- Department of Ecology and Conservation Biology, Texas A&M University, College Station, TX, United States
Despite a large body of literature investigating the effects of long-term climate trends on birds, the effects of short-term weather on individual body condition are less established. Poor body condition is associated with declines in individual fitness for many avian species, thus changes to body condition may result in altered population productivity. We utilized a large existing dataset from the Monitoring Avian Productivity and Survivorship program to analyze the effects of daily maximum temperature, daily minimum temperature, and monthly precipitation on avian body condition over a 15-year period across 79 sampling sites in the southeastern United States. We used a model selection approach with generalized additive models at both species and guild levels and found largely nonlinear responses of avian body condition to weather variables. For many species and guilds, a threshold effect was evident, after which the relationship between body condition and weather changed drastically. As extreme weather becomes more common under climate change, species will be pushed further towards or away from these thresholds. Non-linear effects were also highly species-specific and not easily explained by expected effects on food availability. Thus, avian responses to altered weather may be difficult to predict across species. We discuss the implications of these results for individual fitness and population productivity.
Introduction
Predicting the effects of climate change on wild animals is one of the major challenges facing modern ecologists (Walther et al., 2002; Moritz and Agudo, 2013). Our current rate of climatic warming is already producing changes to climate and weather patterns, including more frequent heatwaves and drought, increased average over-land precipitation, more frequent intense precipitation events, and shifts in storm tracks (IPCC, 2021). The effects of current and future weather patterns on birds are of particular interest because birds are valuable providers of ecosystem services (Sekercioglu, 2006) and perform a diverse array of ecological functions, making them important indicators of ecosystem health (Fischer et al., 2007; Bregman et al., 2016). Moreover, birds are conspicuous to the public, leading to an abundance of available community science data (Neate-Clegg et al., 2020; Binley et al., 2021), and this conspicuousness drives conservation efforts in many regions (Myers et al., 2000; Larsen et al., 2012).
Many bird species are vulnerable to the wide-ranging effects of climate change (Jetz et al., 2007; Langham et al., 2015), through a combination of exposure to changing climatic means and extremes, and intrinsic sensitivity to those changes (McCloy et al., 2022). Although several studies have provided predictions regarding phenological responses of species (Reed et al., 2013) to changing environmental variables (Crick, 2004; Jetz et al., 2007; Reif, 2013; Scridel et al., 2018), and on trait-specific responses to climate change (Jenouvrier, 2013; McLean et al., 2020), less emphasis has been placed on physiological responses to short-term weather, which can vary at fine (e.g., taxonomic grouping) and broad scales (e.g., local community and population; Parmesan et al., 2000; Maxwell et al., 2019). For example, individual physiological changes such as body size and body condition are widely accepted effects of climate change (Gardner et al., 2011), and can also be affected by short-term weather (Romero et al., 2000; Skagen and Adams, 2012; Gardner et al., 2016) and acute disturbance events. They may also indicate potential shifts in fitness and overall health (Parmesan et al., 2000; van de Pol et al., 2016; Kouba et al., 2021). Existing studies regarding the effects of short-term weather on individual wild birds tend to focus on either (a) a single species (Angelier et al., 2011; English et al., 2018), (b) a particular geographic region (McLean et al., 2018), or (c) within the context of migratory ecology (Danner et al., 2013). However, the effects of weather patterns on individual avian physiology can vary greatly by species and region (McLean et al., 2018; Lindenmayer et al., 2019), and understanding these effects is critical to accurately predict animal responses under climate change. For these reasons, more studies are needed- particularly multi-species studies in regions that are vulnerable to an increase in severe weather events, such as the coast of the Gulf of Mexico (Reece et al., 2018).
While the exact definition of body condition varies within the literature, it is commonly used as a wide-ranging indicator of avian health (Stevenson and Woods, 2006; Peig and Green, 2010; English et al., 2018) and can provide insights to disease prevalence and the health of the individual (Granthon and Williams, 2017), while also indicating a phenotypic response to climatic and environmental variables (McLean et al., 2018, 2020; Kouba et al., 2021). An individuals’ body condition can reflect early-life stressor exposure (Grace et al., 2017), and affect survival and intra-specific population dynamics (McLean et al., 2016). Body condition can also indicate phenotypic responses to climatic and environmental variables (McLean et al., 2018, 2020). For example, warmer temperatures are correlated with lower body condition in a variety of songbirds (van Buskirk et al., 2010; Gardner et al., 2016; McLean et al., 2018) an effect that is exacerbated by climate change (van Buskirk et al., 2010).
Body condition is dynamic in birds and can be affected by previous and current environmental conditions and activities (Tonra et al., 2011; Rockwell et al., 2012; Akresh et al., 2019a,b, 2021). Existing literature establishes that temperature and/or precipitation patterns may have lasting, spillover effects from the wintering grounds to breeding grounds in various passerine species (Tonra et al., 2011; Rockwell et al., 2012; Akresh et al., 2019a,b, 2021), with these effects generally being more pronounced in younger birds (Rockwell et al., 2012). The active breeding season may place additional energetic demands on adult birds through incubation and raising young. Adaptive anorexia may occur to cope with these increased energy demands, thus adaptively decreasing body condition (Walsberg, 2003). Additionally, body condition reflects fat storage for thermoregulation as well as energetic storage (Stevenson and Woods, 2006); thus, lower body condition may be adaptive under high temperatures because it results in more rapid cooling (McLean et al., 2020). Temperature and precipitation may vary greatly between exposed and sheltered sites within the same local area and can consequently affect the metabolic rates, and thus body condition, of birds (Weathers, 1979). Microhabitat variation promotes preferential use of microhabitat refugia by a number of taxa (Scheffers et al., 2013), including birds (Walsberg, 1985; Martin et al., 2015). For example, phainopeplas (Phainopepla nitens) use of microhabitat in interior woodland reflects an avoidance of more exposed areas (Walsberg, 1993) while African songbirds have been shown to preferentially select trees with a higher shade density on hot days (Martin et al., 2015).
In this study, we utilize the Monitoring Avian Productivity and Survivorship (MAPS) dataset (Institute for Bird Populations) to evaluate how passerine body condition is influenced by breeding-season weather patterns (i.e., temperature and precipitation). We chose to use breeding season data because of their widespread availability at a large scale, recognizing that high energy expenditures during the breeding season may introduce variability into the dataset. We extracted breeding season data from 79 locations of the MAPS project across the Gulf of Mexico region of the southeastern United States, including: Texas, Louisiana, Mississippi, Alabama, and Florida. We used data from these states because of the strong projected impacts of climate change in this region (Savonis et al., 2008; Anthony et al., 2009) coupled with their high ecological importance as migratory stopover locations for Neotropical migrant songbird species (Moore et al., 1990; Hobson et al., 2007). We generated a series of hypotheses predicting differential responses to weather patterns by bird species, habitat and dietary guild, and migratory status of the species (Figure 1). Keeping in mind the complexities posed by thermal microhabitat refugia, we chose broad guild classifications because of our ability to accurately classify each species and our ability to retain an adequate sample size in each category for analysis.
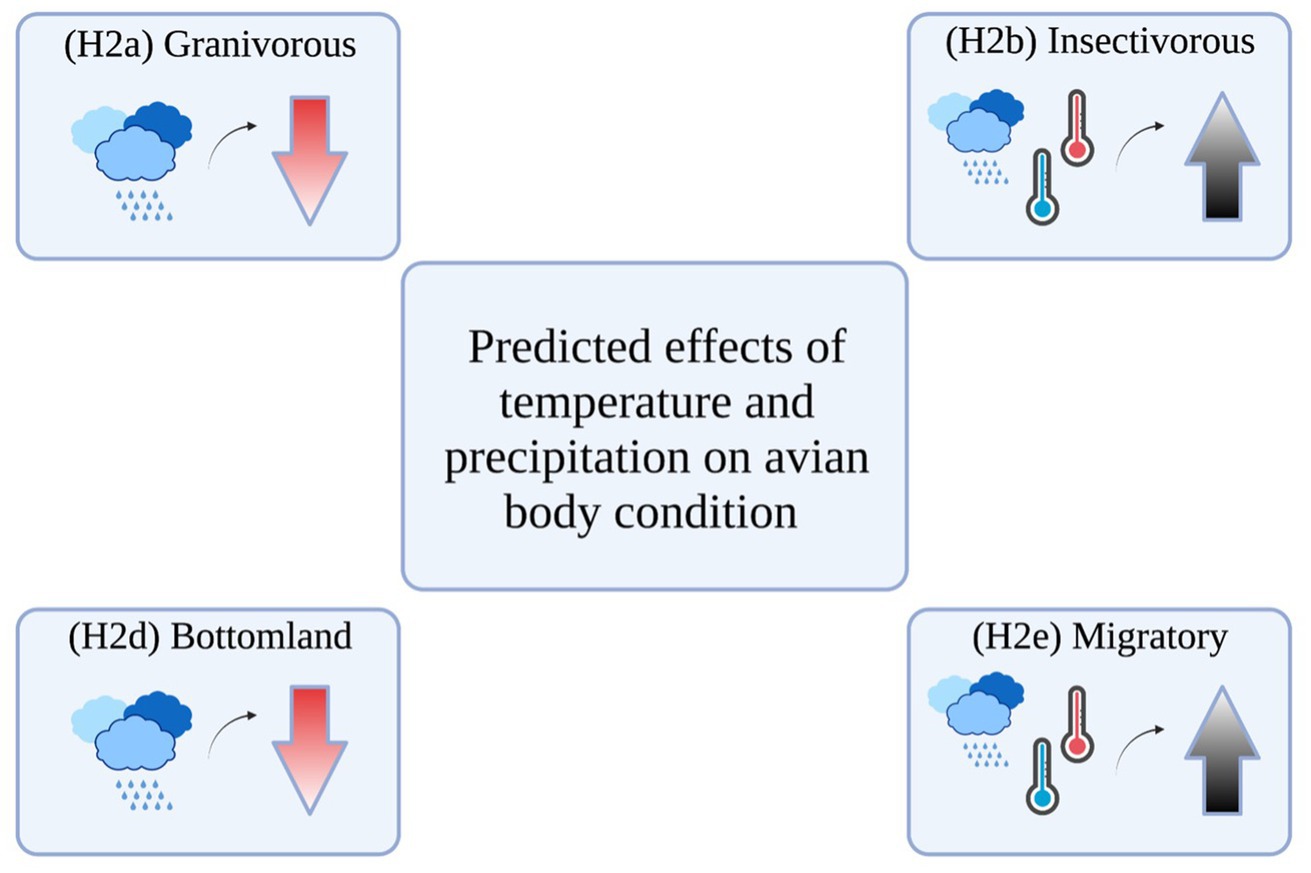
Figure 1. Predicted effects of average daily minimum temperature, average daily maximum temperature, and total monthly precipitation on guilds. (H4) was not included as no effects were expected. Created with BioRender.com.
We predicted that: (H1) precipitation would have a positive effect on granivorous species until a certain threshold is reached because low precipitation typically decreases seed production, after which flooding and excess precipitation destroys seed-producing herbaceous vegetation (Oram et al., 2021); (H2) insectivorous bird species will be positively affected by warmer temperatures and higher precipitation because insects themselves are highly sensitive to temperature and precipitation changes (Rebaudo et al., 2016; Møller, 2019; Ma et al., 2021); and (H3) omnivorous species would show little to no effect of any weather parameter on body condition because of their high foraging plasticity. For habitat guilds, we predicted that: (H4) the body condition of bottomland species would be negatively affected by precipitation after a certain threshold is reached, because flooding can decrease seed and insect abundances (Beja et al., 2010; Oram et al., 2021). Additionally, we predicted that (H5) bottomland species would be less affected by temperature than early-successional species because of the strong dichotomy in overstory cover between these habitats. As a result of the ability of habitat generalists to select a wider range of favorable microhabitats, we also predicted that (H6) temperature and precipitation would have minimal effects on this guild. Lastly, we predicted that (H7) migratory species would be positively affected by warmer temperatures and higher precipitation because the energetic expenditure of migration may make them more sensitive to changes in food sources.
Materials and methods
Avian data collection
Data were collected for this study during a 15-year period from 2005 to 2019 at 79 avian banding stations in Texas, Louisiana, Mississippi, Alabama, and Florida (United States of America) as part of the MAPS program. The MAPS program is a nationwide program operated in adherence to a standardized protocol detailed in DeSante et al. (2015). In brief, ten to twelve 30 mm mesh, 12-m mist nets were operated for one morning in each 10-day period at each station between May and August. Each captured bird was banded with a nine-digit USGS aluminum band, weighed, wing length was measured, and if possible, the species, sex, and age was determined according to Pyle (1997). Not all 79 MAPS stations were operated during the entirety of the 15-year period, but all stations were operated for at least 1 year between 2005 and 2019. Data were downloaded through the Institute for Bird Populations from the MAPSPROG database, formatted in Microsoft Excel, and statistical analyses were performed in program R (v. 3.6.0).
Sampling design
Across all station locations, we analyzed body condition from mass and wing length for 18 avian passerine species in relation to weather data (see “Weather Data” section below). We also categorized each species into three guild classifications (Table 1) using the Birds of the World database species accounts (Billerman et al., 2022). First, we grouped each species by primary habitat: bottomland forest specialist (B), early successional specialist (E), or habitat generalist (G) (Table 1). We also categorized each species by dietary guild: insectivorous (I), granivorous (G), or omnivorous (O) (Table 1). Finally, since many Neotropical migrant species are declining and of conservation concern (Rosenberg et al., 2019) we classified species as either Neotropical migrants (N) or resident (R) species (Table 1). The sample size for each species across all sites and years, after all outliers were removed (see “Data Analysis”), ranged from 300 (Bewick’s wren; Thryomanes bewickii) to 5931 (Northern cardinal; Cardinalis cardinalis), with an average sample size per species of 1963 and total sample size across species of 35326 (Supplementary Table S2). Sample sizes for guilds were: bottomland (n = 10731), early successional (n = 11995), habitat generalist (n = 12685), resident (n = 13226), migratory (n = 22100), omnivorous (n = 1703), granivorous (n = 11541), and insectivorous (n = 22821). Guild-level analyses have long been established as an effective means of analyzing avian data (Gray et al., 2007), and can provide insights that species-specific analyses oftentimes cannot. We chose these specific guilds because of our hypotheses that habitat preference and dietary habits may influence the degree to which temperature and precipitation effect individual body condition, and because of the steep population declines experienced by many North American Neotropical migrant songbird species (Rosenberg et al., 2019).
Weather data
Weather data was downloaded from DayMet (Thornton et al., 2021) via the Single Pixel Extraction Tool, which provides a 1 km2 resolution of surface weather data from each set of MAPS station coordinates. We analyzed total precipitation, average maximum temperature, and average minimum temperature for the 30 days prior to the capture of each individual bird. Thus, if a bird was captured on 30 May, weather data for that bird was collected for the period between 01 May and 30 May. We chose to analyze temperature and precipitation because the existing literature allowed us to make a priori predictions (Gardner et al., 2016; McLean et al., 2018) and there was high availability of data on a fine spatio-temporal scale. To avoid model overfitting, we did not include additional weather variables (e.g., wind, relative humidity, solar radiation) because they were not available for all sites and we had an a priori expectation that these variables would not have strong influences on avian body condition. The effects of weather on animals can be temporally heterogeneous (Salewski et al., 2013; English et al., 2018), and we chose to use a 30-day time frame instead of annualized weather data to capture shorter-term effects of extreme weather (e.g., dehydration due to high heat), along with slightly longer-term changes in resource availability.
Scaled mass index
Numerous condition indices exist for quantifying body condition. Multivariate indices are widely considered to provide the most robust and accurate assessment of the body condition of an individual (Freeman and Jackson, 1990). Although there is still ongoing debate as to which method of quantifying body condition is “best” (Labocha et al., 2014; Akresh et al., 2019b), here we chose to use scaled mass index (Peig and Green, 2009), which is an effective measure of condition in birds (Peig and Green, 2009; Danner et al., 2013; English et al., 2018). The scaled mass index approach scales body mass to body size (Danner et al., 2013), while accounting for changes in the relationship between body mass and size in an individual over time (Peig and Green, 2010). In the scaled mass index equation:
i represents scaled mass index value, Mi is body mass, L0 is the mean of a body size measure (in this study: wing length) across the population, Li is individual wing length, and bSMA is the scaling exponent, calculated as the slope of the Standardized Major Axis (SMA) regression of the log of body mass on the log of wing length (Peig and Green, 2009). We calculated scaled mass index scores separately for each species, and then standardized these scores to a mean of zero through z-scoring for guild analyses.
Data analysis
Exploratory data analysis through linear regression indicated that avian body mass was not strongly affected by time of day, Julian date, amount of body molt, and breeding condition (i.e., cloacal protuberance or brood patch), thus we did not incorporate these variables into our subsequent analyses. To evaluate the effects of temperature, precipitation, and age on avian body condition we used the ‘mgcv’ package (Wood, 2011) in the R statistical programming language (v 4.2.1; R Core Team, 2021) to construct a series of nonparametric generalized additive models (GAMs; Gaussian, identify link function) that separately predicted the scaled mass index of each species and guild. GAMs are a popular technique with large ecological datasets for modeling multiple regression functions (Yee and Mitchell, 1991; Wood, 2013; Wood et al., 2015). Moreover, unlike generalized linear models or linear mixed effects models, GAMs avoid making a priori assumptions regarding the relationship between the dependent variable and covariates. Effects are additive instead of linear, and smooths are fitted via smoothing splines using cubic polynomials.
We used a restricted maximum-likelihood approach to estimate the degree of smoothness of the model terms (Viana and Chase, 2022). Our GAMs included total precipitation, average daily maximum temperature, average daily minimum temperature, year (2005–2019), and age (hatch year or after hatch year, subsequently referred to as “juvenile” or “adult”). The relative strength of the partial effects in each model can be inferred from the y-axis values, in units of scaled mass index (or in the case of guilds, units of z-scored scaled mass index), where higher values represent stronger partial effects of the plotted variable. A positive partial effect which does not bound zero indicates that the given weather variable has a generally positive effect on body condition for that species or guild. We included “station” as a random effect (i.e., the MAPS station from which each bird was captured) to account for potential effects of geographic variation and site-specific human bias on scaled mass index.
We used the ‘dredge’ function in the ‘MuMIn’ package (Bartoń, 2009) in R to perform model selection using Akaike’s Information Criterion (AIC) on all possible models derived from our base model for each species and guild. We composed our global model using our a priori hypotheses and examined each derived model for biological relevance. Thus, we feel that the use of this dredging function was appropriate for this analysis and that our risk of post-hoc hypotheses and inadvertent inclusion of biologically improbable models was low (Delgado-Rodríguez and Llorca, 2004; Johnson and Omland, 2004). We considered all models with Δ AICc <2 (Supplementary Table S4) and then used the top model of each species and guild for assessment of parameters (Supplementary Table S3). We checked for concurvity of weather variables in all top models.
Only initial captures of each individual were analyzed to prevent potential bias from repeated captures of the same individual, an approach that was possible given the large overall sample size of our dataset. Across all species 54.2% of all records were removed, and 51% of these excluded records were recaptures. The remaining excluded records were due to missing values (48.8% of removed records), or outlying measurements (less than 1% of removed records) attributable to human error in data collection or entry. The total percentage of records removed per species ranged from 21.6% (red-eyed vireo; Vireo olivaceous) to 66.3% (prothonotary warbler; Prothonotaria citrea) with a mean of 44.7% of records removed for each of the 18 species analyzed (Supplementary Table S2).
Results
Model selection
Model selection through AICc of all possible parameter combinations indicated multiple competing top models with Δ AICc <2 in 14 of 18 species and 4 of 8 guilds, with as many as 14 models with Δ AICc <2 present for wood thrush. However, 9 of 18 species and all 8 of 8 guilds contained fewer than 5 models with Δ AICc <2. For all species and guilds, the null model (i.e., intercept-only) ranked considerably below the Δ AICc <2 set (intercept only, Supplementary Table S4). Selected top models frequently contained age (23 of 26 species and guilds), and weather parameters of precipitation (19 of 26), maximum temperature (18 of 26), and minimum temperature (17 of 26) were also comparably represented (Supplementary Table S3). The random effect of station was present in 16 of the 26 top models (Supplementary Table S3). Top models that included both minimum temperature and maximum temperature exhibited a high level of concurvity in each case (>0.90). Concurvity between all other pairs of predictor variables was significantly lower (typically <0.40).
Our global model was the top model for Carolina chickadee, white-eyed vireo, Carolina wren, hooded warbler, and northern cardinal, which incorporated the linear effect of age and the random effect of station in addition to the three weather variables. The top model for indigo bunting and prothonotary warbler contained spline effects of only precipitation. For Bewick’s wren and common yellowthroat, the top model contained the spline effect of maximum temperature alongside the linear effect of age. The top model for red-eyed vireo included the spline effect of precipitation alongside the linear effect of age and random effect of station (Supplementary Table S3).
For Acadian flycatcher and Kentucky warbler, the top model included the spline effect of minimum temperature alongside the linear effect of age and random effect of station. For wood thrush, the top model consisted of the spline effects of maximum temperature and minimum temperature along with the linear effect of age. The top model for field sparrow included the spline effects of minimum temperature and precipitation, alongside the linear effect of age and random effect of station. The top models for painted bunting and yellow-breasted chat included only the spline effects of maximum temperature and precipitation. For tufted titmouse, the top model included the spline effects of maximum temperature and minimum temperature alongside the linear effect of age and random effect of station. Finally, the top model for Swainson’s warbler included no weather parameters and only the linear effect of age (Supplementary Table S3).
Our global model containing all three spline effects of maximum temperature, minimum temperature, and precipitation alongside the linear effect of age and random effect of station were the top model for five of the eight guilds: the granivorous and insectivorous dietary guilds, the resident (nonmigratory) guild, the bottomland guild, and the habitat generalist guild. Additionally, the top model for the early successional guild contained all three parameters while dropping the random effect of station. For the migrant and resident guilds, the top model included all three spline effects of maximum temperature, minimum temperature, and precipitation alongside the random effect of station. Finally, the dietary generalist guild had only the spline effect of precipitation along with the linear effect of age in its top model (Supplementary Table S3).
Effects of weather parameters on scaled mass index
Average minimum temperature
We found that average daily minimum temperature had varied effects across species and guilds. For six species (Carolina chickadee, northern cardinal, hooded warbler, tufted titmouse, Carolina wren, and white-eyed vireo), daily minimum temperature had a negative effect on scaled mass index, up to a critical point between 10 and 15°C, after which this negative effect either stabilized (Carolina wren, hooded warbler, and white-eyed vireo) or reversed (Carolina chickadee, northern cardinal, and tufted titmouse; Figure 2). The 95 and 85% CIs for minimum temperature did not overlap zero for any of these six species (Supplementary Table S5). We found a dynamic but generally negative relationship between average daily minimum temperature and scaled mass index for the Acadian flycatcher, field sparrow, and Kentucky warbler (Figure 3). Again, the negative effect was strongest prior to 10–15°C. The 95 and 85% CIs did not overlap zero for the Acadian flycatcher and field sparrow (Supplementary Table S5), but closely approached zero for the Kentucky warbler, indicating a weak effect. The effect of minimum temperature was negligible for wood thrush (Supplementary Figure S4), where both the lower 95 and 85% CIs closely approached zero (lower 95% CI: 2.23e-47; lower 85% CI: 7.92e-36).
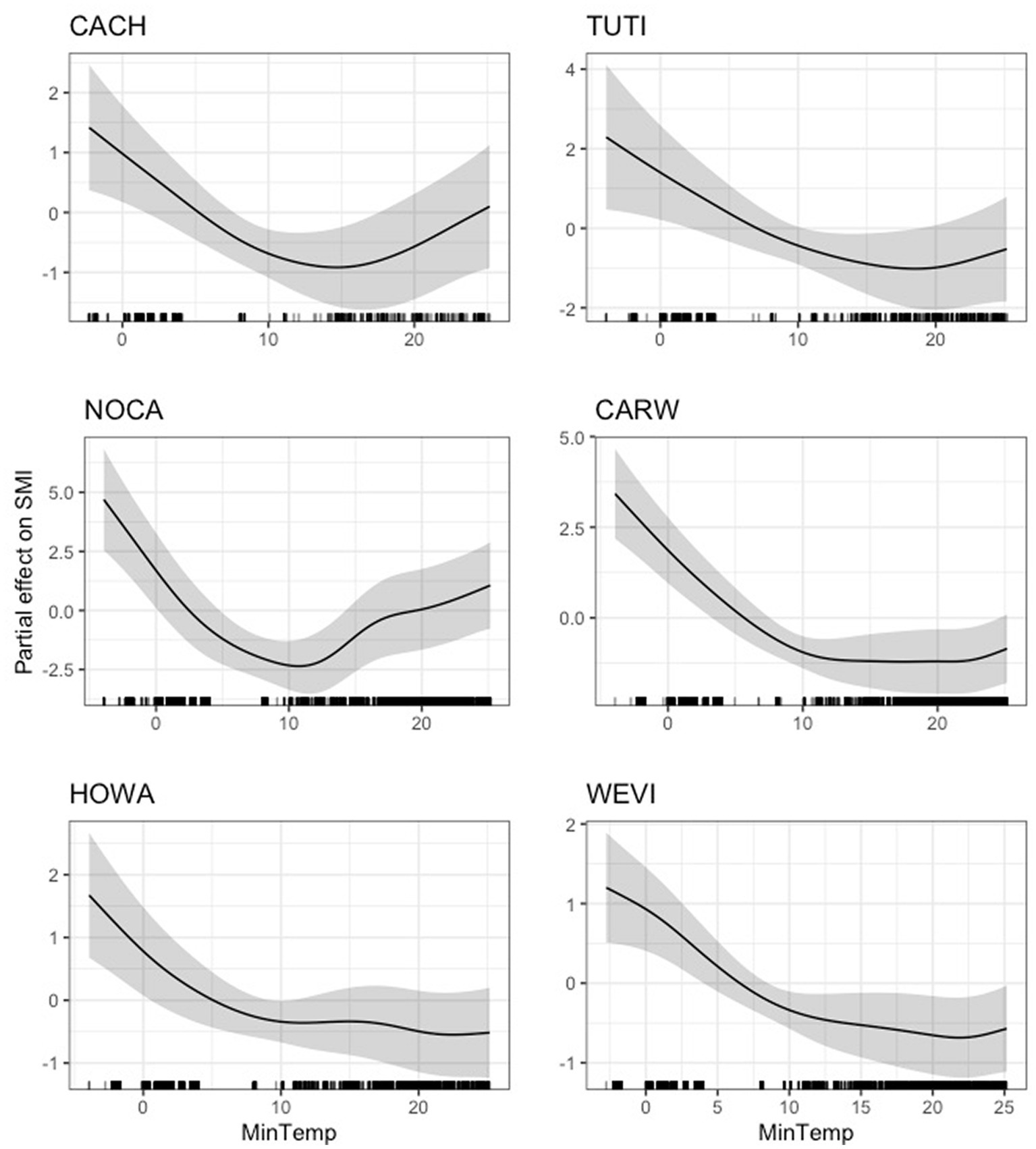
Figure 2. Partial effect of average daily minimum temperature (degrees Celsius) on scaled mass index for six species. Left: Carolina chickadee (CACH, top), northern cardinal (NOCA, center), hooded warbler (HOWA, bottom); Right: tufted titmouse (TUTI, top), Carolina wren (CARW, center), white-eyed vireo (WEVI, bottom). Black lines along the x-axis reflect the distribution of individual data points.
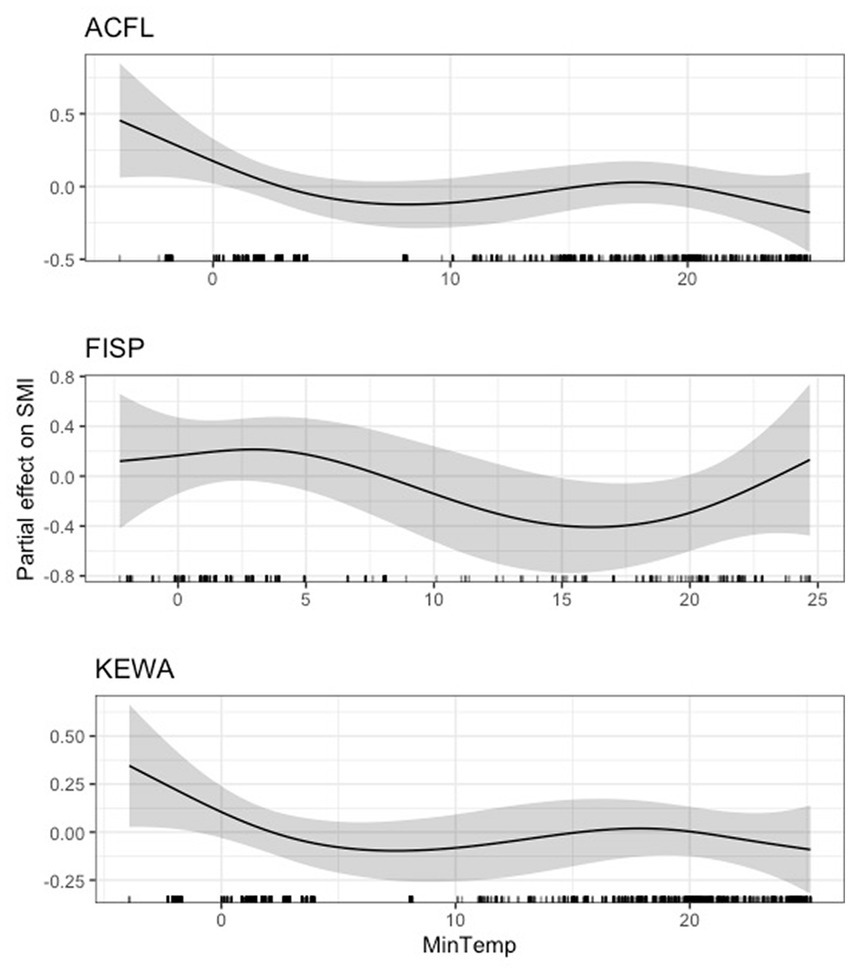
Figure 3. Partial effect of average daily minimum temperature (degrees Celsius) on scaled mass index for Acadian flycatcher (ACFL, top), field sparrow (FISP, center), and Kentucky warbler (KEWA, bottom).
At the guild level, we also found a negative effect of minimum temperature on body condition at very low temperatures (i.e., below 10°C). This inflection point remained relatively even across all guilds. After this there was a generally a weak, positive effect of minimum temperature on body condition (Figures 4, 5). The 95 and 85% CIs for minimum temperature did not overlap zero for any guild analyzed (Supplementary Table S5).
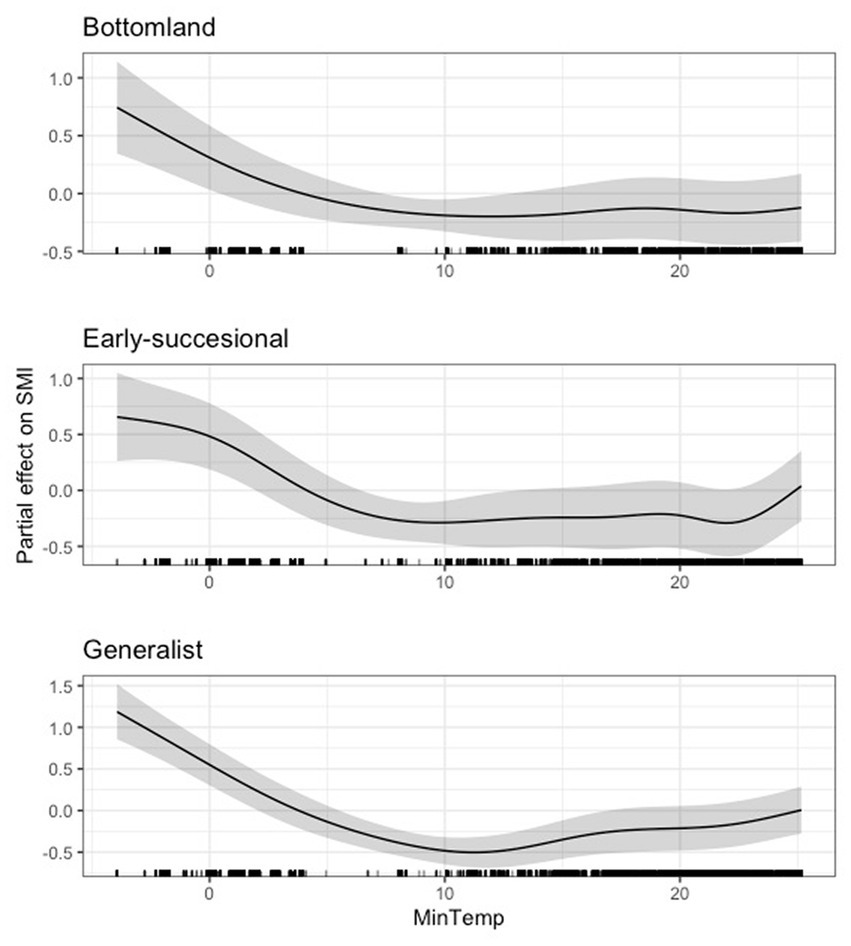
Figure 4. Partial effect of average daily minimum temperature (degrees Celsius) on scaled mass index for the bottomland guild (top), early successional guild (center), and habitat generalist guild (bottom).
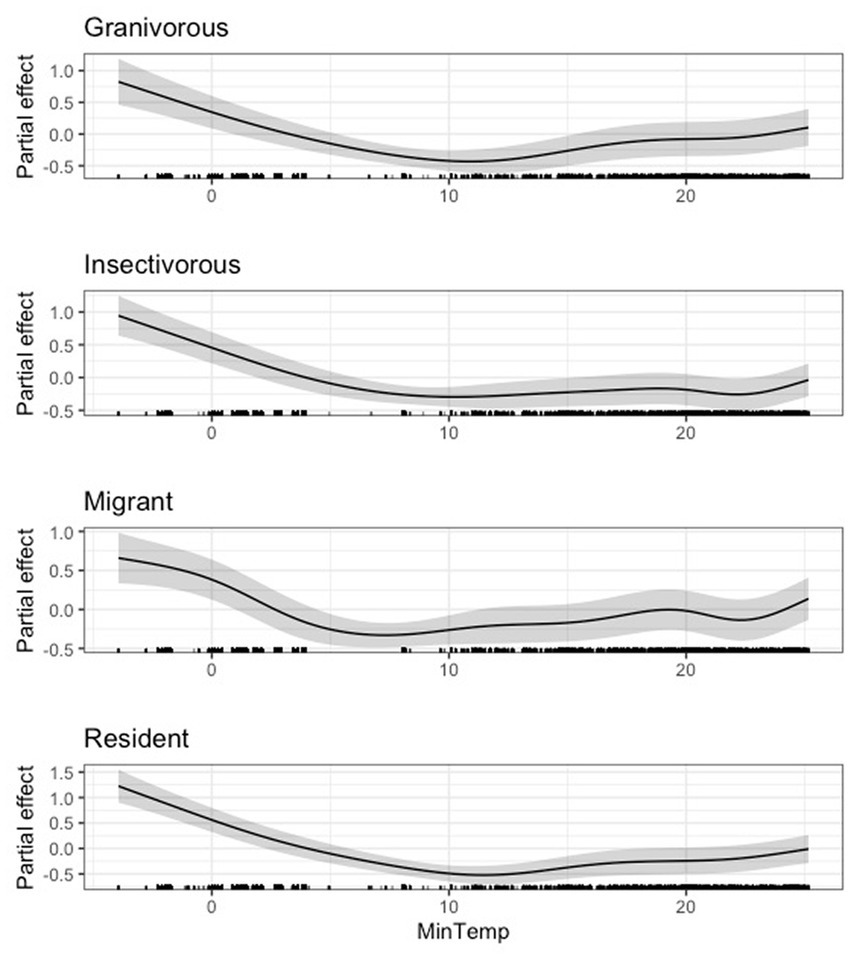
Figure 5. Partial effect of average daily minimum temperature (degrees Celsius) on scaled mass index for the granivorous guild (top), insectivorous guild (second from top), Neotropical migrant guild (third from top), and resident guild (bottom).
Average maximum temperature
For six of the eighteen species (Carolina chickadee, northern cardinal, tufted titmouse, hooded warbler, Carolina wren, and white-eyed vireo), we found that average daily maximum temperature had an inverse relationship with body condition compared to that of daily minimum temperature, such that its effect on scaled mass index was positive until a certain point (between 20 and 30°C), after which the effect either stabilized or became negative (Figure 6). We found a dynamic relationship between average daily maximum temperature and scaled mass index for common yellowthroat, painted bunting, and yellow-breasted chat, with unclear overall directionality (Figure 7). The effect of maximum temperature on scaled mass index was negligible for wood thrush (Supplementary Figure S4), where both the lower 95% CIs (3.07e-37) and 85% CIs (2.29e-28) very closely approached zero (Supplementary Table S3).
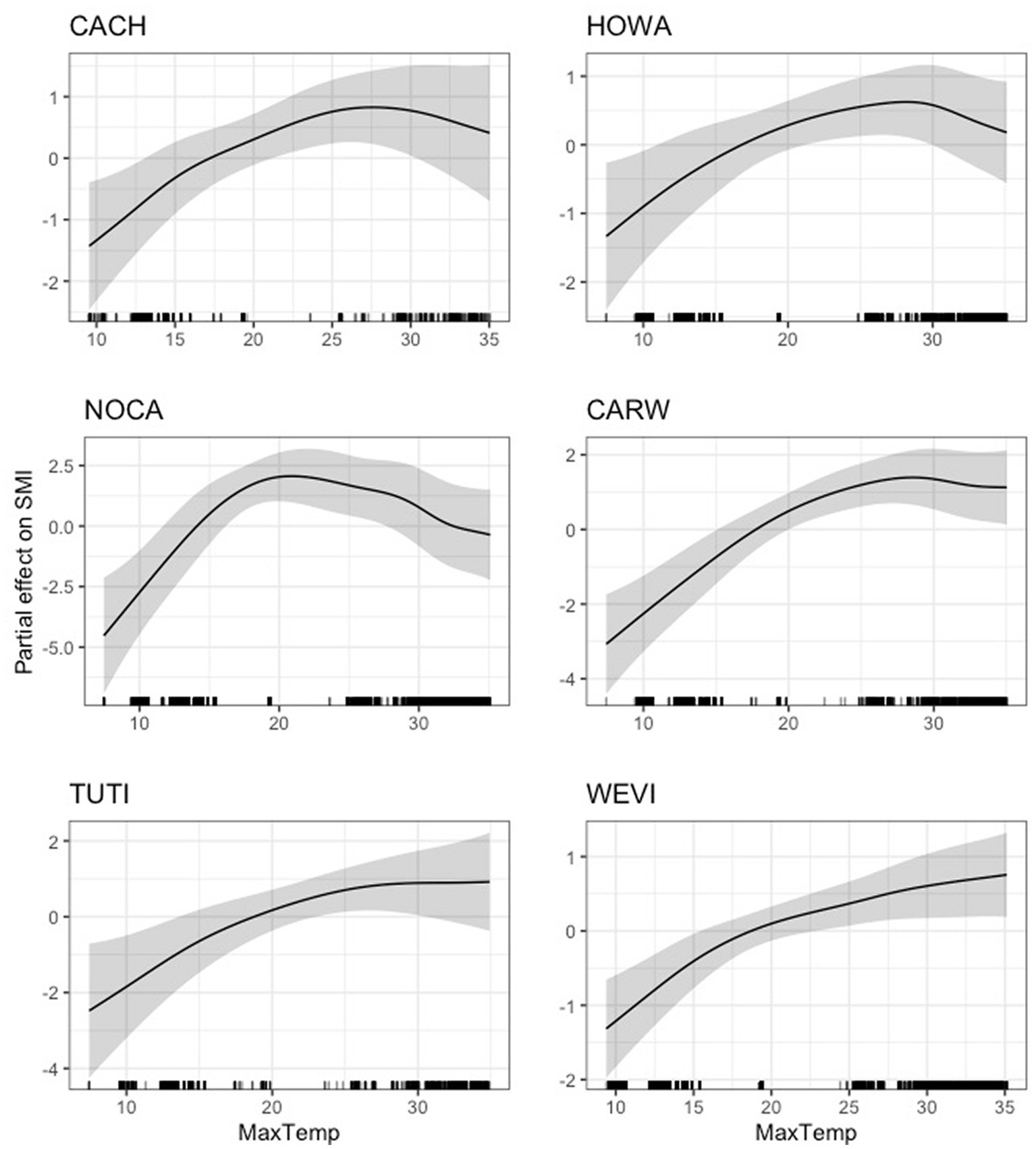
Figure 6. Partial effect of average daily maximum temperature (degrees Celsius) on scaled mass index for six species. Left: Carolina chickadee (CACH, top), northern cardinal (NOCA, center), tufted titmouse (TUTI, bottom); right: hooded warbler (HOWA, top), Carolina wren (CARW, center), white-eyed vireo (WEVI, bottom).
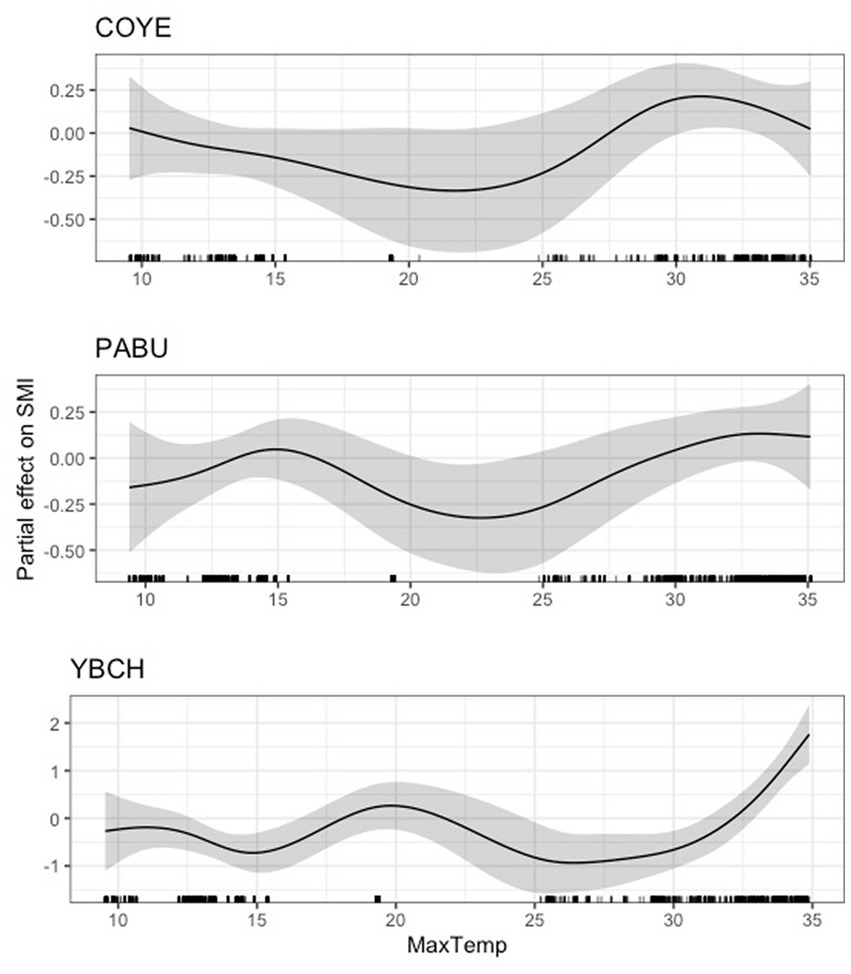
Figure 7. Partial effect of average daily maximum temperature (degrees Celsius) on scaled mass index for common yellowthroat (COYE, top), painted bunting (PABU, center), and yellow-breasted chat (YBCH, bottom).
In terms of guilds, we found a generally positive relationship between average daily maximum temperature and scaled mass index until approximately 20°C, after which the relationship appeared to reach a threshold. This relationship remained remarkably similar across all guilds (Figures 8, 9). For all species and guilds analyzed, the 95 and 85% CIs for maximum temperature did not overlap zero (Supplementary Table S5).
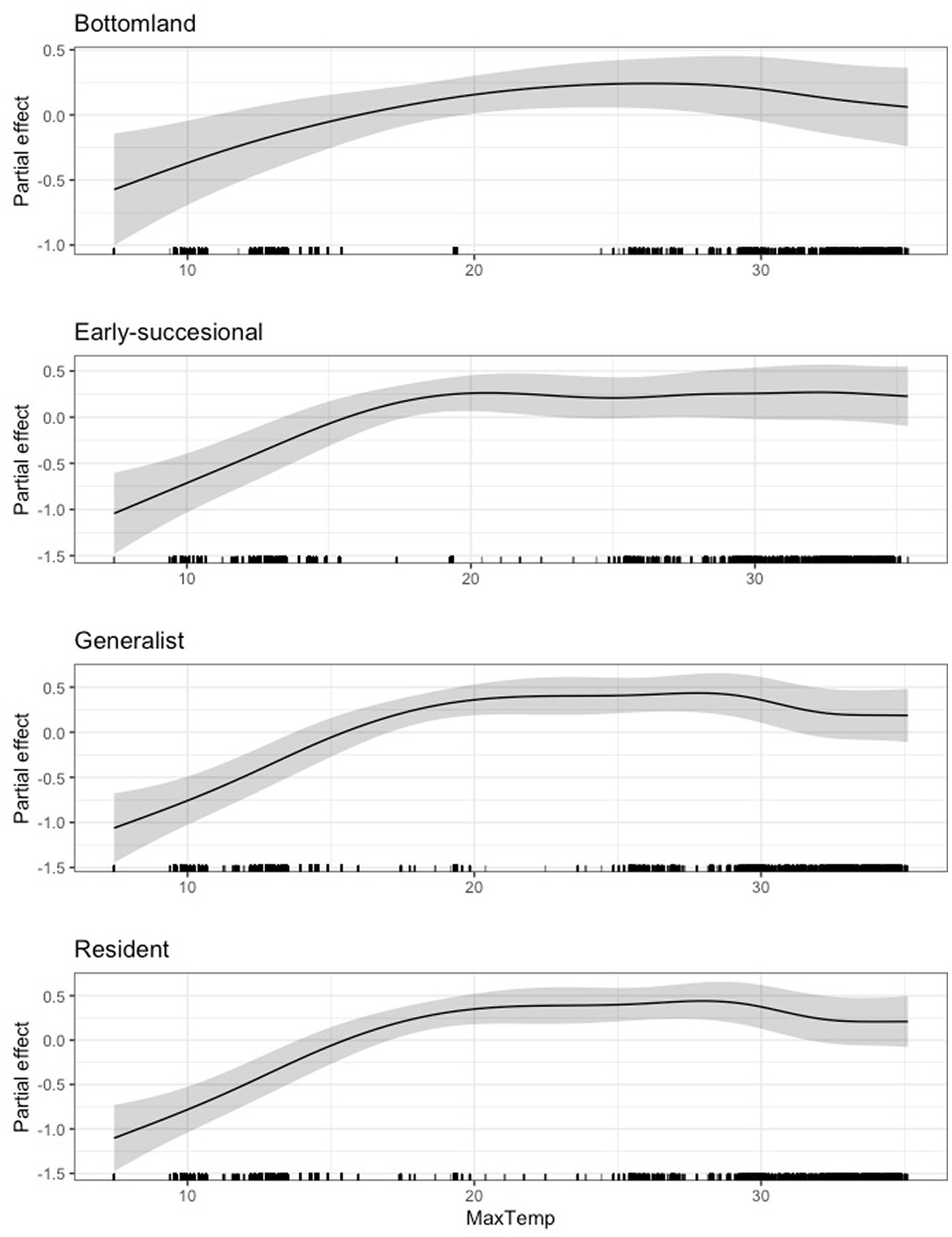
Figure 8. Partial effect of average daily maximum temperature (degrees Celsius) on scaled mass index for the bottomland guild (top), early successional guild (second from top), habitat generalist guild (third from top), and resident guild (bottom).
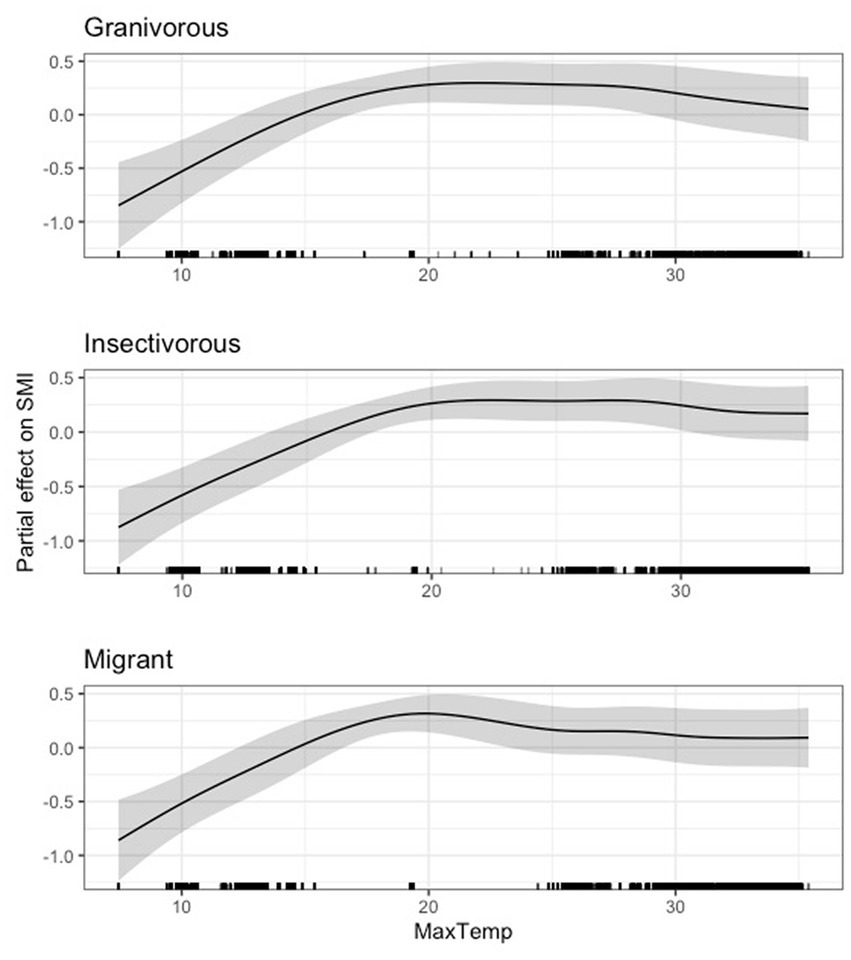
Figure 9. Partial effect of average daily maximum temperature (degrees Celsius) on scaled mass index for the granivorous guild (top), insectivorous guild (center), and Neotropical migrant guild (bottom).
Precipitation
For four species (Carolina wren, indigo bunting, northern cardinal, and hooded warbler), we found that low levels of precipitation were negligibly or negatively related to body condition until a threshold was reached (between 10 and 17 cm), after which precipitation was positively related to body condition (Figure 10). For painted bunting and yellow-breasted chat, we found a highly dynamic, though generally positive, relationship between precipitation and scaled mass index (Figure 10). Neither the 95% nor 85% CIs overlapped zero for Carolina wren, northern cardinal, painted bunting, yellow-breasted chat, or hooded warbler. However, for indigo bunting the lower 95% CI closely approached zero (4.62e-03), suggesting a potentially weak effect of precipitation (Supplementary Table S5). Precipitation was also positively related to scaled mass index for Carolina chickadee, prothonotary warbler, red-eyed vireo, and white-eyed vireo, although these effects were quite weak (Figure 11). The lower 95% CI for Carolina chickadee (2.76e-35) closely approached zero, and for prothonotary warbler, red-eyed and white-eyed vireos both the lower 95% CI (prothonotary warbler: 5.46e-20, red-eyed vireo: 1.94e-06, white-eyed vireo: 1.10e-21) and lower 85% CI (prothonotary warbler: 9.32e-16, red-eyed vireo: 1.58e-05, white-eyed vireo: 4.20e-17) both closely approached zero (Supplementary Table S3). Precipitation was negatively related to scaled mass index for field sparrow (S.5), however this effect was very weak as the lower 95% CI (2.64e-32) and 85% CI (7.51e-25) both closely approached zero (Supplementary Table S5).
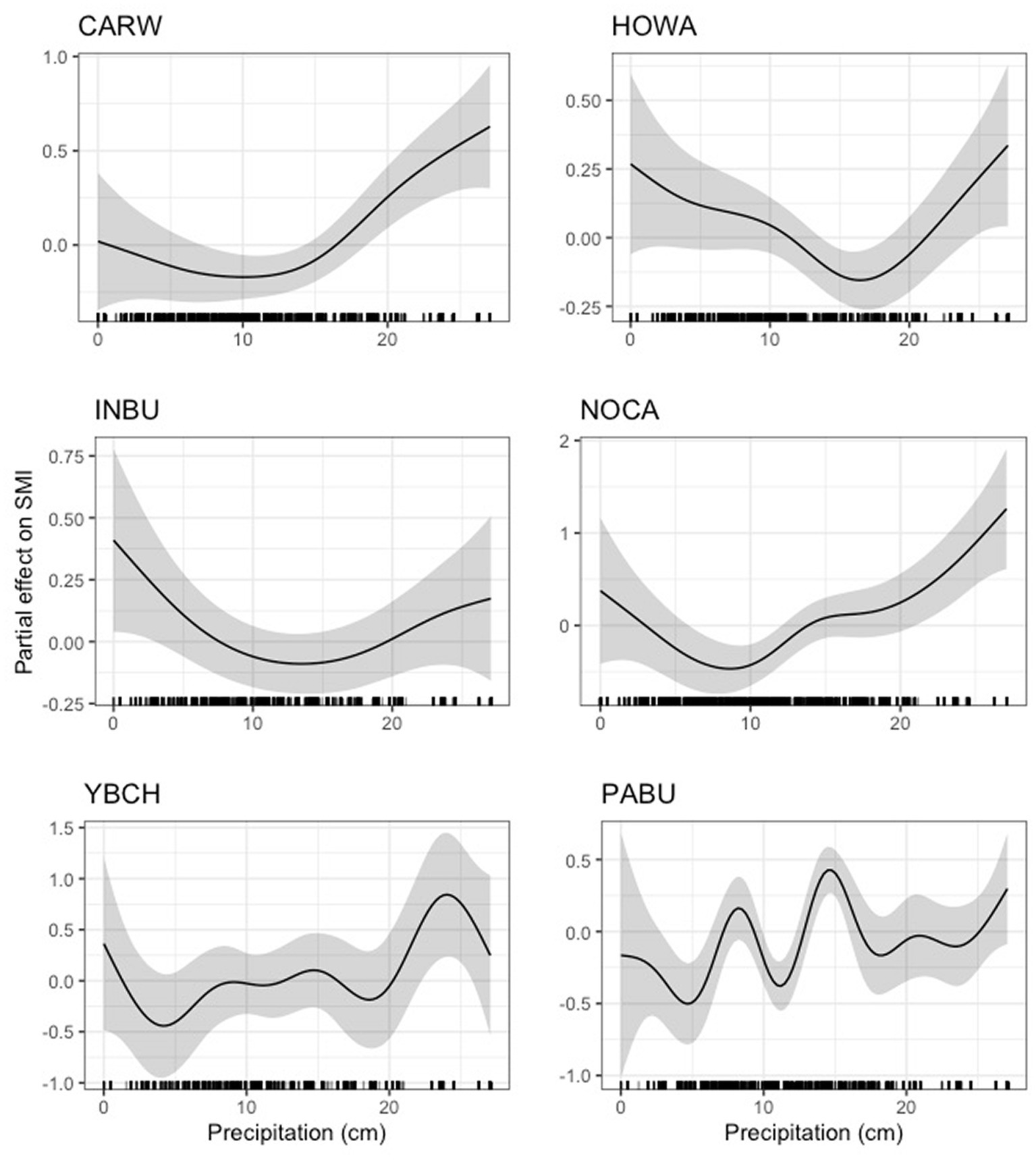
Figure 10. Partial effect of precipitation on scaled mass index for six species. Left: Carolina wren (CARW, top), indigo bunting (INBU, center), yellow-breasted chat (YBCH, bottom); right: hooded warbler (HOWA, top), northern cardinal (NOCA, center), painted bunting (PABU, bottom).
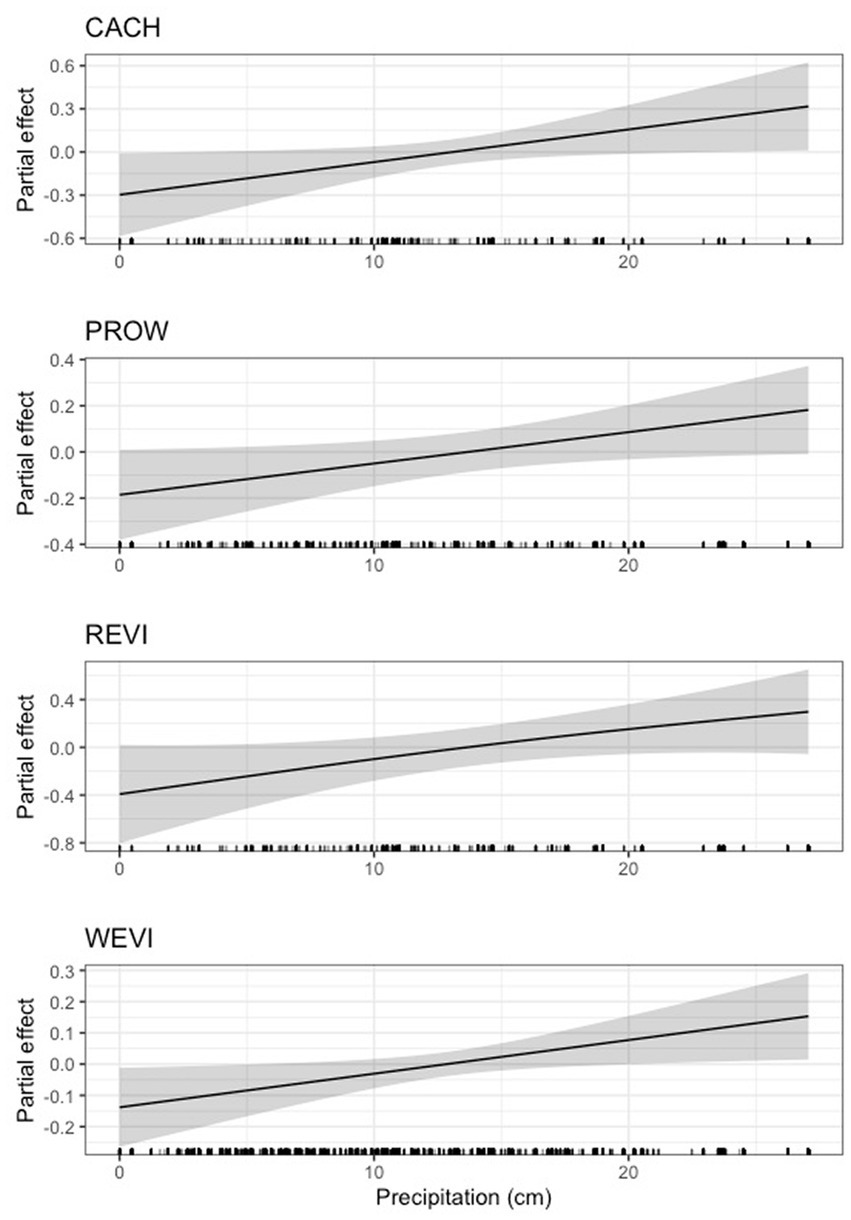
Figure 11. Partial effect of precipitation on scaled mass index for Carolina chickadee (CACH, top), prothonotary warbler (PROW, second from top), red-eyed vireo (REVI, third from top), and white-eyed vireo (WEVI, bottom).
In terms of guilds, we found a generally variable but generally negative effect of precipitation on scaled mass index in the bottomland and granivorous guilds until approximately 17 cm for the bottomland guild and just above 10 cm for the granivorous guild, after which the effect of precipitation was generally positive (Figure 12). Our results for the early successional, insectivorous, and Neotropical migrant guilds largely mirrored those for indigo bunting, Carolina wren, northern cardinal, and hooded warbler in that precipitation was negligibly related to body condition until a certain precipitation threshold was reached (16 - 20cm for these guilds), after which precipitation positively related to body condition (Figure 13). Finally, we noted a strong positive relationship between precipitation and scaled mass index above 10cm for the habitat generalist and resident guilds, while the relationship between precipitation and scaled mass index for the omnivorous guild was weak overall (Figure 14). The 95 and 85% CIs for all guilds analyzed did not overlap zero (Supplementary Table S5).
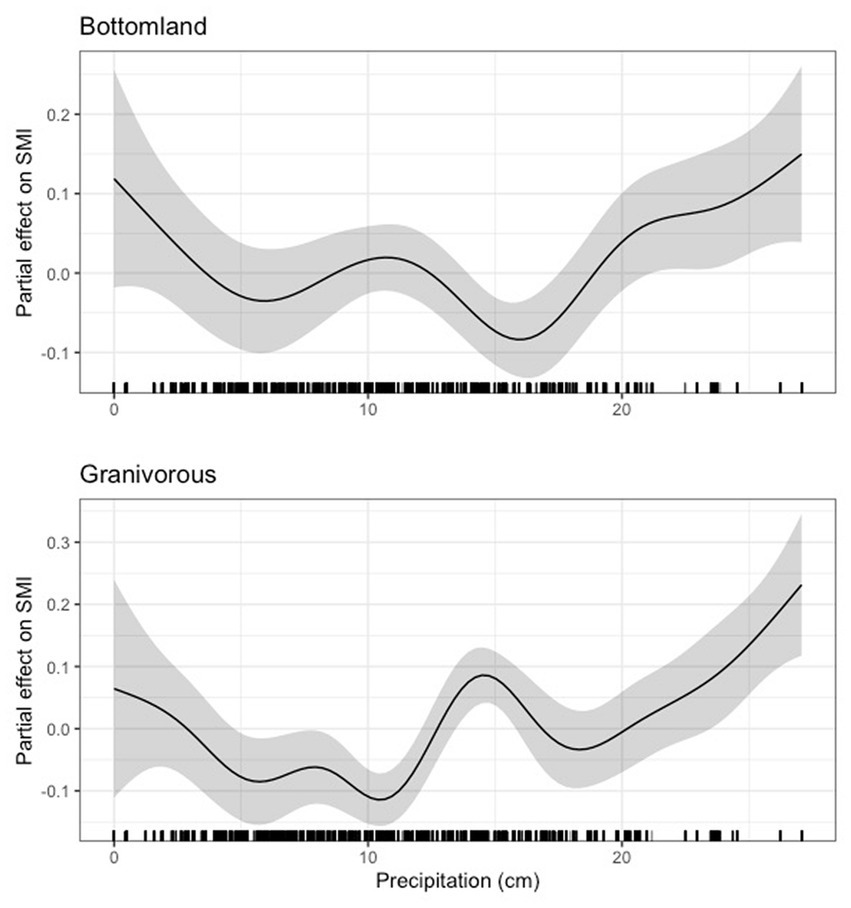
Figure 12. Partial effect of precipitation on scaled mass index for the bottomland guild (top) and the granivorous guild (bottom).
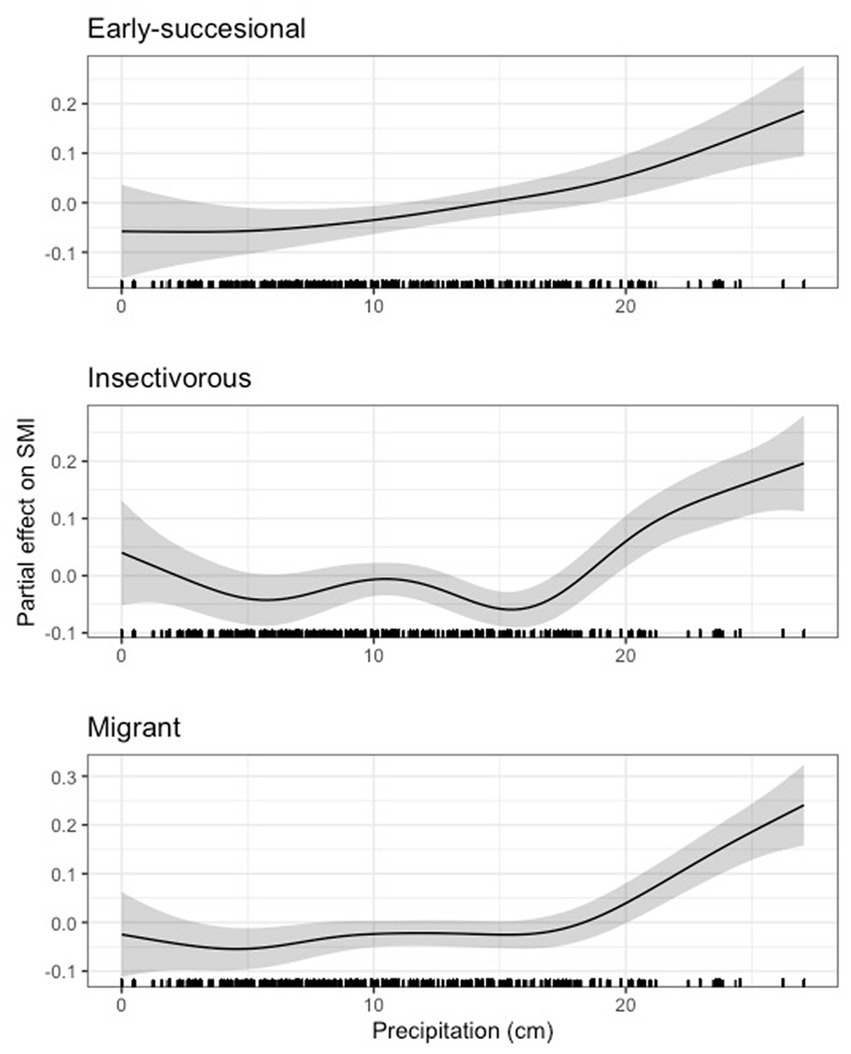
Figure 13. Partial effect of precipitation on scaled mass index for the early successional guild (top), the insectivorous guild (center), and the Neotropical migrant guild (bottom).
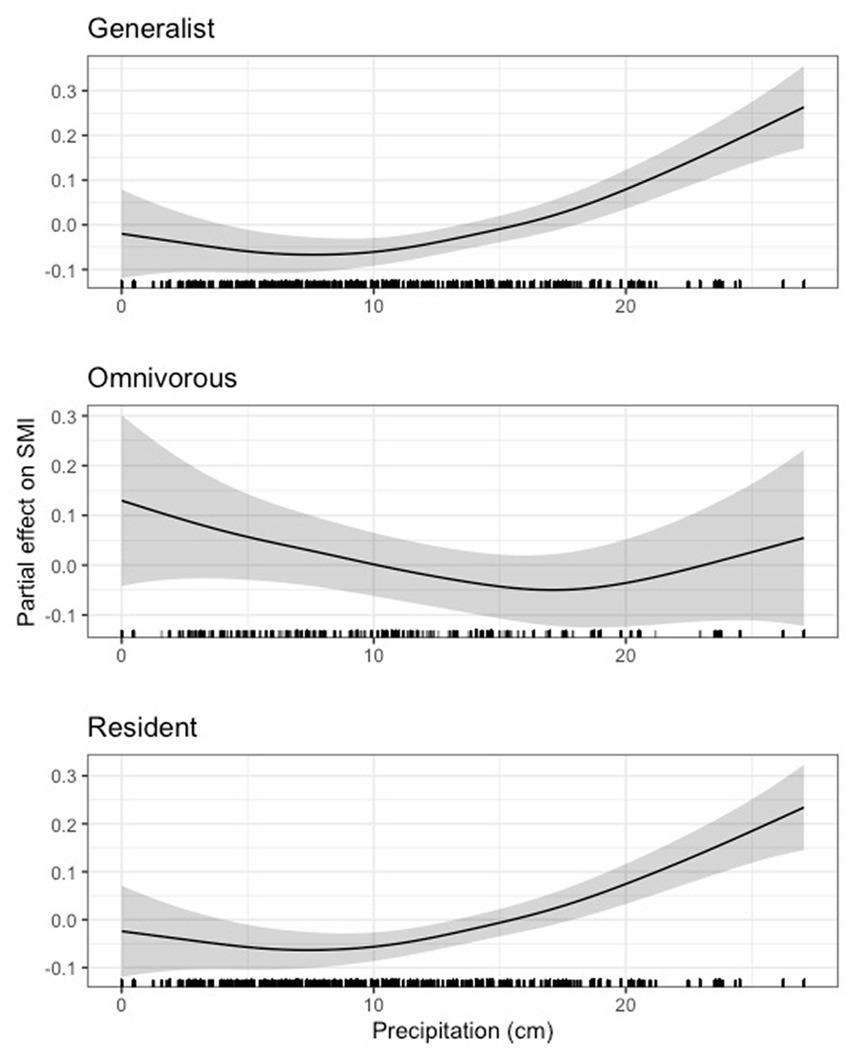
Figure 14. Partial effect of precipitation on scaled mass index for the habitat generalist guild (top), the omnivorous guild (center), and the resident guild (bottom).
Effects of age on scaled mass index
Species
The effect of age was present in the top models for 16 out of the 18 species analyzed (Supplementary Table S3). Juveniles had higher scaled mass indices than adults (i.e., a negative effect of age) for Acadian flycatcher (p < 0.001), hooded warbler (p < 0.01), indigo bunting (p < 0.001), Kentucky warbler (p < 0.001), northern cardinal (p < 0.001), prothonotary warbler (p < 0.01), red-eyed vireo (p < 0.001), Swainson’s warbler (p < 0.001), and white-eyed vireo (p < 0.001; Supplementary Table S6). However, this directionality was not uniform, and scaled mass index was higher in adults than juveniles for Carolina chickadee (p < 0.01), Carolina wren (p < 0.001), common yellowthroat (p < 0.001), field sparrow (p < 0.001), and tufted titmouse (p < 0.01; Supplementary Table S6). Although age was included in the top models for Bewick’s wren and wood thrush, it did not significantly influence scaled mass index for these species (p > 0.05; Supplementary Table S6). The top models for painted bunting and yellow-breasted chat did not include the effect of age (Supplementary Table S3).
Guilds
Age was present in the top models for 7 out of 8 guilds. Scaled mass index was higher in juveniles than adults for the granivorous, bottomland, and early successional guilds (p < 0.001), and the insectivorous guild (p < 0.01; Supplementary Table S6). Conversely, scaled mass index was higher in adults than juveniles for the omnivorous guild (p < 0.001; Supplementary Table S6). Age was in the top models for the resident and habitat generalist guilds, but it did not have a significant effect on scaled mass index for either group (p > 0.05; Supplementary Table S6). The top model for the migrant guild did not include the effect of age.
Discussion
We observed strong heterogeneity in the relationships between scaled mass index and weather parameters across species and guilds. The response of avian body condition to weather has previously been established as dynamic and complex, driven in part by a balance between competing weather variables (Gardner et al., 2018). Our results are consistent with this observation and highlight the role of weather thresholds in relation to avian body condition during the breeding season. For many species and guilds, the body condition response shifted dramatically after reaching a certain threshold of temperature or precipitation. For temperature this threshold tended to occur around 10°C for minimum and 20–30°C for maximum daily temperature, and for precipitation the threshold typically occurred between 10 and 20 cm. As expected for datasets this large and varied, weather parameters typically explained a low amount of overall variance in our top models (r2 < 0.25 for all models). Thus, our results establish that relatively short-term weather parameters are important external drivers of avian body condition, but body condition is complex and likely influenced by many additional variables including seasonality and the availability of local thermal refugia.
Effects of temperature on body condition
We predicted that warmer temperatures would positively impact body condition for insectivorous and migratory species but would have little effect on omnivorous species. Although we did find positive effects of warmer temperatures on body condition for some species, these effects were more dynamic than the predicted linear relationship. Regarding minimum daily temperatures, body condition tended to decrease with increasing minimum temperature until approximately 10°C, after which body condition either increased or became generally unrelated to minimum temperature. At low minimum temperatures, body condition may be influenced by fat storage for thermoregulation and limited activity (Stevenson and Woods, 2006), and thus not follow simple food-availability trends. The scale of the y-axis values for minimum temperature partial effects plots indicates that the observed effects of minimum temperature were generally stronger than those observed for other weather variables. For maximum temperature, we observed a similar threshold effect at 20–30°C, with many species exhibiting a positive relationship between body condition and increasing maximum temperatures until this point, after which the relationship became slightly negative or negligible. At the guild-level, the threshold effect largely mirrored that of individual species with inflection points for minimum temperature occurring around 10°C, and maximum temperature around 20°C. We also found that early-successional species hit their threshold in positive response to maximum temperature sooner than bottomland or generalist species (18 vs. 20°C), and exhibited stronger effects of temperature (indicated by CIs further from zero), which are consistent with our predictions in (H6). High levels of concurvity in top models that included both minimum temperature and maximum temperature indicate large confidence intervals and suggest that detailed interpretations of these results may be limited.
Effects of precipitation on body condition
Like our temperature findings, we observed effects of average daily precipitation on many individual species and some, but not all, guilds. For most of our species and guilds, we observed a threshold effect, where body condition was negatively or negligibly related to body condition until a threshold of precipitation was reached (10–20 cm), after which body condition tended to increase with more precipitation. A smaller proportion of species and guilds exhibited a generally positive relationship between precipitation and body condition, and only one species and guild (i.e., field sparrow and the omnivorous guild, for which the effect was weak) exhibited generally negative relationships between precipitation and body condition. Thus, contrary to our predictions, we found little evidence of high precipitation correlating with a decline in body condition due to flooding for the granivorous (H1) or bottomland guilds (H4). Instead, precipitation tended to have the strongest positive effects after approximately 17 cm. For some species, such as painted bunting, the effects of precipitation appeared to change quickly within relatively small windows of precipitation totals. This variation in response to precipitation seems biologically improbable, and may be due to confounding, unaccounted for factors (e.g., microhabitat selection) or interactions between weather variables which were not considered in this study. Therefore, interpretation of these results is limited.
Age effects
We found that for most species analyzed (14 out of 18), age was an important driver of scaled mass index. Age-dependent responses to weather variables have been demonstrated previously via numerous pathways including differential migratory phenology (Jarjour et al., 2017), phenological (Bonamour et al., 2020) and phenotypic plasticity (Ward et al., 2021), and existing studies on body condition (Gardner et al., 2016, 2018; McLean et al., 2018). A wide body of existing literature also suggests that body condition fluctuates as birds age (Angelier et al., 2011; Rockwell et al., 2012; Welcker et al., 2015), due in part to the fact that adult birds may outcompete juvenile birds for more limited resources in times of extreme weather (Rockwell et al., 2012). For most species (i.e., nine of the 14 species) juvenile birds exhibited higher body condition than adults. This result may have been influenced by both age-specific adaptation and species-specific factors such as fledging date, clutch size, or quality of parental care, for which we were unable to control. Additionally, this finding could reflect a different mass-wing scaling component in younger birds compared to adults. Results from our guild-level analysis largely mirrored this, with the directionality of age effects remaining inconsistent across guilds, although adults had higher body condition for all three dietary guilds.
Implications for fitness
Our findings demonstrate the importance of short-term weather patterns to individual avian body condition, which can have broad ramifications across entire populations, including altered levels of individual fitness. Relationships between avian body condition, external variables such as habitat and climate, and fitness are not well established and frequently inconclusive at best (Kleist et al., 2017; Kouba et al., 2021) or suggest climate changes affect population growth rates predominantly independently of simultaneous body condition declines (McLean et al., 2020). However, body condition has been found to influence individual fitness in a large number of species, including a wide variety of birds (Stevenson and Woods, 2006) and other vertebrate taxa such as marine iguanas (Romero et al., 2001) and bison (Vervaecke et al., 2005). This is a result, in part, of reduced body condition negatively affecting the foraging success of an individual (Jakob et al., 1996) along with increasing susceptibility to disease and predators (Kouba et al., 2021). This uncertainty in the literature may reflect complexity in the interpretation of body condition, which can be influenced by fat storage for thermoregulation as well as energetic storage (Stevenson and Woods, 2006). Thus, high body condition may not always be adaptive, for example under high heat conditions where lower body mass results in more rapid cooling (McLean et al., 2020).
The results of our study indicate that a further increase in body condition past certain temperature and precipitation thresholds (i.e., average daily maximum temperature exceeding 20°C) may no longer be beneficial. Future research may help further elucidate if long-term changes to temperature and precipitation have negative effects on body condition, fitness, and population dynamics. In some cases, subsequent consequences such as reduced nestling survival may become apparent if individuals must spend more time foraging. Future studies could explore the relationship between fitness, body condition, and weather further by pairing adult body condition data with nesting success and annual survival, or by correlating current year population estimates with prior year body condition data. We also anticipate that these effects will differ across scale, species, and geographies, consistent with data from this study.
Conclusion
Overall, our results demonstrate that maximum temperature, minimum temperature, and precipitation frequently relate to avian body condition in a non-linear fashion and that threshold effects of weather are important for many species and guilds. We anticipate that threshold effects, such as those found for several species in this study (e.g., northern cardinal and indigo bunting), will become more relevant in the future as climate change increases extreme weather (Bregman et al., 2016) and pushes species closer to, or further from, these thresholds. Threshold effects are often witnessed at the scale of ecosystems (Burkett et al., 2005) and interactions between ecosystem, community, and species-specific thresholds may be expected and should be investigated in the future. Relationships between weather and body condition were also remarkably similar between most dietary, migratory, or habitat guilds. These trends often did not mirror those for individual species within guilds due to strong species-specific heterogeneity that was masked in a guild-level analysis. Thus, responses of birds to weather may be difficult to predict across species, at least regarding body condition. For migratory species, this may be partially due to spillover effects from conditions on the wintering grounds (Rockwell et al., 2012; Akresh et al., 2019a). For example, migratory species may arrive on their breeding grounds in variable energetic states because of conditions on their wintering grounds or migratory route, that then influence body condition during the breeding season. Finally, our results also suggest that temperature may have stronger effects than precipitation on avian body condition, which is consistent with existing literature indicating stronger effects of temperature than precipitation on avian populations (Pearce-Higgins et al., 2015).
Future directions
The strong species-specific heterogeneity that we observed in this study suggests that caution should be used when evaluating the effects of short-term weather on birds at the guild level, and that species-level approaches may be more informative albeit less consistent. This would be particularly important when dealing with imperiled or engendered species, or species of conservation concern, for which more exact knowledge of their responses are warranted. Dietary and habitat preferences of some species may change across seasons (Billerman et al., 2022), thus future studies should explore alternative guild assignments that may capture more nuance in species response. Future studies at different spatial or temporal scales may also further elucidate the drivers behind species-specific heterogeneity, and scales at which guild-level analyses are appropriate.
We foresee additional future research opportunities investigating the consequences of our observed relationships between weather dynamics and body condition for individual fitness (i.e., reproductive success and survival), and the implications of these relationships for short-term population dynamics and long-term evolutionary processes. Developing a better understanding of how birds respond to short-term weather at the level of the individual complements existing research at the level of the population/community and is an urgent area of research as climate change continues to shift short-term weather patterns (Jenouvrier, 2013; Langham et al., 2015).
Data availability statement
Publicly available datasets were analyzed in this study. This data can be found at: https://datadryad.org/stash/share/sYVnEPMQP-mTS5_-xBBDJDZDT3ocJKik8h53G3HuULw.
Ethics statement
Ethical review and approval was not required because this research was conducted through an existing dataset. No new field work or handling of individual organisms was conducted for this study.
Author contributions
MM spearheaded the study design, data collection of this research, designed figures, and tables. JG and MM both contributed to the analysis of data and contributed equally to the editing process. JG contributed significantly to the discussion section and interpretation of results. All authors contributed to the article and approved the submitted version.
Funding
Funding for this research was made possible by MM’s graduate research fellowship through the Rob and Bessie Welder Wildlife Foundation.
Acknowledgments
The authors would like to thank the Rob and Bessie Welder Wildlife Foundation for fellowship support to MM. This manuscript is Rob and Bessie Welder Wildlife Foundation Contribution number 736. The authors would also like to thank Selma Glasscock along with members of the Grace Lab for feedback on this manuscript, and the two reviewers for providing extensive, constructive comments and suggestions. Finally, the authors would like to thank the Institute for Bird Populations for providing access to the Monitoring Avian Productivity and Survivorship dataset.
Conflict of interest
The authors declare that the research was conducted in the absence of any commercial or financial relationships that could be construed as a potential conflict of interest.
Publisher’s note
All claims expressed in this article are solely those of the authors and do not necessarily represent those of their affiliated organizations, or those of the publisher, the editors and the reviewers. Any product that may be evaluated in this article, or claim that may be made by its manufacturer, is not guaranteed or endorsed by the publisher.
Supplementary material
The Supplementary material for this article can be found online at: https://www.frontiersin.org/articles/10.3389/fevo.2023.1154656/full#supplementary-material
References
Akresh, M. E., King, D. I., and Marra, P. P. (2019a). Examining carry-over effects of winter habitat on breeding phenology and reproductive success in prairie warblers Setophaga discolor. J. Avian Biol. 50, 1–13. doi: 10.1111/jav.02025
Akresh, M. E., King, D. I., and Marra, P. P. (2019b). Rainfall and habitat interact to affect the condition of a wintering migratory songbird in the Bahamas. Ecol. Evol. 9, 8042–8061. doi: 10.1002/ece3.5359
Akresh, M. E., King, D. I., and Marra, P. P. (2021). Hatching date influences winter habitat occupancy: examining seasonal interactions across the full annual cycle in a migratory songbird. Ecol. Evol. 11, 9241–9253. doi: 10.1002/ece3.7500
Angelier, F., Tonra, C. M., Holberton, R. L., and Marra, P. P. (2011). Short-term changes in body condition in relation to habitat and rainfall abundance in American redstarts Setophaga ruticilla during the non-breeding season. J. Avian Biol. 42, 335–341. doi: 10.1111/j.1600-048X.2011.05369.x
Anthony, A., Atwood, J., August, P., Byron, C., Cobb, S., Foster, C., et al. (2009). Coastal lagoons and climate change: ecological and social ramifications in U. S. Atlantic and Gulf Coast ecosystems. Ecol. Soc. 14:8.
Beja, P., Santos, C. D., Santana, J., Pereira, M. J., Marques, J. T., Queiroz, H. L., et al. (2010). Seasonal patterns of spatial variation in understory bird assemblages across a mosaic of flooded and unflooded Amazonian forests. Biodivers. Conserv. 19, 129–152. doi: 10.1007/s10531-009-9711-6
Billerman, S. M., Keeney, B. K., Rodewald, P. G., and Schulenberg, T. S. (2022). Birds of the World. Cornell Laboratory of Ornithology, Ithaca, NY, USA. Available at:https://birdsoftheworld.org/bow/home
Binley, A. D., Proctor, C. A., Pither, R., Davis, S. A., and Bennett, J. R. (2021). The unrealized potential of community science to support research on the resilience of protected areas. Conserv Sci Pract 3:e376. doi: 10.1111/csp2.376
Bonamour, S., Chevin, L. M., Réale, D., Teplitsky, C., and Charmantier, A. (2020). Age-dependent phenological plasticity in a wild bird. J. Anim. Ecol. 89, 2733–2741. doi: 10.1111/1365-2656.13337
Bregman, T. P., Lees, A. C., MacGregor, H. E. A., Darski, B., de Moura, N. G., Aleixo, A., et al. (2016). Using avian functional traits to assess the impact of land-cover change on ecosystem processes linked to resilience in tropical forests. Proc. R. Soc. B Biol. Sci. 283:1289. doi: 10.1098/rspb.2016.1289
Burkett, V. R., Wilcox, D. A., Stottlemyer, R., Barrow, W., Fagre, D., Baron, J., et al. (2005). Nonlinear dynamics in ecosystem response to climatic change: case studies and policy implications. Ecol. Complex. 2, 357–394. doi: 10.1016/j.ecocom.2005.04.010
Crick, H. Q. P. (2004). Impact of climate change on birds. Ibis 146, 48–56. doi: 10.1111/j.1474-919X.2004.00327.x
Danner, R. M., Greenberg, R. S., Danner, J. E., Kirkpatrick, L. T., and Walters, J. R. (2013). Experimental support for food limitation of a short-distance migratory bird wintering in the temperate zone. Ecology 94, 2803–2816. doi: 10.1890/13-0337.1
Delgado-Rodríguez, M., and Llorca, J. (2004). Bias. J Epidemiol Community Health 58, 635–641. doi: 10.1136/jech.2003.008466
DeSante, D. F., Burton, K. M., Velez, P., Froehlich, D., Kaschube, D., and Albert, S. (2015). MAPS manual, 2015 protocol. Point Reyes Station, CA: The Institute for Bird Populations. 1–85.
English, M. D., Robertson, G. J., Peck, L. E., Pirie-Hay, D., Roul, S., and Mallory, M. L. (2018). Body condition of American black ducks (Anas rubripes) wintering in Atlantic Canada using carcass composition and a scaled mass index. Can. J. Zool. 96, 1137–1144. doi: 10.1139/cjz-2017-0329
Fischer, J., Lindenmayer, D. B., Blomberg, S. P., Montague-Drake, R., Felton, A., and Stein, J. A. (2007). Functional richness and relative resilience of bird communities in regions with different land use intensities. Ecosystems 10, 964–974. doi: 10.1007/s10021-007-9071-6
Freeman, S., and Jackson, W. M. (1990). Univariate metrics are not adequate to measure avian body size. Auk 107, 69–74.
Gardner, J. L., Amano, T., Sutherland, W. J., and Clayton, M. (2016). Individual and demographic consequences of reduced body condition following repeated exposure to high temperatures. Ecology 97, 786–795. doi: 10.1890/15-0642.1
Gardner, J. L., Peters, A., Kearney, M. R., Joseph, L., and Heinsohn, R. (2011). Declining body size: a third universal response to warming? Trends Ecol Evol 26, 285–291. doi: 10.1016/j.tree.2011.03.005
Gardner, J. L., Rowley, E., de Rebeira, P., de Rebeira, A., and Brouwer, L. (2018). Associations between changing climate and body condition over decades in two southern hemisphere passerine birds. Clim Chang Responses 5, 1–14. doi: 10.1186/s40665-018-0038-y
Grace, J. K., Froud, L., and Meillère, F. A. (2017). House sparrows mitigate growth effects of post-natal glucocorticoid exposure at the expense of longevity. Gen. Comp. Endocrinol. 253, 1–12. doi: 10.1016/j.ygcen.2017.08.011
Granthon, C., and Williams, D. A. (2017). Avian malaria, body condition, and blood parameters in four species of songbirds. Wilson J Ornithol 129, 492–508. doi: 10.1676/16-060.1
Gray, M. A., Baldauf, S. L., Mayhew, P. J., and Hill, J. K. (2007). The response of avian feeding guilds to tropical forest disturbance. Conserv. Biol. 21, 133–141. doi: 10.1111/j.1523-1739.2006.00557.x
Hobson, K. A., van Wilgenburg, S., Wassenaar, L. I., Moore, F., and Farrington, J. (2007). Estimating origins of three species of neotropical migrant songbirds at a Gulf Coast stopover site: combining stable isotope and GIS tools. Condor 109, 256–267. doi: 10.1093/condor/109.2.256
IPCC (2021). “Summary for Policymakers” in Climate Change 2021: The Physical Science Basis. Contribution of Working Group I to the Sixth Assessment Report of the Intergovernmental Panel on Climate Change. eds. V. Masson-Delmotte, P. Zhai, A. Pirani, S. L. Connors, C. Péan, and S. Berger, et al. Available at: https://www.ipcc.ch/report/ar6/wg1/downloads/report/IPCC_AR6_WGI_SPM.pdf
Jakob, E. M., Marshall, S. D., and Uetz, G. W. (1996). Estimating fitness: a comparison of body condition indices. Oikos 77, 61–67. doi: 10.2307/3545585
Jarjour, C., Frei, B., and Elliott, K. H. (2017). Associations between sex, age and species-specific climate sensitivity in migration. Animal Migration 4, 23–36. doi: 10.1515/ami-2017-0004
Jenouvrier, S. (2013). Impacts of climate change on avian populations. Glob. Chang. Biol. 19, 2036–2057. doi: 10.1111/gcb.12195
Jetz, W., Wilcove, D. S., and Dobson, A. P. (2007). Projected impacts of climate and land-use change on the global diversity of birds. PLoS Biol. 5, e157–e1219. doi: 10.1371/journal.pbio.0050157
Johnson, J. B., and Omland, K. S. (2004). Model selection in ecology and evolution. Trends Ecol Evol 19, 101–108. doi: 10.1016/j.tree.2003.10.013
Kleist, N., Guralnick, R. P., Cruz, A., Lowry, C. A., and Francis, C. D. (2017). Chronic anthropogenic noise disrupts glucocorticoid signaling and has multiple effects on fitness in an avian community. Proc Natl Acad Sci U S A 115, e648–e657. doi: 10.5061/dryad.bt45d
Kouba, M., Bartoš, L., Bartošová, J., Hongisto, K., and Korpimäki, E. (2021). Long-term trends in the body condition in parents and offspring of Tengmalm’s owls under fluctuating food conditions and climate change. Sci. Rep. 11:18893. doi: 10.1038/s41598-021-98447-1
Labocha, M. K., Schutz, H., and Hayes, J. P. (2014). Which body condition index is best? Oikos 123, 111–119. doi: 10.1111/j.1600-0706.2013.00755.x
Langham, G. M., Schuetz, J. G., Distler, T., Soykan, C. U., and Wilsey, C. (2015). Conservation status of north American birds in the face of future climate change. PLoS One 10, 1–16. doi: 10.1371/journal.pone.0135350
Larsen, F. W., Bladt, J., Balmford, A., and Rahbek, C. (2012). Birds as biodiversity surrogates: will supplementing birds with other taxa improve effectiveness? J. Appl. Ecol. 49, 349–356. doi: 10.1111/j.1365-2664.2011.02094.x
Lindenmayer, D. B., Lane, P., Crane, M., Florance, D., Foster, C. N., Ikin, K., et al. (2019). Weather effects on birds of different size are mediated by long-term climate and vegetation type in endangered temperate woodlands. Glob. Chang. Biol. 25, 675–685. doi: 10.1111/gcb.14524
Ma, C., Ma, G., and Pincebourde, S. (2021). Survive a warming climate: insect responses to extreme high temperatures. Annu. Rev. Entomol. 66, 163–184. doi: 10.1146/annurev-ento-041520-074454
Martin, R. O., Cunningham, S. J., and Hockey, P. A. R. (2015). Elevated temperatures drive patterns of fine-scale habitat use in a savanna bird community. J Afr. Ornithol. 86, 127–135. doi: 10.2989/00306525.2015.1029031
Maxwell, S. L., Butt, N., Maron, M., McAlpine, C. A., Chapman, S., Ullmann, A., et al. (2019). “Conservation implications of ecological responses to extreme weather and climate events” in Diversity and Distributions, vol. 25 (Hoboken, NJ: Blackwell Publishing Ltd.), 613–625. doi: 10.1111/ddi.12878
McCloy, M. W. D., Andringa, R. K., and Grace, J. K. (2022). Resilience of Avian Communities to Urbanization and Climate Change: an Integrative Review. Front. Conserv. Sci. 3:918873. doi: 10.3389/fcosc.2022.918873
McLean, N., Lawson, C. R., Leech, D. I., and van de Pol, M. (2016). Predicting when climate-driven phenotypic change affects population dynamics. Ecol. Lett. 19, 595–608. doi: 10.1111/ele.12599
McLean, N., van der Jeugd, H. P., and van de Pol, M. (2018). High intra-specific variation in avian body condition responses to climate limits generalisation across species. PLoS One 13, e0192401–e0192425. doi: 10.1371/journal.pone.0192401
McLean, N., van der Jeugd, H. P., van Turnhout, C. A. M., Lefcheck, J. S., and van de Pol, M. (2020). Reduced avian body condition due to global warming has little reproductive or population consequences. Oikos 129, 714–730. doi: 10.1111/oik.06802
Møller, A. P. (2019). Parallel declines in abundance of insects and insectivorous birds in Denmark over 22 years. Ecol. Evol. 9, 6581–6587. doi: 10.1002/ece3.5236
Moore, F. R., Kerlinger, P., and Simons, T. R. (1990). Stopover on a Gulf Coast Barrier Island by spring trans-gulf migrants. Wilson Bull 102, 487–500.
Moritz, C., and Agudo, R. (2013). The future of species under climate change: resilience or decline? Science 341, 504–508. doi: 10.1126/science.1237190
Myers, N., Mittermeler, R. A., Mittermeler, C. G., da Fonseca, G. A. B., and Kent, J. (2000). Biodiversity hotspots for conservation priorities. Nature 403, 853–858. doi: 10.1038/35002501
Neate-Clegg, M. H. C., Horns, J. J., Adler, F. R., Kemahlı Aytekin, M. Ç., and Şekercioğlu, Ç. H. (2020). Monitoring the world’s bird populations with community science data. Biol. Conserv. 248:108653. doi: 10.1016/j.biocon.2020.108653
Oram, N. J., Sun, Y., Abalos, D., van Groenigen, J. W., Hartley, S., and De Deyn, G. B. (2021). Plant traits of grass and legume species for flood resilience and N2O mitigation. Funct. Ecol. 35, 2205–2218. doi: 10.1111/1365-2435.13873
Parmesan, C., Root, T. L., and Willig, M. R. (2000). Impacts of extreme weather and climate on terrestrial biota. Bull. Am. Meteorol. Soc. 81, 443–450. doi: 10.1175/1520-0477(2000)081<0443:IOEWAC>2.3.CO;2
Pearce-Higgins, J. W., Eglington, S. M., Martay, B., and Chamberlain, D. E. (2015). Drivers of climate change impacts on bird communities. J. Anim. Ecol. 84, 943–954. doi: 10.1111/1365-2656.12364
Peig, J., and Green, A. J. (2009). New perspectives for estimating body condition from mass/length data: the scaled mass index as an alternative method. Oikos 118, 1883–1891. doi: 10.1111/j.1600-0706.2009.17643.x
Peig, J., and Green, A. J. (2010). The paradigm of body condition: a critical reappraisal of current methods based on mass and length. Funct. Ecol. 24, 1323–1332. doi: 10.1111/j.1365-2435.2010.01751.x
Pyle, P. (1997). Identification Guide to North American Birds: Part 1. Bolinas, CA: Slate Creek Press.
R Core Team. (2021). R: A language and environment for statistical computing. Vienna, Austria: R Foundation for Statistical Computing. Available at: https://www.R-project.org/
Rebaudo, F., Faye, E., and Dangles, O. (2016). Microclimate data improve predictions of insect abundance models based on calibrated spatiotemporal temperatures. Front. Physiol. 7:139. doi: 10.3389/fphys.2016.00139
Reece, J. S., Watson, A., Dalyander, P. S., Edwards, C. K., Geselbracht, L., LaPeyre, M. K., et al. (2018). A multiscale natural community and species-level vulnerability assessment of the Gulf coast, USA. PLoS One 13:e0199844. doi: 10.1371/journal.pone.0199844
Reed, T. E., Grotan, V., Jenouvrier, S., and Saether, B. E. (2013). Population growth in a wild bird is buffered against Phenological mismatch. Science 340, 488–491. doi: 10.1126/science.1232870
Reif, A. (2013). Long-term trends in bird populations: A review of patterns and potential drivers in North America and Europe. Acta Ornithol 48, 1–16. doi: 10.3161/000164513X669955
Rockwell, S. M., Bocetti, C. I., and Marra, P. P. (2012). Carry-over effects of winter climate on spring arrival date and reproductive success in an endangered migratory bird, Kirtland’s warbler (Setophaga Kirtlandii). Auk 129, 744–752. doi: 10.1525/auk.2012.12003
Romero, L. M., Reed, J. M., and Wingfield, J. C. (2000). Effects of weather on corticosterone responses in wild free-living passerine birds. Gen. Comp. Endocrinol. 118, 113–122. doi: 10.1006/gcen.1999.7446
Romero, L. M., Wikelski, M., and Levin, S. A. (2001). Corticosterone levels predict survival probabilities of Galá pagos marine iguanas during El Niño events. PNAS 98, 7366–7370. doi: 10.1073/pnas.131091498
Rosenberg, K. V., Dokter, A. M., Blancher, P. J., Sauer, J. R., Smith, A. C., Smith, P. A., et al. (2019). Decline of the north American avifauna. Science 366, 120–124. doi: 10.1126/science.aaw1313
Salewski, V., Hochachka, W. M., and Fiedler, W. (2013). Multiple weather factors affect apparent survival of European passerine birds. PLoS One 8:e59110. doi: 10.1371/journal.pone.0059110
Savonis, M. J., Burkett, V. R., and Potter, J. R. (2008). Impacts of Climate Change and Variability on Transportation Systems and Infrastructure: Gulf Coast Study, Phase I (Issue March) report by the United States Climate Science Program.
Scheffers, B. R., Edwards, D. P., Diesmos, A., Williams, S. E., and Evans, T. A. (2013). Microhabitats reduce animal’s exposure to climate extremes. Glob. Chang. Biol. 20, 495–503. doi: 10.1111/gcb.12439
Scridel, D., Matteo, A., Ahnig, S. J., Caprio, E., Bogliani, G., Pedrini, P., et al. (2018). A review and meta-analysis of the effects of climate change on Holarctic mountain and upland bird populations. Ibis 160, 489–515. doi: 10.1111/ibi.12585
Sekercioglu, C. H. (2006). Increasing awareness of avian ecological function. Trends Ecol. Evol. 21, 464–471. doi: 10.1016/J.TREE.2006.05.007
Skagen, S. K., and Adams, A. A. Y. (2012). Weather effects on avian breeding performance and implications of climate change. Ecol. Appl. 22, 1131–1145. doi: 10.1890/11-0291.1
Stevenson, R. D., and Woods, W. A. (2006). Condition indices for conservation: new uses for evolving tools. Integr. Comp. Biol. 46, 1169–1190. doi: 10.1093/icb/icl052
Thornton, P. E., Shrestha, R., Thornton, M., Kao, S.-C., Wei, Y., and Wilson, B. E. (2021). Gridded daily weather data for North America with comprehensive uncertainty quantification. Scientific Data 8. doi: 10.1038/s41597-021-00973-0
Tonra, C. M., Marra, P. P., and Holberton, R. L. (2011). Migration phenology and winter habitat quality are related to circulating androgen in a long-distance migratory bird. J. Avi. Bio. 42, 397–404. doi: 10.1111/j.1600-048X.2011.05333.x
van Buskirk, J., Mulvihill, R. S., and Leberman, R. C. (2010). Declining body sizes in north American birds associated with climate change. Oikos 119, 1047–1055. doi: 10.1111/j.1600-0706.2009.18349.x
van de Pol, M., Bailey, L. D., McLean, N., Rijsdijk, L., Lawson, C. R., and Brouwer, L. (2016). Identifying the best climatic predictors in ecology and evolution. Methods Ecol. Evol. 7, 1246–1257. doi: 10.1111/2041-210X.12590
Vervaecke, H., Roden, C., and de Vries, H. (2005). Dominance, fatness and fitness in female American bison, Bison bison. Animal Behav. 70, 763–770. doi: 10.1016/j.anbehav.2004.12.018
Viana, D. S., and Chase, J. M. (2022). Ecological traits underlying interspecific variation in climate matching of birds. Glob. Ecol. Biogeogr. 31, 1021–1034. doi: 10.1111/geb.13480
Walsberg, G. E. (1993). Thermal consequences of microhabitat selection in a small bird. Ornis Scand. 24, 174–182. doi: 10.2307/3676733
Walsberg, G. E. (2003). How useful is energy balance as a overall index of stress in animals? Horm. Behav. 43, 16–17. doi: 10.1016/S0018-506X(02)00033-8
Walther, G. R., Post, E., Convey, P., Menzel, A., Parmesank, C., Beebee, T. J. C., et al. (2002). Ecological responses to recent climate change. Nature 416, 389–395. doi: 10.1038/416389a
Ward, G. M., Mahoney, S. M., Joly, S., and Reudink, M. W. (2021). Effects of age and weather during moult on mountain bluebird Sialia currucoides structural colouration. J. Avian Biol. 52, 1–12. doi: 10.1111/jav.02616
Weathers, W. W. (1979). Climate adaptation in avian standard metabolic rate. Oecologica 42, 81–89. doi: 10.1007/BF00347620
Welcker, J., Speakman, J. R., Elliott, K. H., Hatch, S. A., and Kitaysky, A. S. (2015). Resting and daily energy expenditures during reproduction are adjusted in opposite directions in free-living birds. Funct. Ecol. 29, 250–258. doi: 10.1111/1365-2435.12321
Wood, S. N. (2011). Fast stable restricted maximum likelihood and marginal likelihood estimation of semiparametric generalized linear models. J. R. Statist. Soc. B 73, 3–36. doi: 10.1111/j.1467-9868.2010.00749.x
Wood, S. N. (2013). On p-values for smooth components of an extended generalized additive model. Biometrika 100, 221–228. doi: 10.1093/biomet/ass048
Wood, S. N., Goude, Y., and Shaw, S. (2015). Generalized additive models for large data sets. Appl. Statist. 64, 139–155.
Keywords: bird, fitness, songbird, temperature, precipitation, scaled mass index, climate change, physiology
Citation: McCloy MWD and Grace JK (2023) Short-term weather patterns influence avian body condition during the breeding season. Front. Ecol. Evol. 11:1154656. doi: 10.3389/fevo.2023.1154656
Edited by:
Todd Jason McWhorter, University of Adelaide, AustraliaReviewed by:
Keith Alan Hobson, Western University, CanadaDavid John Green, Simon Fraser University, Canada
Copyright © 2023 McCloy and Grace. This is an open-access article distributed under the terms of the Creative Commons Attribution License (CC BY). The use, distribution or reproduction in other forums is permitted, provided the original author(s) and the copyright owner(s) are credited and that the original publication in this journal is cited, in accordance with accepted academic practice. No use, distribution or reproduction is permitted which does not comply with these terms.
*Correspondence: Michael W. D. McCloy, bXdkbWNjbG95QHRhbXUuZWR1