Erebidae). Environ. Entomol. 45, 404–409. doi: 10.1093/ee/nvv222
China Meteorological Data Network (2023) Tianjin. Available at: http://data.cma.cn/dataGis/static/gridgis/#/pcindex (Accessed April 15, 2023).
Cox D. T. C., de Miguel A. S., Dzurjak S. A., Bennie J., Gaston K. J. (2020). National scale spatial variation in artificial light at night. Remote Sens. 12, 17. doi: 10.3390/rs12101591
David S. (2009). Circadian rhythms and the evolution of photoperiodic timing in insects. Physiol. Entomol. 34, 301–308. doi: 10.1111/j.1365-3032.2009.00699.x
Davies T. W., Bennie J., Inger R., De Ibarra N. H., Gaston K. J. (2013). Artificial light pollution: Are shifting spectral signatures changing the balance of species interactions? Global Change Biol. 19, 1417–1423. doi: 10.1111/gcb.12166
Degen T., Mitesser O., Perkin E. K., Weiss N. S., Oehlert M., Mattig E., et al. (2016). Street lighting: Sex-independent impacts on moth movement. J. Anim. Ecol. 85, 1352–1360. doi: 10.1111/1365-2656.12540
De Medeiros B. A. S., Barghini A., Vanin S. A. (2017). Streetlights attract a broad array of beetle species. Rev. Bras. Entomol. 61, 74–79. doi: 10.1016/j.rbe.2016.11.004
Desouhant E., Gomes E., Mondy N., Amat I. (2019). Mechanistic, ecological, and evolutionary consequences of artificial light at night for insects: Review and prospective. Entomol. Exp. Appl. 167, 37–58. doi: 10.1111/eea.12754
Donners M., van Grunsven R. H. A., Groenendijk D., van Langevelde F., Bikker J. W., Longcore T., et al. (2018). Colors of attraction: Modeling insect flight to light behavior. J. Exp. Zool. Part a-Ecological Integr. Physiol. 329, 434–440. doi: 10.1002/jez.2188
Eccard J. A., Scheffler I., Franke S., Hoffmann J. (2018). Off-grid: Solar powered led illumination impacts epigeal arthropods. Insect Conserv. Diversity 11, 600–607. doi: 10.1111/icad.12303
Eisenbeis G. (2006). “Artificial night lighting and insects: Attraction of insects to streetlamps in a rural setting in Germany,” in Ecological consequences of artificial night lighting. Ed. Rich C. (Washington DC: Island Press), 281–304.
Falchi F., Cinzano P., Duriscoe D., Kyba C. C. M., Elvidge C. D., Baugh K., et al. (2016). The new world atlas of artificial night sky brightness. Sci. Adv. 2, e1600377. doi: 10.1126/sciadv.1600377
Farnworth B., Innes J., Kelly C., Littler R., Waas J. R. (2018). Photons and foraging: Artificial light at night generates avoidance behaviour in male, but not female, New Zealand weta. Environ. pollut. 236, 82–90. doi: 10.1016/j.envpol.2018.01.039
Ferrer-Paris J. R., Lozano C., Cardozo-Urdaneta A., Thomas Cabianca A. (2016). Indicative response of oxysternon festivum linn, (coleoptera: Scarabaidae) to vegetation condition in the basin of the orinoco river, Venezuela. J. Insect Conserv. 20, 527–538. doi: 10.1007/s10841-016-9886-6
Gaston K. J., Davies T. W., Nedelec S. L., Holt L. A. (2017). Impacts of artificial light at night on biological timings. Annu. Rev. Ecol. Evol. Syst. 48, 49–68. doi: 10.1146/annurev-ecolsys-110316-022745
Gaston K. J., Visser M. E., Holker F. (2015). The biological impacts of artificial light at night: The research challenge. Philos. Trans. R. Soc. B-Biological Sci. 370, 20140133. doi: 10.1098/rstb.2014.0133
Grubisic M., van Grunsven R. H. A., Manfrin A., Monaghan M. T., Hoelker F. (2018). A transition to white led increases ecological impacts of nocturnal illumination on aquatic primary producers in a lowland agricultural drainage ditch. Environ. pollut. 240, 630–638. doi: 10.1016/j.envpol.2018.04.146
Hoelker F., Wolter C., Perkin E. K., Tockner K. (2010). Light pollution as a biodiversity threat. Trends Ecol. Evol. 25, 681–682. doi: 10.1016/j.tree.2010.09.007
Inger R., Bennie J., Davies T. W., Gaston K. J. (2014). Potential biological and ecological effects of flickering artificial light. PloS One 9, 11. doi: 10.1371/journal.pone.0098631
Jiang W., He G., Long T., Wang C., Ni Y., Ma R. (2017). Assessing light pollution in China based on nighttime light imagery. Remote Sens. 9, 18. doi: 10.3390/rs9020135
Jing X., Lei C. (2004). Progress in the study of insect phototaxis and the mechanisms. Entomol. Knowledge 3, 198–203. (Chinese).
Justice M. J., Justice T. C. (2016). Attraction of insects to incandescent, compact fluorescent, halogen, and led lamps in a light trap: Implications for light pollution and urban ecologies. Entomol. News 125, 315–326. doi: 10.3157/021.125.0502
Kalinkat G., Grubisic M., Jechow A., van Grunsven R. H. A., Schroer S., Hoelker F. (2021). Assessing long-term effects of artificial light at night on insects: What is missing and how to get there. Insect Conserv. Diversity 14, 260–270. doi: 10.1111/icad.12482
Kamei M., Jikumaru S., Hoshino S., Ishikura S., Wada M. (2021). Effects of replacing outdoor lighting with white leds with different correlated color temperatures on the attraction of nocturnal insects. Appl. Entomol. Zool. 56, 225–233. doi: 10.1007/s13355-021-00729-7
Knop E., Gerpe C., Ryser R., Hofmann F., Menz M. H. M., Trosch S., et al. (2018). Rush hours in flower visitors over a day-night cycle. Insect Conserv. Diversity 11, 267–275. doi: 10.1111/icad.12277
Kravchenko V., Pustilnik L., Nagari M., Shtivelman D., Furman G., Poltaysky A., et al. (2021). Application of time-lapse camera situated near a light source, for registration insects' rhythm of attraction to light (lepidoptera: Noctuidae). Shilap-Revista Lepidopterologia 49, 319–325. doi: 10.57065/shilap.302
Lawes M. J., Kotze D. J., Bourquin S. L., Morris C. (2005). Epigaeic invertebrates as potential ecological indicators of afromontane forest condition in South Africa. Biotropica 37, 109–118. doi: 10.1111/j.1744-7429.2005.04054.x
Liao H. J., Liu C. J., Du T., Shi L. (2020). Light intensity affects the reproductive success of danaus chrysippus (lepidoptera: Danaidae) by influencing flight behavior. J. Entomol. Sci. 55, 234–251. doi: 10.18474/0749-8004-55.2.234
Longcore T., Aldern H. L., Eggers J. F., Flores S., Franco L., Hirshfield-Yamanishi E., et al. (2015). Tuning the white light spectrum of light emitting diode lamps to reduce attraction of nocturnal arthropods. Philos. Trans. R. Soc. B-Biological Sci. 370, 20140125. doi: 10.1098/rstb.2014.0125
Longcore T., Rodriguez A., Witherington B., Penniman J. F., Herf L., Herf M. (2018). Rapid assessment of lamp spectrum to quantify ecological effects of light at night. J. Exp. Zool. Part a-Ecological Integr. Physiol. 329, 511–521. doi: 10.1002/jez.2184
Macgregor C. J., Pocock M. J. O., Fox R., Evans D. M. (2019). Effects of street lighting technologies on the success and quality of pollination in a nocturnally pollinated plant. Ecosphere 10, e02550. doi: 10.1002/ecs2.2550
Martin B., Perez H., Ferrer M. (2021). Light-emitting diodes (led): A promising street light system to reduce the attraction to light of insects. Diversity-Basel 13, 89. doi: 10.3390/d13020089
Mi N., Zhang Q., Wang H., Wu S., Lei Z. (2019). The phototaxis behavior of thrips tabaci and trapping effect of different wavelength sticky cards in the field. Scientia Agric. Sin. 52, 1721–1732. (Chinese).
Owens A. C. S., Cochard P., Durrant J., Farnworth B., Perkin E. K., Seymoure B. (2020). Light pollution is a driver of insect declines. Biol. Conserv. 241, 108259. doi: 10.1016/j.biocon.2019.108259
Owens A. C. S., Dressler C. T., Lewis S. M. (2022a). Costs and benefits of "insect friendly" artificial lights are taxon specific. Oecologia 199, 487–497. doi: 10.1007/s00442-022-05189-6
Owens A. C. S., Lewis S. M. (2018). The impact of artificial light at night on nocturnal insects: A review and synthesis. Ecol. Evol. 8, 11337–11358. doi: 10.1002/ece3.4557
Owens A. C. S., van den Broeck M., De Cock R., Lewis S. M. (2022b). Behavioral responses of bioluminescent fireflies to artificial light at night. Front. Ecol. Evol. 10. doi: 10.3389/fevo.2022.946640
Pan H., Liang G., Lu Y. (2021). Response of different insect groups to various wavelengths of light under field conditions. Insects 12, 427. doi: 10.3390/insects12050427
Park J.-H., Lee H.-S. (2017). Phototactic behavioral response of agricultural insects and stored-product insects to light-emitting diodes (leds). Appl. Biol. Chem. 60, 137–144. doi: 10.1007/s13765-017-0263-2
Pawson S. M., Bader M. K. F. (2014). Led lighting increases the ecological impact of light pollution irrespective of color temperature. Ecol. Appl. 24, 1561–1568. doi: 10.1890/14-0468.1
Peng J., Zhao S., Liu Y., Tian L. (2016). Identifying the urban-rural fringe using wavelet transform and kernel density estimation: A case study in beijing city, China. Environ. Model. Softw. 83, 286–302. doi: 10.1016/j.envsoft.2016.06.007
Pimputkar S., Speck J. S., DenBaars S. P., Nakamura S. (2009). Prospects for led lighting. Nat. Photonics 3, 179–181. doi: 10.1038/nphoton.2009.32
Plummer K. E., Hale J. D., 'Callaghan M., Sadler J. P., Siriwardena G. M. (2016). Investigating the impact of street lighting changes on garden moth communities. J. Urban Ecol. 2, 1–10. doi: 10.1093/jue/juw004
Qi H., Zhang Y., Wang J., Peng H., Zhang Z., Cheng D. (2014). Rhythm of rice planthoppers and cyrtorhinus lividipennis to the searchlight trap. Acta Phytophylacica Sin. 41, 277–284. (Chinese).
Reber T., Vahakainu A., Baird E., Weckstrom M., Warrant E., Dacke M. (2015). Effect of light intensity on flight control and temporal properties of photoreceptors in bumblebees. J. Exp. Biol. 218, 1339–1346. doi: 10.1242/jeb.113886
Shimoda M., Honda K.-I. (2013). Insect reactions to light and its applications to pest management. Appl. Entomol. Zool. 48, 413–421. doi: 10.1007/s13355-013-0219-x
Silva F. S., Lobo S., da Silva A. A. (2017). Seasonal abundance and the effect of light trap height on the captures of the vesicating beetles of the genus paederus (coleoptera: Staphylinidae) in Brazil. Entomol. News 127, 21–27. doi: 10.3157/021.127.0104
Steinbauer M. J., Haslem A., Edwards E. D. (2012). Using meteorological and lunar information to explain catch variability of orthoptera and lepidoptera from 250 w farrow light traps. Insect Conserv. Diversity 5, 367–380. doi: 10.1111/j.1752-4598.2011.00170.x
Steinbauer M. J., Weir T. A. (2007). Summer activity patterns of nocturnal scarabaeoidea (coleoptera) of the southern tablelands of new south wales. Aust. J. Entomol. 46, 7–16. doi: 10.1111/j.1440-6055.2007.00579.x
Sun Y. X., Tian A., Zhang X. B., Zhao Z. G., Zhang Z. W., Ma R. Y. (2014). Phototaxis of grapholitha molesta (lepidoptera: Olethreutidae) to different light sources. J. Econ. Entomol. 107, 1792–1799. doi: 10.1603/ec14155
Tataroglu O., Emery P. (2014). Studying circadian rhythms in drosophila melanogaster. Methods 68, 140–150. doi: 10.1016/j.ymeth.2014.01.001
Time and Date (2023) Sun & moon. Available at: https://www.timeanddate.com (Accessed April 15, 2023).
Tu H., Tang N., Hu X., Yao Z., Wang G., Wei H. (2016). Led multispectral circulation solar insecticidal lamp application in rice field. Trans. Chin. Soc. Agric. Eng. 32, 193–197. (Chinese).
Turcsanyi I., Szentkiralyi F., Bernath B., Kadar F. (2009). Flight of mayflies towards horizontally polarised and unpolarised light. Aquat. Insects 31, 301–310. doi: 10.1080/01650420902812222
van der Kooi C. J., Stavenga D. G., Arikawa K., Belusic G., Kelber A. (2021). Evolution of insect color vision: From spectral sensitivity to visual ecology. Annu. Rev. Entomol. 66, 435–461. doi: 10.1146/annurev-ento-061720-071644
van Grunsven R. H. A., Donners M., Boekee K., Tichelaar I., van Geffen K. G., Groenendijk D., et al. (2014). Spectral composition of light sources and insect phototaxis, with an evaluation of existing spectral response models. J. Insect Conserv. 18, 225–231. doi: 10.1007/s10841-014-9633-9
van Langevelde F., Braamburg-Annegarn M., Huigens M. E., Groendijk R., Poitevin O., van Deijk J. R., et al. (2018). Declines in moth populations stress the need for conserving dark nights. Global Change Biol. 24, 925–932. doi: 10.1111/gcb.14008
van Langevelde F., Ettema J. A., Donners M., WallisDeVries M. F., Groenendijk D. (2011). Effect of spectral composition of artificial light on the attraction of moths. Biol. Conserv. 144, 2274–2281. doi: 10.1016/j.biocon.2011.06.004
Wakefield A., Broyles M., Stone E. L., Harris S., Jones G. (2018). Quantifying the attractiveness of broad-spectrum street lights to aerial nocturnal insects. J. Appl. Ecol. 55, 714–722. doi: 10.1111/1365-2664.13004
Wakefield A., Broyles M., Stone E. L., Jones G., Harris S. (2016). Experimentally comparing the attractiveness of domestic lights to insects: Do leds attract fewer insects than conventional light types? Ecol. Evol. 6, 8028–8036. doi: 10.1002/ece3.2527
Welbers A., van Dis N. E., Kolvoort A. M., Ouyang J., Visser M. E., Spoelstra K., et al. (2017). Artificial light at night reduces daily energy expenditure in breeding great tits (parus major)d. Front. Ecol. Evol. 5. doi: 10.3389/fevo.2017.00055
Yang J., Liang Z., Shen B., Zhang Q., Tian C., Huang Y, et al. (2012). Study on the rhythm of insect flapping lamp. J. Anhui Agric. Sci. 40, 210–212. (Chinese).
Keywords: artificial light at night (ALAN), ecological light pollution, nocturnal insects, narrow-spectrum, broad-spectrum, attraction, phototaxis, action spectrum
Citation: Hao Q, Liu G, Wang L, Xin P, Yu J, Yu Z and Chen X (2023) Assessing the attraction of narrow-spectrum and broad-spectrum artificial light to nocturnal insects: patterns and predictive models. Front. Ecol. Evol. 11:1206404. doi: 10.3389/fevo.2023.1206404
Received: 15 April 2023; Accepted: 06 October 2023;
Published: 25 October 2023.
Edited by:
Julian Wang, The Pennsylvania State University (PSU), United StatesReviewed by:
Adam James Bates, Nottingham Trent University, United KingdomEunice Jingmei Tan, National University of Singapore, Singapore
Copyright © 2023 Hao, Liu, Wang, Xin, Yu, Yu and Chen. This is an open-access article distributed under the terms of the Creative Commons Attribution License (CC BY). The use, distribution or reproduction in other forums is permitted, provided the original author(s) and the copyright owner(s) are credited and that the original publication in this journal is cited, in accordance with accepted academic practice. No use, distribution or reproduction is permitted which does not comply with these terms.
*Correspondence: Juan Yu, anVhbi55dUB0anUuZWR1LmNu