- 1Department of Hydrobiology, Institute of Functional Biology and Ecology, Faculty of Biology, University of Warsaw, Warsaw, Poland
- 2Department of Environmental Microbiology and Biotechnology, Institute of Microbiology, Faculty of Biology, University of Warsaw, Warsaw, Poland
- 3Institute of Biology, University of Rzeszow, Rzeszow, Poland
- 4Department of Biological Sciences, College of Science, Sungkyunkwan University, Suwon, Republic of Korea
- 5Biological and Chemical Research Centre, University of Warsaw, Warsaw, Poland
The temperature–size rule (TSR) has been consistently observed in numerous studies, showing that ectotherms reared at higher temperatures experience accelerated growth during the juvenile stage and ultimately reach smaller sizes and younger ages at maturity. One explanation for this response is that it occurs when the effect of temperature on mortality, including predation, outweighs its effect on food intake and metabolism. While several studies have found that the latter effect is close to the expected result based on the Q10 = 2 assumption, confirmation of this hypothesis requires evidence that the effect of temperature on mortality exceeds the Q10 = 2 threshold. To test this hypothesis, we conducted experiments with two fish species: rudd and Malabar danio. We examined the capture rate, which serves as a proxy for mortality, as well as the standard metabolic rate (SMR) and several parameters characterising the mobility of the fish and their planktonic prey (Daphnia) at different temperatures. The results strongly supported our hypothesis, as the capture rate increased significantly more than expected based on the Q10 = 2 assumption, especially for the danio. This substantial effect cannot be attributed solely to the thermal sensitivity of the SMR, as the Q10 for the SMR was only around 2. The most likely explanation seems to be a much more pronounced increase in the fish’s mobility and resulting reaction field volume compared to its planktonic prey at elevated temperatures. This increased mobility leads to an improved attack rate by the fish, which exceeds the prediction made by the Q10 = 2 assumption. This mechanism may explain not only the TSR pattern in zooplankton, but also their reduced mean body size and density at population and community levels at elevated temperatures, and may hypothetically be observed at other predator–prey interfaces.
Highlights
● Confirmed more than twofold increase in predation mortality by planktivorous fish per 10°C increase in temperature.
● Thermal sensitivity of fish attack rate, rather than standard metabolic rate, explains the observed effect.
● The proposed mechanism explains the temperature–size rule in zooplankton.
● Mechanism may also explain smaller average body size and lower population density in zooplankton communities at higher temperatures.
Introduction
Body size has long been considered a fundamental trait of an organism, influencing almost every aspect of its biology, since almost all traits of an individual (e.g. longevity and fecundity), a population (e.g. intraspecific competitive ability, population growth rate) and a community (e.g. interspecific competitive ability) are related to body size (Peters, 1983). Therefore, with the onset of global warming, elucidating the mechanisms responsible for the effects of temperature on the body size of organisms at different levels of organisation (among other ecological and evolutionary responses to recent climate change) is an issue of great importance.
Numerous studies have consistently shown a phenotypically plastic response, known as the temperature–size rule (TSR), demonstrating that ectotherms reared under higher temperatures experience accelerated growth during their juvenile stage and ultimately reach smaller sizes and younger ages upon maturation (Atkinson, 1994; Angilletta and Dunham, 2003). Furthermore, studies have shown that average body size decreases across different ectotherm populations and communities as environmental temperatures increase (Moore and Folt, 1993; Gardner et al., 2011; Sheridan and Bickford, 2011; Maszczyk and Brzezinski, 2018). This pattern is commonly observed along geographic clines, including latitude, altitude and depth (Mauchline, 1972; Green, 1995; Gillooly and Dodson, 2000; Anufriieva and Shadrin, 2014; Havens et al., 2015; Maszczyk and Brzezinski, 2018), as well as in the seasonal and long-term variability of size structure (Sommer et al., 1986; Adrian and Deneke, 1996; Hawkins et al., 2008; Daufresne et al., 2009; Gardner et al., 2011; Maszczyk and Brzezinski, 2018), especially in aquatic environments (Forster et al., 2012; Horne et al., 2015). While the observed patterns of temperature and body size at different levels of biological organisation are widely recognised, the mechanisms responsible for these patterns remain an open question and are the subject of ongoing research.
Two general alternate explanations of the TSR pattern have been proposed. First is attributed to higher metabolic rate, which is seen as an inevitable consequence of elevated temperature, and smaller size at stage are a negative consequence of insufficient energy or oxygen available for growth (Atkinson et al., 2006; Verberk and Atkinson, 2013; Verberk et al., 2021). Insufficient oxygen available for growth is explained by a decrease in oxygen supply as a net effect of temperature-dependent oxygen solubility, viscosity, and the cost of ventilating respiratory surfaces not keeping pace with the increased metabolic demand for oxygen due to increased physiological rates, or the cost of supply being too high (Verberk and Atkinson, 2013). The reduced susceptibility to oxygen limitation in a smaller body may be due to several reasons, including: (1) less demanding oxygen uptake due to a greater surface to volume ratio of the body (Atkinson et al., 2006), (2) shorter distances for oxygen transport within the body, and (3) more efficient diffusion within tissues due to smaller cells (Woods, 1999), as oxygen diffuses more efficiently through a cell membrane than through the cytoplasm (Subczynski et al., 1989). The second explanation emphasises trade-offs between growth and reproduction at different temperatures to maximise individual fitness (Kozłowski, 1992; Kozlowski et al., 2004; Wootton et al., 2022; Thunell et al., 2023). It is proposed that one possible adaptive pathway involves evolved responses to cope with shorter life spans at elevated temperatures due to increased mortality, including predation. These responses may include early maturation or increased allocation of resources to reproduction, allowing organisms to adapt to reduced opportunities for lifetime reproductive success (Kozłowski, 1992; Kozlowski et al., 2004). In support of this notion, one of the optimal resource allocation models has shown that the TSR can occur when mortality risk, including predation, increases with temperature (Kozlowski et al., 2004). Specifically, TSR occurs when the thermal sensitivity of mortality exceeds the thermal sensitivity of intake and metabolic rates. An important question is whether this scenario applies to ectotherms, where the observed thermal sensitivity of body size is consistent with TSR. To our knowledge, there is currently no study in the literature that has directly addressed this question.
TSR has been extensively studied in zooplankton due to their important ecological role as a critical link between primary producers and higher trophic levels in marine and freshwater food webs. The majority of studies have consistently demonstrated the influence of temperature on the plasticity of life history traits supporting TSR in different zooplankton species (Atkinson, 1994; Maszczyk and Brzezinski, 2018; Roman and Pierson, 2022). In addition, several studies have reported earlier maturation at smaller body sizes and increased allocation of resources to reproduction at the expense of somatic growth in zooplankton in response to chemical cues from size-selective fish predators (fish kairomones) (Stibor, 1992; Weetman and Atkinson, 2004; Maszczyk and Bartosiewicz., 2012). The similarity in the phenotypic response of zooplankton to fish kairomones and elevated temperature suggests that the response to TSR may represent an adaptation to increased predation risk at elevated temperatures (Weetman and Atkinson, 2004). However, this hypothesis was not confirmed as Weetman and Atkinson (2004) found no interaction between the two factors tested on life history traits.
In addition, studies focusing on the fish perspective have provided multiple lines of evidence suggesting that increased fish predation is responsible for the negative relationship observed between temperature and zooplankton body size at different ecological levels. It is generally accepted that the seasonal variation in body size of zooplankton communities is driven by increased planktivore mortality that occurs during periods of high juvenile fish densities in early summer (Sommer et al., 1986). A similar explanation has been proposed for latitudinal changes in the size structure of zooplankton communities, where the pattern is attributed to the higher densities of small omnivorous fish, characterised by short life spans, early maturation, high reproductive frequencies and a prolonged spawning season, which efficiently feed on zooplankton in subtropical and tropical latitudes (Jeppesen et al., 2010; Iglesias et al., 2011). Furthermore, research has shown that the increased foraging rate of planktivorous fish and subsequent zooplankton mortality at elevated temperatures can be influenced not only by changes in fish density and species composition, but also by improved fish learning abilities (Babkiewicz et al., 2021), altered fish behaviour (Persson, 1986; Bergman, 1987; Linløkken et al., 2010; Gliwicz et al., 2018), more efficient location of zooplankton patches (Gliwicz and Maszczyk, 2016), and changes in thermocline and oxycline shape that enhance fish access to larger-bodied zooplankton in deeper water layers (Maszczyk et al., 2019).
Elevated temperatures cause an increase in the kinetic energy of biochemical reactions, resulting in a faster rate of metabolic processes (Schmidt-Nielsen, 1979). This acceleration is often measured by the Q10 coefficient, which represents the rate of increase in enzymatic reactions and physiological processes per 10°C increase in temperature (Schmidt-Nielsen, 1979). The Q10 value is determined using the Van’t Hoff equation (formula 1):
where R2 is the measured reaction rate at temperature T2 (where T2 > T1) and R1 is the measured reaction rate at temperature T1. According to the Metabolic Theory of Ecology (MTE), rates of chemical reactions can be used to predict rates and processes at different ecological levels, including individuals, populations, communities and ecosystems (Brown et al., 2004). MTE is widely accepted and is commonly employed in models aimed at predicting species and ecosystem responses to warming (DeLong et al., 2017; Sentis et al., 2021). However, it is worth noting that there has been debate in the past about the underlying mechanisms and the allometric exponent in this theory (Tilman et al., 2004; Hirst et al., 2014). One of the arguments against the Metabolic Theory of Ecology (MTE) as a descriptor of thermal sensitivity is based on evidence from various species showing acclimation of metabolic rates in response to temperature changes (Donelson et al., 2012). The use of Q10 as a descriptor of thermal sensitivity is sometimes considered outdated, as it originated as a simple predictor of how biochemical reaction rates change with temperature. However, Q10 still serves as a useful tool for comparing the thermal sensitivities of different processes, allowing the identification of relative differences between them. It is generally observed that most biological processes and rates have a unimodal relationship with temperature, with a biologically relevant temperature range on the rising part of the curve. Within this range, the Q10 coefficient typically falls between 1 and 3, indicating an exponential increase with increasing body temperature. This pattern is also observed for various rates, including total assimilation rates, respiration rates, and net growth and reproduction rates. For example, similar Q10 values have been reported for filtration rate (Burns, 1969) and respiration rate (Chopelet et al., 2008) in zooplankton, and growth and reproduction rates of algae (Raven and Geider, 1988), zooplankton (Bottrell, 1976) and fish (Schulte, 2011; Schmidt-Nielsen, 1979).
On the other hand, due to inherent differences in the characteristics of fish and zooplankton, such as their visual, cognitive and mobility abilities, it is possible that the increase in foraging rate of planktivorous fish with temperature may exceed the assumption of Q10 = 2, which in turn may be reflected in zooplankton mortality greater than Q10 = 2. This is expected as these characteristics may influence the thermal sensitivity of foraging rate (and hence mortality) asymmetrically to the direct effects of temperature on the metabolic processes of both fish and their prey (Schulte, 2011; Dell et al., 2014). For example, fish tend to have greater mobility, including greater body speed, manoeuvrability and reaction distance, than their planktonic prey. Elevated temperatures could increase the fish’s ability to respond (attack) to a larger field volume than expected based on the Q10 = 2 assumption, while having a minimal effect on the prey’s response (evasion and escape) field volume and corresponding ability to avoid and escape. Consequently, elevated temperatures may increase the attack rate of fish beyond that predicted by the Q1 = 2 assumption. It should be noted that there is currently no experimental verification of these predictions in the available literature.
The aim of this study was to test three hypotheses regarding the thermal sensitivity of foraging and metabolic rates of planktivorous fish. The first hypothesis postulates that elevated temperature leads to a greater increase in foraging rate on zooplankton prey (Daphnia magna) than expected from the Q10 = 2 assumption for a temperate fish, a juvenile rudd (Scardinius erythrophthalmus), and a subtropical fish, the Malabar danio (Devario malabaricus). The second hypothesis proposes that the hypothetical deviation in the thermal sensitivity of the fish’s foraging rate from the Q10 = 2 assumption cannot be explained solely by changes in the metabolic rates of the predator and prey themselves. Lastly, the third hypothesis postulates that the deviation can be explained by the greater temperature sensitivity of the fish’s attack rate than predicted by the Q10 = 2 assumption, due to a much greater effect of temperature on its mobility (i.e. body speed, manoeuvrability and reaction distance), and hence on the reaction (attack) field volume, than on the reaction (evasion and escape) field volume of its prey.
Materials and methods
The approach
The hypotheses were tested in a two-stage experimental procedure, each conducted at three temperature treatments (16, 21 and 26°C). The temperatures used in this experimental study are well within the range of temperatures experienced by both fish species in the field. During Step I, we assessed the capture rate as a proxy for mortality and capture efficiency of 1-year-old rudd (Scardinius erythrophthalmus) and Malabar danio (Devario malabaricus) individuals offered zooplankton prey (Daphnia magna), with two experimental fish allowed to forage at nearly constant prey density. We also assessed the reaction field volume and attack rate of the fish by measuring body speed, reaction (attack or escape) angle and distance of both the fish and the Daphnia during a fish attack on a prey item. In addition, we calculated: (i) the visual field volume and encounter rate of the fish by assessing the body speed, detection angle and distance of the fish, and the body speed of the Daphnia when the fish searched for a prey item, and (ii) handling time. Experiments were conducted with 6 different pairs of juvenile fish of each of the species (each pair kept in separate aquariums for at least two weeks) in an experimental system similar to that used previously (Gliwicz and Maszczyk, 2016; Gliwicz et al., 2018; Maszczyk et al. 2021). In Step II, we measured the standard metabolic rate (SMR) of the same fish individuals kept individually in respiration chambers placed in the same experimental system as in Step I.
Test animals
Juveniles of both fish species were similar in length and fresh weight (3.22 ± 0.17 cm and 1.66 ± 0.47 g for rudd and 3.48 ± 0.31 cm and 2.17 ± 0.24 g for danio, respectively). The rudd is a temperate fish of the family Cyprinidae, widely distributed in Europe and central Asia, around the basins of the North Sea, Baltic Sea, Black Sea, Caspian Sea and Aral Sea. The Malabar danio is a tropical fish of the Cyprinidae family, native to Sri Lanka and the west coast of India. Juveniles of both species are omnivorous and can efficiently feed on planktonic animals. All rudd used in the experiments were the offspring of 10 females and 10 males hatched at the Institute of Inland Fisheries, Żabieniec, Poland. All danio came as a cohort of 100 juveniles from a long-term culture at Sumik Aquarium Fish Breeding in Kraków, Poland, hatched as the offspring of several females and several males. The fish were kept in 6 pairs of each species under laboratory conditions (21.0°C ± 0.1, 12:12 L:D photoperiod) for at least 2 months prior to the experiments. Each of the 6 pairs of each species was kept separately in 6-litre home tanks throughout the experimental period. The fish were acclimated to a given experimental temperature for at least two days before the procedures of both steps were performed. They were fed daily with small amounts of live Daphnia and frozen chironomid larvae, except for 24 h before the experiments. During the experiments, a cohort of 5-day-old Daphnia magna (clone MB01 from the fish-inhabited Großer Binnensee, Germany) was offered to the fish. This clone is highly sensitive to chemical information on fish predation risk (kairomones) (Wilczynski et al., 2019).
The experimental systems
The system used in Step I of the procedure was placed in an air-conditioned laboratory room, which allowed constant temperatures to be maintained (16, 21, 26 ± 0.1°C). It consisted of an 80-litre main aquarium (800 × 667 × 150 mm, length, height and width, respectively), and an annex aquarium (300 × 600 × 300 mm) separated by a removable sliding gate with a 0.16 mm fine mesh net. The main aquarium had an outflow at the bottom with a plankton net at the end to facilitate the collection and counting of all uneaten prey at the end of each replicate of the experiments from Step I. The light source consisted of 4 evenly distributed LED lamps (2.5 W, POLUX®) at a height of 1 m, shining through a semi-transparent Plexiglas diffuser. This provided a light intensity of 0.8 ± 0.2 μmol × m−2 × s−1 throughout the entire aquarium and during each experiment, as confirmed by LI-COR (model 189) measurements taken at depths of 30 and 60 cm. The system also included (1) a colour camera (Panasonic HDC-HS60 full HD) to record the foraging fish, which was separated from the main and annex aquariums by a black curtain, (2) a tube dispenser to replenish consumed prey during the experiment, (3) a small EHEIM 1000.220 pump located in the annex aquarium, which provided a slight water current in the main aquarium, which made it possible to maintain an even distribution of zooplankton during the experiments, and (4) a barrel with an aeration system under the aquarium to hold the experimental water between experiments.
The system used in Step II of the procedure consisted of the same 80 litre main aquarium as the system used in Step I of the procedure. The system also included a flow-through respirometer. It consisted of 5 respirometry chambers, each made of transparent Plexiglas and with a volume of 22 mL. The water in the respirometer circulated in a loop. Each chamber had an inflow connected by plastic tubing to a peristaltic 24-channel peristaltic pump (Ismatec®), which supplied water from an intensively aerated (using a Hailea® ACO-9630 air pump) glass tank (V = 20 L), and an outflow connected to a measurement chamber, from which the water was returned to the glass tank by the same peristaltic pump. The respirometry chambers were placed one above the other in the main aquarium. Dissolved oxygen measurements in the measurement chambers were made with a probe (model 287, UNISENSE) connected to the microsensor amplifier, thermometer and computer.
The experimental design during Step I of the procedure
We conducted a total of 144 experiments, each experiment on a single pair of fish (2 fish species × 3 temperatures × 6 pairs of fish × 4 replicates, Appendix S1; Table 1). On each experimental day, we performed 3 experiments for 3 (out of 6) pairs of each of the 2 fish species in one of the 3 temperature treatments (Table 1). Prior to each experiment, water from the barrel was poured into the aquariums. One pair of fish was then transferred to the main aquarium and, after 10 min, moved to the annex to be locked behind the gate (where the fish were acclimated for the next 15 min). An initial batch of 80 Daphnia (density 1 ind. × L−1) was then added to the main aquarium and the light in the system was switched on. At the start of the experiment, the video recording was started, the fish were released into the main tank and allowed to forage for 5 min. During the experiments, two observers counted the captures. The initial density was maintained throughout the experiment by adding a new portion of 10 prey individuals once 10 had been captured by the two experimental fish. A new portion of 10 prey individuals was then released into the aquarium through a dispenser. At the end of the experiment, the light was switched off, the fish were transferred to their home aquarium using fish net (mesh size = 1.0 cm) and the video recording was stopped. Once the fish were transferred back to their home aquarium, the water containing the uneaten prey was drained through a plankton net (mesh size = 150 µm) suspended in a barrel below the aquarium. The main aquarium was additionally rinsed with several litres of water, and the water was again drained through the plankton net. Uneaten prey were collected for further enumeration. At the end of each experimental day, the entire volume of water (100 L) was replaced with fresh tap water, which was allowed to reach the new temperature for at least of 48 hours.
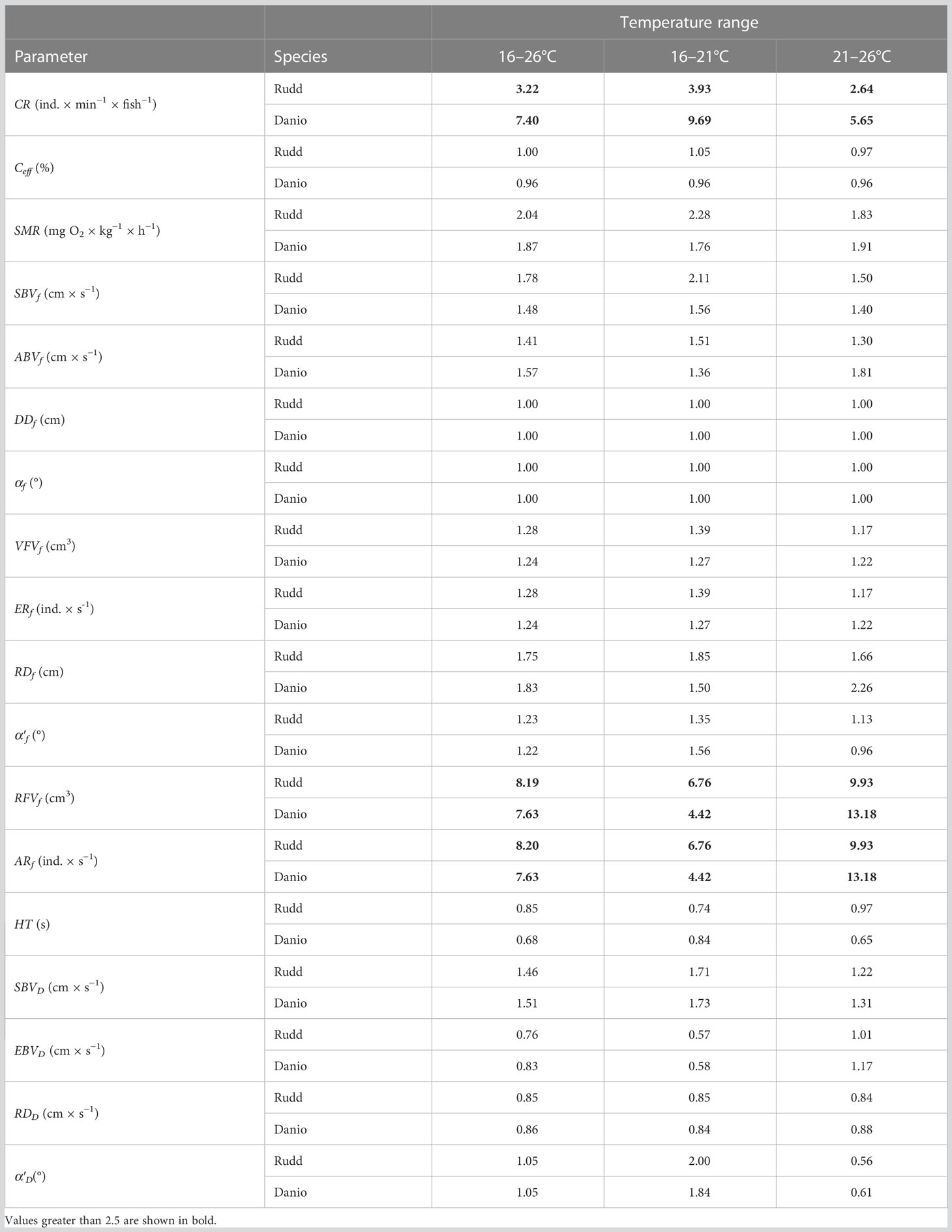
Table 1 The Q10 values for the whole temperature range (16–26°C) and two sub-ranges (16–21 and 21–26°C) for the mean values of capture rate (CR), capture efficiency (Ceff), standard metabolic rate (SMR), fish body speed during search (SBVf), fish body speed during attack (ABVf), their detection distance (DDf), and angle (αf), visual field volume (VFVf), encounter rate (ERf), reaction (i.e. attack) distance (RDf) and angle (α’f), reaction field volume (RFVf), attack rate (ARf) and handling time (HT), at temperatures of 16, 21 and 26°C, as well as the body speed of the Daphnia during search (SBVD) and escape (EBVD) from the fish, and their reaction (i.e. escape) distance (RDD) and angle (α’D) during the attack by the fish at temperatures of 16, 21 and 26°C.
Foraging rate was calculated as the difference between the number of Daphnia prey offered during a trial and the number of uneaten prey collected in the net after the trial, divided by the duration of a trial (5 min) and the number of fish (2 ind.) in the trial. Capture efficiency was calculated as the percentage of the total number of prey captured in relation to the total number of observed captures. The body speed of daphnids and fish was calculated separately while the fish were searching for and attacking the prey. It was based on the distance travelled measured from the archived videos using VirtualDub 1.10.4 software. Manoeuvrability was measured as the mean angle of attack (for fish) or escape (for daphnids) between the trajectories before and after the fish encountered the prey. The reaction (i.e. attack) distance of the fish was measured as the distance between the earliest point at which the fish turned towards the prey and the point of capture. The reaction (i.e. escape and evasion) distance of the daphnids was measured as the distance covered by the daphnids during the time corresponding to the fish attack. Statistics for all of the above parameters were performed on the means (i.e. a single value for each of the Step I trials) of at least four measurements. Fish detection distance and angle were taken as the mean of the top 5% of the largest reaction distances and angles measured for the combined data for all temperature treatments (i.e. assuming that detection distance and angle are independent of temperature) separately for each of the fish species. Handling time was measured as the top 5% of the shortest times between two consecutive captures, separately in each of the temperature treatments for each of the fish species. The visual and reaction field volumes, as well as the encounter and attack rates of the fish were calculated for each of the experiments in Step I. The visual and reaction field volumes of the fish were assumed to have a cylindrical shape combined with a hemisphere. The visual field volume was calculated using the following formula:
where: αf is the detection angle of the fish, DDf is the detection distance of the fish, SBVf is the fish’s body velocity during its search for prey. The encounter rate was expressed as the number of Daphnia appearing in the visual field volume of the fish using the following formula:
Reaction field volume was calculated using the following formula:
where: α′f is the attack angle of the fish, RDf is the reaction distance of the fish, ABVf is the body speed of the fish during its attack on the prey. The attack rate was expressed by the following formula:
where RFVD is described by the formula:
where RFVD = is the reaction field volume of the Daphnia, α′D is the reaction (i.e. escape) angle of the Daphnia, RDD is the reaction distance of the Daphnia, EBVD is the body speed of the Daphnia during its escape from an attacking fish. Equations 2–6 are slight modifications of the previous zooplankton encounter probability models (Gerritsen and Strickler, 1977). They are therefore expected to give similar estimates.
In addition, the effect of temperature on all measured and calculated parameters was expressed as the Q10 value, which was calculated according to the formula (1) given in (Schmidt-Nielsen, 1979).
The experimental design during Step II of the procedure
We performed 36 experiments, each with a control (in a single chamber) and with 4 fish (2 individuals of the 2 fish species) in the remaining 4 chambers at one of the 3 temperatures (Appendix S1; Table 2, 6 sets of fish × 3 temperatures × 2 replicates). Prior to each experiment, the fish were kept in their home tanks for 24 h without food to avoid possible contamination and oxidation of organic matter. Before starting the respirometry procedure, the fresh weight of each fish was measured. The four respirometry chambers were then stocked with fish and one was left without fish. Water flow was then started in the respirometry system. During the preliminary tests, the flow rate provided by a peristaltic pump was set at 0.68 L × hour−1 to maintain the water–oxygen saturation level above 75% inside the respirometry system. To avoid stressing the fish during the experiments, the aquarium containing the respirometry chambers was covered with black material to keep the fish in complete darkness during the experiments. The fish were acclimatised in the respirometry chamber for a period of 23 h before the start of the oxygen measurements. 40 measurements of mass-specific oxygen consumption were taken at 5-minute intervals for each of the fish and the control after 23 h from the start of the experiment. The mass-specific oxygen consumption for each fish (mg O2 kg−1 h−1) was calculated using the following formula (7):
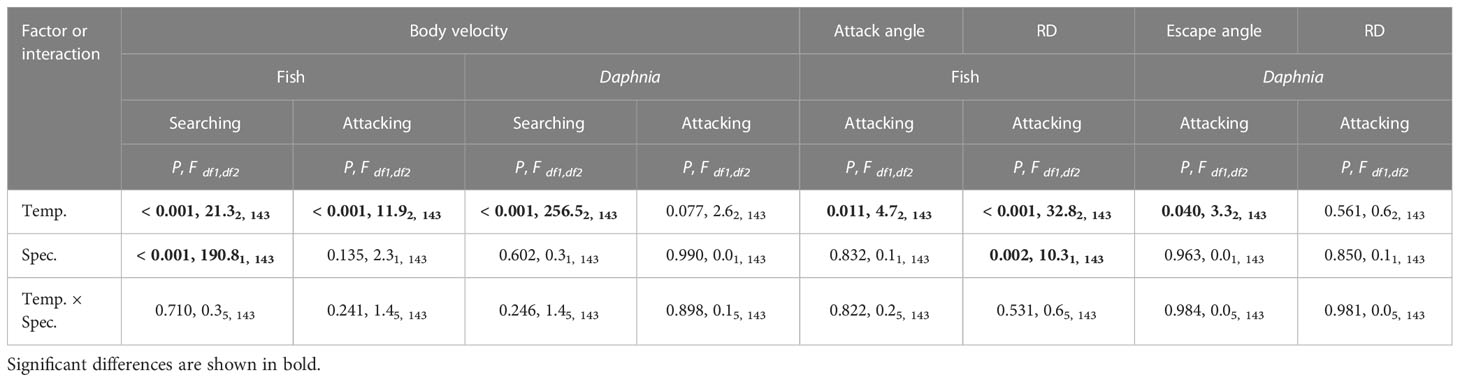
Table 2 Results of the general linear model for the effect of temperature (Temp.), fish species – rudd or Malabar danio (Spec.) and their interactions on the body speed of fish and Daphnia during the search for and attack of the prey by the fish, the reaction (i.e. attack) distance and angle of the fish and the reaction (i.e. escape) distance and angle of Daphnia.
where: O2in is the oxygen content in the control respirometry chamber representing the oxygen content in the water inflow (mg × L−1), O2out is the oxygen content in the water outflow (mg × L−1), F is the water flow rate (L × hour−1), BW is the fresh body weight of the fish placed in a given chamber (kg). The SMR of individual fish was calculated as the average of the lowest 10% of the MO2 measurements (Herrmann and Enders, 2000). In addition, the effect of temperature on fish SMR was expressed as the Q10 value (Schmidt-Nielsen, 1979).
Statistical analyses
To assess the effect of 3 temperatures (temp.), 2 fish species (spec.) and their interactions on fish capture rate, capture efficiency and metabolic rate, we used a general linear model (GLM) with bootstrap (n = 1000). We entered fish species and temperature as factors and capture rate, capture efficiency, metabolic rate, body speed, reaction distance and reaction angle as dependent variables. Dependent variables were transformed as described in Supplementary Material – Data and Analyses. The Bonferroni procedure was used for GLM post-hoc tests. To assess the effect of temperature, fish species and their interactions on the body speed of fish and daphnids during prey seeking and attack by fish, reaction distance and angle of fish and reaction distance and angle of daphnids, we used a modified Welch-ANOVA test, also with bootstrapping (n = 1000). The Games-Howell test was used for post-hoc Welch-ANOVA analyses. Complete statistical analyses, including polynomial linear contrasts, power analysis, and all F and P values, are presented in the Excel file in Supplementary Material – Data and Analyses. Statistics for detection distance and angle, handling time, visual field volume, hit rate, reaction field volume and attack rate were not performed as they were calculated from measurements of other parameters. For all statistics, the significance level was set at α = 0.05 and the confidence level at 95%. Statistics were performed in SPSS 20.0 and G*Power V.3.1.9.6. 56 (Faul et al., 2007). To assess the power of the models, we followed the code available at: https://jakewestfall.shinyapps.io/crossedpower/.
Results
Increased temperature had a positive effect on the capture rate (CR) of the experimental fish (P< 0.001, F = 143.62, 143). The CR of the Malabar danio was greater than that of the rudd (P = 0.007, F = 7.41, 143, Figure 1). The effect of temperature was apparent in each of the three comparisons between temperature treatments (post-hoc test, Figure 1), and was greater in the Malabar danio than in the rudd (P< 0.001, F = 9.55, 143, Figure 1). The Q10 value for the CR was greater than 2 for both fish species in each of the three comparisons between temperature treatments (Table 1). The value was the highest for the Malabar danio between 16 and 21°C (Table 1). Capture efficiency (Ceff) was not affected either by temperature or by fish species (P = 0.846, F = 0.22, 143, Figure 1). The Q10 value for the Ceff was close to 1 for both fish species in each of the three comparisons between temperature treatments (Table 1).
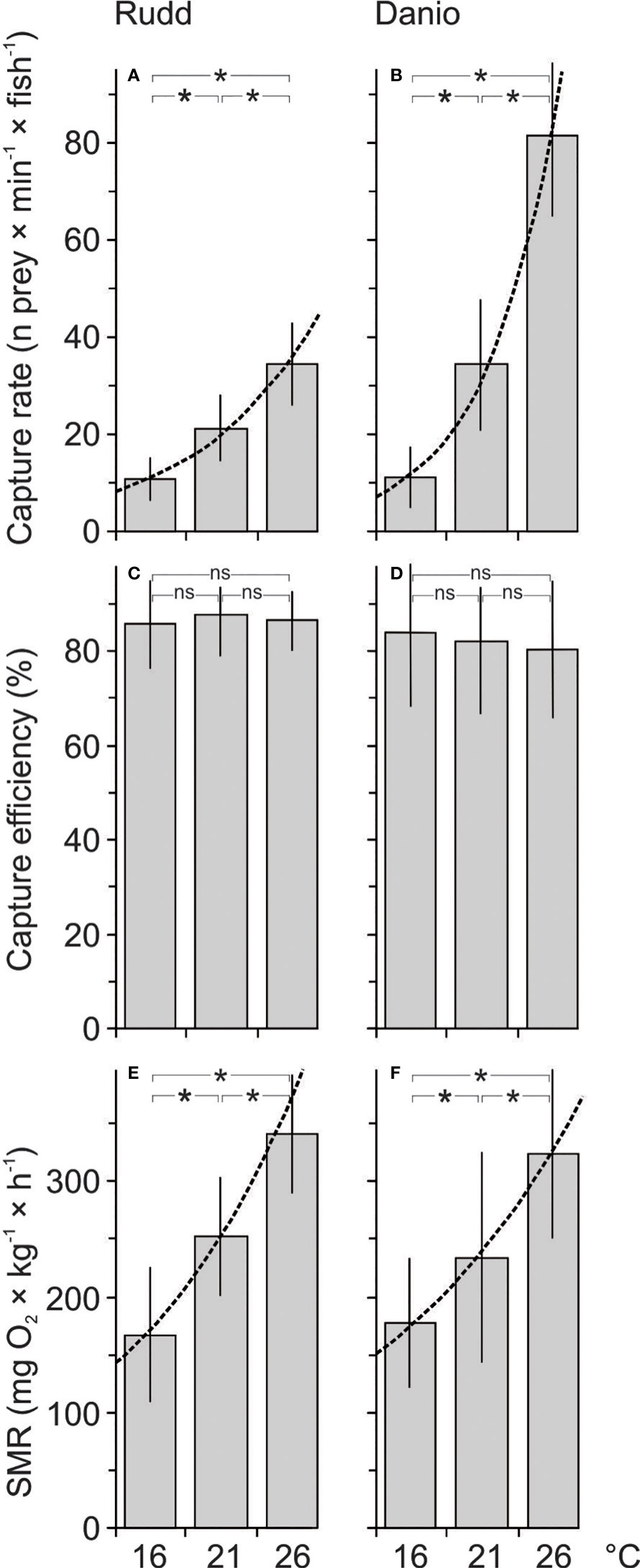
Figure 1 Capture rate (mean ± 1SD, A, B), capture efficiency (mean ± 1SD, C, D) and standard metabolic rate (mean ± 1SD, E, F) of rudd (A, C, E) and danio (B, D, F) at 16, 21 and 26 °C. The lines represent the best fit of the whole data set. Statistical significance of general linear model with the post-hoc test was accepted at *P< 0.05, ns indicates no significance.
Increased temperatures had a positive effect on the standard metabolic rate (SMR) of the fish (P< 0.001, F = 71.92, 143, Figure 1). The effect was evident in each of the three comparisons between temperature treatments (post-hoc test). The SMR was similar in both fish species (P = 0.573, F = 0.31, 143, Figure 1). The Q10 value for the SMR was close to 2 for both fish species in each of the three comparisons between temperature treatments (Table 1).
Increased temperature had a positive effect on the body speed of the fish during search for prey (SBVf, Table 2). The effect was apparent in each of the three comparisons between temperature treatments (post-hoc test), and was greater in the Malabar danio than in the rudd in each of the three comparisons between temperature treatments (post-hoc test). The Q10 coefficient for SBVf was between 1 and 2 for both fish species in each of the three comparisons between temperature treatments (Table 1). Since detection distance and angle were assumed to be independent of temperature, the Q10 coefficient for visual field volume depended only on the SBVf and was similar to the Q10 coefficient for SBVf in each of the three comparisons between temperature treatments (Table 1).
The body speed of Daphnia during prey seeking (SBVD) was significantly affected by temperature (in all of the three comparisons between temperature treatments, post-hoc test) but not by fish species (Table 2). The Q10 coefficient for SBVD was between 1 and 2 in all the comparisons (Table 1). As the SBVD (the only factor differentiating the values of the visual field volume and encounter rate) was much lower than SBVf (i.e. more than an order of magnitude, Figures 2, 3), the Q10 coefficient for the encounter rate was almost identical to that for visual field volume in all of the comparisons between temperature treatments (Table 1).
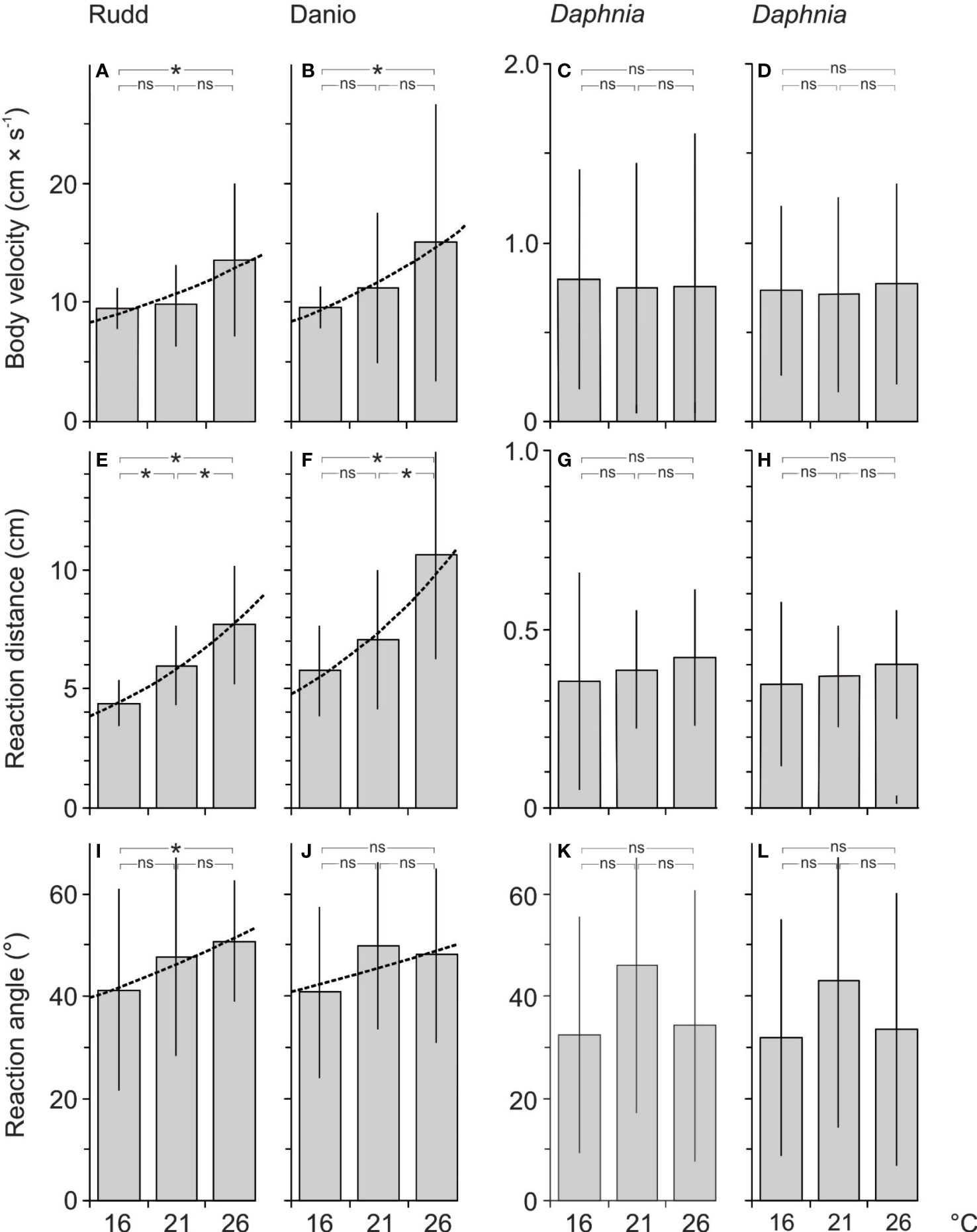
Figure 2 Body speed of rudd (A) and danio (B) during the attack on the prey, body speed of Daphnia during the attack on the prey by the rudd (C) and danio (D), the reaction (i.e. attack) distance of rudd (E) and danio (F), the reaction (i.e. escape) distance of Daphnia during rudd (G) and danio (H) attack on prey, the reaction (i.e. attack) angle of rudd (I) and danio (J) and the reaction (i.e. escape) angle of Daphnia during rudd (K) and danio (L) attack on prey at 16, 21 and 26°C. Statistical significance of the general linear model with the post-hoc test was accepted at *P< 0.05, ns indicates no significance.
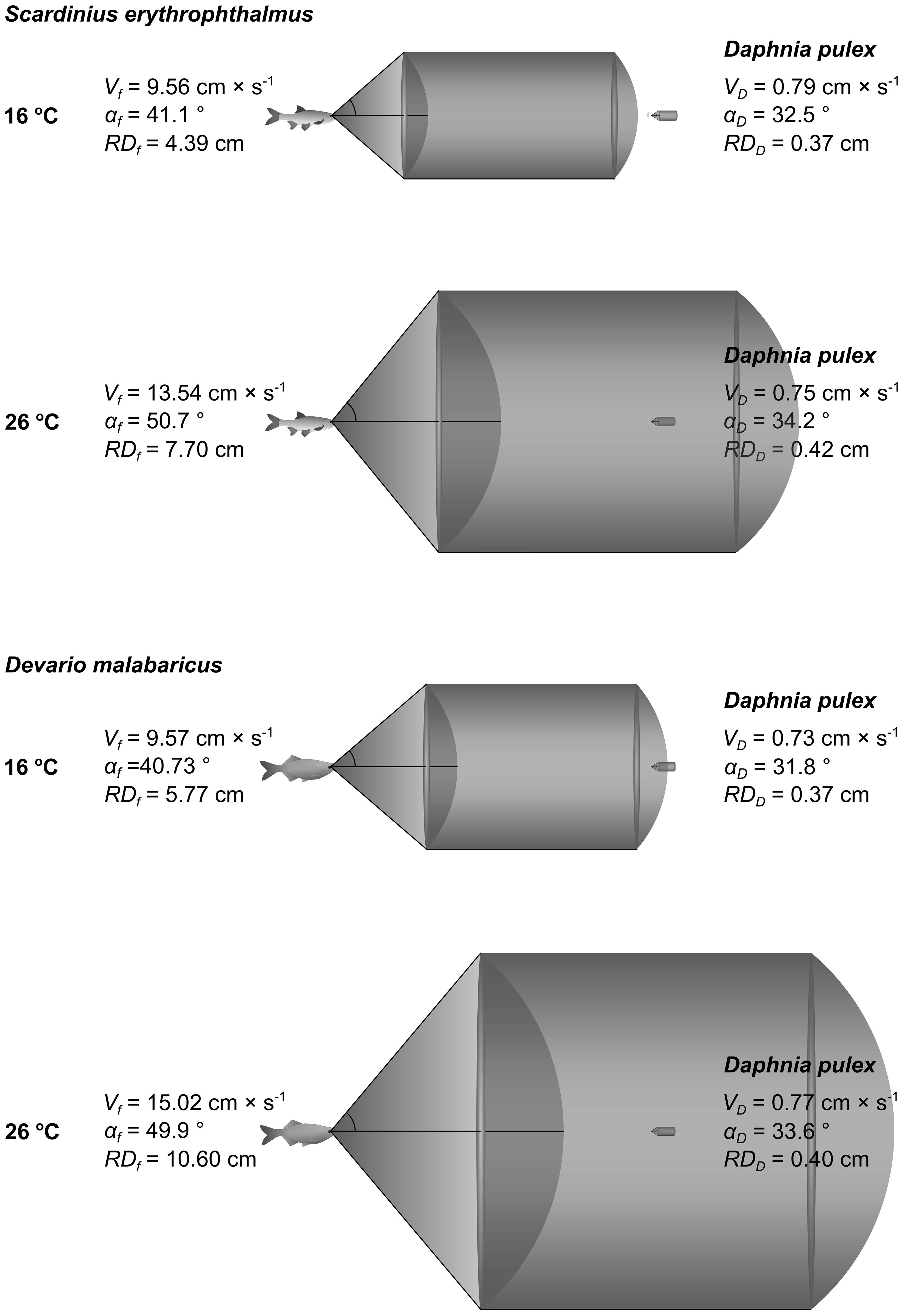
Figure 3 Schematic representation of the reaction field volume (as a function of body speed, reaction distance and reaction angle during the fish’s attack on the prey) of juvenile rudd (S. erythrophthalmus) and Daphnia at 16 and 26°C (upper panels), and of Malabar danio (D. malabaricus) and Daphnia at 16 and 26°C (lower panels).
The body speed of the fish during the attack on the prey (ABVf) was affected by temperature, and was similar for both fish species (Table 2). The effect of temperature for ABVf was only significant between 16 and 26°C (post-hoc test, Figure 2). The Q10 coefficient was between 1 and 2 for both fish species in each of the three comparisons between temperature treatments (Table 1). The reaction distance (RDf) of the fish was affected by temperature, and differed between fish species (Table 2). The effect of temperature on RDf was significant in all of the three comparisons between temperature treatments for each of the fish species (post-hoc test, Figure 2). The only exception was the insignificant effect of temperature between the 16 and 21°C treatments for the danio (post-hoc test, Figure 2). The Q10 coefficient was close to 2 for both fish species in each of the three comparisons between temperature treatments (Table 1). The reaction (attack) angle of the fish (αf) was affected by temperature, and was similar for both fish species (Table 2). Of the three comparisons between temperature treatments, the effect of temperature was only significant between 16 and 26°C for the rudd (post-hoc test, Figure 2). In general, the Q10 coefficient for the αf was slightly greater than 1 for both fish species in each of the three comparisons between temperature treatments (Table 1). The only exception was the value of the Q10 coefficient for the danio between 16 and 26°C, which was slightly less than 1 (Table 1). As all of the three variables affecting the reaction field volume of the fish – RFVf (i.e. ABVf, RDf, αf) were positively affected by temperature, the Q10 coefficient for the RFVf was much greater than 2 for both fish species in all of the three comparisons between temperature treatments (Table 1).
The body velocity of Daphnia during fish’s attack on the prey (EBVD) was not significantly affected either by temperature or by fish species (Table 2). The Q10 coefficient for EBVD was close to 1 in the presence of both fish species in each of the three comparisons between temperature treatments (Table 1). EBVD was more than an order of magnitude lower than ABVf (Figure 2). The reaction (escape) distance of Daphnia (RDD) was significantly affected by either temperature or fish species (Table 2). The Q10 coefficient for the RDD was slightly below 1 in the presence of both fish species in each of the three comparisons between temperature treatments (Table 1). The RDD was more than one order of magnitude lower than the RDf (Figure 2). The reaction (escape) angle of Daphnia (a'D) was significantly affected by temperature (although post-hoc test showed no significant difference between temperature treatments), but not by fish species (Table 2). The Q10 coefficient for a'D was less than 1 for the comparison between 21 and 26°C in the presence of the rudd and danio, slightly greater than 1 for the comparison between 16 and 26°C in the presence of the rudd and danio, and close to 2 for the remaining two comparisons between 16 and 21°C (Table 1). Since the RFVf was much greater than the reaction (escape) field volume of Daphnia prey (i.e. by more than the order of magnitude, Figures 2, 3), and the increase in the RFVf was much greater than the increase in the reaction field volume of Daphnia prey (an increase between 2.3 and 4.4 cm3 for the fish and between 0 and 9.9 × 10−6 cm3 for Daphnia between 16 and 26°C), the Q10 coefficient for the attack rate was almost identical to the RFVf in all of the comparisons between temperature treatments (i.e. being much greater than 2, Table 1).
Handling time was similar in all of the temperature treatments for each of the fish species, ranging from 0.7 to 0.9 seconds (with the Q10 coefficient even slightly less than 1 for each of the comparisons, Table 1).
Discussion
The results strongly supported our first hypothesis, as both fish species (Malabar danio and rudd) exhibited a significantly greater increase in foraging rate with increasing temperature compared to the Q10 = 2 assumption. The observed Q10 values ranged from 2.64 to 9.69 in the different experimental treatments. These results are consistent with previous research on the effect of temperature on fish foraging rate (Persson, 1986; Bergman, 1987; Linløkken et al., 2010). For example, a study of roach (Rutilus rutilus) feeding on dead chironomid larvae reported a Q10 value of approximately 4.8 over a temperature range of 9–17°C (Figure 2A in Linløkken et al., 2010). Previous studies have also shown that Q10 values for metabolic and feeding rates of various Daphnia species can reach up to 2.7 at environmentally relevant temperatures (Burns, 1969; Chopelet et al., 2008,) although they are usually much lower. Consequently, our study shows that elevated environmental temperatures can lead to a greater increase in the foraging efficiency of planktivorous fish and consequently to higher mortality of daphnids compared to their ingestion and metabolic rates. These findings are consistent with the assumptions of the optimal resource allocation model proposed by Kozłowski et al. (2004) and suggest a potential mechanism contributing to the temperature–size rule pattern in Daphnia. It is noteworthy that the temperatures used in our study are within the natural temperature range of both fish species. In particular, Q10 values were particularly high for the Malabar danio, suggesting that it is better adapted to foraging in warmer waters compared to the temperate rudd. Although there is ample evidence in the literature that the temperature sensitivity of metabolic rate in various fish and zooplankton species closely follows the Q10 = 2 assumption (Clarke and Johnston, 1999), suggesting that the difference in thermal sensitivity of metabolic rates between fish and zooplankton cannot account for the substantial (Q10 > 2.64) thermal sensitivity observed in foraging rates, we conducted additional measurements of fish metabolic rates. The aim was to compare the results of metabolic and foraging rates in the same individuals under identical environmental conditions. The results of our study are consistent with previous studies and support our second hypothesis, since the effect of temperature on the metabolic rate of each of the two fish species was in the range of 1.76 and 2.28 in different comparisons of the temperature treatments.
As the simplest explanation for the high thermal sensitivity of the foraging rate, based on the asymmetry in thermal sensitivity of the metabolic rate of the fish and the metabolic rate of its prey, proved invalid (as both are close to Q10 = 2), the next step was to investigate the asymmetric responses to temperature increase in the performance of the fish and its planktonic prey during different foraging phases, such as prey encounter, attack and handling (Dell et al., 2014). Mechanistic models of consumption rate and its temperature dependence predict that temperature affects consumer–resource interactions primarily through its effects on body speed (of either of the consumer, the resource or both), which determines how often consumers and resources encounter each other (including how often a predator attacks its prey), and that temperature effects through its effects on detection distance, capture success and handling time, are of minor importance (Dell et al., 2014). Our results seem to support these predictions. In our study, elevated temperature had a positive effect on all of the measured parameters characterising the fish’s mobility during the attack on the prey, including their body speed, reaction distance and angle. Therefore, the elevated temperature had a large effect on the reaction (attack) field volume of the fish, which was much larger than predicted by the Q10 = 2 assumption. Furthermore, the increase in the reaction (attack) field volume of the fish was much greater than the increase in the reaction (escape) field volume of the Daphnia prey (the increase was between 2.3 and 4.4 cm3 for the fish and between 0 and 9.9 × 10−6 cm3 for the Daphnia), resulting in a Q10 for the attack rate ranging between 4.4 and 13.2. Such a large effect of temperature on the attack rate supports our third hypothesis and suggests that the thermal sensitivity of the fish attack rate is responsible for the large thermal sensitivity of their foraging rate. The effect of temperature on other stages of fish foraging was either negligible (in the case of capture success and handling time) or only a Q10 coefficient of between 1 and 2 (in the case of encounter rate). Therefore, the thermal sensitivity of these stages alone cannot explain the large (Q10 > 2.64) thermal sensitivity of the foraging rate. Although several previous studies have shown a decrease in handling time with increasing temperature (Persson, 1986; Bergman, 1987), the fish in these studies were gape-limited as they were fed large planktonic Chaoborus larvae rather than tiny Daphnia. Although the thermal sensitivity of the encounter rate (and also the visual field volume) in our study was lower than predicted by the Q10 = 2 assumption, it should be pointed out that its calculation in different temperature treatments was made by measuring only the body speed of the fish during their search for prey, while its two remaining components, i.e. detection distance and angle, were not measured. These two components were only estimated as the maximum reaction distance and reaction angle of the fish and were assumed to be independent of temperature. This assumption was based on the results obtained in our previous study, in which we showed an even slightly negative effect of temperature on detection distance in Danio rerio larvae (Babkiewicz et al., 2020). In order to more accurately assess the effect of temperature on encounter rates, future studies should devote more effort to determining the thermal sensitivity of detection distance and angle in juvenile and adult fish.
It should be noted that in our study we determined Q10 for mortality resulting solely from changes in the foraging behaviour of individual fish in a homogeneous environment with respect to biotic and abiotic conditions over a short period of time. In light of previous research suggesting that the increased impact of fish on zooplankton at higher temperatures may also be due to: (i) changes in fish density and species composition (Sommer et al., 1986; Jeppesen et al., 2010; Iglesias et al., 2011), (ii) their enhanced learning abilities (Babkiewicz et al., 2021), (iii) their more efficient localisation of zooplankton patches (Gliwicz and Maszczyk, 2016), and (iv) changes in thermocline and oxycline shape that improve fish access to larger-bodied zooplankton in deeper water layers (Maszczyk et al., 2019), it is plausible to speculate that the thermal sensitivity of mortality resulting from increased foraging by planktivorous fish at elevated temperatures in natural environments may be even greater than assessed in our study.
Conclusion
The literature on the thermal sensitivity of consumer–resource interactions is inconclusive, with numerous examples of studies suggesting that warming either strengthens (Őhlund et al., 2016; Frances and McCauley, 2018; South et al., 2018) or weakens (Rall et al., 2010; Pawar et al., 2012) the interaction. It is therefore important to build experimental datasets to quantify and predict predator effects in different contexts and using a range of different organisms (Dell et al., 2014). Mechanistic models predict that the scaling of interaction strength depends on both the foraging strategy (e.g. active-capture, sit-and-wait, harvesting) and the spatial dimension in which foraging occurs (2D vs. 3D) (Pawar et al., 2012; Dell et al., 2014). In our study, we investigated the thermal sensitivity of the planktivorous fish–Daphnia interface, where the fish is a typical predator–harvester foraging in a three-dimensional environment. This interface is characterised by a large disproportion in body size and mobility between the predator (large and mobile) and its planktonic prey (small and almost static). This large disparity in body size results in a low thermal sensitivity of handling time and a large disparity in the mobility of fish and Daphnia living in a three-dimensional environment, resulting in a large asymmetry in the effect of temperature on the mobility (i.e. body speed, manoeuvrability and reaction distance) of the fish and its prey. The study revealed much greater sensitivity of the foraging rate of the planktivorous fish to temperature increase than was expected from the Q10 = 2 assumption, which is typical of the thermal sensitivity of predator and prey metabolism and their population growth. Therefore, the results suggest that temperature has a greater effect on the fish foraging rate than on consumer–resource metabolism, most likely due to the asymmetric effect on predator and prey mobility. This simple mechanism may have very important consequences, being responsible not only for the life history responses consistent with TSR, but also for the smaller mean body and lower density of zooplankton populations and communities at an elevated temperature. Furthermore, the mechanism may hypothetically be observed in other predator-prey interfaces.
Data availability statement
The original contributions presented in the study are included in the article/Supplementary Material, further inquiries can be directed to the corresponding author/s.
Ethics statement
The animal study was reviewed and approved by First Warsaw Local Ethics Committee for Animal Experimentation.
Author contributions
Study conception and design: PM and ZG. Contribution of data and analysis tools: PM, EB and KL. Collection of data: PM, EB, MZ and WW. Analysis and interpretation of data: PM and KL. Writing – review & editing: PM. Critical review of the text: EB and J-SL. Funding: PM. All authors have read and approved the final manuscript.
Funding
The research described here was supported by grants 2014/15/B/NZ8/00245, 2016/23/D/NZ8/03532 from the National Science Centre, Poland.
Conflict of interest
The authors declare that the research was conducted in the absence of any commercial or financial relationships that could be construed as a potential conflict of interest.
Publisher’s note
All claims expressed in this article are solely those of the authors and do not necessarily represent those of their affiliated organizations, or those of the publisher, the editors and the reviewers. Any product that may be evaluated in this article, or claim that may be made by its manufacturer, is not guaranteed or endorsed by the publisher.
Supplementary material
The Supplementary Material for this article can be found online at: https://www.frontiersin.org/articles/10.3389/fevo.2023.1187404/full#supplementary-material
References
Adrian R., Deneke R. (1996). Possible impact of mild winters on zooplankton succession in eutrophic lakes of the Atlantic European area. Fresh. Biol. 36, 757–770. doi: 10.1046/j.1365-2427.1996.00126.x
Angilletta M. J. Jr., Dunham A. E. (2003). The temperature-size rule in ectotherms: simple evolutionary explanations may not be general. Am. Nat. 162, 332–342. doi: 10.1086/377187
Anufriieva E. V., Shadrin N. V. (2014). Factors determining the average body size of geographically separated Arctodiaptomus salinus (Daday, 1885) populations. Zool. Res. 35, 132. doi: 10.11813/j.issn.0254-5853.2014.2.132
Atkinson D. (1994). Temperature and organism size – a biological law for ectotherms? Adv. Ecol. Res. 3, 1–58. doi: 10.1016/S0065-2504(08)60212-3
Atkinson D., Morley S. A., Hughes R. N. (2006). From cells to colonies: at what levels of body organization does the “temperature–size rule” apply? Evol. Dev. 8, 202–214. doi: 10.1111/j.1525-142X.2006.00090.x
Babkiewicz E., Bazała M., Urban P., Maszczyk P., Markowska M., Gliwicz Z. M. (2020). The effects of temperature on the proxies of visual detection of Danio rerio larvae: observations from the optic tectum. Biol. Open 9, bio047779. doi: 10.1242/bio.047779
Babkiewicz E., Surga K., Gliwicz Z. M., Maszczyk P. (2021). The effect of temperature on the spatial learning rate of zebrafish (Danio rerio). Ethology 127, 632–642. doi: 10.1111/eth.13197
Bergman E. (1987). Temperature-dependent differences in foraging ability of two percids, Perca fluviatilis and Gymnocephalus cernus. Environ. Biol. Fishes 19, 45–53. doi: 10.1007/BF00002736
Bottrell H. C. (1976). A review of some problems in zooplankton production studies. Norw. J. Zool. 24, 419–456.
Brown J. H., Gillooly J. F., Allen A. P., Savage V. M., West G. B. (2004). Toward a metabolic theory of ecology. Ecology 85, 1771–1789. doi: 10.1890/03-9000
Burns C. W. (1969). Relation between filtering rate, temperature, and body size in four species of Daphnia. Limnol. Oceanogr. 14, 693–700. doi: 10.4319/lo.1969.14.5.0693
Chopelet J., Blier P. U., Dufresne F. (2008). Plasticity of growth rate and metabolism in Daphnia magna populations from different thermal habitats. J. Exp. Zool. Part A: Ecol. Genet.Physiol. 309, 553–562. doi: 10.1002/jez.488
Clarke A., Johnston N. M. (1999). Scaling of metabolic rate with body mass and temperature in teleost fish. J. Anim. Ecol. 68, 893–905. doi: 10.1046/j.1365-2656.1999.00337.x
Daufresne M., Lengfellner K., Sommer U. (2009). Global warming benefits the small in aquatic ecosystems. Proc. Nat. Acad. Sci. U.S.A. 106, 12788–12793. doi: 10.1073/pnas.0902080106
Dell A. I., Pawar S., Savage V. M. (2014). Temperature dependence of trophic interactions are driven by asymmetry of species responses and foraging strategy. J. Anim. Ecol. 83, 70–84. doi: 10.1111/1365-2656.12081
DeLong J. P., Gibert J. P., Luhring T. M., Bachman G., Reed B., Neyer A., et al. (2017). The combined effects of reactant kinetics and enzyme stability explain the temperature dependence of metabolic rates. Ecol. Evol. 7, 3940–3950. doi: 10.1002/ece3.2955
Donelson J. M., Munday P. L., McCormick M. I., Pitcher C. R. (2012). Rapid transgenerational acclimation of a tropical reef fish to climate change. Nat. Clim. Change 2, 30–32. doi: 10.1038/nclimate1323
Faul F., Erdfelder E., Lang A.-G., Buchner A. (2007). G*Power 3: a flexible statistical power analysis program for the social, behavioral, and biomedical sciences. Behav. Res. Meth. 39, 175–191. doi: 10.3758/BF03193146
Forster J., Hirst A. G., Atkinson D. (2012). Warming-induced reductions in body size are greater in aquatic than terrestrial species. Proc. Nat. Acad. Sci. U.S.A. 109, 19310–19314. doi: 10.1073/pnas.1210460109
Frances D. N., McCauley S. J. (2018). Warming drives higher rates of prey consumption and increases rates of intraguild predation. Oecologia 187, 585–596. doi: 10.1007/s00442-018-4146-y
Gardner J. L., Peters A., Kearney M. R., Joseph L., Heinsohn R. (2011). Declining body size: a third universal response to warming? Trends Ecol. Evol. 26, 285–291. doi: 10.1016/j.tree.2011.03.005
Gerritsen J., Strickler J. R. (1977). Encounter probabilities and community structure in zooplankton: a mathematical model. J. Fish. Res. Board Can. 34, 73–82. doi: 10.1139/f77-008
Gillooly J. F., Dodson S. I. (2000). Latitudinal patterns in the size distribution and seasonal dynamics of new world, freshwater cladocerans. Limnol. Oceanogr. 45, 22–30. doi: 10.4319/lo.2000.45.1.0022
Gliwicz Z. M., Babkiewicz E., Kumar R., Kunjiappan S., Leniowski K. (2018). Warming increases the number of apparent prey in reaction field volume of zooplanktivorous fish. Limnol. Oceanogr. 63, S30–S43. doi: 10.1002/lno.10720
Gliwicz Z. M., Maszczyk P. (2016). Heterogeneity in prey distribution allows for higher food intake in planktivorous fish, particularly when hot. Oecologia 180, 383–399. doi: 10.1007/s00442-015-3485-1
Green J. (1995). “Altitudinal distribution of tropical planktonic cladocera,” in Cladocera as model organisms in biology (Springer, Dordrecht), 75–84.
Havens K. E., Pinto-Coelho R. M., Beklioğlu M., Christoffersen K. S., Jeppesen E., Lauridsen T. L., et al. (2015). Temperature effects on body size of freshwater crustacean zooplankton from Greenland to the tropics. Hydrobiologia 743, 27–35. doi: 10.1007/s10750-014-2000-8
Hawkins S. J., Moore P. J., Burrows M. T., Poloczanska E., Mieszkowska N., Herbert R. J. H., et al. (2008). Complex interactions in a rapidly changing world: responses of rocky shore communities to recent climate change. Climate Res. 37, 123–133. doi: 10.3354/cr00768
Herrmann J. P., Enders E. C. (2000). Effect of body size on the standard metabolism of horse mackerel. J. Fish Biol. 57, 746–760. doi: 10.1111/j.1095-8649.2000.tb00272.x
Hirst A. G., Glazier D. S., Atkinson D. (2014). Body shape shifting during growth permits tests that distinguish between competing geometric theories of metabolic scaling. Ecol. Lett. 17, 1274–1281. doi: 10.1111/ele.12334
Horne C. R., Hirst A. G., Atkinson D. (2015). Temperature-size responses match latitudinal-size clines in arthropods, revealing critical differences between aquatic and terrestrial species. Ecol. Let. 18, 327–335. doi: 10.1111/ele.12413
Iglesias C., Mazzeo N., Meerhoff M., Lacerot G., Clemente J. M., Scasso F., et al. (2011). High predation is of key importance for dominance of small-bodied zooplankton in warm shallow lakes: evidence from lakes, fish exclosures and surface sediments. Hydrobiologia 667, 133–147. doi: 10.1007/s10750-011-0645-0
Jeppesen E., Meerhoff M., Holmgren K., González-Bergonzoni I., Teixeira-de Mello F., Declerck S. A., et al. (2010). Impacts of climate warming on lake fish community structure and potential effects on ecosystem function. Hydrobiologia 646, 73–90. doi: 10.1007/s10750-010-0171-5
Kozłowski J. (1992). Optimal allocation of resources to growth and reproduction: implications for age and size at maturity. Trends Ecol. Evol. 7, 15–19. doi: 10.1016/0169-5347(92)90192-E
Kozlowski J., Czarnoleski M., Danko M. (2004). Can optimal resource allocation models explain why ectotherms grow larger in cold? Integr. Comparat. Biol. 44, 480–493. doi: 10.1093/icb/44.6.480
Linløkken A. N., Bergman E., Greenberg L. (2010). Effect of temperature and roach Rutilus rutilus group size on swimming speed and prey capture rate of perch Perca fluviatilis and R. rutilus. J. Fish Biol. 76, 900–912. doi: 10.1111/j.1095-8649.2010.02545.x
Maszczyk P., Babkiewicz E., Ciszewski K., Dabrowski K., Dynak P., Krajewski K., et al. (2019). Combined effects of elevated epilimnetic temperature and metalimnetic hypoxia on the predation rate of planktivorous fish. J. Plankton Res. 41, 709–722. doi: 10.1093/plankt/fbz048
Maszczyk P., Bartosiewicz. M. (2012). Threat of treat: the role of fish exudates in the growth and life history of Daphnia. Ecosphere 3, 1–19. doi: 10.1890/ES12-00146.1
Maszczyk P., Brzezinski T. (2018). “Body size, maturation size, and growth rate of crustaceans,” in The natural history of the Crustacea: life histories 5. Eds. Wellborn G. A., Thiel M. (Oxford University Press), 35–72.
Maszczyk P., Talanda J., Babkiewicz E., Leniowski K., Urban P. (2021). Daphnia depth selection in gradients of light intensity from different artificial sources: an evolutionary trap? Limnol. Oceanogr. 66, 1367–1380. doi: 10.1002/lno.11691
Mauchline J. (1972). The biology of bathypelagic organisms, especially Crustacea. Deep-Sea Res. 19, 753–780. doi: 10.1016/0011-7471(72)90097-6
Moore M., Folt C. (1993). Zooplankton body size and community structure: effects of thermal and toxicant stress. Trends Ecol. Evol. 8, 178–183. doi: 10.1016/0169-5347(93)90144-E
Őhlund G., Hedstrőm P., Norman S., Hein C. L., Englund G. (2016). Temperature dependence of predation depends on the relative performance of predators and prey. Proc. R. Soc. B. 282, 2014254. doi: 10.1098/rspb.2014.2254
Pawar S., Dell A. I., Savage V. M. (2012). Dimensionality of consumer search space drives trophic interaction strengths. Nature 486, 485–489. doi: 10.1038/nature11131
Persson L. (1986). Temperature-induced shift in foraging ability in two fish species, roach (Rutilus rutilus) and perch (Perca fluviatilis): implications for coexistence between poikilotherms. J. Anim. Ecol. 55, 829–839. doi: 10.2307/4419
Rall B. C., Vucic-Pestic O., Ehnes R. B., Emmerson M., Brose U. (2010). Temperature, predator–prey interaction strength and population stability. Glob. Change Biol. 16, 2145–2157. doi: 10.1111/j.1365-2486.2009.02124.x
Raven J. A., Geider R. J. (1988). Temperature and algal growth. New Phytol. 110, 441–461. doi: 10.1111/j.1469-8137.1988.tb00282.x
Roman M. R., Pierson J. J. (2022). Interactive effects of increasing temperature and decreasing oxygen on coastal copepods. Biol. Bullet. 243, 171–183. doi: 10.1086/722111
Schmidt-Nielsen K. (1979). Animal physiology: adaptation and environment (Cambridge University Press).
Schulte P. M. (2011). “Effects of temperature: an introduction,” in Encyclopedia of fish physiology: from genome to environment. Ed. Farrell A. P. (Elsevier) 1688–1694.
Sentis A., Montoya J. M., Lurgi M. (2021). Warming indirectly increases invasion success in food webs. Proc. R. Soc B 288, 20202622. doi: 10.1098/rspb.2020.2622
Sheridan J. A., Bickford D. (2011). Shrinking body size as an ecological response to climate change. Nat. Clim. Change 1, 401–406. doi: 10.1038/nclimate1259
Sommer U., Gliwicz Z. M., Lampert W., Duncan A. (1986). The PEG-model of seasonal succession of planktonic events in fresh waters. Archiv Hydrobiolog. 106, 433–471. doi: 10.1127/archiv-hydrobiol/106/1986/433
South J., Welsh D., Anton A., Sigwart J. D., Dick J. T. A. (2018). Increasing temperature decreases the predatory effects of the intertidal shanny Lipophrys pholis on an amphipod prey. J. Fish Biol. 92, 150–164. doi: 10.1111/jfb.13500
Stibor H. (1992). Predator induced life-history shifts in a freshwater cladoceran. Oecologia 92, 162–165. doi: 10.1007/BF00317358
Subczynski W. K., Hyde J. S., Kusumi A. (1989). Oxygen permeability of phosphatidylcholine-cholesterol membranes. Proc. Nat. Acad. Sci. United Stat. America 86, 4474–4478. doi: 10.1073/pnas.86.12.4474
Thunell V., Gårdmark A., Huss M., Vindenes Y. (2023). Optimal energy allocation trade-off driven by size-dependent physiological and demographic responses to warming. Ecology 104, e3967. doi: 10.1002/ecy.3967
Tilman D., HilleRisLambers J., Harpole S., Dybzinski R., Fargione J., Clark C., et al. (2004). Does metabolic theory apply to community ecology? It's a matter of scale. Ecology 85 (7), 1797–1799. doi: 10.1890/03-0725
Verberk W. C., Atkinson D. (2013). Why polar gigantism and Palaeozoic gigantism are not equivalent: effects of oxygen and temperature on the body size of ectotherms. Funct. Ecol. 27, 1275–1285. doi: 10.1111/1365-2435.12152
Verberk W. C., Atkinson D., Hoefnagel K. N., Hirst A. G., Horne C. R., Siepel H. (2021). Shrinking body sizes in response to warming: explanations for the temperature–size rule with special emphasis on the role of oxygen. Biol. Rev. 96, 247–268. doi: 10.1111/brv.12653
Weetman D., Atkinson D. (2004). Evaluation of alternative hypotheses to explain temperature-induced life history shifts in Daphnia. J. Plankton Res. 26, 107–116. doi: 10.1093/plankt/fbh013
Wilczynski W., Dynak P., Babkiewicz E., Bernatowicz P., Maszczyk P. (2019). The combined effects of hypoxia and fish kairomones on several physiological and life history traits of Daphnia. Freshw. Biol. 64, 2204–2220. doi: 10.1111/fwb.13407
Woods H. A. (1999). Egg-mass size and cell size: effects of temperature on oxygen distribution. Am. Zoolog. 39, 244–252. doi: 10.1093/icb/39.2.244
Keywords: body velocity, consumer–resource interaction, global warming, metabolic rate, predation risk, temperature coefficient, temperature–size rule
Citation: Maszczyk P, Wilczynski W, Gliwicz ZM, Leniowski K, Zebrowski ML, Lee J-S and Babkiewicz E (2023) Mechanisms of increasing predation by planktivorous fish with rising temperature may explain the temperature–body size relationships in zooplankton. Front. Ecol. Evol. 11:1187404. doi: 10.3389/fevo.2023.1187404
Received: 16 March 2023; Accepted: 23 June 2023;
Published: 14 July 2023.
Edited by:
Jan Lindstrom, University of Glasgow, United KingdomReviewed by:
Thomas Hrabik, University of Minnesota Duluth, United StatesShannon J. McCauley, University of Toronto Mississauga, Canada
Copyright © 2023 Maszczyk, Wilczynski, Gliwicz, Leniowski, Zebrowski, Lee and Babkiewicz. This is an open-access article distributed under the terms of the Creative Commons Attribution License (CC BY). The use, distribution or reproduction in other forums is permitted, provided the original author(s) and the copyright owner(s) are credited and that the original publication in this journal is cited, in accordance with accepted academic practice. No use, distribution or reproduction is permitted which does not comply with these terms.
*Correspondence: Piotr Maszczyk, cC5tYXN6Y3p5a0B1dy5lZHUucGw=
†ORCID: Piotr Maszczyk, orcid.org/0000-0002-1738-419X
Wojciech Wilczynski, orcid.org/0000-0001-5690-4584
Z. Maciej Gliwicz, orcid.org/0000-0002-6628-4785
Konrad Leniowski, orcid.org/0000-0002-1784-8978
Marcin Lukasz Zebrowski, orcid.org/0000-0002-1554-6474
Jae-Seong Lee, orcid.org/0000-0003-0944-5172
Ewa Babkiewicz, orcid.org/0000-0003-2398-8448