- 1Department of Ecology, Institute of Biological Sciences, University of Brasília, Brasília, Brazil
- 2Department of Biology, Program in Ecology, Evolution and Conservation Biology, University of Nevada, Reno, NV, United States
- 3Institute of Chemistry, University of São Paulo, São Paulo, Brazil
- 4Science Department, Gorongosa National Park, Sofala, Mozambique
- 5College of Earth, Ocean, and Atmospheric Sciences, Oregon State University, Corvallis, OR, United States
Phytochemical diversity is an effective plant defensive attribute, but much more research has focused on genetic and environmental controls of specific defensive compounds than phytochemical diversity per se. Documenting plasticity in phytochemical richness and plant chemical composition as opposed to individual compounds is important for understanding plant defense. This study outlines a multi-site transplant experiment in Cerrado gallery forests in central Brazil, utilizing Piper arboreum (Piperaceae), a prevalent and widespread neotropical shrub. Clones from four distinct populations were planted either at their origin site or in a different forest. Secondary metabolite composition varied between populations initially and then changed after transplanting. Interestingly, clones with chemical profiles that were distinct from the populations where they were introduced experienced reduced specialist chrysomelid herbivory compared to clones that were more chemically similar to the existing P. arboreum populations where they were planted. Specialist Lepidoptera herbivory also declined in clones transplanted to a new forest, but this change could not be ascribed to chemical profiles. In contrast, generalist herbivory was unaffected by chemical dissimilarity and transplanting. This research adds to the expanding body of evidence suggesting that phytochemical diversity is a dynamic trait exerting unique effects on different herbivore guilds.
Introduction
“The world is not colored green, it is colored morphine, caffeine, tannin, phenol, terpene, canavanine, latex …” (Janzen, 1978). Janzen’s observation indicates that insect herbivores perceive plants as distinct chemical mixtures. These mixtures can be highly diverse, and phytochemical profiles differ substantially within as well as among populations (Arany et al., 2009; Zust et al., 2012; Glassmire et al., 2016; Massad et al., 2017; De-la-Cruz et al., 2020; Massad et al., 2022). Phytochemistry is also quickly evolving (Kessler and Kalske, 2018), particularly in response to specialist herbivory (Endara et al., 2015). Specialist herbivores may be locally adapted to the chemical community they interact with (Glassmire et al., 2017), making host plants of the same species but from different populations potentially less palatable to them. In contrast, generalist herbivory may be less affected by unique chemotypes (Massad et al., 2022). Understanding how plant chemistry changes across a landscape and how specialist and generalist herbivory differ with host plant chemistry is an important goal for ecology (Hunter, 2016). As herbivore communities turnover across the ranges of their host plants (Salazar and Marquis, 2012; Fine et al., 2013; Salazar et al., 2018; Campos-Moreno et al., 2021; Serejo Rabelo et al., 2021), one may expect phytochemical diversity and similarity to also vary across a species’ range. It is therefore informative to quantify genetic and environmental controls of plant antiherbivore defenses (Fajer et al., 1992; Laitinen et al., 2005; Osier and Lindroth, 2006; Arany et al., 2009; Massad et al., 2011; Eisenring et al., 2021).
Different species of herbivores are uniquely affected by different classes of chemical defenses, rendering multiple lines of defense necessary (Koricheva et al., 2004; Agrawal and Fishbein, 2006). For example, alkaloids in Datura stramonium effectively limit herbivory by the specialist beetle, Epitrix parvula, while a triterpenoid in the same plant reduces damage by a second specialist beetle, Lema daturaphila (De-la-Cruz et al., 2020). Other well studied examples include glucosinolates, cardenolides, alkaloids, and iridoid glycosides that often limit generalist but not specialist herbivory (Bowers and Puttick, 1988; Malcolm, 1994; van Dam et al., 1995; Agrawal et al., 2012; Jeschke et al., 2017). In addition, synergistic effects of co-occurring compounds often result in mixtures of defenses that are more effective than isolated compounds (Berenbaum and Neal, 1985; Berenbaum and Zangerl, 1993; Dyer et al., 2003; Richards et al., 2010, 2012). Secondary metabolite diversity is therefore an important plant trait (Wetzel and Whitehead, 2020) that has recently become recognized as an effective anti-herbivore defense both at the level of individual plants (α-diversity) and at the level of populations (β-diversity; Kessler and Kalske, 2018). α-diversity can be calculated as Simpson’s index (Richards et al., 2015; Wetzel and Whitehead, 2020), and β-diversity can be calculated with similarity indices that measure changes in community (or chemical) composition (Massad et al., 2017; Wetzel and Whitehead, 2020; Massad et al., 2022).
Quantitative changes in plant defenses are influenced by environmental and genetic variation (Massad et al., 2011). In terms of qualitative changes in defenses, differential herbivore pressure and resource availability across sites affect heritable variation in plant chemical profiles and generate chemically distinct populations (Zust et al., 2012; Fine et al., 2013; Hunter, 2016; Glassmire et al., 2017; De-la-Cruz et al., 2020). While hundreds of studies have looked at quantitative changes in defense driven by environmental variation (e.g., Bryant et al., 1983; Herms and Mattson, 1992; Koricheva, 2002; Massad et al., 2011, 2012; Hattas et al., 2017; Chinder et al., 2020), fewer studies have measured environmental effects on phytochemical diversity (Cadena-Zamudio et al., 2022).
Transplant and common garden experiments are very effective for uncovering genetic and environmental controls of plant defense and growth. For example, a common garden experiment replicated across an environmental gradient shows defense traits, but not growth, are under genetic control in Asclepias speciosa (Hahn et al., 2019). Similarly, when Arabidopsis thaliana individuals from a site with naturally low herbivory were transplanted to a site with high herbivory, they suffered more damage than congeners originating in the high herbivory site. Plasticity in defense also differs between populations, and defense production is less responsive to resource conditions in plants originating from a nutrient poor, high herbivory environment (Arany et al., 2009), where there may be greater selection for high levels of defense (Coley et al., 1985). Transplant experiments with tree species in the Amazon have also demonstrated herbivory is lower in individuals originating from resource poor areas (Fine et al., 2004) where plant defense is greater (Fine et al., 2006, 2013).
To understand environmental versus genetic variation in phytochemical profiles as well as the effects of phytochemical similarity and diversity on specialist and generalist herbivores, we examined changes in phytochemistry across populations in a reciprocal transplant experiment. In contrast to the transplant experiments cited above, we focus on phytochemical diversity and phytochemical similarity as potential antiherbivore defenses. The effects of genotype, transplanting to novel sites, and plant chemistry were evaluated separately for specialist and generalist herbivory. Plant growth was also analyzed in response to genotype, transplanting, and herbivory. This work focused on a chemically rich, widespread neotropical understory species, Piper arboreum Aubl. (Piperaceae), and it combined a classic ecological design with modern methods in LC–MS metabolomics to test the following hypotheses. (1) Populations may be phytochemically distinct, and genotype may have stronger control on phytochemical profiles than the transplant environment. (2) Transplanting may limit specialist herbivory as herbivores may not be adapted to the chemistry of novel genotypes. (3) Chemical dissimilarity between transplanted individuals and naturally occurring conspecifics may limit herbivory, particularly by specialists. (4) Phytochemical diversity may limit generalist herbivory. (5) Growth may be limited by herbivory but be unaffected by genotype.
Methods
Focal species and study sites
Piper arboreum Aubl. is a widespread understory shrub occurring in tropical forests from Mexico through Paraguay (Kew Royal Botanic Gardens, https://powo.science.kew.org/taxon/urn:lsid:ipni.org:names:680435-1; Yuncker, 1972). The possibility that populations of P. arboreum are cryptic species is currently under investigation. The genus Piper is highly abundant in neotropical forest understories, including cerrado gallery forests, and it is also extremely species rich, with about 2,600 described species, mostly in the Neotropics (Callejas-Posada, 2020). The chemistry of many species of Piper has been studied (Dyer et al., 2003; Dyer and Palmer, 2004; Kato and Furlan, 2007; Uckele et al., 2021; Philbin et al., 2022). The genus is known for its phytochemical diversity, both within and between species (Richards et al., 2015; Massad et al., 2017; Glassmire et al., 2019; Massad et al., 2022), so further study of the ecological effects of its chemical diversity may help explain plant–herbivore interactions. Piper arboreum in particular produces at least five antifungal amides, cinnamoyl derivatives (Vasques da Silva et al. 2002) and several terpenoids (Navickiene et al., 2006). Plant–insect herbivore interactions on Piper are also well studied, and patterns of herbivory are known for specialist Lepidoptera, Coleoptera, and generalist Acrididae as we all as other taxa (Dyer and Letourneau, 1999; Connahs et al., 2009; Dyer et al., 2010; Serejo Rabelo et al., 2021). Because of its wide distribution, likely intraspecific phytochemical variation (Richards et al., 2015; Massad et al., 2017, 2022; Glassmire et al., 2019; Uckele et al., 2021), and knowledge of its herbivore community, P. arboreum was chosen as the focal species for the present study.
Piper arboreum clones were created from four populations in gallery forests in four protected areas of the cerrado in the Distrito Federal and Goiás in central Brazil (Supplementary Table S1). The cerrado is the second largest biome in South America, and evergreen gallery forests originally accounted for 5% of the cerrado’s area (Dias, 1996). Distances between sites varied from 7 km to 235 km. Caterpillar communities differ between focal sites (Serejo Rabelo et al., 2021), and mean P. arboreum density (as measured in a minimum of 38 10 m diameter plots per site) varied from 730 to 1,243 plants per ha.
Experimental design
In each of the four gallery forests (populations), we selected three plants of P. arboreum, resulting in a total of 12 mother plants. We cut each mother plant into six clones with one node and three leaves, yielding 72 cuttings. We then planted the clones from each mother plant in one of the four forests, with each forest receiving cuttings from one mother plant from that forest and cuttings from a mother from each of the other three forests. While this method does not allow us to quantify potential confounding factors, such as maternal effects, it provides valuable insights into the relative contributions of genetic and environmental factors (including epigenetic effects) to trait variation. Although we cannot examine specific genotypes, our experimental design enables us to investigate questions related to genotype-environment interactions.
We transplanted 24 cuttings in three of the four gallery forests from which we collected genotypes, namely Estação Ecológica de Águas Emendadas (ESECAE), Reserva Ecológica do Roncador (RECOR), and Parque Nacional de Brasília (PNB). We did not use the fourth site of origin, Parque Nacional da Chapada dos Veadeiros (PNCV), for transplanting due to its distance from the other sites and the logistical challenges of regularly monitoring the clones. Each transplant location was planted with six clones originating from the same site (i.e., from the same mother plant) and 18 clones from the other two sites (six from the same mother plant at one site and six from the same mother plant at the second site). We planted the clones in 10 L buckets using commercial potting soil, and randomly placed the buckets within the experimental sites, with a minimum distance of 2 m between them. The total experimental footprint in each forest was 600 m2. We planted the clones in April 2015, and the experiment ended in April 2016. We measured the initial height of all clones.
During the course of a year, the clones were searched for lepidopteran herbivores, and all leaves were photographed to measure herbivory each month. Percent herbivory was measured using ImageJ.1 Herbivory was separated based on the type of herbivore (classifications included families of Lepidoptera, Chrysomelidae, Acrididae, and other herbivores). Analyses of data from the final month of measurements are presented. Eggs, caterpillars, and pupae of Lepidoptera found on the experimental plants were photographed monthly; herbivores were left on the experimental plants to avoid interrupting herbivory. Plant height and the dry biomass of roots, stems, and leaves were measured at the end of the experiment. Relative growth in height was calculated as the final height minus the initial height divided by the initial height.
Chemical analyses
Leaf samples from each mother plant were collected for chemical analysis at the time the clones were prepared, and leaf samples were collected from plants at the end of the experiment as well. In remote field sites, flash freezing samples is not possible. As our objective was not to quantify or identify all metabolites present at a given point in time but rather to compare phytochemical similarity and richness between samples, all samples were collected following the same sampling protocol at roughly the same time of day to minimize variation associated with sample collection. These methods, although not perfect, have been used effectively in multiple studies in chemical ecology throughout the tropics (Richards et al., 2015; Salazar et al., 2018; Massad et al., 2022). Leaves were dried at ambient temperature in paper bags. Samples were ground, and 100 mg of each leaf were extracted in 800 μL of MeOH. Extracts were centrifuged at 10,000 RPM for 20 min, and the supernatant was collected and dried under N2 flow. Methanol is an effective solvent for extraction compounds of varying polarities (Huang et al., 2010; Mollik et al., 2022).
Samples were redissolved in 1 mL MeOH, and the solution (10 μL) was injected into an HPLC-HRESMS system. The HPLC (Shimadzu, Kyoto, Japan) equipped with an autosampler, binary pump, column oven, and a single-wavelength UV–Vis detector. The analyses were performed using a Kinetex C-18 column (Phenomenex, 100 A, 100 × 1 mm, 2.6 μm PFP) at 40°C. A linear gradient elution was performed using a flow rate of 200 μL/min, with solvent A (water/formic acid, 99.9:0.1 v/v) and solvent B (MeOH:formic acid, 99.9:0.1 v/v). Conditions were held at 85% A from 0 to 2 min, 70% A from 2 to 10 min, and 100% B from 35 to 38 min. MicrOQTof II (Bruker) were performed in positive-ion scan mode (100–1,000 Da), using N2 as the nebulizer gas at 4 Bar and the drying gas at 8 L/min and 4.5 kV capillary voltage at 200°C. The collision and the quadrupole energy were set to 12 and 6 eV, respectively. RF1 and RF2 funnels were programmed to 400 and 200 Vpp, respectively. The HPLC-HRESMS raw data were converted to.netCDF using Data Analysis 4.3 software (Bruker). The data were analyzed with XCMS online software (version 03.03; Tautenhahn et al., 2012; Gowda et al., 2014) for feature detection (min peak width 10; max peak width 60; mz deviation 10), retention time correction (obwarp method; profStep 0.5) and alignment (bw 5 s; minfrac 0.7; mzwd 0.025). A multigroup analysis with netCDF converted data was used to align and extract the data.2 Peak area data were scaled prior to analysis by dividing the absorbance of a given peak by the total absorbance across all peaks.
The metabolites extracted and quantified using this approach are not all assumed to be defensive, but they are likely to be part of metabolic pathways that yield defensive compounds. Overall measures of metabolite complexity or diversity should capture the defensive efficacies of different mixtures without a need for annotation or a requirement to attribute defensive attributes to any one peak (Richards et al., 2015; Dyer et al., 2018).
Data analyses
Chemical similarity between samples from different genotypes and transplant locations was visualized using principal component analysis (PCA) with the prcomp function in R version 4.0.3 (R Core Team, 2020). There were a total of 733 peaks detected across samples, and the area of each peak was used in the PCA. Data were scaled for the analysis. In order to quantify potential differences between groups, an analysis of similarity (ANOSIM) was also conducted using the Bray-Curtis distance and 9,999 permutations. The analysis was run with the vegan package (Oksanen et al., 2022). In addition, a principal coordinate analysis was conducted with the same data (see methods and results in the Supplementary material).
Phytochemical dissimilarity between planted clones at the end of the experiment and the natural population in the transplant location was calculated as the Euclidean distance between samples. The resulting variable was used in analyses of herbivory (see below). First, average peak values were calculated for each genotype-transplant location combination and the original mother plants (to represent the natural population in the planting location). Peak values were then normalized with a z-transformation and the Euclidean distance was calculated using the dist function. Phytochemical diversity was also calculated for each genotype x transplant combination at the end of the experiment and for each original mother plant (to represent the average phytochemical diversity in a given location) with Simpson’s index (Jost, 2006). Peaks were included as species, and their areas were used as abundances.
The effects of genotype (the identity of the mother plant), transplanting (being transplanted to a new site or planted in the site of origin), the transplant location, phytochemical dissimilarity, and phytochemical diversity were tested on different types of herbivory with Bayesian ANCOVA. Herbivory was analyzed separately for Lepidoptera, Chrysomelidae, and Acrididae. Lepidoptera damage came from the families Hesperiidae and Nymphalidae. Members of these families specialize on Piper. Chrysomelid species also specialize on Piper. Acrididae are generalist herbivores (Dyer et al., 2010). Models were run with the R2jags package (Su and Yajima, 2020) using four chains, a burn-in period of 1,000 iterations per chain, and 1,000,000 model iterations per chain. Continuous variables were z-transformed prior to analyses. Model performance was evaluated with trace and density plots and Geweke, Heidelberger–Welch, and Raftery–Lewis convergence diagnostics in the superdiag package (Tsai et al., 2012). Non-predictive covariates were removed until just categorial treatments remained in the models.
A Bayesian structural equation model (BSEM) was constructed to test causal relationships between relative growth rate in height, herbivory from the three distinct groups of herbivores, and the effects of phytochemical diversity (of original mother plants and individuals at the end of the experiment), phytochemical dissimilarity, genotype, and transplanting on herbivory. The direct effects of genotype and transplanting on growth were also tested. Models were built with the lavaan (Rosseel, 2012) package. Bayesian posterior probabilities for the modeled parameter estimates were calculated using the blavaan package (Merkle and Rosseel, 2016). Bayesian analyses were run with two chains of 10,000 samples, a burn-in of 20 iterations, and an adaptation of 100 iterations. Bayesian posterior probabilities are presented with marginal log-likelihood and posterior predictive p-values (PPP values). Non-predictive relationships were successively removed from models, and AIC scores and X2 goodness of fit statistics were used to determine the best fit model. All analyses were run using R version 4.0.3 (R Core Team, 2020).
Results
Peak richness (corresponding to unique chemical compounds) of transplanted clones ranged from 680 to 731. In addition, phytochemical diversity (quantified with Simpson’s index) ranged from 53.8 to 84.8, although there were no patterns in diversity between genotypes or transplant locations. Chemotypes could be distinguished based on their population of origin (ANOSIM R = 0.24, p = 0.0001) and their transplant location (ANOSIM R = 0.27, p = 0.0001), although there was much overlap between plants based on their genotype and transplant location. The first principal component of the PCA explained 24.2% of the variation in the data, and the second explained 15.8% of the variation (Figure 1; see Supplementary Figure S1 for the PCA plot with 95% confidence ellipses). The third PC explained 9.5% of the variation. The retention times and masses of the 10 peaks with the highest loadings on PC1, 2 and 3 are shown in Supplementary Figure S2. Similar results were obtained with the principal coordinates analysis (see Supplementary material).
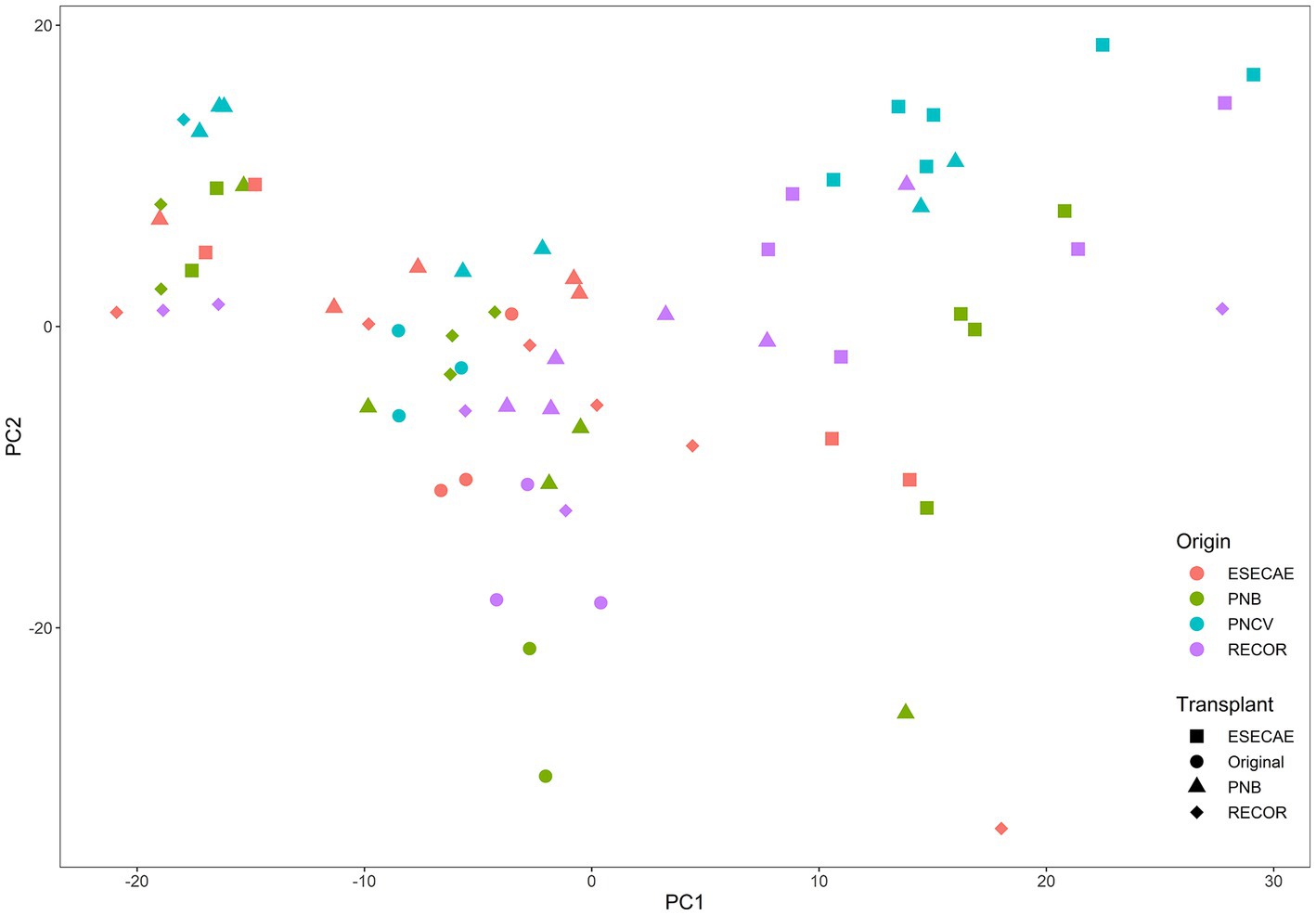
Figure 1. PCA of chemotypes from different populations transplanted in different forests. ‘Origin’ refers to the location of the mother plants that generated experimental clones. ‘Transplant’ refers to where the clones were planted, except for the ‘original’ plants which represent the mother plants from which clones were derived. The chemical profiles of all plants except the ‘originals’ were measured at the end of the experiment. Colors indicate sites of origin, and shapes indicate transplant locations.
No covariates included in the Bayesian ANCOVA were predictive, so the models were simplified to Bayesian ANOVA. Parameter estimates from the Bayesian ANOVA support the hypothesis that transplanting decreased both specialist lepidopteran and chrysomelid herbivory, and Chrysomelidae damage was higher in the Reserva Ecológica do Roncador relative to the other sites (Table 1; Figure 2). No variables were predictive of generalist acridid damage. Based on path coefficients from the Bayesian SEM, specialist lepidopteran herbivory was influenced by transplanting; lepidopteran damage was over 13 times higher on clones planted in their site of origin as opposed to those that were transplanted. Both chrysomelid herbivory and relative growth in height differed between genotypes according to the SEM. Specialist chrysomelid damage also decreased when transplanted clones were less chemically similar to the natural populations surrounding them. Again, in contrast to the specialist herbivores, nothing was predictive of generalist acridid herbivory. Lastly, herbivory did not affect growth in height (X2 = 12.05, df = 13, p = 0.52; marginal log-likelihood = −646.4, PPP = 0.56; Figure 3).

Table 1. Posterior probabilities with 90% credibility intervals from Bayesian ANOVA describing specialist Lepidoptera and chrysomelid herbivory and generalist acridid herbivory on P. arboreum from four populations transplanted across three sites. Bold values have 90% credibility intervals that do not cross zero.
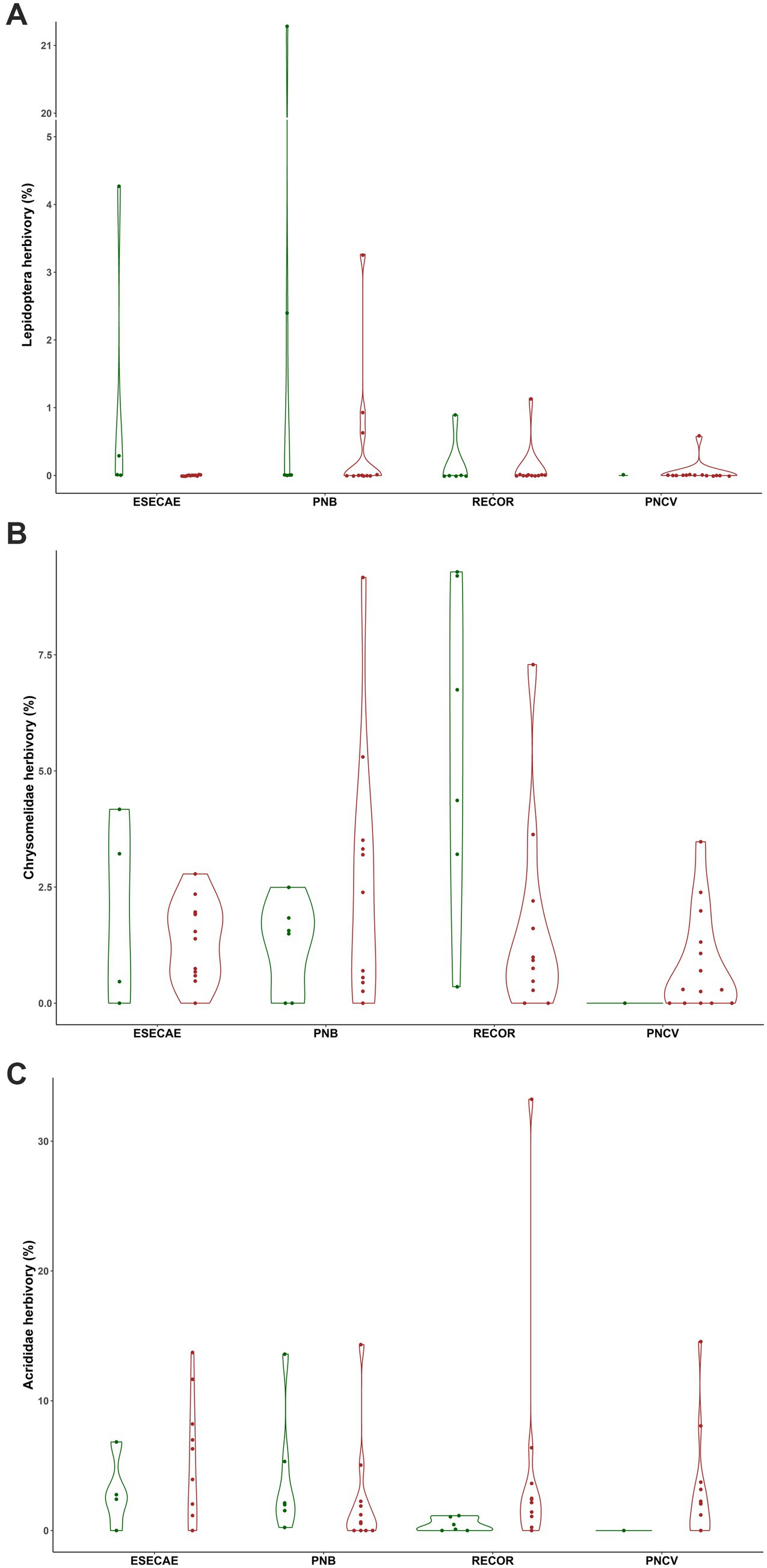
Figure 2. Violin plots and data points of (A) Lepidoptera, (B) Chrysomelidae, and (C) Acrididae herbivory as affected by transplanting and the planting location. Transplanting reduced specialist Lepidoptera and chrysomelid herbivory, and the planting location also influenced chrysomelid damage. Nothing was predictive of generalist acridid herbivory.
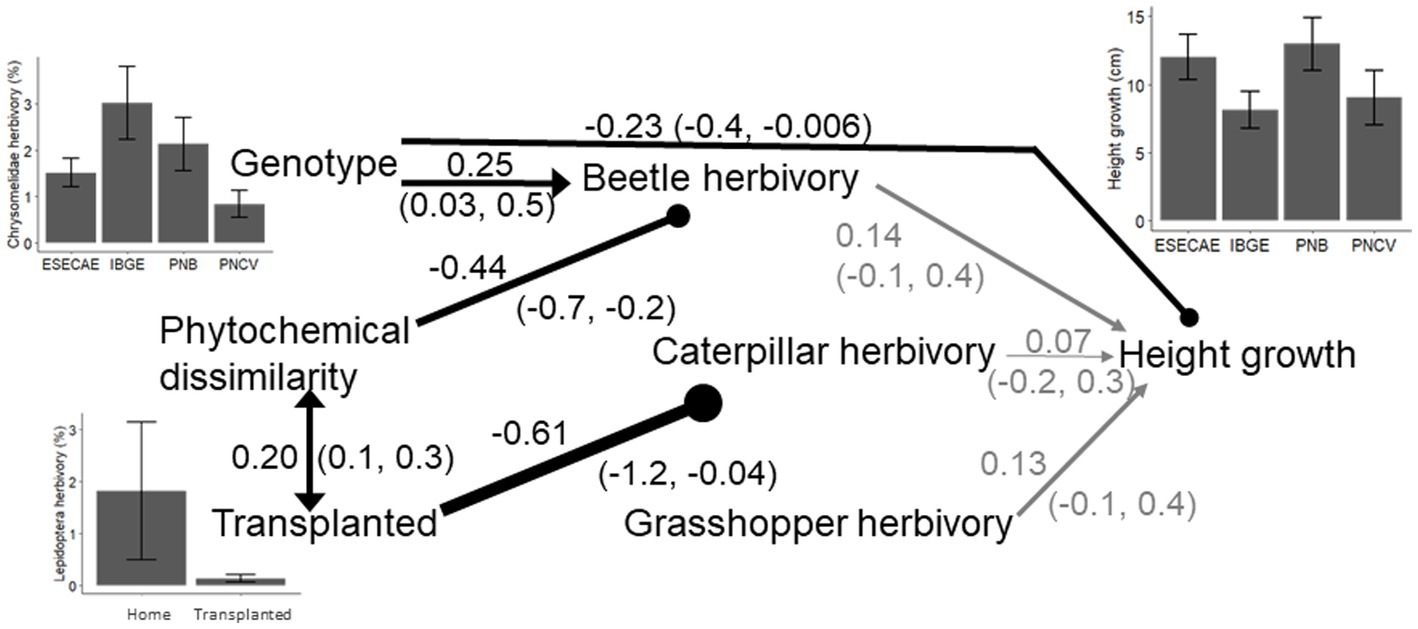
Figure 3. Bayesian structural equation model showing causal relationships (single headed arrows) and correlations (double headed arrow) between genotype, transplanting, phytochemical dissimilarity between experimental clones and native populations of Piper arboreum, herbivory and relative growth in height. Height was only affected by the original genotype. Genotype also affected beetle (chrysomelid herbivory. Beetle damage was reduced when clones were less chemically similar to the native population they were planted into, while transplanting decreased caterpillar (Lepidoptera) herbivory. Generalist grasshopper (acridid) herbivory was not affected by transplanting, phytochemical dissimilarity, or genotype. Values are Bayesian posterior probabilities and 90% credibility intervals; the thickness of the arrows indicates the relative strength of the interactions, and relationships in grey had 90% credibility intervals that crossed zero. Bar charts show the means and SE of relationships between genotype and transplanting and herbivory and growth.
Discussion
Phytochemical diversity can exert community wide effects on terrestrial insects through mediating plant–herbivore interactions and can be highly variable both between and within species (Kursar et al., 2009; Endara et al., 2015; Richards et al., 2015; Massad et al., 2017; Salazar et al., 2018; Glassmire et al., 2019; Massad et al., 2022). In the case of P. arboreum in Cerrado gallery forests, phytochemical diversity was high, differed between isolated populations, and changed with transplanting. Growth of P. arboreum was affected by genotype, which was not hypothesized, although this finding is consistent with other studies (Siemann and Rogers, 2001; Osier and Lindroth, 2006; Zhao et al., 2011; Holeski et al., 2013; Paudel et al., 2019).
As hypothesized for this system, there were population level differences in plant chemistry, changes in phytochemistry after transplanting, and greater effects of novel chemistry on specialist versus generalist herbivores. Despite the antiherbivore effects of phytochemical diversity in other Piper species (Richards et al., 2015), however, chemical diversity did not affect herbivory in our experiment. Interestingly, variation in P. arboreum phytochemistry arose post-transplanting. Although the ANOSIM distinguished between chemotypes based on their origin and transplant location, there was a great deal of overlap between chemotypes at the end of the experiment. These results indicate phytochemical expression in P. arboreum is plastic, both quantitatively and qualitatively. Other work with Piper also shows there is measurable variation in defense between subpopulations; furthermore, this variation is associated with changes in lepidopteran herbivore communities (Glassmire et al., 2016). Changes in plant chemistry in our experiment may have resulted from differential herbivory in addition to influencing herbivory. Work with another Piper species actually shows declines in imides rather than induction with herbivory (Glassmire et al., 2023).
A clear pattern emerged regarding chemical similarity and specialist herbivory on P. arboreum. Specialist chrysomelid damage was lower on clones that differed chemically from plants of the population where they were transplanted. In contrast, chemical similarity was shown to limit specialist herbivory across Piper species in the Mata Atlântica. However, those data combined chrysomelid and lepidoptera damage (Massad et al., 2022), and lepidopteran herbivory was not affected by chemistry in the present study. Nonetheless, lepidopteran herbivory was reduced more than tenfold on transplanted clones relative to clones planted in their location of origin (1.82 ± 1.3% (S.E.) vs. 0.14 ± 0.07%). This suggests that unquantified plant traits affect host selection and consumption by Lepidoptera on P. arboreum. Variation in plant nutrition may be responsible for changes in specialist damage, as specialist herbivores often respond positively to plant nutritional quality (Prudic et al., 2005; Coley et al., 2006; Kurze et al., 2017). Generalists also respond to nutrient quality (Coley et al., 2006), but specialist herbivores, particularly Lepidoptera, are often less negatively affected than generalists by specific chemical compounds (Rothwell and Holeski, 2020), supporting our results.
Generalist herbivory, both on P. arboreum and on other Piper species, is not affected by the diversity or similarity of intrafoliar chemistry (Massad et al., 2022), although generalist damage is reduced by the diversity and similarity of volatile organic compounds, which likely influence host-searching (Massad et al., 2017; Salazar et al., 2018). Overall, the pattern that generalist herbivores are more negatively affected than specialists by specific chemical compounds (Massad et al., 2011; Rothwell and Holeski, 2020) and the lack of effect of intrafoliar phytochemical similarity on generalists suggests phytochemical dissimilarity may be more relevant to specialists, such as Chrysomelidae, adapted to specific compounds in their host plants. In contrast, broadly feeding generalists may be more responsive to specific defenses than the diversity of defenses per se.
Overall, our study shows that P. arboreum exhibits high levels of phytochemical richness and plasticity. The chemical dissimilarity among plants limited specialist chrysomelid herbivory, whereas specialist lepidoptera herbivory was lower on transplanted individuals and not related to phytochemical diversity and similarity. Generalist herbivory is harder to predict, but there are documented relationships between generalists and phytochemical diversity (e.g., Salazar et al., 2018). The high degree of variation in plant chemistry observed between populations and transplanted clones suggests that phytochemical complexity is an important plant trait that may be maintained by the pressure of diverse herbivores that are differentially adapted to unique defense compounds (Kessler and Kalske, 2018; Wetzel and Whitehead, 2020). Our data on this widespread species demonstrate the potential of plants to produce a diversity of chemical compounds and the existence of plasticity in the production of specialized metabolites. Future work should seek to identify chemical compounds in metabolic extracts and continue to test the role of phytochemical diversity and chemical similarity in plant–herbivore interactions.
Data availability statement
The raw data supporting the conclusions of this article will be made available by the authors, without undue reservation.
Author contributions
RR designed the study, collected the field data and chemistry samples, and edited the manuscript. LD designed the study, assisted in the field, advised on analyses, helped write the manuscript, and provided funding. LY analyzed the chemistry samples and helped write the manuscript. ID designed the study and provided funding. WS measured the herbivory data. AK extracted the chemistry samples. MK provide laboratory support and funding and edited the manuscript. TM analyzed the data and wrote the manuscript. All authors contributed to the article and approved the submitted version.
Funding
We gratefully acknowledge the support of grants from FAPESP (2014/50316-7), CAPEA, and NSF (EN 2133818, DEB 1442103, and DEB 2114942).
Acknowledgments
We thank Cintia Lepesqueur and Tácito Barbosa Trindade for support in the field and the lab.
Conflict of interest
The authors declare that the research was conducted in the absence of any commercial or financial relationships that could be construed as a potential conflict of interest.
Publisher’s note
All claims expressed in this article are solely those of the authors and do not necessarily represent those of their affiliated organizations, or those of the publisher, the editors and the reviewers. Any product that may be evaluated in this article, or claim that may be made by its manufacturer, is not guaranteed or endorsed by the publisher.
Supplementary material
The Supplementary material for this article can be found online at: https://www.frontiersin.org/articles/10.3389/fevo.2023.1175590/full#supplementary-material
Footnotes
References
Agrawal, A. A., and Fishbein, M. (2006). Plant defense syndromes. Ecology 87, S132–S149. doi: 10.1890/0012-9658(2006)87[132:PDS]2.0.CO;2
Agrawal, A. A., Petschenka, G., Bingham, R. A., Weber, M. G., and Rasmann, S. (2012). Toxic cardenolides: chemical ecology and coevolution of specialized plant–herbivore interactions. New Phytol. 194, 28–45. doi: 10.1111/j.1469-8137.2011.04049.x
Arany, A. M., de Jong, T. J., Kim, H. K., van Dam, N. M., Choi, Y. H., van Mil, H. G. J., et al. (2009). Genotype-environment interactions affect flower and fruit herbivory and plant chemistry of Arabidopsis thaliana in a transplant experiment. Ecol. Res. 24, 1161–1171. doi: 10.1007/s11284-009-0597-2
Berenbaum, M., and Neal, J. J. (1985). Synergism between myristicin and xanthotoxin, a naturally cooccurring plant toxicant. J. Chem. Ecol. 11, 1349–1358. doi: 10.1007/BF01012136
Berenbaum, M. R., and Zangerl, A. R. (1993). Furanocoumarin metabolism in Papilio polyxenes: biochemistry, genetic variability, and ecological significance. Oecologia 95, 370–375. doi: 10.1007/BF00320991
Bowers, D. M., and Puttick, G. M. (1988). Response of generalist and specialist insects to qualitative allelochemical variation. J. Chem. Ecol. 14, 319–334. doi: 10.1007/BF01022549
Bryant, J., Chapin, F., and Klein, D. (1983). Carbon nutrient balance of boreal plants in relation to vertebrate herbivory. Oikos 40, 357–368. doi: 10.2307/3544308
Cadena-Zamudio, J. D., Monribot-Villanueva, J. L., Pérez-Torres, C.-A., Alatorre-Cobos, F., Jiménez-Moraila, B., Guerrero-Analco, J. A., et al. (2022). The use of ecological analytical tools as an unconventional approach for untargeted metabolomics data analysis: the case of Cecropia obtusifolia and its adaptive responses to nitrate starvation. Funct. Integr. Genomics 22, 1467–1493. doi: 10.1007/s10142-022-00904-1
Callejas-Posada, R (2020) Piperaceae. G. Davidse, C. Ulloa Ulloa, H. M. Hernández Macías, and S. Knapp (Eds.) Flora Mesoamericana, vol. 2, (pp. 1–590), St. Louis: Missouri Botanical Garden Press.
Campos-Moreno, D. F., Dyer, L. A., Salcido, D., Massad, T. J., Perez-Lachaud, G., Tepe, E. J., et al. (2021). Importance of interaction rewiring in determining spatial and temporal turnover of tritrophic (Piper-caterpillar-parasitoid) metanetworks in the Yucatan peninsula, Mexico. Biotropica 53, 1071–1081. doi: 10.1111/btp.12946
Chinder, G. B., Hattas, D., and Massad, T. J. (2020). Growth and functional traits of Julbernardia globiflora (Benth) resprouts and seedlings in response to fire frequency and herbivory in miombo woodlands. S. Afr. J. Bot. 135, 476–483. doi: 10.1016/j.sajb.2020.09.024
Coley, P. D., Bateman, M. L., and Kursar, T. A. (2006). The effects of plant quality on caterpillar growth and defense against natural enemies. Oikos 115, 219–228. doi: 10.1111/j.2006.0030-1299.14928.x
Coley, P., Bryant, J., and Chapin, F. (1985). Resource availability and plant antiherbivore defense. Science 230, 895–899. doi: 10.1126/science.230.4728.895
Connahs, H., Rodríguez-Castañeda, G., Walters, T., Walla, T., and Dyer, L. (2009). Geographic variation in host-specificity and parasitoid pressure of an herbivore (Geometridae) associated with the tropical genus Piper (Piperaceae). J. Insect Sci. 9:28. doi: 10.1673/031.009.2801
De-la-Cruz, I. M., Merilä, J., Valverde, P. L., Flores-Ortiz, C. M., and Núñez-Farfán, J. (2020). Genomic and chemical evidence for local adaptation in resistance to different herbivores in Datura stramonium. Evolution 74, 2629–2643. doi: 10.1111/evo.14097
Dias, BFS (1996) Cerrados: Uma caracterização. B.F.S Dias . (Eds.). Alternativas de desenvolvimento dos Cerrados: manejo e conservação dos recursos naturais renováveis. Fundação Pró-Natureza, Brasília, 11–25.
Dyer, L. A., Dodson, C. D., Stireman, J. O., Tobler, M. A., Smilanich, A. M., Fincher, R. M., et al. (2003). Synergistic effects of three Piper amides on generalist and specialist herbivores. J. Chem. Ecol. 29, 2499–2514. doi: 10.1023/a:1026310001958
Dyer, L. A., and Letourneau, D. K. (1999). Trophic cascades in a complex terrestrial community. Proc. Natl. Acad. Sci. U. S. A. 96, 5072–5076. doi: 10.1073/pnas.96.9.5072
Dyer, L. A., Letourneau, D. K., Chavarria, G. V., and Amoretti, D. S. (2010). Herbivores on a dominant understory shrub increase local plant diversity in rain forest communities. Ecology 91, 3707–3718. doi: 10.1890/08-1634.1
Dyer, L. A., and Palmer, A. D. N. (2004). Piper: a model genus for studies of Phytochemistry, ecology, and evolution. Springer US, Boston, USA
Dyer, L. A., Philbin, C. S., Ochsenrider, K. M., Richards, L. A., Massad, T. J., Smilanich, A. M., et al. (2018). Modern approaches to study plant–insect interactions in chemical ecology. Nat. Rev. Chem. 2, 50–64. doi: 10.1038/s41570-018-0009-7
Eisenring, M., Unsicker, S. B., and Lindroth, R. L. (2021). Spatial, genetic and biotic factors shape within-crown leaf trait variation and herbivore performance in a foundation tree species. Funct. Ecol. 35, 54–66. doi: 10.1111/1365-2435.13699
Endara, M.-J., Weinhold, A., Cox, J. E., Wiggins, N. L., Coley, P. D., and Kursar, T. A. (2015). Divergent evolution in antiherbivore defences within species complexes at a single Amazonian site. J. Ecol. 103, 1107–1118. doi: 10.1111/1365-2745.12431
Fajer, E., Bowers, M., and Bazzaz, F. (1992). The effect of nutrients and enriched CO2 environments on production of carbon-based allelochemicals in plantago – a test of the carbon nutrient balance hypothesis. Am. Nat. 140, 707–723. doi: 10.1086/285436
Fine, P. V. A., Mesones, I., and Coley, P. D. (2004). Herbivores promote habitat specialization by trees in Amazonian forests. Science 305, 663–665. doi: 10.1126/science.1098982
Fine, P. V. A., Metz, M. R., Lokvam, J., Mesones, I., Zuñiga, J. M. A., Lamarre, G. P. A., et al. (2013). Insect herbivores, chemical innovation, and the evolution of habitat specialization in Amazonian trees. Ecology 94, 1764–1775. doi: 10.1890/12-1920.1
Fine, P. V. A., Miller, Z. J., Mesones, I., Irazuzta, S., Appel, H. M., Stevens, M. H. H., et al. (2006). The growth-defense trade-off and habitat specialization by plants in Amazonian forests. Ecology 87, S150–S162. doi: 10.1890/0012-9658(2006)87[150:TGTAHS]2.0.CO;2
Glassmire, A. E., Carson, W. P., Smilanich, A. M., Richards, L. A., Jeffrey, C. S., Dodson, C. D., et al. (2023). Multiple and contrasting pressures determine intraspecific phytochemical variation in a tropical shrub. Oecologia 201, 991–1003. doi: 10.1007/s00442-023-05364-3
Glassmire, A. E., Jahner, J. P., Badik, K. J., Forister, M. L., Smilanich, A. M., Dyer, L. A., et al. (2017). The soil mosaic hypothesis: a synthesis of multi-trophic diversification via soil heterogeneity. Ideas Ecol. Evol. 10, 20–26. doi: 10.4033/iee.2017.10.5.n
Glassmire, A. E., Jeffrey, C. S., Forister, M. L., Parchman, T. L., Nice, C. C., Jahner, J. P., et al. (2016). Intraspecific phytochemical variation shapes community and population structure for specialist caterpillars. New Phytol. 212, 208–219. doi: 10.1111/nph.14038
Glassmire, A. E., Philbin, C., Richards, L. A., Jeffrey, C. S., Snook, J. S., and Dyer, L. A. (2019). Proximity to canopy mediates changes in the defensive chemistry and herbivore loads of an understory tropical shrub, Piper kelleyi. Ecol. Lett. 22, 332–341. doi: 10.1111/ele.13194
Gowda, H., Ivanisevic, J., Johnson, C. H., Kurczy, M. E., Benton, H. P., Rinehart, D., et al. (2014). Interactive XCMS online: simplifying data processing and statistical analyses. Anal. Chem. 86, 6931–6939. doi: 10.1021/ac500734c
Hahn, P. G., Agrawal, A. A., Sussman, K. I., and Maron, J. L. (2019). Population variation, environmental gradients, and the evolutionary ecology of plant defense against herbivory. Am. Nat. 193, 20–34. doi: 10.1086/700838
Hattas, D., Scogings, P. F., and Julkunen-Tiitto, R. (2017). Does the growth differentiation balance hypothesis explain allocation to secondary metabolites in Combretum apiculatum, an African savanna woody species? J. Chem. Ecol. 43, 153–163. doi: 10.1007/s10886-016-0808-6
Herms, D. A., and Mattson, W. J. (1992). The dilemma of plants: to grow or defend. Q. Rev. Biol. 67, 283–335. (Accessed April 2, 2020), doi: 10.1086/417659
Holeski, L. M., Zinkgraf, M. S., Couture, J. J., Whitham, T. G., and Lindroth, R. L. (2013). Transgenerational effects of herbivory in a group of long-lived tree species: maternal damage reduces offspring allocation to resistance traits, but not growth. J. Ecol. 101, 1062–1073. doi: 10.1111/1365-2745.12110
Huang, H., Morgan, C. M., Asolkar, R. N., Koivunen, M. E., and Marrone, P. G. (2010). Phytotoxicity of sarmentine isolated from long pepper (Piper longum) fruit. J. Agric. Food Chem. 58, 9994–10000. doi: 10.1021/jf102087c)
Hunter, M. D. (2016). The phytochemical landscape: linking trophic interactions and nutrient dynamics. Princeton University Press, New Jersey
Janzen, D. H. (1978) Complications in interpreting the chemical defenses of tress against tropical arboreal plant-eating vertebrates, The ecology of arboreal Folivores, Ed. G. G. Montgomerie (Smithsonian Institution Press, Washington, D.C.).
Jeschke, V., Kearney, E. E., Schramm, K., Kunert, G., Shekhov, A., Gershenzon, J., et al. (2017). How glucosinolates affect generalist lepidopteran larvae: growth, development and glucosinolate metabolism. Front. Plant Sci. 8. doi: 10.3389/fpls.2017.01995
Kato, M. J., and Furlan, M. (2007). Chemistry and evolution of the Piperaceae. Pure Appl. Chem. 79, 529–538. doi: 10.1351/pac200779040529
Kessler, A., and Kalske, A. (2018). Plant secondary metabolite diversity and species interactions. Annu. Rev. Ecol. Evol. Syst. 49, 115–138. doi: 10.1146/annurev-ecolsys-110617-062406
Koricheva, J. (2002). Meta-analysis of sources of variation in fitness costs of plant antiherbivore defenses. Ecology 83, 176–190. doi: 10.2307/2680130
Koricheva, J., Nykanen, H., and Gianoli, E. (2004). Meta-analysis of trade-offs among plant antiherbivore defenses: are plants jacks-of-all-trades, masters of all? Am. Nat. 163, E64–E75. doi: 10.1086/382601
Kursar, T. A., Dexter, K. G., Lokvam, J., Pennington, R. T., Richardson, J. E., Weber, M. G., et al. (2009). The evolution of antiherbivore defenses and their contribution to species coexistence in the tropical tree genus Inga. PNAS 106, 18073–18078. doi: 10.1073/pnas.0904786106
Kurze, S., Heinken, T., and Fartmann, T. (2017). Nitrogen enrichment of host plants has mostly beneficial effects on the life-history traits of nettle-feeding butterflies. Acta Oecol. 85, 157–164. doi: 10.1016/j.actao.2017.11.005
Laitinen, M. L., Julkunen-Tiitto, R., Tahvanainen, J., Heinonen, J., and Rousi, M. (2005). Variation in birch (Betula pendula) shoot secondary chemistry due to genotype, environment, and ontogeny. J. Chem. Ecol. 31, 697–717. doi: 10.1007/s10886-005-3539-7
Malcolm, S. B. (1994). Milkweeds, monarch butterflies and the ecological significance of cardenolides. Chemoecology 5–6, 101–117. doi: 10.1007/BF01240595
Massad, T. J., de Moraes, M. M., Philbin, C., Oliveira, C., Torrejon, G. C., Yamaguchi, L. F., et al. (2017). Similarity in volatile communities leads to increased herbivory and greater tropical forest diversity. Ecology 98, 1750–1756. doi: 10.1002/ecy.1875
Massad, T. J., and Dyer, L. A. (2010). A meta-analysis of the effects of global environmental change on plant–herbivore interactions. Arthropod-Plant Interact. 4, 181–188. doi: 10.1007/s11829-010-9102-7
Massad, T. J., Dyer, L. A., and Vega, G. C. (2012). Costs of defense and a test of the carbon-nutrient balance and growth-differentiation balance hypotheses for two co-occurring classes of plant cefense. PLoS One 7:e47554. doi: 10.1371/journal.pone.0047554
Massad, T. J., Fincher, R. M., Smilanich, A. M., and Dyer, L. (2011). A quantitative evaluation of major plant defense hypotheses, nature versus nurture, and chemistry versus ants. Arthropod-Plant Interact. 5, 125–139. doi: 10.1007/s11829-011-9121-z
Massad, T. J., Richards, L. A., Philbin, C., Yamaguchi, L. F., Kato, M. J., Jeffrey, C. S., et al. (2022). The chemical ecology of tropical forest diversity: environmental variation, chemical similarity, herbivory, and richness. Ecology 103:e3762. doi: 10.1002/ecy.3762
Merkle, E. C., and Rosseel, Y. (2016). Blavaan: Bayesian structural equation models via parameter expansion. arXiv. arXiv 1511.05604. Available at: https://arxiv.org/abs/1511.05604
Mollik, M., Rahman, M., Al-Shaeri, M., et al. (2022). Isolation, characterization and in vitro antioxidant activity screening of pure compound from black pepper (Piper nigrum). Environ. Sci. Pollut. Res. 29, 52220–52232. doi: 10.1007/s11356-022-19403-8
Navickiene, H. M. D., Morandim, A. A., Alécio, A. C., Regasini, L. O., Bergamo, D. C. B., Telascrea, M., et al. (2006). Composition and antifungal activity of essential oils from Piper aduncum, Piper arboreum and Piper tuberculatum. Quim Nova 29, 467–470. doi: 10.1590/S0100-40422006000300012
Oksanen, J., Simpson, G. L., Blanchet, F. G., Kindt, R., Legendre, P., Minchin, P. R., et al. (2022). Vegan: Community ecology package. R package version 2, 6–2 Available at: https://CRAN.R-project.org/package=vegan.
Osier, T. L., and Lindroth, R. L. (2006). Genotype and environment determine allocation to and costs of resistance in quaking aspen. Oecologia 148, 293–303. doi: 10.1007/s00442-006-0373-8
Paudel, S., Lin, P.-A., Foolad, M. R., Ali, J. G., Rajotte, E. G., and Felton, G. W. (2019). Induced plant defenses against herbivory in cultivated and wild tomato. J. Chem. Ecol. 45, 693–707. doi: 10.1007/s10886-019-01090-4
Philbin, C. S., Dyer, L. A., Jeffrey, C. S., Glassmire, A. E., and Richards, L. A. (2022). Structural and compositional dimensions of phytochemical diversity in the genus Piper reflect distinct ecological modes of action. J. Ecol. 110, 57–67. doi: 10.1111/1365-2745.13691
Prudic, K. L., Oliver, J. C., and Bowers, M. D. (2005). Soil nutrient effects on oviposition preference, larval performance, and chemical defense of a specialist insect herbivore. Oecologia 143, 578–587. doi: 10.1007/s00442-005-0008-5
R Core Team (2020). R: A language and environment for statistical computing. R Foundation for Statistical Computing, Vienna, Austria.
Richards, L. A., Dyer, L. A., Forister, M. L., Smilanich, A. M., Dodson, C. D., Leonard, M. D., et al. (2015). Phytochemical diversity drives plant–insect community diversity. PNAS 112, 10973–10978. doi: 10.1073/pnas.1504977112
Richards, L. A., Dyer, L. A., Smilanich, A. M., and Dodson, C. D. (2010). Synergistic effects of amides from two Piper species on generalist and specialist herbivores. J. Chem. Ecol. 36, 1105–1113. doi: 10.1007/s10886-010-9852-9
Richards, L. A., Lampert, E. C., Bowers, M. D., Dodson, C. D., Smilanich, A. M., and Dyer, L. A. (2012). Synergistic effects of iridoid glycosides on the survival, development and immune response of a specialist caterpillar, Junonia coenia (Nymphalidae). J. Chem. Ecol. 38, 1276–1284. doi: 10.1007/s10886-012-0190-y
Rosseel, Y. (2012). Lavaan: an R package for structural equation modeling. J. Stat. Softw. 48, 1–36. doi: 10.18637/jss.v048.i02
Rothwell, E. M., and Holeski, L. M. (2020). Phytochemical defences and performance of specialist and generalist herbivores: a meta-analysis. Ecol. Entom. 45, 396–405. doi: 10.1111/een.12809
Salazar, D., Lokvam, J., Mesones, I., Vásquez Pilco, M., Ayarza Zuñiga, J. M., de Valpine, P., et al. (2018). Origin and maintenance of chemical diversity in a species-rich tropical tree lineage. Nat. Ecol. Evol. 2, 983–990. doi: 10.1038/s41559-018-0552-0
Salazar, D., and Marquis, R. J. (2012). Herbivore pressure increases toward the equator. Proc. Natl. Acad. Sci. U. S. A. 109, 12616–12620. doi: 10.1073/pnas.1202907109
Serejo Rabelo, R., Dyer, L. A., Lepesqueur, C., Salcido, D. M., da Silva, T. P., Rodrigues, H. P. A., et al. (2021). Tritrophic interaction diversity in gallery forests: a biologically rich and understudied component of the Brazilian cerrado. Arthropod-Plant Interact. 15, 773–785. doi: 10.1007/s11829-021-09856-y
Siemann, E., and Rogers, W. E. (2001). Genetic differences in growth of an invasive tree species. Ecol. Lett. 4, 514–518. doi: 10.1046/j.1461-0248.2001.00274.x
Su, Y. S., and Yajima, M. (2020). R2jags: using R to run ‘JAGS’. R package version 0, 6–1 Available at: https://CRAN.R-project.org/package=R2jags.
Tautenhahn, R., Patti, G. J., Rinehart, D., and Siuzdak, G. (2012). XCMS online: a web-based platform to process untargeted metabolomic data. Anal. Chem. 84, 5035–5039. doi: 10.1021/ac300698c
Tsai, T-H, Gill, J, and Rapkin, J (2012) Superdiag: R code for testing Markov chain nonconvergence. R package version 1.1. Available at: https://CRAN.R-project.org/package=superdiag
Uckele, K. A., Jahner, J. P., Tepe, E. J., Richards, L. A., Dyer, L. A., Ochsenrider, K. M., et al. (2021). Phytochemistry reflects different evolutionary history in traditional classes versus specialized structural motifs. Sci. Rep. 11:17247. doi: 10.1038/s41598-021-96431-3
van Dam, N. M., Vuister, L. W. M., Bergshoeff, C., de Vos, H., and van Der Meijden, E. (1995). The “raison D’être” of pyrrolizidine alkaloids in Cynoglossum officinale: deterrent effects against generalist herbivores. J. Chem. Ecol. 21, 507–523. doi: 10.1007/BF02033698
Vasques da Silva, R., Navickiene, H. M. D., Kato, M. J., Bolzani, V. da S., Méda, C. I., Young, M. C. M., et al. (2002). Antifungal amides from Piper arboreum and Piper tuberculatum. Phytochemistry 59, 521–527. doi: 10.1016/s0031-9422(01)00431-9
Wetzel, W. C., and Whitehead, S. R. (2020). The many dimensions of phytochemical diversity: linking theory to practice. Ecol. Lett. 23, 16–32. doi: 10.1111/ele.13422
Zhao, K., Tung, C.-W., Eizenga, G. C., Wright, M. H., Ali, M. L., Price, A. H., et al. (2011). Genome-wide association mapping reveals a rich genetic architecture of complex traits in Oryza sativa. Nat. Commun. 2:467. doi: 10.1038/ncomms1467
Keywords: generalist herbivore, phytochemistry, metabolomics, Piper arboreum , transplant experiment, tropical forest, specialist herbivore
Citation: Rabelo RS, Dyer LA, Yamaguchi LF, Diniz I, Simbaña W, Kussano AJM, Kato MJ and Massad TJ (2023) Plasticity in plant defense and the role of phytochemical dissimilarity in limiting specialist herbivory. Front. Ecol. Evol. 11:1175590. doi: 10.3389/fevo.2023.1175590
Edited by:
María-José Endara, University of the Americas, EcuadorReviewed by:
Karin Schrieber, University of Kiel, GermanyDiego Salazar Amoretti, Florida International University, United States
Copyright © 2023 Rabelo, Dyer, Yamaguchi, Diniz, Simbaña, Kussano, Kato and Massad. This is an open-access article distributed under the terms of the Creative Commons Attribution License (CC BY). The use, distribution or reproduction in other forums is permitted, provided the original author(s) and the copyright owner(s) are credited and that the original publication in this journal is cited, in accordance with accepted academic practice. No use, distribution or reproduction is permitted which does not comply with these terms.
*Correspondence: Tara Joy Massad, dG1hc3NhZDc3QGdtYWlsLmNvbQ==