- 1Senckenberg Natural History Collections Dresden, Museum of Zoology, Dresden, Germany
- 2TU Dresden, University Hospital Carl Gustav Carus, Institute of Clinical Chemistry and Laboratory Medicine, Dresden, Germany
- 3TU Dresden, Faculty of Medicine, Institute for Clinical Pharmacology, Dresden, Germany
- 4Department of Biochemistry, Max Planck Institute for Chemical Ecology, Jena, Germany
- 5Department of Archaeology, Max Planck Institute of Geoanthropology, Jena, Germany
The spurge hawkmoth Hyles euphorbiae (Lepidoptera, Sphingidae) is an insect herbivore feeding exclusively on spurges (Euphorbia). These are toxic due to their secondary metabolites including diterpene esters with numerous harmful biological activities, preventing their use by most herbivores. Extracts of frass from H. euphorbiae and of its main larval food plant in Central Europe, the cypress spurge (Euphorbia cyparissias L.), were analyzed via liquid chromatography using a triple-quadrupole (MS/MS) and a high-resolution–quadrupole time-of-flight mass spectrometer (QTOF-MS). We report for the first time the finding of the tigliane (phorbol ester) phorbol-13-acetate and two 12-O-tetradecanoylphorbol-13-acetate (TPA) isomers of unknown structure (compounds 2 and 3) in E. cyparissias. Our data support the assignment of these two compounds as tiglianes and are therefore referred to by us as “putative tiglianes”. Additionally, the distribution of the three reported compounds within the plant parts (leaves, stem and root) was analyzed to gain first insights into the metabolite conditions H. euphorbiae copes with in nature. Phorbol-13-acetate was detected in the plant’s root only, while the other compounds were observed in all three plant parts, although in different distributions. Moreover, our results indicate that the occurrence of compound 3 rises upon larval feeding. Finally, the presence of the plant-derived putative tiglianes in frass from E. cyparissias leaves feeding larvae is confirmed, corroborating the close plant–herbivore interaction.
Introduction
The spurge hawkmoth species Hyles euphorbiae (Lepidoptera: Sphingidae) is well known for its big and aposematic larvae. Whereas the moths are inconspicuous, the larvae are brightly colored in yellow, green, orange, and red with patches of black and oval subdorsal spots of white on every segment (Hundsdoerfer et al., 2011). In organismic interactions, such aposematism has been developed to protect prey from predators, signaling that they are toxic upon ingestion. The larvae feed on several spurge species (Euphorbia, Euphorbiaceae), whereby they utilize cypress spurge (Euphorbia cyparissias L.) as their main food plant in Central Europe (personal observation AKH). E. cyparissias is a small perennial plant with narrow leaves, which is common in a wide range of habitats in Europe but also occurs in other regions such as North America (Ott and Hecker, 1981; Lanzotti et al., 2015). One specific characteristic of this and other Euphorbia species is milky sap or latex (e.g., Metcalfe, 1967), usually leaking from latex vessels upon damaging the plant (Stanković and Zlatić, 2014). Plant latex is known to be a source of diterpenes (Harborne and Held, 1995), which, in the case of Euphorbia plants, were reported to exhibit numerous harmful biological activities including tumor-promoting, cytotoxic, and skin irritant (Shi et al., 2008). Consequently, Euphorbia diterpene esters from several spurges have been shown to exhibit molluscicidal and anti-feedant properties (e.g., E. paralias; Abdelgaleil et al., 2002) and those of Jatropha curcas (Euphorbiaceae; Wink et al., 1997) were shown to exhibit insecticidal activities against several species of, e.g., moths, butterflies, and aphids. There are only a few other herbivores that feed on Euphorbia species in Central Europe (Manojlovic and Keresi, 1997; personal observation AKH), indicating the effectiveness of their chemical defense against herbivores. A very biologically active diterpene found in spurges is 12-O-tetradecanoylphorbol-13-acetate Figure 1B), which was first described in croton oil (Croton tiglium, Euphorbiaceae, Hecker, 1968a and Hecker, 1968b) and is the oil’s most irritant and co-carcinogenic compound. TPA is known to strongly activate protein kinase C (PKC; Nishizuka, 1984), which takes a central role in essential intracellular pathways (e.g., Nakamura and Nishizuka, 1994; Ojani et al., 2016).
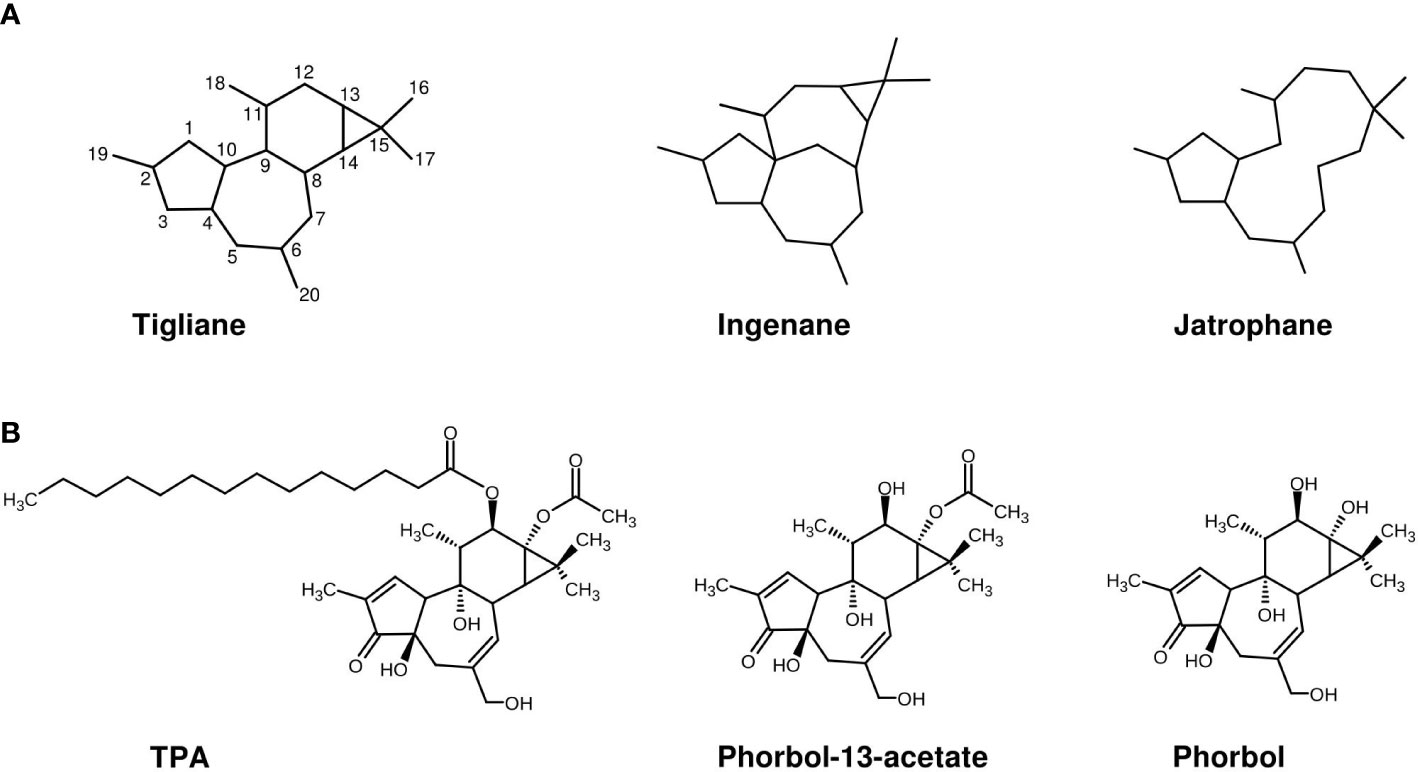
Figure 1 (A) Main structure skeletons of diterpenes from E cyparissias. (B) Representatives of tiglianes: TPA, phorbol-13-acetate, and their parent alcohol phorbol.
An isomer of TPA was reported in E. cyparissias (Ott and Hecker, 1981; see below), which is fed on by H. euphorbiae larvae, despite its toxicity. The food plant’s milky latex was also reported to be irritant to humans (Asilian and Faghihi, 2004). Contrary to the expectation induced by the larval aposematic coloration (Marsh et al., 1984), they do not sequester the toxins from their food plants for chemical protection against predators (Hundsdoerfer et al., 2005). Hundsdoerfer et al. (2005, 2019) studied the sensitivity of Hyles larvae to TPA and postulated that the ability to detoxify this substance represents a plesiomorphic character state in the phylogeny of the genus. Indeed, the study revealed modulated levels of TPA sensitivity depending on the organismic interactions of the species and their food plants in their natural environment. The study demonstrated highest sensitivity to TPA in species that do not use Euphorbia as food plants. Hyles species that include Euphorbia in their food plant spectrum, either as specialists or as part of a polyphagous feeding strategy, showed lowest mortalities and thus insensitivity to TPA. To date, it is not known how H. euphorbiae detoxifies TPA and toxic plant diterpenes. To pave the way for further understanding of their ecological strategy, we are highly interested in the diterpene compounds that H. euphorbiae encounter in their food plant.
A broad number of reviews was published in recent years about diterpenes occurring in the Euphorbiaceae family in general and the Euphorbia genus in particular (Shi et al., 2008; Vasas and Hohmann, 2014; Wang et al., 2015; Xu et al., 2021). One purpose of many studies was not only the interest in natural products for drug discovery, but also the protection of human health against their co-carcinogenicity (Hecker, 1977; Goel et al., 2007; Vasas et al., 2012). Although E. cyparissias was reported to be a diterpene-rich species (Vasas and Hohmann, 2014; Ernst et al., 2019), only a limited number of studies elucidated specific diterpene compounds in this species. The latest report published isolated two diterpenes based on a jatrophane skeleton, named cyparissins A and B (Lanzotti et al., 2015) from the plant. Before, Ott and Hecker described diterpenes of the ingenol type in E. cyparissias root extract (Hecker, 1977; Ott and Hecker, 1981), namely, diesters of 13-hydroxyingenol and triesters of 13, 19-dihydroxyingenol, which were named by them Euphorbia factors (e.g., Cy6, Cy11, and Cy14). The compound Cy11 (3-O-(2,3-dimethylbutyryl)-13-O-iso-decanoyl-13-hydroxyingenol) was described to be at least as active as TPA and as a structural TPA-isomer but was not disclosed as the same substance (Ott and Hecker, 1981). To our knowledge, this was the first and, to date, the only evidence of a TPA-related compound in E. cyparissias, and therefore, our only basis for the assumption that H. euphorbiae is faced with a compound similar to TPA in nature. Nevertheless, the data in that study show that Cy11 is an ingenane and thus of a clearly different chemical structure than TPA (tigliane), since ingenanes and tiglianes are two diterpenoid classes, which are characterized by unique structural skeletons (Figure 1A).
The tiglianes are based on a 5/7/6/3-tetracyclic ring system consisting of five methyl, five methylene, and nine methine groups, as well as one quaternary carbon (Wang et al., 2015). This skeleton is also the basic structure of phorbol (Figure 1B), a common tigliane diterpene and the parent alcohol of an own tigliane subgroup, called phorbol esters. TPA and most tiglianes commonly known belong to the phorbol esters, but we here refer to those substances with the more general term tiglianes, as it includes a broader number of compounds, such as phorbol itself. The common parent alcohol of ingenanes on the contrary is ingenol, a compound also occurring in Euphorbia plants, but not included in this study. As a consequence of the structural differences, Cy11 and TPA also differ in functional groups, as ingenanes are characterized by a ketone bridge between C-8 and C-10 (Vasas and Hohmann, 2014), whereas TPA holds a hydroxyl group at C-9 (see Figure 1A). Moreover, Cy11 and TPA are esterified partly at different positions (Cy11 oppositely at C-3 and C-13, whereas TPA at C-12 and C-13 are adjoined) and with side chains of different lengths (esterification of Cy11 with 10-carbon-long decanoic acid and of TPA with 14-carbon-long myristic/tetradecanoic acid at C-12; both have acetic acid at C-13) leading to a different spatial arrangement and extent of both compounds. Whether the structural differences between TPA and Cy11 lead to different detoxification strategies in Hyles is unknown to us, but appears possible. For this reason, and since neither TPA nor another tigliane was reported for E. cyparissias to date, our first aim was the re-analyses of this plant species’ diterpene spectrum, regarding the presence or absence of TPA or other tiglianes.
The occurrence of such secondary metabolite compounds can vary within plants depending on their function (e.g., Harborne and Held, 1995; Borges et al., 2017). Moreover, distribution of defense compounds in the plant can change as reaction to herbivore feeding. It was further described that the high amount of diterpene diversity for Euphorbia species is associated with Euphorbia-feeding larvae (Ernst et al., 2019). We thus additionally investigate the effect that this herbivore treatment, in the form of feeding H. euphorbiae larvae, would have on plants of E. cyparissias. The three plant parts, leaves, stem, and root, experience a different exposition to herbivore stress and function within the plant system and thus need to be studied separately. Our second aim was, thus, to study the occurrence of TPA and other tiglianes in different parts of E. cyparissias plants and to investigate if the plant produces those compounds only upon herbivore stress or whether they can be found in different (expectedly higher) amounts.
The feeding experiments by Hundsdoerfer et al. (2019) are based on the finding by Hundsdoerfer et al. (2005) that TPA is 70%–90% metabolized by the larvae, whereas 10%–30% was recovered in the feces untransformed. For the experimental setup, toxic tigliane TPA as a model standard compound was chosen, due to its well-investigated biological activity, but most importantly because of its availability as a commercial standard. Only few tiglianes can be purchased as standard compounds and many of them are used in the work at hand. Although phorbol ester sequestration was reported previously for Pachycoris klugii (Wink et al., 2000), neither previous study analyzed the disposition of plant-derived tigliane diterpenes in insect frass. Our third aim of the work reported here was therefore to broaden the finding of TPA in the frass from artificial feeding experiments to additional analyses of such compounds in the frass from H. euphorbiae larvae feeding on their natural food plant E. cyparissias.
Summarizing, our studies were driven by the motivation to broaden our understanding of tiglianes occurring in E. cyparissias native plants as well as plants exposed to herbivore feeding, and thereby to contribute to elucidating the plant–herbivore interaction in this model system.
Materials and methods
The experiments were conducted at the Senckenberg Natural History Collection Dresden (SNSD; plant and larvae breeding, feeding and herbivore stress experiment, extraction of frass and plant samples) and instrumental analytics at the Max-Planck-Institute for Chemical Ecology Jena (MPI-CE; establishment and development of methodological approach) and at the Technische Universität Dresden (TU Dresden; transfer and optimization of method and analyses of frass and plant samples by LC-MS/MS and LC-QTOF-MS), Germany.
Putative tiglianes in E. cyparissias
Plant samples
Plant samples of E. cyparissias were taken from an Euphorbia food plant garden in the SNSD institute (Supplementary Figure S1); the plants originate from natural habitats within ~100 m vicinity of the garden. Various individuals of E. cyparissias plants were sampled, to gain experimental matrix for method establishment, including one individual plant for conduct of standard addition experiments (June 2020). For consecutive targeted and non-targeted analyses of tiglianes, we additionally sampled an extra set of non-flowering E. cyparissias plants in July 2020 as part of the herbivore stress experiment (see below). Furthermore, two complete plants of E. cyparissias were sampled in early summer 2022 (beginning of June) for verification. For sampling, the entire plants including roots were unearthed carefully—washed and dried if necessary—and immediately frozen at −80°C.
Plant sample preparation and extraction
The entire frozen plants were sectioned into fractions according to the compartments “leaves” (including the part of the stem to which the leaves were attached), “stem” (the part without attached leaves, cut into pieces of about 0.3 cm thickness), and “root” (also cut into pieces of about 0.3 cm) and then prepared by lyophilization (freeze drying). For this, the three plant parts (leaves, stem, and root) were frozen again at −40°C immediately before lyophilization. Subsequently, samples were freeze dried for 24–48 h at −56°C and a target pressure of 0.04 mbar. The dry samples were then ground by a hand mill with a ceramic grinder to a fine powder. Extraction was preferably performed immediately after milling. If this was not possible, the milled plant material was stored at −80°C.
The sample material (50 mg of dry plant powder or caterpillar frass, see below) was extracted in five consecutive steps via liquid extraction by use of Eppendorf tubes equipped with metal beads (3 mm). Work was performed under dark conditions for the samples, as far as possible to prevent degradation by light. In each step, 900 µl of solvent was added—methanol:water (50:50, v/v) in steps 1–3, acetonitrile in steps 4–5. The mixture was shaken strongly in a MixBlock for 10 min and then centrifuged (13 min; 20°C; 9,636 ×g/13,000 rpm in a Thermo pico). The residues were extracted by the consecutive steps and the clear supernatants of each step were combined and centrifuged (2 × 13 min; 20°C; 3,853 × g/4,700 rpm in a Thermo Multifuge 1 S-R with a rotor for 5 ml tubes). In a final concentration step, 1,000 µl of that clear supernatant was evaporated under compressed air flow (without heat and light) until dryness and redissolved again in 250 µl of water/methanol/acetonitrile (15:15:70, v/v/v).
LC-MS/MS (triple-quadrupole) analysis
Analyses of our set of plant samples (see Supplementary Table S1) were performed on a Thermo Scientific Ultimate 3000 high-performance liquid chromatography (LC) system (Waltham, MA, USA) coupled to an API 4000 triple-quadrupole mass spectrometer (MS/MS) (AB Sciex, Framingham, MA, USA). The LC-System was equipped with a vacuum degasser, two binary pumps, a high-pressure gradient mixer and a temperature-controlled column compartment. For the LC method, the following settings were applied: column temperature was held at 40°C and a sample volume of 20 µl was injected. A C-18 reversed-phase core-shell column (Kinetex C18, 100 × 3 mm, 2.6 μm; Phenomenex, Aschaffenburg, Deutschland) was used and a water-acetonitrile gradient at a flow rate of 0.5 ml/min was applied. The constitution of the mobile phases was as follows: mobile phase A: acetonitrile/2 mM ammonium acetate solution/formic acid (3/97/0.05, v/v/v); mobile phase B: acetonitrile/2 mM ammonium acetate solution (95/5, v/v). The gradient was as follows: 0–1.6 min 0% (v/v) solvent B; 1.6–4.0 min linear gradient to 80% (v/v) B; 4.0–14.5 min linear gradient to 95% (v/v) solvent B; 14.5–14.6 min solvent B was raised to 100% (v/v) and held at 100% (v/v) B until 15.0 min; 15.0–15.1 a steep gradient of solvent B to 0% (v/v) and hold at 0% (v/v) B until 18.0 min.
The mass spectrometer was equipped with an electrospray ion source operated in negative mode. Detection parameters for each analyte were optimized separately by infusion of a standard solution of 0.1 µg/ml for phorbol-12,13-diacetate and of 1 µg/ml for the other standard compounds (in a mixture of eluent A and B, 50/50, v/v) at a flow rate of 50 μl/min and using an infusion pump. General parameters were fixed as follows: source temperature 450°C, desolvation temperature 400°C, curtain gas flow 30 L/h, and collision gas flow 6 L/h. Nitrogen was used as nebulizer and collision gas. The applied scan modes were multiple reaction monitoring (MRM) and product ion scan. MRM measurements were conducted for identification of tigliane compounds in the biological samples by comparison of retention times and MRM transitions, using the two most intense transitions of the respective standard compound as quantifier and qualifier (see Supplementary Table S2). Standard compounds were dissolved in methanol, combined to a standard mix solution and diluted before injection into the LC-MS/MS (20 µl injection volume). For additional verification of identification and screening for possible matrix effects on detection, standard addition was performed, by spiking leaves and root extracts with standard mix solution (target concentration: 50, 500, and 2,500 ng/ml). To gain further structural information of the detected compounds 2 and 3 in comparison to TPA, product ion scans with precursor ion mass (Q1 target mass) 661 Da were performed. Fragmentation of the other tigliane standard compounds was analyzed by product ion scans with precursor ion mass set to 409 Da (phorbol), 451 Da (phorbol-13-acetate), 493 Da (phorbol-12,13-diacetate), and 435 Da (prostratin). In those MS experiments, Q1 target masses were set to respective formate adduct masses ([M+HCOO]− = Mr + 45 Da), as optimization experiments established that, in our setup, tiglianes are best ionized in their formate adduct form.
Data acquisition, exploration, and quantification were conducted in AnalystTM (AB Sciex, Framingham, MA, USA). Further data processing and visualization were performed in LibreOffice Calc (v 6.0.7.3, The Document Foundation, Berlin, Germany), Microsoft Excel (Microsoft Corporation, Redmond, United States of America), Inkscape (v3; Inkscape Project, 2007), and R Statistical Software (RStudio, v1.2.5033; R Core Team, 2020) using the packages tidyverse (Wickham et al., 2019) and RColorBrewer (Neuwirth, 2022).
LC-QTOF-MS (quadrupole time-of-flight) analysis
For further verification of LC-MS/MS results, high-resolution mass spectrometry analysis of representative plant samples (leaves, stem, and root samples; see Supplementary Table S1) were performed. Samples were analyzed using an Aquity I-class ultra-performance liquid chromatography (LC) system (Waters, Eschborn, Germany) coupled to a high-resolution quadrupole time-of-flight mass spectrometer (QTOF-MS) (Vion IMS QToF, Waters). LC-settings were chosen identically to LC-MS/MS analyses.
The volume of 5 µl of TPA and phorbol-13-acetate standard solution mix was injected into the LC-QTOF-MS to determine analyte-specific characteristics. In addition to a specific retention time (RT), TPA and phorbol-13-acetate were detected by using high-definition data acquisition mode (HDMSE) that includes the determination of an accurate precursor, respective fragment ion mass screening of all ions, and ion mobility separation in conjunction with collision cross section (CCS) data. The observed mass range included mass-to-charge ratios between 50 and 700 Da. Total scan time was set to 0.3, of which 40% of the time a collision energy of 6 eV (low energy) was applied before collision energy was ramped up from 15 to 200 eV (high energy) for the remaining 60% of the time. Repeated injections of a Leucine-Enkephalin solution served as reference for mass correction. For respective data processing, the Waters UNIFI Software package (Version 2.1.2) was used.
Chemicals
The following standard compounds (reference substances) were used for comparisons: 12-O-tetradecanoylphorbol-13-acetate (TPA, also known as phorbol-12-myristate 13-acetate, PMA) was purchased from Alfa Aesar (Thermo Fisher, Kandel, Germany). Phorbol-13-acetate (13-acetylphorbol) and phorbol-12,13-diacetate were bought from Santa Cruz Biotechnology (Dallas & Heidelberg, USA and Germany). Phorbol (4β-Phorbol) was purchased from TRC (Toronto Research Chemicals, Toronto, Canada) and prostratin (12-deoxyphorbol-13-acetate) was bought from Cayman Chemical Company (Michigan, USA). As solvents, methanol (≥99.9% p.a.) from Carl Roth (Karlsruhe, Germany) and acetonitrile (ACN; ≥99.9% HPLC LC-MS grade) from VWR (Darmstadt, Germany) were used. Formic acid (Optima, LC/MS Grade) was obtained from Fisher Scientific (Fisher Chemical; Schwerte, Deutschland) and DMSO (≥99.9%) was purchased from SIGMA (Sigma-Aldrich, Taufkirchen, Germany).
Distribution of tiglianes within the native plant and upon herbivore stress
Herbivore stress experiment
As part of a “herbivore stress” experiment, we sampled a set of E. cyparissias plants that were exposed to feeding by H. euphorbiae larvae in July 2020. The experiment consisted of the placement of one individual 5th instar larva (see below) on one individual non-flowering plant each. Experimental conditions were chosen to correspond to natural conditions; July is the season in which H. euphorbiae larvae can be found on their food plants in Central Europe. The larvae were left to feed under supervision on the plant in the institute food plant garden for 2 h under shaded and humid conditions to avoid stress of the caterpillars. They remained on the same individual plant during the entire experiment. After feeding was interrupted, the entire plant was sampled and stored as described above (see the first section—Putative tiglianes in E. cyparissias—of this part). Three plants treated with this herbivore stress and three non-treated plants (native plants, identical to plant individuals from July 2020 described in the first section of this part) were sampled at the same time. Plants of similar sizes were chosen from the same locality (within 2 m2).
Plant samples of the herbivore stress experiment were prepared, extracted, and analyzed via LC-MS/MS and LC-QTF-MS as described above in the first section of this part (for sample set, see more in Supplementary Table S1), with the exception that herbivore stress samples were extracted and analyzed in duplicates.
Breeding of H. euphorbiae larvae
Hyles euphorbiae larvae from Berbisdorf (Germany) were collected from their food plant E. cyparissias and reared to the adult stage in captivity. The larvae of the F1 generation were reared in the laboratory (in an incubator with long-day conditions: 16.5 h light at 25°C, ramp time of 1 h, 7.5 h darkness at 23°C, see Daneck et al., 2021) on E. segetalis and E. myrsinites (Euphorbiaceae) from the institute Euphorbia food plant garden (Supplementary Figure S1) until the 4th instar and were subsequently used for the experiments after 2–3 days in 5th instar.
Tiglianes in H. euphorbiae frass from feeding experiments with TPA and E. cyparissias
For the feeding experiments with TPA, the 5th instar larvae were kept on an agar-agar-based artificial diet including wheat germ and leaf powder from E. segetalis (recipe was originally developed for larvae of the family Sphingidae with leaf powder sourced from the respective food plants by Harbich, 1994; see also Hundsdoerfer et al., 2019; Table 1 in Hundsdoerfer et al., 2005). As part of the series of feeding experiments conducted by Hundsdoerfer et al. (2019), three individuals were fed with artificial diet containing 2 mg of TPA dissolved in DMSO (100 µg TPA/µl). Three additional individuals were fed with E. cyparissias leaves for 2 h in between feeding on the diet. All frass samples were collected in the time interval between 4 and 6 h after start of the feeding. This time interval was shown in own previous studies (unpublished) to be the one with highest content of excreted TPA. After sampling, frass samples were stored at –80°C until further analyses.
Frass samples were extracted and analyzed via LC-MS/MS and LC-QTOF-MS (sample set see more in Supplementary Table S1) in the same way as described above for the plant samples.
Results
Phorbol-13-acetate and two putative tiglianes in E. cyparissias
We investigated the plant extracts of E. cyparissias via LC-MS/MS and LC-QTOF-MS for the presence of tiglianes through comparative analyses with five commercially available tigliane standard compounds (TPA, phorbol-13-acetate, phorbol-12,13-diacetate, phorbol, prostratin). By means of the two instruments, we identified phorbol-13-acetate (Figure 1B) in E. cyparissias root extracts. In LC-MS/MS, the identification of the underlying peak (peak 1; Figure 2) was based on mass spectrometry-derived signals (same quantifier and qualifier MRM transitions, see Supplementary Table S2) and consistent retention times (phorbol-13-acetate standard: 4.73 min, peak 1: 4.76 min). Additional verification was performed there via standard addition measurements, confirming similarity of retention times (see Supplementary Figure S2). In LC-QTOF-MS, identification was reinforced by determination of the same exact mass (expected mass: 406.1991 Da, median observed m/z of signal 1: 406.1988) and the same CCS values (expected CCS: 207.06 Å2, median observed CCS of signal 1: 205.16 Å2) compared to phorbol-13-acetate standard compound, with mass errors below ±2.0 ppm and CCS delta values below ±2%, as well as a median retention time error of 0.01 min (for detailed information, see Supplementary Table S2).
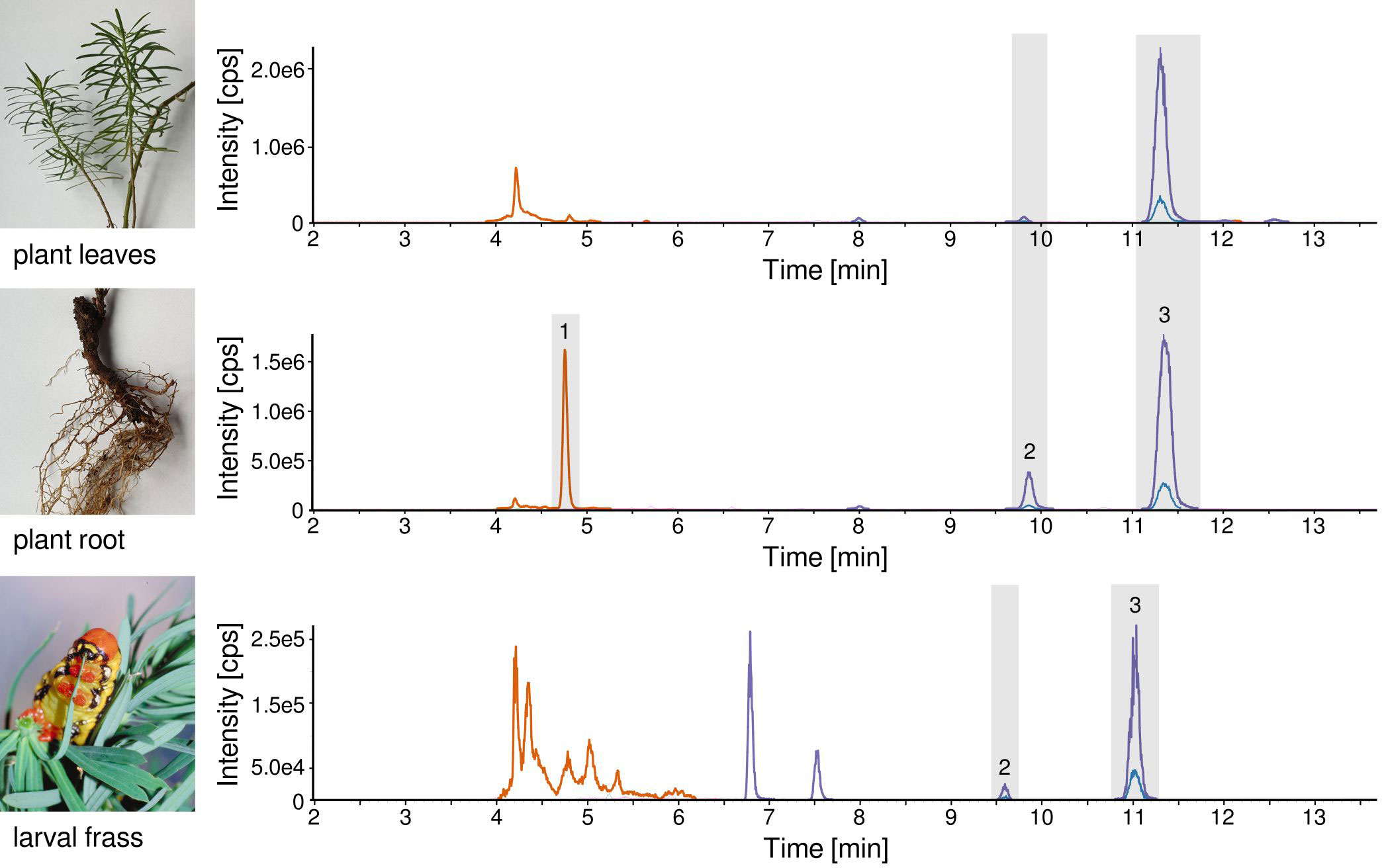
Figure 2 LC-MS/MS chromatograms (MRM transitions of TPA and phorbol-13-acetate) of the sample extracts with peaks 1 to 3 of the detected compounds of interest; top: E. cyparissias leaves, middle: E. cyparissias root, bottom: H. euphorbiae larval frass after feeding on E. cyparissias leaves. A retention time shift of 0.3 min is present between the plant and frass chromatograms as they have been measured at separate weeks.
None of the other analyzed standard compounds could be detected in the plant samples. This reveals that no proof of presence of TPA in early summer E. cyparissias was found. Nevertheless, driven by our question if caterpillars are faced with TPA or, in its absence, with related compounds, when feeding on E. cyparissias, we broadened our search to compounds with similar analytical characteristics as TPA (e.g., same accurate mass, similarities in fragmentation spectra). Respective LC-MS/MS and LC-QTOF-MS analyses revealed two compounds of interest, named here compounds 2 and 3 (see Figure 2). Of both compounds, compound 3 drew most of our attention, as it is represented by the most prominent peak in chromatograms captured by both instruments (retention times LC-MS/MS: 11.32 min, LC-QTOF-MS: 8.88 min) and the retention time of compound 3 was close to the retention time of TPA (LC-MS/MS: 11.09 min, LC-QTOF-MS: 8.71 min). Nevertheless, with a retention time shift of 0.23 min, the two compounds did not elute at the same time, which was also demonstrated in the consecutive standard addition experiments in LC-MS/MS (Figure 3). The second compound of interest, compound 2 is represented by a rather small peak in the chromatograms (peak 2 in Figure 2; retention times LC-MS/MS: 9.82 min, LC-QTOF-MS: 7.66 min), but is still a characteristic signal of the E. cyparissias root extracts.
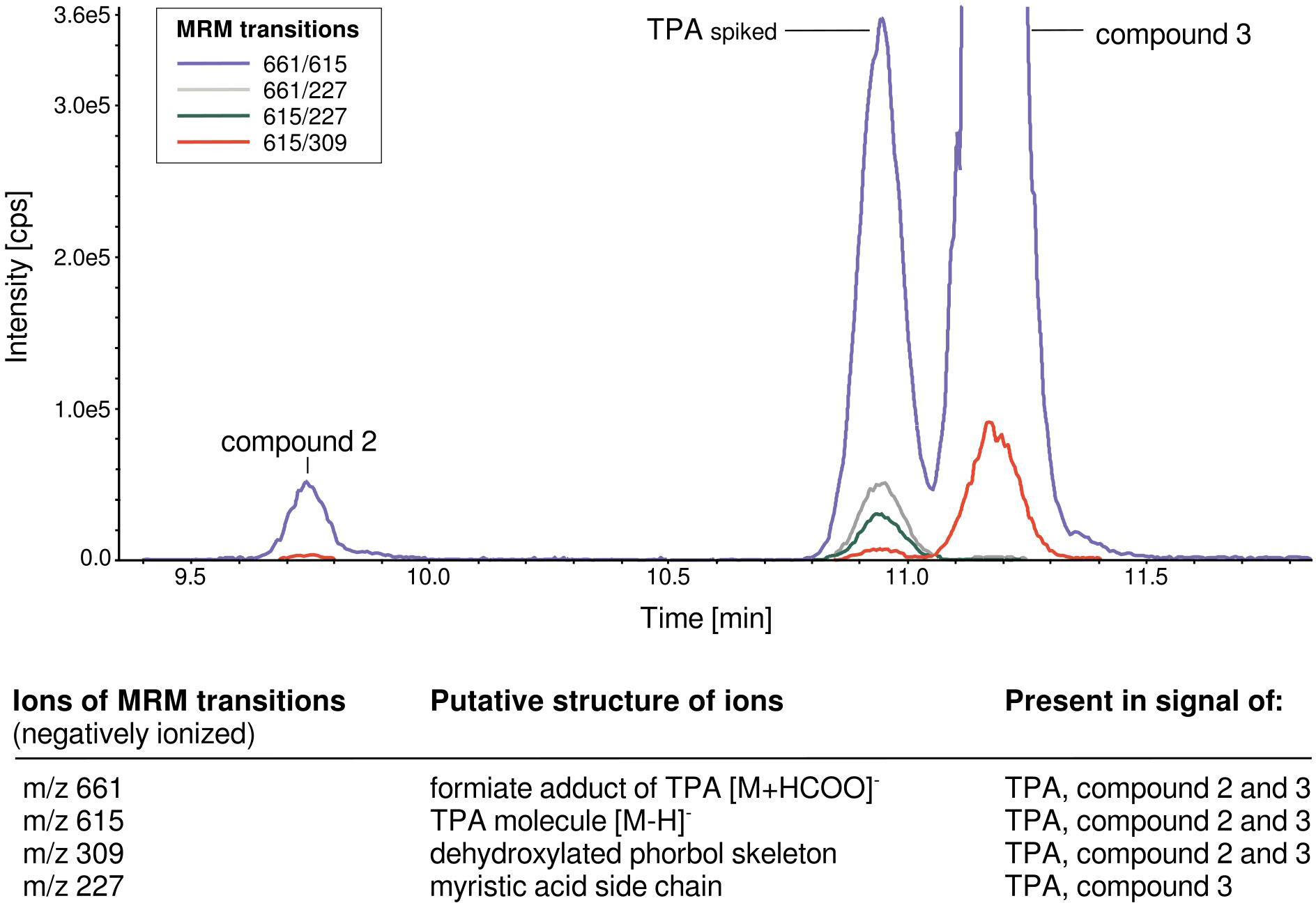
Figure 3 LC-MS/MS standard addition chromatogram of E. cyparissias root extract spiked with TPA standard solution (concentration of standard compound in extract: 500 ng/ml) in multiple reaction monitoring mode (MRM) with MRMs of TPA, including description of MRM transition signal masses.
Both compounds showed interesting analytical characteristics. In LC-QTOF-MS accurate mass screening, the detected neutral mass of the unknown compounds 2 and 3 was the same as that of TPA, with a mass error < 2.0 ppm (expected mass: 616.3975 Da, median observed m/z of compound 2: 616.3980, compound 3: 616.3976; for detailed information, see Supplementary Table S2). It can be assumed that compounds 2 and 3 have the same elemental formula as TPA (C36H56O8), and are thus isomers. Additionally, a high-energy mass spectrum of standard compound TPA was acquired, including fragmentation patterns. As LC-QTOF-MS analyses allow high-resolution measurements of the observed fragment ion masses, theoretical chemical structures could be assigned by the software to the theoretical fragments of TPA (Supplementary Data S2). As expected, theoretical fragments of the phorbol skeleton (observed m/z 327.1602) and its cleavage products (observed m/z 309.1490, m/z 291.1388, and m/z 279.1395) were determined. High-energy mass spectra with theoretical fragmentation patterns were also determined for compounds 2 and 3, with the aim of comparing theoretical fragments to those determined for TPA. At first sight, the mass spectra of both compounds show clear differences to the one determined for TPA. However, some theoretical key fragments of TPA are also present in the high-energy mass spectra of compounds 2 and 3 (see Supplementary Data S2). In particular, the fragment masses of the theoretical main phorbol skeleton (observed m/z 327.1602) were present in both compound 2 and compound 3 mass spectra, while two masses of phorbol cleavage products (observed m/z 309.1490 and m/z 279.1395) were also present in the compound 3 mass spectra.
The results of LC-QTOF-MS were substantiated by LC-MS/MS analyses. The signals of both compounds are formed by the same MRM transitions as determined for TPA (see Figure 3). The underlying masses of the MRM transitions can be assigned to the TPA molecule ([M-H]− = 615) and the formate adduct product ([M+HCOO]− = 661). Additionally, the mass 309, which we know from LC-QTOF-MS analyses as the theoretical phorbol skeleton mass, is present in TPA and compound 2 and 3 MRM transition masses. To gain further structural insight, additional product ion scan measurements (of the respective [M+HCOO]− precursor ion, see methods) were performed, to obtain LC-MS/MS fragmentation mass spectra. We expected to find mass signals of the phorbol skeleton in the LC-MS/MS mass spectra of the tigliane standard compounds. This hypothesis was confirmed as the masses m/z 327, 309, 279, and additionally 291 were present. Furthermore, we observed that the LC-MS/MS mass spectra of compounds 2 and 3 were also characterized by these specific four masses (see Supplementary Figures S3–5).
Distribution of tiglianes within the native plant and upon herbivore stress
To understand where phorbol-13-acetate and compounds 2 and 3 are located in E. cyparissias plants, we analyzed their occurrence in the single plant parts leaves, stem, and root via LC-QTOF-MS (verification of occurrence in the respective plant parts) and LC-MS/MS (determination of peak areas). All three compounds exhibited a different pattern of distribution in the plants (see Figure 4A). Whereas phorbol-13-acetate was only detected in the root samples, and not in leaves and stem (see Figure 2; Supplementary Figure S6), compounds 2 and 3 were present in all parts of the plant (Figure 4A). Compound 2 was detected with considerably higher peak areas in the root fractions than in leaves and stem. For compound 3, no clear differences in occurrence between the three plants parts were found.
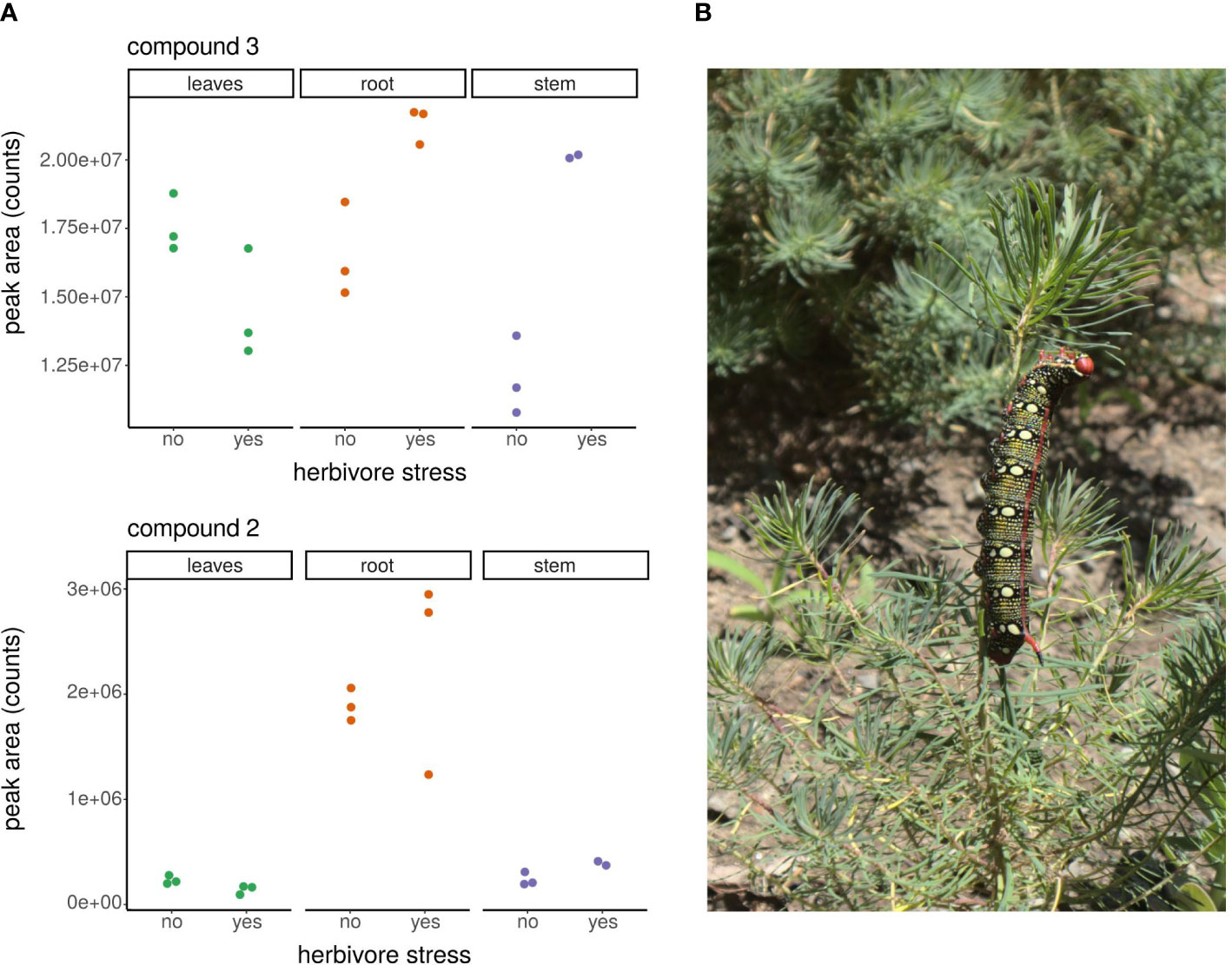
Figure 4 (A) Occurrence of the reported compounds 2 and 3 in the plant parts of E. cyparissias, with (yes) and without (no) herbivore stress. Single points represent the averages of duplicate measurements for one individual plant each. (B) A 5th instar larva of H. euphorbiae feeding on E. cyparissias during the herbivore stress experiment (for photographic recording, the light protection and the insect cage from the experimental setup were removed).
Our data of the plants, which were exposed to Hyles feeding, show that the even distribution of compound 3 changes upon herbivore stress. A clear increase in occurrence of the substance in the stem and a slight increase in the root was induced, as the peak area ranges of non-stressed plants do not overlap with those of the stressed plants (see Figure 4A). However, for compound 2 and phorbol-13-acetate, no clear differences upon herbivore stress were observed (Figure 4A; Supplementary Figure S6). Moreover, we screened the samples for additional occurrence of the other tigliane standard compounds and TPA-related compounds, as production of those substances might be induced by herbivore stress, but such substances, being only present in stressed but not in native plants, were not found.
Tiglianes in H. euphorbiae frass from feeding experiments with TPA and E. cyparissias
As a third methodological approach, we investigated the frass from 5th instar H. euphorbiae larvae feeding either on E. cyparissias leaves or on artificial diet containing TPA for the presence of the plant-derived compounds 2 and 3, as well as of phorbol-13-acetate and TPA. Both LC-MS/MS and LC-QTOF-MS analyses demonstrated compounds 2 and 3 to be present in frass samples from all leaf-fed larvae. Furthermore, measurements using both instrumental approaches showed no signal of phorbol-13-acetate in the frass from leaf-fed insects (see Figure 1; Supplementary Tables S2, S3).
As expected, TPA was detected in the frass samples of larvae that had fed on artificial diet spiked with TPA (see Supplementary Figure S7 and Supplementary Table S2). However, compounds 2 and 3 were not detected as possible TPA by-products in these frass samples.
Discussion
Phorbol-13-acetate and putative tiglianes in E. cyparissias
Phorbol-13-acetate and two compounds of unknown structure but with TPA-related mass spectrometric characteristics, named compound 2 and 3, were detected in E. cyparissias plant extracts. Identification of phorbol-13-acetate was verified by both LC-MS/MS including standard addition and LC-QTOF-MS analyses (see Results).
Additionally, our data provide evidence of a phorbol skeleton of both compounds 2 and 3, due to the detection of mass spectrometric masses, which are assigned to theoretical fragments of phorbol, the parent alcohol of the phorbol esters, a subgroup of the tiglianes (e.g., Wang et al., 2015). These fragment masses of the phorbol skeleton not only were detected by us (see Results) but also had already been reported for phorbol esters (tiglianes) of Croton tiglium and Jatropha curcas and were applied in their detection via LC-MS/MS (although in positive ionization mode and therefore with respective mass values, e.g., m/z 311 in positive mode instead of here reported m/z 309 in negative mode; Vogg et al., 1999; Ichihashi et al., 2010; Punsuvon et al., 2012; Insanu, 2014). Through this classification as a phorbol, and by the definition of a tigliane skeleton, we hypothesize that compounds 2 and 3 are tiglianes. The identification of the specific chemical structures of both compounds will be the purpose of further studies to be conducted, including 1H-NMR analyses. Until final data of their structural identity are present, we intermediately refer to compounds 2 and 3 as putative tiglianes.
To our knowledge, this is the first time that phorbol-13-acetate and tiglianes in general are reported for E. cyparissias. Before, only diterpenes of the ingenane and jatrophane type were described for this species (Ott and Hecker, 1981; Lanzotti et al., 2015). The ingenanes, jatrophanes, and tiglianes are characterized by different structure skeletons, with different parent alcohols each. From those three diterpenoid classes, only the tiglianes are based on a 5/7/6/3-tetracyclic ring system, which is also the basic structure of phorbol (see Figure 1). Considering previous studies (Vasas et al., 2012; Ernst et al., 2019) promoting E. cyparissias as a diterpene-rich species, the additional occurrence of the diterpene class of the tiglianes in E. cyparissias is not unexpected. A broad number of tiglianes was already identified in the family of Euphorbiaceae, including numerous reports in plants of the genus Euphorbia (Shi et al., 2008; Vasas and Hohmann, 2014; Wang et al., 2015). Correspondingly, the tigliane phorbol-13-acetate had also been reported before for other species of the genus, namely, in E. fischeriana (roots; Wang et al., 2017) and in E. tirucalli (aerial parts; Weng et al., 2022). In addition, it was described in croton oil (Croton tiglium, Euphorbiaceae; Marshall and Kinghorn, 1984). Further steps to identify further tiglianes in E. cyparissias are thus promising.
Moreover, our data demonstrate both compounds 2 and 3 to be isomers of TPA, whereby we clearly exclude that compound 2 or 3 is identical with TPA (due to different retention times and different fragmentation patterns, see Results). The presence of another TPA isomer in E. cyparissias root [c.f. 3-O-(2,3-dimethylbutyryl)-13-O-iso-decanoyl-13-hydroxyingenol, named Euphorbia factor Cy11] was described before by Ott and Hecker, 1981. However, Cy11 is an ingenane and thus of a different structure as the TPA isomer compounds 2 and 3 reported here (for comparison, see Figure 1, Introduction, and Ott and Hecker, 1981, page 90).
Distribution of tiglianes within the native plant and upon herbivore stress
Since we expect the amount of secondary plant metabolites to vary within the plant (e.g., Harborne and Held, 1995; Borges et al., 2017), we explored the distribution of the tigliane plant compounds reported here in the respective plant parts of summer season E. cyparissias. Through LC-MS/MS standard addition experiments, we showed that comparison of peak areas in the three different matrices (plant parts) is possible, although limited, as differences of matrix effects were between 20% and 40%. Comparison of the influence of herbivore stress can be carried out without limitations, as it is evaluated for each plant part (matrix) separately. From the stem samples of the stressed plants, only data from two individuals (instead of three) could be evaluated, as the amount of stem material of one stressed E. cyparissias was too low for comparable analyses (E. cyparissias stems are very thin and light weight, as can be seen in Figure 4B). We thus refer to the findings reported here as first insights, which will be corroborated and tested by further studies to follow.
Despite the limitations of the experimental setup, we clearly found phorbol-13-acetate and compound 2 to be mainly present in the roots, irrespective of herbivore treatment. The detection of phorbol-13-acetate only in the root, which was further verified by LC-QTOF-MS analyses, could have several explanations. One would be to protect the roots from general herbivore attack, although this specific species H. euphorbiae does not feed on the root, but there are others, such as rodents, that feed on plant roots. It is also possible that phorbol-13-acetate is stored in the root and transported for protection to other plant parts when herbivory takes place, since phorbol-13-acetate is known to be acute toxic to humans (fatal if swallowed, in contact with skin, and inhaled; National Center for Biotechnology Information, 2023) and was described in the literature as a protein kinase C activator (Bessa et al., 2018), just like TPA (Nishizuka, 1984). Although we did not observe this effect of mobilization through herbivore stress, it could take place under different circumstances than applied in our experimental setup (see below). An additional possible ecological role could be that this compound is a precursor to other tiglianes in the plant. Longer fatty acid chains such as the myristic acid side chain in TPA would be added after synthesis of the main skeletal tigliane structure and acetylation of C-13. Transcriptomics has shown phorbol ester production (in this case of 12-deoxyphorbol-13-acetate, commonly known as prostratin) to take place in the roots of E. fischeriana plants (Barrero et al., 2011). The finding of phorbol esters primarily in roots corresponds to findings of other studies (e.g., Ernst et al., 2019 for E. horrida and E. milii var. hislopii). It is very likely that phorbol ester production and storage also occurs in the roots of E. cyparissias and in this case holds for phorbol-13-acetate. Additional analyses (e.g., transcriptomics) would be necessary to verify the locality of production.
The interpretation of why compound 2 occurs in all parts of the plants but in clearly higher amounts in root samples would be similar, in that we suspect it to be primarily produced and stored in the roots. However, since diterpenoids can also be produced in other plant parts such as young stems in the European Euphorbia subgenus Esula (e.g., E. lathyris; Ernst et al., 2019) and toxic defense compounds are known to be located in the latex of milky sap-containing plants (Harborne and Held, 1995), the localization of diterpenoid production certainly needs further study. Despite not knowing anything about its toxicity, the low amounts of compound 2 in stem and leaves could theoretically provide a first immediate defense against herbivore stress.
Our expectation about the effect of herbivore feeding on the tigliane distribution in E. cyparissias was to find that some substances are only present after herbivore treatment and that amounts of others are increased (c.f. Harborne and Held, 1995; Borges et al., 2017). With rising herbivore stress, further toxic compounds would become mobilized. The first expectation was not met, since we did not encounter new tigliane or TPA-like substances in the herbivore-treated plants only. One reason for this could be that as a specialist, these hawkmoth larvae do not produce particular herbivory cues. Plants exhibit a reduced induction as a response to specialists if these sequester the toxins, as defense may only be indirect in that low toxin levels lead to low protection of the herbivore (Ali and Agrawal, 2012). Another reason could be as simple as not having been able to detect unexpected new compounds with the method applied. Also, in future experiments, the growth conditions will be varied, as well as time of induction, the number of larvae and plants will be increased, and the possible sequestration will be revisited.
We can, however, report observations meeting the latter expectation: compound 3 was measured to occur in higher amounts in parts of the plants upon exposure to larval leaves feeding, as it clearly increases in the stem and slightly in the root extracts analyzed. This finding suggests that compound 3 is indeed produced by the plants as a defense compound. It was detected in all parts of E. cyparissias; thus, the insect is confronted with the compound directly when starting to feed on the plant. We thus hypothesize compound 3 to be toxic. Although H. euphorbiae preferably feeds on the leaves and not on the roots, as they are not reachable for the larvae in nature, compound 3 could be produced by the plant with a generalistic defense purpose. Amounts in the roots would rise due to induced production for further mobilization within the plant and, e.g., amounts in the leaves would rise during longer feeding. Additional experiments have to be carried out, to verify our first observations and additionally characterize its biological activity beside its structure.
The experiments conducted here did not reveal an influence of herbivore feeding on the distribution of phorbol-13-acetate and compound 2. This could have several explanations, one being that the herbivore stress time, i.e., the time the larva was allowed to feed on plants before they were analyzed (in this case 2 h), was not sufficient. It is also possible that tigliane diterpenes produced in the root would be transported to the stem and leaves later on (Engelberth, 2015). An extension of the herbivore stress experiment to corroborate the effects observed would be to apply different time intervals of induced feeding on the plant. Finally, it also cannot be excluded that the compounds reported here are produced by E. cyparissias for other reasons, such as modulating numerous PKC cascades, since the interaction of phorbol ester with PKC affects activities of several enzymes, biosynthesis of protein, DNA, polyamines, cell differentiation processes, and gene expression (Goel et al., 2007). Our first experimental observations open a large field for further studies.
Tiglianes in H. euphorbiae frass from feeding experiments with TPA and E. cyparissias
As a last step, the occurrence of the here reported plant-derived tiglianes and standard compound TPA in the frass from H. euphorbiae larvae feeding on either E. cyparissias or TPA-containing artificial diet was explored. Phorbol-13-acetate was not detected in the frass from leaf-fed insects, which is not surprising, since this compound only occurs in the root, whereas the larvae feed on the leaves. Nevertheless, we cannot exclude that the larvae might need to cope with this compound when feeding on the plant for a longer period than investigated. Our analyses show that both putative tiglianes found in E. cyparissias leaves—compounds 2 and 3—are excreted in the larval frass after consumption. Thus, the putative tiglianes are not fully metabolized by the larvae. The same has previously been shown for TPA (Hundsdoerfer et al., 2005). However, the laboratory experimental setup was rather artificial, since the standard compound TPA had not been demonstrated to have occurred in the food plant E. cyparissias before. To date, the only description of a TPA-related compound in E. cyparissias was the TPA isomer Cy11 from Ott and Hecker, 1981, but this ingenane has a different skeletal structure to TPA and thus possibly deviant detoxification paths (see Introduction). Here, we show that additional putative tiglianes related to TPA are not only found as secondary metabolites in the food plant E. cyparissias but can also be detected in the herbivore frass. More specifically, the two TPA isomers compound 2 and 3 are very probably based on a tigliane skeleton, just like TPA, and are thus chemically much more similar to TPA than Cy11 of the ingenane type.
We firstly show that putative tiglianes and phorbol-13-acetate, being structurally very close to TPA (except for the missing myristic acid side chain, see Figure 1A), occur in E. cyparissias. We also demonstrate leaf-originating substances to be excreted in the frass of H. euphorbiae, just like TPA in the artificial feeding experiments. These findings support the experimental setup of TPA detoxification studies (Hundsdoerfer et al., 2005; Hundsdoerfer et al., 2019) to be close to the natural plant-herbivore interaction and thus to hold in a broader setting, than could only be assumed previously.
Conclusions and outlook
We found out the following:
1. The tigliane phorbol-13-acetate was identified in E. cyparissias. Additionally, two compounds of yet unknown structure, compounds 2 and 3, were detected in the plants. Our data support the assignment of both compounds as tiglianes and are therefore referred to by us as “putative tiglianes”.
2. First experiments indicate that phorbol-13-acetate only occurs in the roots of summer season E. cyparissias plants. A mobilization of the compound to other plant parts due to herbivore feeding was not observed, but cannot be excluded. Compounds 2 and 3 were additionally detected in stem and leaves of the plant. Herbivore feeding by H. euphorbiae increased the occurrence of compound 3 in the stem and slightly in the root.
3. We show that the putative tiglianes—compounds 2 and 3—are excreted by the larvae after feeding on E. cyparissias leaves, just like TPA in artificial feeding experiments, supporting the idea that the results of these can be transferred to understand the mechanisms in the natural setting.
The results reported here pave the way for further analyses towards the understanding of the hawkmoth adaption strategies to food plant defense compounds. Numerous questions remain and larger experiments will be undertaken to strengthen the hypotheses put forward. We plan to study whether, how, and to what extent the plant-derived tiglianes are metabolized by the larvae upon ingestion and metabolization products are excreted. Last but not least, the structure elucidation of compounds 2 and 3 plus possible tigliane metabolites remains a challenge to be met.
Data availability statement
The original contributions presented in this study are included in the article/Supplementary Material. Further inquiries can be directed to the corresponding authors.
Author contributions
The authors contributed in the following manner: conceptualization (SF and AH). Project direction and supervision (AH and DV); and funding acquisition (AH, SF, and DV). SF planned, organized, and evaluated analyses. ML performed, supervised, and evaluated LC-QTOF-MS analyses. RO performed and supervised LC-MS/MS analyses and evaluation. JN performed sample preparation. SF, JN, and AH conducted rearing of hawkmoths and larvae, performed herbivore stress experiment, and sampled plants. LC-MS/MS methods were developed by SF, RO, DV, AH, and JN. The LC-MS/MS method was transferred to LC-QTOF-MS by ML. JG advised scientific methodology. SF and AH drafted the manuscript with input from ML, DV, RO, and JG. All authors contributed to the article and approved the submitted version.
Funding
The Deutsche Forschungsgemeinschaft (DFG) financed the main part of the study (grant number HU1561/7-1) based on preparatory works financed in 2018 by the “Sächsische Aufbaubank—Förderbank” of the “Sächsisches Staatsministerium für Wissenschaft und Kunst” (SAB Antragsnr. 100356414) and the Max Planck Society.
Acknowledgments
We thank Dr. Mirko Peitzsch (TU Dresden, University Hospital Carl Gustav Carus, Institute of Clinical Chemistry and Laboratory Medicine, Facility of Experimental Mass Spectrometry, Germany) for making instruments for analyses available and providing valuable suggestions to the methods, interpretation of results, and the manuscript, as well as Denise Kaden from the same institute for technical support. Special thanks go to Dr. Michael Reichelt (Max Planck Institute for Chemical Ecology, Department of Biochemistry, Germany) for essential help in method development, as well as Bettina Raguschke and Dr. Bhawana Israni from the same institute for technical and scientific support. From the Museum of Zoology (Senckenberg Natural History Collections Dresden, Germany), we thank Dr. Hana Daneck, Johanna Bode, Woorin Kim, Dr. Eduardo Marabuto, and Anja Rauh for technical assistance and help with rearing the larvae and hawkmoth in captivity. We are grateful to Nancy Engel for technical assistance and Dr. Annemarie Lippert (both TU Dresden, Faculty of Medicine, Institute for Clinical Pharmacology, Germany) for scientific advice. Thanks also go to Prof. Dr. Jutta Ludwig-Müller (TU Dresden, Faculty of Biology, Institute of Botany, Germany) for general scientific advice. Last but not least, we greatly thank Prof. emer. Dr. Michael Wink (University of Heidelberg, Institute of Pharmacology and Molecular Biotechnology, Germany) for full scientific and financial support during the initial experimental steps on this topic (2000–2005) and for encouraging this very interdisciplinary approach.
Conflict of interest
The authors declare that the research was conducted in the absence of any commercial or financial relationships that could be construed as a potential conflict of interest.
Publisher’s note
All claims expressed in this article are solely those of the authors and do not necessarily represent those of their affiliated organizations, or those of the publisher, the editors and the reviewers. Any product that may be evaluated in this article, or claim that may be made by its manufacturer, is not guaranteed or endorsed by the publisher.
Supplementary material
The Supplementary Material for this article can be found online at: https://www.frontiersin.org/articles/10.3389/fevo.2023.1197194/full#supplementary-material
References
Abdelgaleil S. A. M., El-Aswad A. F., Nakatani M. (2002). ). molluscicidal and anti-feedant activities of diterpenes from Euphorbia paralias. L. Pest Manage. Sci. 58 (5), 479–482. doi: 10.1002/ps.487
Ali J. G., Agrawal A. A. (2012). Specialist versus generalist insect herbivores and plant defense. Trends Plant Sci. 17 (5), 293–302. doi: 10.1016/j.tplants.2012.02.006
Asilian A., Faghihi G. (2004). Severe irritant contact dermatitis from cypress spurge. Contact Dermatitis 51, 37–39. doi: 10.1111/j.0105-1873.2004.0378e.x
Barrero R. A., Chapman B., Yang Y., Moolhuijzen P., Keeble-Gagnère G., Zhang N., et al. (2011). De novo assembly of Euphorbia fischeriana root transcriptome identifies prostratin pathway related genes. BMC Genomics 12, 1–14. doi: 10.1186/1471-2164-12-600
Bessa C., Soares J., Raimundo L., Loureiro J. B., Gomes C., Reis F., et al. (2018). Discovery of a small-molecule protein kinase cδ-selective activator with promising application in colon cancer therapy. Cell Death Dis. 9 (2), 23. doi: 10.1038/s41419-017-0154-9
Borges C. V., Minatel I. O., Gomez-Gomez H. A., Lima, G. P. P. (2017). "Medicinal Plants: Influence of Environmental Factors on the Content of Secondary Metabolites." in Medicinal Plants and Environmental Challenges, ed. M. Ghorbanpour and A. Varma (Cham: Springer). 259-277. doi: 10.1007/978-3-319-68717-9_15
Daneck H., Barth M. B., Geck M., Hundsdoerfer A. K. (2021). Super cooling point phenotypes and cold resistance in Hyles euphorbiae hawk moths from different climate zones. Diversity 13, 207. doi: 10.3390/d13050207
Engelberth J. (2015). “23: biotic interactions,” in plant physiology and development. Eds. Taiz L., Zeiger E., Møller I. M., Murphy A. (Sunderland: Sinauer Associates, Incorporated, Publishers), 693–729.
Ernst M., Nothias L. F., van der Hooft J. J., Silva R. R., Saslis-Lagoudakis C. H., Grace O. M., et al. (2019). Assessing specialized metabolite diversity in the cosmopolitan plant genus Euphorbia l. Front. Plant Sci. 10. doi: 10.3389/fpls.2019.00846
Goel G., Makkar H., Francis G., Becker K. (2007). Phorbol esters: structure, biological activity, and toxicity in animals. Int. J. Toxicol. 26, 279–288. doi: 10.1080/10915810701464641
Harbich H. (1994). Erfahrungen bei der aufzucht von sphingidenraupen mit einem kombinationsfutter (Lepidoptera: sphingidae). Entomol Z 104, 112–117.
Harborne J. B., Held A. (1995). Ökologische biochemie: eine einführung (Heidelberg, Berlin, Oxford: Spektrum Akademischer Verlag).
Hecker E. (1968a). Cocarcenogeneic principles from the seed oil of Croton tiglium and from other euphorbiaceae. Cancer Res. 28, 2338–2349.
Hecker E. (1977). New toxic irritant and cocarcinogenic diterpene esters from euphorbiaceae and from thymelaeaceae. Pure Appl. Chem. 49, 1423–1431. doi: 10.1351/pac197749091423
Hundsdoerfer A. K., Buchwalder K., O’Neill M. A., Dobler S. (2019). Chemical ecology traits in an adaptive radiation: TPA-sensitivity and detoxification in Hyles and Hippotion (Sphingidae, Lepidoptera) larvae. Chemoecology 29 (1), 35–47. doi: 10.1007/s00049-018-0274-4
Hundsdoerfer A. K., Mende M. B., Harbich H., Pittaway A. R., Kitching I. J. (2011). Larval pattern morphotypes in the Western palaearctic Hyles euphorbiae complex (Lepidoptera: sphingidae: macroglossinae). Insect Systematics Evol. 42 (1), 41–86.
Hundsdoerfer A. K., Tshibangu J. N., Wetterauer B., Wink M. (2005). Sequestration of phorbol esters by aposematic larvae of Hyles euphorbiae (Lepidoptera: sphingidae)? Chemoecology 15, 261–267. doi: 10.1007/s00049-005-0321-9
Ichihashi K., Yuki D., Kurokawa H., Igarashi A., Yajima T., Fujiwara M., et al. (2010). Dynamic analysis of phorbol esters in the manufacturing process of fatty acid methyl esters from Jatropha curcas seed oil. J. Am. Oil Chem. Soc. 88, 851–861. doi: 10.1007/s11746-010-1741-4
Insanu M. (2014) Rational use of jatropha curcas l. in food and medicine. from toxicity problems to safe applications (University of Groningen)
Lanzotti V., Barile E., Scambia G., Ferlini C. (2015). Cyparissins a and b, jatrophane diterpenes from euphorbia cyparissias as pgp inhibitors and cytotoxic agents against ovarian cancer cell lines. Fitoterapia 104, 75-79. doi: 10.1016/j.fitote.2015.05.012
Manojlovic B., Keresi T. (1997). Previous studies of phytophagous insects for biological control of plants from genus Euphorbia l. (Euphorbiales: euphorbiaceae j. st. hill.). Plant Prot. 48, 23–48.
Marsh N., Rothschild M., Evans F. (1984). New look at Lepidoptera toxins. In Symp. R. Entomological Soc. London, 135–139.
Marshall G. T., Kinghorn A. D. (1984). Short-chain phorbol ester constituents of croton oil. J. Am. Oil Chemists' Soc. 61 (7), 1220–1225. doi: 10.1007/BF02636256
Metcalfe C. R. (1967). Distribution of latex in the plant kingdom. Economic Bot. 21, 115–127. doi: 10.1007/BF02897859
Nakamura S. I., Nishizuka Y. (1994). Lipid mediators and protein kinase c activation for the intracellular signaling network. J. Biochem. 115 (6), 1029–1034.
National Center for Biotechnology Information (2023) PubChem compound summary for CID 499953, 13-acetylphorbol. Available at: https://pubchem.ncbi.nlm.nih.gov/compound/499953 (Accessed March 9, 2023).
Neuwirth E. (2022) RColorBrewer: ColorBrewer palettes. Available at: https://CRAN.R-project.org/package=RColorBrewer.
Nishizuka Y. (1984). The role of protein kinase c in cell surface signal transduction and tumour promotion. Nature 308 (5961), 693–698. doi: 10.1038/308693a0
Ojani R., Liu P., Fu X., Zhu J. (2016). Protein kinase c modulates transcriptional activation by the juvenile hormone receptor methoprene-tolerant. Insect Biochem. Mol. Biol. 70, 44–52. doi: 10.1016/j.ibmb.2015.12.001
Ott H. H., Hecker E. (1981). Highly irritant ingenane type diterpene esters from Euphorbia cyparissias l. Experientia 37 (1), 88–91. doi: 10.1007/BF01965588
Punsuvon V., Nokkaew R., Karnasuta S. (2012). Determination of toxic phorbol esters in biofertilizer produced with Jatropha curcas seed cake. ScienceAsia 38, 223. doi: 10.2306/scienceasia1513-1874.2012.38.223
R Core Team (2020). R: a language and environment for statistical computing (Vienna, Austria: R Foundation for Statistical Computing). Available at: https://www.R-project.org/.
Shi Q.-W., Su X.-H., Kiyota H. (2008). Chemical and pharmacological research of the plants in genus Euphorbia. Chem. Rev. 108, 4295–4327. doi: 10.1021/cr078350s
Stanković M. S., Zlatić N. M. (2014). Antioxidant activity and concentration of secondary metabolites in the plant parts of Euphorbia cyparissias l. Kragujevac J. Sci. 36), 121–128. doi: 10.5937/KgJSci1436121S
Vasas A., Hohmann J. (2014). Euphorbia diterpenes: isolation, structure, biological activity, and synthesis, (2008–2012). Chem. Rev. 114, 8579–8612. doi: 10.1021/cr400541j
Vasas A., Rédei D., Csupor D. H., Molnár J., Hohmann J. (2012). Diterpenes from European Euphorbia species serving as prototypes for natural-product-based drug discovery. Eur. J. organic Chem. 2012, 5115–5130. doi: 10.1002/ejoc.201200733
Vogg G., Achatz S., Kettrup A., Sandermann H. (1999). Fast sensitive and selective liquid chromatographic tandem mass spectrometric determination of tumor-promoting diterpene esters. J. Chromatogr. A 855, 563–573. doi: 10.1016/s0021-9673(99)00728-1
Wang H.-B., Wang X.-Y., Liu L.-P., Qin G.-W., Kang T.-G. (2015). Tigliane diterpenoids from the euphorbiaceae and thymelaeaceae families. Chem. Rev. 115, 2975–3011. doi: 10.1021/cr200397n
Wang C. J., Yan Q. L., Ma Y. F., Sun C. P., Chen C. M., Tian X. G., et al. (2017). Ent-abietane and tigliane diterpenoids from the roots of euphorbia fischeriana and their inhibitory effects against mycobacterium smegmatis. J. Natural Products 80 (5), 1248–1254. doi: 10.1021/acs.jnatprod.6b00786
Weng H. Z., Tian Y., Zhang J. S., Huang J. L., Tang G. H., Yin S. (2022). A new tigliane-type diterpenoid from Euphorbia tirucalli. Natural Product Res. 36 (20), 5380–5386. doi: 10.1080/14786419.2021.1938039
Wickham H., Averick M., Bryan J., Chang W., McGowan L. D. A., François R., et al. (2019). Welcome to the tidyverse. J. Open Source software 4 (43), 1686. doi: 10.21105/joss.01686
Wink M., Grimm C., Koschmieder C., Sporer F., Bergeot O. (2000). Sequestration of phorbolesters by the aposematically coloured bug Pachycoris klugii (Heteroptera: scutelleridae) feeding on Jatropha curcas (Euphorbiaceae). Chemoecology 10, 179–184. doi: 10.1007/PL00001820
Wink M., Koschmieder C., Sauerwein M., Sporer F. (1997). "Phorbol esters of J. curcas -biological activities and potential applications." in Biofuel and Industrial Products from Jatropha curcas. ed G. M. Gübitz, M. Mittelbach and M. Trabi (Graz: Dbv-Verlag Univ. Graz) 160-166
Keywords: phorbol-13-acetate, TPA (12-O-tetradecanoylphorbol-13-acetate), cypress spurge, Hyles euphorbiae, herbivore–plant interaction, LC-MS/MS, LC-QTOF-MS, tigliane analysis
Citation: Fiedler S, Langner M, Oertel R, Vassão DG, Nitschke J, Gershenzon J and Hundsdoerfer AK (2023) First insights into herbivore–plant interaction of the spurge hawkmoth and Euphorbia cyparissias. Front. Ecol. Evol. 11:1197194. doi: 10.3389/fevo.2023.1197194
Received: 30 March 2023; Accepted: 16 June 2023;
Published: 12 July 2023.
Edited by:
Michael Wink, Heidelberg University, GermanyReviewed by:
Lora A. Richards, University of Nevada, Reno, United StatesAndréa Sobottka, The University of Passo Fundo, Brazil
Copyright © 2023 Fiedler, Langner, Oertel, Vassão, Nitschke, Gershenzon and Hundsdoerfer. This is an open-access article distributed under the terms of the Creative Commons Attribution License (CC BY). The use, distribution or reproduction in other forums is permitted, provided the original author(s) and the copyright owner(s) are credited and that the original publication in this journal is cited, in accordance with accepted academic practice. No use, distribution or reproduction is permitted which does not comply with these terms.
*Correspondence: Stephanie Fiedler, c3RlcGhhbmllLmZpZWRsZXJAcG9zdGVvLmRl; Anna K. Hundsdoerfer, YW5uYS5odW5kc2RvZXJmZXJAc2VuY2tlbmJlcmcuZGU=