- 1U.S. Geological Survey, South Carolina Cooperative Fish and Wildlife Research Unit, Clemson University, Clemson, SC, United States
- 2The Nature Conservancy, Cold Spring Harbor, NY, United States
- 3South Carolina Cooperative Fish and Wildlife Research Unit, Department of Forestry and Environmental Conservation, Clemson University, Clemson, SC, United States
- 4Center for Environmental Sciences and Engineering, Institute for the Environment, University of Connecticut, Storrs, CT, United States
The northern Gulf of Mexico supports a substantial level of oil and gas extraction in marine waters and experiences acute and chronic exposure to marine pollution events. The region also supports a diverse array of breeding and migratory seabirds that are exposed to these pollutants during foraging and other activities. Among the pollutants of highest concern within the region are polycyclic aromatic hydrocarbons (PAHs) which tend to be toxic, carcinogenic, mutagenic, or teratogenic. We assessed PAH loads in blood from adult brown pelicans and from feathers of adults and chicks of brown pelicans in relation to individual (e.g., body condition, sex) and spatial (e.g., breeding location within the Gulf, home range size, migration distance) factors. Of the 24 PAHs assessed, 17 occurred at least once among all samples. There were no PAHs found in chicks that were not also found in adults. Alkylated PAHs occurred more commonly and were measured at higher summed concentrations compared to parent PAHs in all samples, indicating that exposure to oil and/or byproducts of oil may have been a substantial source of PAH contamination for brown pelicans during this study. Within adults, PAHs were more likely to occur, and to increase in concentration, in blood samples of females compared to males, although no difference was found in feather samples. We also found that occurrence of and concentration of PAHs increased in adults that migrated longer distances. In adults and chicks, the background levels of oil and gas development within the region of the colony was not a consistent predictor of the presence of or concentration of PAHs. We also found correlations of PAHs with hematological and biochemical biomarkers that suggested compromised health. Our results indicate that both short- and long-term exposure (i.e., blood and feathers, respectively) are occurring for this species and that even nest-bound chicks can accumulate high levels of PAHs. Long-term tracking of PAHs, as well as an assessment of sublethal effects of PAHs on pelicans, could enhance our understanding of the persistence and effects of this contaminant in the northern Gulf as could increasing the breadth of species studied.
Introduction
Polycyclic aromatic hydrocarbons (PAHs) are among the pollutants of highest concern for wildlife and ecosystem health (Eisler, 1987). Though often associated with catastrophic events such as oil spills, PAHs are also ubiquitous background contaminants in both aquatic and terrestrial environments (Marvin et al., 2021). In the United States, 16 PAHs are listed as priority environmental pollutants because of their persistence through time, and their propensity to be carcinogenic to humans (Menzie et al., 1992). In wildlife, even relatively low exposure to PAHs can negatively affect growth and metabolism, blood biochemistry, liver health, and immunity (Briggs et al., 1996; King et al., 2021). Parent PAHs, which typically have higher molecular weights usually originate from pyrogenic sources, tend to have higher bioavailability in the marine environment, and are acutely toxic, whereas alkylated PAHs with lower molecular weights tend to be carcinogenic, mutagenic, or teratogenic (Eisler, 1987; Albers, 2006). Even trace exposure to PAH residue can lead to elevated PAH levels, as can exposure to chronic spills and produced waters (King et al., 2021). PAHs tend not to biomagnify or bioaccumulate, however, because most vertebrates can metabolize PAHs within weeks of ingestion (Albers, 2006; Hylland, 2006; Nfon et al., 2008). Nonetheless, both parent (PAR) and alkylated (ALK) PAHs can still accumulate in body tissues at low concentrations when the rate of uptake exceeds elimination, with potentially detrimental effects (Albers, 2003; Laffon et al., 2006; Champoux et al., 2020). Documenting PAHs in wildlife populations therefore requires intensive sampling to detect exposure pathways and trends while accounting for individual, spatial, and temporal variation (Nicholson et al., 2023).
In mobile wildlife, assessing PAH burdens requires sampling across a wide spatial range. This is particularly true in marine systems, in which habitat features are highly dynamic and contaminants can be transported long distances from their sources. The two primary routes of PAH contamination for wildlife are through direct physical interaction (i.e., contact) or foraging on a contaminated item (i.e., ingestion). Wildlife can be exposed to PAHs during daily activities and behaviors such as foraging, movement within and between habitat patches, and bouts of inactivity or resting (e.g., as they come into contact with a contaminated surface). Across the annual cycle, individuals from migratory populations can be exposed to contaminants across a wide spatial range that might also include different ecosystems, food sources, and regulatory environments (i.e., different political jurisdictions with different regulations pertaining to pollutants) (Seegar et al., 2015; Paruk et al., 2016; Champoux et al., 2020). For example, when individuals within a population vary in their migratory strategies or destination (i.e., partial migration), exposure to contaminants may be more difficult to assess or predict for individuals within a population because of the difficulty in knowing which individuals migrate and the locations to which they migrate (Lamb et al., 2017b; Wilkinson and Jodice, 2023). Outside of the migration period, exposure also can be difficult to assess if individuals have extensive home ranges and if the size of the home range is not consistent among populations (Lamb et al., 2017b,2020). Evaluating the effects of environmental contaminants that are temporally or spatially heterogeneous on mobile wildlife therefore requires detailed data on movement patterns, behavior, and foraging activities of the population in question, as well as background information on the potential sources of contamination. Hence, sampling that is spatially and temporally both intensive and extensive is often required.
The northern Gulf of Mexico is a heavily industrialized sea particularly with respect to oil and gas extraction. The region has been under development for marine energy extraction since the early 1940s, and currently there are ∼ 3,200 active rigs1 along with ∼42k km of pipelines and numerous shipping ports throughout the region. Oil and gas activity is heterogeneous across the northern Gulf; most offshore platforms and pipelines are concentrated in the central and western Gulf along the coasts of Louisiana, Alabama, Mississippi, and Texas, while the eastern Gulf along the Florida coast remains undeveloped (Figure 1). Due to the substantial levels of oil and gas activity in the region, the northern Gulf also experiences both acute and chronic levels of oil pollution. While spills that are large in quantity and are spatially and temporally extensive tend to be the focus of most research, monitoring, and mitigation efforts, the many small spills that occur in the region also impact the estuarine, coastal, and marine habitats of the Gulf. For example, from 1972- to 2017, 149 spills occurred in the region with only eight of these classified as large (>1,000 barrels) spills (Absg Consulting Inc, 2018). The release of produced waters, which contain byproducts of oil extraction, are also a source of petrochemical pollution (Beyer et al., 2020). Between 2000 and 2015, ∼558 million barrels of produced oil were released annually into the northern Gulf (Bureau of Ocean Energy Management [BOEM], 2016). Furthermore, ∼1,600 natural oil seeps occur throughout the region, tending to be most common in the central area of the northern Gulf (O’Reilly et al., 2022). Given this extent of oil exposure, the impact of oiling and its resulting contamination, including PAHs has become a primary focus of research and management activities in the region (Fallon et al., 2018; Paruk et al., 2021; Polidoro et al., 2021; Michael et al., 2022; Woodyard et al., 2022).
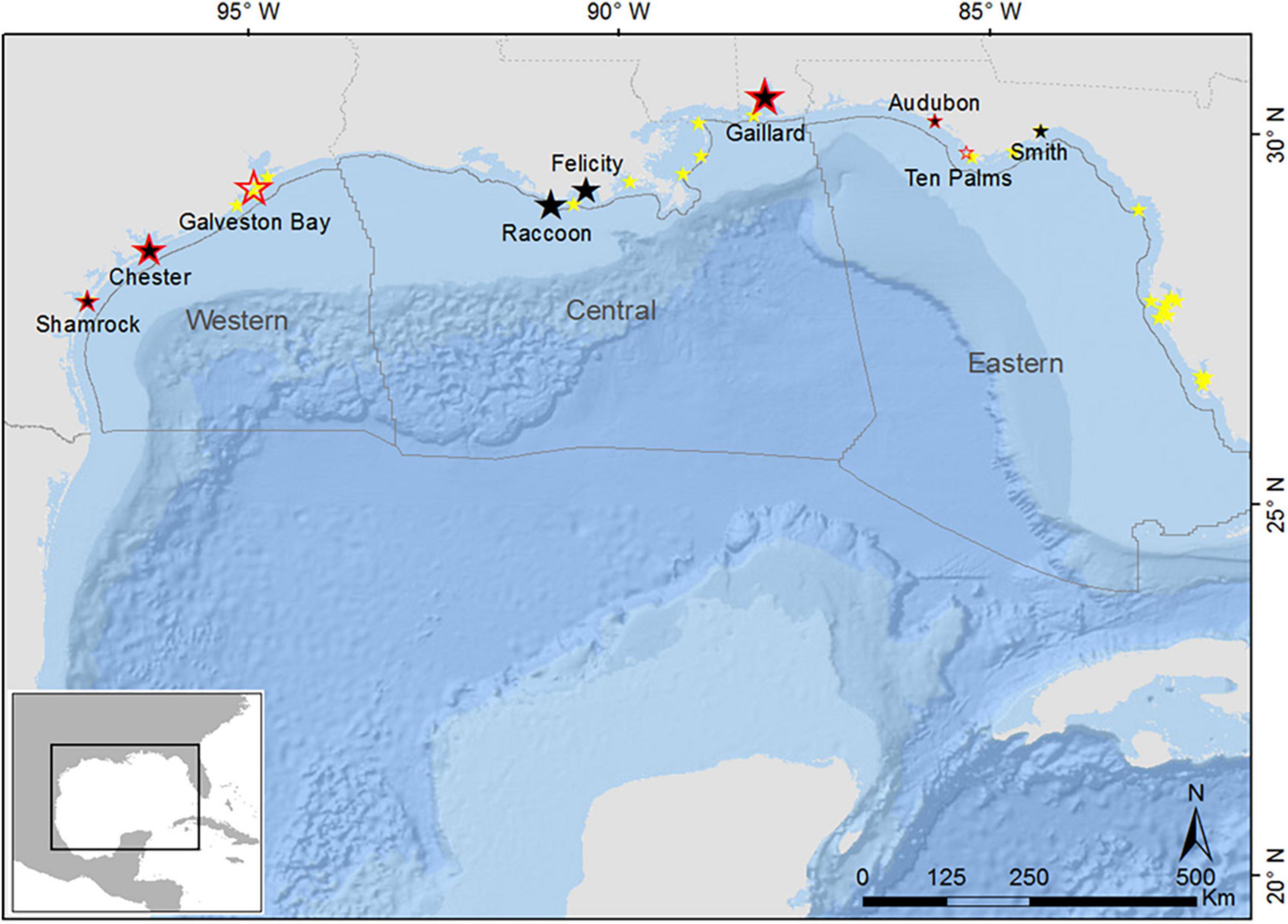
Figure 1. Location of Brown Pelican colonies in the northern Gulf of Mexico. Adults were sampled at colonies marked by a black symbol and chicks were sampled at colonies marked by a red-outlined symbol. Yellow symbols represent colonies not sampled. Size of symbol is relative to colony size (75–5,000 nesting pairs). The three planning areas of the Bureau of Ocean Energy Management (BOEM) are designated. Oil and gas activity is highest in the central, intermediate in the West, and least in the East (Base layer: ESRI, DeLorme, GEBCO, NOAA, NGDC, and other contributors).
The brown pelican (Pelecanus occidentalis) is a high-profile, nearshore seabird that breeds within the northern Gulf of Mexico and throughout the southeastern USA. Due to their documented sensitivity to contaminants and other environmental stressors (both natural and anthropogenic), brown pelicans are recognized as a high priority species for monitoring and research in the Gulf of Mexico (Jodice et al., 2019). For example, the species was reduced to near-extinction by exposure to dichlorodiphenyltrichloroethane (DDT) and other toxic contaminants during the mid-twentieth century (McNease et al., 1992) and continues to experience detrimental effects at the individual and population levels from oil exposure and other environmental stressors (U.S. Fish and Wildlife Service, 2011; Walter et al., 2013; Deepwater Horizon Natural Resource Damage Assessment Trustees, 2016; Fallon et al., 2018; Jodice et al., 2019). The species, therefore, has been and continues to be one of focus for assessing stressors in this geographic area (Jodice et al., 2019). As part of a larger effort to explore various aspects of the ecology and conservation of the species in the northern Gulf (Lamb et al., 2020), we set out to establish baseline levels of health and contaminant exposure for the species. Our prior research (Jodice et al., 2022) indicates that baseline indices of health and condition of pelicans do not consistently match the levels of oil and gas development in the breeding region, suggesting other factors played a role in the physiology of these individuals. PAH exposure could play an important role in mediating physiological parameters. Little is currently known, however, about underlying PAH levels in brown pelicans, nor how individual variability in movement may impact exposure. For example, home range sizes vary substantially across the northern Gulf during the breeding season and, because the species exhibits partial migration, the spatial extent of movements during the non-breeding season can vary greatly as well (Lamb et al., 2017b,2020).
In this paper, we expand on prior efforts to document baseline health and condition of brown pelicans in the northern Gulf of Mexico by examining the levels of PAH contamination in breeding adult pelicans and chicks across a gradient of oil and gas development within the northern Gulf. Because the amount of contamination and the repercussions of contact with a contaminant can differ with the route of contamination, we measured the levels of PAHs in the blood of adults and in the feathers of adults and chicks. Blood samples provide a recent assessment of exposure and represent substances currently circulating within an individual (Paruk et al., 2014, 2016). PAHs in blood likely derive from consumption of contaminated prey or ingestion of preen oil, while bioaccumulation through food webs is thought to be low (Paruk et al., 2014, 2016; Acampora et al., 2018). PAHs detected from adult feathers, however, may provide a different spatial, temporal, or source signature compared to PAHs detected in blood or other fluids (e.g., preen oil) that turnover relatively quickly (Jaspers et al., 2004; Acampora et al., 2018). For example, sources of PAHs in feathers may include blood supply (during feather growth), contact with the external environment, or contact with contaminated preen oil (Jaspers et al., 2004, Acampora et al., 2018). Feather samples provide an assessment of local and/or transboundary contamination in migratory species with a longer temporal signature compared to blood (Acampora et al., 2018). Multiple tissues also respond to different exposure pathways and offer a wider temporal frame of reference, with blood representing more recent exposure (e.g., contamination during the breeding season proximal to breeding colonies) and feathers representing longer term exposure (e.g., migratory or post-breeding locations).
We assessed PAH levels in relation to spatial and individual factors including colony location (adults and chicks), individual attributes (adults and chicks), home range size (adults), migration distance (adults), and select blood biochemistry parameters indicative of health and hepatic damage (adults and chicks). We included both PAR and ALK PAHs in our analyses because petroleum studies have traditionally focused on parent PAHs, and exposure can be underestimated if both PAR and ALK are not assessed (King et al., 2021). We report on the sum of PAHs and not the detection levels of each individual PAH in each individual because our focus was on the total tissue burden among individuals and not necessarily the exact exposure for each individual PAH. By taking this approach, we sought to better understand the PAH tissue burden in brown pelicans throughout the region, which could subsequently enhance our ability to improve targeted mitigation efforts by linking exposure pathways across nesting, foraging, and non-breeding habitats. Ultimately, these data may improve our ability to predict which portions of the Gulf-wide metapopulation are likely to be affected by future contamination events.
Materials and methods
Ethics statement
This study is one component of a broader research program that was focused on the ecology of Brown Pelicans in the northern Gulf of Mexico (Lamb et al., 2020; Jodice et al., 2022). Research was authorized by permits from the Clemson University Animal Care and Use Committee (2013-026), U.S. Geological Survey Bird Banding Lab (#22408), Texas Parks and Wildlife (SPR-0113-005), Audubon Texas, The Nature Conservancy in Texas, Louisiana Department of Wildlife and Fisheries (LNHP-13-058 and LNHP-14-045), and the Florida Fish and Wildlife Conservation Commission (LSSC-13-00005).
Study area
Study sites extended from the Florida panhandle to the Texas coast and represent each of the three planning areas designated by the Bureau of Ocean Energy Management (BOEM; the federal agency responsible for managing oil and gas activities in U.S. waters). These are defined as the Eastern Planning Area (EPA), Central Planning Area (CPA), and Western Planning Area (WPA). The CPA contains the highest level of oil and gas infrastructure (platforms and pipelines) among the three, while the WPA is moderately developed and the EPA is undeveloped. We collected samples from nine sites throughout the study area (Figure 1), although we did not sample both age classes or both tissue types from all sites (Table 1). We sampled adults from seven colonies; Audubon and Smith islands, Florida (EPA); Gaillard Island, Alabama (CPA); Felicity and Raccoon islands, Louisiana (CPA); and Chester and Shamrock islands, Texas (WPA) (Figure 1). We sampled chicks from seven colonies: Audubon and Ten Palms islands, Florida (EPA); Gaillard Island, Alabama (CPA); Marker 52 and North Deer (regrouped as Galveston Bay colonies), Chester, and Shamrock islands, Texas (WPA). Data were analyzed at the spatial scale of the planning areas (i.e., not at the colony level) due to limited samples from some colonies. All study colonies and planning areas were, however, within a single marine ecoregion (Northern Gulf of Mexico within the Temperate North Atlantic Realm: Spalding et al., 2007).
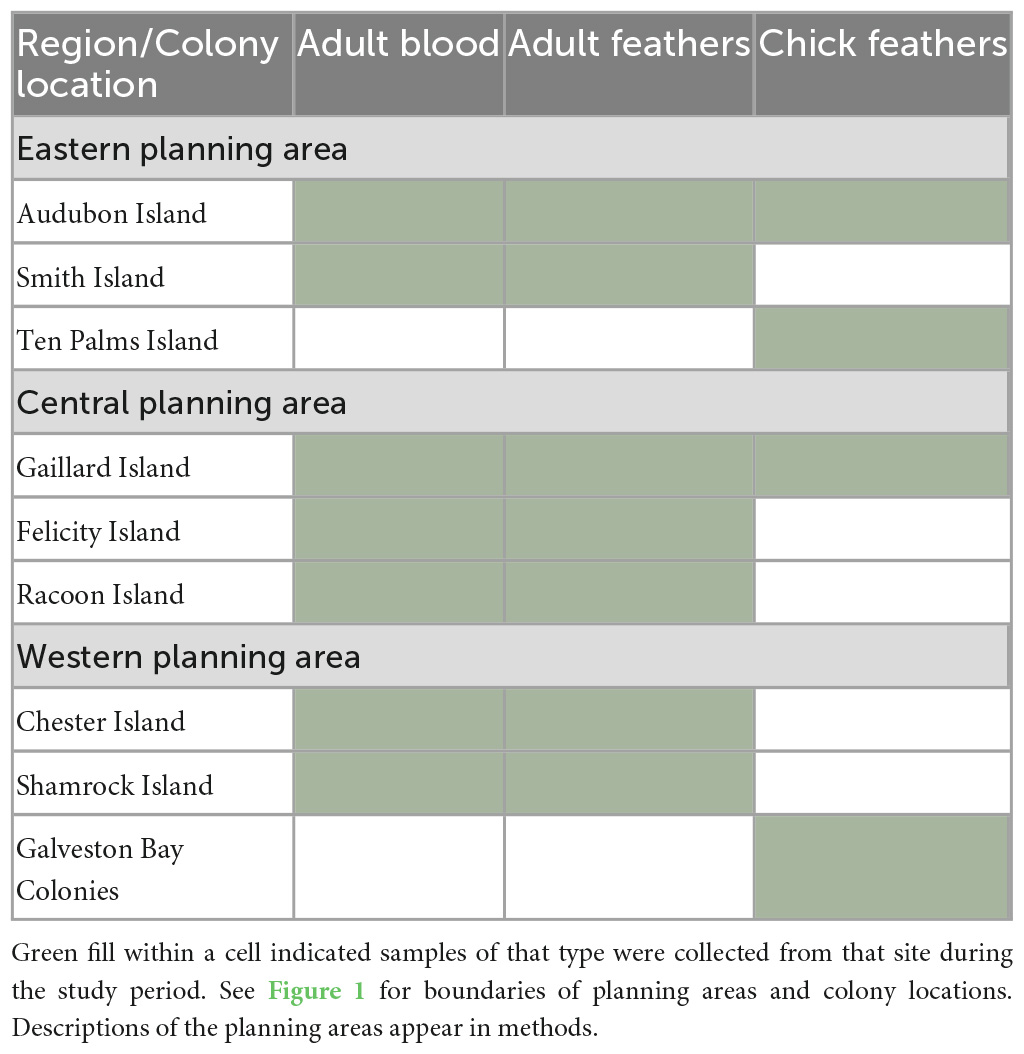
Table 1. Study sites sampled for polycyclic aromatic hydrocarbons (PAHs) in adult and chick brown pelicans from breeding colonies in the northern Gulf of Mexico, 2013–2015.
Sampling
Adult brown pelicans were sampled from active nests during the breeding seasons of 2013–2015. Adults were captured on nests using leg nooses during the late incubation and early chick-rearing stages. If eggs were present in the nest, they were replaced with porcelain eggs during capture to prevent damage. If chicks were present, they were moved to the nest edge to avoid injury. Median handling time was 17.5 min (range = 11–35 min) from capture to release. After release, we observed individuals for several minutes to ensure that they displayed normal flight, swimming, and balance capabilities. Observation methods and results are described in detail in Lamb et al. (2020). We collected 3–4 scapular feathers from 92 breeding adults. We collected blood samples within 2 min of capture from the tarsometatarsal vein. After sterilizing the collection site, we collected a 5 mL blood sample using a 23-gauge needle and VacuTainer tube (Becton Dickinson, Franklin Lakes, NJ, United States) with lithium heparin anticoagulant. We stored samples over cold packs until returning from the field (∼5–10 h), after which we centrifuged samples, separated red blood cells from plasma, and froze samples for storage until lab analyses could be completed. For PAH analysis, we included all adult feather samples (N = 92) as well as red blood cell samples from up to five randomly selected individuals per colony (N = 33).
Pelican chicks were captured by hand at or near nest sites. We collected ∼4 scapular feathers from 606 brown pelican chicks during the breeding season of 2014–2015 of which we randomly selected five individuals per colony (N = 35) for PAH analysis. Since feathers in young chicks represent approximately the same exposure period as blood samples (i.e., the weeks preceding capture), we did not collect blood from chicks for PAH analysis.
For adults and chicks, we measured body mass (±50 g), culmen length (±1 mm), tarsus length (±1 mm), and wing length (±5 mm) and created a body condition index (BCI) specifically for the individuals used in this study. The BCI provides an index for the mass of the bird in relation to its size and is calculated as the residual of the linear relationship between mass and culmen length (Lamb et al., 2017a). In brown pelicans, sex cannot be easily determined in situ. Therefore, the distribution of samples between sexes is opportunistic. Sex of adults was determined from collected blood samples through PCR amplification of sex-linked molecular markers (Itoh et al., 2001). We did not sex chicks.
Sample processing
Analyses of polycyclic hydrocarbons were conducted at the University of Connecticut Center for Environmental Sciences and Engineering (Storrs, Connecticut). In the lab, we weighed 0.2 g of blood sample into a 1.5 mL plastic centrifuge tube. Samples were spiked with quality control standard solutions and vortexed for 1 min at 2,500 rounds per minute. Methanol or acetonitrile (500 μL) were added to each tube along with MgSO4. Samples were then vortexed for 5 min at 2,500 rounds per minute, then centrifuged for 10 min at 14,000 rounds per minute. Next, 190 μL of the supernatant were transferred to a 300 μL liquid-chromatography vial. These samples were then spiked with an internal standard and vortexed.
Following extraction, the samples were analyzed for alkylated PAHs using an Agilent 6890 gas chromatograph (Agilent Technologies Inc., Santa Clara, CA, United States) equipped with a Restek Rxi-5Sil MS column (Restek, Bellefonte, PA, USA; 30 m) using splitless injection coupled to a Waters QuattroMicro triple quadrupole mass spectrometer (Waters Corporation, Milford, MA, USA). Parent PAHs were quantified using a Waters Acquity ultra-performance liquid chromatograph (UPLC; Waters Corporation, Milford, MA, USA) with fluorescence and photo diode array detection, which was equipped with an Acquity UPLC BEH C18 column (Waters Corporation, Milford, MA, USA; 1.7 μm, 2.1 × 100 mm). All peaks were quantified against the internal standard, and the extraction efficiency was evaluated using a surrogate standard of naphthalene-d8. Standard quality assurance procedures were employed, including analysis of duplicate samples, method blanks, post-digestion spiked samples, and laboratory control samples.
Feathers were washed three times in acetone, three times in high performance liquid chromatography water, and one additional time in acetone before allowing them to dry overnight (ca. 10 h). Feathers were weighed (±0.2 g) on folded weighing paper and transferred directly into the accelerated solvent extraction (ASE) cell using forceps when needed. Hydromatrix powder was added to pack the 11 mL ASE cells. Using gelatin as the matrix for the blank and laboratory control samples, a 0.2 g sample was weighed out and transferred to ASE cells. Samples were then spiked with quality control standards. ASE extracts were subsequently run and collected utilizing acetonitrile solvent, and the solution was transferred into the pre-marked conical evaporation vials and evaporated to just below 0.5 mL under a gentle nitrogen stream (set flowrate on N-Evap unit to 180 mL/min). Samples were spiked again with internal standard. The volume was then brought up to 500 μL with acetonitrile and vortexed for a few seconds to mix. Filtered samples were injected into liquid-chromatography vials using 1 mL plastic syringes and 4 mm, 0.2 μm syringe filter. The detection limit for all PAHs was 5 ng g–1 (i.e., parts per billion) and values for PAHs were reported as wet weight for blood and dry weight for feathers.
Statistical analyses
We assessed the concentration of PAHs in adult blood, adult feathers, and chick feathers via three dependent variables: the sum of all PAHs detected (sumPAH), the sum of Parent PAHs detected (sumPAR), and the sum of Alkylated PAHs detected (sumALK). We assessed the relationship for each with a suite of independent variables, including: BCI (adults and chicks; continuous), planning area (adults and chicks; WPA, CPA, or EPA with EPA set as the reference level), sex (adults; male of female), migration class (adults; categorical, see below) and home range size (adults; continuous). Methods for deployment of satellite tags, calculation of home range size, and calculation of migration distance are detailed in Lamb et al. (2017a,b, 2020). Home range was reported as the area of the 95% kernel density contour for any individual from which a sample was collected that was also equipped with a satellite transmitter (n = 64). We selected the 95% use area for PAH analysis rather than a core use area (e.g., 25 or 50%) because we assumed that exposure to PAHs could occur anywhere within an individual’s habitat regardless of intensity of use. Migration distance was calculated as the distance between the center of breeding home range and the center of winter home range as determined from locations of satellite tagging (Lamb et al., 2017b,2020), and classified as short (i.e., resident: < 200 km), medium (200–800 km), and long (>800 km). The migration class defined as long-distance was set as the reference level.
Because the concentration of PAHs was below detectable limits for many samples from both age classes and sample types (i.e., a high proportion of 0’s in the data set, see Results), we analyzed our data using a hurdle model approach. A hierarchical hurdle model approach allows for the consideration of two ecological processes, one that may affect the occurrence of an exposure to a PAH contaminant (i.e., presence/absence) and another that may affect the dose or concentration of the PAH contaminant (i.e., abundance) (Zuur et al., 2009). For step one of the hurdle approach we used a binomial logistic regression with a log link function, using the presence (value > 0) or absence (value = 0, or undetectable) of each of the three PAH variables (sumPAH, sumPAR, sumALK) as the response variable. For step two of the hurdle approach we used a generalized linear model (GLM) to assess the change in the concentration of PAHs only in tissue samples where PAHs exceeded detectable limits. We used a GLM with a gamma distribution and a log link function, with the sum of the concentration of each of the three PAH variables as the response variable.
We used the same set of candidate models in each of the two steps of the hurdle approach and compared the strength of the candidate models using Akaike’s Information Criteria (AIC; Table 2). We built 12 models for analysis of adult blood samples, 16 models for adult feather samples, and three models for chick feather samples. Variables that were correlated were not included within the same model but each independent variable appeared in at least one model. We reported coefficient estimates from the top performing models if no other model was within two points of the model with the lowest AIC value, or averaged coefficient estimates if multiple models were supported (delta AIC ≤ 2.0 relative to the lowest AIC value, n = 2 cases). We provided odds ratios and confidence intervals on odds ratios for coefficient estimates (±SE) from binomial logistic models of PAH detection (the odds of a PAH being detected for a change in categorical levels, or for a one unit increase in a continuous variable) and from gamma models of PAH concentration where present (the odds of the concentration of a PAH increasing by 1 ng g–1 for a change in categorical levels, or for a one unit increase in a continuous variable).
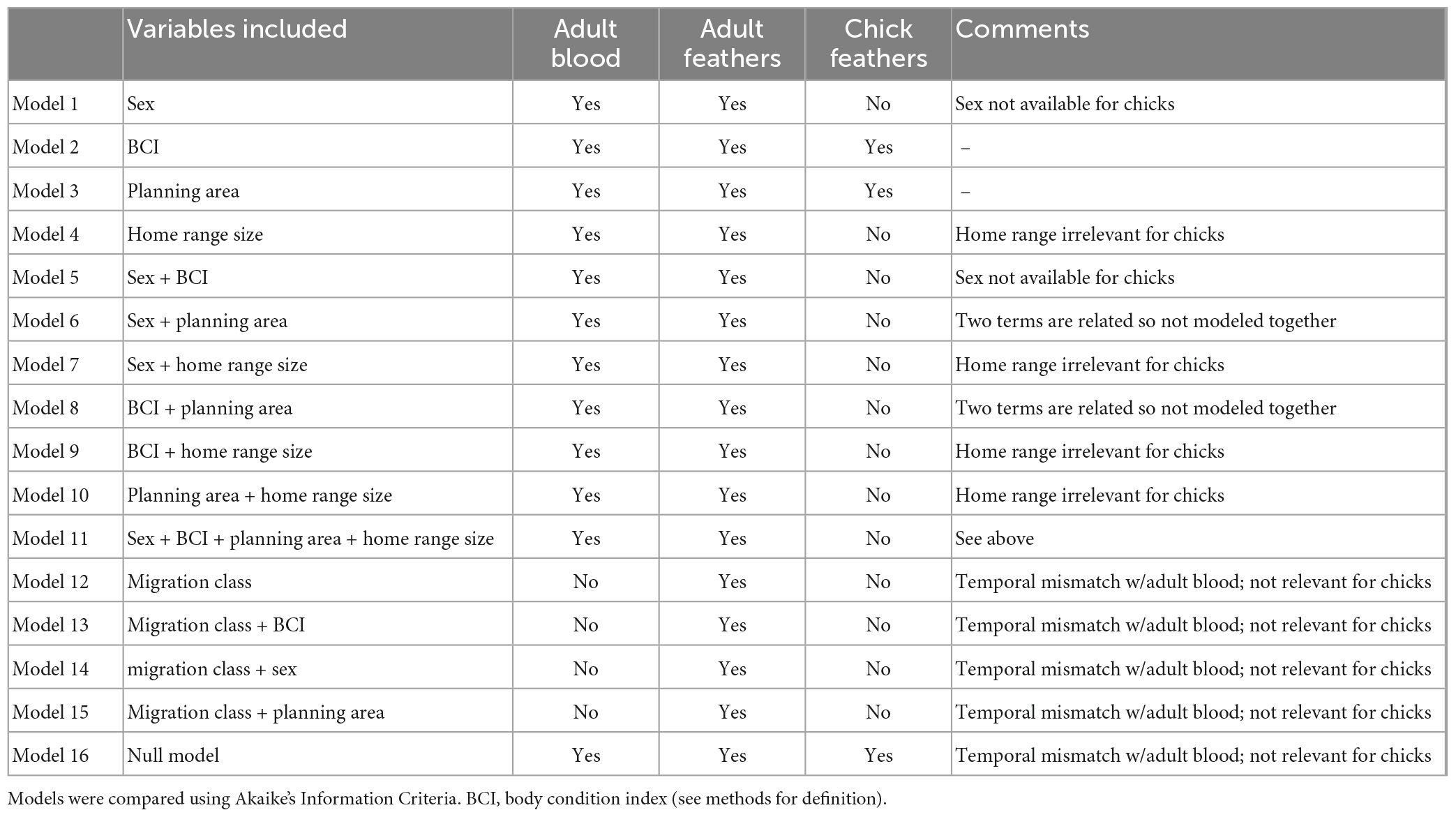
Table 2. Models used in an information theoretic approach to assess relationships among polycyclic aromatic hydrocarbons (PAHs) and independent variables for brown pelican adults and chicks sampled from breeding colonies in the northern Gulf of Mexico, 2013–2015.
Because exposure to PAHs can lead to sublethal effects, we also assessed the correlation between levels of exposure to all PAHs (sumPAH) and attributes that may reflect individual condition or health. We collected blood samples to assess hematological and physiological parameters from many of the same birds from which PAH was measured as part of a component study among these same pelican colonies (Jodice et al., 2022). We assessed the correlation of PAH exposure with total protein (g dL–1), corticosterone (mg dL–1), packed cell volume (PCV; %), heterophil count (10–3 ml–1), lymphocyte count (10–3 ml–1), and the ratio of heterophils to lymphocytes (H:L). We also assessed correlations with AST and CPK, two enzymes indicative of hepatic damage (Harr, 2002; Alonso-Alvarez et al., 2007). Laboratory methods for the measurement of these attributes are described fully in Jodice et al. (2022). Because the distribution of the sumPAH data was non-normal and not able to be transformed to a normal distribution, we used Kendall’s tau to perform a non-parametric correlation. We correlated physiological markers with sumPAH in adult blood, sumPAH in adult feathers, and sumPAH in chick feathers.
Results
PAH profiles in adult blood
We analyzed blood for PAHs from 33 adult pelicans (Table 3). The most frequently occurring PAHs were of intermediate molecular weight (Figure 2). We failed to detect two alkylated compounds and eight parent compounds out of the full test set. The two most frequently detected PAHs were 1,3-dimethylnaphthalene and 2,3,5-trimethylnaphthalene. Of the 24 PAHs assessed, 14 were detected in at least one individual (Table 3). Alkylated PAHs were detected in 48% of individuals and parent PAHs were detected in 30% of individuals. Three alkylated compounds were each detected in >10% of individuals; 2,6-dimethyl naphthalene (n = 4), 1,3-dimethylnaphthalene (n = 5), and 2,3,5-trimethylnaphthalene (n = 6). Of 33 birds sampled, 21 (63.6%) had at least 1 PAH detected (1 PAH: n = 10; 2 PAHs: n = 10; four PAHs: n = 1). Parent compounds were detected in 10 of 33 birds, alkylated compounds were detected in 16 of 33 birds, and both parent and alkylated PAHs were detected in 5 of 33 birds. PAHs were detected in 75% of females sampled and in 57% of males sampled.
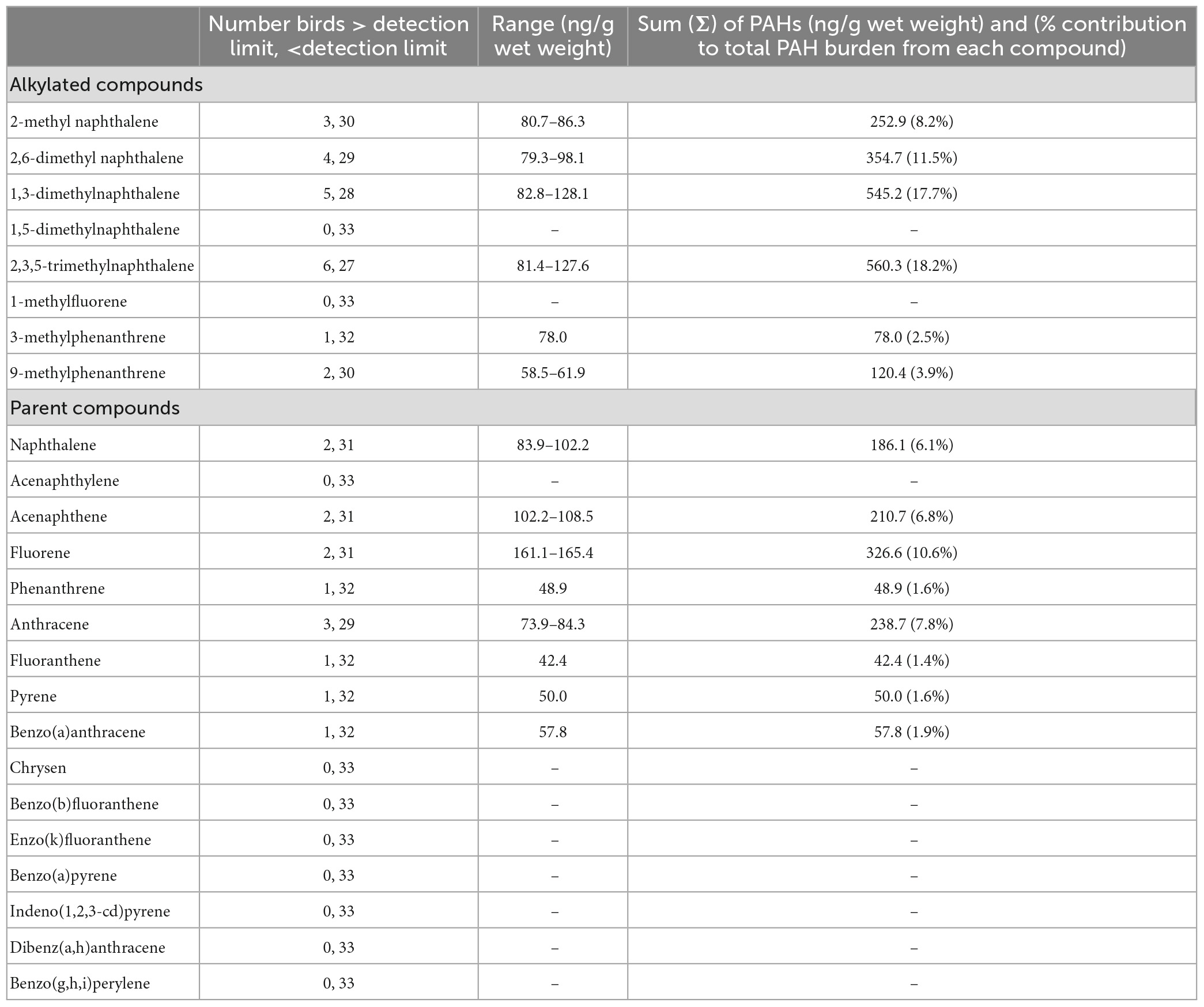
Table 3. Frequency of detection, range and sum of concentration (ng/g wet weight), and percent contribution to total burden of individual polycyclic aromatic hydrocarbons (PAHs) from blood of adult brown pelicans (n = 33) breeding in the northern Gulf of Mexico, 2013–2014.
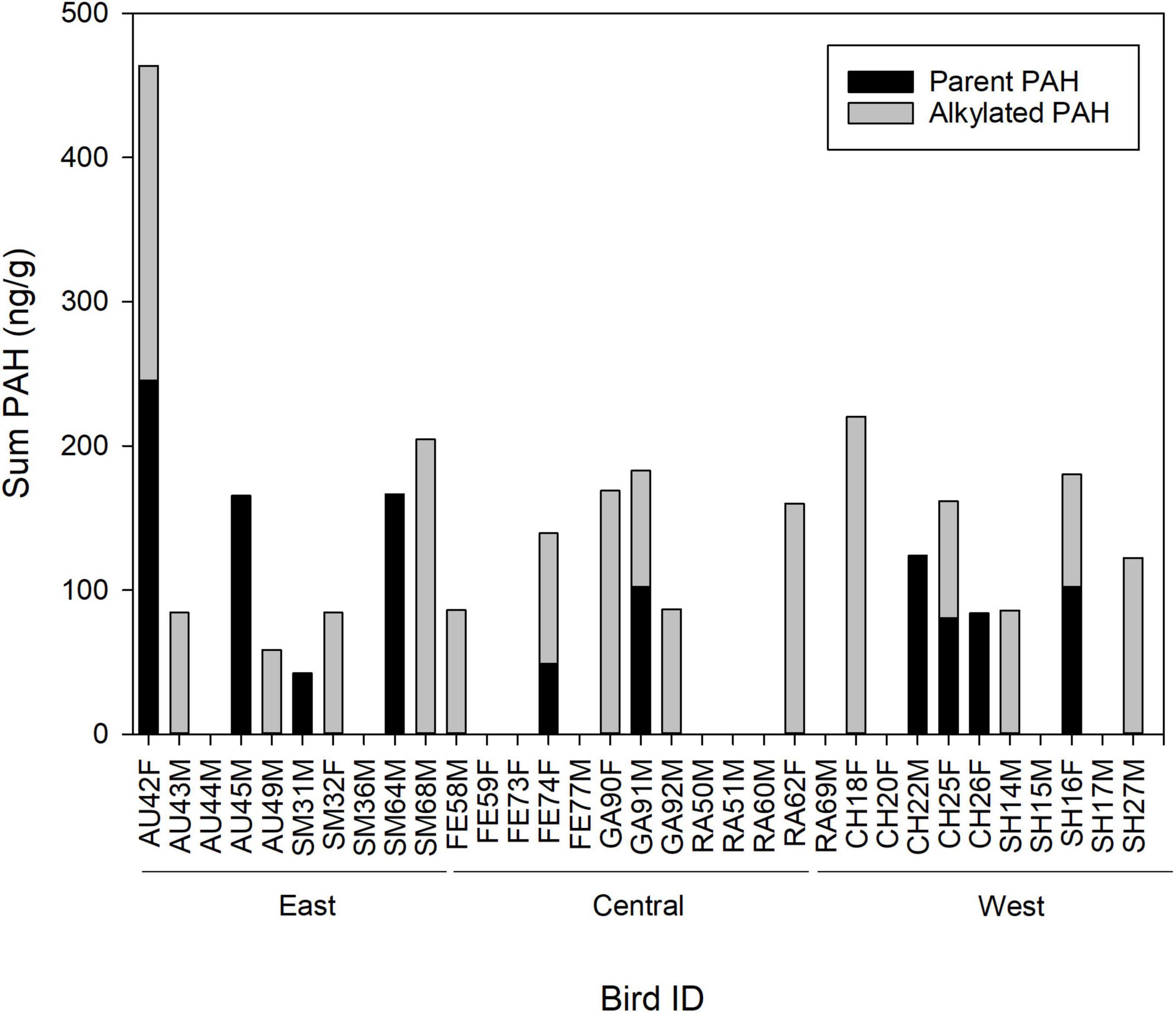
Figure 2. Concentration (ng g–1; wet weight) of parent and alkylated polycyclic aromatic hydrocarbons (PAHs), and the sum of parent plus alkylated PAHs, in blood samples of adult brown pelicans breeding in the northern Gulf of Mexico, 2013–2015. Bird ID, two letter abbreviation for the colony of origin of the sample (AU, Audubon Island; SM, Smith Island; FE, Felicity Island; GA, Gaillard Island; RA. Raccoon Island; CH, Chester Island; SH, Shamrock Island; see Figure 1), a unique identification number, and F, Female or M, Male.
Among adult pelicans with PAH concentrations above detectable limits, the sum of all PAHs ranged from 42.44 to 463.75 ng g–1, the sum of alkylated PAHs ranged from 58.46 to 220.51 ng g–1, and the sum of parent PAHs ranged from 42.44 to 245.47 ng g–1 in blood samples (Figure 2). There was no significant difference in the concentration of parent compared to alkylated PAHs in blood samples from adults using either the full data set or using only data from birds above detectable limits (Wilcoxon rank sum test P > 0.13 for each). When all individuals were considered, there was no correlation between summed concentrations of alkylated and parent PAHs (Kendall tau r = −0.05, Pearson r = −0.09). Similarly, when only individuals with detectable limits of PAHs were considered, there was no correlation between summed concentrations of alkylated and parent PAHs (Pearson r = 0.25). The individual with the highest concentration of summed parent PAHs (245.47 ng g–1) did, however, present with the second highest level of alkylated PAHs (218.28 ng g–1; female, Audubon Island, Florida). The PAH with the highest concentration detected was fluorene (165.4 and 161.1 ng g–1; both birds from Audubon Island, Florida). The two most frequently detected PAHs (1,3-dimethylnaphthalene and 2,3,5-trimethylnaphthalene) also had the widest range in measured concentrations (∼80∼28 ng g–1; Table 3).
Five models for the presence of sumPAH in pelican blood received support, and these included each of the main variables we assessed except planning area (Supplementary Table 1). There was little separation among these five models, and the most supported model only carried an AICc weight = 0.21. The only variable that appeared to have a measurable relationship with sumPAH was sex (model averaged coefficient estimate = −1.12 ± 0.95). Females were 3.1 times more likely to be detected with a PAH compared to males although there was substantial variability around the estimate (90% CI for odds ratio = 0.7, 16.7). The concentration of sumPAH in pelican blood was best described by a single model that included sex and home range size (Supplementary Table 1). There was substantial separation among the first and second ranked model, and the most supported model carried 90% of the AICc weight. There was a strong relationship between sumPAH concentrations and sex (coefficient estimate = −0.73 ± 0.23). The odds of the sumPAH concentration increasing by 1 ng g–1 increased by 2.1 times for females compared to males (90% CI for odds ratio = 1.4, 3.0). There was a weak negative relationship between sumPAH concentrations and home range size which was strongly leveraged by a single individual and therefore discounted (coefficient estimate = −0.00022 ± 0.000099).
Three models for the presence of sumPAR in pelican blood received support, and these included the variables home range, sex, and BCI (Supplementary Table 2). There was little separation among these three models, with the most supported model only carrying an AICc weight = 0.28. Sex was weakly associated with sumPAR (coefficient estimate = −0.94 ± 0.89). Females were approximately 2.5 times more likely to be detected with a PAR PAH compared to males, although there was substantial variability around the estimate (90% CI for odds ratio = 0.6, 11.1). Coefficient estimates for home range size and BCI had standard errors that overlapped zero indicating little evidence of a statistical relationship. Four models for the concentration of sumPAR in pelican blood received support, and these included each of the main variables we assessed (Supplementary Table 1). There was little separation among these four models, and the most supported model carried an AICc weight = 0.38. The only variable with a measurable, yet weak, effect on sumPAR was planning area. Coefficient estimates indicated that the concentrations of sumPAR were lower in the CPA compared to the EPA (coefficient estimate = −0.70 ± 0.41; Figure 3). The odds of the sumPAR concentration increasing by 1 ng g–1 increased by 2.0 times for birds in the EPA compared to the CPA, although there was substantial variability around the estimate (90% CI for odds ratio = 1.0, 4.0).
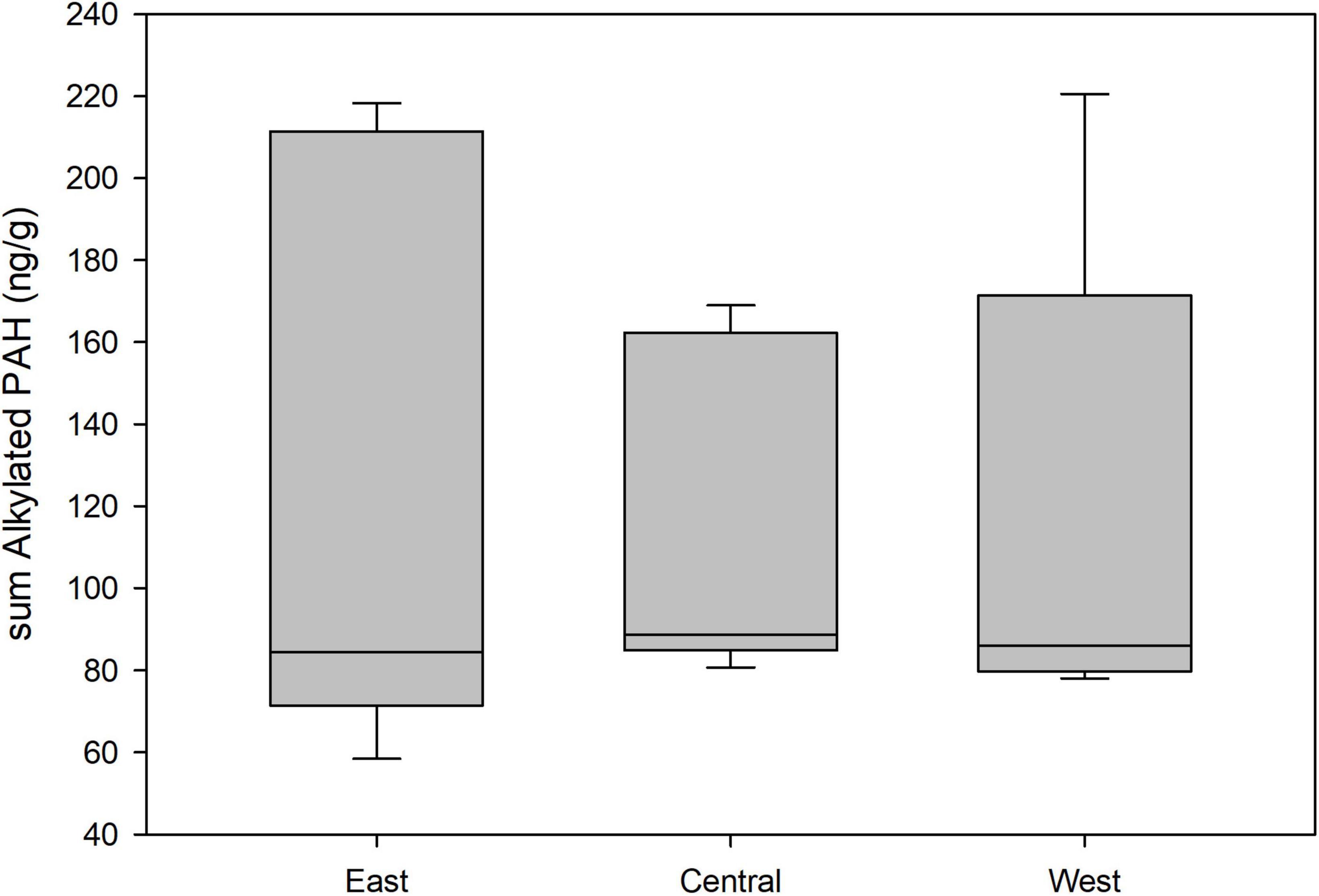
Figure 3. Concentrations (ng g–1; wet weight) of alkylated polycyclic aromatic hydrocarbons (PAHs) by planning area from blood samples of adult brown pelicans breeding in the northern Gulf of Mexico, 2013–2015. Only samples above detectable limits are included.
Two models for the presence of sumALK in pelican blood received support, and these included the variables sex and home range size (Supplementary Table 3). There was little separation among these two models, and the most supported model was 1.5x as likely to be the best model compared to the second-ranked model. Sex had a measurable relationship with sumALK (coefficient estimate = −1.45 ± 0.91) while the coefficient estimate for home range had standard errors that overlapped zero indicating no relationship. Females were 4.3 times more likely to be detected with an ALK PAH compared to males, although there was substantial variability around the estimate (90% CI for odds ratio = 1.1, 25.0). Only the full model for the concentration of sumALK in pelican blood, which included each main variable and carried an AICc weight = 0.86, received support (Supplementary Table 3). Coefficient estimates indicated that the concentrations of ALK PAHs were likely to decrease in the CPA compared to the EPA (coefficient estimate = −0.36 ± 0.24; Figure 3), decrease in males compared to females (coefficient estimate = −0.67 ± 0.21), decrease with an increase in BCI (coefficient estimate = −0.0011 ± 0.0004), and decrease with an increase in home range size (coefficient estimate = −0.00019 ± 0.00011). The odds of the sumALK concentration increasing by 1 ng g–1 increased by 1.4 times for birds in the EPA compared to the CPA (90% CI for odds ratio = 1.0, 2.2), and increased by 1.9 times for females compared to males (90% CI for odds ratio = 1.4, 2.8). The odds of a change in sumALK for BCI and home range did not differ from 1.0 even when each independent variable was scaled up one order of magnitude.
We found a positive correlation between sumPAH in adult blood with total protein (tau = 032, p = 0.02) and with lymphocyte count (tau = 0.25, p = 0.08). All other correlations with hematological variables were not significant.
PAH profiles in adult feathers
We analyzed feathers for PAHs from 92 adult pelicans. The occurrence and concentrations of each PAH are summarized in Table 4 and reported as dry weight (ng g–1). The most frequently occurring PAHs were of intermediate molecular weight. The PAHs with the highest concentrations detected were 1,3-dimethylnaphthalene (256.2 and 221.8 ng g–1; both birds from Felicity Island, Louisiana) and 2-methyl naphthalene (229.0 ng g–1; Shamrock Island, Texas). All of the alkylated compounds were detected in ≥2 birds while nine parent compounds were not detected. 1,3-dimethylnaphthalene, the most frequently detected PAH, also had the widest range in measured concentration (∼72–∼256 ng g–1; Table 4). Of the 24 PAHs assessed, 15 were detected in at least one individual. Alkylated PAHs were detected in 46% of individuals and parent PAHs were detected in 26% of individuals. Three alkylated compounds were detected in >10% of individuals [2,6-dimethyl naphthalene (n = 10), 1,3-dimethylnaphthalene (n = 11), and 2,3,5-trimethylnaphthalene (n = 10)]. Of 92 birds sampled, 56 (60.8%) had at least 1 PAH detected (1 PAH: n = 39; 2 PAHs: n = 13; 3 PAHs: n = 2; 4 and 6 PAHs: n = 1). Parent compounds were detected in 24 of 92 birds, alkylated compounds were detected in 42 of 92 birds, and both parent and alkylated PAHs were detected in 10 of 92 birds. PAHs were detected in 67% of females sampled and in 55% of males sampled.
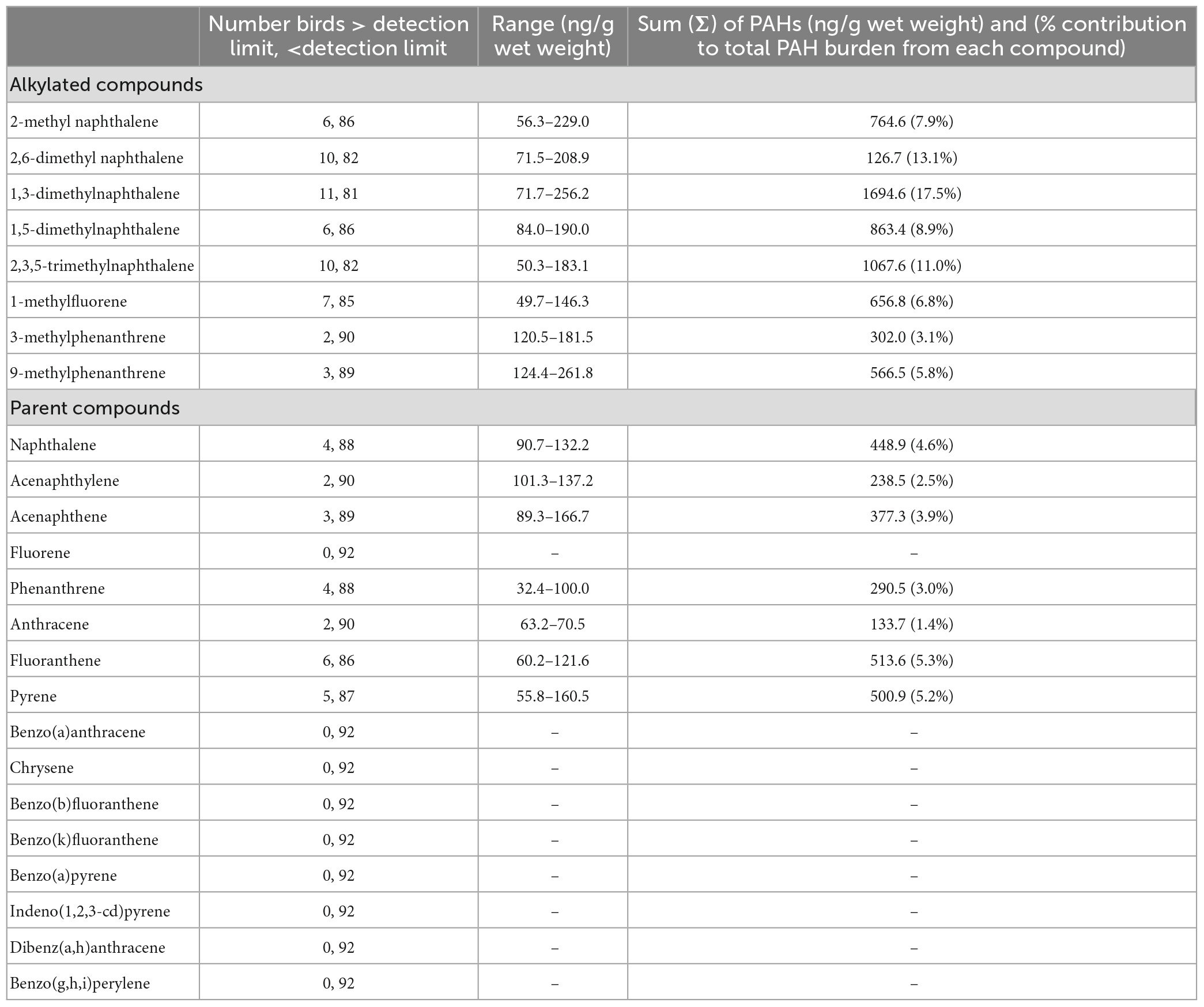
Table 4. Frequency of detection, range and sum of concentration (ng/g wet weight), and percent contribution to total burden of individual polycyclic aromatic hydrocarbons (PAHs) from feathers of adult brown pelicans (n = 92) breeding in the northern Gulf of Mexico, 2013–2014.
Among adult pelicans with detectable limits of PAHs, the sum of all PAHs ranged from 60.20 to 623.80 ng g–1, the sum of alkylated PAHs ranged from 71.50 to 479.00, and the sum of parent PAHs ranged from 32.40 to 166.70 in feather samples (Figure 4). The mean (±SE) of the sum of alkylated PAHs (78.08 ± 10.94) was greater than the sum of parent PAHs (27.21 ± 5.13) for the full data set (i.e., including samples with no detectable PAHs; W = 3135.5, p-value = 0.0004). The mean (± SE) of the sum of alkylated PAHs (171.03 ± 9.41) was greater than the sum of parent PAHs (104.30 ± 3.62) for the subset of samples above detectable limits (W = 235.5, p-value = 0.0003). When all individuals were considered, there was no correlation between summed concentrations of alkylated and parent PAHs (Kendall tau r = −0.06, Pearson r = −0.03). When only individuals with detectable limits of PAHs were considered, there was a moderate positive correlation between summed concentrations of alkylated and parent PAHs (Pearson r = 0.57).
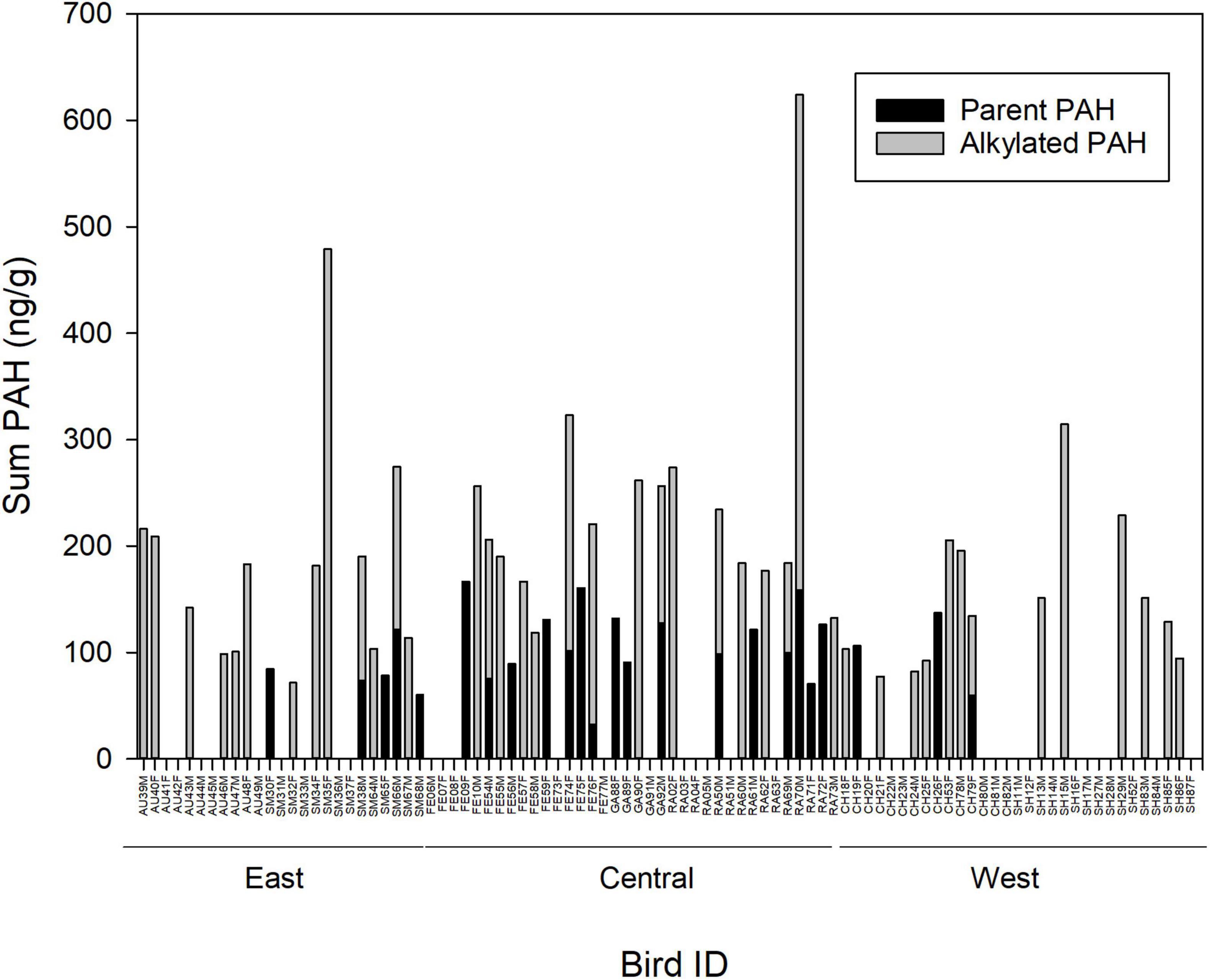
Figure 4. Concentration (ng g–1; dry weight) of parent and alkylated polycyclic aromatic hydrocarbons (PAHs), and the sum of parent plus alkylated PAHs, in feather samples of adult brown pelicans breeding in the northern Gulf of Mexico, 2013–2015. Bird ID, two letter abbreviation for the colony of origin of the sample (AU, Audubon Island; SM, Smith Island; FE, Felicity Island; GA, Gaillard Island; RA, Raccoon Island; CH, Chester Island, SH, Shamrock Island; see Figure 1), a unique identification number, and F, Female or M, Male.
One model for the presence of sumPAH in pelican feathers received support, and it included BCI and migration class (Supplementary Table 4). This two-variable model carried 63% of the AIC weight and was 3x as likely to be the best model compared to the second ranked model (which was a single variable model for migration class). Both BCI (coefficient estimate = −0.002 ± 0.001) and migration class (coefficient estimates: medium −1.58 ± 0.77, short −1.38 ± 0.76) appeared to have a measurable relationship with sumPAH. The odds of a bird having a PAH increased 1.002 times (90% CI for odds ratio = 1.0006, 1.004) for every unit decrease in BCI (i.e., 1.2 times for every 100 unit decrease in BCI). The odds of a bird having a PAH decreased 4.8 times for medium v. long distance migrants (90% CI for odds ratio = 1.4, 20.0) and decreased 4.0 times for short v. long distance migrants (90% CI for odds ratio = 1.2, 16.7). One model for the concentration of sumPAH in pelican feathers received support, and it included BCI and migration class (Supplementary Table 4). Although the best-performing model carried 94% of the AIC weight, neither BCI nor migration class had a measurable effect on the concentration of sumPAH (i.e., SE > coefficient estimate).
Three models for the presence of sumPAR in pelican feathers received support (Supplementary Table 5). The top ranked model carried 31% of the AIC weight and was 1.2 times as likely to be the best model compared to the second ranked model. Home range size appeared to have a measurable relationship with sumPAR (coefficient estimate = 0.00081 ± 0.0003). The odds of a bird having a PAR increased 1.08 times for every 100 km2 unit increase in home range. There was a marginal increase in the odds of a bird having a PAR PAH for females compared to males (2.4 times, 90% CI for odds ratio = 0.8, 7.1). The odds of a bird having a PAR PAH decreased 4.3 times for medium compared to long distance migrants (90% CI for odds ratio = 1.4, 20.0). The odds of a bird having a PAR PAH increased 4.4 times for birds in the CPA compared to the EPA (90% CI for odds ratio = 1.3, 15.9). One model for the concentration of sumPAR in pelican feathers received support, and it included migration class and BCI (Supplementary Table 5). This two-variable model carried 70% of the AIC weight and was 4.4x as likely to be the best model compared to the second ranked model which was >2 AIC points removed. Only migration class (coefficient estimates: medium −0.31 ± 0.21, short −0.39 ± 0.15) had a measurable relationship with sumPAR (Figure 5). The odds of the sumPAR concentration increasing by 1 ng g–1 increased by 1.4 times for birds in the long-distance compared to medium-distance migrant class (90% CI for odds ratio = 0.9, 2.0) while the odds of the sumPAR concentration increasing by 1 ng g–1 increased by 1.5 times for birds in the long-distance compared to short-distance migrant class (90% CI for odds ratio = 1.1, 1.9).
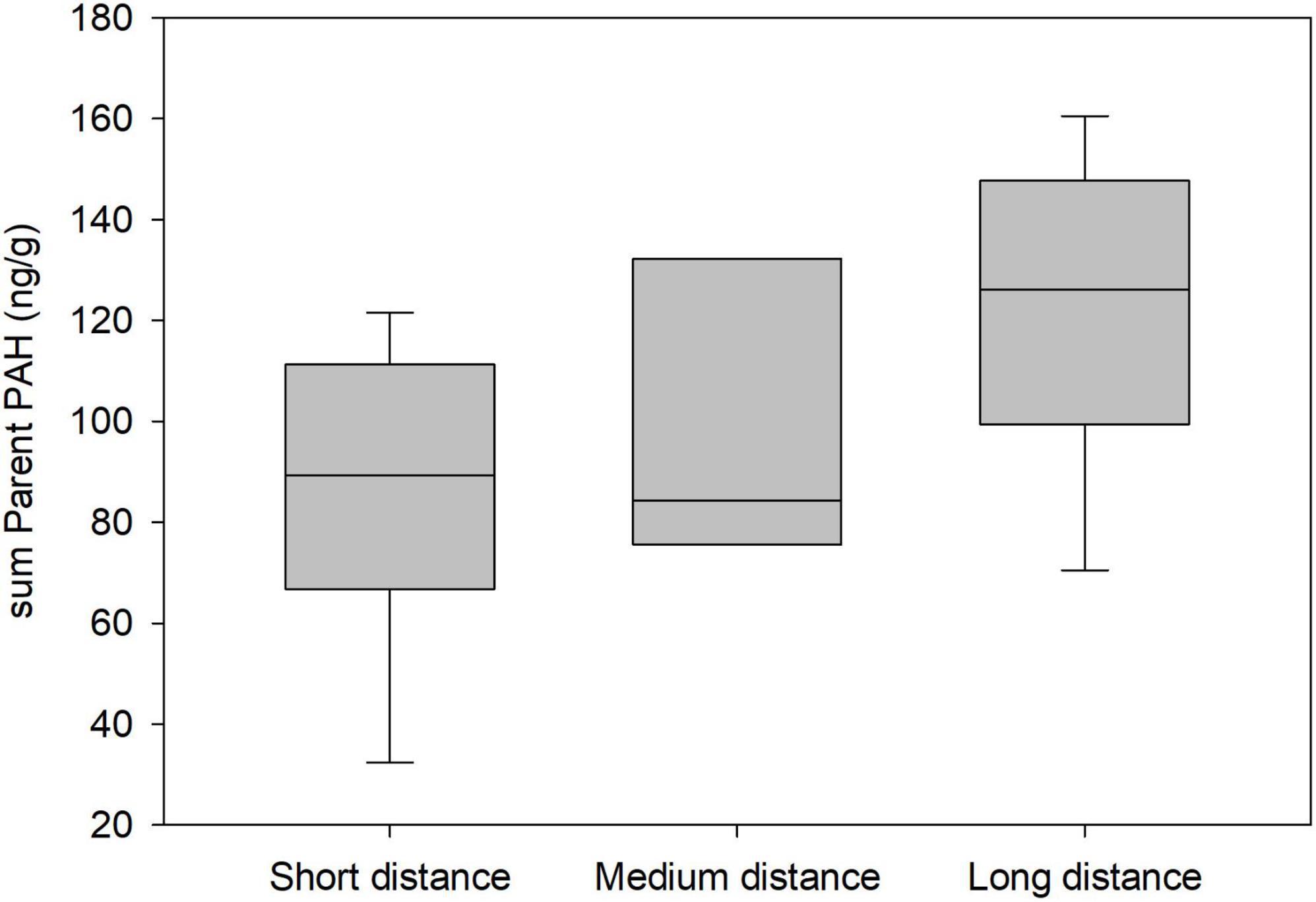
Figure 5. Concentrations (ng g–1 dry wet weight) of Parent polycyclic aromatic hydrocarbons (PAHs) by migration class from feather samples of adult brown pelicans breeding in the northern Gulf of Mexico, 2013–2015. Only samples above detectable limits are included.
Two models for the presence of sumALK received support, and both included migration class (Supplementary Table 6). The first-ranked model carried 68% of the model weights. This top ranked model included migration class and BCI and was 2.6 times as likely to be the best model compared to the second ranked model which included only migration class. BCI was negatively related to the presence of sumALK (coefficient estimate = −0.0014 ± 0.0009). The odds of a bird having an ALK increased 1.001 times (90% CI for odds ratio = 1.0002, 1.003) for every 1 unit decrease in BCI (i.e., 1.15 times for every 100 unit decrease in BCI). Migration class did not have a measurable effect on sumALK. The concentration of sumALK in pelican feathers was represented by one model that included variables for migration class and planning area (Supplementary Table 6). The best performing model carried 74% of the AIC weight and was 5.7 times as likely to be the best model compared to the second ranked model. Neither variable, however, had a measurable effect on the concentration of sumALK (i.e., SE > coefficient estimate).
Table 6 summarizes the model results. Among adults, sex and migration distance appeared as relevant variables for 50% of the dependent PAH variables. In all cases PAH levels were higher in females compared to males, and higher in longer distance migrants compared to short or medium distance migrants. BCI and planning area appeared as relevant variables for 25% of the dependent PAH variables. BCI was negatively related to PAHs in all cases while the relationship with planning area was inconsistent. Home range appeared as a relevant variable for 17% of the dependent PAH variables and had a positive relationship in one case but a negative relationship in the other case.
We found a positive correlation between sumPAH in adult feathers with PCV (tau = 0.20, p = 0.03). All other correlations were not significant (P > 0.10).
PAH profiles in feathers of chicks
We analyzed feathers for PAHs from 35 pelican chicks. The occurrence and concentrations of each PAH are summarized in Table 5 and reported as dry weight (ng g–1). Of the 24 PAHs assessed, 11 were detected in at least one individual (Table 5). Alkylated PAHs were detected in 34% of individuals and each alkylated compound was detected within our sample of individuals. Parent PAHs were detected in 9% of individuals and we failed to detect 13 of the parent compounds within our sample. Two alkylated compounds were each detected in >10% of individuals; 2,6-dimethyl naphthalene (n = 4) and 2,3,5-trimethylnaphthalene (n = 4). Of 35 birds sampled, 13 (37.1%) had at least 1 PAH detected (1 PAH n = 8; 2 PAHs n = 5). Parent compounds were detected in 3 of 35 birds, alkylated compounds were detected in 12 of 35 birds, and both parent and were alkylated PAHs detected in 2 of 35 birds.
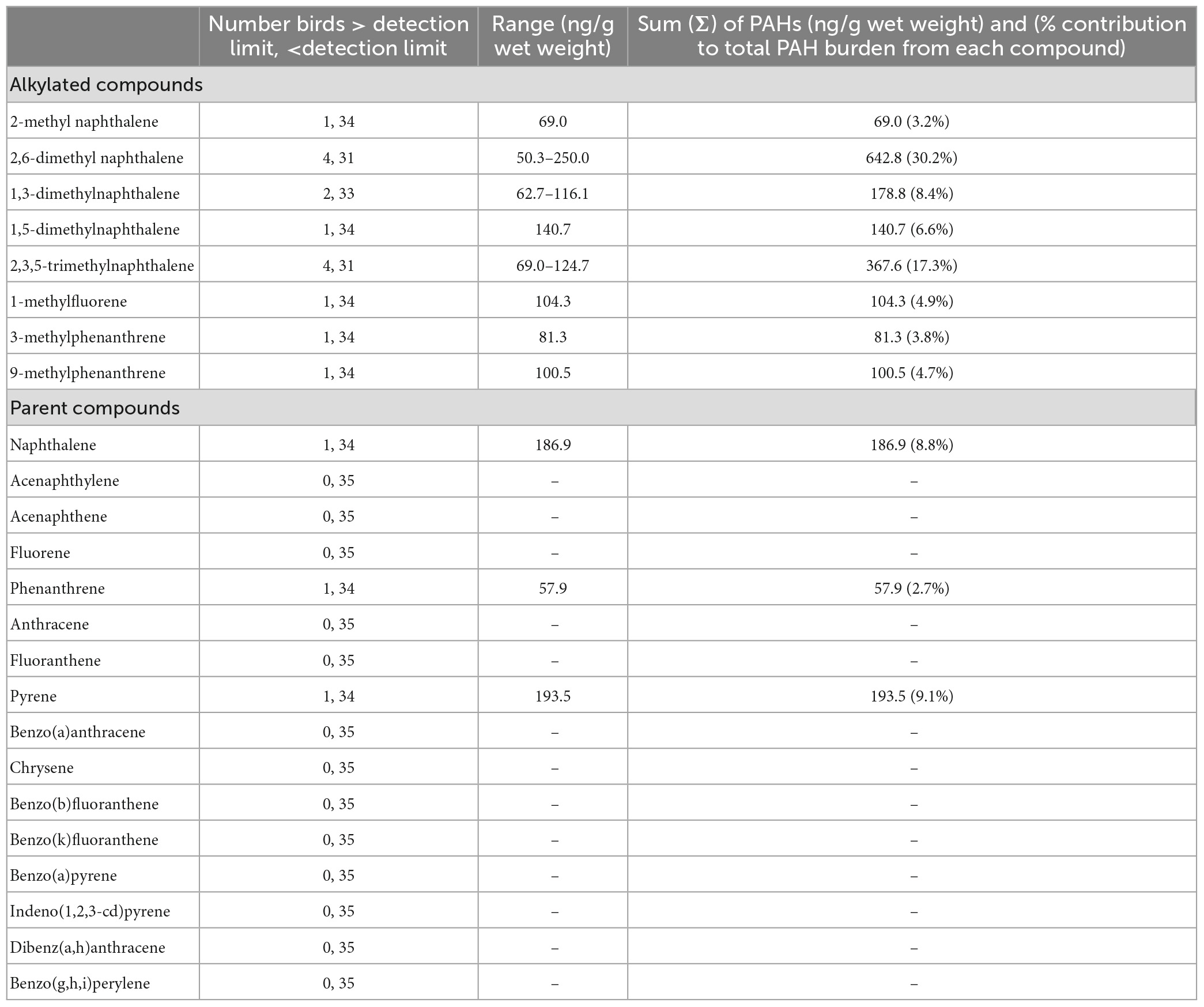
Table 5. Frequency of detection, range and sum of concentration (ng/g wet weight), and percent contribution to total burden polycyclic of individual aromatic hydrocarbons (PAHs) from feathers of chick brown pelicans (n = 35) breeding in the northern Gulf of Mexico, 2014–2015.
Among birds with detectable levels of PAHs, the sum of all PAHs ranged from 100.5 to 309.6, the sum of alkylated PAHs ranged from 50.3 to 265.3, and the sum of parent PAHs ranged from 57.9 to 193.5 (Figure 6). The sum of alkylated PAHs (48.14 ± 77.03) was significantly higher compared to the sum of parent PAHs (12.52 ± 45.44) when using the full data set (W = 458, P = 0.01) but did not differ when only birds above detectable limits were examined (W = 21.0, P = 0.7). When all individuals are considered, there was no correlation between summed concentrations of alkylated and parent PAHs (Kendall tau r = 0.13, Pearson r = 0.05). Sample sizes were insufficient (n = 3 birds) to assess the correlation between alkylated and parent PAHs when only individuals with detectable limits of PAHs were considered.
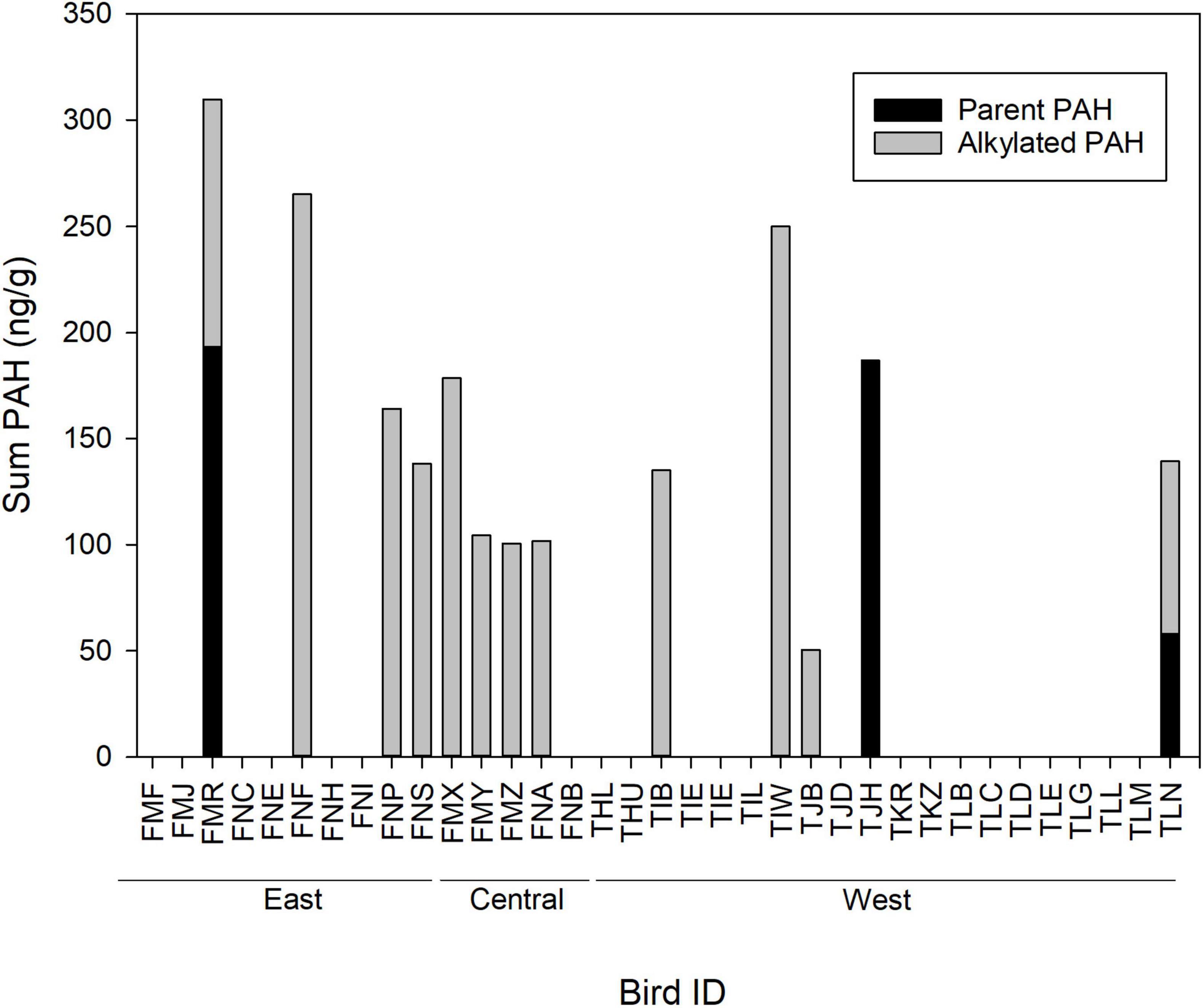
Figure 6. Concentration (ng g–1; dry weight) of parent and alkylated polycyclic aromatic hydrocarbons (PAHs), and the sum of parent plus alkylated PAHs, in feather samples of brown pelican chicks in the northern Gulf of Mexico, 2014–2015. Bird ID, the three-letter combination from the field-readable plastic leg band applied to the chick. F, feather sample.
Two models for the presence of sumPAH in chick feathers received support. The top ranked model included planning area and the null model also was supported (Supplementary Table 7). The planning area model carried 54% of the AIC weight and was 1.9 times as likely to be the best model compared to the second ranked model. Birds in the CPA were six times as likely to have a PAH compared to birds in the EPA (coefficient estimates = 1.79 ± 1.29) although the variability on the estimate was wide (90% CI for odds ratio = 0.9, 75.9). All three models received support for the concentration of sumPAH in chick feathers (Supplementary Table 7). The model including planning area carried 41% of the AIC weight and was 1.1 times as likely to be the best model compared to the null model which ranked second. Planning area (coefficient estimates: central = −0.59 ± 0.29, western = −0.36 ± 0.27) had a measurable relationship with sumPAH. Birds in the EPA were 1.8 times (90% CI for odds ratio = 1.1, 2.9) more likely to have elevated sumPAH concentrations with respect to birds in the CPA and birds in the EPA were 1.4 times (90% CI for odds ratio = 0.9, 2.3) more likely to have elevated sumPAH concentrations with respect to birds in the WPA.
Only three birds had detectable levels of sumPAR. Although the model including BCI received support for the presence of sumPAR, it ranked second to the null model which was 2.6 times as likely to be the best model (Supplementary Table 8). The SE on the estimate of BCI was greater than the coefficient estimate, indicating no measurable relationship. A hurdle model was not conducted for sumPAR due to insufficient sample size.
Only one model supported the presence of sumALK in chick feathers. The top ranked model included planning area (Supplementary Table 9). The planning area model carried 70% of the AIC weight and was 3.5 times as likely to be the best model compared to the null model which ranked second. Birds in the CPA were 6 times more likely (90% CI for odds ratio = 0.9, 75.9) to have a PAH compared to birds in the EPA (coefficient estimate = 1.79 ± 1.29) while birds in the EPA were 2.6 times more likely (90% CI for odds ratio = 0.7, 11.1) to have a PAH compared to birds in the WPA (coefficient estimates = −0.98 ± 0.85). Although the model including BCI received support for the concentration of sumALK, it ranked second to the null model which was 1.9 times as likely to be the best model (Supplementary Table 9).
We found a negative correlation between sumPAH in chick feathers with PCV (tau = −0.54, p = 0.002). All other correlations were not significant (P > 0.10).
Summary of model results
Table 6 summarizes model results from both age classes and both tissues types. Among adults, sex and migration distance appeared as relevant variables for 50% of the dependent PAH variables. In all cases PAH levels were higher in females compared to males, and higher in longer distance migrants compared to short or medium distance migrants. BCI and planning area appeared as relevant variables for 25% of the dependent PAH variables. BCI was negatively related to PAHs in all cases while the relationship with planning area was inconsistent. Home range appeared as a relevant variable for 17% of the dependent PAH variables and had a positive relationship in one case but a negative relationship in the other case.
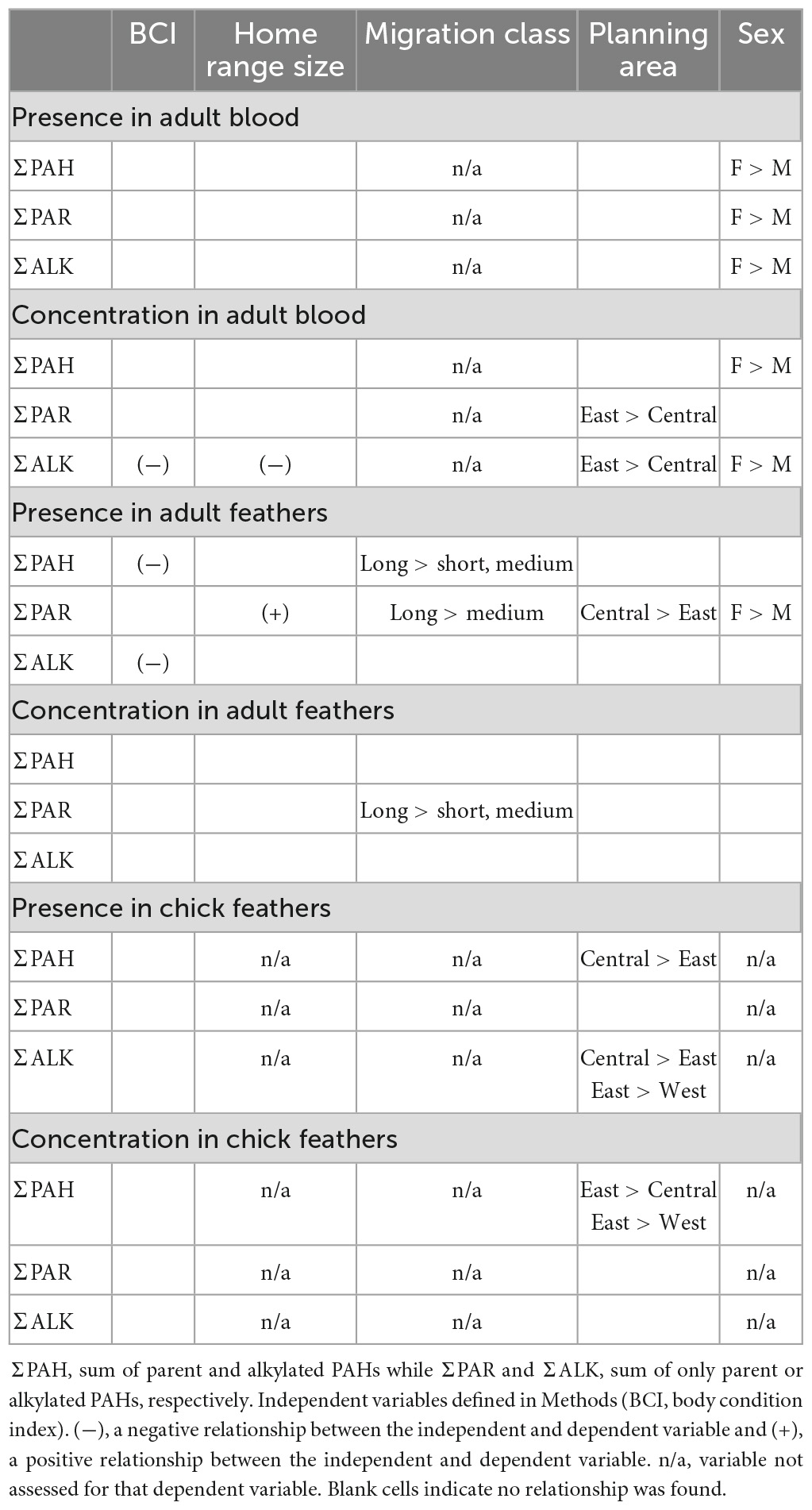
Table 6. Summary of model results from an information theoretic approach to assess relationships among polycyclic aromatic hydrocarbons (PAHs) and independent variables for brown pelican adults and chicks sampled from breeding colonies in the northern Gulf of Mexico, 2013–2015.
Discussion
We found that PAHs occurred frequently, and in high concentrations, in brown pelicans in the Gulf of Mexico, and that higher concentrations of PAHs were associated with adverse outcomes such as lower body condition (adults) and lower packed cell volume (chicks). Of the 24 PAHs assessed, 17 occurred at least once when data were pooled among all three sample sets (i.e., adult blood, adult feathers, chick feathers). Each of the eight alkylated PAHs was detected and six were found in all three sample sets. Nine of the 16 parent PAHs were detected although only three were detected in all three data sets. Although the PAH profile of brown pelicans in the northern GOM was diverse, suggesting a wide range of inputs, 2- and 3-ring PAHs were the most commonly detected, indicating that PAH uptake and sequestration was petrogenic in nature (McConnell et al., 2015). Lesser-ringed PAHs tend to be less carcinogenic compared to higher-ringed PAHs, however, carcinogenic activity tends to increase with the addition of alkyl groups (Abers and Loughlin, 2003). Therefore the adult and chick brown pelicans we sampled, which commonly presented with alkylated versions of two-ring PAHs, may be at risk from carcinogenic PAH exposure. We also found that pathways for exposure varied among age classes and tissue types: adult exposure was higher in females and was positively correlated with larger home ranges and longer migrations, while chick exposure was positively correlated with the region in which the colony was located. This suggests that PAH exposure in adults incorporates variation in individual behavior across the annual cycle, while PAH levels in chicks reflect conditions in and around the breeding site.
PAH profiles in adults
We analyzed concentrations of PAHs in both blood and feathers from adults to provide a complementary assessment of PAH exposure in the environment. Alkylated PAHs occurred more commonly and were measured at higher summed concentrations compared to parent PAHs in adult samples from both blood and feathers. The most common and concentrated PAHs in brown pelican samples were all two-ring alkylated PAHs present in crude oil (2,3,5-trimethylnaphthalene, 1,3-dimethylnaphthalene, and 2,6-dimethyl naphthalene), suggesting that exposure to oil and/or byproducts of oil may have been a substantial source of PAH contamination for brown pelicans during this study. Alkylated PAHs are more abundant in crude oil, more persistent, and less prone to metabolization compared to parent PAHs (Seegar et al., 2015). Liu et al. (2012) found that alkylated PAHs had a stronger signature in crude oil, weathered oil, and oil mousse compared to parent PAHs. 2,6-dimethyl naphthalene was also identified as a known component of oil released during blowouts in the northern Gulf of Mexico (Seegar et al., 2015). This profile pattern indicates that exposure to oil and/or byproducts of oil may have been a substantial source of PAH contamination for brown pelicans during this study. Similar to the general profile we observed, alkylated PAHs also were more common compared to parent PAHs in blood samples of Common Loons (Gavia immer) and Northern Gannets (Morus bassanus) sampled during their non-breeding seasons in the northern Gulf of Mexico (Paruk et al., 2014, 2016; Champoux et al., 2020).
The presence or absence of PAHs in adult blood samples was most frequently predicted by sex, although this relationship was stronger for alkylated PAHs than for parent or the sum of all PAHs. Sex also had a positive effect on the increase in concentration of sumPAH and sumALK in adult blood samples. A dimorphic result based on sex could occur through differential resource selection by males and females with respect to habitat occupied and/or prey consumed (Seegar et al., 2015). In contrast, we did not observe a sex effect on either the occurrence of or concentration of PAHs in adult feathers. Given that blood reflects a relatively recent signature compared to feathers, our results indicate that during incubation and early chick-rearing, females are being exposed to PAHs more frequently or perhaps more recently than males but that over the course of the annual cycle exposure may be more similar.
A unique attribute of our samples was the opportunity to use tracking data to assess the extent of movement, as measured by home range size and migration strategy, on PAH exposure. Migration class predicted the occurrence and concentration of PAHs in adult feathers more frequently than the other independent variables we assessed. Three of the six model steps showed that longer distance migrants were more likely to be found with a PAH (sumPAH and sumPAR) and that the level of PAHs was likely to increase in that group (sumPAR). Most long-distance migrants in our study departed the northern Gulf and occupied wintering areas in the southern Gulf (i.e., Mexico EEZ), south Texas coast, Cuba, or the Pacific coast of Mexico (Lamb et al., 2018, 2020). Most long-distance migrants also attended distinct staging or molting sites separate from both their breeding and wintering areas, and approximately 75% of birds in our sample occupied ranges in the Louisiana Delta during the post-breeding period (Lamb et al., 2020). An investigation into potential sources of parent PAHs in molting and wintering areas may be warranted but likely would need to consider exposure to both pyrogenic sources (which could be quite varied) and petrogenic sources in these locations.
In contrast to migration class, home range during the breeding season rarely had an ecological relationship with PAH presence and was only related (positively) to the concentration of parent PAHs on adult feathers. Our results suggest that the extent of daily movement patterns (i.e., on the order of 10s of km) may not occur over a spatial scale that consistently experiences substantial heterogeneity in PAH availability. When considered with the results of migration class, our data suggest that PAH availability for adult brown pelicans is more heterogeneous at the larger spatial resolutions typical of migration (e.g., ≥100 km) compared to smaller spatial resolutions typical of home ranges (e.g., ≤100 km). Jodice et al. (2022) also did not report a consistent effect of home range size on measures of blood biochemistry and hematology in these same populations of brown pelicans.
We also assessed the relationship between PAH exposure and regional oil and gas development intensity, using the three jurisdictional planning areas of the U.S. Bureau of Ocean and Energy Management. The proximity to oil and gas activity is often used when predicting or assessing contaminant loads or the likelihood of risk exposure to marine wildlife (e.g., Nicholson et al., 2023), with an underlying assumption that exposure would increase with the level of oil and gas activity (i.e., highest in the central, intermediate in the west, and least in the east). During our study, however, planning area was never a strong predictor of the presence of PAHs in adult pelicans and when planning area was relevant, the results were not consistent with the background levels of oil and gas development among the regions. Similarly, Nicholson et al. (2023) found that proximity to active oil and gas activity was not a strong predictor of PAH levels in red snapper (Lutjanus campechanus) in the western Gulf. We detected a slightly higher concentration of sumPARs in the eastern planning area, the least developed with respect to oil and gas activity. This result was driven by two individuals from Florida, each with a high level of the parent PAH fluorene (n = 2 birds, 161.1 ng g–1 and 165.4 ng g–1). The individuals from which these samples were collected were both nesting adults on Audubon Island, Florida, with home ranges centered in and proximate to bays surrounding Panama City, Florida, an area with substantial industrial, military, and agricultural development. Similarly, the three highest levels of sumALK occurred in birds nesting in the eastern planning area in Florida. The lack of a consistent relationship between PAHs and the level of oil and gas development within the planning areas agrees with our prior observation that breeding region is not a consistent predictor of pelican health parameters (Jodice et al., 2022). These results indicate that exposure to PAHs in pelicans is driven by a complex set of internal and external factors affecting exposure probability and cannot be distilled solely to the planning area (i.e., regional level of oil and gas development) within which individuals breed.
Of the health-related variables we assessed, the only relationship of significance was between the presence of PAHs in adult feathers and BCI. Individuals in poorer body condition were more likely to be found with a PAH, and it was more likely that the PAH was from the alkylated group. Paruk et al. (2016) found that in common loons sampled for PAHs during their non-breeding season in the northern Gulf of Mexico, individuals for which PAHs were detected were lighter in body mass compared to individuals for which PAHs were not detected. Similarly, Champoux et al. (2020) found that condition of northern gannets, as assessed through blood analytes, was negatively correlated with PAH concentration. Taken together, these results support the supposition that PAHs can result in sublethal effects within individuals (e.g., reduced body condition) even when concentrations are not at maximum levels.
The average concentration (i.e., excluding individuals where sumPAH = 0) of both parent (116.1 ± 61.8 ng g–1) and alkylated (119.57 ± 55.6 ng g–1) PAHs in blood samples from pelicans in this study appears to be higher compared to blood samples collected from other waterbirds from the Gulf of Mexico during a similar time frame. Paruk et al. (2016) reported means ± SEs for parent and alkylated PAHs in wintering common loons of 83.1 ± 15.2 and 15.2 ± 4.3, respectively. Champoux et al. (2020) reported means ± SEs for parent and alkylated PAHs in wintering northern gannets of 2.5 ± 2.4 and 11.1 ± 3.3 ng g–1, respectively. Comparisons of PAH levels in feathers of adult pelicans from this study to other studies of PAH levels in feathers of free-ranging birds from this region are, to the best of our knowledge, unavailable at this time.
PAH profiles in chicks
Only feathers were assessed for PAHs in chicks. As with adults, however, each of the eight alkylated PAHs was detected in chicks but only three of the 16 parent PAHs were detected (and each only once). The most commonly occurring PAHs were the alkylated PAHs 2,3,5-trimethylnaphthalene, 1,3-dimethylnaphthalene, and 2,6-dimethyl naphthalene, the same common PAHs found in adult feathers. As with adults, 2- and 3-ring PAHs were the most commonly detected and therefore pelican chicks also may be at risk from carcinogenic PAH exposure (Abers and Loughlin, 2003). There were no PAHs found in chicks that were not also found in adults, suggesting that chicks represent a subset of the adult data although the profile and total concentration of PAHs of chicks are less diverse and lower, respectively, compared to adults.
Planning area irregularly and weakly predicted PAH levels in feathers of chicks. The presence of alkylated PAHs was more likely in the central planning area, which has a higher concentration of oil and gas activity compared to the western and eastern planning areas. Alkylated PAHs tend to be associated with petrogenic sources and may be more likely to occur from contact with recently foraging parents (Paruk et al., 2014; Seegar et al., 2015). The three highest concentrations of PAHs in chick feathers, however, were not recorded from the central planning area. These three high values occurred in birds sampled in Galveston Bay, Texas, and from the Florida Panhandle, suggesting that local factors may also be driving individual exposure to PAHs. For example, between 2004 and 2017, 17% of sediment samples from the Houston Ship Channel in Galveston Bay exceeded state standards for Pyrene2 which was the second highest PAH we recorded in pelican chicks. The heterogeneity we observed in chick exposure suggests that specific and highly localized exposure events, such as consuming contaminated prey item or contacting contaminated material at or near nest sites, may substantially affect PAH levels over the relatively short time frame (i.e., weeks) of nestling development. Because of relative infrequency of PAHs in chick feathers, however, caution should be applied when interpreting these results.
Comparable data sets assessing PAH loads in chicks are, to the best of our knowledge, few in number. Fernie et al. (2018a) measured total PAH loads of 31–106 ng g–1 ww in muscle and feces of nestling tree swallows at oil sand sites. While not directly comparable the sumPAH values we measured (57.9–642.8 ng g–1 dry weight) appear to be substantially higher. Chick feathers likely represent contact transfer of PAHs from adults, deposition from aerial sediments, or contact with PAHs in ground/nesting material or prey (Fernie et al., 2018a,b; Perez-Umphrey et al., 2018). Fernie et al. (2018b) found that Tree swallow nestlings showed decreased growth rates and that reproductive success (% eggs that led to fledged chicks) was lower at oil sand sites with higher levels of PAHs compared to reference sites. Uptake and deposition of PAHs in the birds’ muscle was related to diet and also higher at oil sand sites compared to reference sites (Fernie et al., 2018a). These results demonstrate that nest-bound chicks can accumulate detrimental levels of PAHs from the surrounding environment. Given that the route of contamination for PAHs on chick feathers is not entirely clear, we suggest that additional assessments be considered to determine if short- or long-term changes may occur in PAH loads dependent upon chick age or parental activity such as provisioning or brooding (i.e., activities that may enhance contact with chicks).
Correlates with other biomarkers
Exposure to contaminants such as PAHs can result in sublethal effects of varying severity. Fallon et al. (2018) identified several physiologic correlates of oiling in Brown Pelicans and other waterbirds following exposure to oiling including reduced PCV, reduced Hb, decreased counts of red blood cells, oxidative injury to erythrocytes, and formation of Heinz bodies. We assessed physiologic correlates of PAH exposure in two tissue types and two age classes. We found a positive correlation of PAH exposure with total proteins and with lymphocyte count in adults. An increase in total proteins or lymphocyte counts can occur with inflammation and infection (Newman et al., 2000; Grimaldi et al., 2015), both attributes of PAH exposure. Paruk et al. (2016, 2021) found positive correlations between PAH levels and total protein, and between PAH levels and counts of eosinophils, lymphocytes, and monocytes in common loons wintering in the Gulf of Mexico. Similarly, Champoux et al. (2020) found a positive correlation between lymphocyte count and PAH exposure in northern gannets wintering in the Gulf of Mexico.
We found that PCV was lower in chicks when PAH levels in their feathers were higher. A decrease in PCV can be indicative of sublethal physiological responses to environmental stressors. For example, Paruk et al. (2016) found a weak negative correlation between PCV and PAH exposure as measured in blood of adult common loons wintering in the Gulf of Mexico and Fallon et al. (2018) found decreased PCV in brown pelicans in areas exposed to oiling compared to reference areas. Each suggested that the decrease in PCV was symptomatic of environmental stressors related to exposure to PAHs and oiling. Given that feathers are more likely to represent longer term exposure, our results indicate that pelican chicks may be experiencing long term physiological stress correlated with exposure to PAHs. In contrast, we found that PCV was higher in adults when PAH levels in their feathers were higher. Pulmonary and renal diseases can also lead to relative increases in PCV and such diseases may occur with long term exposure to pollutants such as PAHs (Troisi et al., 2006; Jones, 2015; Bursian et al., 2017).
Conclusion
We assessed PAH loads in blood and feathers from adult brown pelicans during the breeding season, and from feathers of chicks of brown pelicans. Both alkylated and parent PAHs were commonly detected in both age groups and both sample types, indicating that both short- and long-term exposure (i.e., blood and feathers, respectively) are occurring for this species. The high level of oil and gas activity in waters of the northern Gulf of Mexico, as well as industrial, military, and agricultural activity in the coastal region, make it challenging to identify a primary source for PAH exposure although the higher frequency of alkylated PAHs in samples does suggest substantial exposure from a petrogenic source (Pereira et al., 2009; McConnell et al., 2015; Jesus et al., 2022). Our data provide reference levels for a time period following a major spill event, and further assessments conducted additional years out from the event (i.e., a time series) may also provide context for the stability of these initial levels (National Research Council [NRC], 2003). Long-term tracking of PAHs, as well as an assessment of sublethal effects of PAHs on pelicans, can also enhance our understanding of the persistence and effects of this contaminant in the northern Gulf. Recent studies also suggest that preen oil can also be used to assess PAH levels and assessing PAH levels through multiple matrices (e.g., blood, feathers, preen oil) may provide a more complete picture of contamination (Acampora et al., 2018). Lastly, the taxonomic diversity of marine avifauna assessed for PAH exposure in the northern Gulf remains narrow. Along with our study, PAHs have also been measured in free-ranging common loons and northern gannets, both species that migrate to the Gulf during their non-breeding seasons. Additional data from other marine avifauna that breed within the northern Gulf would provide stakeholders with a more complete view of PAH exposure, particularly if species representing different foraging modes and diets were included [e.g., gulls and terns (Laridae), or American oystercatchers (Haematopus palliates)].
Data availability statement
The datasets presented in this study can be found in online repositories. The names of the repository/repositories and accession number(s) can be found below: Data on PAH levels and individual attributes from this research are available as a data release through the U.S. Geological Survey at https://doi.org/10.5066/P94GBJ4G (Lamb et al., 2019).
Ethics statement
This animal study was reviewed and approved by Clemson University Animal Care and Use Committee.
Author contributions
PJ: conceptualization, methodology, formal analysis, resources, writing (original and reviews), visualization, supervision, project administration, and funding acquisition. JL: conceptualization, methodology, validation, formal analysis, investigation, data collection and management, sample preparation, writing (original and reviews), supervision, and project administration. YS: validation, data collection and management, sample preparation, formal analysis, writing (original and reviews), and visualization. CP: methodology, laboratory analysis, and writing (original and reviews). All authors contributed to the article and approved the submitted version.
Funding
This study was funded by the U.S. Department of the Interior, Bureau of Ocean Energy Management, Environmental Studies Program, Washington, DC, through Interagency Agreement Number M12PG00014 with the U.S. Geological Survey South Carolina Cooperative Fish and Wildlife Research Unit, and through the U.S. Geological Survey Outer Continental Shelf Program. JL was supported by the University of Rhode Island and Centre d’Ecologie Fonctionnelle et Evolutive while conducting earlier research in support of this manuscript. Carolyn Wakefield and Brenna Byler provided administrative support. Katherine McFadden (now deceased) was originally a Co-PI on the project and developed the initial research plan for health and contaminants. The South Carolina Cooperative Fish and Wildlife Research Unit is jointly supported by the U.S. Geological Survey, South Carolina DNR, and Clemson University. Any use of trade, firm, or product names is for descriptive purposes only and does not imply endorsement by the U.S. Government Parts of this manuscript were released as a Final Report for the aforementioned Interagency Agreement through the Bureau of Ocean Energy Management (see Lamb et al., 2020 in the reference list).
Acknowledgments
Special thanks to Jeff Gleason for his efforts in conceptualizing and initiating this research. Dave Moran (BOEM) provided administrative and contract support. Field research was conducted with permission from the Clemson University Animal Care and Use Committee (2013-026). Permitting for field data collection was provided by the U.S. Geological Survey Bird Banding Laboratory (22408), Texas Parks and Wildlife (SPR-0113-005), Audubon Texas, the Nature Conservancy in Texas, Louisiana Department of Wildlife and Fisheries (LNHP-13-058 and LNHP-14-045), and the Florida Fish and Wildlife Conservation Commission (LSSC-13-00005). Genetic sexing of pelicans was conducted in collaboration with Amy MacMillan (Buffalo State University) and Ludovic Besaury (Clemson University). Plasma chemistry and hematology samples were analyzed at the Avian & Wildlife Laboratory, University of Miami, Miami, Florida. For assistance and logistical support with field research and laboratory activities, we thank Elizabeth Ford, Stève Desaivre, Tim and Peggy Wilkinson, Mark Dumesnil, Beau Hardegree, Amanda Hackney, ScottWalter, John Dindo, Caroline Poli, Ludovic Besaury, Tommy King, and technicians from Texas Audubon Society and The Nature Conservancy. We thank Jim Paruk, two reviewers, and our handling TM, for providing reviews on this manuscript.
Conflict of interest
The authors declare that the research was conducted in the absence of any commercial or financial relationships that could be construed as a potential conflict of interest.
Publisher’s note
All claims expressed in this article are solely those of the authors and do not necessarily represent those of their affiliated organizations, or those of the publisher, the editors and the reviewers. Any product that may be evaluated in this article, or claim that may be made by its manufacturer, is not guaranteed or endorsed by the publisher.
Supplementary material
The Supplementary Material for this article can be found online at: https://www.frontiersin.org/articles/10.3389/fevo.2023.1185659/full#supplementary-material
Footnotes
References
Abers, P. H., and Loughlin, T. R. (2003). “Effects of PAHs on marine birds, mammals, and reptiles,” in PAHs: An Ecotoxicological Perspective, ed. P. T. Douben (West Sussex: Wiley), 243–262. doi: 10.1016/j.aquatox.2016.09.016
Absg Consulting Inc. (2018). U.S. Outer Continental Shelf Oil Spill Statistics. Arlington, VA: Bureau of Ocean Energy Management.
Acampora, H., White, P., Lyashevska, O., and O’Connor, I. (2018). Contrasting congener profiles for persistent organic pollutants and PAH monitoring in European storm petrels (Hydrobates pelagicus) breeding in Ireland: a preen oil versus feathers approach. Environ. Sci. Pollut. Res. 25, 16933–16944. doi: 10.1007/s11356-018-1844-2
Albers, P. H. (2003). “Birds and polycyclic aromatic hydrocarbons,” in Handbook of Ecotoxicology, 2nd Edn, eds D. J. Hoffman, B. A. Rattner, G. A. Burton Jr., and J. Cairns (New York, NY: Lewis Publishers), 341–372.
Albers, P. H. (2006). Birds and polycyclic aromatic hydrocarbons. Avian Poult. Biol. Rev. 17, 125–140.
Alonso-Alvarez, C., Munilla, I., López-Alonso, M., and Velando, A. (2007). Sublethal toxicity of the Prestige oil spill on yellow-legged gulls. Environ. Int. 33, 773–781.
Beyer, J., Goksøyr, A., Hjermann, D. Ø, and Klungsøyr, J. (2020). Environmental effects of offshore produced water discharges: a review focused on the Norwegian continental shelf. Mar. Environ. Res 162:105155. doi: 10.1016/j.marenvres.2020.105155
Briggs, K. T., Yoshida, S. H., and Gershwin, M. E. (1996). The influence of petrochemicals and stress on the immune system of seabirds. Reg. Toxicol. Pharmacol. 23, 145–155. doi: 10.1006/rtph.1996.0036
Bureau of Ocean Energy Management [BOEM] (2016). Gulf of Mexico OCS Oil and Gas Lease Sale: 2017, in Central Planning Area Lease Sale 245 Final Supplemental Environmental Impact Statement. OCS EIS/EA BOEM 2016-061. New Orleans, LA: Bureau of Ocean Energy Management.
Bursian, S. J., Dean, K. M., Harr, K. E., Kennedy, L., Link, J. E., Maggini, I., et al. (2017). Effect of oral exposure to artificially weathered Deepwater Horizon crude oil on blood chemistries, hepatic antioxidant enzyme activities, organ weights and histopathology in western sandpipers (Calidris mauri). Ecotoxicol. Environ. Saf. 146, 91–97. doi: 10.1016/j.ecoenv.2017.03.045
Champoux, L., Rail, J. F., Houde, M., Giraudo, M., Lacaze, É, Franci, C. D., et al. (2020). An investigation of physiological effects of the Deepwater Horizon oil spill on a long-distance migratory seabird, the northern gannet. Mar. Pollut. Bull. 153:110953. doi: 10.1016/j.marpolbul.2020.110953
Deepwater Horizon Natural Resource Damage Assessment Trustees (2016). Deepwater Horizon oil spill: Final programmatic damage assessment and restoration plan and final programmatic environmental impact statement. Available online at: http://www.gulfspillrestoration.noaa.gov/restoration-planning/gulf-plan
Eisler, R. (1987). Polycyclic Aromatic Hydrocarbon Hazards to Fish, Wildlife, and Invertebrates: a Synoptic Review. Laurel, MD: U.S. Department of the Interior, Fish and Wildlife Service. U.S. Fish and Wildlife Service Biological Report 85 (1.11).
Fallon, J. A., Smith, E. P., Schoch, N., Paruk, J. D., Adams, E. A., Evers, D. C., et al. (2018). Hematological indices of injury to lightly oiled birds from the Deepwater Horizon oil spill. Environ. Toxicol. Chem. 37, 451–461. doi: 10.1002/etc.3983
Fernie, K. J., Marteinson, S. C., Chen, D., Eng, A., Harner, T., Smits, J. E. G., et al. (2018a). Elevated exposure, uptake, and accumulation of polycyclic aromatic hydrocarbons by nestling tree swallows (Tachycineta bicolor) through multiple exposure routes in active mining-related areas of the Athabasca oil sands region. Sci. Total Environ. 624, 250–261. doi: 10.1016/j.scitotenv.2017.12.123
Fernie, K. J., Marteinson, S. C., Soos, C., Chen, D., Cruz-Martinez, L., and Smits, J. E. G. (2018b). Reproductive and developmental changes in tree swallows (Tachycineta bicolor) are influenced by multiple stressors, including polycyclic aromatic compounds, in the Athabasca Oil Sands. Environ. Pollut. 238, 931–941. doi: 10.1016/j.envpol.2018.03.074
Grimaldi, W. W., Hall, R. J., White, D. D., Wang, J., Massaro, M., and Tompkins, D. M. (2015). First report of a feather loss condition in Adelie penguins (Pygoscelis adeliae) on Ross Island, Antarctica, and a preliminary investigation of its cause. Emu 115, 185–189.
Hylland, K. (2006). Polycyclic aromatic hydrocarbon (PAH) ecotoxicology in marine ecosystems. J. Toxicol. Environ. Heal. Part A. 69, 109–123.
Itoh, Y., Suzuki, M., Ogawa, A., Munechika, I., Murata, K., and Mizuno, S. (2001). Identification of the sex of a wide range of carinatae birds by PCR using primer sets selected from chicken Eeo.6 and its related sequences. J. Hered. 92, 315–321. doi: 10.1093/jhered/92.4.315
Jaspers, V., Dauwe, T., Pinxten, R., Bervoets, L., Blust, R., and Eens, M. (2004). The importance of exogenous contamination on heavy metal levels in bird feathers. a field experiment with free-living great tits, Parus major. J. Environ. Monit. 6, 356–360. doi: 10.1039/b314919f
Jesus, F., Pereira, J. L., Campos, I., Santos, M., Ré, A., Keizer, J., et al. (2022). A review on polycyclic aromatic hydrocarbons distribution in freshwater ecosystems and their toxicity to benthic fauna. Sci. Tot. Environ. 820:153282. doi: 10.1016/j.scitotenv.2022.153282
Jodice, P. G. R., Adams, E. M., Lamb, J. S., Satgé, Y. G., and Gleason, J. S. (2019). “Strategic bird monitoring guidelines for the Northern Gulf of Mexico: seabirds,” in Strategic Bird Monitoring Guidelines for the Northern Gulf of Mexico, eds R. R. Wilson, A. Fournier, J. S. Gleason, J. E. Lyons, and M. S. Woodrey (Starkville, MI: Mississippi State University), 133–172.
Jodice, P. G. R., Lamb, J. S., Satgé, Y. G., and Fiorello, C. V. (2022). Blood biochemistry and hematology of adult and chick brown pelicans in the northern Gulf of Mexico: baseline health values and ecological relationships. Conserv. Physiol. 10:coac064. doi: 10.1093/conphys/coac064
King, M. D., Elliott, J. E., and Williams, T. D. (2021). Effects of petroleum exposure on birds: a review. Sci. Total Environ. 755:142834.
Laffon, B., Fraga-Iriso, R., Pérez-Cadahía, B., and Méndez, J. (2006). Genotoxicity associated to exposure to prestige oil during autopsies and cleaning of oil-contaminated birds. Food Chem. Toxicol. 44, 1714–1723. doi: 10.1016/j.fct.2006.05.010
Lamb, J. S., Newstead, D. J., Koczur, L. M., Ballard, B. M., Green, C. M., and Jodice, P. G. R. (2018). A bridge between oceans: Overland migration of marine birds in a wind energy corridor. J. Avian Biol. 49:e01474. doi: 10.1111/jav.01474
Lamb, J. S., Satgé, Y. G., Fiorello, C. V., and Jodice, P. G. R. (2017a). Behavioral and reproductive effects of bird-borne data logger attachment on Brown Pelicans (Pelecanus occidentalis) on three temporal scales. J. Ornithol. 158, 617–627.
Lamb, J. S., Satgé, Y. G., and Jodice, P. G. R. (2017b). Influence of density-dependent competition on foraging and migratory behavior of a subtropical colonial seabird. Ecol. Evol. 7, 6469–6481. doi: 10.1002/ece3.3216
Lamb, J. S. Satgé, Y. G., and Jodice, P. G. R. (2019). Physiological ecology of Brown Pelican in the Gulf of Mexico, 2013-2016. U.S. Geological Survey data release. Washington, DC: US Geological Survey. doi: 10.5066/P94GBJ4G
Lamb, J. S., Satgé, Y. G., Streker, R. A., and Jodice, P. G. R. (2020). Ecological Drivers of Brown Pelican Movement Patterns, Health, and Reproductive Success in the Gulf of Mexico. New Orleans, LA: Bureau of Ocean Energy Management.
Liu, Z., Liu, J., Zhu, Q., and Wu, W. (2012). The weathering of oil after the Deepwater Horizon oil spill: insights from chemical composition of the oil from the sea surface, salt marshes and sediments. Environ. Res. Lett. 7:035302.
Marvin, C. H., Berthiaume, A., Burniston, D. A., Chibwe, L., Dove, A., Evans, M., et al. (2021). Polycyclic aromatic compounds in the Canadian environment: aquatic and terrestrial environments. Environ. Pollut. 285:117442.
McConnell, H. M., Gartrell, B. D., Chilvers, B. L., Finlayson, S. T., Bridgen, P. C. E., and Morgan, K. J. (2015). Baseline hydrocarbon levels in New Zealand coastal and marine avifauna. Mar. Poll. Bull. 94, 290–298. doi: 10.1016/j.marpolbul.2015.02.001
McNease, L., Richard, D., and Joanen, T. (1992). Reintroduction and colony expansion of the Brown Pelican in Louisiana. Proc. Annu. Conf. Southeast Fish Wildl Agencies 46, 223–229.
Menzie, C. A., Potocki, B. B., and Santodonato, J. (1992). Exposure to carcinogenic PAHs in the environment. Environ. Sci. Technol. 26, 1278–1284.
Michael, P. E., Hixson, K. M., Haney, J. C., Satgé, Y. G., Gleason, J. S., and Jodice, P. G. R. (2022). Seabird vulnerability to oil: exposure potential, sensitivity, and uncertainty in the northern Gulf of Mexico. Front. Mar. Sci. 9:880750. doi: 10.3389/fmars.2022.880750
National Research Council [NRC] (2003). Oil in the Sea III: Inputs, Fates, and Effects. Washington, D.C: National Academic Press.
Newman, S. H., Anderson, D. W., Ziccardi, M. H., Trupkiewicz, J. G., Tseng, F. S., Christopher, M. M., et al. (2000). An experimental soft-release of oil-spill rehabilitated American coots (Fulica americana): II. effects on health and blood parameters. Environ. Poll. 107, 295–304. doi: 10.1016/s0269-7491(99)00171-2
Nfon, E., Cousins, I. T., and Broman, D. (2008). Biomagnification of organic pollutants in benthic and pelagic marine food chains from the Baltic Sea. Sci. Total. Environ. 397, 190–204.
Nicholson, T. J., Pulster, E. L., Murawski, S. A., and Judkins, H. L. (2023). A comparison of PAH exposure in red snapper (Lutjanus campechanus) around natural and artificial reefs in the northwestern Gulf of Mexico. Cont. Shelf. Res. 258:104972.
O’Reilly, C., Silva, M., Asl, S. D., Meurer, W. P., and Macdonald, I. R. (2022). Distribution, magnitude, and variability of natural oil seeps in the Gulf of Mexico. Remote Sens. 14:3150.
Paruk, J. D., Adams, E. M., Uher-Koch, H., Kovach, K. A., Long, D., Perkins, C., et al. (2016). Polycyclic aromatic hydrocarbons in blood related to lower body mass in common loons. Sci. Total. Environ. 565, 360–368. doi: 10.1016/j.scitotenv.2016.04.150
Paruk, J. D., Long, D., Perkins, C., East, A., Sigel, B. J., and Evers, D. C. (2014). Polycyclic aromatic hydrocarbons detected in Common Loons (Gavia immer) wintering off coastal Louisiana. Waterbirds 37, 85–93.
Paruk, J. D., Uher-Koch, H., Kovach, K., Byrd, A., Dolley, A., Cray, C., et al. (2021). Evidence of subclinical inflammation relates to PAH exposure in overwintering common loons (Gavia immer). Waterbirds 44, 317–323. doi: 10.1675/063.044.0306
Pereira, M. G., Walker, L. A., Wright, J., Best, J., and Shore, R. F. (2009). Concentrations of Polycyclic Aromatic Hydrocarbons (PAHs) in the eggs of predatory birds in britain. Environ. Sci. Tech. 43, 9010–9015. doi: 10.1021/es901805e
Perez-Umphrey, A. A., Bergeon Burns, C. M., Stouffer, P. C., Woltmann, S., and Taylor, S. S. (2018). Polycyclic aromatic hydrocarbon exposure in seaside sparrows (Ammodramus maritimus) following the 2010 Deepwater Horizon oil spill. Sci. Total Environ. 630, 1086–1094. doi: 10.1016/j.scitotenv.2018.02.281
Polidoro, B., Matson, C. W., Ottinger, M. A., Renegar, D. A., Romero, I. C., Schlenk, D., et al. (2021). A multi-taxonomic framework for assessing relative petrochemical vulnerability of marine biodiversity in the Gulf of Mexico. Sci. Total Environ. 763:142986. doi: 10.1016/j.scitotenv.2020.142986
Seegar, W. S., Yates, M. A., Doney, G. E., Jenny, J. P., Seegar, T. C. M., Perkins, C., et al. (2015). Migrating tundra Peregrine Falcons accumulate polycyclic aromatic hydrocarbons along Gulf of Mexico following Deepwater Horizon oil spill. Ecotox 24, 1102–1111. doi: 10.1007/s10646-015-1450-8
Spalding, M. D., Fox, H. E., Allen, G. R., Davidson, N., Ferdana, Z. A., Finlayson, M., et al. (2007). Marine ecoregions of the world: a bioregionalization of coastal and shelf areas. Bioscience 57, 573–583. doi: 10.1111/cla.12453
Troisi, G. M., Bexton, S., and Robinson, I. (2006). Polyaromatic hydrocarbon and PAH metabolite burdens in oiled common guillemots (Uria aalge) stranded on the east coast of England (2001-2002). Environ. Sci. Technol. 40, 7938–7943. doi: 10.1021/es0601787
U.S. Fish and Wildlife Service (2011). FWS Deepwater Horizon Oil Spill Response. 2011. Bird Impact Data and Consolidated Wildlife Reports. Washington, DC: U.S. Fish and Wildlife Service.
Walter, S. T., Carloss, M. R., Hess, T. J., and Leberg, P. L. (2013). Hurricane, habitat degradation, and land loss effects on brown pelican nesting colonies. J. Coast. Res. 29, 187–195.
Wilkinson, B. P., and Jodice, P. G. R. (2023). Support for the fasting endurance hypothesis of partial migration in a nearshore seabird. Ecosphere 14:e4365.
Woodyard, M., Polidoro, B. A., Matson, C. W., McManamay, R. A., Saul, S., Carpenter, K. E., et al. (2022). A comprehensive petrochemical vulnerability index for marine fishes in the Gulf of Mexico. Sci. Total Environ. 820:152892. doi: 10.1016/j.scitotenv.2021.152892
Keywords: body condition, brown pelicans, Gulf of Mexico (GOM), home range, migration, petrogenic, polycyclic aromatic hydrocarbons (PAHs)
Citation: Jodice PGR, Lamb JS, Satgé YG and Perkins C (2023) Spatial and individual factors mediate the tissue burden of polycyclic aromatic hydrocarbons in adult and chick brown pelicans in the northern Gulf of Mexico. Front. Ecol. Evol. 11:1185659. doi: 10.3389/fevo.2023.1185659
Received: 13 March 2023; Accepted: 12 May 2023;
Published: 02 June 2023.
Edited by:
Terri Maness, Louisiana Tech University, United StatesReviewed by:
Brianna R. Beechler, Oregon State University, United StatesMary Ann Ottinger, University of Houston, United States
Copyright © 2023 Jodice, Lamb, Satgé and Perkins. This is an open-access article distributed under the terms of the Creative Commons Attribution License (CC BY). The use, distribution or reproduction in other forums is permitted, provided the original author(s) and the copyright owner(s) are credited and that the original publication in this journal is cited, in accordance with accepted academic practice. No use, distribution or reproduction is permitted which does not comply with these terms.
*Correspondence: Patrick G. R. Jodice, cGpvZGljZUBnLmNsZW1zb24uZWR1