- 1Departamento de Genética Molecular y Microbiología, Pontificia Universidad Católica de Chile, Santiago, Chile
- 2Departamento de Química Ambiental, Universidad Católica de la Santísima Concepción, Concepción, Chile
- 3Departamento de Medio Ambiente y Energía, Facultad de Ingeniería, Centro de Investigación en Biodiversidad y Ambientes Sustentables (CIBAS), Universidad Católica de la Santísima Concepción, Concepción, Chile
- 4Instituto de Ciencias Ómicas y Biotecnología Aplicada, Pontificia Universidad Católica del Perú, Lima, Peru
- 5Departamento de Ciencias, Pontificia Universidad Católica del Perú, Lima, Peru
- 6Research Group Plant Defense Physiology, Max Planck Institute for Chemical Ecology, Jena, Germany
One of the major impacts of climate change is increasing global temperatures. Because warming is expected to affect plant morphological and chemical traits, it may therefore also influence plant interactions with other trophic levels, including herbivores. Here, we simulated a climate warming scenario of +2.7°C in the field using open-top chambers and assessed the effects of warming on plant performance (growth, leaf area, and chlorophyll), leaf nutrients (nitrogen and carbon), and primary (amino acids and carbohydrates) and secondary (toxic aristolochic acids) metabolites in the plant Aristolochia chilensis. We performed untargeted metabolomics analyses for estimating general changes in foliar metabolites between ambient control and warming-treated plants. Bioassays were additionally conducted to evaluate how changes in host plant chemistry affected growth and nutritional parameters in first-instar larvae of the specialist lepidopteran herbivore Battus polydamas archidamas. We found that warming did not significantly affect plant performance, but did result in significant changes in leaf nutrients, and primary and secondary metabolites, although in opposite directions. While primary metabolites (specifically, nitrogen-containing compounds) decreased in response to treatment, aristolochic acids increased. Untargeted metabolomics analyses showed that, of a total of 824 features, 50 were significantly different between ambient control and warming-treated plants; some of these were identified by MS/MS spectra as amino acids. Larvae feeding on warming-treated plants, showed significantly enhanced growth, food conversion efficiency, and lipid concentration. Our study contributes to current understanding of climate change impacts on trophic interactions, showing that projected temperature increases lead to changes in the resistance phenotype of the host plant, favoring nutrition and growth of a unique specialist herbivore.
Introduction
Increasing atmospheric temperatures linked to global climate change are expected to have major implications for terrestrial biological processes. Average air temperature is projected to rise 2–4°C by 2,100 under current rates of climate change [Intergovernmental Panel on Climate Change (IPCC), 2018]. Warming is likely to alter morphological and chemical plant traits that affect plant performance. Some meta-analyses have shown that global warming generally enhances plant growth, photosynthesis, dark respiration, specific leaf area, as well as other plant metabolic processes (Wu et al., 2011; Poorter et al., 2012; Fu et al., 2015). In addition to direct effects on plant growth and physiology, warming may alter leaf primary and secondary metabolites by modifying carbon metabolism and the translocation and accumulation of plant nutrients (Ali et al., 2020), which collectively affect host plant quality. Given that warming may strongly influence plant morphological and chemical traits, changes in air temperature can subsequently affect plant interactions with other trophic levels.
Leaf chemical traits (here defined as primary and secondary metabolites) mediate interactions between plants and herbivores (Kudo, 2003; Pérez-Harguindeguy et al., 2003; Njovu et al., 2019). Primary metabolites often relate to the plant’s nutritional component for insect performance (Awmack and Leather, 2002). While the nitrogen (N) and amino acids are linked to proteins fundamental for insect growth and fecundity, carbon (C), and sugars (=nonstructural carbohydrates) are a proxy for energy sources (Awmack and Leather, 2002; Schoonhoven et al., 2005). Secondary metabolites (i.e., alkaloids, glucosinolates, terpenoids, and phenolic compounds), in contrast, act as deterrents and/or digestibility reducers for herbivores, restricting herbivore feeding and growth (Mithöfer and Boland, 2012). Since herbivore performance is frequently explained by the components of host plant quality (Descombes et al., 2020; Kuczyc et al., 2021), variations in primary and secondary metabolites likely imply consequences for plant-herbivore interactions.
Plant-herbivore interactions can be reshaped by climate change (Hamann et al., 2020); it is therefore crucial to understand how climate change scenarios may alter host plant quality and palatability, either increasing or decreasing plant susceptibility to herbivores. Leaf chemical traits vary under elevated temperatures. For example, nitrogen level and some amino acids have been shown to decrease in response to warming (Du et al., 2014; Liu et al., 2022), while C:N ratio, carbohydrate concentrations and some secondary metabolites, including flavonoids, phenols, terpenes, and glucosinolates often increased (Sallas et al., 2003; Bidart-Bouzat and Imeh-Nathaniel, 2008; Kuczyc et al., 2021; Liu et al., 2022). Changes in leaf chemical traits can alter plant susceptibility to herbivory. For example, Dury et al. (1998) reported that elevated temperatures reduced leaf nitrogen but increased condensed tannin levels in Quercus robur (Fagaceae), negatively affecting development and fecundity of generalist insect herbivores. Similarly, a recent study on Sinapis alba (Brassicaceae) showed that warming increased the C:N ratio and concentrations of glucosinolates in leaves, which led to increased feeding, but lower performance in larvae of the specialist herbivore Pieris napi (Lepidoptera; Kuczyc et al., 2021). Understanding climate-mediated shifts in plant interactions involving specialist herbivores is of particular interest because they are monophagous and have evolved sophisticated mechanisms to overcome host plant toxins (Ali and Agrawal, 2012).
The system Aristolochia chilensis and Battus polydamas archidamas is suitable to evaluate plant-mediated indirect effects on performance of a specialist herbivore (Figure 1). Aristolochia chilensis is a perennial herb endemic to Chile, and occurs naturally from hyper-arid to Mediterranean-like climates. Aristolochia chilensis is highly toxic due to its high concentrations of secondary metabolites, specifically aristolochic acids (AAs; Urzúa et al., 2013; Santander et al., 2015), which typically deter herbivory by non-specialist plant herbivores (Jeude and Fordyce, 2014). Battus polydamas archidamas, however, has evolved the ability to sequester AAs (Sime et al., 2000; Pinto et al., 2011), and selectively targets A. chilensis as a food source and for oviposition (Peña and Ugarte, 1996). Of the seven recognized AAs synthesized by A. chilensis (Santander et al., 2015), AAI and AAII are particularly genotoxic, mutagenic, and nephrotoxic (Michl et al., 2014). AAI and AAII have even been found to positively affect feeding behavior and development of first instar larvae of B. polydamas archidamas (Pinto et al., 2009). Here, we simulated climate warming (+2.7°C) in the field using open-top chambers (OTCs) and assessed the effects of short-term temperature increases (3 months) on plant performance (chlorophyll, leaf area, and above-ground biomass), leaf nutrition (nitrogen and carbon level), and leaf primary (amino acids and carbohydrates), and secondary metabolites (toxic aristolochic acids) in the plant A. chilensis. Changes in the leaf metabolomic profile in response to warming were also monitored. Untargeted metabolomic analysis involves simultaneous unbiased measurement of as many metabolites as possible from biological samples (Patti et al., 2012), which is advantageous for obtaining a broad overview of how metabolite profiles change between treatments. We performed untargeted metabolomics analyses for estimating general changes in foliar chemistry between ambient control and warming-treated plants. Additionally, bioassays were conducted to assess how changes in host plant chemistry affect growth and nutritional parameters of B. polydamas archidamas. Specifically, we asked the following questions: (1) Is performance of A. chilensis positively impacted by warming? (2) Does warming affect leaf primary and secondary metabolites in A. chilensis? and (3) Do changes in leaf chemical traits cause variations in growth and nutritional parameters of B. polydamas archidamas?
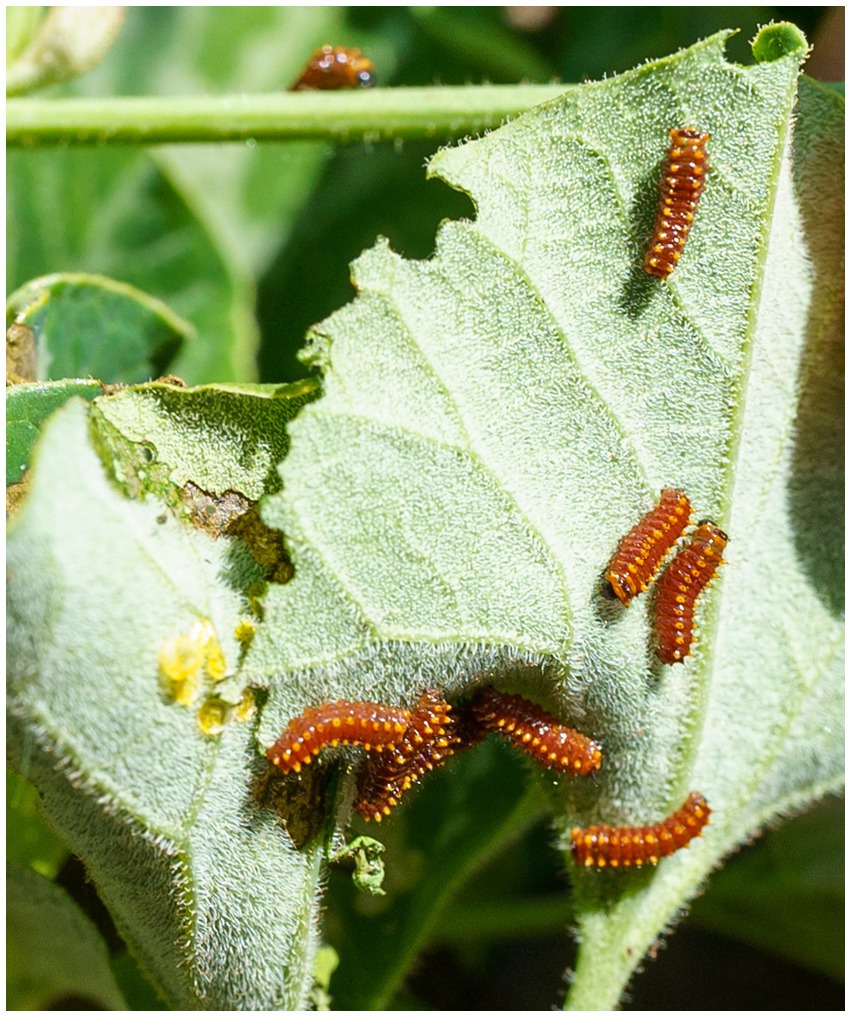
Figure 1. Battus polydamas archidamas first instar larvae feeding on an Aristolochia chilensis leaf in the field.
Materials and methods
Study system
Aristolochia chilensis (Bridges ex Lindl.) is a short-lived perennial herb endemic to Chile. It is distributed across a large climate gradient, from the hyper-arid Atacama Desert at the northern extent of its range (27° 30’ S) to a Mediterranean-type climate in its southern extent in central Chile (33° 29’ S; Ruiz, 2001). Aristolochia chilensis has been described as a therophyte (Figueroa et al., 2016), i.e., a species that completes its life cycle rapidly during periods favorable for growth and reproduction and survives unfavorable conditions by entering a state of dormancy. Growth and flowering of A. chilensis occur from September to December/January (Stotz and Gianoli, 2013; González-Teuber, personal observations). A. chilensis contains high concentrations of seven toxic AAs, being AAI and AAII the most abundant (Urzúa et al., 2013; Santander et al., 2015). Battus polydamas archidamas (Lepidoptera: Papilionidae: Troidini), the only species of this genus in Chile, is monophagous and selectively targets Aristolochia plants for feeding and oviposition (Peña and Ugarte, 1996). Its range extends from the Atacama Desert (27° S) to the Mediterranean region (34° S), and is coextensive with the distribution of the host plant A. chilensis (Peña and Ugarte, 1996).
Field experiment
In September 2020, 20 A. chilensis plants were selected in the field at Praderas de lo Aguirre (33°27′40.8”S 70°54′33.0”W), Región Metropolitana, Santiago, Chile. The climate at the study site is Mediterranean, with mean annual rainfall of 183 mm and an annual temperature of 22°C (Chilean Meteorology Service). A completely randomized experimental design was applied, with 10 plants used for each warming treatment. Warming was simulated using open-top chambers (OTCs), passive warming systems widely used to simulate climate warming in the field (Welshofer et al., 2018). A group of 10 plants was completely enclosed within a chamber (W = warming-treated plants), while a second group of 10 plants was excluded and designated as an ambient control (C = control plants). Newly emerged plants were selected, which showed similar size, expressed in the total number of branches and leaves. The chambers are an open-topped structure hexagonal in shape, constructed of transparent Plexiglass® with dimensions of 30 cm (height) by 92 cm (diameter). Chamber walls were perforated with 20 holes 1.5 cm in diameter to allow natural air movement and prevent excessive temperature increases within the structure. Air temperature and soil humidity in control and warming treatments were measured throughout the duration of the experiment. Temperature measurements were performed using iButtons (MCIelectronics) placed 15 cm above the ground in proximity to seven control and seven warming-treated plants. Diurnal and nocturnal temperatures were calculated as the mean of measurements performed between the hours of 08.00 AM–08.00 PM and 08.00 PM–08.00 AM, respectively. OTCs significantly increased daily mean air temperature by 2.7°C, but no significant differences in nocturnal mean air temperature were observed inside OTCs relative to ambient conditions (Supplementary Figure 1S). Soil humidity (%), measured for all plants using a soil moisture meter (PMS710), did not differ between ambient control and warming-treated plants (C = 41.1 ± 0.4; W = 42.1 ± 0.7). In January 2021, prior to senescence of above-ground biomass, leaves and roots from both treatments (N = 10 plants per treatment) were collected for chemical analyses. Leaf and root samples were immediately submerged in liquid nitrogen after collection. Once in the lab, tissues were ground, lyophilized and maintained at −20°C until analyses. Additionally, above-ground plant biomass was harvested for all plants comprising both control and warming treatments.
Plant growth and chlorophyll measurements
For leaf area measurements, the three youngest leaves per plant were measured and averaged to obtain an individual value. Leaf area (cm2) was estimated using ImageJ software. Shoots of plants from both treatments were collected and oven-dried for 72 h at 80° C and above-ground dry biomass (g) was determined to estimate growth during the experiment. To determine chlorophyll, leaves (100 mg per sample) were extracted using 80% acetone and absorbance was measured at 664 and 647 nm, using a microplate reader spectrophotometer (TECAN, Infinite2000pro, Austria). Total chlorophyll (mg mL−1) level was determined using established formulae described by Steubing et al. (2002).
Untargeted metabolomics
Leaf samples for mass spectrometry measurements were obtained from both plant groups in the field (N = 10 plants per treatment). Leaf samples were shock-frozen in liquid nitrogen immediately after dissection for transportation, lyophilized, and subsequently stored at −80°C. Stock solutions were prepared by grinding 40 mg of leaves under cold conditions. The leaf powder was dissolved in 1 mL of MeOH, vortexed for a period of 5 min, then filtered with a 0.20 μM syringe disk filter. Stock solutions were stored at −20°C until measurement. A dilution solvent, comprising a mixture of MeOH and ddH2O containing 0.5% formic acid (FA; 1:1), was used to dilute stock solutions at a ratio of 1:200. The samples were directly injected into an Orbitrap Q-Exactive HF mass spectrometer (MS; Thermo Fisher Scientific, United States) at a flow rate of 20 μL/min. The MS data were acquired at a resolution of 60,000 in positive mode data-dependent acquisition (DDA) with a scan range of m/z 50–750. Raw data from this experiment were first extracted using an in-house data processing tool built in MatLab (vR2022a). Tentative identification was performed using the Metlin and the mzCloud repository.
Plant nutritional and secondary metabolite analyses
Percentage (%) of carbon (C) and nitrogen (N) was measured in leaf (N = 10 plants per treatment) and root (N = 5 plants per treatment) samples (1 mg per sample) by dry combustion with a Perkin Elmer Elemental Analyzer (EA 2400 Series II CHNS/O Analyzer). Both leaf and root protein content were estimated based on the assumption that proteins comprise 16% nitrogen (Templeton and Laurens, 2015). Amino acid concentration (mg g−1 dry mass) was determined in 100 mg of leaves (N = 10 plants) by reverse phase HPLC-DAD using the derivatization method with diethyl ethoxymethylenemalonate (DEEMM), as described by Gómez-Alonso et al. (2007), following hydrolyzation for 24 h at 110°C with 6 N HCl. Subsequently, solid-free samples were treated with DEEMM to transform amino acids into aminoenones at pH 9.0. Amino acids were then identified according to their retention times and spectral characteristics of their derivatization products (280, 269, or 300 nm). A total of 16 amino acids (arginine, aspartic acid, cysteine, glycine, glutamic acid, histidine, isoleucine, alanine, lysine, leucine, phenylalanine, valine, methionine, serine, tyrosine, and threonine) were identified and quantified by comparison with retention times and peak areas of standards (Sigma-Aldrich). Leaf carbohydrate concentrations (sucrose, glucose, and fructose) were determined in 100 mg of leaves (N = 10 plants) by HPLC/RI, using an Aminex HPX-87P (300 mm × 7.8 mm) column and water as eluent (0.6 mL/min) at a temperature of 85°C. Samples were previously freeze-dried, powdered and extracted for 10 min with milli-Q water by ultrasound, followed by heating at 60°C for a period of 30 min. Proteins were then precipitated with Carrez reagent (Pico et al., 2015). Samples were finally centrifuged and filtered (0.20 μm) to be analyzed by HPLC. Carbohydrates (mg g−1 dry mass) were identified and quantified by comparison with retention times and peak areas of standards (Sigma-Aldrich). Concentrations of AAI and AAII (mg g−1 dry mass; N = 10 plants) were measured using HPLC-DAD following the method described by Araya et al. (2018). A methanol extraction was performed on the lyophilized and pulverized sample (100 mg per sample). Liquid–liquid separations and a subsequent solid phase extraction (SPE) C18 were performed using 100 μL of the extract. Calibration curves were constructed for each aristolochic acid by plotting the peak area versus concentration of each standard (Sigma-Aldrich).
No-choice feeding assays
To further investigate effects of warming-induced metabolic changes in A. chilensis on herbivores, feeding experiments using first instar larvae of B. polydamas archidamas were conducted. We assessed growth, nutritional parameters, and total lipid concentration in larvae fed on the youngest leaves harvested from ambient control and warming-treated plants. Four leaves per plant were obtained from both plant groups and immediately transported to the lab in separate plastic bags (under humid conditions) for feeding assays. No-choice assays were initiated after 1 h of leaf collection. First instar larvae of B. polydamas archidamas were collected a day prior to the assays from wild Aristolochia plants not included in the experiment. All larvae were reared individually in plastic vials at room temperature. Before starvation (4 h), larvae were fed with fresh young A. chilensis leaves. A single randomly selected larva was weighed and placed in a Petri dish containing one leaf of either an ambient control or a warming-treated plant. Leaves were similarly weighed immediately prior to the assay and kept hydrated by placing the petiole in wet cotton. All treatments were completely randomized (N = 20 larvae per diet treatment). The trial was run for 48 h and checked at regular intervals to ensure that leaves were hydrated and that caterpillars had sufficient food, replenishing consumed leaves as necessary. Larval weight (g), leaf weight (g), and feces weight (g) were recorded at intervals of 24 and 48 h to evaluate short-term growth and nutritional parameters. Here, we focused on short-term growth and nutritional parameters of Battus larvae. An earlier study on intermediate temperature effects on lepidopteran performance reported that both short- and long-term experiments showed similar results (Kingsolver and Woods, 1998). Growth and nutritional parameters comprised relative growth rate (RGR: grams of tissue gained per gram of larva per day), relative consumption rate (RCR: grams of leaf consumed per gram of larva per day), efficiency of conversion of ingested food to body matter (ECIF: ability of larva to convert ingested food into tissue), and efficiency of conversion of digested food to body matter (ECDF: ability of larva to convert digested food into tissue), calculated using standard procedures described in Waldbauer (1968):
where G is fresh larval weight gained during the feeding period, T is duration of the feeding period, A is fresh mean larval weight gain (g) during the feeding period (obtained by averaging the initial and final weight of the larva for each feeding period), F is fresh weight of food ingested (g), and H is fecal weight (g) per larva. Individual caterpillars were lyophilized and weighted to obtain dry mass, and total lipids were extracted from approx. 30–40 mg dry weight of whole larvae. This procedure was developed using vanillin reagent according to the method described by Van Handel (1985). Lyophilized samples were resuspended in chloroform/methanol (1:1) for 5 min and the supernatant extracted and evaporated on a hot plate at 96° C, following which 120 μL of sulfuric acid was added. After cooling, vanillin reagent was added and mixed for several minutes. Samples were quantified against a standard dilution of commercial canola oil (Sigma Aldrich). Samples and standards were assayed in a spectrophotometer (TECAN, Infinite2000pro, Austria) set at 525 nm at room temperature. Total lipid concentration was determined by referencing the canola oil standard curve and expressed in mg g−1 dry larval tissue. The total lipid concentration was measured in both larvae fed on ambient control plants (N = 14 larvae) and warming-treated plants (N = 17 larvae).
Statistics
Effect of warming (fixed effect) on plant performance and leaf chemical traits was determined using a One-way ANOVA. Differences in the composition of amino acids between control and warming-treated plants were determined using a permutational multivariate analysis of variance (PERMANOVA; 9,999 permutations; Anderson, 2001). To visualize differences in amino acid composition and aid in the interpretation of PERMANOVA results, a principal component analysis (PCA), based on the Euclidean matrix of dissimilarity, was performed using the “ggplot2” package. One-way ANOVAs were performed using all detected metabolomic features to determine how many were significantly different between ambient control and warming-treated plants. Larval growth, nutritional parameters, and total lipid concentration were analyzed using a generalized linear mixed model analysis using warming treatment (ambient control and warming) as fixed factor and plants as a random factor. Assumptions of normality and homogeneity of variance were tested prior to statistical analyses. Since root carbon level did not show homogeneity of variance, data were analyzed using the Mann–Whitney test. One-way ANOVAs and Mann–Whitney test were performed in Statistica 7.0 software (StatSoft, Inc.). PERMANOVA and PCA were analyzed using PAST 4.03 software (Hammer et al., 2001).
Data were analyzed using a generalized linear mixed model using pollination treatment (presence and absence of animal pollinators) and slope-type (steep vs. gentle slope zones) as fixed factors. Individual plants were included as a random factor.
Results
Plant growth and chlorophyll measurements
Total above-ground biomass (F1,18 = 1.21; p = 0.284), leaf area (F1,18 = 0.00; p = 0.979), and total chlorophyll (F1,18 = 0.37; p = 0.546) were not significantly different between ambient control and warming-treated plants (Table 1).
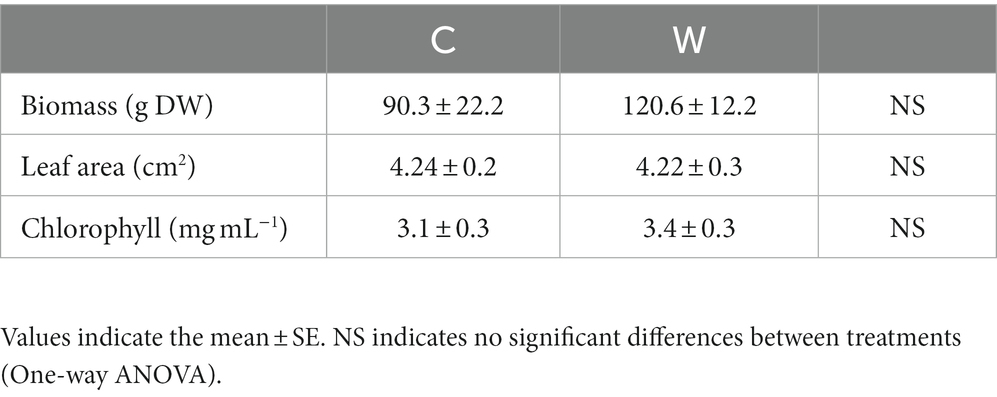
Table 1. Plant performance of Aristolochia chilensis subjected to ambient temperature (C: ambient control plants) and climate warming (W: warming-treated plants) in the field.
Metabolomics
Metabolome analysis showed that most features did not significantly differ between control and warming treatments (Supplementary Figure 2S). Of a total of 824 features, only 50 were found to be significantly different in this respect (p < 0.05, One-way ANOVA). The clustergram shows a general pattern in terms of how feature intensity of these 50 metabolites varied according to treatment type (Figure 2). Individual plants with similar metabolomic patterns were positioned closer together, showing that ambient control versus warming-treated plants grouped separately (Figure 2). Of the 50 distinct features, three were identified by MS/MS spectra as amino acids, comprising proline (m/z = 116,070), glutamine (m/z = 147,076), and arginine (m/z = 175,118). Signal intensity of these three amino acids was found to be significantly lower in warming-treated plants than in ambient control plants (Proline: mean ± SE: C = 8.22 ± 0.09, W = 7.86 ± 0.11, F1,18 = 5.94, p = 0.025; Glutamine: mean ± SE: C = 8.43 ± 0.08, W = 8.15 ± 0.08, F1,18 = 5.75, p = 0.027; Arginine: mean ± SE: C = 6.75 ± 0.50, W = 4.38 ± 0.48, F1,18 = 11.50, p = 0.003), showing the same general tendency for amino acid measurements by HPLC (Supplementary Table S1).
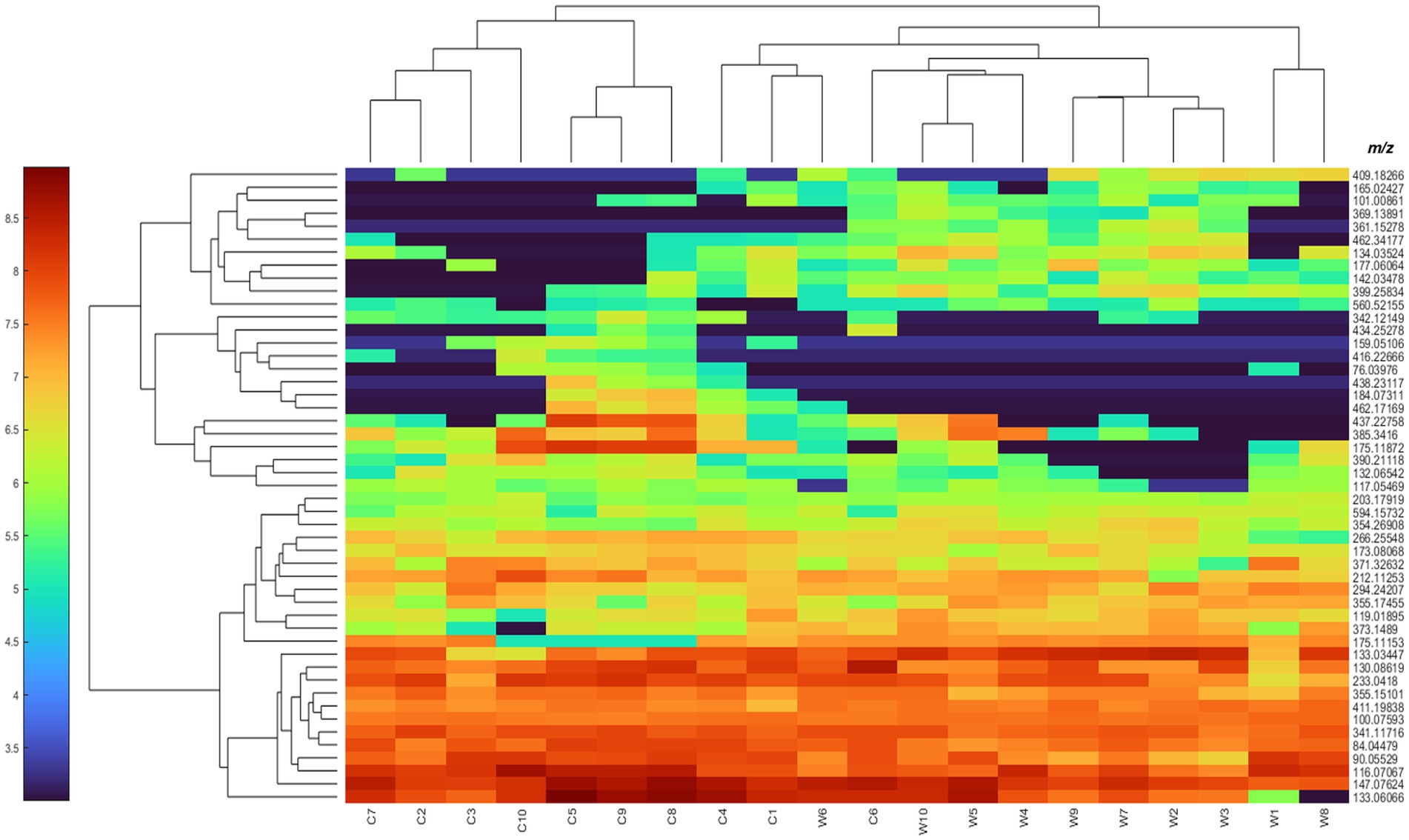
Figure 2. Clustergram showing changes in intensity of 50 features exhibiting significant differences (One-way ANOVA) between ambient control (C) and warming-treated (W) Aristolochia chilensis plants. m/z (mass-to-charge ratio) of features is indicated. Red color denotes upregulation of features while blue color denotes downregulation of features across the specific samples/conditions.
Plant nutritional and secondary metabolite analyses
Leaf and root carbon level did not significantly differ between ambient control and warming-treated plants (leaves: F1,18 = 4.38; p = 0.051; roots: Z = 0.41; p = 0.676). In contrast, nitrogen level decreased significantly in both tissues in response to warming (leaves: F1,18 = 10.52; p = 0.004; roots: F1,8 = 6.73; p = 0.031; Figures 3A,B). A significant increase in the C:N ratio from 8 to 10 was observed in leaves (F1,18 = 10.64; p = 0.004). For roots, no significant changes in the C:N ratio were observed (F1,8 = 0.42; p = 0.531). The total amino acid concentration was significantly lower in warming-treated plants than in the ambient control (F1,18 = 12.23; p = 0.002; Figure 4A). Among the 16 amino acids that could be measured, 14 decreased significantly in response to warming, whereas two were not significantly affected (Supplementary Table S1). PERMANOVA indicated that the amino acid composition differed significantly between treatments (F = 3.94; p = 0.018). This was visualized by PCA, showing a clear separation of amino acid composition between ambient control and warming-treated plants (Figure 4B). Regarding carbohydrates, sucrose (F1,18 = 0.38; p = 0.545), fructose (F1,14 = 1.12; p = 0.307), and glucose (F1,14 = 0.86; p = 0.369) concentrations were not significantly different between treatments (Figure 5); however, there was a trend toward higher concentrations in warming-treated plants. For secondary metabolites, in contrast, warming significantly affected the concentration of both AAI (F1,14 = 5.06; p = 0.041) and AAII (F1,14 = 9.69; p = 0.007), with higher accumulations of both AAs detected in warming-treated plants (Figure 6).
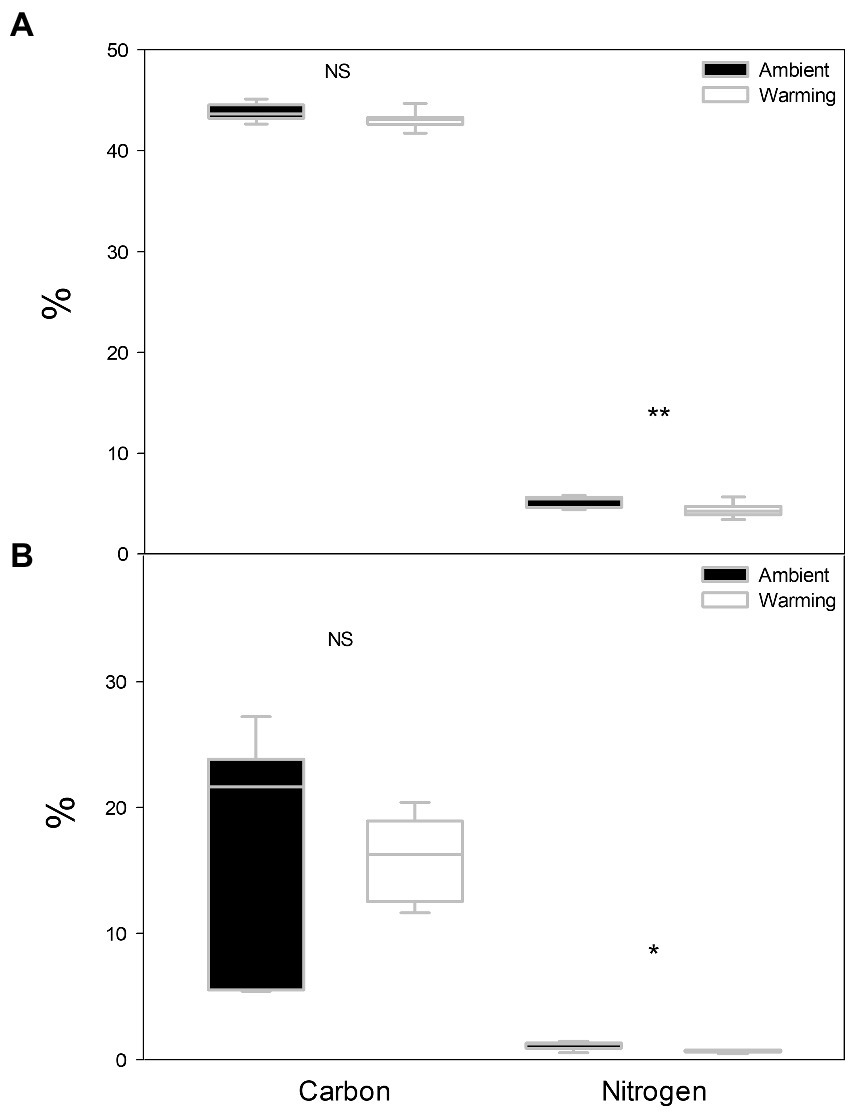
Figure 3. Effect of warming on carbon and nitrogen percentage in leaves (A) and roots (B) of Aristolochia chilensis plants. Asterisks indicate the significance level of differences in the elemental composition between ambient control and warming-treated plants: **p < 0.01, *p < 0.05, NS, no significant difference (One-way ANOVA).
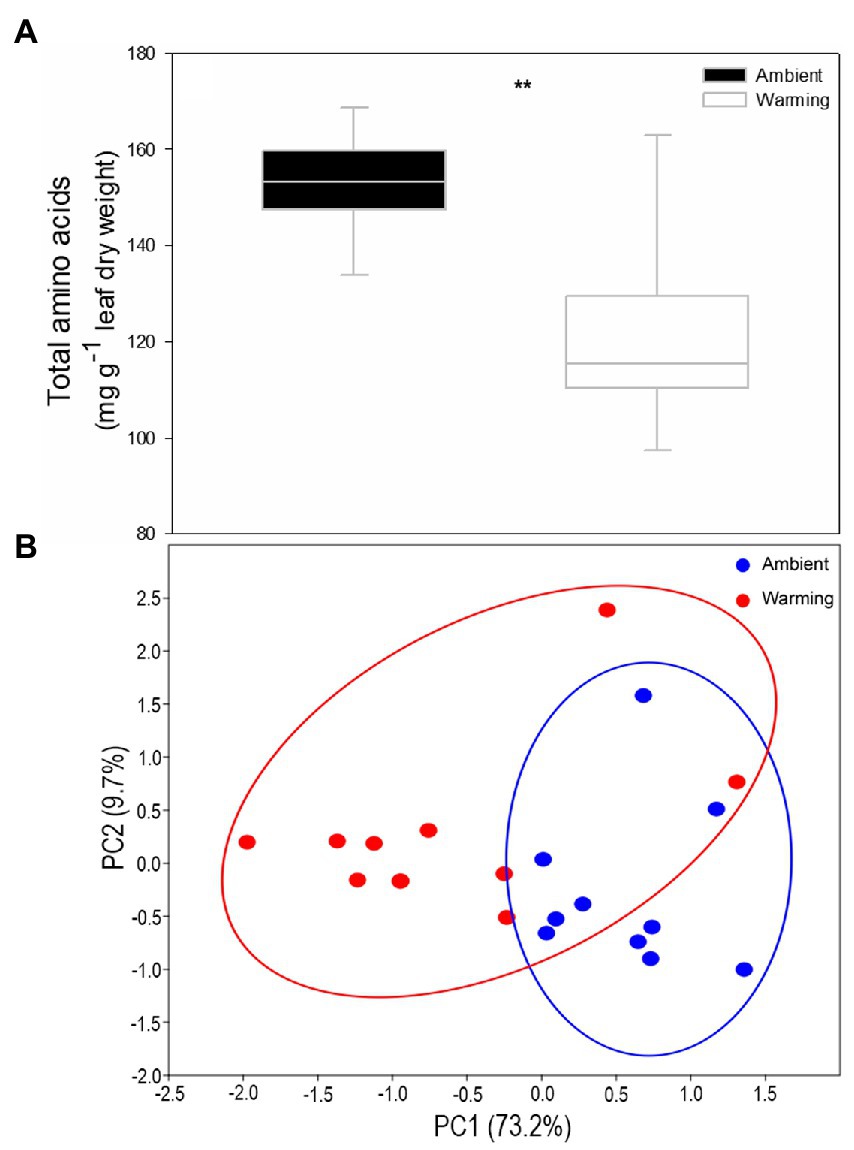
Figure 4. Effect of warming on total foliar amino acid concentration (mg g−1 dry weight) in Aristolochia chilensis plants. Asterisks indicate the significance level of differences in total amino acids between ambient control and warming-treated plants: **p < 0.01 (One-way ANOVA) (A). Principal component analysis (PCA) of foliar amino acid composition between ambient control and warming-treated plants is shown (B).
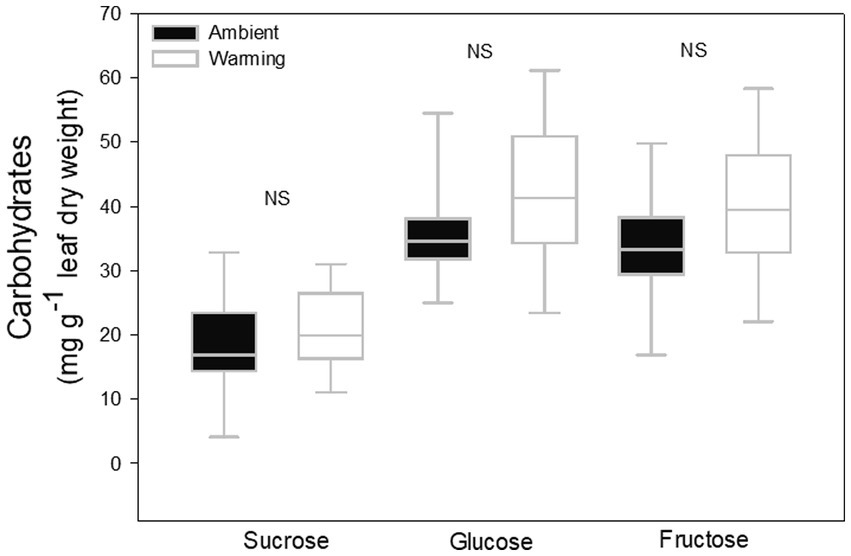
Figure 5. Effect of warming on foliar carbohydrate concentrations (sucrose, glucose, and fructose; mg g−1 dry weight) in Aristolochia chilensis plants. NS indicates no significant difference between ambient control and warming-treated plants (One-way ANOVA).
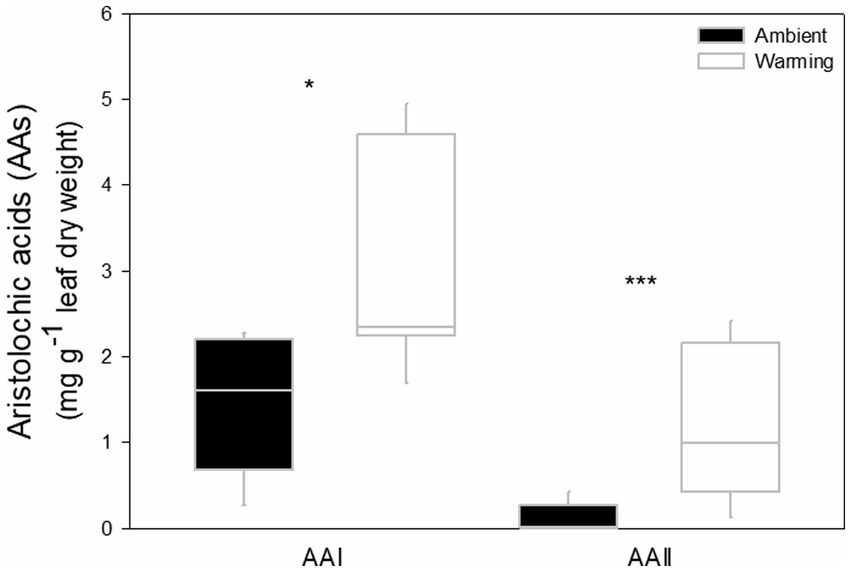
Figure 6. Effect of warming on the concentration of foliar aristolochic acids (AAs) I and II (mg g−1 dry weight) in Aristolochia chilensis. Asterisks indicate the significance level of differences in aristolochic acids between ambient control and warming-treated plants: **p < 0.01, *p < 0.05 (One-way ANOVA).
Growth and nutritional parameters for Battus polydamas archidamas
No-choice feeding assays showed no significant differences after 24 h in RGR (F1,21 = 3.77; p = 0.065), RCR (F1,21 = 0.03; p = 0.843), ECIF (F1,21 = 2.42; p = 0.347), or ECDF (F1,21 = 1.55; p = 0.226) of larvae fed on either ambient control or warming-treated leaves (Table 2). However, after 48 h, larvae fed on warming-treated leaves showed significant increases in RGR (F1,16 = 4.83; p = 0.042), ECIF (F1,16 = 6.28; p = 0.023), and ECDF (F1,16 = 7.63; p = 0.013; Table 2). Moreover, total lipid concentration was significantly higher in larvae fed on warming-treated plants than those fed on ambient control plants (F1,13 = 13.63; p = 0.002; Figure 7).
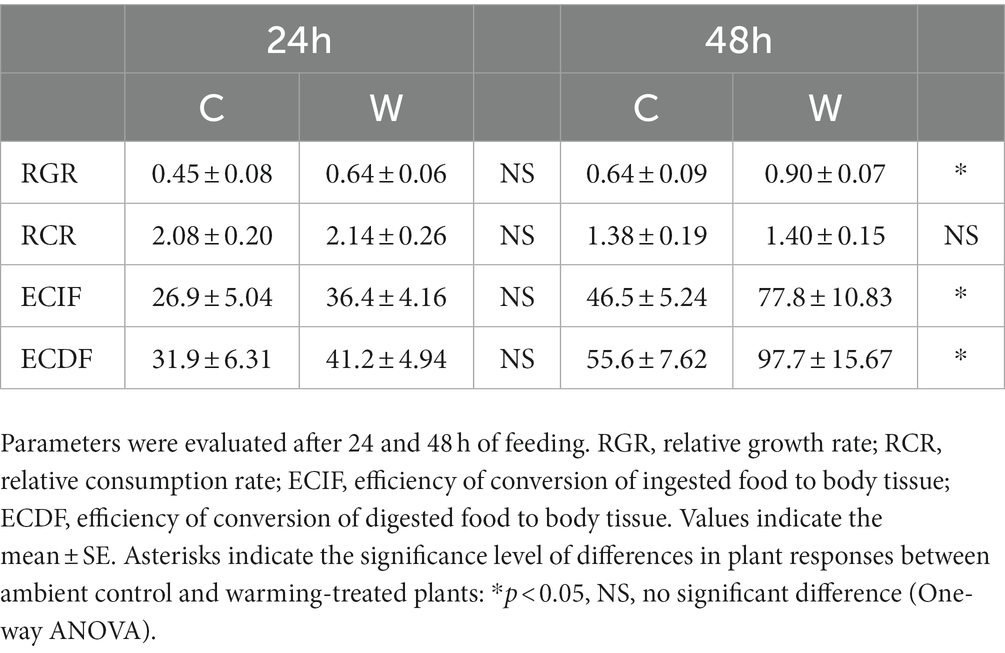
Table 2. Growth and nutritional parameters of Battus polydamas archidamas first instar larvae fed on either ambient control (C) or warming-treated (W) Aristolochia chilensis leaves.
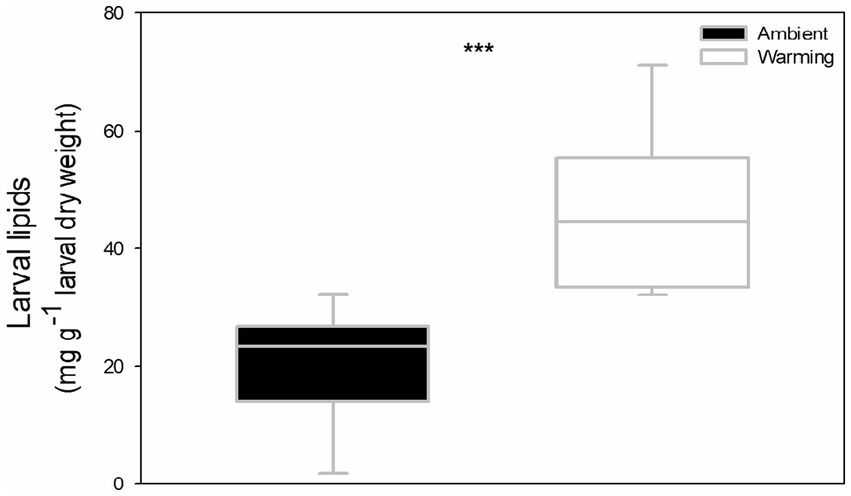
Figure 7. Total lipids (mg g−1 dry weight) in Battus polydamas archidamas first instar larvae after 48 h of feeding on Aristolochia chilensis leaves from either ambient control (C) or warming-treated plants (W). Asterisks indicate the significance level of differences in aristolochic acids between ambient control and warming-treated plants: ***p < 0.001 (One-way ANOVA).
Discussion
Our results showed that an increase in air temperature, as it is predicted by global climatic projections, does not negatively impact the performance of A. chilensis under natural conditions. However, when subjected to warming, A. chilensis showed considerable changes in essential primary and secondary metabolites associated with nitrogen balance and defensive responses. These changes in plant metabolites seem to be involved in herbivore-resistance phenotype alterations in this species, leading to shifts in growth and nutrition of the specialist herbivore.
Plant growth and chlorophyll were not affected by warming in A. chilensis, suggesting that an increase of around 3°C is still within the temperature optimal range of growth for this species. Considering that chlorophyll level is highly correlated with photosynthesis (Fleischer, 1935; Croft et al., 2017) and that no increase in carbohydrate concentrations was observed in A. chilensis in response to warming, our data suggest that the photosynthetic activity was maintained under elevated temperatures. This is supported by the lack of change in growth parameters (leaf area and biomass) in A. chilensis under warming. Previous research and meta-analyses have shown that a mild increase in mean temperature of 2–4°C, as predicted by climate models, generally promotes photosynthesis and growth parameters in plants (Wu et al., 2011; Poorter et al., 2012; Descamps et al., 2018). For example, in the annual plant Borago officinalis a temperature increases of 3°C stimulated gas exchange, chlorophyll level and vegetative development (Descamps et al., 2018), at least when warming was acting as a single factor. In contrast, Descamps et al. (2020) showed for two Echium species that an increase temperature of 3°C negatively affected photosynthesis and leaf production. The lack of a growth stimulation in response to warming observed in this study in A. chilensis may be linked to the geographic distribution of this desert-adapted plant, which comprises a large environmental gradient, including hyper- and semi-arid environments (Guevara-Araya et al., 2022). In our study, with the exception of elevated temperature, no additional drought stress was present in treated plants of A. chilensis with OTCs. OTCs may, however, affect plants by changing other bioclimatic factors, including plant surface temperature, photosynthetically active radiation (PAR), wind speed, nutrient inputs, and soil temperature and moisture (Hollister et al., 2023).
Metabolome analysis showed that most features were not different between treatments. 50 features, however, significantly differed between ambient control and warming-treated plants. Of these, a first cluster is clearly over-expressed in warming-treated plants, whereas a second cluster is less expressed in the same plant group. Some of these less expressed features were identified as amino acids, which showed the same general tendency as HPLC measurements. Approximately 1.8% of untargeted metabolomics analyses can currently be identified (Da Silva et al., 2015). Although the lack of metabolite characterization limits understanding of function (Patti et al., 2012), it nevertheless offers a novel approach to capture phytochemical profiles, which is particularly useful when comparing plant groups subjected to different experimental treatments. In addition, selected primary and secondary metabolites were strongly impacted by experimental warming, but in opposite directions: while leaf N and amino acids declined significantly, aristolochic acid concentrations increased. These results are consistent with previous studies showing that warming leads to declines in leaf nitrogen (Tjoelker et al., 1999; Zhang et al., 2016; Liu et al., 2022) and increases in the concentration of secondary metabolites, including some glucosinolates, terpenes, phenolics, and flavonoids (Zvereva and Kozlov, 2006; Kuczyc et al., 2021; Liu et al., 2022). Other studies, however, have reported that warming increased leaf nitrogen in tree species and grasses (Grogan and Chapin, 2000; Hobbie et al., 2001; Luomala et al., 2003).
Concentrations of most amino acids (with the exception of methionine and tyrosine, which remained unchanged) were lower in warming-treated plants relative to control plants, which may be attributed to a variety of factors. Leaf and root N level was approximately 20 and 40% lower, respectively, in treated than control plants. Moreover, we showed by identification with MS/MS that glutamine decreased significantly under warming. Glutamine is the first organic nitrogen compound derived from the assimilation of ammonium and is a major amino donor for the synthesis of amino acids and other nitrogen-containing compounds in plants (Forde and Lea, 2007). Our results therefore suggest that warming-treated plants were N limited, which consequently affected the synthesis of amino acids and proteins. Previous studies have also reported decreases in N uptake by roots and/or foliar nitrogen in several species in response to warming (Mainali et al., 2014; Jamieson et al., 2015; Liu et al., 2022), and may be attributable to reductions in N-uptake and assimilation protein levels in roots (Giri et al., 2017). Additionally, elevated temperatures may induce changes in root system architecture (Luo et al., 2020), which is considered a major factor in nutrient uptake efficiency in plants (Fitter et al., 1991). In our study foliar aristolochic acids (AAs) were found to increase in response to warming. AAs are biosynthetically related to benzylisoquinoline alkaloids, which are derived from tyrosine (Comer et al., 1969). Strikingly, tyrosine was not significantly affected by increased temperature, in contrast to the trend observed for almost all other measured amino acids. Thus, the increase in aristolochic acid concentrations under warming is likely associated with temperature-dependent synthesis (da Silva et al., 2022).
On the herbivore side, results indicated that growth, food conversion efficiency, and total lipid concentration in B. polydamas archidamas larvae were significantly enhanced following ingestion of warming-treated plant leaves, while leaf consumption was not affected. Compared with ambient control plants, leaves of warming-treated plants were poorer in nitrogen-containing compounds, but richer in aristolochic acids. Nitrogen is a key limiting nutrient for many herbivorous insects (Mattson, 1980), and previous research has shown that compensatory feeding occurs when insects are provided with artificial diets poor in nitrogen and/or protein level (Simpson and Simpson, 1990; Raubenheimer and Simpson, 1993). In contrast to these findings, our data showed that B. polydamas archidamas larvae did not exhibit a compensatory feeding to declines in nutritional quality (= poor in N level and amino acids). Interestingly, toxic aristolochic acids were positively associated with increased growth and efficiency of conversion of ingested and digested food into body tissue. Specialist herbivores often show a preference for host plants with higher concentrations of secondary metabolites (i.e., glucosinolates), using these defensive compounds for host plant recognition and oviposition (Nieminen et al., 2003; Badenes-Perez et al., 2014). Nevertheless, the breakdown of toxic defensive compounds such as glucosinolates is recognized as costly to herbivores in terms of toxicity and growth (Wittstock et al., 2003; Kuczyc et al., 2021). Our results contradict this, showing rather that a higher sequestration rate of aristolochic acids did not imply a cost for B. polydamas archidamas in terms of growth and nutrition. This is consistent with earlier research, which showed that the presence or higher concentrations of aristolochic acids in artificial diets led to enhanced performance and survival of Battus larvae (Miller and Feeney, 1989; Pinto et al., 2009). A recent study involving the specialist herbivore Ceratomia catalpae showed that higher catapol levels were positively linked to several growth and nutrition-related processes, but negatively associated with larval respiration rates (Lampert, 2020). Additionally, other studies have reported that higher concentrations of toxic secondary metabolites generate a cost to specialist larvae in terms of oxidative state and immunity (Smilanich et al., 2009; Blount et al., 2023). Increased conversion efficiency triggered by aristolochic acids in B. polydamas archidamas might be related to physiological responses to sequestration that have yet to be identified.
Our study provides evidence of plant-mediated indirect positive effects of climate warming on growth and nutrition of a specialist herbivore. Despite the fact that direct temperature effects may also play a role in influencing herbivore performance, often interacting with indirect effects (Kharouba and Yang, 2021), the former were not assessed in this study. The consideration of both direct and indirect effects of climate change is key to determining the overall outcome on species interactions. We showed that elevated temperatures induced changes in the nutritional quality and palatability of A. chilensis through modifications in primary and secondary metabolites. Further studies may consider the integration of different omics techniques, including transcriptomic and metabolomic to gain insight into gene-metabolite networks in plant metabolism under a scenario of climate change. Understanding how changes in transcript abundance link to changes in targeted metabolites is a novel way for identification of gene function for expression of specific plant metabolites. Moreover, since warming increases the risk of drought events, future studies should explore combinations of different abiotic factors to improve understanding of climate change effects on plant metabolism and plant-herbivore interactions.
Data availability statement
The raw data supporting the conclusions of this article is available in the Supplementary material.
Author contributions
MG-T and AM designed the research. MG-T analyzed the data. MG-T and VP-O carried out the field work. CA, AI, and VP-O developed chemical measurements and analyses. MG-T and AM wrote the manuscript. All authors contributed to the article and approved the submitted version.
Funding
This work was supported by the ANID-Max Planck Society Grant, MPG190015 (MG-T and AM) and by the Max Planck Partner Group (MG-T).
Acknowledgments
We are grateful to Alejandra Vergara, Manuel Caro, Erika Calla-Quispe and Hammerly Lino Fuentes-Rivera for their valuable help in the field and lab. We thank Praderas Lo Aguirre for granting permits to work in the field. MG-T acknowledges the Max Planck Society (MPS) through the Max Planck Partner Group. CA is grateful to DI-FME 09/2021 (UCSC).
Conflict of interest
The authors declare that the research was conducted in the absence of any commercial or financial relationships that could be construed as a potential conflict of interest.
Publisher’s note
All claims expressed in this article are solely those of the authors and do not necessarily represent those of their affiliated organizations, or those of the publisher, the editors and the reviewers. Any product that may be evaluated in this article, or claim that may be made by its manufacturer, is not guaranteed or endorsed by the publisher.
Supplementary material
The Supplementary material for this article can be found online at: https://www.frontiersin.org/articles/10.3389/fevo.2023.1152489/full#supplementary-material
References
Ali, J. G., and Agrawal, A. A. (2012). Specialist versus generalist insect herbivores and plant defense. Trends Plant Sci. 17, 293–302. doi: 10.1016/j.tplants.2012.02.006
Ali, S., Rizwan, M., Arif, M. S., Ahmad, R., Hasanuzzaman, M., Ali, B., et al. (2020). Approaches in enhancing thermotolerance in plants: an updated review. J. Plant Growth Regul. 39, 456–480. doi: 10.1007/s00344-019-09994-x
Anderson, M. J. (2001). A new method for non-parametric multivariate analysis of variance. Austral Ecol. 26, 32–46. doi: 10.1111/j.1442-9993.2001.01070.pp.x
Araya, M., García, S., and González-Teuber, M. (2018). Rapid identification and simultaneous quantification of aristolochic acids by HPLC-DAD and confirmations by MS in Aristolochia chilensis using a limited biomass. J. Anal. Methods Chem. 2018, 1–8. doi: 10.1155/2018/5036542
Awmack, C. S., and Leather, S. R. (2002). Host plant quality and fecundity in herbivorous insects. Annu. Rev. Entomol. 47, 817–844. doi: 10.1146/annurev.ento.47.091201.145300
Badenes-Perez, F. R., Gershenzon, J., and Heckel, D. G. (2014). Insect attraction versus plant defense: young leaves high in glucosinolates stimulate oviposition by a specialist herbivore despite poor larval survival due to high saponin content. PLoS One 9:e95766. doi: 10.1371/journal.pone.0095766
Bidart-Bouzat, M. G., and Imeh-Nathaniel, A. (2008). Global change effects on plant chemical defenses against insect herbivores. J. Integr. Plant Biol. 50, 1339–1354. doi: 10.1111/j.1744-7909.2008.00751.x
Blount, J. D., Rowland, H. M., Mitchell, C., Speed, M. P., Ruxton, G. D., Endler, J. A., et al. (2023). The price of defence: toxins, visual signals and oxidative state in an aposematic butterfly. Proc. R. Soc. B 290:20222068. doi: 10.1098/rspb.2022.2068
Comer, F., Tiwari, H. P., and Spenser, I. D. (1969). Biosynthesis of aristolochic acid. Can. J. Chem. 47, 481–487. doi: 10.1139/v69-070
Croft, H., Chen, J. M., Luo, X., Bartlett, P., Chen, B., and Staebler, R. M. (2017). Leaf chlorophyll content as a proxy for leaf photosynthetic capacity. Glob. Chang. Biol. 23, 3513–3524. doi: 10.1111/gcb.13599
da Silva, G. C., de la Cruz-Chacón, I., Honório, A. B. M., Martin, B. C., Sousa, M. C., Campos, F. G., et al. (2022). Temperature and GA3 as modulating factors in the biosynthesis of alkaloids during imbibition and early development of Annona x atemoya Mabb cv. ‘Gefner’ seedlings. Horticulturae 8:766. doi: 10.3390/horticulturae8090766
da Silva, R. R., Dorrestein, P. C., and Quinn, R. A. (2015). Illuminating the dark matter in metabolomics. Proc. Natl. Acad. Sci. U. S. A. 112, 12549–12550. doi: 10.1073/pnas.1516878112
Descamps, C., Marée, S., Hugon, S., Quinet, M., and Jacquemart, A. (2020). Species-specific responses to combined water stress and increasing temperatures in two bee-pollinated congeners (Echium, Boraginaceae). Ecol. Evol. 10, 6549–6561. doi: 10.1002/ece3.6389
Descamps, C., Quinet, M., Baijot, A., and Jacquemart, A.-L. (2018). Temperature and water stress affect plant-pollinator interactions in Borago officinalis (Boraginaceae). Ecol. Evol. 8, 3443–3456. doi: 10.1002/ece3.3914
Descombes, P., Kergunteuil, A., Glauser, G., Rasmann, S., and Pellissier, L. (2020). Plant physical and chemical traits associated with herbivory in situ and under a warming treatment. J. Ecol. 108, 733–749. doi: 10.1111/1365-2745.13286
Du, B., Jansen, K., Junker, L. V., Eiblmeier, M., Kreuzwieser, J., Gessler, A., et al. (2014). Elevated temperature differently affects foliar nitrogen partitioning in seedlings of diverse Douglas fir provenances. Tree Physiol. 34, 1090–1101. doi: 10.1093/treephys/tpu074
Dury, S. J., Good, J. E. G., Perrins, C. M., Buse, A., and Kaye, T. (1998). The effects of increasing CO2 and temperature on oak leaf palatability and the implications for herbivorous insects. Glob. Chang. Biol. 4, 55–61. doi: 10.1046/j.1365-2486.1998.00102.x
Figueroa, J. A., Teillier, S., Guerrero-Leiva, N., Ray-Bobadilla, C., Rivano, S., Saavedra, D., et al. (2016). Vascular flora in public spaces of Santiago, Chile. Gayana Bot. 73, 85–103. doi: 10.4067/S0717-66432016000100011
Fitter, A. H., Stickland, T. R., Harvey, M. L., and Wilson, G. W. (1991). Architectural analysis of plant root systems 1. Architectural correlates of exploitation efficiency. New Phytol. 118, 375–382. doi: 10.1111/j.1469-8137.1991.tb00018.x
Fleischer, W. (1935). The relation between chlorophyll content and rate of photosynthesis. J. Gen. Physiol. 18, 573–597. doi: 10.1085/jgp.18.4.573
Forde, B. G., and Lea, P. J. (2007). Glutamate in plants: metabolism, regulation, and signaling. J. Exp. Bot. 58, 2339–2358. doi: 10.1093/jxb/erm121
Fu, G., Shen, Z.-X., Sun, W., Zhong, Z.-M., Zhang, X.-Z., and Zhou, Y.-T. (2015). A meta-analysis of the effects of experimental warming on plant physiology and growth on the Tibetan plateau. J. Plant Growth Regul. 34, 57–65. doi: 10.1007/s00344-014-9442-0
Giri, A., Heckathorn, S., Mishra, S., and Krause, C. (2017). Heat stress decreases levels of nutrient-uptake and -assimilation proteins in tomato roots. Plan. Theory 6:6. doi: 10.3390/plants6010006
Gómez-Alonso, S., Hermosín-Gutiérrez, I., and García-Romero, E. (2007). Simultaneous HPLC analysis of biogenic amines, amino acids, and ammonium ion as aminoenone derivatives in wine and beer samples. J. Agric. Food Chem. 55, 608–613. doi: 10.1021/jf062820m
Grogan, P., and Chapin, F. S. (2000). Initial effects of experimental warming on above- and belowground components of net ecosystem CO2 exchange in arctic tundra. Oecologia 125, 512–520. doi: 10.1007/s004420000490
Guevara-Araya, M. J., Escobedo, V. M., Palma-Onetto, V., and González-Teuber, M. (2022). Changes in diversity and community composition of root endophytic fungi associated with Aristolochia chilensis along an aridity gradient in the Atacama Desert. Plan. Theory 11:1511. doi: 10.3390/plants11111511
Hamann, E., Blevins, C., Franks, S. J., Jameel, M. I., and Anderson, J. T. (2020). Climate change alters plant-herbivore interactions. New Phytol. 229, 1894–1910. doi: 10.1111/nph.17036
Hammer, Ø., Harper, D. A. T., and Ryan, P. D. (2001). PAST: paleontological statistics software package for education and data analysis. Palaeontol. Electron. 4:9.
Hobbie, E. A., Olszyk, D. M., Rygiewicz, P. T., Tingey, D. T., and Johnson, M. G. (2001). Foliar nitrogen concentrations and natural abundance of 15N suggest nitrogen allocation patterns of Douglas-fir and mycorrhizal fungi during development in elevated carbon dioxide concentration and temperature. Tree Physiol. 21, 1113–1122. doi: 10.1093/treephys/21.15.1113
Hollister, R. D., Elphinstone, C., Henry, G. H. R., Bjorkman, A. D., Klanderud, K., Björk, R. G., et al. (2023). A review of open top chamber (OTC) performance across the ITEX network. Arct. Sci. doi: 10.1139/as-2022-0030
Intergovernmental Panel on Climate Change (IPCC) (2018). “Annex IV: Expert reviewers of the IPCC special report on global warming of 1.5°C” in An IPCC special report on the impacts of global warming of 1.5 °C above pre-industrial levels and related global greenhouse gas emission pathways, in the context of strengthening the global response to the threat of climate change, sustainable development, and efforts to eradicate poverty. (eds.) Masson-Delmotte, P., Zhai, H-O., Pörtner, D., Roberts, J., Skea, P. R., Shukla, A., et al. (Geneva, Switzerland: World Meteorological Organization).
Jamieson, M. A., Schwartzberg, E. G., Raffa, K., Reich, P. B., and Lindroth, R. L. (2015). Experimental climate warming alters aspen and birch phytochemistry and performance traits for an outbreak insect herbivory. Glob. Chang. Biol. 21, 2698–2710. doi: 10.1111/gcb.12842
Jeude, S. E., and Fordyce, J. A. (2014). The effects of qualitative and quantitative variation of aristolochic acids on preference and performance of a generalist herbivore. Entomol. Exp. Appl. 150, 232–239. doi: 10.1111/eea.12159
Kharouba, H. M., and Yang, L. H. (2021). Disentangling the direct, indirect, and combined effects of experimental warming on a plant-insect herbivore interaction. Ecosphere 12:e03778. doi: 10.1002/ecs2.3778
Kingsolver, J. G., and Woods, H. A. (1998). Interactions of temperature and dietary protein concentration in growth and feeding of Manduca sexta caterpillars. Physiol. Entomol. 23, 354–359. doi: 10.1046/j.1365-3032.1998.00105.x
Kuczyc, J., Müller, C., and Fischer, K. (2021). Plant-mediated indirect effects of climate change on an insect herbivore. Basic Appl. Ecol. 53, 100–113. doi: 10.1016/j.baae.2021.03.009
Kudo, G. (2003). Variations in leaf traits and susceptibility to insect herbivory within a Salix miyabeana population under field conditions. Plant Ecol. 169, 61–69. doi: 10.1023/A:1026209017627
Lampert, E. C. (2020). Relationships among catalpol sequestration, metabolism and nutitional efficiencies on the catalpa sphinx, Ceratomia catalpae (Lepidoptera: Sphingidae). Entomol. Sci. 23, 196–203. doi: 10.1111/ens.12413
Liu, Z., Yu, H., Sun, X., and Ding, J. (2022). Effects of elevated temperature on chemistry of an invasive plant, its native congener and their herbivores. J. Plant Ecol. 15, 450–460. doi: 10.1093/jpe/rtab013
Luo, H., Xu, H., Chu, C., He, F., and Fang, S. (2020). High temperature can change root system architecture and intensify root interactions of plant seedlings. Front. Plant Sci. 11:160. doi: 10.3389/fpls.2020.00160
Luomala, E. M., Laitinen, K., Kellomäki, S., and Vapaavuori, E. (2003). Variable photosynthetic acclimation in consecutive cohorts of scots pine needles during 3 years of growth at elevated CO2 and elevated temperature. Plant Cell Environ. 26, 645–660. doi: 10.1046/j.1365-3040.2003.01000.x
Mainali, K. P., Heckathorn, S. A., Wang, D., Weintraub, M. N., Frantz, J. M., and Hamilton, E. W. (2014). Impact of a short-term heat event on C and N relations in shoots vs. roots of the stress-tolerant C4 grass. J. Plant. Physiol. 171, 977–985. doi: 10.1016/j.jplph.2014.04.006
Mattson, W. J. (1980). Herbivory in relation to plant nitrogen content. Annu. Rev. Ecol. Syst. 11, 119–161. doi: 10.1146/annurev.es.11.110180.001003
Michl, J., Ingrouille, M. J., Simmonds, M. S., and Heinrich, M. (2014). Naturally occurring aristolochic acid analogues and their toxicities. Nat. Prod. Rep. 31, 676–693. doi: 10.1039/c3np70114j
Miller, J. S., and Feeney, P. P. (1989). Interspecific differences among swallowtail larvae (Lepidoptera: Papilionidae) in susceptibility to aristolochic acids and berberine. Ecol. Entomol. 14, 287–296.
Mithöfer, A., and Boland, W. (2012). Plant defense against herbivores: chemical aspects. Annu. Rev. Plant Biol. 63, 431–450. doi: 10.1146/annurev-arplant-042110-103854
Nieminen, M., Suomi, J., van Nouhuys, S., Sauri, P., and Riekkola, M. L. (2003). Effect of iridoid glycoside content on oviposition host plant choice and parasitism in a specialist herbivore. J. Chem. Ecol. 29, 823–844. doi: 10.1023/A:1022923514534
Njovu, H. K., Peters, M. K., Schellenberger Costa, D., Brandl, R., Kleyer, M., and Steffan-Dewenter, I. (2019). Leaf traits mediate changes in invertebrate herbivory along broad environmental gradients on Mt. Kilimanjaro. J. Anim. Ecol. 88, 1777–1788. doi: 10.1111/1365-2656.13058
Patti, G. J., Yanes, O., and Siuzdak, G. (2012). Innovation: metabolomics: the apogee of the omics trilogy. Nat. Rev. Mol. Cell Biol. 13, 263–269. doi: 10.1038/nrm3314
Pérez-Harguindeguy, N., Díaz, S., Vendramini, F., Cornelissen, J. H. C., Gurvich, D. E., and Cabido, M. (2003). Leaf traits and herbivore selection in the field and in cafeteria experiments. Austral Ecol. 28, 642–650. doi: 10.1046/j.1442-9993.2003.01321.x
Pico, J., Martínez, M. M., Martin, M. T., and Gómez, M. (2015). Quantification of sugars in wheat flours with an HPAEC-PAD method. Food Chem. 173, 674–681. doi: 10.1016/j.foodchem.2014.10.103
Pinto, C. F., Troncoso, A. J., Urzua, A., and Niemeyer, H. M. (2009). Aristolochic acids affect the feeding behaviour and development of Battus polydamas archidamas larvae (Lepidoptera: Papilionidae: Troidini). Eur. J. Entomol. 106, 357–361. doi: 10.14411/eje.2009.044
Pinto, C. F., Urzua, A., and Niemeyer, H. M. (2011). Sequestration of aristolochic acids from meridic diets by larvae of Battus polydamas archidamas (Papilionidae: Troidini). Eur. J. Entomol. 108, 41–45. doi: 10.14411/eje.2011.005
Poorter, H., Niklas, K. J., Reich, P. B., Oleksyn, J., Poot, P., and Mommer, L. (2012). Biomass allocation to leaves, stems and roots: meta-analyses of interspecific variation and environmental control. New Phytol. 193, 30–50. doi: 10.1111/j.1469-8137.2011.03952.x
Raubenheimer, D., and Simpson, S. J. (1993). The geometry of compensatory feeding in the locust. Anim. Behav. 45, 953–964. doi: 10.1006/anbe.1993.1114
Ruiz, E. (2001). “Aristolochiaceae Juss” in Flora de Chile. eds. C. Marticorena and R. Rodríguez, vol. 2 (Concepción: Editorial Concepción, Universidad de Concepción), 33–34.
Sallas, L., Luomala, E. M., Utriainen, J., Kainulainen, P., and Holopainen, J. K. (2003). Contrasting effects of elevated carbon dioxide concentration and temperature on rubisco activity, chlorophyll fluorescence, needle ultrastructure and secondary metabolites in conifer seedlings. Tree Physiol. 23, 97–108. doi: 10.1093/treephys/23.2.97
Santander, R., Urzúa, A., Olguín, A., and Sánchez, M. (2015). Temporal variation of Aristolochia chilensis aristolochic acids during spring. Molecules 20, 20391–20396. doi: 10.3390/molecules201119704
Schoonhoven, L. M., van Loon, J. J. A., and Dicke, M. (2005) Insect Plant Biology. Oxford: Oxford University Press
Sime, K. R., Feeny, P. P., and Haribal, M. M. (2000). Sequestration of aristolochic acids by the pipevine swallowtail, Battus philenor (L.): evidence and ecological implications. Chemoecology 10, 169–178. doi: 10.1007/PL00001819
Simpson, S. J., and Simpson, C. L. (1990). “The mechanism of nutritional compensation by phytophagous insects” in Insect Plant Interactions. ed. E. A. Bernays, vol. II (Boca Raton: CRC Press), 111–160.
Smilanich, A. G., Dyer, L. A., Chambers, J. Q., and Bowers, M. D. (2009). Immunological cost of chemical defence and the evolution of herbivore diet breath. Ecol. Lett. 12, 612–621. doi: 10.1111/j.1461-0248.2009.01309.x
Steubing, L., Godoy, R., and Alberdi, M. (2002). Métodos de Ecología Vegetal. Santiago de Chile: Editorial Universitaria
Stotz, G. C., and Gianoli, E. (2013). Pollination biology and floral longevity of Aristolochia chilensis in an arid ecosystem. Plant Ecol. Divers. 6, 181–186. doi: 10.1080/17550874.2013.770934
Templeton, D. W., and Laurens, L. M. L. (2015). Nitrogen-to-protein conversion factors revisited for applications of microalgal biomass conversion to food, feed and fuel. Algal Res. 11, 359–367. doi: 10.1016/j.algal.2015.07.013
Tjoelker, M. G., Reich, P. B., and Oleksyn, J. (1999). Changes in leaf nitrogen and carbohydrates underlie temperature and CO2 acclimation of dark respiration in five boreal tree species. Plant Cell Environ. 22, 767–778. doi: 10.1046/j.1365-3040.1999.00435.x
Urzúa, A., Espinoza, J., Olguin, A., and Santander, R. (2013). Phenolic aristolactams from leaves and stems of Aristolochia chilensis. Bol. Latinoam. Caribe Plantas Med. Aromat. 12, 537–542.
Van Handel, E. (1985). Rapid determination of total lipids in mosquitoes. J. Am. Mosq. Control Assoc. 1, 302–304.
Waldbauer, G. P. (1968). The consumption and utilization of food by insects. Adv. Insect Physiol. 5, 229–288. doi: 10.1016/S0065-2806(08)60230-1
Welshofer, K. B., Zarnetske, P. L., Lany, N. K., and Thompson, L. A. E. (2018). Open-top chambers for temperature manipulation in taller-stature plant communities. Methods Ecol. Evol. 9, 254–259. doi: 10.1111/2041-210X.12863
Wittstock, U., Kliebenstein, D. J., Lambrix, V., Reichelt, M., and Gershenzon, J. (2003). Glucosinolate hydrolysis and its impact on generalist and specialist herbivores. Recent Adv. Phytochem. 37, 101–125. doi: 10.1016/S0079-9920(03)80020-5
Wu, Z. T., Dijkstra, P., Koch, G. W., Penuelas, J., and Hungate, B. A. (2011). Responses of terrestrial ecosystems to temperature and precipitation change: a meta-analysis of experimental manipulation. Glob. Chang. Biol. 17, 927–942. doi: 10.1111/j.1365-2486.2010.02302.x
Zhang, T., Yang, S., Guo, R., and Guo, J. (2016). Warming and nitrogen addition alter photosynthetic pigments, sugars and nutrients in a temperate meadow ecosystem. PLoS One 11:e0155375. doi: 10.1371/journal.pone.0155375
Keywords: Aristolochia chilensis, Battus polydamas archidamas, climate change, plant defenses, amino acids, aristolochic acids, herbivory, specialist herbivore
Citation: González-Teuber M, Palma-Onetto V, Aguirre C, Ibáñez AJ and Mithöfer A (2023) Climate change-related warming-induced shifts in leaf chemical traits favor nutrition of the specialist herbivore Battus polydamas archidamas. Front. Ecol. Evol. 11:1152489. doi: 10.3389/fevo.2023.1152489
Edited by:
Lukasz Lech Stelinski, University of Florida, United StatesReviewed by:
Colin Mark Orians, Tufts University, United StatesJames D. Blande, University of Eastern Finland, Finland
Copyright © 2023 González-Teuber, Palma-Onetto, Aguirre, Ibáñez and Mithöfer. This is an open-access article distributed under the terms of the Creative Commons Attribution License (CC BY). The use, distribution or reproduction in other forums is permitted, provided the original author(s) and the copyright owner(s) are credited and that the original publication in this journal is cited, in accordance with accepted academic practice. No use, distribution or reproduction is permitted which does not comply with these terms.
*Correspondence: Marcia González-Teuber, bWdvbnphbGV6dEBiaW8ucHVjLmNs; Axel Mithöfer, YW1pdGhvZWZlckBpY2UubXBnLmRl