- 1Marine Science Discipline, Eckerd College, St. Petersburg, FL, United States
- 2Marine Science and Biology Disciplines, Eckerd College, St. Petersburg, FL, United States
Microplastics have been recognized as an emerging contaminant. Copepods are abundant primary consumers in marine food webs. Interactions between copepods and microplastics can lead to negative health effects to the individual and may have implications for populations and ecosystems through biomagnification. Laboratory and field studies have observed various species of zooplankton ingesting microplastics, however, this is the first study to observe microplastic-copepod interactions in Tampa Bay. Over 2 years (November 2017-January 2020), 14 sampling cruises were conducted with seven stations throughout Tampa Bay. At each station copepods were collected by towing a 200 μm mesh ring net (0.5 m diameter) for 3 min. 1,000 individual Acartia tonsa copepods were picked from each sample and digested to release gut contents. Gut contents were stained in a Nile Red solution and then visualized using epifluorescent microscopy, quantified, photographed and sized using image analysis. In Tampa Bay, A. tonsa consumed fragments over fibers, ranging from 0.018 to 0.642 mm, with an average particle size of 0.076 mm. An overall average of 15.38 particles were ingested per 1,000 copepods, or 6.48 particles m–3 when normalized for environmental copepod concentrations. While significant differences were detected between stations and months, no clear spatial (from head to mouth of estuary) or temporal (between wet and dry seasons) trends in ingestion rate or ingested particle size were evident. These results show that A. tonsa ingested microplastics throughout Tampa Bay. These robust baseline data, for a copepod species that dominates estuarine zooplankton communities around the world, set the stage for valuable comparisons between estuaries with different physical mechanisms and levels of anthropogenic impact, allowing for exploration of how the environmental conditions impact ecological interactions.
1. Introduction
Microplastics are small fibers, fragments or granules of plastic, 0.001–5 mm in diameter (reviewed in GESAMP, 2019). Primary microplastics are small beads and fibers, specifically manufactured for use in various products, as well as pellets known as “nurdles,” which are used in plastic manufacturing (Ellison, 2007; GESAMP, 2019). Secondary microplastics derive from macroplastics that have broken into smaller pieces through processes of thermal degradation, photo-oxidative degradation from UV exposure, and via mechanical degradation from stressors such as abrasion and impact from waves, rocks, sand and ocean currents (Andrady, 2015; GESAMP, 2019). Hence, it is estimated that the concentration of microplastics in the marine environment is negatively correlated with microplastic size, as the proportion of smaller particles increases relative to the number of larger plastics fragmenting (Cózar et al., 2014; Enders et al., 2015; GESAMP, 2019). Based on increasing production and consumption of plastics, projections indicate that up to 53 million metric tonnes of plastic per year may enter the ocean from land by 2030 (Borrelle et al., 2020). Several studies using environmental data and modeling have estimated that between 7,000–93,000 tons or 1.7–4.85 trillion microplastic particles are present in surface waters of the world’s oceans (Cózar et al., 2014; Eriksen et al., 2014; van Sebille et al., 2015; Lebreton et al., 2018), with greater concentrations near continents as opposed to offshore (Tonhua et al., 2020).
With the widespread abundance of plastics in the ocean, there is growing concern for the threats they may pose to marine organisms and ecosystems. Microplastics can transport invasive species (reviewed in García-Gómez et al., 2021) and cause intestinal blockage, pseudo-satiation or even starvation when ingested (reviewed in Egbeocha et al., 2018). Plastics also contain harmful toxins, originating from the manufacturing process, or sorbed hydrophobic toxins from the environment (Engler, 2012; reviewed in Crawford and Quinn, 2017; reviewed in Gallo et al., 2018). Studies have found that if ingested, microplastics and associated toxins can lead to a number of negative reproductive, growth, health, and behavioral impacts (Derriak, 2002; Rochman et al., 2013, 2014; Lönnstedt and Eklöv, 2016; Coppock et al., 2019; Bucci et al., 2020). In the marine environment, an array of animals ingest microplastics, including fishes (Davison and Asch, 2011), seabirds (Van Franeker, 2011), bivalves (Phuong et al., 2018), marine turtles (Duncan et al., 2018), marine mammals (Nelms et al., 2019; Ortega-Borchardt et al., 2023), and zooplankton (reviewed in Botterell et al., 2019). Ecological effects of plastic pollution in the marine environment are less clear and not always detected (Bucci et al., 2020).
The transfer of microplastics from zooplankton to secondary consumers has been observed in laboratory studies (Farrell and Nelson, 2013; Setälä et al., 2014; Athey et al., 2020; Costa et al., 2020; Van Colen et al., 2020; Domínguez-López et al., 2022; Kim et al., 2022), raising concern that plastics and associated toxins have the potential for biomagnification, moving up through trophic levels and accumulating in top consumers. Microscopic plankton represent a potential entry point of microplastics at the base of the food web (Botterell et al., 2019) and may act as bioindicators for overall health of aquatic ecosystems (Hemraj et al., 2016; Araujo et al., 2017). Copepods serve as a vital link between primary producers and higher trophic levels throughout the ocean (Vroom et al., 2017; reviewed in Mauchline, 1998). Investigating copepod interactions with microplastics will not only increase our understanding of the impact of plastics on zooplankton but will also shed light on the potential for zooplankton to serve as vectors of plastic transfer through marine food webs. While investigating grazing rates and size-selection, using microplastics particles as inert “prey,” several early laboratory experiments unintentionally demonstrated that copepods ingest microplastics under certain conditions (Burns, 1968; Frost, 1972; Wilson, 1973). More recent laboratory experiments have found that exposure to high concentrations of microplastics can lead to changes in copepod feeding behavior and prey selection (Cole et al., 2013, 2014, 2019; Coppock et al., 2019; Koski et al., 2021), reproductive output (Cole et al., 2014), molting cycles (Cole et al., 2019), lipid production (Cole et al., 2019), and fecal pellet sinking rates (Coppock et al., 2019).
There has been limited research, however, on ingestion of microplastics by copepods in the field. Ingestion rates (particles per copepod) have been estimated for large oceanic copepod species in northern Pacific (Desforges et al., 2015), Arctic (Howell, 2019; Botterell et al., 2022), and Black Sea (Aytan et al., 2022) offshore waters. Despite differences in prey size spectra as function of copepod size and feeding strategy, ingestion rate estimates have also been made for mixed copepod assemblages in coastal waters of the South China Sea (Sun et al., 2017; Amin et al., 2020), Gulf of Thailand (Buathong et al., 2020; Taha et al., 2021), Andaman Sea (Goswami et al., 2020), and western Indian Ocean (Kosore et al., 2018). Notably, few observations have been made of microplastic ingestion by copepods in estuaries, where high biological productivity and high surface microplastic concentrations, resulting from the semi-enclosed shape and proximity to river discharge and anthropogenic activities (GESAMP, 2019), facilitate such interactions. Microplastic ingestion rate estimates were generated from small sample sizes collected over a series of days for copepod assemblages in Malaysia (Taha et al., 2021) and the southeastern United States (Payton et al., 2020), as well as for dominant copepod species in the mid-Atlantic United States (Sipps et al., 2022). In contrast, periodic sampling over the course of many months accounted for seasonal variability in tropical rainfall when measuring microplastic ingestion by copepod assemblages from estuaries in India (Rashid et al., 2022) and China (Zheng et al., 2021).
This study aims to expand understanding of microplastic-copepod interactions by comprehensively characterizing the ingestion of environmental microplastics by a cosmopolitan estuarine calanoid copepod. Tampa Bay is located in the transition zone between subtropical and temperate climates along Florida’s west-central coast. Spanning a surface area of 1,036 km2, it is the largest open-water estuary in Florida. A watershed of approximately 5,700 km2 feeds into Tampa Bay, including four major rivers and numerous smaller tributaries (Cicchetti and Greening, 2011). However, non-point source stormwater runoff appears to be the greatest input of freshwater to the Bay (Zhu et al., 2015). Pinellas County, to the west, is the most densely populated county in Florida1 and the Bay supports high levels of tourism, fishing and boating. Additionally, Tampa Bay is the only deep water cargo port on Florida’s west coast and the largest cargo port in Florida.2 This collection of features suggests that Tampa Bay may be especially prone to plastic accumulation; McEachern et al. (2019) estimated an average of 940 particles m–3 in Tampa Bay surface waters. Here, we report on the quantities, sizes and types (e.g., fragment or fiber) of microplastic ingested by Acartia tonsa over the course of 2 years in Tampa Bay, providing a substantial baseline for comparison to future studies in both developed and pristine estuaries.
2. Materials and methods
From November 2017 through January 2020 copepod samples were collected bi-monthly (14 cruises total) at seven stations in Tampa Bay, FL, USA (Figure 1). All stations, except for Boca Ciega Bay, were co-located with established Environmental Protection Commission of Hillsborough County (EPC-HC) water quality monitoring sites and selected to represent sub-regions of the Bay with different flushing times and physical mechanisms (e.g., tidal excursion, river influx, and winds). Because Tampa Bay is long and the four major rivers enter on the eastern side, residence times increase from mouth to head (south to north) and east to west (Zhu et al., 2015). Many tidal inlets between barrier islands keep the Boca Ciega Bay region well-flushed with a short residence time (Zhu et al., 2015). Skyway, Pinellas Point, and MacDill Air Force Base stations follow a south to north axis through the open mid-Bay region. The two heads of the Bay differ greatly in residence time. Hillsborough Bay residence time is shorter (100–120 days) due to freshwater input from the Hillsborough and Alafia Rivers, whereas Old Tampa Bay residence time is longest (120–200 days) due to its location in the northwest and the multiple causeways that restrict flow (Zhu et al., 2015). The Egmont Key station sits at the transition between Tampa Bay and open shelf waters.
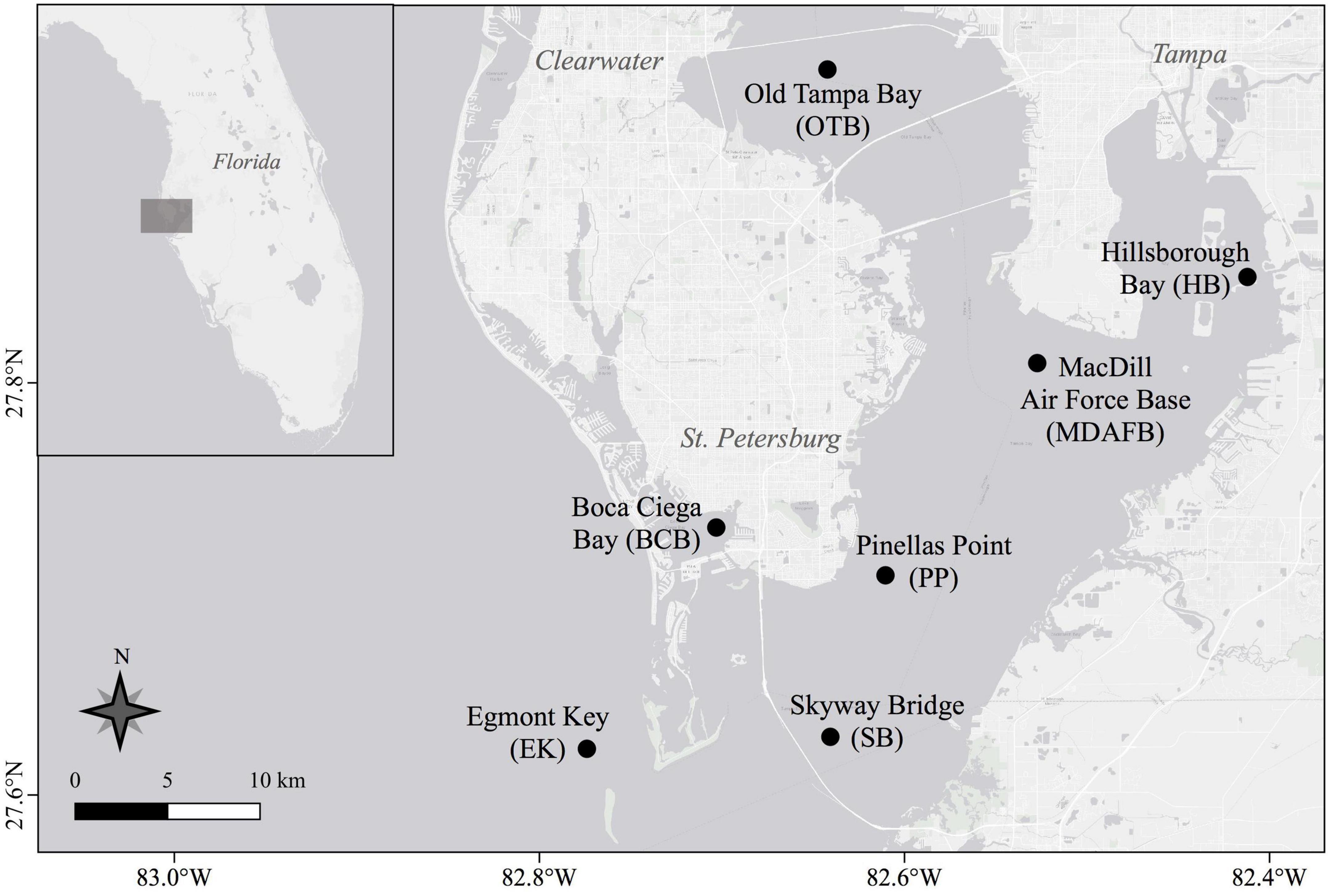
Figure 1. Sampling locations in Tampa Bay, Florida, USA. Station map was created using QGIS software (QGIS 3.4.14-Madeira).
Copepods were collected using a Sea-Gear Corporation (Florida, USA) model 9000 conical ring net (0.5 m diameter, 200 μm mesh) towed horizontally while submerged just below the surface for 3 min at approximately 1.5 knots. Tow distance was calculated based on straight line distance between beginning and ending GPS coordinates and tow volume was estimated from tow distance and area of the plankton net mouth. Each zooplankton sample was immediately concentrated on a 200 μm mesh sieve and preserved with ethanol to halt ingestion or egestion of microplastic particles.
In the lab, each sample was concentrated on a 200 μm mesh sieve and resuspended in a known volume of Milli-Q water to determine biovolume. From each sample, 1,000 copepods, with full guts and of the dominant species, were individually selected using a glass Pasteur pipet. Each copepod was passed through several Milli-Q water rinses and examined under a dissecting microscope with bright-field, white light to confirm there were no externally adhered microplastic particles. Copepods were transferred in batches of 500 individuals to 2 mL microcentrifuge tubes for an initial room temperature decomposition period of 14 days. Subsequently, the batches were dried for approximately 24 h in a covered heating block set to 60°C. Microcentrifuge tube caps were closed but not firmly sealed to allow for evaporation during the drying step. An enzymatic digestion protocol adapted from Cole et al. (2014), with a ratio of 500 μg mL–1 proteinase-K to 0.2 g dry-weight of sample, was used to break apart exoskeletons and release gut content from copepods into solution. For the contents in each tube, 750 μL homogenizing solution (0.25 L stock homogenizing solution consisting of 15.77 g Tris-HCL, 4.38 g EDTA, 1.53 g NaCl, and 1.26 g SDS, with 15 mL of homogenizing solution being 40 mM Tris-HCL, 60 mM EDTA, 105 mM NaCl and 1% SDS) were added. Sample tubes were then vortexed for at least 30 sec and incubated for 1–2 h at 60°C. 40 μL of proteinase-K solution was added and samples were incubated for at least 6–8 h with periodic vortexing. 250 μL of 5M NaClO4 were added and samples were vortexed for several minutes before incubating at 60°C for 24 hrs.
Nile Red [9-diethylamino-5H-benzo(a) phenoxazine-5-one], a selectively fluorescent dye that binds to lipids (Maes et al., 2017; Shim et al., 2017), was used to stain copepod gut content in preparation for visual identification of microplastics. Preliminary protocol testing with plastics of known composition indicated that plastics glowed brightly red, orange, yellow or green, depending on composition, and chitin or cellulose material fluoresced a faint red. It is important to note that the staining pattern may cause semi-synthetic or regenerated cellulosic fibers to be overlooked and the presence of manufacturing dyes can negatively impact the ability of Nile Red to stain some plastic particles (Stanton et al., 2019). Therefore, the quantification of microplastics using this method may represent an underestimation of particles present in a sample.
Digested copepod batches were stained with Nile Red for 30 min in clean and covered glass beakers containing a ratio of 20 mL Milli-Q water to 4 mL Nile Red stock solution (5 mg Nile Red powder dissolved in 1 L acetone). Each batch was then transferred to a 47 mm diameter, 5 μm pore size black polycarbonate filter using vacuum filtration and stored in a clean petri dish with lid. To eliminate contamination, cotton clothing or lab coats were worn at all times and samples and reagent solutions were kept covered while handling. Wet filters left exposed in a variety of locations around the lab for 24 h did not accumulate microplastic particles.
A dissecting microscope equipped with a digital camera and Stereo Microscope Fluorescence Adapter system (NightSea, Lexington, MA, USA) was used to quantify microplastic particles. Sample filters were exposed to cyan light (excitation wavelength: 490–515 nm) and viewed through an orange emission filter (550 nm long pass). Similar to reports by others (Erni-Cassola et al., 2017; Nalbone et al., 2021), brightly glowing microplastic particles clearly contrasted with the faint red of stained copepod exoskeleton fragments remaining after digestion (Figure 2). Before imaging a particle and adding its photo to the archive, each particle was also examined at higher magnification under bright-field, white light and physically manipulated with a metal probe to assess hardness. Microplastic particles were categorized as fragments or fibers. ImageJ (Schneider et al., 2012) was used to measure the length of the longest axis.
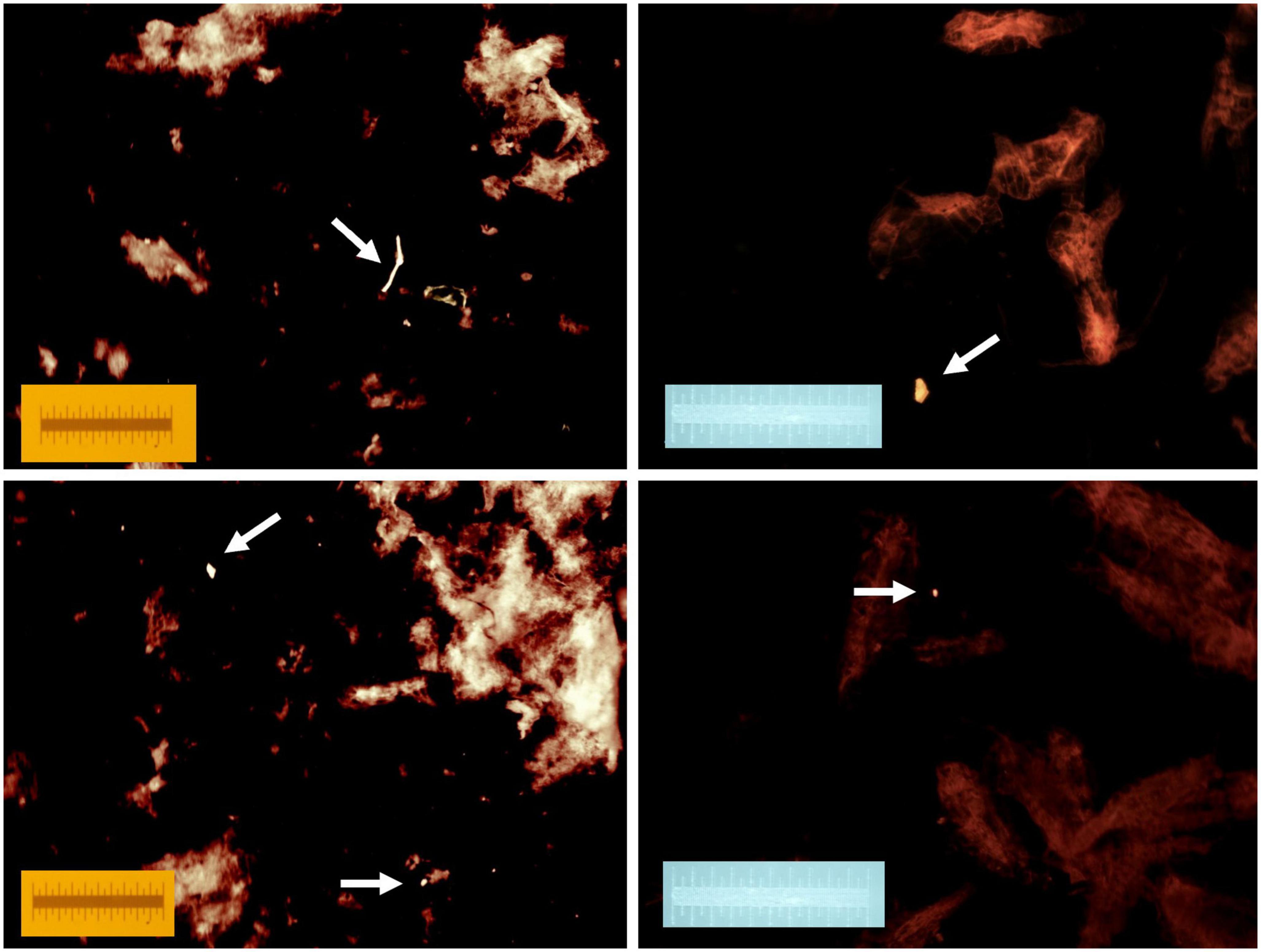
Figure 2. Four separate examples of fluorescent microplastics released during copepod digestion. Samples were stained with Nile red following digestion and photographed with a cyan light (490–515 nm) through an orange long pass filter (550 nm). Each image includes a 1 mm scale.
In addition to directly measured microplastic particle length and number of ingested particles per 1,000 copepods, the number of ingested particles per m3 was estimated to account for differences in mesozooplankton concentration between samples. Based on observations made while picking copepods for digestion, a conversion of 1 mL biovolume to 1,000 individuals was used to estimate the total number of individuals in each tow. The calculation also assumed that each copepod contained at most a single ingested microplastic particle. As none of these direct-measurement and estimated datasets were normally distributed, single-factor Kruskal–Wallis tests were used to assess differences between groups (e.g., stations, months, and seasons) and Dunn Method for Joint Ranking tests were used to make pairwise comparisons. All data analyses were performed in JMP Version 16 (SAS Institute Inc., Cary, NC, USA).
3. Result
A total of 1,507 ingested microplastic particles were collected from 97,500 copepods. A. tonsa dominated mesozooplankton communities and accounted for all 1,000 copepods picked from samples at all stations, except one–the May 2018 sample from Egmont Key, where 500 copepods representing multiple species were picked in the absence of abundant A. tonsa. Most ingested microplastic particles were fragments (96.88%); the remainder of ingested particles were fibers, which were most commonly ingested at the Skyway Bridge and Egmont Key stations. The mean size of all ingested microplastic particles was 0.076 mm (SE ± 0.001; Figure 3). Fragment length ranged from 0.018 to 0.351 mm (mean = 0.072 mm; SE ± 0.001), whereas fiber length ranged from 0.019 to 0.642 mm (mean = 0.202 mm; SE ± 0.022). Significant differences in ingested particle size were detected between sampling stations (H = 22.105, 6 d.f., p = 0.0012; Figure 4A) and months (H = 58.903, 5 d.f., p < 0.0001; Figure 4B). Pairwise comparisons indicated that ingested particle size was significantly smaller at the Boca Ciega Bay station than at the Pinellas Point (p = 0.0106), Old Tampa Bay (p = 0.0275) and Hillsborough Bay stations (p = 0.0359). Ingested particle size was significantly smaller during May and November compared with January (May: p = 0.0039; Nov.: <0.0001), March (May: p = 0.0124; Nov.: p < 0.0001) and September (May/Nov.: p < 0.0001) as well as during July compared with September (p = 0.0153). All other comparisons between stations and months resulted in no significant differences.
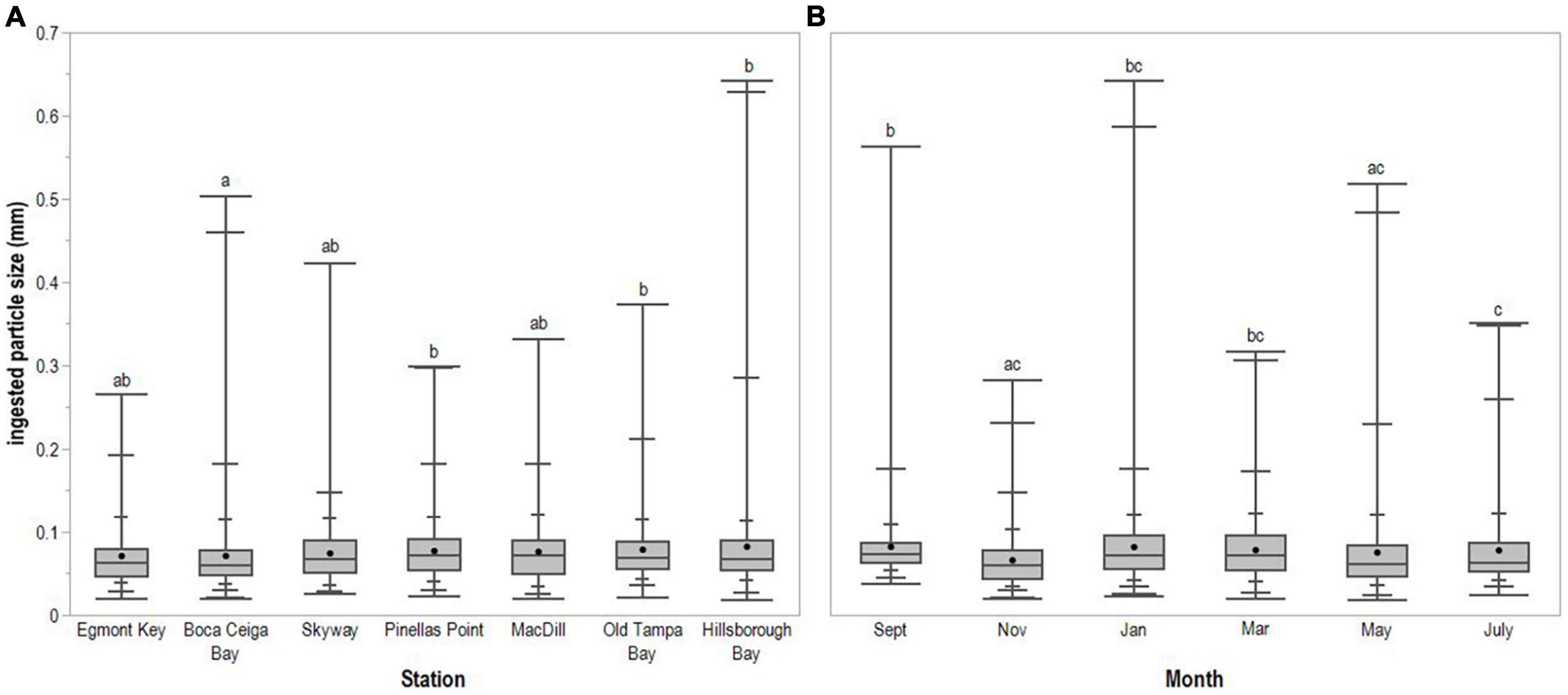
Figure 4. Ingested microplastic particle size (mm) across (A) sampling stations and (B) months. Horizontal line spanning box indicates median, while point indicates mean. Whiskers indicate maximum and minimum values, with intermediate quantiles marked. Letters identify statistically significant differences between stations or months.
The average number of ingested microplastic particles per 1,000 copepods was 15.38 (SE ± 0.85), with a mode of 11 microplastic particles per 1,000 copepods. The Hillsborough Bay sample from May 2018 contained 55 ingested microplastic particles per 1,000 copepods, the maximum observed in this study. The minimum was 4 ingested microplastic particles per 1,000 copepods in the January 2018 MacDill Air Force Base and September 2018 Old Tampa Bay samples. There were no significant differences in the number of microplastic particles ingested per 1,000 copepods between stations (H = 3.214, 6 d.f., p = 0.7815; Figure 5A). However, the number of ingested microplastic particles per 1,000 copepods was significantly lower during the dry season (August to June) compared with the wet season (May to July; H = 4.868, 1 d.f., p = 0.0274). Specifically, the number of ingested particles per 1,000 copepods was lower during September compared with May (p = 0.0274; Figure 5B); no other pairwise comparisons between months were significantly different.
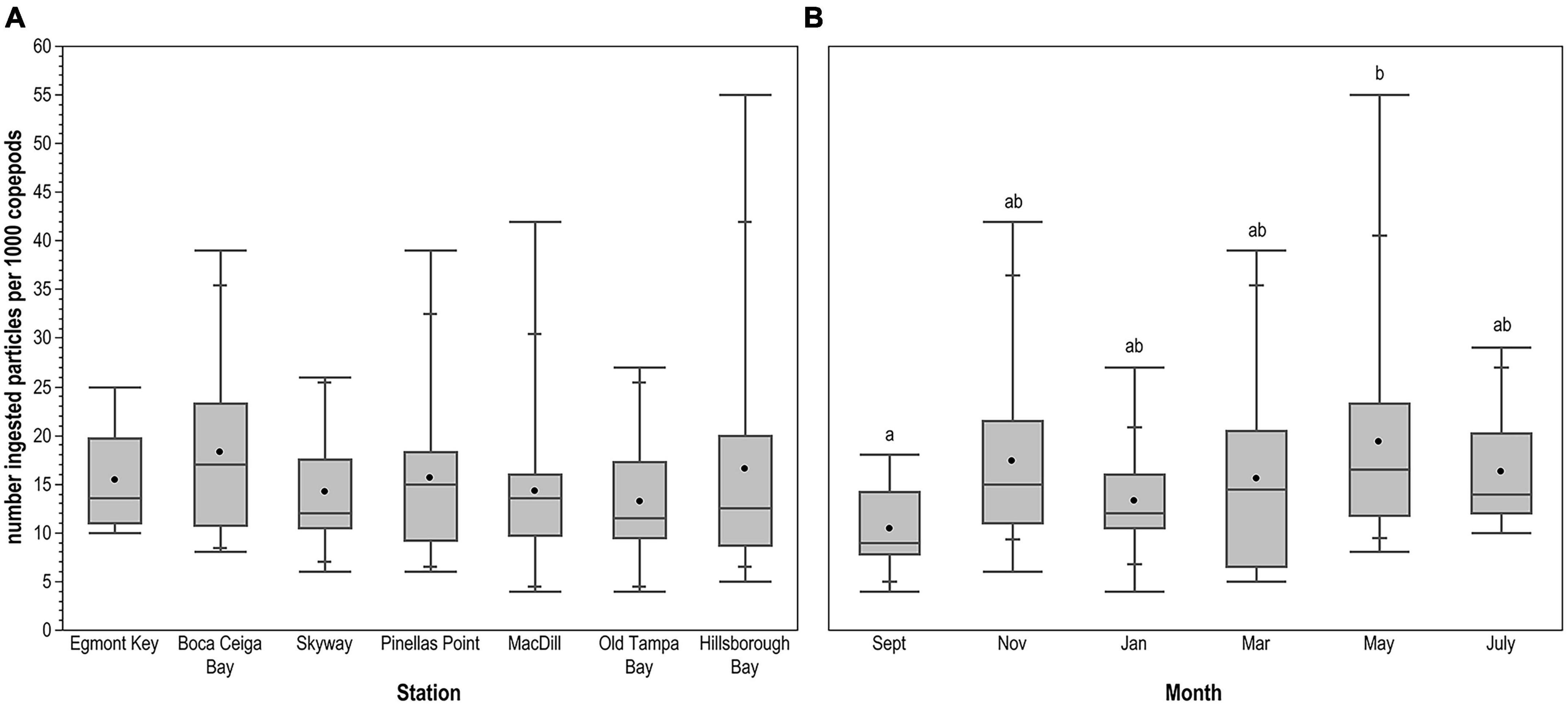
Figure 5. Number of ingested microplastic particles per 1,000 copepods across (A) sampling stations and (B) months. Horizontal line spanning box indicates median, while point indicates mean. Whiskers indicate maximum and minimum values, with intermediate quantiles marked. Letters identify statistically significant differences between months; there were no significant differences between stations.
Variable wind and tidal current speed and direction resulted in tow volumes ranging from 7.27 to 83.64 m3 (mean = 31.81 m3; SE ± 1.42). Mesozooplankton biovolume per tow ranged from 1.2 to 76 mL (mean = 12.73 mL; SE ± 1.42) after removing one sample with abundant diatoms that skewed the measurement. There was no relationship between tow volume and mesozooplankton biovolume; tow volumes were close to the mean at the maximum and minimum biovolume stations. The estimated number of microplastic particles ingested per m3 was not significantly different between stations (H = 1.853, 6 d.f., p = 0.9327; Figure 6A) or sampling months (H = 7.742, 5 d.f., p = 0.1710; Figure 6B). However, fewer particles were ingested per m3 during the dry as compared to the wet season (H = 6.429, 1 d.f., p = 0.0112).
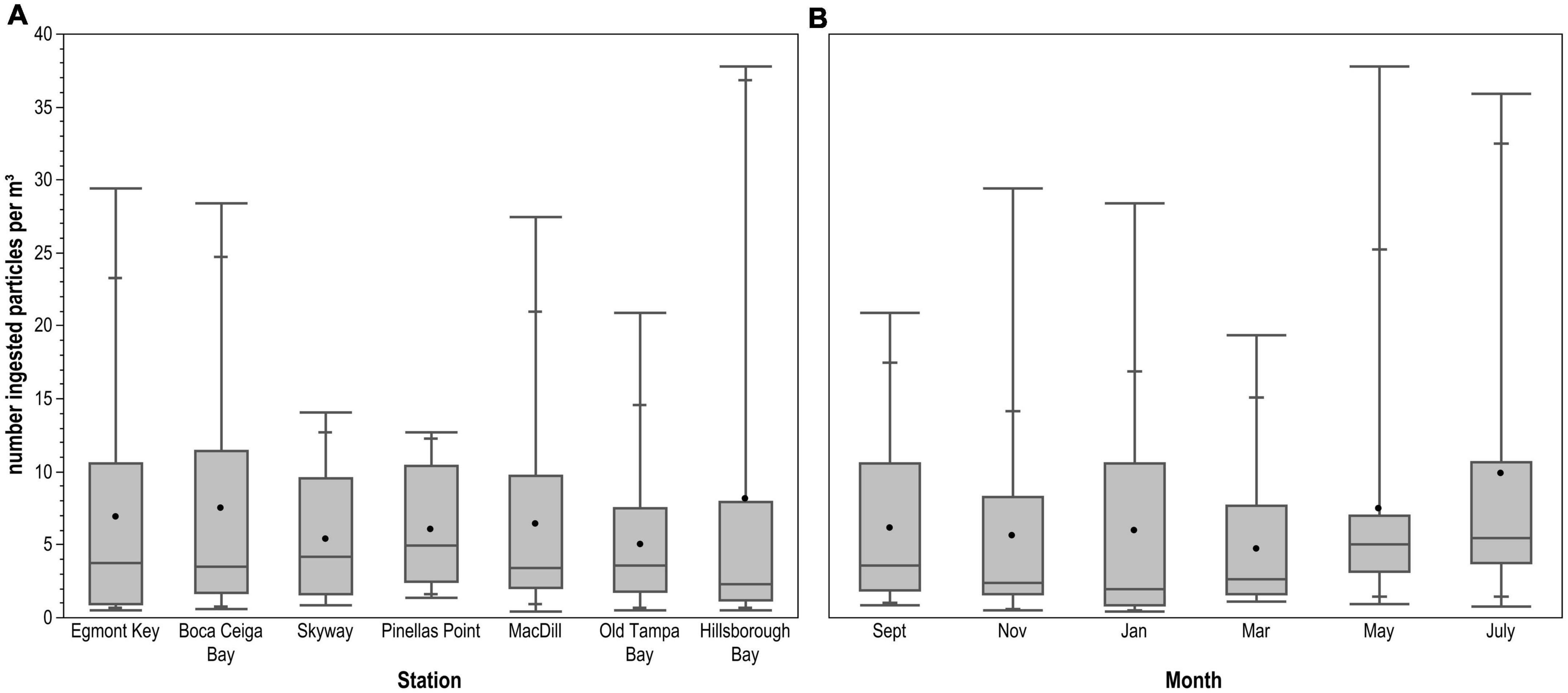
Figure 6. Number of ingested microplastic particles per m3 across (A) sampling stations and (B) months. Horizontal line spanning box indicates median, while point indicates mean. Whiskers indicate maximum and minimum values, with intermediate quantiles marked. There were no statistically significant differences between stations or months.
4. Discussion
A. tonsa copepods in Tampa Bay primarily ingested microplastic fragments. Fibers accounted for <4% of ingested particles, despite having been reported as the most common microplastic type in surface waters of the Bay (McEachern et al., 2019). This pattern of dominant fibers in seawater samples with dominant fragments in concurrent copepod samples has been observed by others (Amin et al., 2020; Aytan et al., 2022) and while fibers were abundantly present in seawater of the Fram Strait, copepods ingested only fragments (Botterell et al., 2022). Overall, however, ingestion of greater proportions of fibers to fragments has been more frequently reported. Kosore et al. (2018) and Taha et al. (2021) found that fibers were dominant in both seawater and copepod samples. Similarly, a greater proportion of microplastic fibers to fragments were ingested by copepods in the other published field studies (Sun et al., 2017; Buathong et al., 2020; Payton et al., 2020; Zheng et al., 2021; Rashid et al., 2022). Amin et al. (2020) suggested that if zooplankton were not preserved immediately after collection, fragments could pass through the digestive system more easily than fibers, leading to reporting of elevated fiber to fragment ingestion ratios. Yet, most methods in the aforementioned studies include a preservation step. Furthermore, A. tonsa selected for nylon fibers over polystyrene beads in a laboratory setting (Borrelle et al., 2020). In this particular case, the nylon fibers were smaller than the polystyrene beads, possibly making the fibers more easily ingested. In the field, fibers tend to be larger/longer than fragments (Aytan et al., 2022; Botterell et al., 2022), with lengths that can exceed ingestion capacities. The shape of ingested microplastic appears to be limited both by particle size and availability in the surrounding water. Thus, Desforges et al. (2015) found that a single large copepod species switched from more frequently ingesting fragments at offshore sites to only ingesting fibers at coastal sites, and given the mismatch in shape and size of fibers to the typical estuarine copepod diet of phytoplankton and microzooplankton, more frequent ingestion of fragments by A. tonsa was expected.
The average size of particles ingested by A. tonsa was comparable to that of their natural phytoplankton prey (0.002–0.106 mm or 2–5% of prosome length; Berggreen et al., 1988), as well as to estimates for microplastic ingestion by A. tonsa in the Hudson-Raritan Estuary, USA (Sipps et al., 2022) and for the closely related A. clausi in the Black Sea (Aytan et al., 2022). These three estimates, however, were smaller than estimates reported for copepods in most other field studies. Ingested particle size clearly scales with copepod size (Hansen et al., 1994). Aytan et al. (2022) observed Calanus euxinus, a species of copepod larger than A. tonsa, ingesting microplastic particles that were 1.8 times the size of those ingested by A. tonsa in Tampa Bay. The largest microplastics (2.485 mm) ingested by copepods were found during a study conducted in Jiaozhou Bay, Yellow Sea (Zheng et al., 2021). Although the copepod species was not reported, the authors indicated that typical prey size for the dominant copepods was approximately 0.5 mm. While the maximum recorded fragment (0.351 mm) and fiber (0.642 mm) lengths ingested by copepods in Tampa Bay were exceptional, Buathong et al. (2020) also measured large particles ingested by calanoid copepods of similar size to A. tonsa and Davis (1977) reported an observation of A. longiremus (1.16 mm) ingesting a larval Chaetognatha (approx. 2.6 mm), making the prey 2.23 times longer than the predator. Desforges et al. (2015) also suggested that fibers are not always elongated and could be folded into a ball, effectively decreasing the overall size and facilitating ingestion. Alternatively, a few large particles may have remained adhered to exoskeletons even through the extensive rinsing process and been measured along with the ingested particles released during enzymatic digestion. Nevertheless, due to the variety of individual species examined and/or the grouping of copepods into mixed assemblages for ingestion estimates, it is difficult to make further comparisons between studies and assess any potential environmental impacts on copepod-microplastic interactions.
In Tampa Bay, the size of particles ingested by A. tonsa exhibited some spatial and temporal variability, but did not show a clear trend. The size of the particles ingested was significantly smaller in Boca Ciega Bay than Pinellas Point, Old Tampa Bay and Hillsborough Bay. This could be caused by slower circulation in Pinellas Point, Old Tampa Bay and Hillsborough Bay (Weisberg and Zheng, 2006) and retention of buoyant microplastic particles from riverine inputs to these areas, whereas water in Boca Ciega Bay exchanges more frequently with the open Gulf of Mexico. Nevertheless, all ingested particles were still within the size range of food particles preferred by A. tonsa (Berggreen et al., 1988). Ingested particle size distribution in Hudson-Raritan Estuary was also significantly different based on location (Sipps et al., 2022). However, no clear pattern was observed or viable explanation offered in either of these studies. Temporally, the particle size ingested by A. tonsa was significantly smaller in May and November compared to January, March and September. Studies in Fram Strait (Botterell et al., 2022) and Hudson-Raritan Estuary (Sipps et al., 2022) also detected temporal variability that was difficult to explain, while other studies reported no significant differences between the size of ingested particles and season (Zheng et al., 2021; Rashid et al., 2022). Future work could explore the potential relationship between the size of seasonally available phytoplankton and the size of microplastic ingested as well as seasonal variation in microplastic sizes in surface waters.
The overall average microplastic ingestion rate by A. tonsa in Tampa Bay was 0.015 particles per individual, which was lower than estimates from estuarine studies conducted in the Hudson-Raritan Estuary, USA (0.56 particles per individual; Sipps et al., 2022), Kochi backwaters, India (0.41 particles per individual; Rashid et al., 2022), and Jiaozhou Bay, China (0.21 particles per individual; Zheng et al., 2021), but on par with estimates from studies in Terengganu Estuary, Malaysia (0.02 particles per individual; Taha et al., 2021) and Charleston Harbor, USA (0.009 particles per individual; Payton et al., 2020). Prey ingestion rates vary by copepod species, as well as prey concentration and nutritional quality (reviewed in Mauchline, 1998). For example, without the presence of infochemicals or biofilms on microplastic particles copepods could be deterred from ingesting microplastics (Vroom et al., 2017; Borrelle et al., 2020). The differences in ingestion rates between studies could also result from differences in proximity to large industrial areas or large populations and likely greater microplastic loads. Similar to Hudson-Raritan Estuary, calanoid copepods ingested 0.45 particles per individual near an industrial complex in Chonburi Province, Thailand (Buathong et al., 2020). However, other studies have observed that the amount of microplastics present in the water does not correlate with the ingestion rate (Amin et al., 2020; Taha et al., 2021). Moreover, the ingestion rate in the present study was lower than estimates for some offshore environments (Desforges et al., 2015; Sun et al., 2017; Botterell et al., 2022). Ingestion rates could also be affected by the current strength and flux of water in and out of the sampling location. Rashid et al. (2022) observed that ingestion rates for calanoid copepods increased during the pre-monsoon season in the Kochi backwaters of India, when Barrage shutters are closed restricting the flow of water into the estuary. Alternatively, Sipps et al. (2022) found that ingestion rate in the Hudson-Raritan Estuary was lowest in July when the river flow was slow. Compared to other estuaries, Tampa Bay has low river input and long residence time (Zhu et al., 2015).
The ingestion rate of A. tonsa showed no significant differences between stations in Tampa Bay. Similar to the present study, there was no spatial pattern to ingestion rates in Terengganu coastal waters and estuary (Amin et al., 2020; Taha et al., 2021), Black Sea (Aytan et al., 2022), Fram Strait (Botterell et al., 2022), northeast Pacific Ocean (Desforges et al., 2015), and South China Sea (Sun et al., 2017). For some, the lack of significance was attributed to a small sample size and a small number of particles ingested by zooplankton (Desforges et al., 2015; Botterell et al., 2022). On the other hand, Zheng et al. (2021) observed significantly greater ingestion rates in the estuary than farther from the coast and attributed this pattern to proximity of estuarine sampling sites to a major river carrying effluent from a large wastewater treatment facility. In contrast, freshwater flow, likely the greatest source of microplastics, into Tampa Bay is dominated by non-point source stormwater runoff (Zhu et al., 2015). Seasonally, in Tampa Bay, there was a significantly lower number of microplastic particles ingested during the dry season (around September) compared to the wet season (around May). This could be attributed to the amount of run-off from the surrounding coastline during the wet season. McEachern et al. (2019) observed that months with increased precipitation levels correlate with increased microplastic concentration in surface waters of Tampa Bay. Regardless of whether or not there is a correlation between microplastic concentrations in surface waters and ingestion rates, freshwater runoff can foster phytoplankton growth (Corcoran et al., 2017), which in turn could increase copepod suspension feeding activity and ingestion of microplastics suspended in the bloom. While other studies also detected significant seasonal differences in ingestion rates, there were no consistent patterns across studies.
In Tampa Bay, the average microplastic ingestion rate normalized for copepod concentration was 6.48 particles m–3 and there were no significant differences between stations or sampling months. McEachern et al. (2019) provides the only estimate of microplastic concentration for comparison in Tampa Bay waters, 940 particles m–3. Of note, this microplastic concentration is over twice the average concentration of copepods collected during the present study (440 copepods per m–3). Based on the present results, copepods in Tampa Bay may be ingesting 0.7% of the available microplastics each hour, assuming that is a viable gut passage time (Kiørboe and Tiselius, 1987; Tirelli and Mayzaud, 2005). Few environmental microplastic ingestion studies normalize for zooplankton concentration (Sun et al., 2017; Amin et al., 2020; Taha et al., 2021; Sipps et al., 2022). Our estimate of normalized ingestion rates and our subsequent extrapolation of proportional ingestion impact on microplastic concentration in Tampa Bay were approximately on the same order of magnitude as those for other studies. However, 10 times greater estimates were reported for the Hudson-Raritan Estuary. Future ingestion studies should also consider accounting for zooplankton concentration in their analyses, as this type of calculation is more directly applied to understanding the degree of environmental impact.
In addition to being the most abundant metazoans in the ocean, calanoid copepods can exhibit higher environmental microplastic ingestion rates than other zooplankton (Sun et al., 2017; Amin et al., 2020; Buathong et al., 2020). Microplastic ingestion can have negative effects on copepod populations via decreased egg production and survival (Cole et al., 2014; Shore et al., 2021). In turn, population level impacts on copepods could lead to adverse effects further up the food web. However, trophic transfer of microplastics from lower trophic levels to higher trophic levels have only been observed in laboratory experiments. A recent study even demonstrated that A. tonsa, specifically, can serve as a vector for microplastic transmission to seahorses, which then retain the microplastic particles and could lead to obstructions in the gastro-intestinal tract (Domínguez-López et al., 2022). Similarly, microplastics in copepod fecal pellets could be transferred to benthic organisms in shallow coastal waters that rely on sinking pellets as an important component of their diet, thus redistributing plastic from the surface to the benthos. However, the presence of microplastics in fecal pellets may decrease their sinking rates (Cole et al., 2016; Coppock et al., 2019; Wieczorek et al., 2019; Shore et al., 2021). Shore et al. (2021) also found that copepods egest smaller and fewer pellets when exposed to high concentrations of microplastics; together these factors could reduce the potential for concentrated redistribution of microplastics from surface to benthos.
5. Conclusion
This study is the first characterization of microplastic-plankton interactions in Tampa Bay Estuary. The microplastic ingestion rate by A. tonsa was spatially consistent but temporal differences seem to be driven by rainy/dry seasons. However, no trends were evident once the data were normalized for copepod concentrations in the environment. The size of microplastic ingested varied spatially and temporally without a consistent pattern, however, the size of particles ingested was consistent with the prey size spectra for A. tonsa. Additionally, A. tonsa ingested significantly more fragments than fibers, and further research is needed to understand the selective behavior of A. tonsa in the presence of fragments and fibers. With a focus on A. tonsa, a species that dominates estuarine zooplankton communities around the world, this study provides a useful baseline for comparison. Additional data on the same species from estuaries with different physical mechanisms and levels of anthropogenic impact will prove useful in teasing apart the role of the environment in these interactions. Finally, future studies should further investigate the potential for and impacts of trophic transfer of microplastics across multiple trophic levels.
Data availability statement
The raw data supporting the conclusions of this article will be made available by the authors, without undue reservation.
Author contributions
ANSS and SG directed the research and oversaw the project. ANSS conceived of the research. MCF and DC conducted the sample collection and processing. All authors contributed to the data analysis and writing of the manuscript.
Funding
This work was provided by two grants from the Tampa Bay Environmental Restoration Fund to ANSS and SG. Additional support was provided by Eckerd College’s Ford Apprentice Scholars Program (DC and ANSS) and Natural Sciences Summer Research Program (ANSS and SG).
Acknowledgments
We thank the many undergraduate student interns on the Microplastics in Tampa Bay Project at Eckerd College for assisting with sample collection. We particularly thank Karsen Henwood for sustained assistance with sample processing.
Conflict of interest
The authors declare that the research was conducted in the absence of any commercial or financial relationships that could be construed as a potential conflict of interest.
Publisher’s note
All claims expressed in this article are solely those of the authors and do not necessarily represent those of their affiliated organizations, or those of the publisher, the editors and the reviewers. Any product that may be evaluated in this article, or claim that may be made by its manufacturer, is not guaranteed or endorsed by the publisher.
Footnotes
References
Amin, R. M., Sohami, E. S., Anuar, S. T., and Bachok, Z. (2020). Microplastic ingestion by zooplankton in Terengganu coastal waters, southern South China Sea. Mar. Pollut. Bull. 150:110616. doi: 10.1016/j.marpolbul.2019.110616
Andrady, A. (2015). “Persistence of Plastic Litter in the Oceans,” in Marine anthropogenic litter, eds M. Bergmann, L. Gutow, and M. Klages (Cham: Springer International Publishing), 57–72. doi: 10.1007/978-3-319-16510-3_3
Araujo, A. V., Dias, C. O., and Bonecker, S. L. C. (2017). Differences in the structure of copepod assemblages in four tropical estuaries: Importance of pollution and the estuary hydrodynamics. Mar. Pollut. Bull. 115, 412–420. doi: 10.1016/j.marpolbul.2016.12.047
Athey, S. N., Albotra, S. D., Gordon, C. A., Monteleone, B., Seaton, P., Andrady, A. L., et al. (2020). Trophic transfer of microplastics in an estuarine food chain and the effects of a sorbed legacy pollutant. Limnol. Oceanogr. Lett. 5, 154–162. doi: 10.1002/lol2.10130
Aytan, U., Esensoy, F. B., and Senturk, Y. (2022). Microplastic ingestion and egestion by copepods in the Black Sea. Sci. Total Environ. 806:150921. doi: 10.1016/j.scitotenv.2021.150921
Berggreen, U., Hansen, B., and Kiørboe, T. (1988). Food size spectra, ingestion and growth of the copepod Acartia tonsa during development: Implications for determination of copepod production. Mar. Biol. 99, 341–352. doi: 10.1007/BF02112126
Borrelle, S. B., Ringma, J., Law, K. L., Monnahan, C. C., Lebreton, L., McGivern, A., et al. (2020). Predicted growth in plastic waste exceeds efforts to mitigate plastic pollution. Science 369, 1515–1518. doi: 10.1126/science.aba3656
Botterell, Z. L. R., Beaumont, N., Dorrington, T., Steinke, M., Thompson, R., and Lindeque, P. K. (2019). Bioavailability and effects of microplastics on marine zooplankton: A review. Environ. Pollut. 245, 98–110. doi: 10.1016/j.envpol.2018.10.065
Botterell, Z. L. R., Bergmann, M., Hildebrandt, N., Krumpen, T., Steinke, M., Thompson, R. C., et al. (2022). Microplastic ingestion in zooplankton from the Fram Strait in the Arctic. Sci. Total Environ. 831:154886. doi: 10.1016/j.scitotenv.2022.154886
Buathong, D., Sriwisait, P., Pnengsakun, S., Chamchoy, C., Mue-suae, O., Phoaduang, S., et al. (2020). Accumulation of microplastics in zooplankton from Chonburi Province, the Upper Gulf of Thailand. RIST 3, 1–12.
Bucci, K., Tulio, M., and Rochman, C. M. (2020). What is known and unknown about the effects of plastic pollution: A meta-analysis and systematic review. Ecol. App. 30:e02044. doi: 10.1002/eap.2044
Burns, C. W. (1968). The relationship between body size of filter-feeding Cladocera and the maximum size of particle ingested. Limnol. Oceanogr. 13, 675–678. doi: 10.4319/lo.1968.13.4.0675
Cicchetti, G., and Greening, H. (2011). Estuarine biotope mosaics and habitat management goals: An application in Tampa Bay, FL, USA. Estuar. Coasts 34, 1278–1292. doi: 10.1007/s12237-011-9408-4
Cole, M., Coppock, R., Lindeque, P. K., Altin, D., Reed, S., Pond, D. W., et al. (2019). Effects of nylon microplastic on feeding, lipid accumulation, and molting in a coldwater copepod. Environ. Sci. Technol. 53, 7075–7082. doi: 10.1021/acs.est.9b01853
Cole, M., Lindeque, P., Fileman, E., Halsband, C., Goodhead, R., Moger, J., et al. (2013). Microplastic ingestion by zooplankton. Environ. Sci. Technol. 47, 6646–6655. doi: 10.1021/es400663f
Cole, M., Lindeque, P. K., Fileman, E., Clark, J., Lewis, C., Halsband, C., et al. (2016). Microplastics alter the properties and sinking rates of zooplankton faecal pellets. Environ. Sci. Technol. 50, 3239–3246. doi: 10.1021/acs.est.5b05905
Cole, M., Webb, H., Lindeque, P. K., Fileman, E. S., Halsband, C., and Galloway, T. S. (2014). Isolation of microplastics in biota-rich seawater samples and marine organisms. Sci. Rep. 4:4528. doi: 10.1038/srep04528
Coppock, R., Galloway, T. S., Cole, M., Fileman, E. S., Queirós, A. M., and Lindeque, P. K. (2019). Microplastics alter feeding selectivity and fecal density in the copepod, Calanus helgolandicus. Sci. Total Environ. 687, 780–789. doi: 10.1016/j.scitotenv.2019.06.009
Corcoran, A. A., Wolny, J., Leone, E., Ivey, J., and Murasko, S. (2017). Drivers of phytoplankton dynamics in old Tampa Bay, FL (USA), a subestuary lagging in ecosystem recovery. Estuar. Coast Shelf Sci. 185, 130–140. doi: 10.1016/j.ecss.2016.11.009
Costa, E., Piazza, V., Lavorano, S., Faimali, M., Garaventa, F., and Gambardella, C. (2020). Trophic transfer of microplastics from copepods to jellyfish in the marine environment. Front. Environ. Sci. 8:571732. doi: 10.3389/fenvs.2020.571732
Cózar, A., Echevarría, F., González-Gordillo, J. I., Irigoien, X., Úbeda, B., Hernández-León, S., et al. (2014). Plastic debris in the open ocean. PNAS 11, 10239–10244. doi: 10.1073/pnas.1314705111
Davison, P., and Asch, R. G. (2011). Plastic ingestion by mesopelagic fishes in the North Pacific Subtropical Gyre. Mar. Ecol. Prog. Ser. 432, 172–180. doi: 10.3354/meps09142
Derriak, J. G. B. (2002). The pollution of the marine environment by plastic debris: A review. Mar. Pollut. Bull. 44, 842–852. doi: 10.1016/S0025-326X(02)00220-5
Desforges, J. W., Galbraith, M., and Ross, P. S. (2015). Ingestion of microplastics by zooplankton in the Northeast Pacific Ocean. Arch. Environ. Contam. Toxicol. 69, 320–330. doi: 10.1007/s00244-015-0172-5
Domínguez-López, M., Bellas, J., Sánchez-Ruiloba, L., Planas, M., and Hernández-Urcera, J. (2022). First evidence of ingestion and retention of microplastics in seahorses (Hippocampus reidi) using copepods (Acartia tonsa) as transfer vectors. Sci. Tot. Env. 818:151688. doi: 10.1016/j.scitotenv.2021.151688
Duncan, E., Broderick, A. C., Fuller, W. J., Galloway, T. S., Godfrey, M. H., Hamann, M., et al. (2018). Microplastic ingestion ubiquitous in marine turtles. Glob. Chang. Biol. 25, 744–752. doi: 10.1111/gcb.14519
Egbeocha, C. O., Malek, S., Emenike, C. U., and Milow, P. (2018). Feasting on microplastics: Ingestion by and effects on marine organisms. Aquat. Biol. 27, 93–106. doi: 10.3354/ab00701
Ellison, K. (2007). The trouble with nurdles. Front. Ecol. Environ. 5:396. doi: 10.1890/1540-9295(2007)5[396:TTWN]2.0.CO;2
Enders, K., Lenz, R., Stedmon, C. A., and Nielsen, T. G. (2015). Abundance, size and polymer composition of marine microplastics ≥10 μm in the Atlantic Ocean and their modeled vertical distribution. Mar. Pollut. Bull. 100, 70–81. doi: 10.1016/j.marpolbul.2015.09.027
Engler, R. E. (2012). The complex interaction between marine debris and toxic chemicals in the ocean. Environ. Sci. Technol. 46, 12302–12315. doi: 10.1021/es3027105
Eriksen, M., Lebreton, L. C. M., Carson, H. S., Thiel, M., Moore, C. J., Borerro, J. C., et al. (2014). Plastic pollution in the world’s oceans: More than 5 trillion plastic pieces weighing over 250,000 tons afloat at sea. PLoS One 9:e111913. doi: 10.1371/journal.pone.0111913
Erni-Cassola, G., Gibson, M. I, Thompson, R. C., and Christie-Oleza, J. A. (2017). Lost, but found with Nile Red: A novel method for detecting and quantifying small microplastics (1 mm to 20 μm) in environmental samples. Environ. Sci. Technol. 51, 13641–13648. doi: 10.1021/acs.est.7b04512
Farrell, P., and Nelson, K. (2013). Trophic level transfer of microplastic: Mytilus edulis (L.) to Carcinus maenas (L.). Environ. Pollut. 177, 1–3. doi: 10.1016/j.envpol.2013.01.046
Frost, B. W. (1972). Effects of size and concentration of food particles on the feeding behavior of the marine planktonic copepod Calanus pacificus. Limnol. Oceanogr. 17, 805–815. doi: 10.4319/lo.1972.17.6.0805
Gallo, F., Fossi, C., Weber, R., Santillo, D., Sousa, J., Ingram, I., et al. (2018). Marine litter plastics and microplastics and their toxic chemicals components: The need for urgent preventive measures. Environ. Sci. Eur. 30:13. doi: 10.1186/s12302-018-0139-z
García-Gómez, J. C., Garrigós, M., and Garrigós, J. (2021). Plastic as a vector of dispersion for marine species with invasive potential. A review. Front. Ecol. Evol. 9:629756. doi: 10.3389/fevo.2021.629756
GESAMP (2019). “Guidelines for the monitoring and assessment of plastic litter and microplastics in the ocean,” in GESAMP joint group of experts on the scientific aspects of marine environmental, eds P. Kershaw, A. Turra, and F. Galani (Nairobi: United Nations Environment Programme (UNEP)).
Goswami, P., Vinithkumar, N. V., and Dharani, G. (2020). First evidence of microplastics bioaccumulation by marine organisms in the Port Blair Bay, Andaman Islands. Mar. Pollut. Bull. 155:111163. doi: 10.1016/j.marpolbul.2020.111163
Hansen, B., Bjornsen, P. K., and Hansen, P. J. (1994). The size ratio between planktonic predators and their prey. Limnol. Oceanogr. 39, 395–403. doi: 10.4319/lo.1994.39.2.0395
Hemraj, D. A., Hossain, M. A., Ye, Q., Qin, J. G., and Leterme, S. C. (2016). Plankton bioindicators of environmental conditions in coastal lagoons. Estuar. Coast Shelf Sci. 184, 102–114. doi: 10.1016/j.ecss.2016.10.045
Howell, L. M. (2019). Microplastic pollution in the Arctic Ocean: Assessing ingestion and potential health effects in Calanus and Neocalanus copepods. Ph.D thesis. Burnaby, BC: Simon Fraser University.
Kim, L., Cui, R., Kwak, J. I., and An, Y.-J. (2022). Sub-acute exposure to nanoplastics via two-chain trophic transfer: From brine shrimp Artemia franciscana to small yellow croaker Larimichthys polyactis. Mar. Pollut. Bull. 175:113314. doi: 10.1016/j.marpolbul.2021.113314
Kiørboe, T., and Tiselius, P. T. (1987). Gut clearance and pigment destruction in a herbivorous copepod, Acartia tonsa, and the determination of in situ grazing rates. J. Plankton Res. 9, 525–534. doi: 10.1093/plankt/9.3.525
Koski, M., Søndergaard, J., Christensen, A. M., and Nielsen, T. G. (2021). Effect of environmentally relevant concentrations of potentially toxic microplastic on coastal copepods. Aquat. Toxicol. 230:105713. doi: 10.1016/j.aquatox.2020.105713
Kosore, C., Ojwang, L., Maghanga, J., Kamau, J., Kimeli, A., Omukoto, J., et al. (2018). Occurrence and ingestion of microplastics by zooplankton in Kenya’s marine environment: First documented evidence. Afr. J. Mar. Sci. 40, 225–234. doi: 10.2989/1814232X.2018.1492969
Lebreton, L., Slat, B., Ferrari, F., Sainte-Rose, B., Aitken, J., Marthouse, R., et al. (2018). Evidence that the Great Pacific Garbage Patch is rapidly accumulating plastic. Sci. Rep. 8:4666. doi: 10.1038/s41598-018-22939-w
Lönnstedt, O. M., and Eklöv, P. (2016). Environmentally relevant concentrations of microplastic particles influence larval fish ecology. Science 352, 1213–1216. doi: 10.1126/science.aad8828
Maes, T., Jessop, R., Wellner, N., Haupt, K., and Mayes, A. G. (2017). A rapid-screening approach to detect and quantify microplastics based on fluorescent tagging with Nile Red. Sci. Rep. 7:44501. doi: 10.1038/srep44501
McEachern, K., Alegria, H., Kalagher, A. L., Hansen, C., Morrison, S., and Hastings, D. (2019). Microplastic in Tampa Bay, Florida: Abundance and variability in estuarine waters and sediments. Mar. Pollut. Bull. 432, 173–180. doi: 10.1016/j.marpolbul.2019.07.068
Nalbone, L., Panebianco, A., Giarratana, F., and Russell, M. (2021). Nile Red staining for detecting microplastics in biota: Preliminary evidence. Mar. Pollut. Bull. 172:112888. doi: 10.1016/j.marpolbul.2021.112888
Nelms, S. E., Barnett, J., Brownlow, A., Davison, N. J., Deaville, R., Galloway, T. S., et al. (2019). Microplastics in marine mammals stranded around the British coast: Ubiquitous but transitory? Sci. Rep. 9:1075. doi: 10.1038/s41598-018-37428-3
Ortega-Borchardt, J. A., Ramírez-Alvarez, N., Rios Mendoza, L. M., Gallo-Reynoso, J. P., Barba-Acuna, I. D., García-Hernandez, J., et al. (2023). Detection of microplastic particles in scats from different colonies of California sea lions (Zalophus californianus) in the Gulf of California, Mexico: A preliminary study. Mar. Pollut. Bull. 186:114433. doi: 10.1016/j.marpolbul.2022.114433
Payton, T. G., Beckingham, B. A., and Dustan, P. (2020). Microplastic exposure to zooplankton at tidal fronts in Charleston Harbor, SC USA. Estuar. Coast Shelf Sci. 232:106510. doi: 10.1016/j.ecss.2019.106510
Phuong, N. N., Poirier, L., Pham, Q. T., Lagarde, F., and Zalouk-Vergnoux, A. (2018). Factors influencing the microplastic contamination of bivalves from the French Atlantic coast: Location, season and/or mode of life? Mar. Pollut. Bull. 129, 664–674. doi: 10.1016/j.marpolbul.2017.10.054
Rashid, C. P., Jyothibabu, R., Arunpandi, N., Santhikrishnan, S., Vidhya, V., Sarath, S., et al. (2022). Microplastics in copepods reflects the manmade flow restrictions in the Kochi backwaters, along the southwest coast of India. Mar. Pollut. Bull. 177:113529. doi: 10.1016/j.marpolbul.2022.113529
Rochman, C. M., Hentschel, B. T., and Teh, S. J. (2014). Long-term sorption of metals is similar among plastic types: Implications for plastic debris in aquatic environments. PLoS One 9:e85433. doi: 10.1371/journal.pone.0085433
Rochman, C. M., Hoh, E., Kurobe, T., and Teh, S. J. (2013). Ingested plastic transfers hazardous chemicals to fish and induces hepatic stress. Sci. Rep. 3:3263. doi: 10.1038/srep03263
Schneider, C. A., Rasband, W. S., and Eliceiri, K. W. (2012). NIH Image to ImageJ: 25 years of image analysis. Nat. Methods 9, 671–675. doi: 10.1038/nmeth.2089
Setälä, O., Fleming-Lehtinen, V., and Lehtiniemi, M. (2014). Ingestion and transfer of microplastics in the planktonic food web. Environ. Pollut. 185, 77–83. doi: 10.1016/j.envpol.2013.10.013
Shim, W. J., Hong, S. H., and Eo, S. E. (2017). Identification methods in microplastic analysis: A review. Anal. Methods 9, 1384–1391. doi: 10.1039/C6AY02558G
Shore, E. A., deMayo, J. A., and Pespeni, M. H. (2021). Microplastics reduce net population growth and fecal pellet sinking rates for the marine copepod, Acartia tonsa. Environ. Pollut. 284:117379. doi: 10.1016/j.envpol.2021.117379
Sipps, K., Arbuckle-Keil, G., Chant, R., Fahrenfeld, N., Garzio, L., Walsh, K., et al. (2022). Pervasive occurrence of microplastics in Hudson-Raritan estuary zooplankton. Sci. Total Environ. 817:152812. doi: 10.1016/j.scitotenv.2021.152812
Stanton, T., Johnson, M., Nathanail, P., Gomes, R. L., Needham, T., and Burson, A. (2019). Exploring the efficacy of Nile Red in microplastic quantification: A costaining approach. Environ. Sci. Tech. Lett. 6, 606–611. doi: 10.1021/acs.estlett.9b00499
Sun, X., Li, Q., Zhu, M., Liang, J., Zheng, S., and Zhao, Y. (2017). Ingestion of microplastics by natural zooplankton groups in the northern South China Sea. Mar. Pollut. Bull. 115, 217–224. doi: 10.1016/j.marpolbul.2016.12.004
Taha, Z. D., Amin, R. M., Anuar, S. T., Nasser, A. A. A., and Sohaimi, E. S. (2021). Microplastics in seawater and zooplankton: A case study from Terengganu estuary and offshore waters, Malaysia. Sci. Total Environ. 786:147466. doi: 10.1016/j.scitotenv.2021.147466
Tirelli, V., and Mayzaud, P. (2005). Relationship between functional response and gut transit time in the calanoid copepod Acartia clausi: Role of food quantity and quality. J. Plankton Res. 27, 557–568. doi: 10.1093/plankt/fbi031
Tonhua, T., Gutekunst, S. B., and Biastoch, A. (2020). A near-synoptic survey of ocean microplastic concentration along an around-the-world sailing race. PLoS One 15:e0243203. doi: 10.1371/journal.pone.0243203
Van Colen, C., Vanhove, B., Diem, A., and Moens, T. (2020). Does microplastic ingestion by zooplankton affect predator-prey interactions? An experimental study on larviphagy. Environ. Pollut. 256:113479. doi: 10.1016/j.envpol.2019.113479
Van Franeker, J. A. (2011). Reshape and relocate: Seabirds as transformers and transporters of microplastics. NOAA Tech. Memo. 38, 278–280.
van Sebille, E., Wilcox, C., Lebreton, L., Maximenko, N., Hardesty, B. D., Van Franeker, J. A., et al. (2015). A global inventory of small floating plastic debris. Environ. Res. Lett. 10:124006. doi: 10.1088/1748-9326/10/12/124006
Vroom, R. J. E., Koelmans, A. A., Besseling, E., and Halsband, C. (2017). Aging of microplastics promotes their ingestion by marine zooplankton. Environ. Pollut. 231, 987–996. doi: 10.1016/j.envpol.2017.08.088
Weisberg, R. H., and Zheng, L. (2006). Circulation of Tampa Bay driven by buoyancy, tides, and winds, as simulated using a finite volume coastal ocean model. J. Geophys. Res. 111:C01005. doi: 10.1029/2005JC003067
Wieczorek, A. M., Croot, P. L., Lombard, F., Sheahan, J. N., and Doyle, T. K. (2019). Microplastic ingestion by gelatinous zooplankton may lower efficiency of the biological pump. Environ. Sci. Technol. 53, 5387–5395. doi: 10.1021/acs.est.8b07174
Zheng, S., Zhao, Y., Liu, T., Liang, J., Zhu, M., and Sun, X. (2021). Seasonal characteristics of microplastics ingested by copepods in Jiaozhou Bay, the Yellow Sea. Sci. Total Environ. 776:145936. doi: 10.1016/j.scitotenv.2021.145936
Keywords: microplastics, zooplankton, copepod, field ingestion rates, Tampa Bay, estuary
Citation: Fibbe MC, Carroll D, Gowans S and Siuda ANS (2023) Ingestion of microplastics by copepods in Tampa Bay Estuary, FL. Front. Ecol. Evol. 11:1143377. doi: 10.3389/fevo.2023.1143377
Received: 12 January 2023; Accepted: 22 March 2023;
Published: 12 April 2023.
Edited by:
Frank S. Gilliam, University of West Florida, United StatesReviewed by:
Daria Martynova, Zoological Institute (RAS), RussiaRachel Louise Coppock, Plymouth Marine Laboratory, United Kingdom
Copyright © 2023 Fibbe, Carroll, Gowans and Siuda. This is an open-access article distributed under the terms of the Creative Commons Attribution License (CC BY). The use, distribution or reproduction in other forums is permitted, provided the original author(s) and the copyright owner(s) are credited and that the original publication in this journal is cited, in accordance with accepted academic practice. No use, distribution or reproduction is permitted which does not comply with these terms.
*Correspondence: Amy N. S. Siuda, siudaan@eckerd.edu; Shannon Gowans, gowanss@eckerd.edu