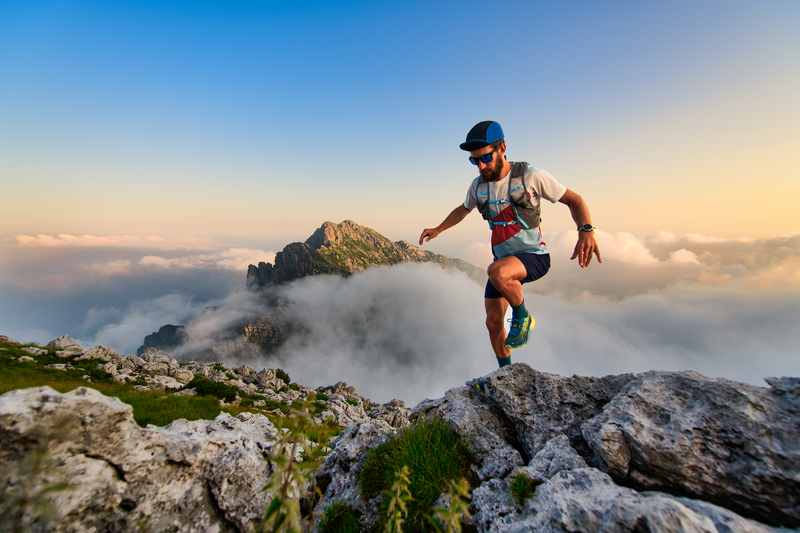
95% of researchers rate our articles as excellent or good
Learn more about the work of our research integrity team to safeguard the quality of each article we publish.
Find out more
ORIGINAL RESEARCH article
Front. Ecol. Evol. , 30 May 2023
Sec. Biogeography and Macroecology
Volume 11 - 2023 | https://doi.org/10.3389/fevo.2023.1160598
This article is part of the Research Topic The Ecological Niche at Different Spatial Scales View all 7 articles
Coleoptera are key elements of terrestrial trophic interactions and generate significant economic and ecological benefits, but their representatives also represent severe pest species. Understanding how invasive species operate is indispensable to identify and anticipate potential invasion areas. However, few studies have explored niche dynamics and drivers of invasions in this group. Here we examined niche dynamics across 54 invasive beetle species native to Europe and assessed whether factors such as human influence index, feeding habits, body size, and niche breadth are associated with the degree of invasion. The realized niches had low similarity in invasive and native ranges (i.e., invaded areas are climatically dissimilar to native ranges). This included a high degree of niche expansion in invaded areas but also environments occupied in the native ranges but unoccupied in the invasive range (unfilling), suggesting that altered species–climate relationships during invasion processes are common. Niche expansions showed positive association with small native niche breadth sizes and movements from highly disturbed native areas to less disturbed invaded ranges; unfilling was associated with invaded niche breadth size and frequency of species occurrence. Both were related to dissimilar realized climatic niches in invaded ranges. Colonization of invaded areas might be triggered by low quality resources in native areas. Unfilling levels might be related to the year of introduction and loss of biotic constraints present in their native distribution, leading to the use of different climatic spaces in the invasive areas. This idea is reinforced by larger invasive climatic niche breadth. Our results provide insight into patterns of invasive species, and initial holistic exploration towards the understanding of invasive species dynamics.
Insects are among the most predominant invasive species in the animal kingdom (Liebhold et al., 2016). Once established in non-native habitats, populations can become difficult to contain and/or manage, leading to economic, ecological, and human-health impacts (Bradshaw et al., 2016; Liebhold et al., 2016; McLaughlin and Dearden, 2019). Thus, as the first step to preventing large-scale economic and environmental damage, it is crucial to understand the factors affecting invasions. Understanding niche dynamics and mechanisms underlying invasion success is crucial in predicting potential invasion areas of non-native species and their impacts (Montagnani et al., 2022). Thus, assessing species ecological niches and their dynamics can help to elucidate patterns of species distributions and global diversity (Wiens and Graham, 2005; Wiens et al., 2010; Liu et al., 2020).
Ecological niche models (ENMs) are highly effective tools for assessing invasive species’ potential distributions (Graham et al., 2007; Araújo et al., 2011; Peterson et al., 2011; Piquet et al., 2021). However, two key assumptions are that species are in equilibrium with climate (Araújo et al., 2005) and that the niche is conserved in time and space, i.e., niche conservatism; (Peterson et al., 1999), but invasive species may undergo evolutionary niche shifts through genetic drift, selection, or hybridization; these can modify their environmental requirements to match the available conditions in invasive ranges (Stohlgren and Schnase, 2006). Given this, niche space can either be maintained, expanded, or contracted as a consequence of changes in the biotic interactions, dispersal limitation, and evolution of their niche (Chase and Leibold, 2004). This can be of particular concern as it entangles our ability to extrapolate models into climates for which native-range data are unavailable (Atwater et al., 2018).
A growing number of studies have demonstrated niche shifts during invasions (Broennimann et al., 2007; Petitpierre et al., 2012; Parravicini et al., 2015; Atwater et al., 2018), challenging niche-based invasion risk assessments as well as predictions of alien species’ potential distributions and utility of ENMs in conservation biogeography (Liu et al., 2020). Specifically to non-native invasive insect species, several studies have reported niche shifts (Parravicini et al., 2015; Hill et al., 2017; Torres et al., 2018; Bates et al., 2020), prevalence of niche expansion, and unfilling during invasions correlated to human disturbance (Hill et al., 2017). However, these changes were apparently unrelated to niche breadth (Bates et al., 2020), a factor often suggested to confer plasticity to invasive species, i.e., the “niche-breadth hypothesis” (Vazquez, 2006; Hulme and Bernard-Verdier, 2018). Nonetheless, despite general assessments (e.g., Hill et al., 2017; Bates et al., 2020), little relevant information remains available for specific groups, with validation of such patterns being limited to few insect orders.
Beetles, for instance, are the largest insect order (> 400,000 species; McKenna et al., 2019). They occupy an enormous variety of niches, display a broad range of traits, and are key elements of terrestrial trophic interactions, generating significant economic and ecological benefits (Bouchard et al., 2017). This order serves as a crucial information source for expanding and enhancing conservation strategies, addressing biological research inquiries, and offering subgroups that can be used as model organisms to investigate specific questions (e.g., Carabidae; Koivula, 2011). Species such as the lily leaf beetle, Lilioceris lilii, the Colorado potato beetle, Leptinotarsa decemlineata, the emerald ash borer, Agrilus planipennis, and the Asian Long-horned beetle, Anoplophora glabripennis are infamous for their ecological and economic impacts (Herms and McCullough, 2014; Orlova-Bienkowskaja et al., 2020). However, the niche dynamics during invasions have been examined for only a few of these species, and these show contrasting results (e.g., Medina, 2016; Silva et al., 2016; Hill et al., 2017; Srivastava et al., 2019). This places serious limitations on our ability to derive generalizations regarding trends and dynamics of invasion patterns.
Within this context, an additional major challenge remains in understanding which life-history traits might set effective colonists apart from those that are less successful and why only some species become invasive (van Kleunen et al., 2010; Davidson et al., 2011; Hänfling et al., 2011; Renault et al., 2022). Understanding functional characteristics that predispose species to become invasive (i.e., invasiveness; Lamarque et al., 2011) can improve predictions of future species’ range shifts in response to climate change (Guisan et al., 2014; Estrada et al., 2016; Simões et al., 2021), enlighten interactions among range-shifting species (Murray et al., 2002; Davidson et al., 2011; Hänfling et al., 2011; Alexander et al., 2015), and expand the power of ecosystem function and community assembly prediction (Pavoine and Bonsall, 2011; Fountain-Jones et al., 2015). So far the association between functional traits and invasiveness has been broadly studied in plant communities (Drenovsky et al., 2012; te Beest et al., 2015; Funk et al., 2016; Fried et al., 2019; Palma et al., 2021), vertebrates (Marino et al., 2022), and soil invertebrates (Thouvenot et al., 2021), but less commonly tested in beetles (Laparie et al., 2010, 2013, 2012; Evans, 2012).
Here, we investigated patterns of niche dynamics and functional traits related to invasiveness in beetles. We compiled a database of 54 invasive species representing 17 beetle families and explored climate signatures of native and invasive populations to characterize general patterns of invasive species within this group. We assessed the association between niche properties of native and invasive populations with factors that have been linked to change in niche dynamics between populations in other groups, such as human influence (Hill et al., 2017), niche breadth (Vazquez, 2006), and species life history traits (Davidson et al., 2011). In light of our results, we discussed generalizations regarding niche dynamics and similarity of the native and invaded realized niches within beetles.
A preliminary list of species was assembled based on the inventory of known invasive terrestrial arthropods of Europe (Roques et al., 2009), result of the DAISIE project (Delivering Alien Invasive Species Inventories for Europe; http://www.europe-aliens.org; Hulme and Roy, 2010), including 140 species. Based on the availability of occurrence data representing native and invasive distributions, information on pest status, and features that might assist the establishment o invasive species, 54 species were retained for further analysis.
Invasive species status (i.e., being colonizer species that can establish populations outside their native distribution with the potential to spread and negatively affect native ecosystems or local human-mediated systems; Lockwood et al., 2011) was cross-checked with information available at the invasive species databases Centre for Agriculture and Bioscience International (CABI; http://www.cabi.org) and Center for Invasive and Ecosystems health.1 Pest status was also cross-checked for each species, (i.e., organism that causes annoyance or injury to human beings, human possessions, or human interests, and causes economic loss; Hill, 1997), with information at the database Forest Pests of North America,2 CABI, and Interactive Agricultural Ecological Atlas of Russia and Neighboring Countries (AgroAtlas; http://www.agroatlas.ru; see Supplementary Table 1).
For these species, we extracted information from the literature (see Supplementary Table 1) on body size and feeding habits, which are features that, in combination with climatic suitability, can assist establishment of alien invasive insects (Peacock and Worner, 2008). When the mean value body size was not available, we estimated the mean adult size known for the species, based on the maximum and minimum sizes available in the literature (see Supplementary Table 1). To simplify the complexity of feeding habits of some species in our dataset, we distinguished three adult feeding habit categories: (1) phytophagous (i.e., feeding on the tissues of living plants, including consumption of pollen or nectar), (2) parasitic/predator (i.e., using other animals as food), and (3) detritivores (i.e., diet is primarily composed of detritus such as decaying organic matter). For phytophagous species, information regarding the number of hosts was not available. Nevertheless, it was possible to assemble information regarding host specificity for 36 species, further divided into generalists (i.e., polyphagous species that feed on different host plant families) and specialists (i.e., species that feed on host plants from single family; for references used see Supplementary Table 1).
Occurrence records for the species’ native and invaded areas were obtained from the Global Biodiversity Information System (GBIF.org; 03 June 2021 GBIF Occurrence Download https://doi.org/10.15468/dl.6jyj4v; see Supplementary Table 2), and complemented with occurrence data obtained from literature (Yu et al., 2001; Orlova-Bienkowskaja, 2013b, 2013a; Michalcewicz and Ciach, 2015; Dieni et al., 2016; Brzica, 2017; Jendek and Nakládal, 2019; Orlova-Bienkowskaja et al., 2020). Duplicates were excluded; dubious records were corrected (e.g., reversed latitude and longitude fields) or manually removed following Cobos et al. (2018).
Native ranges (i.e., the indigenous area; Pereyra, 2020) were defined as described in Beenen and Roques (2010), Cox (2007), and Löbl and Löbl (2015). Invasive ranges were considered as all the remaining points that fell outside the native ranges, and previously recorded as invaded areas in databases (i.e., CABI.org, invasive.org) and literature. In total, we obtained 138,870 occurrence records which were rarefied through spatial thinning using a 10 km distance to avoid problems derived from spatial autocorrelation, resulting in a final count of 17,341 for native populations and 9,906 records for invasive population (see Supplementary Tables 1, 2) for number of occurrences for each species). The thinning distance was chosen considering the effect of geographic clustering, the spatial resolution of variables (~5 km), and the number of remaining points after exploring distinct distance alternatives (i.e., 5 km, 10 km, 20 km, and 50 km). All steps for data cleaning and manipulation were performed using the statistical software R 3.6.2 (Team, 2021) and packages “raster” (Hijmans et al., 2014), “rgdal” (Bivand et al., 2015), and “spThin” (Aiello-Lammens et al., 2015).
We obtained environmental data for the current period from WorldClim version 2.0 (https://www.worldclim.org/; Fick and Hijmans, 2017) at 2.5 arc-minute (~5 km) spatial resolution. From the 19 climatic variables available in this database, we used eight that are known to represent environmental dimensions that limit the distribution of terrestrial invertebrates (De Meyer et al., 2010; Hill et al., 2017). These variables have been applied to a range of arthropod species, particularly invasive insects (e.g., De Meyer et al., 2010; Hill et al., 2017): mean diurnal temperature range, temperature seasonality, maximum temperature of the warmest month, minimum temperature of the coldest month, temperature annual range, precipitation of the wettest month, precipitation seasonality, and precipitation of the driest month.
To explore niche characteristics, native and invasive ranges were compared by accounting for niche overlap, similarity, and niche dynamic metrics (i.e., niche stability, expansion, and unfilling; Broennimann et al., 2012). We used the methodology of Broennimann et al. (2012), applying the R package “ecospat” (Di Cola et al., 2017), which is robust to biases due to spatial resolution and sampling efforts (Guisan et al., 2014; Atwater et al., 2018). We conducted principal components analysis (PCA) for each native and invasive geographical background. The first two PCA axes were rescaled (Broennimann et al., 2012; Petitpierre et al., 2012) to calculate the density of species occurrence points for each range using kernel smoothing methods [see Di Cola et al., 2017 for details], and then projected onto the rescaled PCA surface to create two-dimensional surfaces for native and invasive ranges. This process allows for the direct comparison among different ranges, while reducing effects of sampling bias and missing data, maximizing environmental differences between ranges, and allowing for any differences in range size to be largely discounted (Broennimann et al., 2012).
To calculate niche similarity, we used Schoener’s (1968), ranging from 0 (complete dissimilarity/no overlap) to 1 (total similarity/complete overlap). Then we applied a niche similarity test (Warren et al., 2008) to examine whether the observed overlap between the compared niches is different from the overlap between the observed niche in one range and randomly selected niches in the other range (Broennimann et al., 2012). We fixed the native niche as reference and permuted the occurrence of the species in the invasive range, which represents the null hypothesis that the environmental niche occupied in the invasive range is more similar to the environmental niche occupied in the native range than expected by chance, given the available set of environments in the invaded area. This permutation test (n = 1,000) was conducted to test the one-sided hypothesis that occupied niches have greater similarity assuming an α equal to 0.05.
For assessing niche dynamic metrics, a set of environments represents a niche expansion if it is available in both native and invasive ranges but is only occupied in the invasive range (Guisan et al., 2014). Similarly, a set of environments is considered to result from niche stability if it is occupied in both native and invaded ranges, and niche unfilling if it is used in the native range and available, but not yet exploited, in the invaded range (Figure 1; Broennimann et al., 2012; Guisan et al., 2014). Values for expansion, stability, and unfilling range from 0 to 100% considered significant at >10% (Petitpierre et al., 2012; Strubbe and Matthysen, 2014; Hill et al., 2017). This method is very sensitive to the selection of background extents (i.e., space accessible to the species and that has been sampled; Barve et al., 2011). Thus, three geographical backgrounds were tested, so to have a perspective on coherent calibration areas intersected with our presence points, i.e., biomes (using the definition of Olson et al., 2001), Köppen–Geiger climate zones (Rubel and Kottek, 2010), and buffer zone of 50 km, previously reported as the mean spread rate per year of invasive insect species (Fahrner and Aukema, 2018). For meaningful interpretation of niche dynamics, we focused on the shared climatic envelope (i.e., analogue climate) between the two ranges being considered (Fitzpatrick and Hargrove, 2009; Guisan et al., 2014). However, to evaluate whether invasive populations have expanded into non-analogous climatic space as well, we also calculated the metrics for non-analogous parts, by progressively excluding the rare climatic conditions (5, 15, and 25 percentiles of outlying climatic conditions) following (Guisan et al., 2014).
Figure 1. Schematic representation of niche dynamic metrics. Dotted lines show available environments in the native range (light gray) and invaded range (light green), and the overlap shows the analogous environments present in both ranges. Dark gray and green circles show the native and invaded niches that represent the environmental space occupied in the native and invaded ranges, respectively. The grey horizontal lines depict niche unfilling (U), with analogue conditions filled by the native niche but not filled by the introduced niche; white vertical lines depict niche stability (S) with conditions filled in both native and invaded range; and black diagonal lines depict areas of niche expansion (E), showing conditions in the introduced niche not occupied by the native niche. Figure redrawn from Guisan et al. (2014).
To estimate the occupied niche volume of populations (i.e., niche breadth), ellipsoid envelope models were created using the “ellipsenm” R package (Cobos et al., 2020). Compared to other packages, “ellipsenm” was favored due to its simplicity in estimating niche breadth and not requiring other modeling techniques or methods, as it only needs presence as species distribution data. To characterize the ellipsoid of each species, we used the centroid and a matrix of covariances of the two first principal components created with the eight environmental variables, with 95% of pairwise confidence regions for the ellipsoid.
To visually evaluate differences in niche dynamics of beetle species, the relationship between the loge ratios of native and invasive breaths (BR) were plotted against the similarity index among niches (Liu et al., 2020). BR is calculated as
We used Bayesian generalized linear models (GLM) to evaluate which set of anthropogenic factors and species traits best explains niche expansion and unfilling. Because both niche expansion and unfilling are bounded between zero and one (i.e., percentage data), we used beta regressions with a Logit link function (Geissinger et al., 2022). We also fit Bayesian generalized mixed-effects models (GLMM) including the random effect of species family (both random intercept and slopes), and additive models (GAM) to the data. In order to avoid overfitting, the number of splines in GAMs were limited to a maximum of three (Hill et al., 2017). Both additive and interaction models of the fixed effects were considered. We assumed weakly informative Gaussian priors (μ = 0, σ = 2) for the fixed effects and for the φ parameter in the beta regression, a gamma distribution with both scale and shape parameters equals to 0.01. For random effects, we used Student-t distribution priors (μ = 0, σ = 2.5, and df = 3).
Because variables related to anthropogenic impacts are calculated for both invaded and native environments, we used the loge ratios of these variables in the analyses as described in equation 1. These factors should be interpreted as the relative change in the native environment in relation to the invaded one. The anthropogenic factors included in the analysis were human population density (Oak Ridge National Laboratory; http://www.ornl.gov/sci/landscan), human modification of terrestrial systems (HTE; Kennedy et al., 2020), and human influence index (HII; Kennedy et al., 2020). Because HTE and HII were highly correlated (i.e., Pearson’s r = 0.93), HTE was excluded from the final analyses. Because the frequency of species occurrence is usually an important factor affecting both niche expansion and unfilling (Liu et al., 2020), the loge ratio of species frequency was also included in the analysis. Both native and invaded breadth, and niche status (similar or dissimilar niche) were also included as covariates. To evaluate the effects of functional traits, mean body size and feeding habits were used. Because the distribution of species body masses was slightly skewed, the loge of body mass was used. Unfortunately, data on species dispersal ability and other functional traits for beetle species are scarce (Fountain-Jones et al., 2015). Therefore, information on other traits were not available for all species evaluated in this work and consequently could not be included in the analysis.
In total, 277 models were fitted to both niche expansion and unfilling. Model selection was performed based on leave-one-out cross-validation (LOO) information criteria and LOO weights, as described in Vehtari et al. (2017). Models were fitted using Markov Chain Monte Carlo (MCMC) methods with a Hamiltonian sampler algorithm. Four independent chains were used in parallel with initial 2,500 iterations for adaptation, followed by another 5,000 iterations. Chain convergences were assessed based on Rhat values and visual inspection of diagnosing plots of the parameter estimates. To test the one-sided hypothesis that parameters were significantly different from zero, evidence ratios (ER) were used. All analyses and figures were performed in the R environment and models were fitted with the “brms” package (Bürkner, 2018). The R script used to execute all analysis is available at https://tinyurl.com/46bhdmbr.
The dataset assembled included 54 invasive beetle species, representing 17 families (Figure 2A) of two suborders. 23 species were agricultural pests; the majority were phytophagous (36 species), followed by detritivores (9) and parasitic/predator species (9). Of the phytophagous species, 19 were classified as specialists and 17 were classified as generalists. Mean body size ranged from ca. 1–30 mm (Supplementary Table 1).
Figure 2. Summary of niche dynamic metrics. (A) Results of niche dynamics recovered by expansion, stability, and unfilling. (B) Niche overlap between climatic space of native and invaded range. Asterisks indicate species with significant similarity between realized native and invasive climatic niche.
The analysis of niche dynamics was heavily influenced by the choice of geographical background. To simplify discussion, we focus here on the results from analyses using geographical background based on biomes, which showed fewer extreme values on the measures estimated (for results with other backgrounds see Supplementary Table 3). The use of biomes has been recommended in previous studies as those represent areas of ecological relevance and has been utilized in different studies (e.g., Guisan et al., 2014; Ancillotto et al., 2016).
Realized native and invasive niches were dissimilar in half of the species included in our dataset (i.e., 27 species, Figure 2B; Supplementary Table 1). Niche similarity, and niche dynamic indices (i.e., niche stability, expansion, and unfilling) were significantly different between species, but no consistent signature at the family level was recovered (Figures 2A,B, Supplementary Table 1). Climatic niches of all invasive ranges showed moderate to high degrees of niche stability when compared to the native (mean with standard deviation: 0.498 ± 0.306; Figure 2A). However, on average niche expansion and unfilling were equally high (0.502 ± 0.306 and 0.447 ± 0.382, respectively), but idiosyncratic within species (Figure 2A). Niche similarity ranged from 0 to 0.684 (Figure 2B; 0.162 ± 0.116; Supplementary Table 1). Among the species with zero similarity and highest expansion levels and unfilling are Onthophagus illyricus (Scopoli, 1763; Scarabeidae), Melanotus dichrous (Erichson, 1841; Elateridae), and Sitona cinnamomeus Allard, 1863 (Curculionidae; Figure 2A), while the species with highest similarity between ranges was Neocrepidodera brevicollis (Daniel, 1904; Chrysomelidae: 0.684; Figure 2B). Removal of rare climatic conditions hardly affected niche dynamic values (Supplementary Table 1), and contribution of variables for niche dynamics estimations of each species are given in Supplementary Figure 1).
In total, we recovered eight scenarios linking niche dynamics and similarity (Figure 3; Supplementary Figures 2, 3). In most cases, species presented a larger invasive niche and dissimilar niches (62.9%), whereas only a few species presented a larger native niche and similar niches (3.7%; Figure 3). Combinations ranged from significant realized niche similarity, but high expansion and unfilling between their native and invasive range [e.g., N. brevicollis, Rhytideres plicatus (Olivier, 1790)], to significant niche dissimilarity, but low expansion and unfilling [i.e., Onthophagus taurus (Schreber, 1759), Carabus auratus (Linnaeus, 1761)].
Figure 3. Relationships between niche similarity and breadth ratio for all species. Breadth ratio is the loge transformed ratio of the breadth of native niche to that of the invaded niche. In each panel, points with breadth ratio larger than 0 indicate that the native niche is larger than the introduced niche, and niche similarity larger than 0.5 indicates the two niches occupy a similar position in the environmental space. The number of points in each panel are shown in each corner. Black points indicate species with similar realized niches in native and invasive ranges and white circles indicate species with dissimilar realized niches.
The best fit model for niche expansion, based on LOO information criteria, was the GLM that included the additive effects of niche status, native niche breadth, and the loge ratios of HII (Figures 4A–C; parameters estimate: Supplementary Table 4, model selection analyses: Supplementary Table 5). Dissimilar niche status (ER > 1,000) and loge ratios of HII (ER = 32) have strong positive effect, and native niche breadth (ER = 665) have strong negative effect on niche expansion (Figures 4A–C). Regarding niche unfilling, the best model was the GAM that included the effects of niche status (positive effect of dissimilarity; ER > 1,000), invaded niche breadth (negative; ER > 1,000), and the loge ratio of species frequency occurrence (positive; ER > 1,000) (Figures 4D–F; parameters estimate: Supplementary Table 6; model selection analyses: Supplementary Table 7). For both niche unfilling and expansion, the random effect of species family was not included in the best selected models based on the LOO information criteria.
Figure 4. Results of the best fit models evaluating the effects of anthropogenic factors and species traits on niche expansion and unfilling: effects of (A) niche status on niche expansion; (B) native niche breadth on niche expansion; (C) loge ratio of human influence index (HII) on niche expansion; (D) niche status on niche unfilling; (E) invaded niche breadth on niche unfilling; and (F) the loge ratio of species frequency on niche unfilling. Larger points in (A,D) represent the estimated mean, and error bars represent the 95% fitted credible interval. In (B,C,E,F), solid lines represent the fitted model, and gray shaded areas represent the 95% fitted credible interval.
Invasive species are one of the main causes of biodiversity loss and global change. They frequently alter ecosystem functions, change community structures through competition with native species, and reduce native species diversity (Chapin et al., 2000; Medina, 2016; Hill et al., 2017; Fortuna et al., 2022). Despite the large diversity of beetles, the incredible diversity of life histories across terrestrial and aquatic habitats (Bouchard et al., 2017), and the fact that there are 10 times as many non-native species established worldwide as there are in any other animal taxon (Seebens et al., 2018), knowledge on the ecology of beetle invasions is limited and requires extensive exploration (Liebhold et al., 2021). Previous studies have focused on the study of spatial ecological patterns (e.g., Hanski, 1980; Burel, 1989; Roslin and Koivunen, 2001; Kautz et al., 2016), but this is the first multi-species study to explore beetles’ invasion niche dynamics and patterns. Overall, our results recovered low niche overlap between invaded and native ranges, and niche dissimilarity in half of the species studied. Further, native niche was found to be in general dissimilar and of smaller breadth when compared to invaded niche breadth (Figures 2A,B), while niche expansion and unfilling were generally high, suggesting that altered species–climate relationships during beetle invasions are common. Expansion and unfilling were also related to lack of realized native niche similarity to invaded ranges, indicating that invasive beetles expand into new climates in their invasive ranges. At the same time, levels of expansions showed positive associations with niche breadth, dissimilarity, and native ranges that are more disturbed than invaded ranges. On the other hand, unfilling was associated with invaded niche breadth size and the relative species frequency.
Our findings reinforce trends seen in studies on other groups of organisms, including reptiles (e.g., Li et al., 2014), crustaceans (e.g., Torres et al., 2018), plants (e.g., Petitpierre et al., 2012; Goncalves et al., 2014), marine fishes (e.g., Parravicini et al., 2015), and, most importantly, insects (e.g., Kumar et al., 2015; Medina, 2016; Hill et al., 2017). Local adaptation of an introduced species in new geographic areas can occur because of the frequency and magnitude of local processes in native distribution (e.g., disturbance), absence of natural enemies, competitors (e.g., congeneric native species, “Darwin naturalization hypothesis”; Darwin, 1859), predators or pathogens (Mitchell and Power, 2003). For instance, there is plenty of evidence that pests and disease vectors can develop resistance to chemicals (Hoffmann et al., 2017; Garnas, 2018; Sotka et al., 2018), likely due to the short generation time and large population size, allowing species to evolve rapidly in function of environmental stress, such as climatic conditions (Hoffmann et al., 2017). At the same time, these trends could also be related to differential availability of habitat and/or non-stationarity of species distributions in the invaded area (Vsevolodova-Perel and Sizemskaya, 2007).
Species with dissimilar realized niches in native and introduced ranges were frequently paired with high expansion and unfilling (i.e., > 10%; Petitpierre et al., 2012), indicating occupation of “exotic” climates in the invasive ranges (i.e., realized niche shift) and large availability of climatic conditions that species may further colonize in the introduced range after a certain time. High niche expansion and low unfilling could be related to the year of species’ introduction to new areas (Liu et al., 2020).
Because many invasive beetles are also agricultural pests and/or specialists, the host distribution could also be an important factor affecting the niche dynamics of beetles (Charlery de la Masselière et al., 2017). Indeed, most species that invaded dissimilar niches were pests, not only constrained by environmental conditions, and likely using a common and abundant resource (Supplementary Figure 3). A good example is Otiorhynchus cribricollis (Curculionidae), native to the Mediterranean, but a pest of apple orchards and vineyards. This species feeds on leaves and is currently widespread throughout five continents and at least eight countries (CABI.org). However, our results did not recover species traits as an important factor explaining niche dynamics (Supplementary Tables 5–7). Besides, host range was not formally included in our analysis as most species have multiple hosts from different plant families (e.g., generalists), limiting our ability to evaluate such effects. Therefore, further investigation regarding distribution in future studies could shed light into patterns regarding host range.
However, we also recovered niche similarity between realized climatic niches of the distinct ranges, regardless of the generally low overlap. This indicates that species are using similar environments in its invaded and native areas despite the availability of different environments. This might explain, for example, the high similarity and stability (i.e., 0.595 and 0.977, respectively; Supplementary Table 1) recovered for the native and invaded range of Liparus glabrirostris (Curculionidae). This species is distributed in mountains with their foothills of central and south-western Europe, from the Pyrenees across the Alps to the Carpathians, east to Ukraine (Mitrović et al., 2016), but as a montane to subalpine species absent from the plains and distributed up to 2,000 m a.s.l. This type of range might impose strong limitation regarding available landscapes for its expansion. Additionally, niche similarity within our dataset (i.e., the overlap of climatic environments between native and invasive ranges) might be aided by the fact that species introduced to climates similar to those in their native ranges are more likely to establish self-sustaining populations, thus reducing the possibility of later niche shift into “exotic” climates (Peterson et al., 1999; Wiens and Graham, 2005; Liu et al., 2020).
Anthropogenic disturbances (e.g., habitat destruction, industrialized agriculture, urbanization) are well-known to mediate the spread of invasive species, often imposing severe resource restrictions that limit productivity and niche space (Martínez-Meyer et al., 2013; Sánchez-Bayo and Wyckhuys, 2019). Here, niche expansion was found to be positively associated with higher disturbance in the native habitat accompanied by lower disturbance in the invaded range (Figure 4C). The detection of movements of species from disturbed to less disturbed areas has been commonly reported in forest ecosystems where disturbances promote migration of species to locations with less severe or frequent disturbances than the surrounding landscape (“disturbance refugia”; Krawchuk et al., 2020). Particularly in Europe, these patterns are expected due to the expansion of urban land around larger cities and along the Mediterranean coast (Kuemmerle et al., 2016). Consequently, niche expansion was positively related to dissimilar realized native and invaded climatic niches and negatively related to native niche breadth, a pattern which is supported by previous work (e.g., Bates et al., 2020; Montgomery et al., 2022). This might further indicate that ecological limits on native niche breadth could be related to constraints in the realized native niche, such as through interspecific competition and/or adaptation to a host in the invaded range.
Niche unfilling was negatively associated with invaded niche breadth, and positively to niche shift (Figure 4E). Niche unfilling refers to known suitable climate that is available in invaded ranges but not currently occupied, or species’ “opportunity to invade” (Strubbe et al., 2015; Cardador et al., 2019), and thus is usually strongly associated with the year of introduction (Liu et al., 2020). Consequently, high rates of unfilling should be positively correlated with recent introductions due to lack of time to occupy the available climatic niche of the invasive range. Unfortunately, information regarding the year of introduction of beetles is scarce due to non-reporting or the lag between establishment and discovery (Kiritani and Yamamura, 2003; Essl et al., 2011; Liebhold et al., 2021), and therefore could not be tested. Further, the ratio of records (native vs. invasive range) was also a major factor affecting niche unfilling and is reasonably coupled with the fact that a larger native niche causes higher niche unfilling (Figure 4F). Similar patterns were also found for most species from different groups (Liu et al., 2020). However, this also could be due to a statistical bias in sample size as the number of records of a species is related to the available information about it; this information is unequally distributed over species. Therefore, future studies should evaluate the effects of records of both native and invaded ranges on niche dynamics.
Functional traits (i.e., size and feeding habit) were not among the main factors related to niche dynamics. It has been suggested that the degree of invasiveness could be related to different functional traits that confer high plasticity and adaptability to new conditions, favoring the establishment and spread in new environments. Nahrung and Swain (2015) found that insects that had become successful invaders were generally those with smaller body size, more generations per year, lower incidence of diapause, longer flight season, and with close host association. Body size is also hypothesized to be mechanistically related to the increased ability to exploit resources, higher rates of intrinsic growth, quick establishment (Gaston and Lawton, 1988; Williamson and Fitter, 1996; Forys and Allen, 1999; Peacock and Worner, 2008; Devictor et al., 2010), and the ability to cope with stress (Gutiérrez-Cánovas et al., 2015). Adult feeding habits has also been considered as a relevant trait that should influence the process of colonization and settlement, with host generalists (i.e., detritivores) being more likely to encounter suitable resources and establish successfully than host specialists (Crowder and Snyder, 2010). We did not recover a clear effect of either size or feeding habit as has been demonstrated by previous studies (e.g., Liebhold et al., 2021). As several of these traits are quantified very coarsely in the literature (e.g., mean values, binary instead of quantified trait values), they might not be sufficiently precise to detect potentially existing associations.
Future studies investigating invasion dynamics should also take into account additional functional traits, such as the reproductive rate or length of different life stages, which may offer valuable insights into the mechanisms that facilitate colonization and establishment of invasive species in new ranges. In particular, as holometabolous insects, beetles may exhibit variation in the length of their immature stages. Shorter larval periods could potentially confer a competitive advantage to invasive beetles, as they would reach maturity and reproduce more quickly, enabling them to complete their life cycle faster and be less susceptible to environmental disturbances (Skarpaas and Økland, 2009; Kajita and Evans, 2010; Demidko et al., 2021). It should be noted that our results must be interpreted with caution, as estimations depend upon the environmental variables included (Strubbe and Matthysen, 2014; Liu et al., 2020); nevertheless, methodological artifacts are the same in the native and invaded areas. For example, Peterson and Nakazawa (2007) showed that models produce different predictions when using different environmental datasets. We tested only a single combination of climatic predictors known to represent environmental dimensions that limit the distribution of terrestrial invertebrates (e.g., De Meyer et al., 2010; Hill and Terblanche, 2014; Hill et al., 2017). Further, any test for niche similarity will run the risk of interpreting differences in environmental representation across areas accessible for a particular species as niche shifts (Peterson et al., 2011). Due to the lack of knowledge regarding the dispersal capability of most of the species included in the study, we used biomes to define the calibration area, what has been recommended in previous studies (e.g., Guisan et al., 2014; Ancillotto et al., 2016). However, we are aware that this representation for some species is likely more environmentally restricted than the fundamental niche, leading to the non-detection of analogous climates in invasive ranges (Pili et al., 2020). This debate is not just a technical detail, as niches indeed shift easily during species’ invasions, making predictions of the geographic potential of invasive species much more difficult. Thus, our findings have relevant implications for the use of species distribution models on the study of biological invasions.
Finally, although insects have contributed significantly to the understanding of some key issues in invasion ecology (Roderick and Navajas, 2015; Roy et al., 2016; Hill et al., 2017), geographic and taxonomic biases perceive (Hill et al., 2016). Thus, most focus has been placed in agricultural pests or vectors of human disease, representing only a small portion of the group’s diversity (Hill et al., 2016). The present study represents a substantial advance on the knowledge of invasive beetle species dynamics. However, broader exploration of niche dynamics patterns must be carried out to obtain a more complete overview of the many facets of their invasion ecology.
Overall, our findings reveal that beetle species exhibit high levels of climatic instability and are invading climatically dissimilar areas. Most species that are going through expansion are related to areas that show higher disturbance than invaded ranges, indicating that colonization might be motivated by low quality resource areas. This is extremely worrisome, as most of these species are pests and responsible for economic and ecological service losses. On the other side, unfilling levels could be related to two factors, year of introduction and loss of biotic or abiotic constraints present in the native range of their fundamental niche, leading to the use of different climatic spaces in the invasive range. This is further reinforced by the results related to niche breath, which revealed a larger invasive climatic niche breadth in comparison to native climatic niche breadth. Empirical field evidence of climatic niche shifts during biological invasions and demography dynamics is still lacking, and biomonitoring programs and natural history studies are essential to assess functional traits related to the patterns recovered in our study. Consequently, our results provide a substantial contribution to the knowledge regarding niche dynamics of invasive species in a rapidly changing world.
The original contributions presented in the study are included in the article/Supplementary material, further inquiries can be directed to the corresponding author.
MS: conceptualization, data curation, formal analysis, investigation, methodology, project administration, resources, supervision, writing – original draft, and review and editing. CN-P: conceptualization, formal analysis, investigation, methodology, and writing – review and editing. DW: conceptualization, methodology, and writing – review and editing. TS: conceptualization-supporting, resources, and writing – review and editing. MK: conceptualization, data curation, formal analysis, investigation, methodology, writing – original draft, and review and editing-equal. All authors contributed to the article and approved the submitted version.
We thank Jorge Soberón and Marlon Cobos (University of Kansas) for comments on an earlier version of the manuscript.
The authors declare that the research was conducted in the absence of any commercial or financial relationships that could be construed as a potential conflict of interest.
All claims expressed in this article are solely those of the authors and do not necessarily represent those of their affiliated organizations, or those of the publisher, the editors and the reviewers. Any product that may be evaluated in this article, or claim that may be made by its manufacturer, is not guaranteed or endorsed by the publisher.
The Supplementary material for this article can be found online at: https://www.frontiersin.org/articles/10.3389/fevo.2023.1160598/full#supplementary-material
Aiello-Lammens, M. E., Boria, R. A., Radosavljevic, A., Vilela, B., and Anderson, R. P. (2015). spThin: an R package for spatial thinning of species occurrence records for use in ecological niche models. Ecography 38, 541–545. doi: 10.1111/ecog.01132
Alexander, J. M., Diez, J. M., and Levine, J. M. (2015). Novel competitors shape species’ responses to climate change. Nature 525, 515–518. doi: 10.1038/nature14952
Ancillotto, L., Strubbe, D., Menchetti, M., and Mori, E. (2016). An overlooked invader? Ecological niche, invasion success and range dynamics of the Alexandrine parakeet in the invaded range. Biol. Invasions 18, 583–595. doi: 10.1007/s10530-015-1032-y
Araújo, M. B., Alagador, D., Cabeza, M., Nogués-Bravo, D., and Thuiller, W. (2011). Climate change threatens European conservation areas. Ecol. Lett. 14, 484–492. doi: 10.1111/j.1461-0248.2011.01610.x
Araújo, M. B., Pearson, R. G., Thuiller, W., and Erhard, M. (2005). Validation of species-climate impact models under climate change. Glob. Chang. Biol. 11, 1504–1513. doi: 10.1111/j.1365-2486.2005.01000.x
Atwater, D. Z., Ervine, C., and Barney, J. N. (2018). Climatic niche shifts are common in introduced plants. Nat. Ecol. Evol. 2, 34–43. doi: 10.1038/s41559-017-0396-z
Barve, N., Barve, V., Jiménez-Valverde, A., Lira-Noriega, A., Maher, S. P., Peterson, A. T., et al. (2011). The crucial role of the accessible area in ecological niche modeling and species distribution modeling. Ecol. Model. 222, 1810–1819. doi: 10.1016/j.ecolmodel.2011.02.011
Bates, O. K., Ollier, S., and Bertelsmeier, C. (2020). Smaller climatic niche shifts in invasive than non-invasive alien ant species. Nat. Commun. 11:5213. doi: 10.1038/s41467-020-19031-1
Beenen, R., and Roques, A. (2010). Leaf and seed beetles (Coleoptera, Chrysomelidae). Chapter 8.3. BioRisk 4, 267–292. doi: 10.3897/biorisk.4.52
Bivand, R., Keitt, T., Rowlingson, B., Pebesma, E., Summer, M., Hijmans, R., et al. (2015). Package ‘rgdal’. Bindings for the geospatial data abstraction Library. CRAN.
Bouchard, P., Smith, A. B., Douglas, H., Gimmel, M. L., Brunke, A. J., and Kanda, K. (2017). Biodiversity of Coleoptera. Insect Biodivers. Sci. Soc., 337–417. doi: 10.1002/9781118945568.ch11
Bradshaw, C. J. A., Leroy, B., Bellard, C., Roiz, D., Albert, C., Fournier, A., et al. (2016). Massive yet grossly underestimated global costs of invasive insects. Nat. Commun. 7:12986. doi: 10.1038/ncomms12986
Broennimann, O., Fitzpatrick, M. C., Pearman, P. B., Petitpierre, B., Pellissier, L., Yoccoz, N. G., et al. (2012). Measuring ecological niche overlap from occurrence and spatial environmental data. Glob. Ecol. Biogeogr. 21, 481–497. doi: 10.1111/j.1466-8238.2011.00698.x
Broennimann, O., Treier, U. A., Müller-Schärer, H., Thuiller, W., Peterson, A. T., and Guisan, A. (2007). Evidence of climatic niche shift during biological invasion. Ecol. Lett. 10, 701–709. doi: 10.1111/j.1461-0248.2007.01060.x
Brzica, M. (2017). Bio-ecological research of lily leaf beetle Lilioceris lilii Scopoli (Coleoptera: Chrysomelidae) in Bosnia and Herzegovina. Entomol. Hell. 20, 55–67. doi: 10.12681/eh.11509
Burel, F. (1989). Landscape structure effects on carabid beetles spatial patterns in western France. Landsc. Ecol. 2, 215–226. doi: 10.1007/BF00125092
Bürkner, P.-C. (2018). Advanced bayesian multilevel modeling with the R package brms. R J. 10:395. doi: 10.32614/RJ-2018-017
Cardador, L., Tella, J. L., Anadón, J. D., Abellán, P., and Carrete, M. (2019). The European trade ban on wild birds reduced invasion risks. Conserv. Lett. 12:e12631. doi: 10.1111/conl.12631
Chapin, F. S., Zavaleta, E. S., Eviner, V. T., Naylor, R. L., Vitousek, P. M., Reynolds, H. L., et al. (2000). Consequences of changing biodiversity. Nature 405, 234–242. doi: 10.1038/35012241
Charlery de la Masselière, M., Ravigné, V., Facon, B., Lefeuvre, P., Massol, F., Quilici, S., et al. (2017). Changes in phytophagous insect host ranges following the invasion of their community: long-term data for fruit flies. Ecol. Evol. 7, 5181–5190. doi: 10.1002/ece3.2968
Chase, J. M., and Leibold, M. A. (2004). Ecological niches: linking classical and contemporary approaches. Biodivers. Conserv. 13, 1791–1793. doi: 10.1023/B:BIOC.0000029366.24837.fc
Cobos, M. E., Jiménez, L., Nuñez-Penichet, C., Romero-Alvarez, D., and Simoes, M. (2018). Sample data and training modules for cleaning biodiversity information. Biodivers. Inform. 13, 49–50. doi: 10.17161/bi.v13i0.7600
Cobos, M. E., Osorio-Olvera, L., Soberón, J., Peterson, A. T., Barve, V., and Barve, N. (2020). Ellipsenm: Ecological niche’s characterizations using ellipsoids. R package.
Cox, M. (2007). Atlas of the seed and leaf beetles of Britain and Ireland (Coleoptera, Bruchidae, Chrysomelidae, Megalopodidae and Orsodacnidae). Newbury, Berkshire: Pisces Publications.
Crowder, D. W., and Snyder, W. E. (2010). Eating their way to the top? Mechanisms underlying the success of invasive insect generalist predators. Biol. Invasions 12, 2857–2876. doi: 10.1007/s10530-010-9733-8
Darwin, C. (1859). On the origins of species by means of natural selection, or the preservation of favored races in the struggle for life. John Murray, London
Davidson, A. M., Jennions, M., and Nicotra, A. B. (2011). Do invasive species show higher phenotypic plasticity than native species and, if so, is it adaptive? A meta-analysis. Ecol. Lett. 14, 419–431. doi: 10.1111/j.1461-0248.2011.01596.x
De Meyer, M., Robertson, M. P., Mansell, M. W., Ekesi, S., Tsuruta, K., Mwaiko, W., et al. (2010). Ecological niche and potential geographic distribution of the invasive fruit fly Bactrocera invadens (Diptera, Tephritidae). Bull. Entomol. Res. 100, 35–48. doi: 10.1017/S0007485309006713
Demidko, D. A., Demidko, N. N., Mikhaylov, P. V., and Sultson, S. M. (2021). Biological strategies of invasive bark beetles and borers species. Insects 12:367. doi: 10.3390/insects12040367
Devictor, V., Whittaker, R. J., and Beltrame, C. (2010). Beyond scarcity: citizen science programmes as useful tools for conservation biogeography. Divers. Distrib. 16, 354–362. doi: 10.1111/j.1472-4642.2009.00615.x
Di Cola, V., Broennimann, O., Petitpierre, B., Breiner, F. T., D’Amen, M., Randin, C., et al. (2017). Ecospat: an R package to support spatial analyses and modeling of species niches and distributions. Ecography 40, 774–787. doi: 10.1111/ecog.02671
Dieni, A., Brodeur, J., and Turgeon, J. (2016). Reconstructing the invasion history of the lily leaf beetle, Lilioceris lilii, in North America. Biol. Invasions 18, 31–44. doi: 10.1007/s10530-015-0987-z
Drenovsky, R. E., Grewell, B. J., D’Antonio, C. M., Funk, J. L., James, J. J., Molinari, N., et al. (2012). A functional trait perspective on plant invasion. Ann. Bot. 110, 141–153. doi: 10.1093/aob/mcs100
Essl, F., Dullinger, S., Rabitsch, W., Hulme, P. E., Hülber, K., Jarošík, V., et al. (2011). Socioeconomic legacy yields an invasion debt. Proc. Natl. Acad. Sci. U. S. A. 108, 203–207. doi: 10.1073/pnas.1011728108
Estrada, A., Morales-Castilla, I., Caplat, P., and Early, R. (2016). Usefulness of species traits in predicting range shifts. Trends Ecol. Evol. 31, 190–203. doi: 10.1016/j.tree.2015.12.014
Evans, M. R. (2012). Modelling ecological systems in a changing world. Philos. Trans. R. Soc. Lond. Ser. B Biol. Sci. 367, 181–190. doi: 10.1098/rstb.2011.0172
Fahrner, S., and Aukema, B. H. (2018). Correlates of spread rates for introduced insects. Glob. Ecol. Biogeogr. 27, 734–743. doi: 10.1111/geb.12737
Fick, S. E., and Hijmans, R. J. (2017). WorldClim 2: new 1-km spatial resolution climate surfaces for global land areas. Int. J. Climatol. 37, 4302–4315. doi: 10.1002/joc.5086
Fitzpatrick, M. C., and Hargrove, W. W. (2009). The projection of species distribution models and the problem of non-analog climate. Biodivers. Conserv. 18, 2255–2261. doi: 10.1007/s10531-009-9584-8
Fortuna, T. M., Le Gall, P., Mezdour, S., and Calatayud, P.-A. (2022). Impact of invasive insects on native insect communities. Curr. Opin. Insect Sci. 51:100904. doi: 10.1016/j.cois.2022.100904
Forys, E. A., and Allen, C. R. (1999). Biological invasions and deletions: community change in South Florida. Biol. Conserv. 87, 341–347. doi: 10.1016/S0006-3207(98)00073-1
Fountain-Jones, N. M., Baker, S. C., and Jordan, G. J. (2015). Moving beyond the guild concept: developing a practical functional trait framework for terrestrial beetles. Ecol. Entomol. 40, 1–13. doi: 10.1111/een.12158
Fried, G., Carboni, M., Mahaut, L., and Violle, C. (2019). Functional traits modulate plant community responses to alien plant invasion. PPEES 37, 53–63. doi: 10.1016/j.ppees.2019.02.003
Funk, J. L., Standish, R. J., Stock, W. D., and Valladares, F. (2016). Plant functional traits of dominant native and invasive species in mediterranean-climate ecosystems. Ecology 97, 75–83. doi: 10.1890/15-0974.1
Garnas, J. R. (2018). Rapid evolution of insects to global environmental change: conceptual issues and empirical gaps. Curr. Opin. Insect Sci. 29, 93–101. doi: 10.1016/j.cois.2018.07.013
Gaston, K. J., and Lawton, J. H. (1988). Patterns in body size, population dynamics, and regional distribution of bracken herbivores. Am. Nat. 132, 662–680. doi: 10.1086/284881
Geissinger, E. A., Khoo, C. L. L., Richmond, I. C., Faulkner, S. J. M., and Schneider, D. C. (2022). A case for beta regression in the natural sciences. Ecosphere 13:e3940. doi: 10.1002/ecs2.3940
Goncalves, E., Herrera, I., Duarte, M., Bustamante, R. O., Lampo, M., Velásquez, G., et al. (2014). Global invasion of Lantana camara: has the climatic niche been conserved across continents? PLoS One 9:e111468. doi: 10.1371/journal.pone.0111468
Graham, C. H., Elith, J., Hijmans, R. J., Guisan, A., Townsend Peterson, A., Loiselle, B. A., et al. (2007). The influence of spatial errors in species occurrence data used in distribution models. J. Appl. Ecol. 45, 239–247. doi: 10.1111/j.1365-2664.2007.01408.x
Guisan, A., Petitpierre, B., Broennimann, O., Daehler, C., and Kueffer, C. (2014). Unifying niche shift studies: insights from biological invasions. Trends Ecol. Evol. 29, 260–269. doi: 10.1016/j.tree.2014.02.009
Gutiérrez-Cánovas, C., Sánchez-Fernández, D., Velasco, J., Millan, A., and Bonada, N. (2015). Similarity in the difference: changes in community functional features along natural and anthropogenic stress gradients. Ecology 96, 2458–2466. doi: 10.1890/14-1447.1
Hänfling, B., Edwards, F., and Gherardi, F. (2011). Invasive alien Crustacea: dispersal, establishment, impact and control. BioControl 56, 573–595. doi: 10.1007/s10526-011-9380-8
Hanski, I. (1980). Spatial patterns and movements in coprophagous beetles. Oikos 34, 293–310. doi: 10.2307/3544289
Herms, D. A., and McCullough, D. G. (2014). Emerald ash borer invasion of North America: history, biology, ecology, impacts, and management. Annu. Rev. Entomol. 59, 13–30. doi: 10.1146/annurev-ento-011613-162051
Hijmans, R. J., Etten, J, and van Mattiuzzi, M., Sumner, M., Greenberg, J. A., Lamigueiro, O. P., Bevan, A., Racine, E. B., and Shortridge, A. (2014). Package “raster.” R.
Hill, D. S. (ed.). (1997). “Pest definitions” in The economic importance of insects (Dordrecht: Springer Netherlands), 51–63.
Hill, M. P., Clusella-Trullas, S., Terblanche, J. S., and Richardson, D. M. (2016). Drivers, impacts, mechanisms and adaptation in insect invasions. Biol. Invasions, 18, 883–891. doi: 10.1007/s10530-016-1088-3
Hill, M. P., Gallardo, B., and Terblanche, J. S. (2017). A global assessment of climatic niche shifts and human influence in insect invasions. Glob. Ecol. Biogeogr. 26, 679–689. doi: 10.1111/geb.12578
Hill, M. P., and Terblanche, J. S. (2014). Niche overlap of congeneric invaders supports a single-species hypothesis and provides insight into future invasion risk: implications for global management of the Bactrocera dorsalis complex. PLoS One 9:e90121. doi: 10.1371/journal.pone.0090121
Hoffmann, A. A., Sgrò, C. M., and Kristensen, T. N. (2017). Revisiting adaptive potential, population size, and conservation. Trends Ecol. Evol. 32, 506–517. doi: 10.1016/j.tree.2017.03.012
Hulme, P. E., and Bernard-Verdier, M. (2018). Comparing traits of native and alien plants: can we do better? Funct. Ecol. 32, 117–125. doi: 10.1111/1365-2435.12982
Hulme, P., and Roy, D. (2010). DAISIE and arthropod invasions in Europe. BioRisk 4, 1–3. doi: 10.3897/biorisk.4.41
Jendek, E., and Nakládal, O. (2019). Revision of citrus trees pests of Agrilus angulatus species–group (Coleoptera, Buprestidae) from South and Southeast Asia with description of one new species. J. Asia Pac. Entomol. 22, 316–332. doi: 10.1016/j.aspen.2019.01.004
Kajita, Y., and Evans, E. W. (2010). Relationships of body size, fecundity, and invasion success among predatory lady beetles (Coleoptera: Coccinellidae) inhabiting alfalfa fields. Ann. Entomol. Soc. 103, 750–756. doi: 10.1603/AN10071
Kautz, M., Imron, M. A., Dworschak, K., and Schopf, R. (2016). Dispersal variability and associated population-level consequences in tree-killing bark beetles. Mov. Ecol. 4, 1–12. doi: 10.1186/s40462-016-0074-9
Kennedy, C. M., Oakleaf, J. R., Theobald, D. M., Baruch-Mordo, S., and Kiesecker, J. (2020). Documentation for the Global Human Modification of Terrestrial Systems. Palisades, NY: NASA Socioeconomic Data and Applications Center (SEDAC).
Kiritani, K., and Yamamura, K. (2003) in “Exotic insects and their pathways for invasion” in invasive species: Vectors and management strategies. eds. G. M. Ruiz and J. T. Carlton (Washington, DC: Island Press), 44–67.
Koivula, M. J. (2011). Useful model organisms, indicators, or both? Ground beetles (Coleoptera, Carabidae) reflecting environmental conditions. ZooKeys 100, 287–317. doi: 10.3897/zookeys.100.1533
Krawchuk, M. A., Meigs, G. W., Cartwright, J. M., Coop, J. D., Davis, R., Holz, A., et al. (2020). Disturbance refugia within mosaics of forest fire, drought, and insect outbreaks. Front. Ecol. Environ. 18, 235–244. doi: 10.1002/fee.2190
Kuemmerle, T., Levers, C., Erb, K., Estel, S., Jepsen, M. R., Müller, D., et al. (2016). Hotspots of land use change in Europe. Environ. Res. Lett. 11:064020. doi: 10.1088/1748-9326/11/6/064020
Kumar, S., LeBrun, E. G., Stohlgren, T. J., Stabach, J. A., McDonald, D. L., Oi, D. H., et al. (2015). Evidence of niche shift and global invasion potential of the tawny crazy ant, Nylanderia fulva. Ecol. Evol. 5, 4628–4641. doi: 10.1002/ece3.1737
Lamarque, L. J., Delzon, S., and Lortie, C. J. (2011). Tree invasions: a comparative test of the dominant hypotheses and functional traits. Biol. Invasions 13, 1969–1989. doi: 10.1007/s10530-011-0015-x
Laparie, M., Larvor, V., Frenot, Y., and Renault, D. (2012). Starvation resistance and effects of diet on energy reserves in a predatory ground beetle (Merizodus soledadinus; Carabidae) invading the Kerguelen Islands. Comp. Biochem. Physiol, Part A Mol. Integr. Physiol. 161, 122–129. doi: 10.1016/j.cbpa.2011.09.011
Laparie, M., Lebouvier, M., Lalouette, L., and Renault, D. (2010). Variation of morphometric traits in populations of an invasive carabid predator (Merizodus soledadinus) within a sub-Antarctic island. Biol. Invasions 12, 3405–3417. doi: 10.1007/s10530-010-9739-2
Laparie, M., Renault, D., Lebouvier, M., and Delattre, T. (2013). Is dispersal promoted at the invasion front? Morphological analysis of a ground beetle invading the Kerguelen Islands, Merizodus soledadinus (Coleoptera, Carabidae). Biol. Invasions 15, 1641–1648. doi: 10.1007/s10530-012-0403-x
Li, Y., Liu, X., Li, X., Petitpierre, B., and Guisan, A. (2014). Residence time, expansion toward the equator in the invaded range and native range size matter to climatic niche shifts in non-native species. Glob. Ecol. Biogeogr. 23, 1094–1104. doi: 10.1111/geb.12191
Liebhold, A. M., Turner, R. M., Blake, R. E., Bertelsmeier, C., Brockerhoff, E. G., Nahrung, H. F., et al. (2021). Invasion disharmony in the global biogeography of native and non-native beetle species. Divers. Distrib. 27, 2050–2062. doi: 10.1111/ddi.13381
Liebhold, A. M., Yamanaka, T., Roques, A., Augustin, S., Chown, S. L., Brockerhoff, E. G., et al. (2016). Global compositional variation among native and non-native regional insect assemblages emphasizes the importance of pathways. Biol. Invasions 18, 893–905. doi: 10.1007/s10530-016-1079-4
Liu, C., Wolter, C., Xian, W., and Jeschke, J. M. (2020). Most invasive species largely conserve their climatic niche. Proc. Natl. Acad. Sci. U. S. A. 117, 23643–23651. doi: 10.1073/pnas.2004289117
Löbl, I., and Löbl, D. (2015). Catalogue of Palaearctic Coleoptera. Hydrophiloidea-Staphylinoidea. Vol. 2. Brill, Leiden, Boston.
Lockwood, J. L., Hoopes, M. F., and Marchetti, M. P. (2011). Non-natives: plusses of invasion ecology. Nature 475:36. doi: 10.1038/475036c
Marino, C., Leclerc, C., and Bellard, C. (2022). Profiling insular vertebrates prone to biological invasions: what makes them vulnerable? Glob. Chang. Biol. 28, 1077–1090. doi: 10.1111/gcb.15941
Martínez-Meyer, E., Díaz-Porras, D., Peterson, A. T., and Yáñez-Arenas, C. (2013). Ecological niche structure and range wide abundance patterns of species. Biol. Lett. 9:20120637. doi: 10.1098/rsbl.2012.0637
McKenna, D. D., Shin, S., Ahrens, D., Balke, M., Beza-Beza, C., Clarke, D. J., et al. (2019). The evolution and genomic basis of beetle diversity. Proc. Natl. Acad. Sci. U. S. A. 116, 24729–24737. doi: 10.1073/pnas.1909655116
McLaughlin, G. M., and Dearden, P. K. (2019). Invasive insects: management methods explored. J. Insect Sci. 17, 1–9. doi: 10.1093/jisesa/iez085
Medina, A. M. (2016). What can an invasive dung beetle tell us about niche conservatism? Environ. Entomol. 45, 1141–1145. doi: 10.1093/ee/nvw092
Michalcewicz, J., and Ciach, M. (2015). Current distribution of the Rosalia longicorn Rosalia alpina (Linnaeus, 1758) (Coleoptera: Cerambycidae) in Poland. Pol. J. Entomol. 84, 9–20. doi: 10.1515/pjen-2015-0002
Mitchell, C. E., and Power, A. G. (2003). Release of invasive plants from fungal and viral pathogens. Nature 421, 625–627. doi: 10.1038/nature01317
Mitrović, M., Tomanović, Ž., Jakovljević, M., Radović, D., Havelka, J., and Stary, P. (2016). Genetic differentiation of Liparus glabrirostris (Curculionidae: Molytinae) populations from the fragmented habitats of the Alps and Carpathian Mountains. Bull. Entomol. Res. 106, 651–662. doi: 10.1017/S0007485316000377
Montagnani, C., Casazza, G., Gentili, R., Caronni, S., and Citterio, S. (2022). Kudzu in Europe: niche conservatism for a highly invasive plant. Biol. Invasions 24, 1017–1032. doi: 10.1007/s10530-021-02706-1
Montgomery, W. I., Elwood, R. W., and Dick, J. T. A. (2022). Invader abundance and contraction of niche breadth during replacement of a native gammarid amphipod. Ecol. Evol. 12:e8500. doi: 10.1002/ece3.8500
Murray, B. R., Thrall, P. H., Gill, A. M., and Nicotra, A. B. (2002). How plant life-history and ecological traits relate to species rarity and commonness at varying spatial scales. Austral. Ecol. 27, 291–310. doi: 10.1046/j.1442-9993.2002.01181.x
Nahrung, H. F., and Swain, A. J. (2015). Strangers in a strange land: do life history traits differ for alien and native colonizers of novel environments? Biol. Invasions 17, 699–709. doi: 10.1007/s10530-014-0761-7
Olson, D. M., Dinerstein, E., Wikramanayake, E. D., Burgess, N. D., Powell, G. V. N., Underwood, E. C., et al. (2001). Terrestrial ecoregions of the world: a new map of life on earth. Bioscience 51:933. doi: 10.1641/0006-3568(2001)051[0933:TEOTWA]2.0.CO;2
Orlova-Bienkowskaja, M. J. (2013a). Dramatic expansion of the range of the invasive ash pest, buprestid beetle Agrilus planipennis Fairmaire, 1888 (Coleoptera, Buprestidae) in European Russia. Entmol. Rev. 93, 1121–1128. doi: 10.1134/S0013873813090042
Orlova-Bienkowskaja, M. J. (2013b). Dynamics of the range of lily leaf beetle (Lilioceris lilii, Chrysomelidae, Coleoptera) indicates its invasion from Asia to Europe in the 16th–17th century. Russ. J. Biol. Invasions 4, 93–104. doi: 10.1134/S2075111713020082
Orlova-Bienkowskaja, M. J., Drogvalenko, A. N., Zabaluev, I. A., Sazhnev, A. S., Peregudova, E. Y., Mazurov, S. G., et al. (2020). Current range of Agrilus planipennis Fairmaire, an alien pest of ash trees, in European Russia and Ukraine. Ann. For. Sci. 77:29. doi: 10.1007/s13595-020-0930-z
Palma, E., Vesk, P. A., White, M., Baumgartner, J. B., and Catford, J. A. (2021). Plant functional traits reflect different dimensions of species invasiveness. Ecology 102:e03317. doi: 10.1002/ecy.3317
Parravicini, V., Azzurro, E., Kulbicki, M., and Belmaker, J. (2015). Niche shift can impair the ability to predict invasion risk in the marine realm: an illustration using Mediterranean fish invaders. Ecol. Lett. 18, 246–253. doi: 10.1111/ele.12401
Pavoine, S., and Bonsall, M. B. (2011). Measuring biodiversity to explain community assembly: a unified approach. Biol. Rev. Camb. Philos. Soc. 86, 792–812. doi: 10.1111/j.1469-185X.2010.00171.x
Peacock, L., and Worner, S. P. (2008). Biological and ecological traits that assist establishment of alien invasive insects. N. Z. Plant Prot. 61, 1–7. doi: 10.30843/nzpp.2008.61.6824
Pereyra, P. J. (2020). Rethinking the native range concept. Conserv. Biol. 34, 373–377. doi: 10.1111/cobi.13406
Peterson, A. T., and Nakazawa, Y. (2007). Environmental data sets matter in ecological niche modelling: an example with Solenopsis invicta and Solenopsis richteri. Glob. Ecol. Biogeogr. 17, 135–144. doi: 10.1111/j.1466-8238.2007.00347.x
Peterson, A. T., Soberón, J., Pearson, R. G., Anderson, R. P., Martínez-Meyer, E., Nakamura, M., et al. (2011). Ecological niches and geographic distributions (MPB-49). Princeton, New Jersey: Princeton University Press.
Peterson, A. T., Soberón, J., and Sanchez-Cordero, V. (1999). Conservatism of ecological niches in evolutionary time. Science 285, 1265–1267. doi: 10.1126/science.285.5431.1265
Petitpierre, B., Kueffer, C., Broennimann, O., Randin, C., Daehler, C., and Guisan, A. (2012). Climatic niche shifts are rare among terrestrial plant invaders. Science 335, 1344–1348. doi: 10.1126/science.1215933
Pili, A. N., Tingley, R., Sy, E. Y., Diesmos, M. L. L., and Diesmos, A. C. (2020). Niche shifts and environmental non-equilibrium undermine the usefulness of ecological niche models for invasion risk assessments. Sci. Rep. 10:7972. doi: 10.1038/s41598-020-64568-2
Piquet, J. C., Warren, D. L., Saavedra Bolaños, J. F., Sánchez Rivero, J. M., Gallo-Barneto, R., Cabrera-Pérez, M. Á., et al. (2021). Could climate change benefit invasive snakes? Modelling the potential distribution of the California Kingsnake in the Canary Islands. J. Environ. Manag. 294:112917. doi: 10.1016/j.jenvman.2021.112917
Renault, D., Hess, M. C. M., Braschi, J., Cuthbert, R. N., Sperandii, M. G., Bazzichetto, M., et al. (2022). Advancing biological invasion hypothesis testing using functional diversity indices. Sci. Total Environ. 834:155102. doi: 10.1016/j.scitotenv.2022.155102
Roderick, G. R., and Navajas, M. (2015). “Invasions of terrestrial arthropods: mechanisms, pathways, and dynamics,” in Biological Invasions in Changing Ecosystems Vectors, Ecological Impacts, Management and Predictions. ed. J. Canning-Clode (Warsaw: De Gruyter Open Ltd.), 75–87.
Roslin, T., and Koivunen, A. (2001). Distribution and abundance of dung beetles in fragmented landscapes. Oecologia 69–77. doi: 10.1007/s004420000565
Roques, A., Rabitsch, W., Rasplus, J.-Y., Lopez-Vaamonde, C., Nentwig, W., and Kenis, M. (2009). “Alien terrestrial invertebrates of Europe,” in Handbook of Alien Species in Europe. Netherlands, Dordrecht: Springer, 63–79.
Roy, H. E., Brown, P. M., Adriaens, T., Berkvens, N., Borges, I., Clusella-Trullas, S., et al. (2016). The harlequin ladybird, Harmonia axyridis: global perspectives on invasion history and ecology. Biol Invasions. 18, 997–1044. doi: 10.1007/s10530-016-1077-6
Rubel, F., and Kottek, M. (2010). Observed and projected climate shifts 1901-2100 depicted by world maps of the Köppen-Geiger climate classification. Meteorol. Z. 19, 135–141. doi: 10.1127/0941-2948/2010/0430
Sánchez-Bayo, F., and Wyckhuys, K. A. G. (2019). Worldwide decline of the entomofauna: a review of its drivers. Biol. Conserv. 232, 8–27. doi: 10.1016/j.biocon.2019.01.020
Schoener, T. W. (1968). The anolis lizards of bimini: resource partitioning in a complex fauna. Ecology 49, 704–726. doi: 10.2307/1935534
Seebens, H., Blackburn, T. M., Dyer, E. E., Genovesi, P., Hulme, P. E., Jeschke, J. M., et al. (2018). Global rise in emerging alien species results from increased accessibility of new source pools. Proc. Natl. Acad. Sci. U. S. A. 115, E2264–E2273. doi: 10.1073/pnas.1719429115
Silva, D. P., Vilela, B., Buzatto, B. A., Moczek, A. P., and Hortal, J. (2016). Contextualized niche shifts upon independent invasions by the dung beetle Onthophagus taurus. Biol. Invasions 18, 3137–3148. doi: 10.1007/s10530-016-1204-4
Simões, M. V. P., Saeedi, H., Cobos, M. E., and Brandt, A. (2021). Environmental matching reveals non-uniform range-shift patterns in benthic marine Crustacea. Clim. Chang. 168:31. doi: 10.1007/s10584-021-03240-8
Skarpaas, O., and Økland, B. (2009). Timber import and the risk of forest pest introductions. J. Appl. Ecol. 46, 55–63. doi: 10.1111/j.1365-2664.2008.01561.x
Sotka, E. E., Baumgardner, A. W., Bippus, P. M., Destombe, C., Duermit, E. A., Endo, H., et al. (2018). Combining niche shift and population genetic analyses predicts rapid phenotypic evolution during invasion. Evol. Appl. 11, 781–793. doi: 10.1111/eva.12592
Srivastava, V., Lafond, V., and Griess, V. C. (2019). Species distribution models (SDM): applications, benefits and challenges in invasive species management. CAB Rev. 14, 1–13. doi: 10.1079/PAVSNNR201914020
Stohlgren, T. J., and Schnase, J. L. (2006). Risk analysis for biological hazards: what we need to know about invasive species. Risk Anal. 26, 163–173. doi: 10.1111/j.1539-6924.2006.00707.x
Strubbe, D., Beauchard, O., and Matthysen, E. (2015). Niche conservatism among non-native vertebrates in Europe and North America. Ecography 38, 321–329. doi: 10.1111/ecog.00632
Strubbe, D., and Matthysen, E. (2014). Patterns of niche conservatism among non-native birds in Europe are dependent on introduction history and selection of variables. Biol. Invasions 16, 759–764. doi: 10.1007/s10530-013-0539-3
te Beest, M., Esler, K. J., and Richardson, D. M. (2015). Linking functional traits to impacts of invasive plant species: a case study. Plant Ecol. 216, 293–305. doi: 10.1007/s11258-014-0437-5
Thouvenot, L., Ferlian, O., Beugnon, R., Künne, T., Lochner, A., Thakur, M. P., et al. (2021). Do invasive earthworms affect the functional traits of native plants? Front. Plant Sci. 12:627573. doi: 10.3389/fpls.2021.627573
Torres, U., Godsoe, W., Buckley, H. L., Parry, M., Lustig, A., and Worner, S. P. (2018). Using niche conservatism information to prioritize hotspots of invasion by non-native freshwater invertebrates in New Zealand. Divers. Distrib. 24, 1802–1815. doi: 10.1111/ddi.12818
van Kleunen, M., Dawson, W., Schlaepfer, D., Jeschke, J. M., and Fischer, M. (2010). Are invaders different? A conceptual framework of comparative approaches for assessing determinants of invasiveness. Ecol. Lett. 13, 947–958. doi: 10.1111/j.1461-0248.2010.01503.x
Vazquez, D. P. (2006). “Exploring the relationship between niche breadth and invasion success” in Conceptual ecology and invasion biology: reciprocal approaches to nature. eds. M. W. Cadotte, S. M. Mcmahon, and T. Fukami (Newbury, Berkshire), 307–322.
Vehtari, A., Gelman, A., and Gabry, J. (2017). Practical Bayesian model evaluation using leave-one-out cross-validation and WAIC. Stat. Comput. 27, 1413–1432. doi: 10.1007/s11222-016-9696-4
Vsevolodova-Perel, T. S., and Sizemskaya, M. L. (2007). Spatial structure of soil macrofauna in the northern Caspian clay semidesert. Biol. Bull. Russ. Acad. Sci. 34, 629–634. doi: 10.1134/S1062359007060143
Warren, D. L., Glor, R. E., and Turelli, M. (2008). Environmental niche equivalency versus conservatism: quantitative approaches to niche evolution. Evolution 62, 2868–2883. doi: 10.1111/j.1558-5646.2008.00482.x
Wiens, J. J., Ackerly, D. D., Allen, A. P., Anacker, B. L., Buckley, L. B., Cornell, H. V., et al. (2010). Niche conservatism as an emerging principle in ecology and conservation biology. Ecol. Lett. 13, 1310–1324. doi: 10.1111/j.1461-0248.2010.01515.x
Wiens, J. J., and Graham, C. H. (2005). Niche conservatism: integrating evolution, ecology, and conservation biology. Annu. Rev. Ecol. Evol. Syst. 36, 519–539. doi: 10.1146/annurev.ecolsys.36.102803.095431
Williamson, M. H., and Fitter, A. (1996). The characters of successful invaders. Biol. Conserv. 78, 163–170. doi: 10.1016/0006-3207(96)00025-0
Keywords: ecospat, ellipsenm, functional traits, invasive beetle species, niche breadth, niche unfilling
Citation: Simões MVP, Nuñez-Penichet C, Warren D, Schmitt T and Krull M (2023) Regional anthropogenic disturbance and species-specific niche traits influence the invasiveness of European beetle species. Front. Ecol. Evol. 11:1160598. doi: 10.3389/fevo.2023.1160598
Received: 07 February 2023; Accepted: 02 May 2023;
Published: 30 May 2023.
Edited by:
Matteo Marcantonio, Université catholique de Louvain, BelgiumReviewed by:
Philippe Vernon, University of Rennes, FranceCopyright © 2023 Simões, Nuñez-Penichet, Warren, Schmitt and Krull. This is an open-access article distributed under the terms of the Creative Commons Attribution License (CC BY). The use, distribution or reproduction in other forums is permitted, provided the original author(s) and the copyright owner(s) are credited and that the original publication in this journal is cited, in accordance with accepted academic practice. No use, distribution or reproduction is permitted which does not comply with these terms.
*Correspondence: Marianna V. P. Simões, bWFyaWFubmEuc2ltb2VzQHNlbmNrZW5iZXJnLmRl
Disclaimer: All claims expressed in this article are solely those of the authors and do not necessarily represent those of their affiliated organizations, or those of the publisher, the editors and the reviewers. Any product that may be evaluated in this article or claim that may be made by its manufacturer is not guaranteed or endorsed by the publisher.
Research integrity at Frontiers
Learn more about the work of our research integrity team to safeguard the quality of each article we publish.