- 1Posgrado en Ciencias Biológicas, Unidad de Posgrado, Universidad Nacional Autónoma de México, Ciudad de México, Mexico
- 2Instituto de Geografía, Universidad Nacional Autónoma de México, Ciudad de México, Mexico
Seed long-distance dispersal (LDD) is essential to explain plant migration. However, few studies have addressed the magnitude and frequency of LDD events given the difficulty of measuring them in situ. Computational simulation offers an alternative to the in situ methods. In this study we proposed a simulation model of seed dispersal for two anemochoric conifers, which includes wind patterns, seed and tree traits. We proposed and measured 18 dispersal traits for Abies religiosa and Pinus hartwegii and analyzed variation between traits and species through a principal component analysis. We used the Weather Research and Forecasting (WRF) atmospheric simulation model to obtain wind speed and direction data at the study zone (Iztaccihuatl volcano, central Mexico). We performed linear regression models to simulated seed dispersal events considering horizontal wind speed, seed traits and seed release height, and using a mechanistic model, we integrated vertical wind speed and wind direction. Seeds of both species presented similar morphology but were sorted into two groups. The relationship between wing size and seed weight may be a key element to dispersal, as it influences the interaction of the seed with the wind. Although we expected that P. hartwegii, seed traits and higher distribution would promote more and longer LDD events, A. religiosa presented more and longer LDD. The maximum dispersal distance was 105 m for A. religiosa and 64 m for P. hartwegii. Both species showed differences in dispersal capacity, which may be related to their seed traits. The frequency of LDD events indicates that a low proportion of seeds would travel more than 20 m away from the parent tree. This suggests that, under migration scenarios, new trees movement up would take place gradually.
1. Introduction
Global warming is affecting the reproduction, growth, and phenology of plants in temperate ecosystems (Kreyling, 2010), causing changes in their distribution (Boisvert-Marsh et al., 2014; Osland et al., 2021). In mountain systems, the elevation-climate gradient regulates the distribution of plants; therefore, climatic variations may affect the distribution of populations (Tape et al., 2006; Kelly and Goulden, 2008). Accumulated evidence over the past few decades related to tree line shifts is a clear example of plant distribution changes and upward migration (Grace et al., 2002; Harsch et al., 2009). However, the factors and mechanisms underlying migration require further study.
Environmental conditions may limit plant migration by preventing establishment or by increasing the mortality rate. However, there are other mechanisms that can also limit migration, such as seed dispersal. Experimentally, it has been shown that the artificial addition of seeds outside the limits of their original distribution promotes colonization through the exploration and occupation of new ecological niches (Zobel et al., 2000; Foster et al., 2011). Therefore, migration is limited not only by environmental conditions, but also by seed dispersal.
Plant migration can occur slowly through a gradual advance or quite suddenly through long-distance dispersal (LDD) jumps (Neilson et al., 2005). It has been suggested that LDD events may explain past and current migrations (Clark et al., 1998; Powell and Zimmermann, 2004), but measurement of LDD is not easy because of the difficulty of finding enough seeds and of linking the seed to the parent plant (Nathan et al., 2011; Jordano, 2017; Beckman et al., 2020). It is known that wind dispersal (anemochory) has great potential for achieving LDD (Svenning and Sandel, 2013), but little is known about the maximum distance wind dispersal may reach and about the frequency of the maximum distance events.
Seed traits are related to their dispersal capacity, defining different dispersal syndromes. For anemochoric seeds, wind patterns play a fundamental role. But also seed traits as the mass, shape and appendages, as well as other traits related to the mother plant, as release height, seem to play an important role in its dispersal trajectory. For example, seeds with less mass have the potential to be dispersed greater distances, similarly a discoidal shape has advantages over a spherical shape by interacting with wind currents and achieving greater displacement (Cousens and Rawlinson, 2001; Hewitt and Kellman, 2002; Zhu et al., 2016; Wyse and Hulme, 2021).
Computational modeling offers an alternative to dispersal studies in situ because it can simulate multiple dispersal events that can then be evaluated (Kuparinen, 2006; Nathan et al., 2011). Modeling also provides climate information in regions where meteorological instrumentation is scarce. For example, numerical weather prediction models, such as the Weather Research and Forecasting (WRF) model, can predict wind patterns in one or more space-time domains. However, the use of these kinds of models to simulate dispersal trajectories of anemochoric seeds have not yet been explored in-depth.
Abies religiosa and Pinus hartwegii are two conifer species widely distributed across 3,000 m a.s.l. in central Mexico, mainly over the Trans-Mexican Volcanic Belt (TMVB). At around 4,000 m a.s.l., P. hartwegii forms the tree line. During the final one-third of the Holocene, the tree line upward approximately 500 to 700 m as a result of glacier advances and retreats that changed climatic conditions (Biondi, 2001; Lozano-Garcial and Vázquez-Selem, 2005). Models have indicated that under warming scenarios, these two conifer species may migrate upward in mountain systems (Sáenz-Romero et al., 2012; Manzanilla-Quiñones et al., 2019; Gómez-Pineda et al., 2020). There is already evidence in Mexico of their advance to elevations above their usual distribution range (Torres-Beltrán, 2013; Astudillo-Sánchez et al., 2019), but the extent to which seed dispersal may limit the migration of these two species has not been assessed.
To address the dispersal mechanisms involved in the upward advance of the two species, we asked the following questions: what is the mean distance of a dispersal event? How long and frequent are the LDD events? Do the two species differ in their dispersal capacity? Could LDD favor the migration of each species to higher altitudes? To answer these questions, we proposed the following specific objectives: (1) to identify seed traits related to dispersal and to compare the dispersal capacity of the two species, (2) to simulate wind patterns in a mountain region during the season of seed release of A. religiosa and P. hartwegii in order to model dispersal events, and (3) to calculate a probability of LDD that would promote altitudinal migration for each species. Our hypotheses were: (1) P. hartwegii with lower seed mass will favor larger distance of dispersal than A. religiosa, (2) wind patterns will favor more LDD events at higher elevations, and (3) LDD events will accelerate the migration of both species.
In this context, in the present study, we integrated the results of the WRF model into a mechanistic model that simulates dispersal events to estimate the distance and direction of the dispersal trajectories of anemochoric seeds of A. religiosa and P. hartwegii. Our model constructs dispersal trajectories based on the results of displacement experiments. It also considers different wind patterns for seed dispersal, such as the vertical wind component and two levels of the horizontal component. Even though, in this paper, we do not intend to extrapolate our model to a warming scenario, we consider that it can be fed with different runs of future scenarios to project changes in migration patterns.
2. Materials and methods
2.1. Area and species studied
The study area is located in the TMVB in central region of México. The TMVB includes the highest peaks in the country; like the Iztaccíhuatl volcano, third highest with its summit at 5,284 m a.s.l., is at 19.172°N −98.641°W (Figure 1). Iztaccíhuatl volcano has been inactive since the late 19th century, and this has allowed forest migration to be regulated by factors other than volcanic activity. The climate on Iztaccíhuatl ranges from temperate to cold and very cold; the mean temperature of the coldest month ranges from −2 to 18°C, according to elevation, with a decrease of 0.68°C per 100 m (Jasso-Flores et al., 2019). Mean annual precipitation is between 659.3 and 1,186 mm, concentrated during June to October (Comisión Nacional de Áreas Naturales Protegidas [CONANP], 2013).
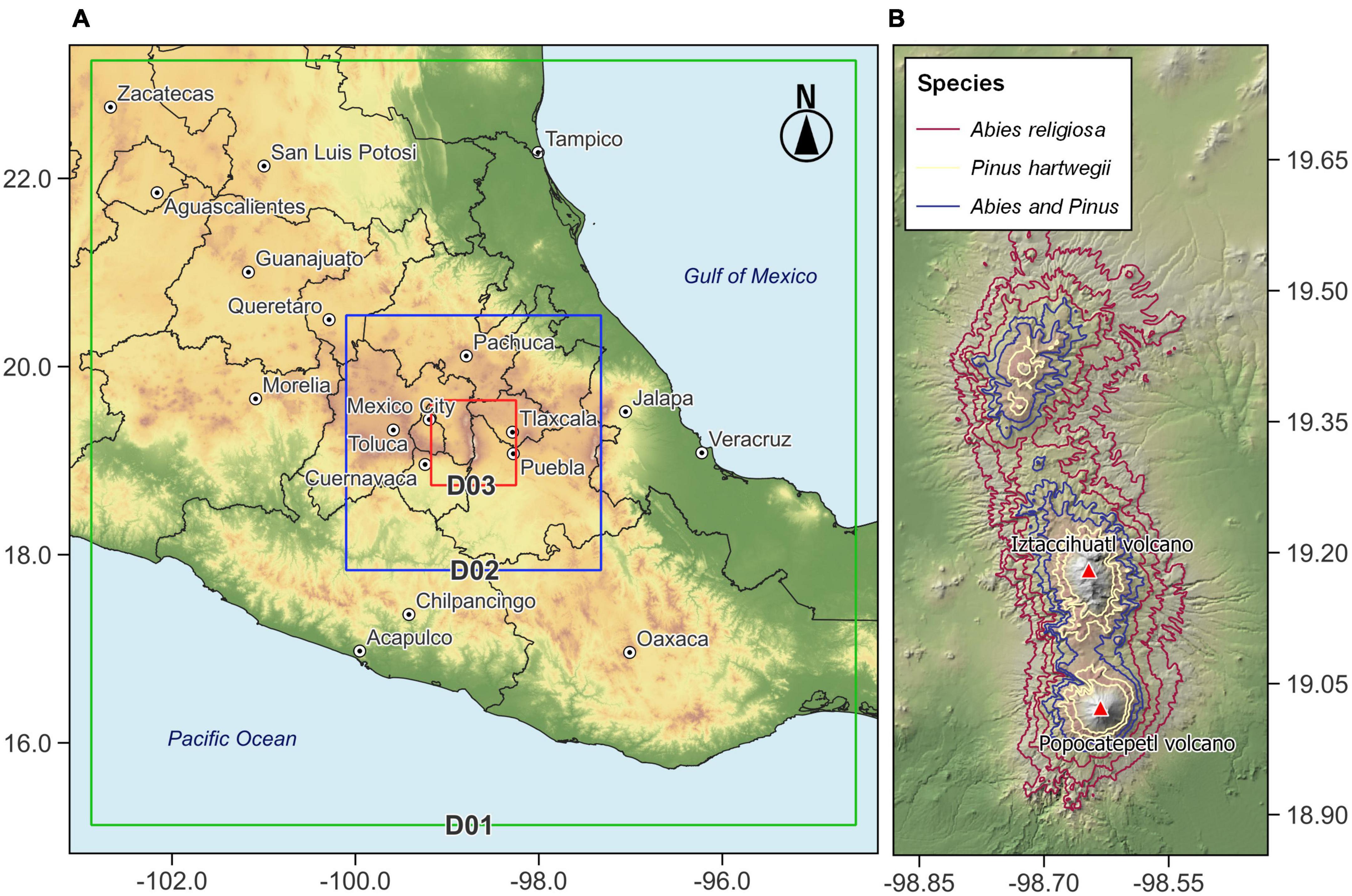
Figure 1. Map of the study area showing (A) spatial configuration of the domains D01, D02, and D03 used in the simulation with WRF-ARW and (B) distribution of Abies religiosa and Pinus hartwegii.
The vegetation in the area is mainly coniferous forests with the dominant species differing according to altitude. Abies religiosa (Kunth) Schltdl. and Cham., Pinaceae (hereafter referred to as Ar) is dominant between 3,000 and 3,700 m a.s.l. Pinus hartwegii Lindl., Pinaceae (hereafter referred to as Ph) is dominant between 3,400 and 4,000 m a.s.l., forming the tree line. Above 3,500 m and up to 4,350 m a.s.l., alpine grassland is the dominant vegetation. Ar trees can reach 40 m in height and sometimes up to 60 m, while Ph trees can reach 25 m in height (Espinosa-Garduño, 2005; Comisión Nacional de Áreas Naturales Protegidas [CONANP], 2013; Jasso-Flores et al., 2019). Both species have seeds with wings that favor wind dispersal (anemochory). In this context, the term diaspore refers to the wing and the seed together, while the term seed excludes the wing. Diaspore dispersal can happen from January to June, with a peak from March to April for Ar, and from February to March for Ph, with a biennial seed production seed production or longer (Benítez-Ortega, 2014; Arriola-Padilla et al., 2015).
2.2. Traits related to dispersal
We measured 18 seed traits in 50 diaspores for each species (Figure 2 and Table 1). Area, circularity, and linear dimensions were measured with ImageJ version 1.53. We evaluated the correlation of the seed traits and performed a principal component analysis (PCA). To represent the variation of the data, we used the first two components, which together accounted for 82.2% of the variation. R version 4.2.0. was used for all statistical analyzes.
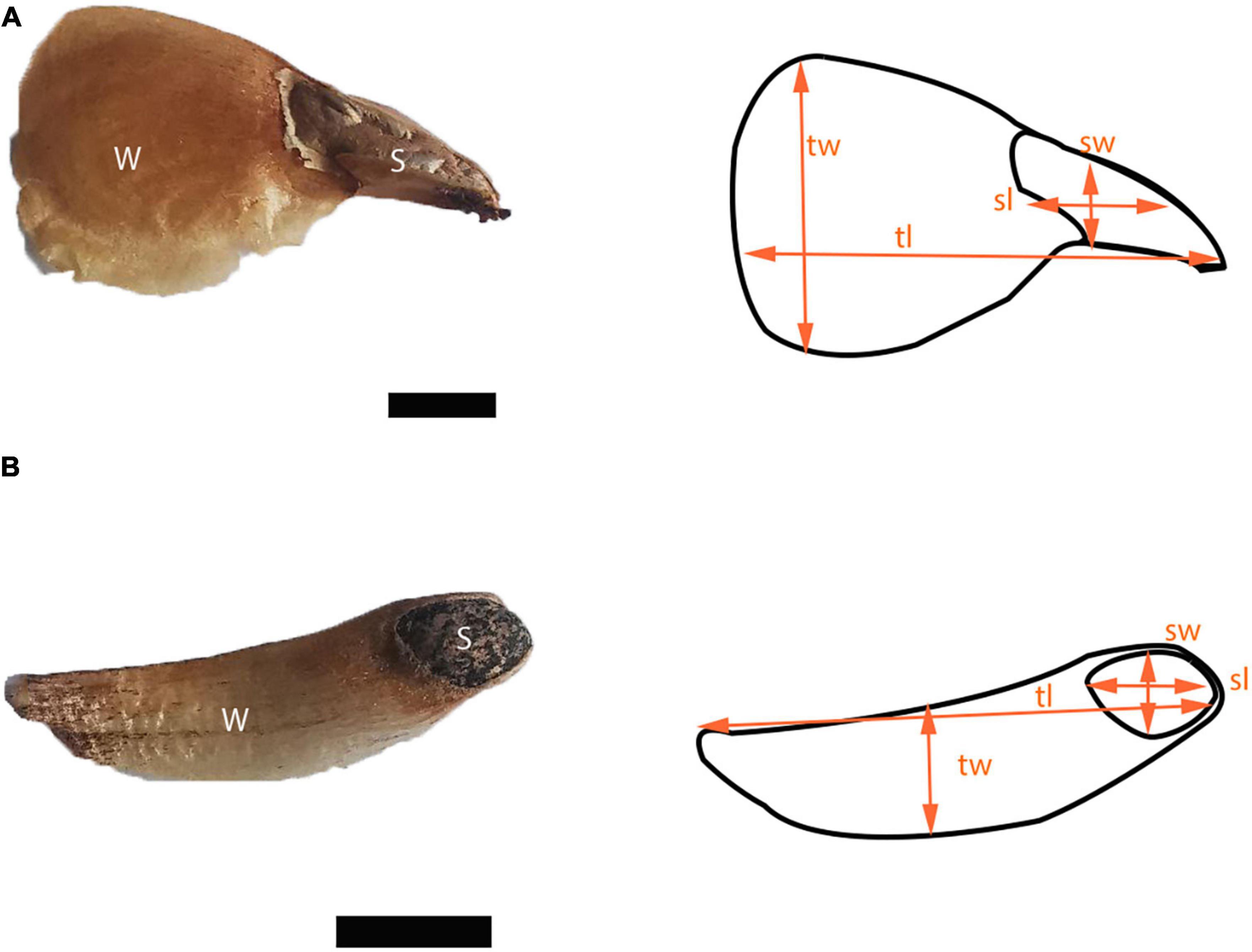
Figure 2. Diaspores of (A) Abies religiosa and (B) Pinus hartwegii. For both diaspores seed (s) and wing (w) are pointed out, and some linear dimensions like seed width (sw), seed length (sl), total width (tw), and total length (tl) are indicated schematically. Scale = 5 mm.
2.3. Biophysical traits
To evaluate the falling time and dispersal distance of the diaspores, we used a floor fan (Mytek 3348; 18 inches, 3 speed levels) to produce different horizontal wind speeds (1, 3, and 5 m/s) that we checked with an anemometer (Gain Express Ane-272) at the point where diaspores were released. The diaspores were released at a height of 1.5 m from the floor and 1 m from the fan. The dispersal distance was the horizontal distance traveled by the diaspore from its release point to the point where it touched the ground, not including subsequent displacements by sliding. According to the dispersal distance of each diaspore, we calculated the terminal velocity as the speed a diaspore reached when it was moving. Diaspore falling time, the time that elapsed between seed release and contact with the ground, was measured at different seed release heights (1, 2, and 3 m) and in the absence of a horizontal wind flow. For the three wind velocities and the three seed release heights, we performed 50 replicates for each species. To compare the terminal velocity and falling time by wind speed, release height, and species, we used two-way ANOVA and Tukey’s tests. Additionally, we tested the data for normality and homoscedasticity between groups. All statistical analyses were performed considering a significance of 0.05.
2.4. Configuration of the WRF-ARW model
We used the WRF model (Skamarock et al., 2019) with the ARW dynamic core version 3.8.1 to simulate weather conditions, including wind patterns in the Iztaccíhuatl–Popocatépetl region. We defined a 3-year period with no significant extreme events (2017, 2018, and 2019) to capture normal weather conditions in the area. To simulate the seasonal variation in wind patterns, we used the first week of each month of seed dispersal (January to June). Total simulation time was 3,024 h, iterated to consider 168-h periods, i.e., 7 days of simulation per numerical experiment.
The spatial configuration of the model defined a parent domain (D01) and two nested domains (D02 and D03) with a spatial resolution of 9, 3, and 1 km, respectively (Figure 1). This domain configuration seeks to increase the resolution for in D03 and represent, in the best possible way, the local processes and wind patterns, which are significantly influence by topography. D01 used a grid of 100 × 100 points, D02 88 × 88 points, and D03 76 × 76 points. The centroid of all spatial domains corresponds to coordinates 19.173°N and −98.635°W and represents the location of the highest point of the Iztaccíhuatl volcano. Finally, we defined 34 hybrid levels vertically, from 1,000 to 100 hPa, which supplied information across the entire atmospheric column and not only values on the surface.
The numerical models were initialized with NCEP GDAS/FNL 0.25 Degree Global Tropospheric Analyses and Forecast Grids (ds083.3) reanalysis data (National Centers for Environmental Prediction/National Weather Service/NOAA/U.S. Department of Commerce, 2015), which have a one-quarter degree (0.25°) spatial resolution and a 6-h time step. Physical parameterizations were based on previous research into the use of the WRF-ARW model in complex orographic regions of Mexico (Méndez Pérez et al., 2016; León-Cruz et al., 2019). In this regard, we selected the WRF Single-Moment 5-class scheme for microphysics, the RRTM scheme for long-wave radiation, the Dudhia scheme for short-wave radiation, Kain-Fritsch for cumulus parameterization, and the Yonsei University scheme (YSU) for the planetary boundary layer (Dudhia, 1989; Mlawer et al., 1997; Hong et al., 2004, 2006; Kain, 2004). The wind was corrected for topography (topo_wind) for the three domains in order to improve the representativeness of this variable in the study area (Jiménez et al., 2012).
From the model outputs, we extracted the wind speed and direction on the surface and interpolated and calculated the values at 50 m above ground level, as well as the vertical component. These variables were written in separate netCDF files in 6, 3, and 1 h time steps for D01, D02, and D03, respectively. The hourly data allowed us to represent the frequencies of the wind values over the simulated period.
2.5. Simulating dispersal events
We simulated dispersal events during a period from January to June. The simulations considered the following components: seed release height according to the elevation gradient and the vertical structure of Ar and Ph forests; the results of the WRF-ARW model; empirical results of dispersal trajectory to estimate falling time and terminal velocity; a mechanistic displacement equation; and wind direction to evaluate the probability of altitudinal dispersal.
For the vertical structure, we proposed a probability matrix including species, altitude, and six height ranges for reproductive trees (2 to 5 m, 6 to 10 m, 11 to 15 m, 16 to 20 m, 21 to 25 m, and 26 to 30 m). Probability was calculated for each of seven altitudinal floors (3,000—3,200 m a.s.l.; 3,201—3,400 m a.s.l.; 3,401—3,600 m a.s.l.; 3,601—3,600 m a.s.l.; 3,601—3,800 m a.s.l.; 3,801—4,000 m a.s.l.) according to the density of trees for the different ranges reported in the literature (Hoch and Körner, 2003; Iglesias-Andreu and Tivo-Fernández, 2006; Cuevas-Guzmán et al., 2011; Murrieta-Hernández et al., 2014) (Additional file).
Simulated dispersal trajectories consider multiple elements: wind speed data generated by the WRF-ARW model, tree height generated by the first component, and linear models for the biophysical traits of each species. Linear models for terminal velocity and falling time used the following equations:
where Vt is the terminal velocity of the diaspore during dispersal, Vv is horizontal wind speed, Tc is time to fall, h is tree height, β0 is the intercept of the respective model, β1 is the slope coefficient and e is the species (quantitative variable).
A linear model was generated for each dispersal simulation based on a random selection of an empirical dispersal trajectory. Each model considers an outcome of displacement or falling time for each experimental wind speed (1, 3, and 5 m/s) or release height (1, 2, and 3 m). If the slope coefficient was negative the model was discarded, and a new linear model was generated, thereby minimizing negative trajectories. When a linear model was selected, it was used to extrapolate terminal velocity and falling time based on the wind speed and height data provided by the WRF-ARW model. We calculated the dispersal distance for each mesh point in D03 matching the distribution of Ar and Ph (606 points for Ar and 365 points for Ph). For each point, we randomly selected one of the outcomes of the temporal iterations of wind speed of the WRF-ARW model. Before calculating the dispersal distance, the simulation selects a dispersal scenario based on the value of the vertical wind component and the height of the reproductive tree. If tree height exceeds 20 m and an ascending wind flow of >0.2 m/s occurs, this is considered an “UP” scenario in which the diaspore interacts with the horizontal wind component at 50 m; otherwise, it is a “NORMAL” scenario in which the diaspore interacts with horizontal wind at 10 m. Once the dispersal scenario had been selected, the dispersal distance was calculated as the product of the terminal velocity and the time to fall:
where Dd is the seed dispersal distance, Vt is the extrapolation of the seed terminal velocity according to the horizontal wind corresponding to the dispersal scenario, and Tc is the extrapolation of the time taken for the seed to reach the ground according to the height of the reproductive tree.
Each dispersal simulation includes the horizontal wind direction selected according to the dispersal scenario. For each simulation, we compared the wind direction with an ideal range of wind directions that would favor altitudinal dispersal according to the location of the reproductive tree. The ideal direction is an interval of possible dispersal directions that promote the arrival of the seed to the next altitudinal floor. To calculate it, we established a point located at the highest altitude of our study site and drew lines that originated from each mesh point of the grid; then for each line we calculated the angle and added a range to estimate the ideal direction interval. Hence, our model simulates individual dispersal events, yielding, as outcome variables, the dispersal distance, and the dispersal direction. The outcome variables indicate whether the dispersal event could enable the diaspore to reach a higher altitude (altitudinal dispersal).
The altitudinal dispersal was evaluated by running 100 iterations of the model. We set samples of 10 trees for each mesh point. We used the 3,024 simulation hours of the WRF-ARW model to make random selections of each mesh point, which were then evaluated to choose the dispersal scenario and carry out extrapolations to calculate the dispersal distance. Finally, we set a range of ± 60° from the ideal direction to evaluate the altitudinal dispersal.
Additionally, we ran the model monthly favoring the “UP” scenarios, fixing the values of the first component, and adjusting a unique model for each species to simulate its dispersal distance. Regardless of the altitudinal floor, we set Ar height to 30 m and Ph height to 20 m. We selected wind values associated with the maximum monthly value of the vertical component for each mesh point and the linear models with the maximum slope. For these simulations, we set a range of ± 40° from the ideal direction to evaluate the altitudinal dispersal.
3. Results
3.1. Morphological traits
Pinus hartwegii and Ar diaspores overlapped in their linear dimensions, area, and mass, but they differed in the PCA. This analysis separated the two species according to their main morphological traits (Figure 3). The components 1 and 2 accounted for 68.7 and 13.5% of the total variation. The variables that had the highest contribution in component 1 were the ratio of wing size and seed size to total diaspore area, whereas for component 2, the variables with the highest contributions were the ratio of diaspore and wing area to mass. Distance was the variable with the least contribution in the two components (Additional file). Ar had higher values of length, mass, circularity, and ratio of total area to seed area, while Ph had a longer shape and a proportionally larger wing area (Table 2). The area of Ar diaspores was twice that of Ph, but Ph relative wing size was double that of Ar. The Total Area:Seed Area (ta:m) relation for Ph was twice that for Ar, whose diaspores were on average four times heavier than those of Ph (Table 2).
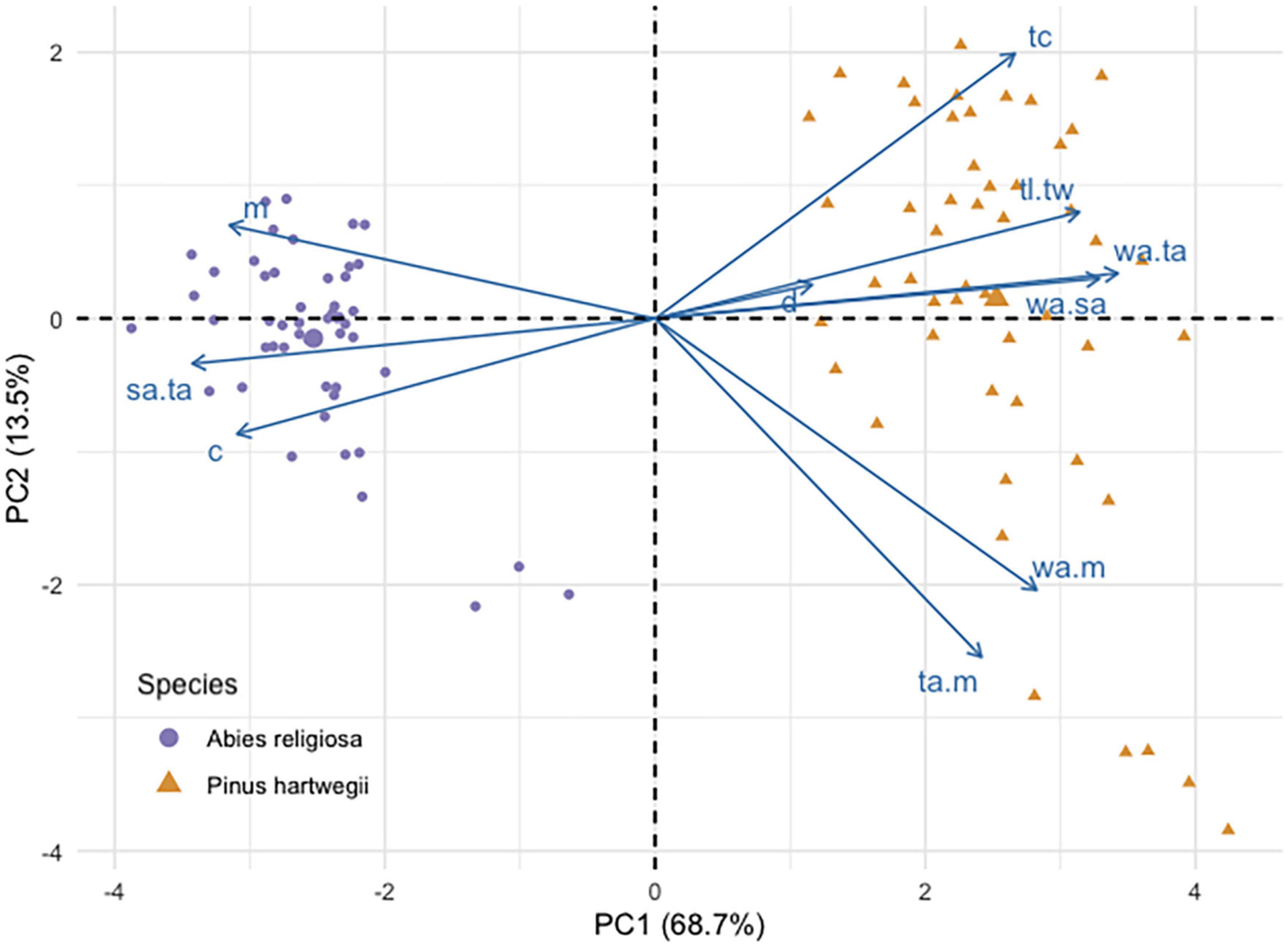
Figure 3. Principal component analysis biplot of seed traits of Abies religiosa and Pinus hartwegii.
The horizontal dispersal distance of Ar almost completely overlapped that of Ph (refer to the Attachments); its distribution was bimodal for each species, with a prevailing displacement of <50 cm (Additional file), and its correlation coefficient with the other traits was low. Falling time (tc) for Ph was almost twice that of Ar; it was negatively correlated with circularity (r = −0.745), seed length (r = −0.815), and seed area (r = −0.809), and to a lesser extent with mass (r = −0.524).
a: Cuevas-Guzmán et al. (2011), b: López-López (unpublished), c: Ortiz-Bibian et al. (2019), d: Hoch and Körner (2003), e: Iglesias-Andreu and Tivo-Fernández (2006), f: Murrieta-Hernández et al. (2014), g: Alfaro-Ramírez et al. (2007), h: Iglesias et al. (2012).
3.2. Wind patterns
The model outputs showed a mean horizontal wind speed of 2.81 m/s, with a peak value of 17.5 m/s during the entire period analyzed (Additional file). In D03, the wind pattern varied between valleys and mountains, with the most intense winds prevailing on mountain slopes (Figure 4). Diurnal variations reflected the differential solar radiation throughout the day, i.e., anabatic, and katabatic winds that are typical of complex orographic regions.
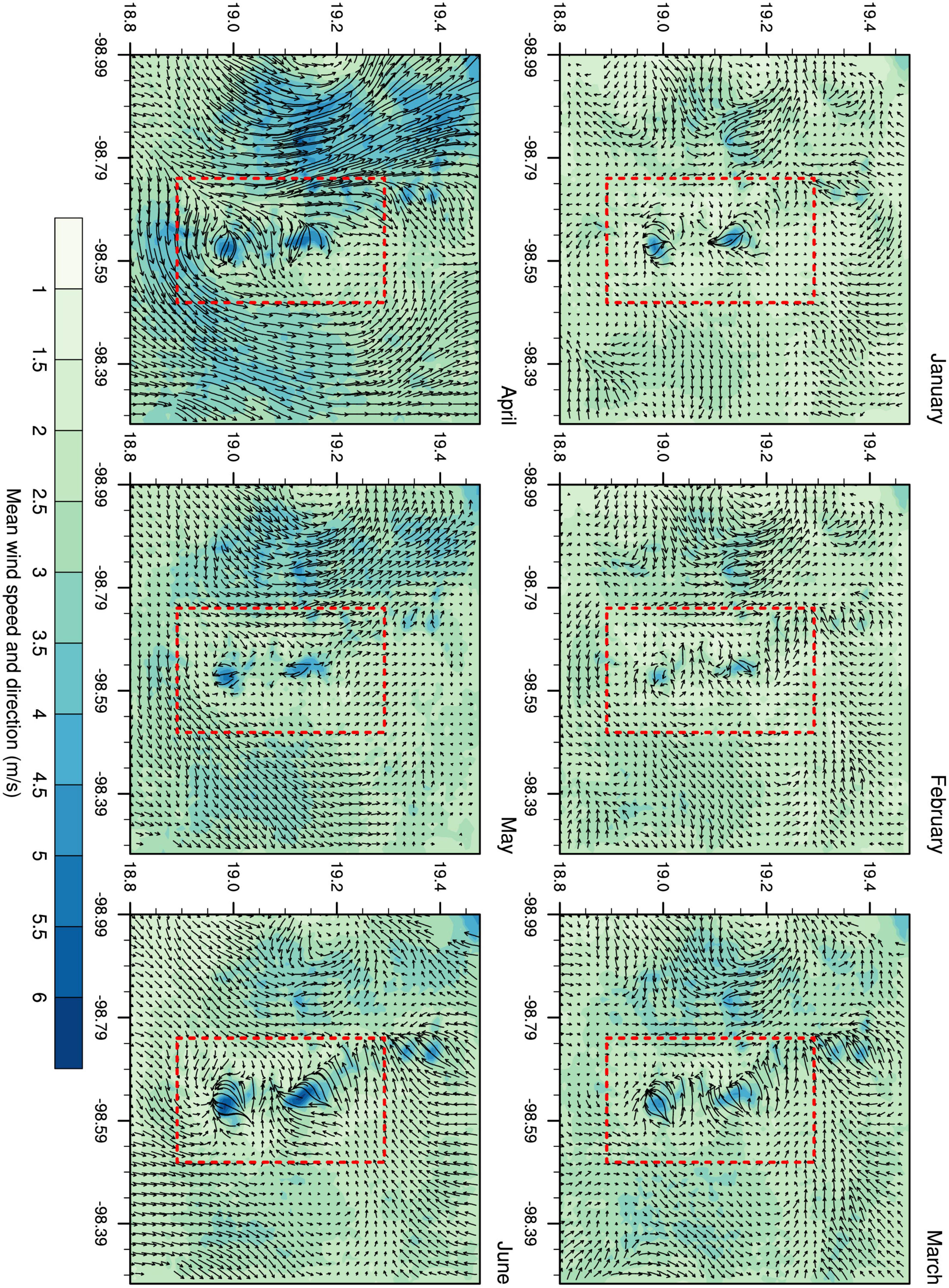
Figure 4. Mean wind speed and direction for D03. The Iztaccíhuatl-Popocatepetl volcanic area is marked in red.
In the mountain area, the model suggested differences between wind patterns at 10 m and 50 m height. The horizontal wind component at 50 m was significantly larger than at 10 m (t = −355.59, df = 4,222,373, p-value < 2.2e-16); at these two strata, horizontal wind speed tended to increase slightly with altitude (Figure 5). Mean horizontal wind speed (at both 10 and 50 m) was higher during the first months of the year, peaking in April and then decreasing gradually over time; however, peak values were indicated in May. For each month except April, the frequency of wind direction was evenly distributed, with a slight pre-dominance of the E and NE directions; for April, SW and S directions prevailed. For the maximum horizontal wind speed, the pre-dominant directions were SW, NE, W, and E (Figure 5).
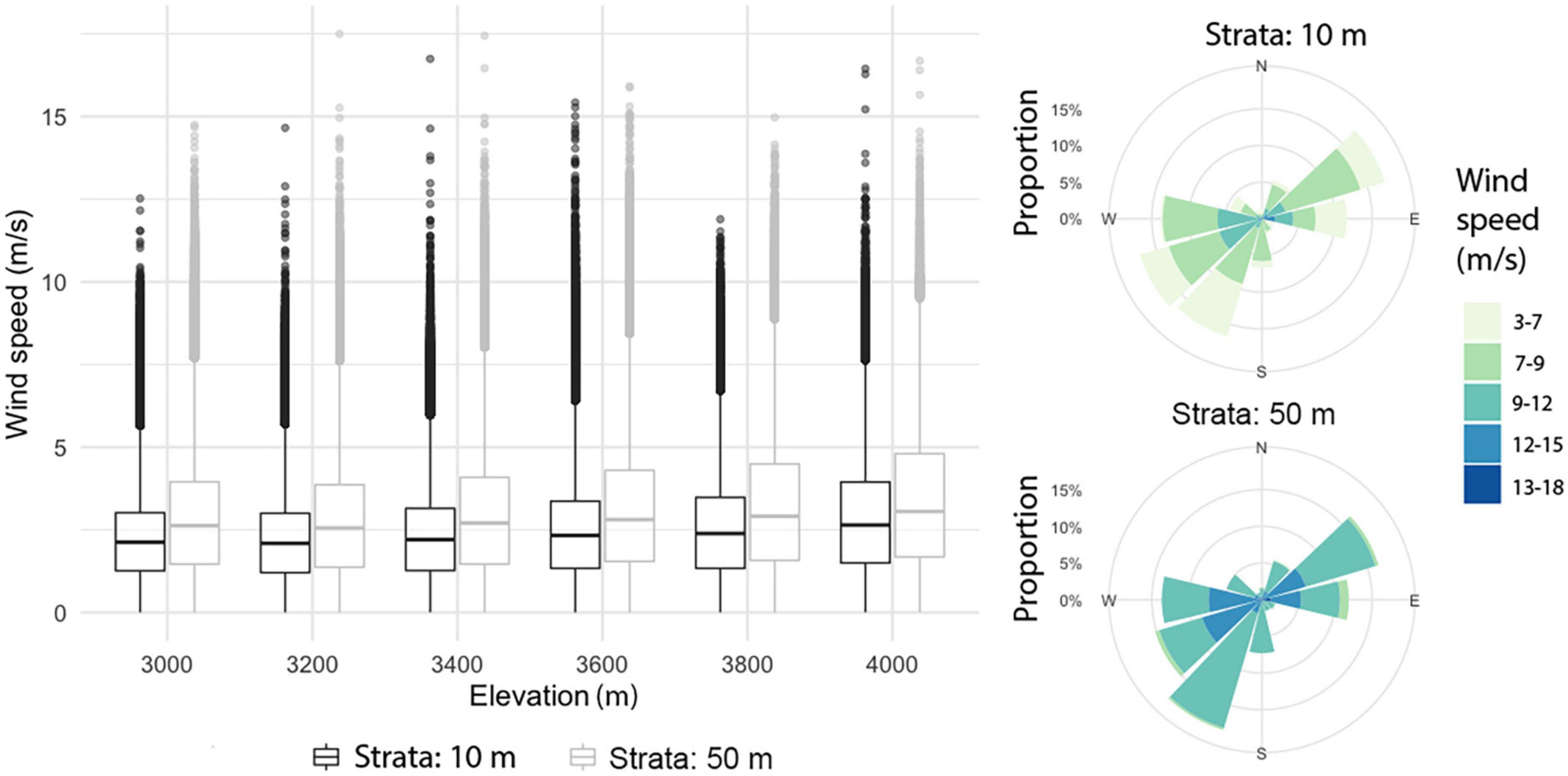
Figure 5. Boxplots of horizontal wind by elevation at 10 and 50 m above the surface (left). Compass roses for the highest wind speeds at 10 m and 50 m above the surface (right).
Horizontal wind speed had a mean value of 0.016 m/s, with a minimum of 0.9 m/s and a maximum of 4.27 m/s, with descending flows pre-dominating, represented by negative values. Values tended to become increasingly extreme with altitude. Both the highest monthly mean speed (0.004 m/s) and the highest maximum (4.28 m/s) occurred in June.
3.3. Probability of dispersal at higher altitude
Among 594,000 dispersal events simulated, dispersal distance ranged from 0 to 23.3 m, with a mean of 2.24 m (Figure 6); distance (mean, maximum) was further for Ar (2.2 m, 23.3 m) than for Ph (1.2 m, 9.94 m). The above-canopy (“UP”) dispersal scenario occurred in only 11% of the dispersal events for Ar and 16% for Ph. Adequate wind direction to promote altitudinal displacement of diaspores occurred in approximately one-fourth of the simulated dispersal events in either species. Only 7% of the dispersal events for Ar and 3% for Ph showed potential for the altitudinal dispersal of diaspores; in each species, such events were near the upper limit of its present distribution. For individuals near this upper limit, the mean probability of an altitudinal dispersal event was 26% for each species, with points reaching probabilities of 48% for Ar and 42% for Ph (Figure 7). Of the events with altitudinal dispersal potential, only 24% for Ar and 45% for Ph corresponded to an above-canopy (“UP”) dispersal scenario.
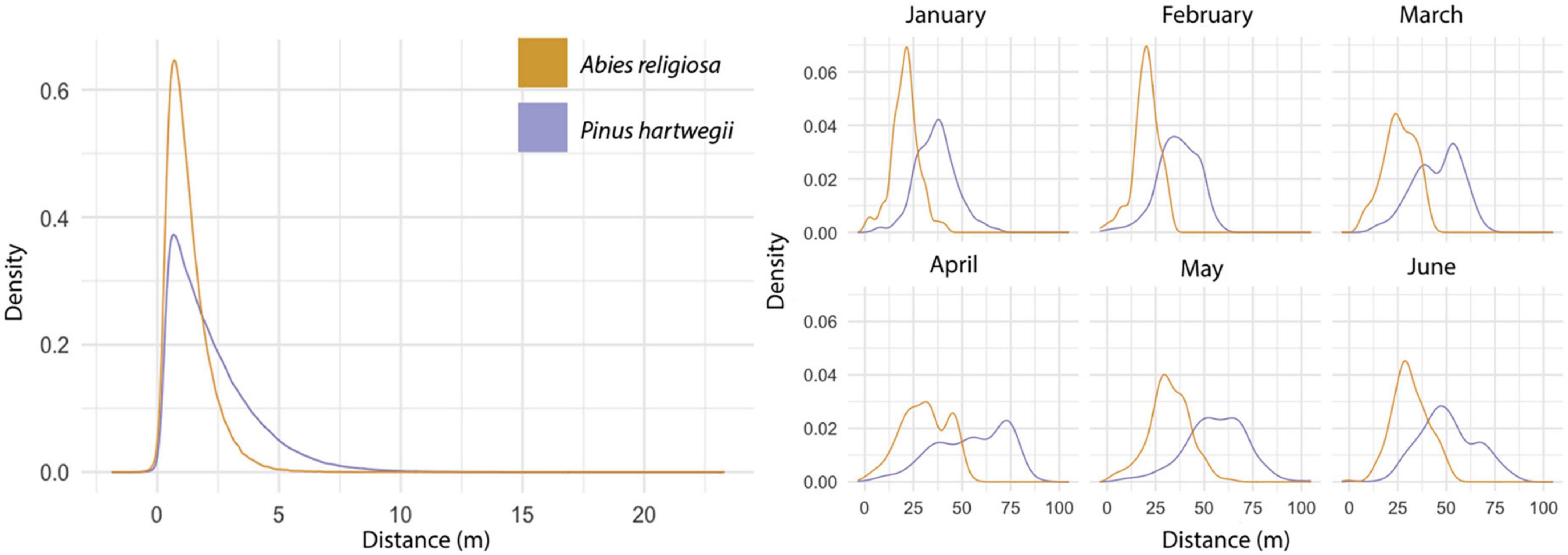
Figure 6. Simulated dispersal distance. Results of the dispersal distance of the simulations iterated 100 times (left). Results of the dispersal distance of simulations with maximum wind and height values by month (right). Colors correspond to each species.
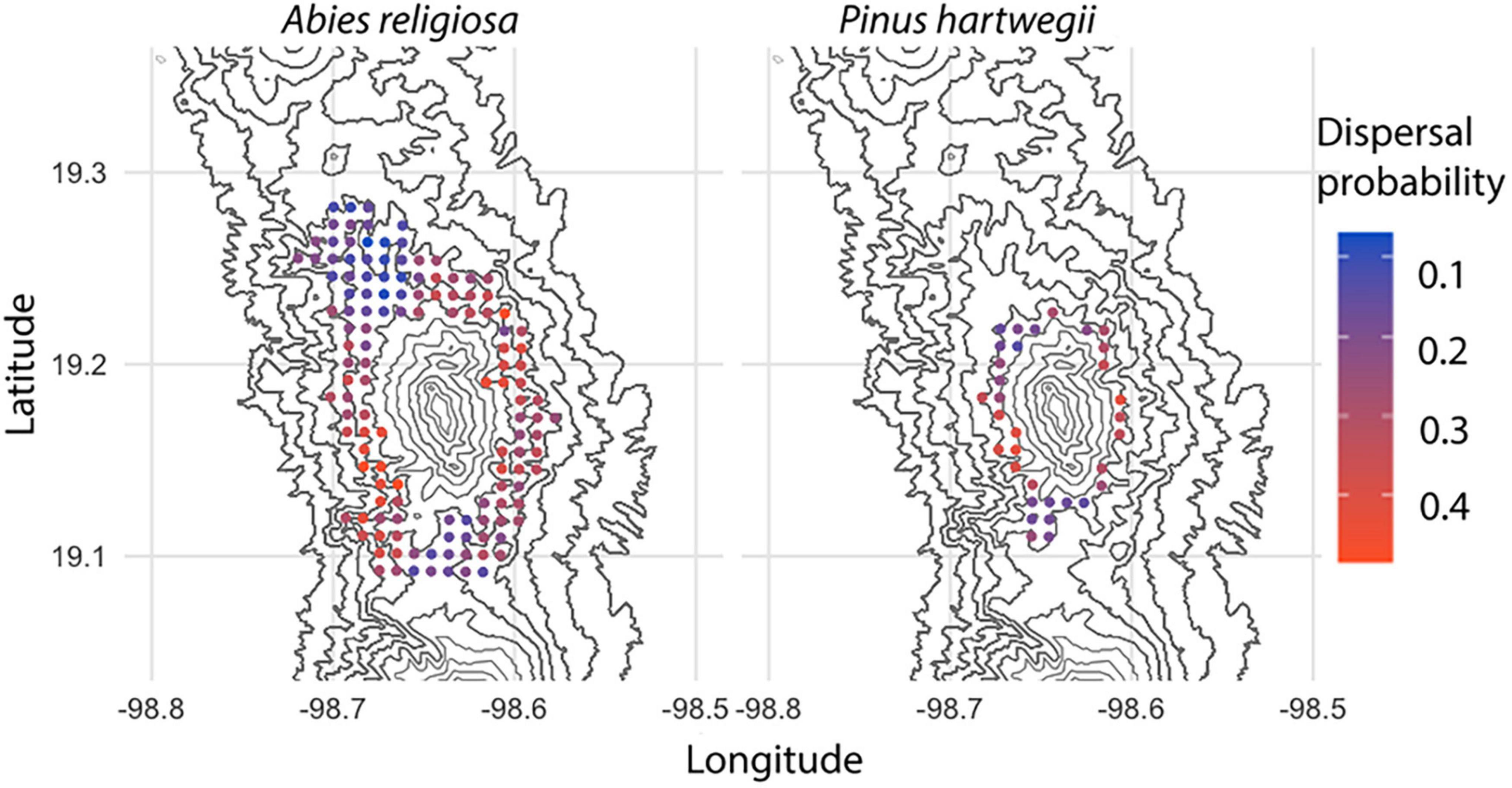
Figure 7. Probability of altitudinal dispersal for Abies religiosa and Pinus hartwegii. Contour lines of the study area are indicated and the probability of altitudinal dispersal per point grid correspond to a simulation with 100 iterations.
In 3,564 simulations to fix the maximum values for the vertical wind component, dispersal distances (mean, maximum) tended to be further: 46.6 m, 105 m for Ar and 26.8 m, 64.4 m for Ph (Figure 6). The greatest displacement was recorded at an altitude between 3,600 and 3,800 m a.s.l. in May for either species. Note that when the model was configured with the highest vertical wind values and the maximum tree heights, all dispersal scenarios were classified as “UP,” but there were dispersal distances of 0 m. Although a wind direction suitable for altitudinal dispersal occurred in ∼40% of events for each species, only 20% of events for Ar and 9% for Ph showed potential for altitudinal dispersal. Altitudinal dispersal events were identified near the upper limit of the present distribution of each species. For individuals near this limit, the probability of an altitudinal dispersal event was ∼55% for Ar and ∼65% for Ph. The highest probability of altitudinal dispersal for Ar was 63% in March, and for Ph it was 69% in January.
4. Discussion
4.1. Traits related to dispersal
Variation in diaspore morphological traits can have significant impacts on wind dispersal distributions when comparing species (Kim et al., 2022). Our results suggest that even though the seeds of Abies religiosa and Pinus hartwegii have similar appendages to assist in wind dispersal, the two species differ in their dispersal potential. But even though we hypothesize that Ph distance of dispersal would be larger because of its smaller and lighter seeds, simulations showed a larger dispersal for Ar. Dispersal capacity depends on the combination of seed traits and its interaction with wind patterns (Zhu et al., 2016). The greater displacement of Ar diaspores may be attributable to other variables as seed dimensions and shape, that were more important drivers for dispersal than mass and, therefore modify the dispersal capacity.
The relation between seed traits had been used to explain dispersal capacity. For example, low mass:area ratios may enhanced the aerodynamic transport of diaspores by increasing drag and hence the travel time through the air (Nathan et al., 2011). Similarly, wind carries Parthenium hysterophorus diaspores further when the achene:pappus size ratio decreases (Mao et al., 2022); and in a wind-tunnel study that included 14 anemochoric species with winged seeds suggested that the larger ratio mass:wing area was the best predictor of secondary dispersal capacity through lift-off from the ground (Liang et al., 2020). In our study, the ratios of wing area:diaspore area and of wing area:mass were the traits in which the two species differed most. Ph had smaller seeds and larger wings relative to seed size than did Ar, suggesting that Ph would display a slight advantage in its movement capacity. However, the greater dispersal distances indicated for Ar in the simulation suggest that absolute wing size is more important than the relative proportions of wing and seed.
For other North American tree species, it has been suggested that the variation in tree height has a significant impact on dispersal distance (Nathan et al., 2011). The height range of our studied species prevented the inclusion of this in our experiments, but we included it in the model. In terms of diaspore release height, Ar has a slight advantage over Ph, since Ar individuals can reach twice the height (Table 2). However, even when equivalent release heights were set for the two species, Ar showed some advantage in diaspore displacement. This indicates that, regardless of height, diaspore morphology is important for dispersal, and that dispersal strategies may differ according to their interaction with wind patterns. This finding is consistent with the experimental results obtained by Jongejans and Telenius (2001), who observed that the height of anemochoric species offers an alternative explanation to the greater displacement that characterizes them, so that the height of diaspore release may be a more important factor for dispersal than seed morphology.
Even though our results address differences in the dispersal capacity of our species, it is important to highlight that our empirical data of seed width, length, and mass exceeded those recorded for other populations in central Mexico (Table 2), which suggests that the morphological variation of diaspores may be wider than has literature been noted. Also, in both species, seed mass showed a multimodal distribution, reflecting the morphological variation of diaspores in the two species. Therefore, we suggest considering larger samples of seeds with different populations of origin to continue studying the role of morphological variations in the dispersal capacity of both species.
4.2. The role of wind in dispersal
The role of the wind on seed dispersal distance resulting in different vertical and horizontal components of air flow including turbulence, and complex updrafts that potentially increasing wind dispersal within a canopy (Kim et al., 2022). Contrary to what we considered in our second hypothesis, the elevation by itself was not determinant in the frequency of LDD events, but various factors participated such as the vertical component and the horizontal component of the wind speed, the direction of the wind and the site from which the seeds were released. A positive value of the vertical wind component can favor dispersal above the forest canopy (”UP” scenario). In the UP scenario the diaspore interacted with stronger horizontal wind currents that favored longer displacements than in the “Normal” scenario (dispersal within the canopy). However, even these “UP” dispersal scenarios yielded very short or even nil diaspore displacement, due to the interaction with low-speed horizontal wind even at 50 m above the ground. That is, even above the canopy, diaspores may interact with very low wind speeds that would not lead to great displacement. This finding is like the experimental results obtained by Qin et al. (2022), who observed that low horizontal wind speed will decrease diaspore dispersal distance, but an updraft or vertical wind speed can increase dispersal time, thereby increasing diaspore dispersal distance. This demonstrates the importance of horizontal wind speed since it directly influences the magnitude of diaspore displacement; however, our simulations show that diaspore displacement will also depend on the attributes of each species. For instance, Ph distributed at higher altitudes than Ar, has contact with significantly greater horizontal wind speeds, and nonetheless its diaspores showed a lower mean displacement than in Ar diaspores. The importance of vertical wind has been explored in the model comparison by Soons et al. (2004), showing that autocorrelated turbulent fluctuations in vertical wind speed are the key mechanism for LDD.
Our model prediction of dispersal events with a mean displacement of 1.2 to 46.6 m assigned a low displacement potential to the species studied here in comparison with other models. In a seedling recruitment model, Ribbens et al. (1994) proposed mean dispersal distances of 8 m for Acer rubrum, 9 m for A. saccharum, 15 m for Pinus strobus, 16 m for Fraxinus americana, and >66 m for Betula alleghaniensis, whereas Ph and Ar here attained a mean dispersal distance of <5 m. The maximum simulated displacement here, 105 m, is also lower than the displacement predicted by other models reported in the literature. For example, the mechanistic model for Pinus halepensis predicted that 3.5% of the dispersal events would exceed 100 m (Nathan et al., 2001); another study for Pinus taeda estimated that 70 seeds/ha could travel distances of >1 km (Williams et al., 2006). Despite the differences in the magnitude of displacements, for all models, including ours, the frequency of dispersal events reaching a displacement of at least 100 m is minimal. It should also be emphasized that the input data for wind patterns come from an atmospheric model that contemplates orographic complexity, so the presence of mountains has a significant impact on the generation of strong wind episodes and turbulence on the surface and the first meters above it due to friction. Also, Ar and Ph have a different density and canopy structure (Jasso-Flores et al., 2019), that will cause differences in turbulence, which may further affect the distance of seed wind dispersal.
In addition to wind speed, the timing between seed release and maximum wind speed events should also be considered. The dispersal simulations conducted by Heydel et al. (2015) found that species of Asteraceae, Populus and Salix showed a high potential for LDD; however, in natural conditions, these species lacked synchronization between seed release and higher wind speed events, so LDD would not happen in nature. The months for which we recorded higher wind speeds (April and May) may not coincide with the diaspore dispersal peak of Ar and Ph. Hence, the reproductive phenology of both species should be studied to determine accurate patterns in the timing of diaspore release events, considering particularly the frequency of years of high seed yield. Some studies suggest that the diaspore release season spans from March to April for Ar and from February to March for Ph (Benítez-Ortega, 2014; Arriola-Padilla et al., 2015). If so, there would be a partial temporal coincidence of higher wind speed events with the dispersal of Ar diaspores, while no such coincidence occurs for Ph. However, it has also been suggested that both species can release their seeds for longer periods of time, thus coinciding with the months in which maximum wind speed would occur.
Dispersal to a higher altitude depends on wind direction as well as speed. According to our model, only 40% of all dispersal events that may potentially favor altitudinal migration had a wind direction that might favor such migration. However, wind direction is difficult to model since it is highly variable over very short periods of time, ultimately affecting the trajectory of the diaspore. Therefore, we suggest further exploring the implications of wind direction in future dispersal models.
4.3. Dispersal and altitudinal migration
According to our results, the prevailing dispersal events for Ar and Ph involve diaspores that fall near the reproductive plant. Short-distance dispersal is a common phenomenon of anemochoric diaspores in closed forests because of obstacles such as branches, trunks, and even other seeds. Studying Betula pendula forests, Liu and Evans (2021) observed that the average dispersal distance in an open area is 65 m but decreases to 38 m in a closed area. However, although the possibility of LDD is low, the number of LDD events may increase depending on the number of seeds produced. For P. halepensis, although a low frequency of LDD was predicted, the large seed production suggests that even a small fraction of this large production would result in a considerable number of seeds being dispersed far from the parent tree (Nathan et al., 2001). The seed production reported for Ar ranges between 235 and 574 seeds per cone, while Ph produces between 187 and 199 seeds per cone on the western slope of Popocatépetl (Madrigal Sánchez, 1967; Landa et al., 2003). But it should also be considered that coniferous seed production varies between years, sites, and individual trees, with low seed production triggers as high temperatures and dry weather (Krannitz and Duralia, 2004). Therefore, the proportion of dispersal events that could reach a higher altitude depends on the changing total seed production, so it would be important to study the variations in seed yield, particularly between years of high and low seed production.
One of the main questions in evaluating dispersal events is to define the magnitude of a LDD event. One characteristic of LDD is that it crosses the boundaries of the distribution of a species (Jordano, 2017), so we can consider that any elevation dispersal event is a LDD regardless of the distance. In this way, the potential of LDD events increases toward the upper altitudinal limit of the distribution range of the species, while a distance further below reduces the potential for LDD and depends on the dispersal distance. According to the data derived from our model, the maximum dispersal distance for Ar is 105 m and for Ph is 64 m; therefore, only trees less than 105 m below the upper limit for Ar and 64 m below for Ph could produce diaspores for the altitudinal migration of each species. This may be an advantage for Ar as it implies that a higher number of trees could provide diaspores for migration. Another advantage for Ar is that its displacement is nearly twice that of Ph, so Ar may approach the altitudinal range of Ph, while Ph may advance more slowly toward a higher altitude. In this sense, and answering our third hypothesis, we could consider that the LDD events could only accelerate the migration of Ar. However, this must be contextualized and the density of the forests of the two species compared, as well as the differences between them in terms of reproductive phenology and seed production. Conditions that affect seeds and seedlings include abiotic factors and competition with the vegetation already present. The altitudinal migration of Ar is leading to interactions with Ph, while the altitudinal migration of Ph is advancing onto terrain dominated by alpine grasslands (Figure 8). The variety of potential responses for each species will be a key driver for the success of its altitudinal migration.
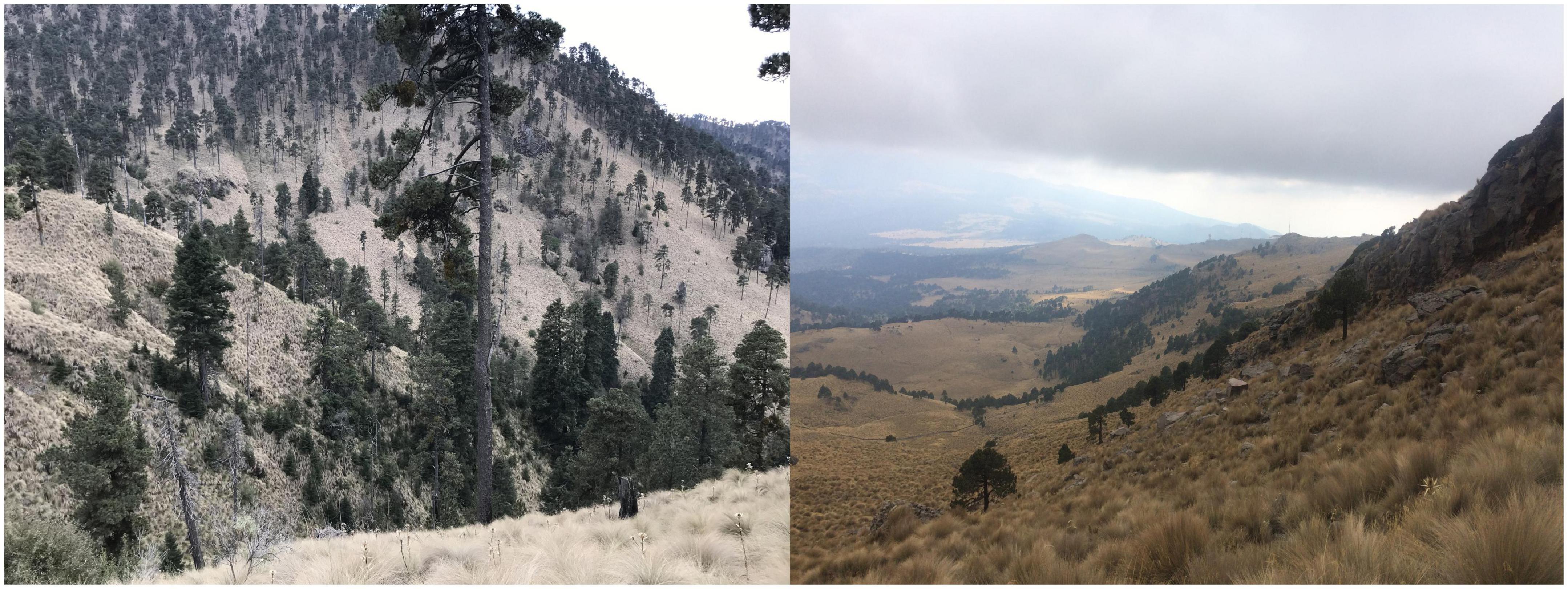
Figure 8. Valley of Alcalican, at approximately 3,800 MASL, where individuals of Abies religiosaaged 5 to 10 years are colonizing slopes currently dominated by Pinus hartwegii (left). A slope at 4,100 MASL showing some individuals of P. hartwegii that have migrated beyond the tree line (right).
Even when the proportion of LDD is low, they could favor altitudinal migration. To maximize the probability of altitudinal dispersal events high seed production, the release of seeds during the months with the highest wind speed and the adequate wind direction are required. However, there are some limitations to LDD for our species: (1) the periodicity of seed production is usually biennial, with seed production peaks that may require for longer times (Benítez-Ortega, 2014; Arriola-Padilla et al., 2015); (2) events of maximum wind speed are infrequent and it is common for the seed to find obstacles in its trajectory depending on the density of trees (Liu and Evans, 2021); and (3) the direction of the wind is a highly variable and could easily return the seed to the forest. Therefore, we would expect altitudinal migration to occur mostly slowly by gradually advancing, with few “jumps” accelerating the migration.
Abies religiosa and Ph are among the few trees able to grow under the climatic conditions of the high-mountain systems of the Neotropics (>3,000 m a.s.l.). The impact of the shift in environmental conditions on their regeneration dynamics will have repercussions on their altitudinal migration. The consequences of global warming on the reproduction of temperate species include a lag in reproductive phenology or changes in propagule production (Kreyling, 2010). Dendrological studies of the effects of temperature and precipitation on growth in P. hartwegii have indicated that environmental warming would have an adverse effect on the distribution of this species in the higher mountains of central and northern Mexico (Carlón Allende et al., 2021). Also notable is the differential effect that changes in soil moisture and temperature related to warming can have on the establishment of species such as Ph in the vicinity of the study region (Astudillo-Sánchez et al., 2019).
5. Conclusion
Modeling has suggested differences in the dispersal capacity of two species that may be related to their seed traits. Seed traits related to shape could be more informative for the dispersal capacity than only seed mass. We also highlight the importance of evaluating seed traits variability for each species, to approach pathways in the seed dispersal capacity. The frequency of LDD decreases with increasing distance below the upper altitudinal limit of the distribution range of each species, especially in the case of Ph. In addition to wind speed, wind direction determines whether the seed is dispersed at a higher altitude. However, the high variability of the wind direction requires future approaches to modeling this variable. The apparently greater dispersal potential of Ar may favor its advance onto the Ph terrain at a faster pace than the migration of Ph onto higher altitudes. However, it is important to consider other factors such as seed production, the density of the forest, germination, seedling development and interactions with other plants already present in the migration area. The results from this study can help explain the altitudinal migration in the complex terrain systems along the TMVB, where similar conditions are observed. The study area’s geographical location, which represents a transition zone between Neotropical and Nearctic conditions, provides valuable information poorly explored in the literature. Likewise, the results obtained can be helpful in future analysis contextualized in a warming scenario.
Data availability statement
The original contributions presented in this study are included in the article/Supplementary material, further inquiries can be directed to the corresponding author.
Author contributions
IG-V and LG: conception and design. IG-V and JL-C: analysis and interpretation. IG-V, JL-C, and LG: drafting and revising. All authors contributed to the article and approved the submitted version.
Funding
This work was funded by Mexico’s Consejo Nacional de Ciencia y Tecnologia (National Council of Science and Technology, CONACyT) graduate scholarship to the IG-V (Grant No. 762543) and, through the PAPIT-DGAPA project, IN206121, Interacción cambio climático-ecofisiología-variación ambiental en el desplazamiento y recolonización de especies arbóreas en paisajes de alta montaña: comprendiendo los mecanismos de la migración (Interaction between climate change-ecophysiology-environmental variation in the displacement and recolonization of tree species in high-mountain landscapes: Understanding the mechanisms of migration).
Acknowledgments
This article is part of the requirements for obtaining a Doctoral degree at the Posgrado en Ciencias Biológicas of the Universidad Nacional Autónoma de México (UNAM) of IG-V. María Elena Sánchez-Salazar translated the manuscript into English.
Conflict of interest
The authors declare that the research was conducted in the absence of any commercial or financial relationships that could be construed as a potential conflict of interest.
Publisher’s note
All claims expressed in this article are solely those of the authors and do not necessarily represent those of their affiliated organizations, or those of the publisher, the editors and the reviewers. Any product that may be evaluated in this article, or claim that may be made by its manufacturer, is not guaranteed or endorsed by the publisher.
Supplementary material
The Supplementary Material for this article can be found online at: https://www.frontiersin.org/articles/10.3389/fevo.2023.1150137/full#supplementary-material
Abbreviations
Ar, Abies religiosa; Ph, Pinus hartwegii; LDD, long distance dispersal; TMVB, trans-Mexican volcanic belt.
References
Alfaro-Ramírez, F. U., Arredondo-Moreno, J. T., Pérez-Suárez, M., and Endara-Agramont, Á. R. (2007). Ecotono del límite superior del bosque de Pinus hartwegii Lindl.: Estructura y límites altitudinales en el Nevado de Toluca, México. Rev. Chapingo Ser. Cienc. For. Ambiente 23, 261–273.
Arriola-Padilla, V., Flores, A., Hernández, A., Ojeda, T., Jacob, V., and Pola, C. (2015). Producción de planta de Abies religiosa (Kunth) Schltdl. & Cham. en vivero. México: Comisión Nacional Forestal.
Astudillo-Sánchez, C. C., Fowler, M. S., Villanueva-Díaz, J., Endara-Agramont, A. R., and Soria-Díaz, L. (2019). Recruitment and facilitation in Pinus hartwegii, a Mexican alpine treeline ecotone, with potential responses to climate warming. Trees 33, 1087–1100. doi: 10.1007/s00468-019-01844-3
Beckman, N. G., Aslan, C. E., Rogers, H. S., Kogan, O., Bronstein, J. L., Bullock, J. M., et al. (2020). Advancing an interdisciplinary framework to study seed dispersal ecology. AoB Plants 12:lz048. doi: 10.1093/aobpla/plz048
Benítez-Ortega, D. (2014). Proyecto de inversión para el establecimiento de un vivero tecnificado para la producción de plantas forestales en el municipio de Tlalmanalco, Estado de México. Tesis de Licenciatura. México: Universidad Nacional Autónoma de México.
Biondi, F. (2001). A 400-year tree-ring chronology from the tropical treeline of North America. Ambio 30, 162–166. doi: 10.1579/0044-7447-30.3.162
Boisvert-Marsh, L., Périé, C., and de Blois, S. (2014). Shifting with climate? Evidence for recent changes in tree species distribution at high latitudes. Ecosphere 5, 1–33. doi: 10.1890/ES14-00111.1
Carlón Allende, T., Villanueva Díaz, J., Soto Castro, G., Mendoza, M. E., and Macías, J. L. (2021). Tree rings as indicators of climatic variation in the Trans-Mexican Volcanic Belt, central Mexico. Ecol. Indic. 120:106920. doi: 10.1016/j.ecolind.2020.106920
Clark, J. S., Fastie, C., Hurtt, G., Jackson, S. T., Johnson, C., King, G. A., et al. (1998). Reid’s paradox of rapid plant migration dispersal theory and interpretation of paleoecological records. BioScience 48, 13–24. doi: 10.2307/1313224
Comisión Nacional de Áreas Naturales Protegidas [CONANP] (2013). Programa de Manejo Parque Nacional Iztaccíhuatl Popocatépetl. México: Comisión Nacional de Áreas Naturales Protegidas.
Cousens, R. D., and Rawlinson, A. A. (2001). When will plant morphology affect the shape of a seed dispersal “kernel”? J. Theor. Biol. 211, 229–238. doi: 10.1006/jtbi.2001.2341
Cuevas-Guzmán, R., Cisneros–Lepe, E. A., Jardel–Peláez, E. J., Sánchez–Rodríguez, E. V., Guzmán–Hernández, L., Núñez–López, N. M., et al. (2011). Análisis estructural y de diversidad en los bosques de Abies de Jalisco, México. Rev. Mex. Biodivers. 82, 1219–1233.
Dudhia, J. (1989). Numerical study of convection observed during the winter monsoon experiment using a Mesoscale two-dimensional model. J. Atmospheric Sci. 46, 3077–3107. doi: 10.1175/1520-04691989046<3077:NSOCOD<2.0.CO;2
Espinosa-Garduño, J. (2005). “Gymnospermae,” in Flora fanerogámica del Valle de México, eds J. Rzedowski and G. Calderón Flora (Pátzcuaro: Instituto de Ecología, A.C. y Comisión Nacional para el Conocimiento y Uso de la Biodiversidad), 44–55.
Foster, B. L., Questad, E. J., Collins, C. D., Murphy, C. A., Dickson, T. L., and Smith, V. H. (2011). Seed availability constrains plant species sorting along a soil fertility gradient. J. Ecol. 99, 473–481. doi: 10.1111/J.1365-2745.2010.01769.X
Gómez-Pineda, E., Sáenz-Romero, C., Ortega-Rodríguez, J. M., Blanco-García, A., Madrigal-Sánchez, X., Lindig-Cisneros, R., et al. (2020). Suitable climatic habitat changes for Mexican conifers along altitudinal gradients under climatic change scenarios. Ecol. Appl. 30:e02041. doi: 10.1002/eap.2041
Grace, J., Berniger, F., and Nagy, L. (2002). Impacts of climate change on the tree line. Ann. Bot. 90, 537–544. doi: 10.1093/AOB/MCF222
Harsch, M. A., Hulme, P. E., McGlone, M. S., and Duncan, R. P. (2009). Are treelines advancing? A global meta-analysis of treeline response to climate warming. Ecol. Lett. 12, 1040–1049. doi: 10.1111/J.1461-0248.2009.01355.X
Hewitt, N., and Kellman, M. (2002). Tree seed dispersal among forest fragments: II. Dispersal abilities and biogeographical controls. J. Biogeogr. 29, 351–363. doi: 10.1046/j.1365-2699.2002.00679.x
Heydel, F., Cunze, S., Bernhardt-Römermann, M., and Tackenberg, O. (2015). Seasonal synchronization of seed release phenology promotes long-distance seed dispersal by wind for tree species with medium wind dispersal potential. J. Veg. Sci. 26, 1090–1101. doi: 10.1111/jvs.12305
Hoch, G., and Körner, C. (2003). The carbon charging of pines at the climatic treeline: A global comparison. Oecologia 135, 10–21. doi: 10.1007/s00442-002-1154-7
Hong, S.-Y., Dudhia, J., and Chen, S.-H. (2004). A revised approach to ice microphysical processes for the bulk parameterization of clouds and precipitation. Mon. Weather Rev. 132, 103–120. doi: 10.1175/1520-04932004132<0103:ARATIM<2.0.CO;2
Hong, S.-Y., Noh, Y., and Dudhia, J. (2006). A new vertical diffusion package with an explicit treatment of entrainment processes. Mon. Weather Rev. 134, 2318–2341. doi: 10.1175/MWR3199.1
Iglesias, L. G., Solís-Ramos, L. Y., and Viveros-Viveros, H. (2012). Variación morfométrica en dos poblaciones naturales de Pinus hartwegii Lindl. del estado de Veracruz. Phyton B Aires 81, 239–247.
Iglesias-Andreu, L. G., and Tivo-Fernández, Y. (2006). Caracterización morfométrica de la población de Pinus hartwegii lindl. del cofre de perote, Veracruz, México. Ra Ximhai 2, 449–468. doi: 10.35197/rx.02.02.2006.08.li
Jasso-Flores, I., Galicia, L., García-Oliva, F., and Martínez-Yrízar, A. (2019). Are vegetation–soil systems drivers of ecosystem carbon contents along an elevational gradient in a highland temperate forest? Can. J. For. Res. 49, 296–304. doi: 10.1139/cjfr-2018-0245
Jiménez, P. A., Dudhia, J., González-Rouco, J. F., Navarro, J., Montávez, J. P., and García-Bustamante, E. (2012). A revised scheme for the WRF surface layer formulation. Mon. Weather Rev. 140, 898–918. doi: 10.1175/MWR-D-11-00056.1
Jongejans, E., and Telenius, A. (2001). Field experiments on seed dispersal by wind in ten umbelliferous species (Apiaceae). Plant Ecol. 152, 67–78. doi: 10.1023/A:1011467604469
Jordano, P. (2017). What is long-distance dispersal? And a taxonomy of dispersal events. J. Ecol. 105, 75–84. doi: 10.1111/1365-2745.12690
Kain, J. S. (2004). The Kain–Fritsch convective parameterization: An update. J. Appl. Meteorol. Climatol. 43, 170–181. doi: 10.1175/1520-04502004043<0170:TKCPAU<2.0.CO;2
Kelly, A. E., and Goulden, M. L. (2008). Rapid shifts in plant distribution with recent climate change. Proc. Natl. Acad. Sci. U.S.A. 105, 11823–11826. doi: 10.1073/PNAS.0802891105
Kim, M., Lee, S., Lee, S., Yi, K., Kim, H. S., Chung, S., et al. (2022). Seed dispersal models for natural regeneration: A review and prospects. Forests 13:659. doi: 10.3390/f13050659
Krannitz, P. G., and Duralia, T. E. (2004). Cone and seed production in Pinus ponderosa: A review. West. N. Am. Nat. 64, 208–218.
Kreyling, J. (2010). Winter climate change: A critical factor for temperate vegetation performance. Ecology 91, 1939–1948. doi: 10.1890/09-1160.1
Kuparinen, A. (2006). Mechanistic models for wind dispersal. Trends Plant Sci. 11, 296–301. doi: 10.1016/j.tplants.2006.04.006
Landa, J. A., Rentería, A. A., and Ramírez, J. M. (2003). Potencial y eficiencia de producción de semillas de Pinus Hartwegii lindl. de dos poblaciones de México. For. Veracruzana 5, 25–28.
León-Cruz, J. F., Carbajal, N., and Pineda-Martínez, L. F. (2019). The role of complex terrain in the generation of tornadoes in the west of Mexico. Nat. Hazards 97, 335–353. doi: 10.1007/s11069-019-03647-8
Liang, W., Liu, Z., Liu, M., Qin, X., Baskin, C. C., Baskin, J. M., et al. (2020). Wing loading, not terminal velocity, is the best parameter to predict capacity of diaspores for secondary wind dispersal. J. Exp. Bot. 71, 4298–4307. doi: 10.1093/jxb/eraa170
Liu, Z., and Evans, M. (2021). Effect of Tree Density on Seed Production and Dispersal of Birch (Betula pendula Roth and Betula pubescens Ehrhs). Forests 12, 929. doi: 10.3390/f12070929
López-López, A. (unpublished). Variación clinal en Pinus hartwegii a partir de caracteres fenotípicos para la Estación Forestal Experimental Zoquiapan, México. Chapingo: Maestría en Ciencias Forestales, División de Ciencias Forestales, Universidad Autónoma Chapingo.
Lozano-Garcial, S., and Váizquez-Selem, L. (2005). A high-elevation Holocene pollen record from Iztaccihuatl volcano, central Mexico. Holocene 15, 329–338. doi: 10.1191/0959683605hl814rp
Madrigal Sánchez, X. (1967). Contribucion al conocimiento de la ecologia de los bosques de Oyamel (Abies religiosa (H. B. K.) Schl. et Cham.) en el Valle de Mexico. Available online at: https://scholar.google.com/scholar_lookup?title=Contribucion+al+conocimiento+de+la+ecologia+de+los+bosques+de+Oyamel+%28Abies+religiosa+%28H.+B.+K.%29+Schl.+et+Cham.%29+en+el+Valle+de+Mexico&author=Madrigal+Sa%CC%81nchez%2C+Xavier.&publication_year=1967 (accessed October 24, 2022).
Manzanilla-Quiñones, U., Aguirre-Calderón, ÓA., Jiménez-Pérez, J., Treviño-Garza, E. J., and Yerena-Yamallel, J. I. (2019). Distribución actual y futura del bosque subalpino de Pinus hartwegii Lindl en el Eje Neovolcánico Transversal. Madera Bosques 25:e2521804. doi: 10.21829/myb.2019.2521804
Mao, R., Osunkoya, O. O., Campbell, S., and Adkins, S. W. (2022). Wind dispersal of seeds of Parthenium hysterophorus L. (Asteraceae) contributes to its steady invasion and spread. Austral Ecol. 47, 791–803. doi: 10.1111/aec.13159
Méndez Pérez, J. M., Ramírez López, A. K., and Luévano de la Cruz, A. C. (2016). “Evaluación del desempeño del modelo WRF sobre la región montañosa central de Veracruz, México, durante una campaña de mediciones en el verano del 2015,” in Clima, sociedad, riesgos y ordenación del territorio, (Alicante: Servicio de Publicaciones de la UA), 237–245. doi: 10.14198/XCongresoAECAlicante2016-22
Mlawer, E. J., Taubman, S. J., Brown, P. D., Iacono, M. J., and Clough, S. A. (1997). Radiative transfer for inhomogeneous atmospheres: RRTM, a validated correlated-k model for the longwave. J. Geophys. Res. Atmospheres 102, 16663–16682. doi: 10.1029/97JD00237
Murrieta-Hernández, D. M., Pineda-López, M. R., Noa-Carrazana, J. C., Mata-Rosas, M., Zulueta-Rodríguez, R., and Flores-Estévez, N. (2014). The structure of Pinus hartwegii at the Cofre de Perote, Veracruz, Mexico. Open J. For. 04:291. doi: 10.4236/ojf.2014.44035
Nathan, R., Katul, G. G., Bohrer, G., Kuparinen, A., Soons, M. B., Thompson, S. E., et al. (2011). Mechanistic models of seed dispersal by wind. Theor. Ecol. 4, 113–132. doi: 10.1007/s12080-011-0115-3
National Centers for Environmental Prediction/National Weather Service/NOAA/U.S. Department of Commerce (2015). NCEP GDAS/FNL 0.25 Degree Global Tropospheric Analyses and Forecast Grids (Updated daily) [Dataset]. Boulder, CO: Research Data Archive at the National Center for Atmospheric Research, Computational and Information Systems Laboratory. Available online at: doi: 10.5065/D65Q4T4Z (accessed May 16, 2022).
Nathan, R., Safriel, U. N., and Noy-Meir, I. (2001). Field validation and sensitivity analysis of a mechanistic model for tree seed dispersal by wind. Ecology 82, 374–388. doi: 10.1890/0012-96582001082[0374:FVASAO]2.0.CO;2
Neilson, R. P., Pitelka, L. F., Solomon, A. M., Nathan, R., Midgley, G. F., Fragoso, J. M. V., et al. (2005). Forecasting regional to global plant migration in response to climate change. BioScience 55, 749–759. doi: 10.1641/0006-35682005055[0749:FRTGPM]2.0.CO;2
Ortiz-Bibian, M. A., Castellanos-Acuña, D., Gómez-Romero, M., Lindig-Cisneros, R., Silva-Farías, M. Á, Sáenz-Romero, C., et al. (2019). Variación entre poblaciones de Abies religiosa (H.B.K.) Schl. et Cham a lo largo de un gradiente altitudinal. I. Capacidad germinativa de la semilla. Rev. Fitotec. Mex. 42, 301–308.
Osland, M. J., Stevens, P. W., Lamont, M. M., Brusca, R. C., Hart, K. M., Waddle, J. H., et al. (2021). Tropicalization of temperate ecosystems in North America: The northward range expansion of tropical organisms in response to warming winter temperatures. Glob. Change Biol. 27, 3009–3034. doi: 10.1111/GCB.15563
Powell, J. A., and Zimmermann, N. E. (2004). Multiscale analysis of active seed dispersal contributes to resolving Reid’s Paradox. Ecology 85, 490–506. doi: 10.1890/02-0535
Qin, X., Liang, W., Liu, Z., Liu, M., Baskin, C. C., Baskin, J. M., et al. (2022). Plant canopy may promote seed dispersal by wind. Sci. Rep. 12:63. doi: 10.1038/s41598-021-03402-9
Ribbens, E., Silander, J., and Pacala, S. (1994). Seedling recruitment in forests: Calibrating models to predict patterns of tree seedling dispersion. Ecology 75, 1794–1806. doi: 10.2307/1939638
Sáenz-Romero, C., Rehfeldt, G. E., Duval, P., and Lindig-Cisneros, R. A. (2012). Abies religiosa habitat prediction in climatic change scenarios and implications for monarch butterfly conservation in Mexico. For. Ecol. Manag. 275, 98–106. doi: 10.1016/j.foreco.2012.03.004
Skamarock, W. C., Klemp, J. B., Dudhia, J., Gill, D. O., Liu, Z., Berner, J., et al. (2019). A description of the advanced research WRF model version 4, Vol. 145. Boulder, CO: National Center for Atmospheric Research, 550.
Soons, M. B., Heil, G. W., Nathan, R., and Katul, G. G. (2004). Determinants of long-distance seed dispersal by wind in grassland. Ecology 85, 3056–3068. doi: 10.1890/03-0522
Svenning, J.-C., and Sandel, B. (2013). Disequilibrium vegetation dynamics under future climate change. Am. J. Bot. 100, 1266–1286. doi: 10.3732/AJB.1200469
Tape, K., Sturm, M., and Racine, C. (2006). The evidence for shrub expansion in Northern Alaska and the Pan-Arctic. Glob. Change Biol. 12, 686–702. doi: 10.1111/j.1365-2486.2006.01128.x
Torres-Beltrán, C. (2013). Análisis de los cambios del límite superior del bosque en el volcán Iztaccíhuatl. Tesis de Licenciatura. México: Universidad Nacional Autónoma de México.
Williams, C. G., LaDeau, S. L., Oren, R., and Katul, G. G. (2006). Modeling seed dispersal distances: Implications for transgenic Pinus Taeda. Ecol. Appl. 16, 117–124. doi: 10.1890/04-1901
Wyse, S. V., and Hulme, P. E. (2021). Limited evidence for a consistent seed mass-dispersal trade-off in wind-dispersed pines. J. Ecol. 109, 284–293. doi: 10.1111/1365-2745.13477
Zhu, J., Liu, M., Xin, Z., Zhao, Y., and Liu, Z. (2016). Which factors have stronger explanatory power for primary wind dispersal distance of winged diaspores: The case of Zygophyllum xanthoxylon (Zygophyllaceae)? J. Plant Ecol. 9, 346–356. doi: 10.1093/jpe/rtv051
Keywords: seed traits, long-distance dispersal, wind patterns, distribution ranges, mountain systems
Citation: Guzmán-Vázquez I, León-Cruz JF and Galicia L (2023) Role of dispersal in the altitudinal migration of Pinus hartwegii and Abies religiosa in mountain systems. Front. Ecol. Evol. 11:1150137. doi: 10.3389/fevo.2023.1150137
Received: 23 January 2023; Accepted: 04 April 2023;
Published: 24 April 2023.
Edited by:
Marcial Escudero, University of Sevilla, SpainReviewed by:
Mariana Valoy, Fundación Miguel Lillo, ArgentinaMaria Luz Thomann, Universidad Nacional de Tucumán, Argentina
Copyright © 2023 Guzmán-Vázquez, León-Cruz and Galicia. This is an open-access article distributed under the terms of the Creative Commons Attribution License (CC BY). The use, distribution or reproduction in other forums is permitted, provided the original author(s) and the copyright owner(s) are credited and that the original publication in this journal is cited, in accordance with accepted academic practice. No use, distribution or reproduction is permitted which does not comply with these terms.
*Correspondence: Leopoldo Galicia, bGdhbGljaWFAZ2VvZ3JhZmlhLnVuYW0ubXg=