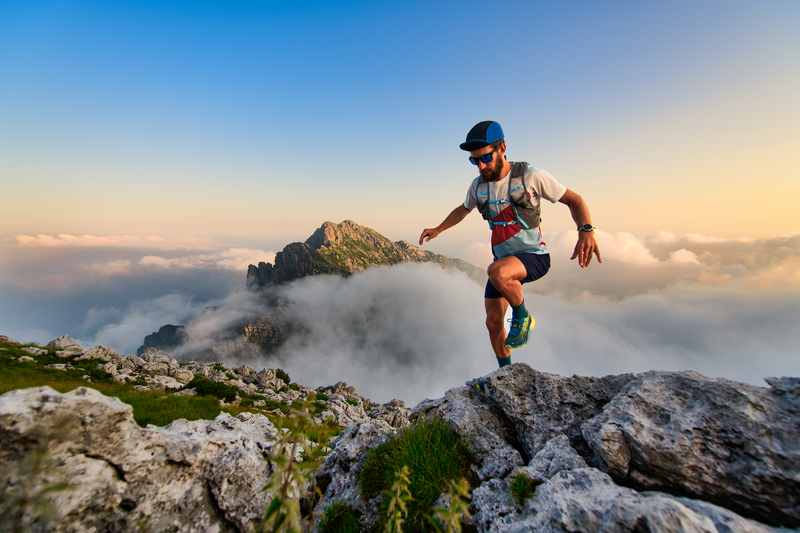
95% of researchers rate our articles as excellent or good
Learn more about the work of our research integrity team to safeguard the quality of each article we publish.
Find out more
HYPOTHESIS AND THEORY article
Front. Ecol. Evol. , 28 September 2023
Sec. Ecophysiology
Volume 11 - 2023 | https://doi.org/10.3389/fevo.2023.1199281
This article is part of the Research Topic Large Scale Coastal Processes and Their Interactions With Changing Coastal Environments View all 7 articles
Tidal wetlands are highly dynamic ecosystems that are susceptible to changes in sea level and flooding from storm surges. Among them, salt marshes play a key role in coastal protection as they contribute to wave attenuation through their regulating ecosystem services, thereby promoting sediment deposition and shoreline stabilization. However, the resilience of salt marshes, particularly those that have been modified and cultivated for centuries, is questionable in the face of accelerated sea-level rise (SLR) and increasing run-up heights of storm surges. In this context, this study aims to investigate the historical foraminiferal records of two sedimentary salt marsh archives from the Wadden Sea area (Dithmarschen and North Frisia, Germany) that have been modified to varying degrees by human management activities over the last century. The foraminiferal records document how physico-chemical traits of salt marshes of the central Wadden Sea have responded to storm tide inundation over the last century, providing information about salt marsh stability and vulnerability. Abnormally grown tests of the salt marsh indicator species Entzia macrescens increased in number between 1950 CE and the late 1980s, indicating the concurrent increase of environmental stress caused by the effects of times of increased salt marsh flooding. These trends can be linked to observations of amplified North Sea storm surges, corroborating that salt marsh ecosystems respond to changing climate conditions. Differences in the number of abnormal foraminifera between the studied salt marshes suggest a particularly high vulnerability of intensively human-modified coastal wetland ecosystems to amplified storm climate conditions.
Salt marsh ecosystems are known for their unstable conditions, characterized by large fluctuations and gradients in various environmental parameters, which could give rise to different stressors (e.g., Fagherazzi et al., 2012). Important stressors encompass shifts in temperature and salinity, as well as specific hydrodynamic conditions including storm-tide frequency, exposure to wave energy, and deposition of sediment event layers. Although salt marsh systems are generally well adapted to such an environment, extreme variations in abiotic factors may constrain the optimal or tolerance ranges of the system (Davy, 2002; Davy et al., 2011). The natural feedbacks between plant growth, sedimentation and morphodynamics, which allows a salt marsh to adapt to sea-level rise, have been altered at many coasts by human activities, such as diking, drainage, and transition to pasture land (Kirwan and Megonigal, 2013). While natural stressors already affect the salt marsh ecosystem, anthropogenic impacts further contribute to these pressures. However, our understanding of how these human-altered salt marshes influence various ecosystem components and salt marsh organisms remains incomplete.
Most of today’s mainland salt marshes in the German Wadden Sea have been affected through land reclamation measures (Meier, 2004). Of these, the most important measures include the construction of groin fields made of brushwood fences in the foreshore of the dikes, which ensures a hydrodynamically calmed settlement space in which fine sediment can be deposited. This strategy results in accelerated accretion of the terrain, and thereby promoting the colonization of the tidal flats by halophytic pioneer plants (Hofstede and Schirmacher, 1996; Gettner, 2003; Hofstede, 2003). Given the economic viability as agricultural land and for resource extraction, the reclaimed land was then traditionally used for livestock grazing as well as haymaking and harvesting marsh sods (Meier, 2004; Esselink et al., 2009; Esselink et al., 2017). However, some measures changed the morphology of the salt marshes (such as diking and drainage), others influenced the local erosion and sedimentation processes (such as groins or dredging channels for navigation purposes) or even changed the ecological and biological conditions of the marshes (such as disrupting natural tidal patterns, water movement, and nutrient cycling; Suchrow et al., 2012; Kirwan et al., 2016a; Siemes et al., 2020; Gläsner, 2023). Although intensive foreshore management measures in Schleswig-Holstein (Germany) have been reduced since the establishment of the Wadden Sea National Park in 1985 CE and are nowadays mainly restricted to the areas near the dike base, the formerly cultivated salt marshes still show a rather poor conservation status according to the EU Habitats Directive (LLUR, State Office for Agriculture, Environment and Rural Areas, 2020). Yet the regularly flooded salt marshes provide various ecosystem services and therefore urgently need to be protected, conserved, and ecologically restored (Bromberg Gedan et al., 2009; Barbier et al., 2011). In principle, massive dikes that border the shoreline are the most common coastal protection structures in northern Germany (Kelletat, 1992). On the seaward side of the dikes, however, salt marshes provide regulating services by serving as a natural buffer zone against heavy swells and storm surges from the North Sea and by attenuating incoming wave energy and current-induced shear stress (Feagin et al., 2009; Kirwan and Megonigal, 2013; Möller et al., 2014). Thus, salt marshes likewise contribute to coastal protection and play an essential role for future-proof and sustainable coastal protection concepts as so-called ‘Nature-based Solution’ (NbS) approach (Narayan et al., 2016; Schoonees et al., 2019). This principle involves using green and soft infrastructure, such as salt marshes, to supplement or replace conventional coastal protection structures, thereby simultaneously enhancing the ecological value of the coast while preserving its protective function (Pontee et al., 2016). Another important regulating ecosystem service is the incorporation of organic carbon-rich suspended matter during flooding of the marshes at high and storm tides, making salt marshes one of the most important ‘Blue Carbon’ players (Kathilankal et al., 2008; Mcleod et al., 2011; Kirwan and Mudd, 2012; Müller et al., 2018).
The resilience of salt marshes depends largely on their ability to grow vertically by incorporating suspended sediments introduced by recurrent flooding around the mean high water level (MHW; Van Proosdij et al., 2006), ensuring sediment accretion rates higher than sea-level rise (SLR), and on their potential to migrate laterally further inland as sea level rises (Allen, 1990; Andersen et al., 2011; Kirwan and Megonigal, 2013; Kirwan et al., 2016b). In fact, an important response, and thus the underlying natural long-term stability of salt marshes, through dynamic adaptation to changing hydrodynamic conditions, is their migration to adjacent uplands (salt marsh transgression; Miller et al., 2021; Walters et al., 2021). A slowed SLR combined with sufficient sediment supply is the key driver of salt marsh formation, both by creating accommodation and by filling the newly formed space through sediment accretion (Watson et al., 2014; Fagherazzi et al., 2020). Nevertheless, coastal salt marshes are in urgent need of conservation and protection (Esselink et al., 2009), as ecosystems are expected to be more frequently and intensively exposed to the effects of extreme high sea levels (ESLs) under the influence of global warming by the year 2100 CE (Arns et al., 2015; Arns et al., 2017; IPCC, 2019; Lang and Mikolajewicz, 2020; IPCC, 2022). Although salt marshes are, in principle and to some degree, capable of creating new equilibria between their vertical growth and water level fluctuations through self-reinforcing interactions between plant communities and morphodynamics (Fagherazzi et al., 2020; Van de Ven et al., 2023), there is an upper limit to optimal SLR rates for salt marsh growth (Morris et al., 2002). If SLR rates are too high, the vegetation will no longer be able to achieve sufficient elevation gain to be still in equilibrium with sea level; a limitation that is further exacerbated when regional sediment availability is low (Morris et al., 2002; Bartholdy et al., 2010; Fagherazzi et al., 2013; Fagherazzi et al., 2020). Furthermore, the rate of transgression of salt marshes also depends on local morphology, aboveground vegetation, and engineered barriers in the hinterland (Kirwan et al., 2016a; Fagherazzi et al., 2020).
In this context, the question remains as to how and to what extent anthropogenically modified coasts and their cultivated ecosystems can withstand more frequent and more intense occurring ESLs and the associated increased run-up heights of storm surges. This question arises given that in the human-altered salt marshes on the Wadden Sea coast, natural features such as a varying morphology and diverse plant communities are no longer present, but instead the salt marshes are characterized by flattened and drained areas with altered soil chemical properties and mature successional stages of mono-dominant plant species (e.g., Van Wijnen et al., 1997; Morris et al., 2002; Bromberg Gedan et al., 2009; Davy et al., 2009). According to the Flora-Fauna-Habitat Directive (or Habitats Directive), such cultivated salt marshes show considerable deficits with regard to their conservation status, and it is assumed that this in turn may degrade the ecosystem services of the salt marshes in the long term (Wessels, 2021). Furthermore, the artificially straightened marsh areas that lie well above the MHW level hardly experience any tide-induced sedimentation and are thus almost detached from the marine environment – a circumstance whose long-term effects are not yet fully understood (Broome et al., 1988; Doody, 2008; Bromberg Gedan et al., 2009; Bunzel et al., 2021). Ultimately, the massive dikes prevent the spatio-temporal dynamics of the salt marshes accompanying SLRs (Kirwan et al., 2016a). Accordingly, today’s cultivated salt marshes along the German Wadden Sea coast are suspected of being particularly vulnerable under rising sea levels, as coastal narrowing and habitat loss can occur if the salt marshes are prevented from migrating upland by anthropogenic structures (Van der Wal and Pye, 2004; Pontee, 2011), and at the same time experience hardly any marine sediment input (Bunzel et al., 2021). Human interventions have thus significantly affected the natural physico-chemical gradients as well as the plant communities of today’s Wadden Sea salt marshes, and the cultivation of salt marshes will also have a considerable impact on their future development and survival.
To better assess the evolution of salt marshes under climatic and anthropogenic influences, it is crucial to reconstruct salt marsh evolution over the past 20th century, when climatic and non-climatic factors increasingly influence coastal ecosystems (He and Silliman, 2019). Tidal flats and salt marshes are colonized by a variety of benthic foraminiferal species. Benthic foraminifera are unicellular organisms that can account for up to 50% of eukaryotic biomass in coastal areas (Moodley et al., 2000). Along the tidal frame, foraminiferal taxa exhibit distinct vertical zonation, and similar to salt marsh plants, they closely follow physico-chemical gradients along salt marsh successional stages (such as salinity, substrate, and pH that are linked to inundation and elevation of the terrain; Scott and Medioli, 1986; Scott and Leckie, 1990; Müller-Navarra et al., 2016). In the differentiation and taxonomic identification of the foraminiferal species, the test material and wall structure are of particular relevance. The test materials can be i) non-mineralized and organic, ii) agglutinated (i.e., they collect sediment particles from the surrounding habitat), or iii) consisting of calcium carbonate (porcellaneous or hyaline; Marszalek et al., 1969; Goldstein, 1999). These tests have a high potential to be preserved in the sediment for a long time, making foraminifera good bioindicators (e.g., Marszalek et al., 1969; Francescangeli et al., 2016). In the intertidal zone, the calcareous foraminifera occur preferentially in the tidal flats, which are very frequently to permanently flooded, while in the adjacent vegetated salt marshes, which are rarely to not flooded at all, primarily agglutinated foraminifera live (Horton et al., 1999a). In this regard, dead or fossilized tests of benthic foraminifera deposited at the coastal environments and integrated into the sedimentary archives provide information that can be used to reconstruct past ecosystems (Alve et al., 2009).
Although the use of foraminifera to reconstruct environmental conditions in different salt marsh areas has proven successful, comparatively little information is available from the Wadden Sea on the distribution of recent and fossil salt marsh foraminifera. This may be due to the fact that the cultivated salt marshes along the German Wadden Sea coast lack a pronounced landward rise in surface elevation, resulting not only in the absence of distinct vertical salt marsh zonation but also in the absence of foraminiferal zonation with respect to the tidal frame (Müller-Navarra et al., 2016), complicating the use of foraminifera as a proxy for reconstructing past salt marsh development and environmental change. Therefore, the question arises how past environmental changes and the associated responses of a coastal ecosystem can be reconstructed and assessed in this region using intertidal benthic foraminifera. Commonly, a general differentiation between tidal influences and marsh development can be reconstructed based on the relative abundance of calcareous tidal flat and agglutinated salt marsh foraminifera. However, as foraminifera are considered to be one of the microorganism groups most sensitive to coastal environmental degradation (Capotondi et al., 2015), intertidal foraminifera may also be suitable for assessing environmental and anthropogenic stressors on (cultivated) Wadden Sea salt marshes during the 20th century. Hence, further information on changes in the ecosystem state can be derived by abnormalities in the test morphology of benthic foraminifera, as foraminifera can encounter unfavorable environmental conditions by modifying their test shapes (e.g., Geslin et al., 2000; Geslin et al., 2002; Polovodova and Schönfeld, 2008; Brunner et al., 2013; Martínez et al., 2023).
In this study, the historical benthic foraminiferal records of two sedimentary salt marsh archives from Schleswig-Holstein, central Wadden Sea, have been evaluated. The selected study sites have been heavily modified over the last century by human activities such as foreshore management measures and coastal protection structures. Today, the two sites differ such that since the establishment of the Wadden Sea National Park, many management activities have largely ceased at one site, while the other site continues to be managed. Since both sites have undergone different histories of intensive land use and degrees of modification (e.g., drainage frequency, grazing intensity, times of dike construction), whereas the influence of long-term climate change and associated storm surge dynamics along the central Wadden Sea coast should have exerted similar environmental stress at both sites, this study is twofold: i) to test whether intertidal benthic foraminifera (calcareous and agglutinated indicator species) can be used to reconstruct and to assess the evolution of local coastal ecosystems in the Wadden Sea area over the past century, and ii) to investigate how cultivated salt marshes in the Wadden Sea have responded to different non-climatic (foreshore management and coastal protection measures) but similar natural external influences (such as hydrodynamics), potentially indicated by test modifications of representative foraminifera in response to stress.
In Schleswig-Holstein, Germany, most of the mainland salt marshes have been anthropogenically modified to some degree (Figure 1A). This includes the typical use of groins made out of brushwood fences to promote foreshore development. The fences are installed on the tidal flats in front of the salt marshes, where they form a hydrodynamically calmed settlement space in which fine sediments can be deposited (Hofstede, 2003; Doody, 2008). At the same time, it is a common practice to construct drainage ditches on salt marshes, which improves soil aeration and reduces soil waterlogging, thus favoring the occurrence of species that are well adapted to dry to moderately moist and well-drained soils, such as couch grass (Elymus spp.; Bockelmann and Neuhaus, 2002). In this way, a mature vegetation stage of the salt marsh succession is achieved at an accelerated rate (Schröder et al., 2002; Rupprecht et al., 2015). The drainage ditches also reduce tidal currents so that fine sediments are deposited within the artificial channels. For maintenance, it is necessary to dredge the material accumulated in the ditches, subsequently placing the material on the surrounding salt marsh areas (Hofstede, 2003; Stock and Maier, 2016). In this way, drained groin fields in the foreshore enable a spatial expansion of the land–sea transition, and equally lead to improved accretion of the terrain and acceleration of salt marsh maturing (Erchinger, 1971; Von Lieberman et al., 1998). The areas are usually grazed, with mainly sheep being used for this purpose (pers. comm. LKN.SH, 2022). Since 1985 CE, however, German intertidal systems are under national nature protection and belong to the Wadden Sea National Park (Stock, 2003; Esselink et al., 2009). With the aim of ecologically restoring the salt marsh areas, the maintenance of artificial drainage ditches has been abandoned in many areas and grazing reduced or stopped. Since the original goal of land reclamation has been given up, no new groins are being built on the Schleswig-Holstein mainland coast today and the maintenance of existing groins has been gradually reduced since the 1980s (Esselink et al., 2009).
Figure 1 Southeastern North Sea coastline with the investigated sites in Schleswig-Holstein. Location map of the observational wind and tide gauge stations (A), as well as the setting of the evaluated salt marshes at the Bay of Tümlau (TB13-1; B) and Friedrichskoog (GeoHH-FK; C). For cross-section profiles (red lines, b and c), see Figure 2. Arrows indicate the counter-clockwise mean tidal current vectors (dimensionless; Callies et al., 2017).
However, the investigated coastal salt marsh north of the embanked Friedrichskoog polder (Figure 1C), located in Dithmarschen of the federal state Schleswig-Holstein, is still actively managed, leaving the area streaked by drainage ditches and intensively grazed by sheep (Bunzel et al., 2020). Thus, the ~6 km2 territory in the northern part of the polder is characterized by a short-grazed, monotonous vegetation consisting of a few grass species (i.e., Festuca rubra and Puccinellia maritima) and a flat but elevated surface morphology lacking the typical and naturally pronounced landward surface increase (Stock et al., 2005; Bunzel et al., 2020). As the salt marsh is bordered by a dike in the south and due to its unsheltered exposition to wave action, the seaward edge of the marsh is further characterized by a prominent erosional cliff (Figure 2, bottom). Due to the proximity to the Elbe estuary, the coastal waters near Friedrichskoog also contain high amounts of suspended sediment, causing a rapid infilling of the local artificial drainage ditches in the salt marshes during high tides. Consequently, the network of parallel ditches is dredged regularly every two to three years at Friedrichskoog (pers. comm. LKN.SH, 2020). The average tide levels recorded at the nearby tide gauge (Friedrichskoog Hafen, no. 110021) for the observation period 1986–2018 CE, provided by the Federal Maritime and Hydrographic Agency of Germany (BSH), are as follows: highest astronomical tide (HAT): 2.1 m above NHN (‘Normalhöhennull’ datum level); mean high water spring (MHWS): 1.7 m NHN; mean high water (MHW): 1.6 m NHN; mean low water (MLW): −0.4 m NHN; and mean low water spring (MLWS): −0.3 m NHN. Since the surface of the salt marsh at Friedrichskoog is nowadays 2.8 m NHN and thus well above the level of MHWS (Figure 2, bottom), the marsh is only flooded during storm tides when the water level is exceeding MHW by 1.5 m and severe storms push the water ashore (Gerber et al., 2016).
Figure 2 Salt marsh cross-section profiles. Elevation of the salt marsh platforms relative to the tidal frame in the Bay of Tümlau (TB13-1; top – b in Figure 1) and Friedrichskoog (GeoHH-FK; bottom – c in Figure 1), and the erosional cliffs at which the sediment sequences were taken. The lateral extent of the salt marshes is given here as the distance between the seaward side of the dike base and the seaward salt marsh edge. The salt marsh heights as well as the tidal data (HAT = highest astronomical tide, MHWS = mean high water spring, MHW = mean high water) are given in relation to the German reference level ‘Normalhöhennull’ (NHN). Salt marsh elevation data were acquired with the Leica Geosystems GNSS field controller (Viva Uno CS10) equipment (in the Bay of Tümlau in 2013) and with LiDAR sensing (in Friedrichskoog from 2005 to 2007; non-vegetation corrected). Elevation data were provided by Müller-Navarra et al. (2016) and the Schleswig-Holstein Agency for Coastal Defence, National Park and Marine Conservation (LKN.SH), tidal data were provided by the Federal Maritime and Hydrographic Agency of Germany (BSH).
To assess the extent to which foraminiferal data reflect local environmental factors and site-specific conditions caused by different land use histories, or are more likely to reflect super-regional factors such as storm climate variability, a second salt marsh site was considered for comparison (Bay of Tümlau), with the data collected there being based on the studies by Müller-Navarra et al. (2019) and made available by the authors for this work. The Bay of Tümlau is located on the west coast of the Eiderstedt peninsula in North Frisia, Schleswig-Holstein (Figure 1B). The salt marshes in the Bay of Tümlau were also intensively managed in the last century, and the drainage ditches were maintained (dredged) every three to seven years (pers. comm. LKN.SH, 2017). Since the founding of the National Park, however, these foreshore management measures have been largely limited to the area near the dike, leaving the salt marshes largely untouched (Hofstede and Schirmacher, 1996). As a result, the artificial drainage ditches have filled themselves in over time or have been actively filled in and are now only relics, as are the brushwood fences on the tidal flats in front of the salt marshes (Esselink et al., 2017). Compared to the salt marsh site at Friedrichskoog, there is also a return of more diverse plant communities (i.e., the Atriplex portulacoides/Artemisia-type, Atriplex portulacoides/Puccinella-type and Festuca rubra) as well as a few naturally meandering tidal creeks in the Bay of Tümlau (Stock et al., 2005). Erosion also takes place in the Bay of Tümlau on the seaward side of the salt marsh, as can be seen from an erosional cliff, which is, however, far less pronounced and steep compared to Friedrichskoog (Figure 2, top). The average tide levels recorded at the nearby tide gauge (Tümlauer Hafen, no. 110016) for the observation period 2001–2013 CE, provided by the BSH, are as follows: HAT: 2.0 m NHN; MHWS: 1.6 m NHN; MHW: 1. 4 m NHN; MLW: −1.7 m NHN; MLWS: no data available. The surface of the salt marsh in the Bay of Tümlau is located 2.1 m NHN, which is also well above the MHWS level, meaning that flooding of the salt marsh can only occur during storm tides (Figure 2, top). For more details of the regional setting, see Müller-Navarra et al. (2016, 2019).
This study builds on the investigation of the 1.16 m long sediment-filled and U-shaped plastic profile (‘U-channel’) named GeoHH-FK that was retrieved from the erosional cliff face at the seaward side of the salt marsh at Friedrichskoog in November 2016 CE (54°2’35.02’’N, 8°52’20.41’’E; Figure 1C). For further details see Bunzel et al. (2020). The 1.06 m long sediment-filled U-channel from the Bay of Tümlau (TB13-1) was retrieved from the local erosion cliff by Müller-Navarra et al. (2019) in August 2013 CE (54°21’51.07’’N, 8°40’35.09’’E; Figure 1B). The terrain heights of the salt marshes and position of the sampled erosional cliffs were surveyed with the Leica Geosystems GNSS field controller (Viva Uno CS10) equipment. The surveyed data were referred to the nearby triangulation stations (no. 191908800, Friedrichskoog; no. 161803110, Bay of Tümlau), and post-processing was realized with the Leica Geo Office 8.3 software package. The resulting vertical uncertainty is less than 1 cm. Details for the triangulation station were provided by the State Office for Surveying and Geoinformation Schleswig-Holstein (LVermGeo SH).
The obtained sediment sequence GeoHH-FK was sampled at 0.5 cm spacing, in which every second sample was considered for foraminiferal analyses, giving 116 samples in total with a sediment volume of ~1.75 cm3 each. Sediment sequence TB13-1 was sampled in 1 cm steps and performed by Müller-Navarra et al. (2019), resulting in a sediment volume of ~2.56 cm3 per sample. After sampling took place, all samples were preserved in 96% ethanol to avoid degradation of the organic components and buffered with a carbonate solution to prevent dissolution of the calcareous components. Counting of foraminiferal individuals was carried out on subsamples (splits) from the wet-sieved 63–500 µm size fraction. The splits were obtained by means of a wet-splitter (Scott and Hermelin, 1993), into which each sample was placed for at least one hour to allow the sediment to fully and evenly settle down, producing eight equal splits per sample. The number of splits that need to be counted for their foraminiferal content depends on the minimum number of total individuals (Schönfeld et al., 2012). As foraminiferal species richness is rather low in salt marshes (Murray, 2006), foraminiferal analyses within this study are based on a target value of at least 100 individuals per split, since a number of 100 counted foraminiferal individuals is sufficient to provide statistical reliability, which is at least valid for those species making up >5% of the total fauna (Fatela and Taborda, 2002; Kemp et al., 2020). Taxonomical identification of the foraminifera from the GeoHH-FK samples was mainly based on Feyling-Hanssen (1972); Wright et al. (2011); Milker et al. (2015); Müller-Navarra et al. (2016), and Schönfeld (2018). As the analyzed sediments are rich in different genera whose individuals were often poorly preserved and seemed to be reworked, identification up to species level has often been impossible. Hence, corresponding individuals were grouped by genus, from which most genera making up less than 1% of the total fauna, while the total sum of this group accounted for 3% on average. This concerns, among others, genera such as Bolivinita, Bulimina, Cibicides, and Cibicidoides.
Based on the observations of Müller-Navarra et al. (2017), who identified abnormally grown tests of Entzia macrescens in active salt marshes on the island of Sylt, regular and irregular tests of E. macrescens were also distinguished from each other in this study and counted separately. The irregularly grown individuals were referred to as E. macrescensirregular. To avoid the quantitative integration of potential intra-specific morphological variations, following Alve (1991), only severe test irregularities were counted for this study (Figures 3A–C). High-resolution images of E. macrescensirregular originating from Friedrichskoog were generated by using a Keyence VHX-6000 microscope (Figures 3A–C). The preparation of the sediment samples from the Bay of Tümlau and the identification of the local foraminifera species were carried out by Müller-Navarra et al. (2019), who then also provided the foraminiferal counting data for this study and for the analyses that follow herein. Due to the different average quantities of individuals within a group, i.e., E. macrescensirregular versus E. macrescens, as well as their trends over the entire period under consideration, the ratio between abnormal and regular E. macrescens tests was also calculated. Since the distribution of foraminifera is closely dependent on various abiotic factors (such as substrate, organic matter, salinity, oxygen or pH), which in turn are significantly controlled and constrained by tides and currents in the intertidal region (Camacho et al., 2015; Francescangeli et al., 2017), the relationship between the biota (foraminifera) and abiotic factors (coastal flooding) was statistically evaluated. In more detail, cross-correlations between absolute abundances of E. macrescensirregular and storm tide frequency in the German Bight were calculated for both sites (Friedrichskoog and Bay of Tümlau) to compare the temporal variability and potential lags and leads between the occurrence of abundant abnormal tests and years with more frequent storm tide events. Cross-correlation analyses (with t-tests) were performed by using the PAleontological STatistics (PAST) software version 4.02 (Hammer et al., 2001) and on the previously resampled records (Δt = 1 yr) by applying a simple linear interpolation processed with the AnalySeries software 2.0, version 05/2005 (Paillard et al., 1996). Required age model compilations for the sediment successions (GeoHH-FK and TB13-1) build on the establishment of an integrated stratigraphic framework for active foreshore salt marshes, in which age markers were mainly obtained by radionuclides (137Cs) and the human-induced mercury (Hg) pollution history (for further details see Bunzel et al., 2020).
Figure 3 Regular and irregular tests of Entzia macrescens (Brady, 1870). Light microscope images of E. macrescensirregular (spiral view) showing different degrees of morphological abnormalities. The individuals were derived from 24 cm (A), 25 cm (B), and 44 cm (C) sediment depth of the sediment sequence GeoHH-FK at Friedrichskoog. Also shown are regularly grown individuals, derived from surface samples at the Bay of Tümlau, showing the spiral side (D, E) and umbilical side (F) of E. macrescens. All scale bars = 100 µm.
Since the North Sea coast is basically exposed to several storm surges per year (Gerber et al., 2016), but the flood risk itself additionally depends on the prevailing wind direction and strength during a storm surge, both parameters were considered in this study. In addition, it is pointed out in literature that when assessing the impact of storm surges, strong wind conditions should last for at least six hours to account for both the different timing of MHW along the North Sea coast (Gerber et al., 2016) and the tidal phase, as there is also a positive relationship between rising tides and storm surges (Horsburgh and Wilson, 2007). In order to define meteorologically forced changes in sea level (storm surge events) that should have flooded the coasts with high probability, the German Meteorological Service (DWD) supplied historical wind data (for the stations at Husum, Büsum, and Cuxhaven) and the BSH provided seawater level observations (for the tide gauge at Cuxhaven; Figure 1A). All datasets were filtered to only consider severe wind conditions (hereafter referred to as storminess) and high seawater levels (hereafter referred to as storm tides) in this study. These include the daily mean values of the westerly winds (≥180° to 360° azimuth), which have wind speeds of ≥7 Beaufort (Bft), and the annual number of extreme sea level highs where the observed seawater level (daily peak values) exceeds the MHW by ≥1.5 m (Gerber et al., 2016). A correction with respect to tidal predictions, i.e., the subtraction of the full tidal cycles from the observed seawater level (Pugh, 1987), was not carried out for the Cuxhaven tide gauge record, because the tide surge interaction is rather complex and the generation of surges is associated with rising tides (Horsburgh and Wilson, 2007). Accordingly, the high seawater levels shown and discussed in here are the result of both storm surges and tides, i.e., storm tides.
Within the sediment sequence GeoHH-FK from Friedrichskoog, a total of 48 foraminiferal taxa were identified (27 taxa at genus level and another 21 taxa at species level). Of these, the majority belong to the recent calcareous benthic tidal flat foraminifera (on average ~71%), mainly consisting of Ammonia spp., Cribroelphidium selseyense, and Haynesina germanica. It was observed that the highest number of calcareous foraminifera occurred in the lower part of the sedimentary sequence (below 86 cm depth and chronologically before 1940 CE), with absolute abundance here reaching up to 3,255 specimens cm−3 (Figure 4A). The total amount of agglutinated foraminifera averaged 20% of the total fauna, with a maximum density of 795 specimens cm−3. Among the group of agglutinated foraminifera, E. macrescens is the most dominant species (on average ~13%), reaching a maximum density within the sediment of about 615 specimens cm−3 (Figure 4B). In contrast to the group of calcareous foraminifera, the agglutinated foraminifera represented by E. macrescens are somewhat more evenly distributed across the sediment sequence GeoHH-FK in terms of their absolute abundance, but slightly more E. macrescens individuals occurred in the central part of the sequence and after 1930 CE (Figure 4B). The separately counted foraminifera, i.e., the abnormally grown tests of E. macrescens, reached values of up to 250 specimens cm−3 at Friedrichskoog, which accounts for >30% of the total fauna in the respective sediment samples. The density of abnormally grown tests successively increased from about 78 cm depth in the sediment sequence (which chronologically corresponds to about the 1950s) until maximum values are reached at 43 cm depth (about the mid-1980s). Subsequently, the values drop back to the previous low level (Figure 5B).
Figure 4 Benthic foraminiferal abundances. (A) Absolute abundance (numbers per cm3) of calcareous benthic foraminifera (CBF), mainly comprising common tidal flat taxa such as Ammonia spp., C. selseyense, and H. germanica (sketches not to scale), displaying a general decrease after the embankment of the polder ‘Tümlauer-Koog’ at the Bay of Tümlau in 1935 CE and construction of the dam ‘Trischendamm’ at Friedrichskoog between 1934 and 1936 CE. (B) Salt marsh evolution at Friedrichskoog (GeoHH-FK, dark green) and the Bay of Tümlau (TB13-1, light green) during the past century, represented by the abundance of the salt marsh indicator species E. macrescens. Data from the Bay of Tümlau were taken from Müller-Navarra et al. (2019).
Figure 5 Time series of storm-climate observations with response of foraminifera. (A) Number of storm tide events observed per year (dark grey) when the seawater level at the Cuxhaven tide gauge exceeds the mean high water (MHW) by ≥1.5 m, and number of storm days per year (referred to as storminess) observed at Husum, Büsum, and Cuxhaven (light grey circles) when westerly winds exceed ≥7 Bft (corresponding to ≥14 m s−1). (B) Abundances (numbers cm−3) of abnormally grown tests of E. macrescens in the intense human-modified salt marsh at Friedrichskoog (GeoHH-FK, dark green) and in the comparatively less modified salt marsh at the Bay of Tümlau (TB13-1, light green); note the different y-axes scaling. The foraminiferal sketch is not to scale. (C) Ratio of abnormal to regularly grown E. macrescens individuals. The yellow shaded area indicates enhanced storm climate activity in the North Sea, with the dark yellow area marking the maximum storm tide activity during the 1990s. Data from the Bay of Tümlau were taken from Müller-Navarra et al. (2019).
For the sediment sequence TB13-1 from the Bay of Tümlau, Müller-Navarra et al. (2019) identified 24 foraminiferal taxa in total (12 taxa at genus level and another 12 taxa at species level). The proportion of calcareous (on average ~49%) and agglutinated (on average ~48%) foraminifera of the total fauna is almost equal here. According to the authors, the most common calcareous species are Ammonia batava, Cribroelphidium selseyense, Cribroelphidium williamsoni, and H. germanica, while the most abundant agglutinated species are B. pseudomacrescens, E. macrescens, and Trochammina inflata. Similar to the sequence of GeoHH-FK, it was observed for TB13-1 that the highest number of calcareous foraminifera occurred in the lower part of the sedimentary sequence, below about 90 cm depth (also corresponding approximately to the time before 1940 CE). The calcareous foraminiferal density in TB13-1 is with maximum 20,000 specimens cm−3 considerably higher than in GeoHH-FK (Figure 4A). With about 700 specimens cm−3, average abundances of agglutinated foraminifera within sediment sequence TB13-1 are similar to those of GeoHH-FK. The same applies to E. macrescens that reached up to 685 specimens cm−3 (on average ~37%). However, the absolute abundance trends of E. macrescens over time are not consistent with GeoHH-FK. For example, TB13-1 shows slightly higher densities from about 67 cm depth bottom-up (starting from the second half of the last century; Figure 4B). Further details on the foraminifera faunal composition found in the Bay of Tümlau can be obtained from Müller-Navarra et al. (2019). Among the abnormally grown E. macrescens, up to 30 specimens cm−3 could be identified at the Bay of Tümlau, which accounts for >9% of the total fauna in the respective sediment samples. Thus, the relative abundance of E. macrescensirregular in sequence TB13-1 is much lower than in GeoHH-FK. Nevertheless, the trend over time is very similar to that of GeoHH-FK, so that the density of individuals increases gradually, i.e., from the mid-1960s to the 1990s (Figure 5B), corresponding to a depth range of 67 to 26 cm, reaching a maximum in the late 1980s and followed by relatively low values until today. However, E. macrescensirregular appeared somewhat later in the Bay of Tümlau (in the mid-1960s), whereas at Friedrichskoog an increase of E. macrescensirregular was already recorded from the first half of the 20th century.
The results of the statistical cross-correlation analyses between E. macrescensirregular (specimens density at both study sites) and tide gauge records of storm tides (number of events per year) showed that the best correlations (Bay of Tümlau: p-value = 0.005; Friedrichskoog: p-value = 0.001) were obtained when the highest numbers of abnormally grown foraminifera occurred three to five years after a period of frequent storm tides (Bay of Tümlau: lag = −3; Friedrichskoog: lag = −5).
Benthic salt marsh foraminifera have a very short life cycle, often reproducing once a year between May and June (Lehmann, 2000; Schönfeld et al., 2012). Foraminifera are thus particularly sensitive and usually respond very quickly to certain environmental factors (such as seawater chemistry, temperature, substrate or water motion) and their spatio-temporal changes (Scott and Leckie, 1990; Boltovskoy et al., 1991; Hayward et al., 2014). The distribution of intertidal benthic foraminifera, inhabiting various tidal flat and salt marsh habitats in large numbers, are increasingly used as proxy to track past (paleo-)environmental changes (based on dead or fossil faunas) or to perform recent biomonitoring (based on living faunas) in the coastal zone (e.g., Alve, 1995; De Rijk and Troelstra, 1997; Geslin et al., 2000; Schönfeld et al., 2012; Francescangeli et al., 2016). While the vegetated and less tidally influenced salt marshes are typically inhabited by agglutinated taxa such as E. macrescens, Miliammina fusca, and T. inflata, the muddy to sandy tidal flats, ponds, and drainage ditches are dominated by taxa that have a calcareous test, such as Ammonia spp., Cribroelphidium spp., and Haynesina spp. (Francescangeli et al., 2017; Müller-Navarra et al., 2017). In natural coastal interfaces, the occurrence of modern benthic foraminifera thus reflects their different species-specific tolerance ranges to gradients in salinity, pH or substrate, which are mainly controlled by tidal inundation (or hydrodynamics) and elevation of the salt marsh platform (or morphodynamics; Gehrels and Newman, 2004; Horton and Edwards, 2006; Kemp et al., 2009). While the calcareous species can thus provide information about marine conditions (such as tidal flat deposition or sediment relocation through storm surges) or sediment redistribution through dredged drainage ditches (Müller-Navarra et al., 2019), the occurrence of agglutinated species on the salt marsh surface follows a similar vertical zonation relative to the tidal frame as it is known from the halophytic salt marsh plants (Horton et al., 1999b). Hence, observations of changes in species composition within sediment sequences allow for the assessment of modern in situ conditions in salt marshes, but also the reconstruction of past depositional and environmental changes (Horton et al., 1999b; Horton and Edwards, 2006; Scheder et al., 2019).
Among the agglutinated species, E. macrescens (previously named Jadammina macrescens) is of particular importance, as this species colonizes the salt marshes along the southeastern North Sea coast in large numbers (Müller-Navarra et al., 2016; Armynot du Châtelet et al., 2018; Francescangeli et al., 2018; Francescangeli et al., 2021). Entzia macrescens is described as a species that has a trochospiral chambers arrangement, but is almost planispiral in the adult stage (Figures 3D–F; Kaminski et al., 2020). The spiral test design keeps the distance between the aperture, foramen, and proloculus as close as possible, which allows for optimized signal transmission to the nucleus (Hottinger, 2000). During foraminiferal morphogenesis, the test construction follows the morphospace concept, meaning that the test form is primarily controlled by an increase in volume till a certain factor and by rotation around a certain vector (and shifting the origin of the rotation vector; Tyszka et al., 2005; Tyszka, 2006). The finely agglutinated and cosmopolitan taxon lives epifaunal to infaunal and is adapted to survive in extreme marine marginal environments (Milker et al., 2015; Kaminski et al., 2020). Entzia macrescens is usually appearing in sediments of the upper intertidal marsh zone between MHW and HAT (Haslett et al., 1997; Horton, 1999; Lal et al., 2020), which are rich in organic carbon (Armynot du Châtelet et al., 2009; Francescangeli et al., 2017). In fact, E. macrescens seems to be able to withstand long periods of subaerial exposure (on average ~90% of the day; Francescangeli et al., 2017). Furthermore, E. macrescens exhibits an epiphytal life cycle related to salt marsh plants of the Carex acuta type (Alve and Murray, 1999).
Although E. macrescens specimens occur in similar average absolute quantities at Friedrichskoog and the Bay of Tümlau, the temporal trends exhibit specific differences (Figure 4B). The latter may be due to the different salt marsh development histories at the sites (see Section 5.2). At both sites, however, there is also a conspicuous appearance in the number of irregularly grown tests of E. macrescens specimens (here referred to as E. macrescensirregular), revealing striking abnormal chambers together with abnormal twisted tests (Figures 3A–C, 5B). As foraminifera usually react very quickly to any kind of environmental changes, E. macrescens seems to have responded directly to environmental disturbances at both sites with an increasing number of abnormally grown tests (see Section 5.3).
The composition of foraminiferal assemblages is primarily determined by abiotic environmental factors (Sen Gupta, 1999). Similarly, abiotic stressors likely also determine the occurrence of morphological abnormalities with enhanced stress leading to increased numbers of abnormally grown foraminifera (see also Nigam et al., 2008). In fact, morphological abnormalities of another high marsh species (Trochamminita irregularis) are known to occur in naturally formed estuarine salt marshes on the Pacific coast (Oregon, USA; Milker, pers. observ.) as well as observed in small numbers in semi-natural salt marshes and lagoons of the North Sea and Baltic Sea regions (Schleswig-Holstein, Germany; Lehmann, 2000). However, test abnormalities are well known here and characteristically occur for this species. Another agglutinated salt marsh species that has irregularly designed tests is Polysaccammina ipohalina (Lehmann, 2000). Interestingly, its uniserial chamber construction with frequent directional changes bears some resemblance to the test abnormalities observed for E. macrescens in this study. However, P. ipohalina is actually also more common in the mangroves and marshes of the Atlantic coast (i.e., Santa Catarina and Paraná, Brazil; Barbosa et al., 2005) and Pacific coast (i.e., Oregon, USA; Jennings and Nelson, 1992). In summary, however, apart from the preferred latitude of the above-mentioned species, namely rather in tropical and subtropical areas, they are described as unevenly grained and with distended chambers (T. irregularis) or as very fine-grained and without a spiral part of the test (P. ipohalina), which is not in agreement with the observations made in this study for the abnormally grown E. macrescens. Furthermore, the two species mentioned tend to occur in the more elevated areas of the wetlands (Lehmann, 2000; Barbosa et al., 2005), while E. macrescens has a high abundance across the entire marsh zonation. Lehmann (2000) also refers to test abnormalities of E. macrescens and describes that the spiral construction can be abandoned already in the juvenile stage for unknown reasons, but at the same time mentions that this is rather rarely the case. This supports the assumption that the observed morphological abnormalities may be due to environmental disturbances (see Section 5.3), which means that the foraminiferal individuals are reaching their upper tolerance range, where they can still live, but react stressed to it (e.g., Martínez-Colón and Hallock, 2010).
At many salt marsh sites, it can be observed that annual storm surges that temporarily inundate the salt marshes are necessary for the required sediment supply, which allows the salt marshes to grow vertically (Stumpf, 1983; Allen, 1990; Temmerman et al., 2003; Bungenstock and Schäfer, 2009), but also laterally as a function of changing sea levels (Balke et al., 2016). Thus, the long-term conservation of salt marshes is highly dependent on the persistence of a delicate balance between sediment input and hydrodynamics (such as storm surges, tides, waves, and SLR; Leonardi et al., 2016). The resulting sediment exchange between the tidal flats and the salt marshes allows for the onshore deposition and stratification of allochthonous sediments (Leonardi et al., 2018).
However, the tidal wetlands in front of the dikes at Friedrichskoog have experienced increased erosion since the completion of the polder embankment in 1852–1854 CE (Fischer, 1957). This is attributed to a prolonged period of severe storminess, which triggered the propagation of storm waves to the coastline (Röhrs, 1938; Dangendorf et al., 2014). Indeed, wave energy conditions are found to be the largest contributor to salt marsh erosion (Leonardi et al., 2016). Favored by the salt marsh retreat, a nearby narrow channel (called ‘Altfelder Priel’) migrated steadily further towards the coast, contributing to accelerated erosional processes in front of the dike (Röhrs, 1938). The high abundance of typical calcareous benthic foraminiferal tidal flat indicator species in the lower part of the sedimentary record at Friedrichskoog between 1900 CE and until the mid-1930s suggests a period of increased deposition of marine sediments due to more frequent and prolonged tidal inundation (GeoHH-FK; Figure 4A). The group of calcareous taxa, consisting mainly of Ammonia spp., C. selseyense, and H. germanica, indicates a period of dynamic depositional processes in an unvegetated tidal flat to shallow subtidal environment (Francescangeli et al., 2018). As a countermeasure to the increasing loss of land and accompanied flood risk, a 2,200 m long W–E-oriented dam (called ‘Trischendamm’) was built and completed in 1934–1936 CE (Figure 1C; Röhrs, 1938). The resulting cut-off and diversion of the previously southwards flowing channel ‘Altfelder Priel’ prevented progressive erosion and instead favored the intended near-shore sedimentation processes, as the previously prevailing high current velocities were now attenuated or diverted (Lorenzen, 1960). As a result, the amount of calcareous tidal flat foraminifera has been comparatively low at the Friedrichskoog salt marsh site (GeoHH-FK) since the mid-1930s until today (Figure 4A). The subsequent formation of a salt marsh is evident not only from the decline in calcareous tidal flat foraminifera, but also from the simultaneous occurrence of the agglutinated salt marsh foraminifera mainly dominated by the indicator species E. macrescens (Figure 4B). Consequently, the construction of the ‘Trischendamm’ successfully contributed to a hydrodynamically calmed settlement space in the proximate area of the mainland and thus promoted the local accumulation of sediment.
A similar pattern could be observed for the total time span considered (~1920–2013 CE) of the sedimentary salt marsh record in the Bay of Tümlau (TB13-1; Müller-Navarra et al., 2019). More specifically, this means that the lower part of the TB13-1 record is likewise dominated by typical calcareous tidal flat foraminifera. They indicate deposition of sediments under marine influences until about 1940 CE, and then again temporarily between the late 1940s to the mid-1950s (Figure 4A; Müller-Navarra et al., 2019). From the end of the 1950s at the latest, however, the values of calcareous foraminifera are similarly low as those in Friedrichskoog (Figure 4A). The embankment of the local polder (named ‘Tümlauer-Koog’) in 1935 CE and the subsequent drainage measures of the foreshore apparently further weakened the marine influence, which in turn favored a progressive development of the marshes fronting dikes at the Bay of Tümlau (Figure 1B). This is illustrated by the contemporaneous increase of the agglutinated foraminifera, specifically the cosmopolitan salt marsh species E. macrescens (Figure 4B; Müller-Navarra et al., 2019). While from the second half of the 20th century the foreshore areas were intensively managed, and grazing and drainage in particular played a major role, these measures were largely abandoned when the Wadden Sea National Park was established in 1985 CE (LLUR, State Office for Agriculture, Environment and Rural Areas, 2001). Since 2001 CE at the latest, a (re-)establishment of semi-natural high marsh conditions has been observed, which can be identified by an increased heterogeneous salt marsh vegetation (Stock et al., 2005) and the corresponding agglutinated foraminifera, such as B. pseudomacrescens (Müller-Navarra et al., 2019).
In addition, and considering the periods of salt marsh development, the established coastal salt marshes at Friedrichskoog and in the Bay of Tümlau have essentially been able to keep pace with the rise in mean sea level (MSL) through their vertical growth over the past century. During this period, both sites have received sufficient sediment carried in by the storm tides. These findings are documented by local sediment accretion rates of 1.16 cm yr−1 (GeoHH-FK) and 1.31 cm yr−1 (TB13-1) on average (Bunzel et al., 2020), exceeding the regional MSL of 2.4 ± 0.1 mm yr−1 at all times over the 20th century (Dangendorf et al., 2013b). It is questionable whether the ability of the salt marshes to migrate laterally with the MSL changes could also compensate for the increasing MSL trend. The point is that due to the occurrence of increased storm surge activity in the last century, extensive dike improvements were initiated, especially in response to the severe storm surge in 1962 CE (Von Storch et al., 2008). These dike raising and stabilization measures along the Wadden Sea coast may have impeded the natural ability of coastal wetlands to expand laterally and migrate further inland as the MSL rises (Kelletat, 1992; Dangendorf et al., 2013a). In recent decades, the lateral dynamics of salt marshes indicate losses rather than expansions (Gläsner, 2023). Accordingly, the seaward sides of the salt marshes are more and more exposed to erosion in the course of increased storm tide and wave activity and MSL, as also evidenced by the pronounced erosional cliffs (Schuerch et al., 2013; Müller-Navarra et al., 2019; Bunzel et al., 2020). It can be deduced that the combination of amplified hydrodynamics and grey interventions (engineered coastal protection infrastructures) at the more exposed sites at Friedrichskoog and in the Bay of Tümlau has a negative impact on the lateral growth dynamics of the Wadden Sea salt marshes, which is locally reflected in a reduction of their area sizes (Gläsner, 2023). However, given the simultaneous occurrence of abnormalities of agglutinated foraminiferal tests in high numbers, it can be assumed that the positive effects of storm tide events in terms of sediment supply for the vertical growth of salt marshes obviously also coincide with increased stress levels due to more frequent hydrodynamic disturbances of the salt marsh surface (see Section 5.3).
In modern environments, the appearance of abnormally grown foraminifera is often thought to be due to environmental shifts, such as those triggered by man-made chemical or heavy metal pollution (Yanko et al., 1998; Stouff et al., 1999; Frontalini and Coccioni, 2008; Elshanawany et al., 2011; Martínez-Colón et al., 2018). Nevertheless, irregularly grown tests can also occur at low extent (~1–3%) in non-impacted environments (Alve, 1991; Alve, 1995). Previous studies on test abnormalities focusing on intertidal foraminifera usually involved calcareous species of the genera Ammonia or (Cribro-)Elphidium. Here, the abundance of abnormally grown individuals is often associated mainly with heavy metal pollution (Martínez-Colón et al., 2009; Martínez-Colón et al., 2018), as the incorporation of alien elements (heavy metals) into the microstructure of calcareous tests during certain ontogenetic stages leads to growth disturbances (Frontalini et al., 2009). When considering the selected study areas in the Wadden Sea, heavy metal pollution seems rather unlikely as the sole cause for the observed increasing number of morphological abnormalities. Although previous studies documented heavy metal pollution levels for both Friedrichskoog and the Bay of Tümlau that were well above natural background levels during the last century (i.e., mercury concentrations >100 µg kg−1 on average), an increasing trend towards the end of the 1980s, as indicated by the foraminiferal test abnormalities, is missing (Bunzel et al., 2020). Also in previous studies, high numbers of test abnormalities of agglutinated benthic foraminifera were observed at heavily oil-polluted salt marsh sites along the Mississippi Sound and Barataria Bay (Mississippi and Louisiana, USA) and in the typical high marsh indicator species Balticammina pseudomacrescens (Brunner et al., 2013). Indeed, with the increase in maritime traffic and the growing demand and use of heavy fuel oil, the number of oil spills and illegal dumping of oil residues at sea in the southern North Sea area has increased, especially between 1969 and 1991 CE (Fleet and Reineking, 2000; Ferraro et al., 2009; Camphuysen and Vollaard, 2015). Accordingly, the salt marshes from the central Wadden Sea may have been increasingly affected by polluted water masses in times of regular high tide or storm tide inundation. Although chemical and heavy metal pollution cannot be excluded as a contributing factor, it is hypothesized that it does not appear to be the primary cause for the increasing abnormalities in E. macrescens in the salt marshes studied. Other studies also show that natural instability (such as extreme changes in abiotic factors) may be more important than anthropogenic influences (such as heavy metal exposure) for the development of abnormal foraminiferal tests (Polovodova and Schönfeld, 2008).
Both records of E. macrescensirregular exhibit a strong conformity with the regional changes in storminess and storm tide activity in the southeastern North Sea (Figure 5). While no significant long-term trends can be identified for atmospheric and astronomically driven water-level changes at the global scale for the past century, there are considerable fluctuations on a quasi-decadal time scale and at the regional level (Trenberth et al., 2007; Matulla et al., 2008; Weisse et al., 2012; Dangendorf et al., 2013a). Indeed, regional climate model simulations and historical observations showed a marked increase in storminess and the frequency of storm tide events for the North Sea area, starting in the 1960s, peaking around 1990 CE, and declining again to comparatively low levels after 1990 CE (Figure 5A; Matulla et al., 2008; Weisse et al., 2012; Mudersbach et al., 2013). It is stated that while MSL provides a kind of reference frame for storm surges (Dangendorf et al., 2013b), the fluctuations of transient extreme sea levels (such as storm surges) are in turn mainly controlled by meteorological forcing as well as by the tidal regime, upon which regional storminess can take action (Dangendorf et al., 2013a; Mudersbach et al., 2013). The pattern in the frequency and intensity of storms is thus associated with certain atmospheric and ocean configurations, but at the same time is most likely influenced in part by continuous SLR. Yet, when a positive Atlantic Multidecadal Oscillation (AMO) mode coincides with shifts from a positive to a negative North Atlantic Oscillation (NAO) mode (or vice versa), storminess appears to be highest for the NW European region (Van Vliet-Lanoë et al., 2014).
The occurrence of the E. macrescensirregular, which vary in number per site but follow the same trend of regional storm tide fluctuation, as it is further reflected by the ratio of abnormal versus regular grown tests (Figure 5C), could therefore be primarily of mechanical origin. The amplification of hydrodynamics by storm tides until the 1990s CE is most likely to have contributed to simultaneously increased turbulences and sediment re-deposition. It is likely that this effect also has caused damage to the tests of living specimens of E. macrescens, resulting in abnormal tests in their later growth cycle due to regeneration phenomena, as is reported for calcareous tidal flat foraminifera, for example (Stouff et al., 1999; Geslin et al., 2002). Interestingly, the E. macrescensirregular records are offset in time compared to the storm climate activity and are three (Bay of Tümlau; p-value = 0.005) to five years (Friedrichskoog; p-value = 0.001) behind those years when severe storm tide events occurred more frequent. This may indicate either that it takes a few years for a salt marsh system to respond, or these time lags may have been caused by the uncertainties of the age models (Bunzel et al., 2020).
As storm surges can be provoked by strong atmospheric low-pressure systems over the North Atlantic moving across the North Sea, this often also leads to high precipitation rates over Europe (e.g., Müller-Navarra and Giese, 1999; Svensson and Jones, 2002). As a result, the pore water salinity in the salt marsh soils can temporarily drop to extreme low levels, both during the periods when storm surges do not inundate the marshes and evaporation in the soil is rather small, and due to the increased precipitation associated with the storm tracks (Northrup et al., 2018). Among the abiotic factors, salinity is considered a key factor that controls the growth and reproduction of benthic foraminifera, thus setting their tolerance limits (Sen Gupta, 1999; Saraswat et al., 2015). The effect of salinity, or fresh water influx respectively, yields a lower salinity tolerance limit of about 10–15‰, at which mortality of foraminifera from coastal marine environments has been observed after only a few days (Nigam et al., 2006; Saraswat et al., 2015). Other studies, however, have shown that foraminifera can still be found alive even at 100‰ salinity, while optimal living conditions are around 33–35‰ (Nigam et al., 2006; Nigam et al., 2008). With regard to E. macrescens, it was shown by De Rijk (1995) that this species is euryhaline, but that the highest numbers of individuals occur preferentially at high seawater salinity levels (R = 0.56). It is therefore assumed that the much more critical factor, which can quickly become harmful to the foraminifera, lies at the lower salinity levels. In fact, foraminifera experience osmotic stress when temporarily exposed to fresh water, causing the cell membranes and vacuole membranes to rupture at worst (Movellan et al., 2012; Lintner et al., 2021). Therefore, extreme salinities (i.e., values at the upper and lower limits) and/or strong changes in salinity are often discussed as the most critical ecological stressor to which foraminifera respond with morphological test abnormalities for both calcareous (Geslin et al., 2000; Geslin et al., 2002; Polovodova and Schönfeld, 2008; Martínez-Colón et al., 2009) and agglutinated species (Müller-Navarra et al., 2017). In particular, strong fluctuations in salinity can lead to disturbances that cause numerous test abnormalities, if these disturbances occur during the early ontogenetic development phase of a species (Stouff et al., 1999). Consequently, it can be assumed that the salinity gradients on the salt marsh surfaces were more pronounced since the second half of the last century until the 1990s. These fluctuations were likely triggered by more frequent storm tide events as well as precipitation, causing a more drastic change between very high and very low salinity levels at ever shorter intervals.
What exactly caused the abnormalities of the investigated foraminifera and whether primarily mechanical stress (high-energy hydrodynamics) or ecological stress (salinity fluctuations) were decisive, cannot be conclusively answered in this study. Rather, it is more likely that a combination of both factors must have played a decisive role. The question arises, however, as to why the total number of abnormally grown individuals varied greatly from site to site. Reasons for this could be the different land use histories and the associated extent of human intervention. With respect to this, the main difference between the sites is that despite the establishment of the Wadden Sea National Park in the mid-1980s, the coastal management measures at Friedrichskoog were continued, at least in the area studied here, while foreshore management measures were abandoned in the Bay of Tümlau, which is a common passive habitat restoration strategy. This means that the artificial drainage ditches at Friedrichskoog are still regularly maintained today (every two to three years; pers. comm. LKN.SH, 2020), whereas in the Bay of Tümlau this has stopped. Before the National Park was established, however, the ditches had to be renewed and dredged much less frequently in the more sheltered Bay of Tümlau (every three to seven years; pers. comm. LKN.SH, 2017). Grazing also differs and is still practiced intensively in Friedrichskoog through the use of sheep, resulting in a very short sward here, while in the Bay of Tümlau it has only been practiced near the dike since the mid-1980s. Both sites therefore differ significantly in their intensive (Friedrichskoog) versus extensive (Bay of Tümlau) form of management. D’Alpaos et al. (2011) also showed, that salt marshes are considered more vulnerable to rapid increases in sediment availability, which in cultivated marshes may equally be caused by frequent dredging for drainage ditch maintenance. The effects of a strongly grazed vegetation cover with simultaneous (man-made) high sediment supply, is also reflected in the lower absolute abundance of non-abnormally grown E. macrescens specimens in the salt marsh at Friedrichskoog during the last century (around 60 specimens cm−3 on average; Figure 4B). The number of non-abnormally grown E. macrescens specimens in the salt marsh of the Bay of Tümlau is comparatively much higher (around 130 specimens cm−3 on average). Beyond that, the salt marsh in the Bay of Tümlau and the associated foraminiferal fauna seems to have benefited from the aforementioned passive restauration measures; at least a return of more diverse vegetation communities with corresponding agglutinated foraminifera indicator species could be documented from 2001 CE at the latest (Stock et al., 2005; Müller-Navarra et al., 2019). In addition, exposure to high (open coast at Friedrichskoog) or low (semi-enclosed Bay of Tümlau) energy conditions during severe swells will also have played a significant role and may be a reason for the different E. macrescensirregular numbers per site. Hence, the combination of various disturbances to the salt marsh at Friedrichskoog, such as frequent upkeep of the artificial drainage ditches, intensive sheep grazing (resulting in a short-grass vegetation cover of low biodiversity), and unsheltered exposure to currents and wave action, probably led to an increased vulnerability of the ecosystem to environmental stress. The loss of biodiversity caused by intensive foreshore cultivation in recent decades has also led to an increasing threat to the salt marsh plants belonging to the Carex type (Mierwald and Romahn, 2006): a salt-bearing sedge species on which E. macrescens prefers to occur during its epiphytic life cycle (Alve and Murray, 1999). Accordingly, this can also put E. macrescens under pressure.
Summing up, individual factors (hydrodynamics, salinity, exposure, and human interventions) and their changes or intensifications may well be within the tolerance range of E. macrescens, but in combination environmental perturbations seem to have exceeded the ecological limit (threshold), such that E. macrescens responded with increased numbers of abnormally grown tests (Figure 6A). On this basis, it can be argued that the increased rates in morphological abnormalities of E. macrescens are likely to be caused by both natural (of mechanical and ecological origins) and human-induced environmental stress. This finding is consistent with other studies that have concluded that abrupt and highly variable natural environmental changes (or interventions) are more likely to be the main cause of abnormal foraminifera growth than the effects of environmental pollution alone (e.g., Stouff et al., 1999; Geslin et al., 2002; Polovodova and Schönfeld, 2008; Martínez-Colón et al., 2009). In order to be able to give a simplified overview of the combination and effects of the stressors, a conceptual model was outlined, partly following the portfolio analysis of McKinsey & Company (e.g., ECA, Economics of Climate Adaptation, 2009). The two dimensions of the matrix represent the magnitude of the environmental stress and time between impacts (Δ time) and allow for the assessment of the influence of disturbances with different frequency and amplitude on E. macrescens (Figure 6B). Transferring this to the benthic foraminiferal records of the two salt marsh sites means that the higher the stress impact (of mechanical, physico-chemical and anthropogenic quality) in ever shorter time intervals, the more foraminiferal indicator species react to it in the form of morphological test abnormalities. Consequently, and based on the results, morphological abnormalities of typical and widespread salt marsh foraminifera can provide a powerful biomonitoring tool for assessing the extent of environmental stressors on cultivated coastal wetlands from the German Wadden Sea.
Figure 6 Conceptual links between environmental stressors and foraminiferal test abnormalities. (A) Simplified impact diagram demonstrating the various natural and human-induced environmental-stress factors on abnormal tests of E. macrescens. (B) Conceptual model outlining the increased rates in morphological abnormalities of E. macrescens (green lines) in relation to the combined influence of environmental-stress factors, particularly when disturbances occur at increasingly higher frequency and amplitude. The nine-field portfolio denotes different scenarios of low to high stress levels in dependence on the time intervals considered, and corresponding effects on the amount of abnormally grown foraminifera. Illustrations are not to scale.
The evaluation of benthic foraminiferal records from two different Wadden Sea salt marshes in Schleswig-Holstein (Germany) contributes to a better understanding of the linkages and responses between foreshore cultivation and changes in storm climate. The main conclusions are as follows:
● The sediment records of Friedrichskoog and the Bay of Tümlau reveal a similar pattern concerning the dominance of calcareous foraminifera in the lower part of the sediment sequences. Here, their abundance can be attributed to the prevailing marine depositional conditions at that time. Construction of dikes and dams at both sites in the mid-1930s to prevent land loss and flood damage resulted in a decline in calcareous tidal flat foraminifera and an increase in agglutinated salt marsh indicator species, suggesting the development of marshes fronting dikes.
● Although foreshore areas were intensively managed in Schleswig-Holstein in the mid-20th century, the establishment of the Wadden Sea National Park in 1985 CE led to a (re-)establishment of more natural high marsh conditions in recent years, as evidenced by an increase in heterogeneous salt marsh vegetation and corresponding agglutinated foraminiferal species. At least this can be observed in places for the Bay of Tümlau. In Friedrichskoog, only a low diversity of salt marsh indicator species was observed, both in terms of vegetation and agglutinated foraminifera, which is attributed to the intensive foreshore management activities.
● This study has shown that the intensely human-modified salt marsh at Friedrichskoog has the highest number of abnormally grown salt marsh foraminifera (here referred to as E. macrescensirregular) at times of increased storminess and storm tide activity from the mid-20th century until the late 1980s. In the less modified and somewhat extensively managed salt marsh of the Bay of Tümlau, the same species exhibited comparatively less numbers of abnormal tests during the same period of intensified storm climate conditions. The results suggest that the more the salt marsh ecosystems of the central Wadden Sea have been cultivated, the more vulnerable they appear to be to the impacts of increased natural environmental stresses (such as changes in hydrodynamics or salinity). It also follows that the effects of environmental stress are amplified when both natural drivers and anthropogenic influences are involved and both are closely linked in some way. Accordingly, combined environmental disturbances resulting from hydrodynamics, salinity, exposure, and various human activities (e.g., such as draining or grazing), occurring at high magnitude and with high frequency, have caused morphological test abnormalities of E. macrescens. As such this species is a powerful indicator for assessing or reconstructing the impact of environmental stress on coastal wetlands.
● The results also illustrate the ecological sensitivity and vulnerability of human-modified coastal salt marshes from the central Wadden Sea to more frequent and intensified storm-climate conditions, although the salt marshes themselves currently appear to be able to cope with ongoing climate changes. The results of this study highlight the need for further research to assess and quantify the extent to which human interventions affect the resilience of cultivated coastal ecosystems. The results also highlight the importance of preserving and restoring salt marshes as a critical component of coastal protection strategies (such as Nature-based Solutions, NbS; Schoonees et al., 2019) in the face of current and future climate change.
The datasets presented in this study can be found in online repositories. The names of the repository/repositories and accession number(s) can be found below: PANGAEA Open Access library: https://doi.pangaea.de/10.1594/PANGAEA.927336.
The manuscript presents research involving animals (single-celled protists) that does not require ethical approval for its study.
Field work, sample preparation and foraminifera analyses were carried out by DB, who also designed the study, wrote the main body of the article and designed the figures. FF contributed to the discussion of the foraminifera data. YM and GS contributed to field work and were involved in all data discussions, providing critical comments and major revisions during the preparation phase of the article. All authors contributed to the article and approved the submitted version.
This study was financially supported by grants SCHM1180/19 and MI1508/4 from the German Research Foundation (DFG) through the Special Priority Program (SPP-1889) ‘Regional Sea Level Change and Society (SeaLevel)’ under the ‘SEASTORM’ project. The first author is further financed by the Ministry for Science and Culture of Lower Saxony (MWK) and the Volkswagen Foundation within the framework of the project ‘Gute Küste Niedersachsen’ (FKZ: 76251-17-5/19).
We thank the State Office for Surveying and Geoinformation Schleswig-Holstein (LVermGeo SH), the German Meteorological Service (DWD), the Agency for Coastal Defence, National Park and Marine Conservation (LKN.SH), and the Federal Maritime and Hydrographic Agency of Germany (BSH) for providing historical observation data and for discussions. We also thank C. Wiedemann, M. Stock, and M. Padlat (National Park of the Schleswig-Holstein Wadden Sea) for the authorization to work in the coastal salt marsh at Friedrichskoog, and for providing surface elevation data of the salt marsh platform in Friedrichskoog. A very special thank you goes to K. Müller-Navarra, who provided us with the foraminiferal data and salt marsh elevation data for the Bay of Tümlau and thus added a valuable contribution to this study. And special thanks go to J. Schönfeld, for the substantial and valuable discussions. We also thank the two anonymous reviewers for their critical comments, which greatly improved the quality of the manuscript.
The authors declare that the research was conducted in the absence of any commercial or financial relationships that could be construed as a potential conflict of interest.
All claims expressed in this article are solely those of the authors and do not necessarily represent those of their affiliated organizations, or those of the publisher, the editors and the reviewers. Any product that may be evaluated in this article, or claim that may be made by its manufacturer, is not guaranteed or endorsed by the publisher.
Allen J. R. L. (1990). Salt-marsh growth and stratification: a numerical model with special reference to the Severn Estuary, southwest Britain. Mar. Geology 95, 77–96. doi: 10.1016/0025-3227(90)90042-I
Alve E. (1991). Benthic foraminifera in sediment cores reflecting heavy metal pollution in Soerfjord, western Norway. J. Foraminiferal Res. 21, 1–19. doi: 10.2113/gsjfr.21.1.1
Alve E. (1995). Benthic foraminiferal responses to estuarine pollution: a review. J. Foraminiferal Res. 25, 190–203. doi: 10.2113/gsjfr.25.3.190
Alve E., Lepland A., Magnusson J., Backer-Owe K. (2009). Monitoring strategies for re-establishment of ecological reference conditions: possibilities and limitations. Mar. Pollut. Bull. 59, 297–310. doi: 10.1016/j.marpolbul.2009.08.011
Alve E., Murray J. W. (1999). Marginal marine environments of the Skagerrak and Kattegat: a baseline study of living (stained) benthic foraminiferal ecology. Palaeogeography Palaeoclimatology Palaeoecol. 146, 171–193. doi: 10.1016/S0031-0182(98)00131-X
Andersen T. J., Svinth S., Pejrup M. (2011). Temporal variation of accumulation rates on a natural salt marsh in the 20th century – the impact of sea level rise and increased inundation frequency. Mar. Geology 279, 178–187. doi: 10.1016/j.margeo.2010.10.025
Armynot du Châtelet É., Bout-Roumazeilles V., Riboulleau A., Trentesaux A. (2009). Sediment (grain size and clay mineralogy) and organic matter quality control on living benthic foraminifera. Rev. Micropaléontologie 52, 75–84. doi: 10.1016/j.revmic.2008.10.002
Armynot du Châtelet É., Francescangeli F., Frontalini F. (2018). Definition of benthic foraminiferal bioprovinces in transitional environments of the Eastern English Channel and the Southern North Sea. Rev. Micropaléontologie 61, 223–234. doi: 10.1016/j.revmic.2018.04.001
Arns A., Dangendorf S., Jensen J., Talke S., Bender J., Pattiaratchi C. (2017). Sea-level rise induced amplification of coastal protection design heights. Sci. Rep. 7, 40171. doi: 10.1038/srep40171
Arns A., Wahl T., Dangendorf S., Jensen J. (2015). The impact of sea level rise on storm surge water levels in the northern part of the German Bight. Coast. Eng. 96, 118–131. doi: 10.1016/j.coastaleng.2014.12.002
Balke T., Stock M., Jensen K., Bouma T. J., Kleyer M. (2016). A global analysis of the seaward salt marsh extent: the importance of tidal range. Water Resour. Res. 52, 3775–3786. doi: 10.1002/2015WR018318
Barbier E. B., Hacker S. D., Kennedy C., Koch E. W., Stier A. C., Silliman B. R. (2011). The value of estuarine and coastal ecosystem services. Ecol. Monogr. 81, 169–193. doi: 10.1890/10-1510.1
Barbosa C. F., Scott D. B., Seoane J. C. S., Turcq B. J. (2005). Foraminiferal zonations as base lines for Quaternary sea-level fluctuations in south-southeast Brazilian mangroves and marshes. J. Foraminiferal Res. 35, 22–43. doi: 10.2113/35.1.22
Bartholdy A. T., Bartholdy J., Kroon A. (2010). Salt marsh stability and patterns of sedimentation across a backbarrier platform. Mar. Geology 278, 31–42. doi: 10.1016/j.margeo.2010.09.001
Bockelmann A.-C., Neuhaus R. (2002). Competitive exclusion of Elymus athericus from a high-stress habitat in a European salt marsh. J. Ecol. 87, 503–513. doi: 10.1046/j.1365-2745.1999.00368.x
Boltovskoy E., Scott D. B., Medioli F. S. (1991). Morphological variations of benthic foraminiferal tests in response to changes in ecological parameters: a review. J. Paleontology 65, 175–185. doi: 10.1017/S0022336000020394
Brady G. S. (1870). XXVI.—The Ostracoda and Foraminifera of tidal rivers. With an analysis and descriptions of the Foraminifera, by Henry B. Brady, F.L.S. Annals and Magazine of Natural History 6, 273–309.
Bromberg Gedan K., Silliman B. R., Bertness M. D. (2009). Centuries of human-driven change in salt marsh ecosystems. Annu. Rev. Mar. Sci. 1, 117–141. doi: 10.1146/annurev.marine.010908.163930
Broome S. W., Seneca E. D., Woodhouse W. W. Jr. (1988). Tidal salt marsh restoration. Aquat. Bot. 32, 1–22. doi: 10.1016/0304-3770(88)90085-X
Brunner C. A., Yeager K. M., Hatch R., Simpson S., Keim J., Briggs K. B., et al. (2013). Effects of oil from the 2010 Macondo well blowout on marsh foraminifera of Mississippi and Louisiana, USA. Environ. Sci. Technol. 47, 9115–9123. doi: 10.1021/es401943y
Bungenstock F., Schäfer A. (2009). The Holocene relative sea-level curve for the tidal basin of the barrier island Langeoog, German Bight, southern North Sea. Global Planetary Change 66, 34–51. doi: 10.1016/j.gloplacha.2008.07.007
Bunzel D., Milker Y., Müller-Navarra K., Arz H. W., Friedrich J., Lahajnar N., et al. (2020). Integrated stratigraphy of foreland salt-marsh sediments of the south-eastern North Sea region. Newsletters Stratigraphy 53, 415–442. doi: 10.1127/nos/2020/0540
Bunzel D., Milker Y., Müller-Navarra K., Arz H. W., Schmiedl G. (2021). North Sea salt-marsh archives trace past storminess and climate variability. Global Planetary Change 198, 103403. doi: 10.1016/j.gloplacha.2020.103403
Callies U., Groll N., Horstmann J., Kapitza H., Klein H., Maßmann S., et al. (2017). Surface drifters in the German Bight: model validation considering windage and stokes drift. Ocean Sci. 13, 799–827. doi: 10.5194/os-13-799-2017
Camacho S., Moura D., Connor S., Scott D., Boski T. (2015). Ecological zonation of benthic foraminifera in the lower Guadiana Estuary (southeastern Portugal). Mar. Micropaleontology 114, 1–18. doi: 10.1016/j.marmicro.2014.10.004
Camphuysen K., Vollaard B. (2015). “Oil pollution in the Dutch sector of the North Sea,” in Oil pollution in the North Sea, vol. 41. Eds. Carpenter A. (Switzerland: Springer International Publishing), 117–140.
Capotondi L., Bergami C., Orsini G., Ravaioli M., Colantoni P., Galeotti S. (2015). Benthic foraminifera for environmental monitoring: a case study in the central Adriatic continental shelf. Environ. Sci. Pollut. Res. 22, 6034–6049. doi: 10.1007/s11356-014-3778-7
D’Alpaos D., Mudd S. M., Carniello L. (2011). Dynamic response of marshes to perturbations in suspended sediment concentrations and rates of relative sea level rise. J. Geophysical Res. 116, F04020. doi: 10.1029/2011JF002093
Dangendorf S., Mudersbach C., Jensen J., Anette G., Heinrich H. (2013a). Seasonal to decadal forcing of high water level percentiles in the German Bight throughout the last century. Ocean Dynamics 63, 533–548. doi: 10.1007/s10236-013-0614-4
Dangendorf S., Mudersbach C., Wahl T., Jensen J. (2013b). Characteristics of intra-, inter-annual and decadal sea-level variability and the role of meteorological forcing: the long record of Cuxhaven. Ocean Dynamics 63, 209–224. doi: 10.1007/s10236-013-0598-0
Dangendorf S., Müller-Navarra S., Jensen J., Schenk F., Wahl T., Weisse R. (2014). North Sea storminess from a novel storm surge record since AD 1843. J. Climate 27, 3582–3595. doi: 10.1175/JCLI-D-13-00427.1
Davy A. J. (2002). “Development and structure of salt marshes: community patterns in time and space,” in Concepts and controversies in tidal marsh ecology. Eds. Weinstein M. P., Kreeger D. A. (Dordrecht: Springer), 137–156.
Davy A. J., Bakker J. P., Figueroa M. E. (2009). “Human modification of European salt marshes,” in Human impacts on salt marshes – a global perspective. Eds. Silliman B. R., Grosholz E. D., Bertness M. D. (Berkeley, Los Angeles, London: University of California Press), 311–335.
Davy A. J., Brown M. J. H., Mossman H. L., Grant A. (2011). Colonization of a newly developing salt marsh: disentangling independent effects of elevation and redox potential on halophytes. J. Ecol. 99, 1350–1357. doi: 10.1111/j.1365-2745.2011.01870.x
De Rijk S. (1995). Salinity control on the distribution of salt marsh foraminifera (Great Marshes, Massachusetts). J. Foraminiferal Res. 25, 156–166. doi: 10.2113/gsjfr.25.2.156
De Rijk S., Troelstra S. R. (1997). Salt marsh foraminifera from the Great Marshes, Massachusetts: environmental controls. Palaeogeography Palaeoclimatology Palaeoecol. 130, 81–112. doi: 10.1016/S0031-0182(96)00131-9
Doody J. P. (2008). Saltmarsh conservation, management and restoration (Dordrecht: Springer), 219 pp.
ECA, Economics of Climate Adaptation. (2009). Shaping climate-resilient development: a framework for decision-making (Climate Works Foundation, Global Environment Facility, European Commission, McKinsey & Company, Rockefeller Foundation, Standard Chartered Bank and Swiss Re).
Elshanawany R., Ibrahim M. I., Milker Y., Schmiedl G., Badr N., Kholeif S. E. A., et al. (2011). Anthropogenic impact on benthic foraminifera, Abu-Qir Bay, Alexandria, Egypt. J. Foraminiferal Res. 41, 326–348. doi: 10.2113/gsjfr.41.4.326
Esselink P., Petersen J., Arens S., Bakker J. P., Bunje J., Dijkema K. S., et al. (2009). “Salt marshes, Thematic Report No. 8,” in Quality Status Report 2009, Wadden Sea Ecosystem No. 25. Eds. Marencic H., Vlas J. (Wilhelmshaven, Germany: Common Wadden Sea Secretariat, Trilateral Monitoring and Assessment Group).
Esselink P., van Duin W. E., Bunje J., Cremer J., Folmer E. O., Frikke J., et al. (2017). “Salt marshes,” in Wadden Sea Quality Status Report 2017. Ed. Kloepper S., et al (Wilhelmshaven, Germany: Common Wadden Sea Secretariat, Trilateral Monitoring and Assessment Group).
Fagherazzi S., Kirwan M. L., Mudd S. M., Guntenspergen G. R., Temmerman S., D’Alpaos A., et al. (2012). Numerical models of salt marsh evolution: ecological, geomorphic, and climatic factors. Rev. Geophysics 50, RG1002. doi: 10.1029/2011RG000359
Fagherazzi S., Mariotti G., Leonardi N., Canestrelli A., Nardin W., Kearney W. S. (2020). Salt marsh dynamics in a period of accelerated sea level rise. J. Geophysical Research: Earth Surface 125, e2019JF005200. doi: 10.1029/2019JF005200
Fagherazzi S., Mariotti G., Wiberg P. L., McGlathery K. J. (2013). Marsh collapse does not require sea level rise. Oceanography 26, 70–77. doi: 10.5670/oceanog.2013.47
Fatela F., Taborda R. (2002). Confidence limits of species proportions in microfossil assemblages. Mar. Micropaleontology 45, 169–174. doi: 10.1016/S0377-8398(02)00021-X
Feagin R. A., Lozada-Bernard S. M., Ravens T. M., Möller I., Yeager K. M., Baird A. H. (2009). Does vegetation prevent wave erosion of salt marsh edges? PNAS 106, 10109–10113. doi: 10.1073/pnas.0901297106
Ferraro F., Meyer-Roux S., Muellenhoff O., Pavliha M., Svetak J., Tarchi D., et al. (2009). Long term monitoring of oil spills in European seas. Int. J. Remote Sens. 30, 627–645. doi: 10.1080/01431160802339464
Feyling-Hanssen R. W. (1972). The foraminifer Elphidium excavatum (Terquem) and its variant forms. Micropaleontology 18, 337–354. doi: 10.2307/1485012
Fischer O. (1957). Das Wasserwesen an der schleswig-holsteinischen Nordseeküste, Dithmarschen (Berlin: Dritter Teil: das Festland. Verlag von Dietrich Reimer).
Fleet D. M., Reineking B. (2000). “Have efforts to clean up the marine environment been successful? – German beached bird surveys provide an index for oil pollution levels in the southern North Sea,” in Oil and hydrocarbon spills II: modelling, analysis and control. Eds. Brebbia C. A., Rodriguez G. R. (Southampton, Boston: WIT Press), 117–126.
Francescangeli F., Armynot du Chatelet E., Billon G., Trentesaux A., Bouchet V. M. P. (2016). Palaeo-ecological quality status based on foraminifera of Boulognesur-Mer harbour (Pas-de-Calais, Northeastern France) over the last 200 years. Mar. Environ. Res. 117, 32–43. doi: 10.1016/j.marenvres.2016.04.002
Francescangeli F., Bouchet V. M. P., Trentesaux A., Armynot du Chatelet E. (2017). Does elevation matter? Living foraminiferal distribution in a hyper tidal salt marsh (Canche Estuary, Northern France). Estuarine Coast. Shelf Sci. 194, 192–204. doi: 10.1016/j.ecss.2017.06.023
Francescangeli F., Milker, Bunzel D., Thomas H., Norbisrath M., Schönfeld J., et al. (2021). Recent benthic foraminiferal distribution in the Elbe Estuary (North Sea, Germany): a response to environmental stressors. Estuarine Coast. Shelf Sci. 251, 107198. doi: 10.1016/j.ecss.2021.107198
Francescangeli F., Portela M., Armynot du Chatelet E., Billon G., Andersen T. J., Bouchet V. M. P., et al. (2018). Infilling of the Canche Estuary (eastern English Channel, France): insight from benthic foraminifera and historical pictures. Mar. Micropaleontology 142, 1–12. doi: 10.1016/j.marmicro.2018.05.003
Frontalini F., Buosi C., Da Pelo S., Coccioni R., Cherchi A., Bucci C. (2009). Benthic foraminifera as bio-indicators of trace element pollution in the heavily contaminated Santa Gilla lagoon (Cagliari, Italy). Mar. pollut. Bull. 58, 858–877. doi: 10.1016/j.marpolbul.2009.01.015
Frontalini F., Coccioni R. (2008). Benthic foraminifera for heavy metal pollution monitoring: a case study from the central Adriatic Sea coast of Italy. Estuarine Coast. Shelf Sci. 76, 404–417. doi: 10.1016/j.ecss.2007.07.024
Gehrels W. R., Newman S. W. G. (2004). Salt-marsh foraminifera in Ho Bugt, western Denmark, and their use as sea-level indicators. Geografisk Tidsskrift Danish J. Geogr. 104, 97–106. doi: 10.1080/00167223.2004.10649507
Gerber M., Ganske A., Müller-Navarra S., Rosenhagen G. (2016). Categorisation of meteorological conditions for storm tide episodes in the German Bight. Meteorologische Z. 25, 447–462. doi: 10.1127/metz/2016/0660
Geslin E., Debenay J.-P., Duleba W., Bonetti C. (2002). Morphological abnormalities of foraminiferal tests in Brazilian environments: comparison between polluted and non-polluted areas. Mar. Micropaleontology 45, 151–168. doi: 10.1016/S0377-8398(01)00042-1
Geslin E., Stouff V., Debenay J.-P., Lesourd M. (2000). “Environmental variation and foraminiferal test abnormalities,” in Environmental micropaleontology: the application of microfossils to environmental geology. Ed. Martin R. E. (New York: Kluwer Academic/Plenum Publishers), 191–215.
Gettner. (2003). Vegetationsveränderungen in Festland-Salzmarschen an der Westküste Schleswig-Holsteins – elf Jahre nach Änderung der Nutzung Vol. 30 (Kieler Notizen zur Pflanzenkunde in Schleswig-Holstein und Hamburg 30 (Kiel)), 69–83.
Gläsner D. (2023). The impacts of management measures on the lateral dynamics of salt marshes in the Wadden Sea region. Master’s thesis. (Hannover (Germany): Leibniz University Hannover, Ludwig-Franzius-Institute of Hydraulic, Estuarine and Coastal Engineering), 97 pp.
Goldstein S. T. (1999). “Foraminifera: a biological overview,” in Modern foraminifera. Ed. Sen Gupta B. K. (Dordrecht, The Netherlands: Kluwer Academic Publishers), 37–55.
Hammer Ø., Harper D. A. T., Ryan P. D. (2001). PAST: paleontological statistics software package for education and data analysis. Palaeontologia Electronica 4, 1–9.
Haslett S. K., Davies P., Strawbridge F. (1997). Reconstructing Holocene sea-level change in the Severn Estuary and Somerset Levels: the foraminifera connection. Archaeology Severn Estuary 8, 29–40.
Hayward B. W., Figueira B. O., Sabaa A. T., Buzas M. A. (2014). Multi-year life spans of high salt marsh agglutinated foraminifera from New Zealand. Mar. Micropaleontology 109, 54–65. doi: 10.1016/j.marmicro.2014.03.002
He Q., Silliman B. R. (2019). Climate change, human impacts, and coastal ecosystems in the Anthropocene. Curr. Biol. 29, R1021–R1035. doi: 10.1016/j.cub.2019.08.042
Hofstede J. L. A. (2003). Integrated management of artificially created salt marshes in the Wadden Sea of Schleswig-Holstein, Germany. Wetlands Ecol. Manage. 11, 183–194. doi: 10.1023/A:1024248127037
Horsburgh K., Wilson C. (2007). Tide-surge interaction and its role in the distribution of surge residuals in the North Sea. J. Geophysical Res. 112, C08003. doi: 10.1029/2006JC004033
Horton B. P. (1999). The distribution of contemporary intertidal foraminifera at Cowpen Marsh, Tees Estuary, UK: implications for studies of Holocene sea-level changes. Palaeogeography Palaeoclimatology Palaeoecol. 149, 127–149. doi: 10.1016/S0031-0182(98)00197-7
Horton B. P., Edwards R. J. (2006). Quantifying Holocene sea-level change using intertidal foraminifera: lessons from the British Isles (Cushman Foundation for Foraminiferal Research, Fredericksburg, Special Publication). 40, 97 pp.
Horton B. P., Edwards R. J., Lloyd J. M. (1999a). UK intertidal foraminiferal distributions: implications for sea-level studies. Mar. Micropaleontology 36, 205–223. doi: 10.1016/S0377-8398(99)00003-1
Horton B. P., Edwards R. J., Lloyd J. M. (1999b). A foraminiferal-based transfer function: implications for sea-level studies. J. Foraminiferal Res. 29, 117–129. doi: 10.2113/gsjfr.29.2.117
Hottinger L. C. (2000). Functional morphology of benthic foraminiferal shells, envelopes of cells beyond measure. Micropaleontology 46, 57–86.
IPCC (2019). IPCC special report on the ocean and cryosphere in a changing climate. Eds. Pörtner H.-O., Roberts D. C., Masson-Delmotte V., Zhai P., Tignor M., Poloczanska E., Mintenbeck K., Alegría A., Nicolai M., Okem A., Petzold J., Rama B., Weyer N. M. (Cambridge, UK and New York, NY, USA: Cambridge University Press). doi: 10.1017/9781009157964
IPCC. (2022). Climate change 2022: Impacts, adaptation, and vulnerability. Contribution of working group II to the sixth assessment report of the Intergovernmental Panel on Climate Change. Eds. Pörtner H.-O., Roberts D. C., Tignor M., Poloczanska E. S., Mintenbeck K., Alegría A., Craig M., Langsdorf S., Löschke S., Möller V., Okem A., Rama B. (Cambridge, UK and New York, NY, USA: Cambridge University Press). doi: 10.1017/9781009325844
Jennings A. E., Nelson A. R. (1992). Foraminiferal assemblage zones in Oregon tidal marshes – relation to marsh floral zones and sea level. J. Foraminiferal Res. 22, 13–29. doi: 10.2113/gsjfr.22.1.13
Kaminski M. A., Amao A. O., Garrison T. F., Fiorini F., Magliveras S., Tawabini B. S., et al. (2020). An Entzia-dominated marsh-type agglutinated foraminiferal assemblage from a salt marsh in Tubli Bay, Bahrain. Geology Geophysics Environ. 46, 189–204. doi: 10.7494/geol.2020.46.3.189
Kathilankal J. C., Mozdzer T. J., Fuentes J. D., D’Odorico P., McGlathery K. J., Zieman J. C. (2008). Tidal influences on carbon assimilation by a salt marsh. Environ. Res. Lett. 3, 44010. doi: 10.1088/1748-9326/3/4/044010
Kelletat D. (1992). Coastal erosion and protection measures at the German North Sea coast. J. Coast. Res. 8, 699–711.
Kemp A. C., Horton B. P., Culver S. J. (2009). Distribution of modern salt-marsh foraminifera in the Albemarle-Pamlico estuarine system of North Carolina, USA: implications for sea-level research. Mar. Micropaleontology 72, 222–238. doi: 10.1016/j.marmicro.2009.06.002
Kemp A. C., Wright A. J., Cahill N (2020). Enough is enough, or more is more? Testing the influence of foraminiferal count size on reconstructions of paleo-marsh elevation. J. Foraminiferal Res. 50, 266–278. doi: 10.2113/gsjfr.50.3.266.
Kirwan M. L., Mudd S. M. (2012). Response of salt-marsh carbon accumulation to climate change. Nature 489, 550–553. doi: 10.1038/nature11440
Kirwan M. L., Temmerman S., Skeehan E. E., Guntenspergen G. R., Fagherazzi S. (2016b). Overestimation of marsh vulnerability to sea level rise. Nat. Climate Change 6, 253–260. doi: 10.1038/NCLIMATE2909
Kirwan M. L., Walters D. C., Reay W. G., Carr J. A. (2016a). Sea level driven marsh expansion in a coupled model of marsh erosion and migration. Geophysical Res. Lett. 43, 4366–4373. doi: 10.1002/2016GL068507
Lal K. K., Bonetti C., Woodroffe C. D., Rogers K. (2020). Contemporary distribution of benthic foraminiferal assemblages in coastal wetlands of south-eastern Australia. Estuarine Coast. Shelf Sci. 245, 106949. doi: 10.1016/j.ecss.2020.106949
Lang A., Mikolajewicz U. (2020). Rising extreme sea levels in the German Bight under enhanced CO2 levels: a regionalized large ensemble approach for the North Sea. Climate Dynamics 55, 1829–1842. doi: 10.1007/s00382-020-05357-5
Lehmann G. (2000). Vorkommen, Populationsentwicklung, Ursache fleckenhafter Besiedlung und Fortpflanzungsbiologie von Foraminiferen in Salzwiesen und Flachwasser der Nord- und Ostseeküste Schleswig – Holsteins (Kiel (Germany: Faculty of Mathematics and Natural Sciences, Kiel University).
Leonardi N., Carnacina I., Donatelli C., Ganjuc N. K., Plater A. J., Schuerch M., et al. (2018). Dynamic interactions between coastal storms and salt marshes: a review. Geomorphology 301, 92–107. doi: 10.1016/j.geomorph.2017.11.001
Leonardi N., Ganju N. K., Fagherazzi S. (2016). A linear relationship between wave power and erosion determines salt-marsh resilience to violent storms and hurricanes. PNAS 113, 64–68. doi: 10.1073/pnas.1510095112
Lintner M., Lintner B., Wanek W., Keul N., von der Kammer F., Hofmann T., et al. (2021). Effects of heavy elements (Pb, Cu, Zn) on algal food uptake by Elphidium excavatum (Foraminifera). Heliyon 7, e08427. doi: 10.1016/j.heliyon.2021.e08427
LLUR, State Office for Agriculture, Environment and Rural Areas. (2020). FFH-Bericht 2019 des Landes Schleswig-Holstein. (Methodik, Ergebnisse und Konsequenzen. Ministerium für Energiewende, Landwirtschaft, Umwelt, Natur und Digitalisierung des Landes Schleswig-Holstein (Kiel)), 132 pp. Available at: https://www.schleswig-holstein.de/D.
LLUR, State Office for Agriculture, Environment and Rural Areas. (2001). Vorlandmanagementkonzept – Erfahrungsbericht 1995–2000 (Landesamt für Landwirtschaft, Umwelt und ländliche Räume (Kiel)), 40.
Marszalek D. S., Wright R. C., Hay W. W. (1969). Function of the test in foraminifera. Gulf Coast. Assoc. Geological Societies Trans. 19, 341–352.
Martínez L. T., Marchant M., Urbina M. (2023). Are physiological responses in foraminifera reliable environmental stress bioindicators? A systematic review. Environ. Res. 216, 114515. doi: 10.1016/j.envres.2022.114515
Martínez-Colón M., Hallock P. (2010). Preliminary survey on foraminiferal responses to pollutants in Torrecillas Lagoon Puerto Rico. Caribbean J. Sci. 46, 1–6. doi: 10.18475/cjos.v46i1.a14
Martínez-Colón M., Hallock P., Green-Ruíz C. (2009). Strategies for using shallow-water benthic foraminifers as bioindicators of potentially toxic elements: a review. J. Foraminiferal Res. 39, 278–299. doi: 10.2113/gsjfr.39.4.278
Martínez-Colón M., Hallock P., Green Ruíz C., Smoak J. M. (2018). Benthic foraminifera as bioindicators of potentially toxic element (PTE) pollution: Torrecillas lagoon (San Juan Bay Estuary), Puerto Rico. Ecol. Indic. 89, 516–527. doi: 10.1016/j.ecolind.2017.10.045
Matulla C., Schöner W., Alexandersson H., von Storch H., Wang X. L. (2008). European storminess: late nineteenth century to present. Climate Dynamics 31, 125–130. doi: 10.1007/s00382-007-0333-y
Mcleod E., Chmura G. L., Bouillon S., Salm R., Björk M., Duarte C. M., et al. (2011). A blueprint for blue carbon: toward an improved understanding of the role of vegetated coastal habitats in sequestering CO2. Front. Ecol. Environ. 9, 552–560. doi: 10.1890/110004
Meier D. (2004). Man and environment in the marsh area of Schleswig–Holstein from Roman until late Medieval times. Quaternary Int. 112, 55–69. doi: 10.1016/S1040-6182(03)00065-X
Mierwald U., Romahn K. (2006). Die Farn- und Blütepflanzen Schleswig-Holsteins, Rote Liste Band 1 (Kiel: Landesamt für Natur und Umwelt des Landes Schleswig-Holstein).
Milker Y., Horton B. P., Nelson A. R., Engelhart S. E., Witter R. C. (2015). Variability of intertidal foraminiferal assemblages in a salt marsh, Oregon, USA. Mar. Micropaleontology 118, 1–16. doi: 10.1016/j.marmicro.2015.04.004
Miller C. B., Rodriguez A. B., Bost M. C. (2021). Sea-level rise, localized subsidence, and increased storminess promote saltmarsh transgression across low-gradient upland areas. Quaternary Sci. Rev. 265, 107000. doi: 10.1016/j.quascirev.2021.107000
Möller I., Kudella M., Rupprecht F., Spencer T., Paul M., van Wesenbeeck B. K., et al. (2014). Wave attenuation over coastal salt marshes under storm surge conditions. Nat. Geosci. 7, 727–731. doi: 10.1038/NGEO2251
Moodley L., Boschker H. T. S., Middelburg J. J., Pel R., Herman P. M. J., de Deckere E., et al. (2000). Ecological significance of benthic foraminifera: 13C labelling experiments. Mar. Ecol. Prog. Ser. 202, 289–295. doi: 10.3354/meps202289
Morris J. T., Sundareshwar P. V., Nietch C. T., Kjerfve B., Cahoon D. R. (2002). Responses of coastal wetlands to rising sea level. Ecology 83, 2869–2877. doi: 10.1890/0012-9658(2002)083[2869:ROCWTR]2.0.CO;2
Movellan A., Schiebel R., Zubkov M. V., Smyth A., Howa H. (2012). Protein biomass quantification of unbroken individual foraminifers using nano-spectrophotometry. Biogeosciences 9, 3613–3623. doi: 10.5194/bg-9-3613-2012
Mudersbach C., Wahl T., Haigh I. D., Jensen J. (2013). Trends in high sea levels of German North Sea gauges compared to regional mean sea level changes. Continental Shelf Res. 65, 111–120. doi: 10.1016/j.csr.2013.06.016
Müller P., Ladiges N., Jack A., Schmiedl G., Kutzbach L., Jensen K., et al. (2018). Assessing the long-term carbon-sequestration potential of the semi-natural salt marshes in the European Wadden Sea. Ecosphere 10, e02556. doi: 10.1002/ecs2.2556
Müller-Navarra S., Giese H. (1999). Improvements of an empirical model to forecast wind surge in the German Bight. German J. Hydrography 51, 385–405. doi: 10.1007/BF02764162
Müller-Navarra K., Milker Y., Bunzel D., Lindhorst S., Friedrich J., Arz H. W., et al. (2019). Evolution of a salt marsh in the southeastern North Sea region – anthropogenic and natural forcing. Estuarine Coast. Shelf Sci. 218, 268–277. doi: 10.1016/j.ecss.2018.12.022
Müller-Navarra K., Milker Y., Schmiedl G. (2016). Natural and anthropogenic influence on the distribution of salt marsh foraminifera in the Bay of Tümlau, German North Sea. J. Foraminiferal Res. 46, 61–74. doi: 10.2113/gsjfr.46.1.61
Müller-Navarra K., Milker Y., Schmiedl G. (2017). Applicability of transfer functions for relative sea-level reconstructions in the southern North Sea coastal region based on salt-marsh foraminifera. Mar. Micropaleontology 135, 15–31. doi: 10.1016/j.marmicro.2017.06.003
Murray J. (2006). Ecology and applications of benthic foraminifera (Cambridge, New York, Melbourne: Cambridge University Press).
Narayan S., Beck M. W., Reguero B. G., Losada I. J., van Wesenbeeck B., Pontee N., et al. (2016). The effectiveness, costs and coastal protection benefits of natural and nature-based defences. PloS One 11, e0154735. doi: 10.1371/journal.pone.0154735
Nigam R., Kurtarkar S. R., Saraswat R., Linshy V. N., Rana S. S. (2008). Response of benthic foraminifera Rosalina leei to different temperature and salinity, under laboratory culture experiment. J. Mar. Biol. Assoc. United Kingdom 88, 699–704. doi: 10.1017/S0025315408001197
Nigam R., Saraswat R., Kurtarkar S. R. (2006). Laboratory experiment to study the effect of salinity variations on benthic foraminiferal species - Pararotalia nipponica (Asano). J. Geological Soc. India 67, 41–46.
Northrup K., Capooci M., Seyfferth A. L. (2018). Effects of extreme events on arsenic cycling in salt marshes. J. Geophysical Research: Biogeosciences 123, 1086–1100. doi: 10.1002/2017JG004259
Paillard D., Labeyrie L., Yiou P. (1996). Macintosh program performs time-series analysis. Eos 77, 379. doi: 10.1029/96EO00259
Polovodova I., Schönfeld J. (2008). Foraminiferal test abnormalities in the western Baltic Sea. J. Foraminiferal Res. 38, 318–336. doi: 10.2113/gsjfr.38.4.318
Pontee N. (2011). Reappraising coastal squeeze: a case study from north-west England. Maritime Eng. 164, 127–138. doi: 10.1680/maen.2011.164.3.127
Pontee N., Narayan S., Beck M. W., Hosking A. H. (2016). Naturebased solutions: lessons from around the world. Proc. Institution Civil Engineers - Maritime Eng. 169, 29–36. doi: 10.1680/jmaen.15.00027
Pugh D. T. (1987). Tides, surges, and mean sea level (Chichester, New York, Brisbane, Toronto, Singapore: John Wiley & Sons).
Röhrs W. (1938). Der Dammbau zur Sicherung des Seedeiches an der Friedrichskoogspitze in Süderdithmarschen. Westküste 2, 1–15.
Rupprecht F., Wanner A., Stock M., Jensen K. (2015). Succession in saltmarshes – large-scale and long-term patterns after abandonment of grazing and drainage. Appl. Vegetation Sci. 18, 86–98. doi: 10.1111/avsc.12126
Saraswat R., Kouthanker M., Kurtarkar S. R., Nigam R., Naqvi S. W. A., Linshy V. N. (2015). Effect of salinity induced pH/alkalinity changes on benthic foraminifera: a laboratory culture experiment. Estuarine Coast. Shelf Sci. 153, 96–107. doi: 10.1016/j.ecss.2014.12.005
Scheder J., Frenzel P., Bungenstock F., Engel M., Brückner H., Pint A. (2019). Vertical and lateral distribution of foraminifera and ostracoda in the East Frisian Wadden Sea – developing a transfer function for relative sea-level change. Geologica Belgica 22, 99–110. doi: 10.20341/gb.2019.007
Schönfeld J. (2018). Monitoring benthic foraminiferal dynamics at Bottsand coastal lagoon (western Baltic Sea). J. Micropalaeontology 37, 383–393. doi: 10.5194/jm-37-383-2018
Schönfeld J., Alve E., Geslin E., Jorissen F., Korsun S., Spezzaferri S., et al. (2012). The FOBIMO (FOraminiferal BIo-MOnitoring) initiative – towards a standardized protocol for soft-bottom benthic foraminiferal monitoring studies. Mar. Micropaleontology 94-95, 1–13. doi: 10.1016/j.marmicro.2012.06.001
Schoonees T., Mancheño A. G., Scheres B., Bouma T. J., Silva R., Schlurmann T., et al. (2019). Hard structures for coastal protection, towards greener designs. Estuaries Coasts 42, 1709–1729. doi: 10.1007/s12237-019-00551-z
Schröder H. K., Kiehl K., Stock M. (2002). Directional and non-directional vegetation changes in a temperate salt marsh in relation to biotic and abiotic factors. Appl. Vegetation Sci. 5, 33–44. doi: 10.1111/j.1654-109X.2002.tb00533.x
Schuerch M., Vafeidis A., Slawig T., Temmerman S. (2013). Modeling the influence of changing storm patterns on the ability of a salt marsh to keep pace with sea level rise. J. Geophysical Research: Earth Surface 118, 84–96. doi: 10.1029/2012JF002471
Scott D. B., Hermelin J. O. R. (1993). A device for precision splitting of micropaleontological samples in liquid suspension. J. Paleontology 67, 151–154. doi: 10.1017/S0022336000021302
Scott D. K., Leckie R. M. (1990). Foraminiferal zonation of Great Sippewissett salt marsh (Falmouth, Massachusetts). J. Foraminiferal Res. 20, 248–266. doi: 10.2113/gsjfr.20.3.248
Scott D. B., Medioli F. S. (1986). “Foraminifera as sea-level indicators,” in Sea-level research: a manual for the collection and evaluation of data. Ed. van de Plassche O. (Norwich England: International Geological Correlation Project 64, Geo Books), 435–456.
Sen Gupta B. K. (1999). “Foraminifera in marginal marine environments,” in Modern foraminifera. Ed. Sen Gupta B. K. (Dordrecht, The Netherlands: Kluwer Academic Publishers), 141–159.
Siemes R. W. A., Borsje B. W., Daggenvoorde R. J., Hulscher S. J. M. H. (2020). Artificial structures steer morphological development of salt marshes: a model study. J. Mar. Sci. Eng. 8, 326. doi: 10.3390/jmse8050326
Stock M. (2003). Salzwiesenschutz im Schleswig-Holsteinischen Wattenmeer. Vogelkundlicher Bericht Niedersachsen 35, 115–124.
Stock M., Gettner S., Hagge M., Heinzel K., Kohlus J., Stumpe H. (2005). Salzwiesen an der Westküste von Schleswig-Holstein 1988-2001. Schriftenreihe Des. Nationalparks Schleswig-Holsteinisches Wattenmeer (Tönning), Heft 15, 239.
Stock M., Maier M. (2016). Salzwiesenschutz im Nationalpark Wattenmeer: ein Überblick (Conservation of salt marshes within the National Park Wadden Sea – an overview). Vogelkundliche Berichte aus Niedersachsen 44, 131–156.
Stouff V., Geslin E., Debenay J.-P., Lesourd M. (1999). Origin of morphological abnormalities in Ammonia (foraminifera): studies in laboratory and natural environments. J. Foraminiferal Res. 29, 152–170. doi: 10.2113/gsjfr.29.2.152
Stumpf R. P. (1983). The process of sedimentation on the surface of a salt marsh. Estuarine Coast. Shelf Sci. 17, 495–508. doi: 10.1016/0272-7714(83)90002-1
Suchrow S., Pohlmann N., Stock M., Jensen K. (2012). Long-term surface elevation changes in German North Sea salt marshes. Estuarine Coast. Shelf Sci. 98, 71–83. doi: 10.1016/j.ecss.2011.11.031
Svensson C., Jones D. A. (2002). Dependence between extreme sea surge, river flow and precipitation in eastern Britain. Int. J. Climatology 22, 1149–1168. doi: 10.1002/joc.794
Temmerman S., Govers G., Meire P., Wartel S. (2003). Modelling long-term tidal marsh growth under changing tidal conditions and suspended sediment concentrations, Scheldt estuary, Belgium. Mar. Geology 193, 151–169. doi: 10.1016/S0025-3227(02)00642-4
Trenberth K. E., Jones P. D., Ambenje P., Bojariu R., Easterling D., Klein Tank A., et al. (2007). “Observations: surface and atmospheric climate change,” in Climate change 2007: the physical science basis. Contribution of Working Group I to the Fourth Assessment Report of the Intergovernmental Panel on Climate Change. Eds. Solomon S., Qin D., Manning M., Chen Z., Marquis M., Averyt K. B., Tignor M., Miller H. L. (Cambridge, United Kingdom and New York, NY, USA: Cambridge University Press), 1–102.
Tyszka J. (2006). Morphospace of foraminiferal shells: results from the moving reference model. Lethaia 39, 1–12. doi: 10.1080/00241160600575808
Tyszka J., Topa P., Saczka K. (2005). State-of-the-art in modelling of foraminiferal shells: searching for an emergent model. Studia Geologica Polonica 124, 143–157.
Van der Wal D., Pye K. (2004). Patterns, rates and possible causes of saltmarsh erosion in the Greater Thames area (UK). Geomorphology 61, 373–391. doi: 10.1016/j.geomorph.2004.02.005
Van de Ven C. N., Reijers V. C., Lammers C., van Belzen J., Chung Y., Bouma T. J., et al. (2023). Establishing cordgrass plants cluster their shoots to avoid ecosystem engineering. Funct. Ecol. 37, 1339–1349. doi: 10.1111/1365-2435.14302
Van Proosdij D., Davidson-Arnott R. G. D., Ollerhead J. (2006). Controls on spatial patterns of sediment deposition across a macro-tidal salt marsh surface over single tidal cycles. Estuarine Coast. Shelf Sci. 69, 64–86. doi: 10.1016/j.ecss.2006.04.022
Van Vliet-Lanoë B., Penaud A., Hénaff A., Delacourt C., Fernane A., Goslin J., et al. (2014). Middle- to late-Holocene storminess in Brittany (NW France): part II – the chronology of events and climate forcing. Holocene 24, 434–453. doi: 10.1177/0959683613519688
Van Wijnen H. J., Bakker J. P., de Vries Y. (1997). Twenty years of salt marsh succession on a Dutch coastal barrier island. J. Coast. Conserv. 3, 9–18. doi: 10.1007/BF02908174
Von Lieberman N., Schwarze H., Zimmermann C. (1998). Ausführung und Wirkungsweise von Lahnungen. Die Küste 60, 191–225.
Von Storch H., Gönnert G., Meine M. (2008). Storm surges – an option for Hamburg, Germany, to mitigate expected future aggravation of risk. Environ. Sci. Policy 11, 735–742. doi: 10.1016/j.envsci.2008.08.003
Walters D. C., Carr J. A., Hockaday A., Jones J. A., McFarland E., Kovalenko K. E., et al. (2021). Experimental tree mortality does not induce marsh transgression in a Chesapeake Bay low-lying coastal forest. Front. Mar. Sci. 8. doi: 10.3389/fmars.2021.782643
Watson E. B., Oczkowski A. J., Wigand C., Hanson A. R., Davey E. W., Crosby S. C., et al. (2014). Nutrient enrichment and precipitation changes do not enhance resiliency of salt marshes to sea level rise in the northeastern U.S. Climatic Change 125, 501–509. doi: 10.1007/s10584-014-1189-x
Weisse R., von Storch H., Niemeyer H. D., Knaack H. (2012). Changing North Sea storm surge climate: an increasing hazard? Ocean Coast. Manage. 68, 58–68. doi: 10.1016/j.ocecoaman.2011.09.005
Wessels T.-F. (2021). Addressing the challenges of climate change for coastal protection in Lower Saxony using the example of a coastal section near Neßmersiel (Aurich district) (Hannover (Germany: Leibniz University Hannover, Institute of Environmental Planning).
Wright A. J., Edwards R. J., van de Plassche O. (2011). Reassessing transfer-function performance in sea-level reconstruction based on benthic salt-marsh foraminifera from the Atlantic coast of NE North America. Mar. Micropaleontology 81, 43–62. doi: 10.1016/j.marmicro.2011.07.003
Keywords: cultural landscapes, environmental stress, ecological indicators, foraminiferal test abnormalities, Entzia macrescens
Citation: Bunzel D, Milker Y, Francescangeli F and Schmiedl G (2023) Braving the extremes: foraminifera document changes in climate-induced and anthropogenic stress in Wadden Sea salt marshes. Front. Ecol. Evol. 11:1199281. doi: 10.3389/fevo.2023.1199281
Received: 03 April 2023; Accepted: 08 September 2023;
Published: 28 September 2023.
Edited by:
Ming Li, University of Liverpool, United KingdomReviewed by:
Veronica Rossi, University of Bologna, ItalyCopyright © 2023 Bunzel, Milker, Francescangeli and Schmiedl. This is an open-access article distributed under the terms of the Creative Commons Attribution License (CC BY). The use, distribution or reproduction in other forums is permitted, provided the original author(s) and the copyright owner(s) are credited and that the original publication in this journal is cited, in accordance with accepted academic practice. No use, distribution or reproduction is permitted which does not comply with these terms.
*Correspondence: Dorothea Bunzel, YnVuemVsQGx1ZmkudW5pLWhhbm5vdmVyLmRl
Disclaimer: All claims expressed in this article are solely those of the authors and do not necessarily represent those of their affiliated organizations, or those of the publisher, the editors and the reviewers. Any product that may be evaluated in this article or claim that may be made by its manufacturer is not guaranteed or endorsed by the publisher.
Research integrity at Frontiers
Learn more about the work of our research integrity team to safeguard the quality of each article we publish.