- 1Cornell Wildlife Health Center, College of Veterinary Medicine, Cornell University, Ithaca, NY, United States
- 2Durrell Institute of Conservation and Ecology, School of Anthropology and Conservation, University of Kent, Canterbury, United Kingdom
The contraction of the global tiger population over the last 100 years into small, often isolated subpopulations has made them increasingly vulnerable to the impact of disease. Despite this, the health of wild tigers continues to be insufficiently funded and explored. For example, canine distemper virus (CDV), has been associated with localized declines and increased risk of extinction, and yet has received little research attention in most tiger range countries. The emergence of new pathogenic threats has posed fresh challenges, including African swine fever virus (ASFV), which has the potential to devastate wild boar populations, and severe acute respiratory syndrome coronavirus (SARS-CoV2) with implications for tiger conservation that remain unknown. The objective of this review is to synthesize current research on the health of tigers and their prey that impacts the conservation of tigers in the wild. Published sources are interpreted based on three mechanisms through which disease can affect the viability of tiger populations: (1) by reducing the survival of adult tigers, (2) by reducing breeding productivity, and (3) by reducing the carrying capacity of tiger habitat through decreased prey abundance. Examples of CDV, SARS-CoV2, carnivore protoparvovirus 1 and ASFV are used to illustrate these processes and inform discussion of research and mitigation priorities.
1. Introduction
Tigers (Panthera tigris) now occupy just 7% of their historic distribution (Sanderson et al., 2006), and despite some recent successes, many populations continue to decline (Goodrich et al., 2022). Current estimates place the global population between 2,608 and 3,905 mature individuals, with 76% in the Indian Subcontinent (Goodrich et al., 2022). Poaching and habitat degradation have been the primary drivers of these declines and the focus of most conservation interventions. However, with remaining tigers now confined to fragmented, often isolated patches of habitat, vulnerability to disease has never been greater. As such, there is an increasing need to integrate health monitoring and disease surveillance into wider tiger conservation strategies.
Disease – the disruption of structure and/or function of living organisms – is an inherent feature of all life and an important part of the ecosystem. However, few habitats remain in their natural state, with most having been altered by anthropogenic forces that have reduced biodiversity, altered climatic conditions, and fragmented landscapes. Modified habitats create new species interfaces providing opportunities for pathogens to emerge and rapidly disseminate along global transport and trade networks, taking root in distant locations with unpredictable outcomes. This has resulted in a loss of resilience, with depleted and isolated populations now vulnerable to stochastic effects. Outbreaks of disease that were once incidental, are now capable of triggering population collapse.
Long-lived, slow-breeding species like tigers are particularly vulnerable to increases in adult mortality. Studies often use a binary classification of tiger mortality as either “human-caused” or “natural” (Goodrich et al., 2008; Singh et al., 2015; Sadhu et al., 2017), however this is somewhat reductive and discourages researchers from investigating further. In reality many so-called ‘natural’ disease processes are affected by human activity, whether through the introduction of exotic pathogens, new opportunities for disease transmission in modified habitats, or climate-related perturbations. In this context it is helpful to understand what disease processes are present, how they impact the tiger population and, particularly, whether they may be preventable.
The objective of this paper is to review ways in which disease can impact tiger conservation, highlighting examples that encourage researchers to focus on those of greatest potential significance. While the death of any individual tiger in a small and genetically impoverished population is important, such isolated incidents are unpredictable and difficult to mitigate against. Therefore, we have chosen to focus on disease processes that can produce declines at a population level. Pathogens which meet this criterion should be considered priorities for investigation and potential targets for intervention.
There are three fundamental mechanisms through which disease can threaten tiger populations:
i. Reducing the survival of breeding tigers–Late-maturing, slow-breeding species like tigers are sensitive to increases in mortality, particularly of adult breeding females, and populations will decline if their survival drops below 0.85 per year (Chapron et al., 2008). While most tigers die from anthropogenic causes (Goodrich et al., 2008), disease-related mortality can be additive to other factors, narrowing a population’s capacity to withstand the negative effects of humans (Robinson et al., 2015). The presence of non-breeding tigers without territories may buffer against declines in a breeding population, as individuals quickly move to replace territory holders that die (Goodrich et al., 2010). However, turnover in the breeding population causes wider social disruptions and can lead to further mortality through fighting or infanticide (Goodrich et al., 2008; Gilbert et al., 2015). Cubs are dependent on their mothers until late in their second year, therefore relatively long periods of stability are required for productive breeding.
ii. Reducing the productivity of breeding tigers–Population maintenance or growth, requires a steady supply of recruits into the breeding population. Lifetime productivity can be as high as 11.9 to 15.4 cubs (Smith and McDougal, 1991; Kerley et al., 2003), but many breeding females do not achieve this (Kerley et al., 2003) and many cubs will not survive to adulthood. Reproductive disease that prevents conception or causes abortion, and neonatal and juvenile conditions that result in a failure to raise cubs to independence threatens this recruitment. Repeated loss of litters can halt recruitment, effectively stalling population growth and potentially precipitating declines.
iii. Reducing the carrying capacity of tiger habitat–Prey availability defines the carrying capacity of habitat, therefore any disease that reduces prey abundance places limits on tiger populations (Karanth et al., 2004; Miller et al., 2014). Epizootic waves of infection can produce temporary declines in prey density, which reduces cub survival as mothers fail to meet their energetic needs (Miller et al., 2014). Extended circulation of enzootic pathogens can impact prey abundance in the long term, reducing the number of breeding tigers that a landscape can support.
These concepts provide a useful starting point to assess the potential conservation significance of individual diseases. To be significant, pathogens must contribute to one or more of these processes and do so at an epidemiological scale that impacts population viability. This review is structured around each of these mechanisms and uses topical examples to illustrate the processes involved, priorities for research and prospects for mitigation. It must be emphasized that these pathogen case examples have been selected for illustrative purposes and are not the only pathogens with the potential to threaten tiger populations. We have elected to treat all tiger populations as biologically equivalent, as there is little evidence to suggest differences in inherent disease susceptibility between subspecies, although there may be differences in exposure geographically.
2. Literature review
To obtain a comprehensive picture of tiger health research we conducted a review of indexed literature published between 1928 and 2022 within the Web of Knowledge database using a series of relevant search strings (see Supplementary Table S1 for methodology, a full list of Boolean searches and criteria for inclusion). Overall, publications tended to focus on captive animals and on hazards that are of limited importance to the viability of wild tiger populations. Of the 280 publications considered relevant to tiger health, 256 focused on captive tigers (91.4%) and just 35 referred to wild tigers (12.5%). Publications addressing viral pathogens were most represented (40.0%), followed by non-infectious disease (26.4%), bacterial pathogens (16.8%), protozoa (11.8%), helminths (8.9%), fungi (4.6%), toxins (4.6%), ectoparasites (2.1%), prions (1.1%) and Acanthocephalans (0.7%). Among viral pathogens, more publications addressed severe acute respiratory syndrome coronavirus 2 (SARS-CoV 2) (37 papers, although only 22 of those reported on primary research), followed by canine distemper virus (CDV) (26 papers including 15 that referred to wild tigers). Studies addressing non-infectious diseases were predominantly focused on neoplasia (39 studies) or other disease of individual tigers and were not considered relevant to the conservation of free-ranging animals. An annotated account of the main health issues identified are summarized in Supplementary Table S2, and a full bibliography of papers reviewed is available https://doi.org/10.7298/rpe5-wa81.
Of the 35 publications that addressed free-ranging tigers, 27 reported primary research, while the remaining eight included reviews, textbooks, and news commentaries. Subjects covered by the primary literature sources included virology (n = 12), parasitology (n = 9), mixed pathogens (n = 3), dental disease (n = 2) and toxicology (n = 1). Virological studies focused on CDV (n = 8), feline immunodeficiency virus (FIV, n = 2), carnivore protoparvovirus 1 (n = 1) and mixed viruses (n = 2). In most cases, parasitological studies described incidental findings based on post-mortem examinations or fecal analysis, but in two cases the parasite was the primary cause of mortality (Galoncus perniciosus and Taenia solium). Serological surveys of wild tigers were described in eight studies (Table 1). Only one of the 17 pathogens included in the serosurveys is known to cause mortality in free-ranging tigers (CDV), a further five have caused mortality in captive tigers (feline parvovirus [FPV], feline coronavirus [FCoV], feline herpesvirus [FHV], feline calicivirus [FCV] and influenza A virus), and another (FIV) in a captive lion (P. leo) and a captive snow leopard (P. uncia). Several of these pathogens (FPV, FCoV, FHV, FCV and FIV) have been associated with reproductive failure or mortality in neonates.
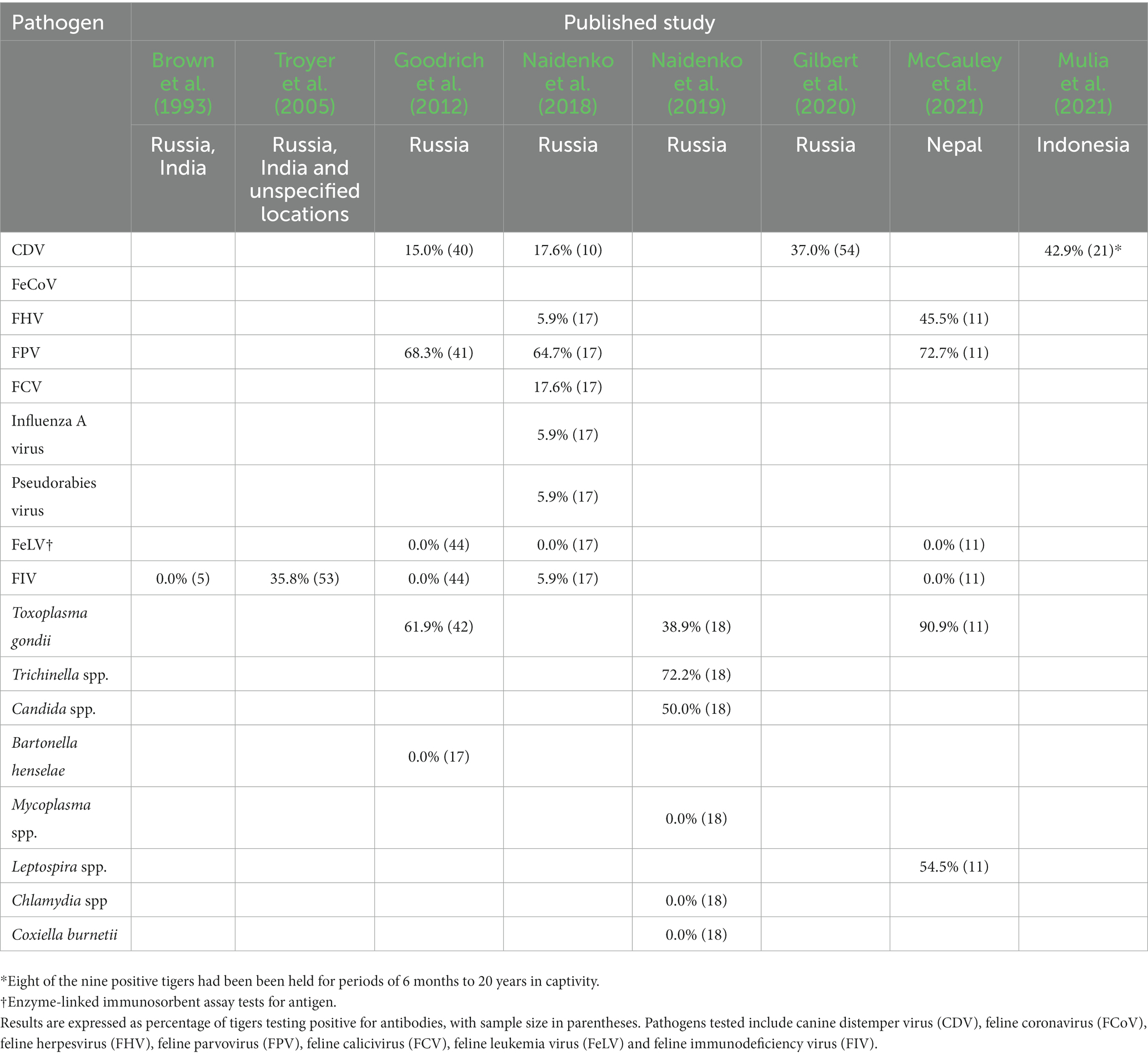
Table 1. Summary of published serological survey findings of free-ranging tigers, indicating location of sample collection.
3. Disease that reduces the survival of breeding tigers
Among the pathogens reported in wild tigers, only CDV has been associated with mortality in breeding adults coincident with population declines, and therefore warrants more detailed attention (Seimon et al., 2013; Gilbert et al., 2015, 2020; Kadam et al., 2022). Furthermore, the global prevalence, high morbidity and observed mortality in captive tigers suggests that SARS-CoV2 has the potential to become an emerging threat in the future. Both pathogens are discussed in the following sections.
3.1. Canine distemper virus
It is now almost 20 years since CDV was first detected in wild tigers, yet our understanding of the threat that it represents across tiger subpopulations remains incomplete. The impact of the virus has been studied most extensively in Russia, following the death of a young female Amur tiger in 2004 and a series of subsequent cases diagnosed since 2010 (Quigley et al., 2010; Seimon et al., 2013; Gilbert et al., 2020). Death from CDV is additive to other causes of tiger mortality (Robinson et al., 2015) and a population viability model has shown that infection increased the 50-year extinction probability of small Russian populations by up to 65% (Gilbert et al., 2014). Recent tiger cases have been confirmed in Malaysia (Ten et al., 2021) and India (Kadam et al., 2022), while antibodies indicating prior infection have been detected in tigers in Indonesia (Mulia et al., 2021) and in Nepal (Bodgener et al., 2023). Although only a small number of cases have been diagnosed, the presence of infection in at least five of the 10 tiger range states suggests that all populations are probably at risk of infection.
Canine distemper is caused by an enveloped, single-stranded RNA virus in the genus Morbillivirus (giving rise to its contemporary common name, canine morbillivirus). The viral envelope reduces environmental stability (particularly in warmer temperatures), limiting opportunities for indirect transmission (Wilkes, 2023). However, CDV is readily transmitted during direct contact, via inhalation of aerosolized respiratory droplets or possibly during predation of infected individuals (Gilbert et al., 2020). Once infected, the virus replicates in immune cells (leading to immunosuppression) and then in epithelial tissue (causing respiratory and gastrointestinal disease). Tigers that survive these initial stages of infection can progress to develop severe neurological disease as the virus becomes established in the central nervous system. Mortality is high, with approximately 30% of clinically affected tigers ultimately dying, including 100% of those that develop neurological signs (Appel et al., 1994; Nagao et al., 2012; Linhares et al., 2021).1 Survivors develop an immune response that should protect them from further infection. While immunologically naïve tigers of any age can die from infection with CDV, mortality of breeding adults has the greatest impact on population viability (Figure 1).
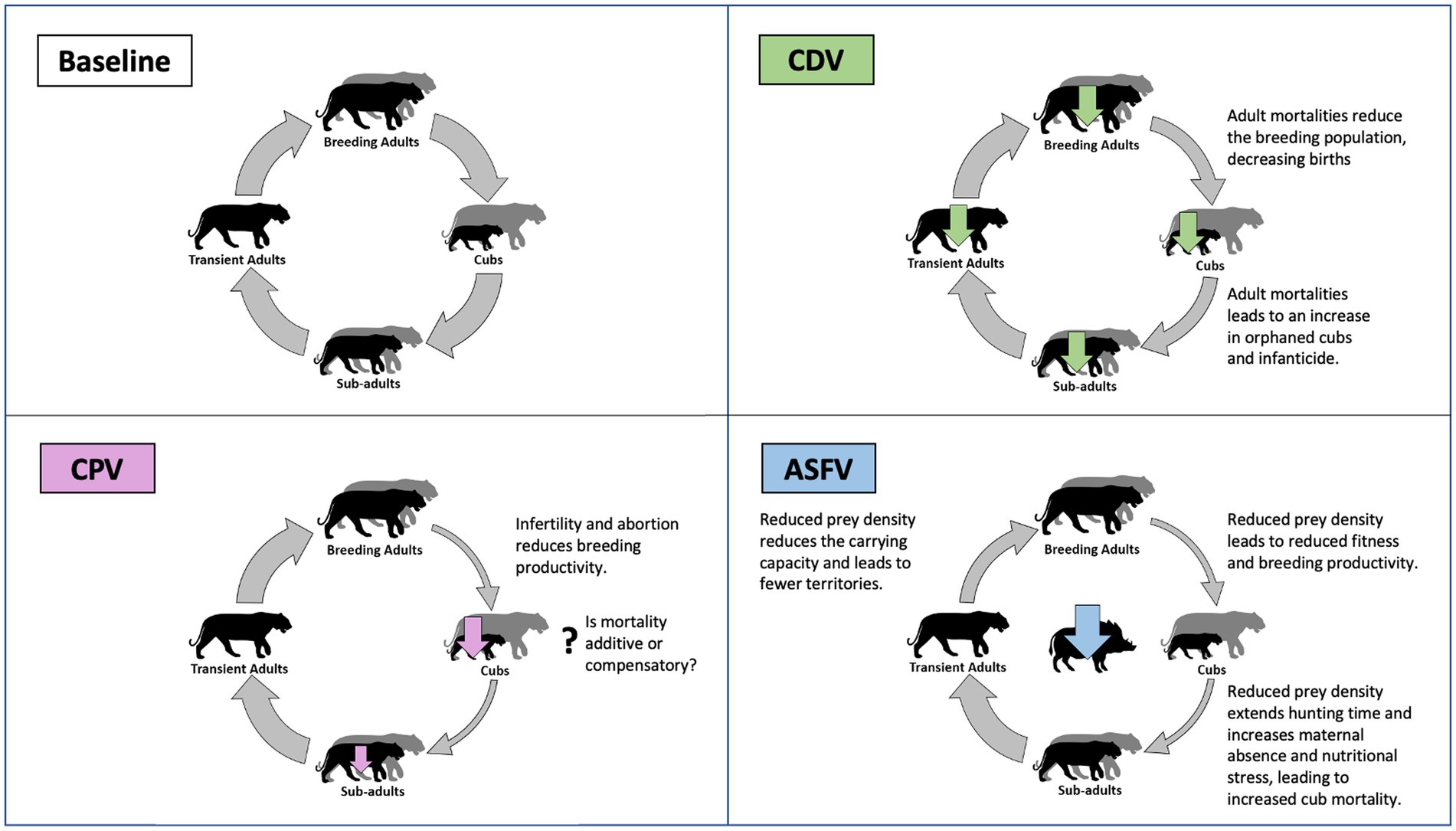
Figure 1. Schematic illustration of ways in which disease can threaten tiger populations compared to a baseline population where disease is absent. Populations consist of breeding adults (with territories), cubs (0–1 year), subadults (1 year–independence) and non-breeding transient adults (without territories). Example pathogens are used to illustrate three ways in which disease can impact tiger populations and include canine distemper virus (CDV–Reduces survival of breeding tigers), canine protoparvovirus 1 (CPV–Reduces productivity of breeding tigers), and African swine fever virus (ASFV–Reduces the carrying capacity of tiger habitat). Disease-related mortality is represented using vertical arrows (over tiger life stages, or wild boar prey) and is scaled according to relative severity. Transition between tiger life stages is represented by circular arrows, which are colored to represent transitions that are constrained by disease and scaled to indicate severity.
There are three epidemiological features, which combine to make CDV a pathogen of conservation importance for tigers: (1) high mortality, (2) high transmissibility and (3) high diversity of susceptible host species. This latter is of particular importance as it enables the virus to perpetuate in reservoirs of more abundant hosts that includes most members of the order Carnivora, which act as a continual source of exposure for tigers. In Russia, a multi-species community of wild carnivores appears to play a dominant role in the CDV reservoir for Amur tigers (Gilbert et al., 2020). However, the species composition of CDV reservoirs depends on local conditions, and in other areas large populations of unvaccinated and free-roaming domestic dogs (Canis familiaris) may constitute important CDV reservoirs (Acosta-Jamett et al., 2011; Belsare et al., 2014). Spillover could initiate short chains of tiger-tiger transmission, but these fade out rapidly in a species with few intra-specific contacts. Consequently, the extensive epizootics that occur in more social species are not a feature of CDV in tigers. Famously, the 1994 outbreak in Serengeti, Tanzania coincided with the disappearance of an estimated 1,000 lions (Roelke-Parker et al., 1996) and at least 68 lion cases occurred during an outbreak in Gujarat, India in 2018 (Mourya et al., 2019). Although less conspicuous, CDV in tigers can reach comparable scales. With more than a third of Amur tigers carrying antibodies that indicate prior infection (Gilbert et al., 2020), spillovers must be occurring with some regularity. In a population of between 265 and 486 tigers (Goodrich et al., 2022) the five CDV cases that have been confirmed to date clearly represent an underestimate of true mortality (Gilbert et al., 2020).
The most immediate priority for CDV research remains a risk assessment for tiger populations outside of Russia. Fundamentally, this requires two key questions to be addressed: “what proportion of tigers are exposed to CDV?,” and then, “is exposure at a level that threatens population viability?.” Collection of blood and preservation of serum should be routinely incorporated into tiger handling protocols (whether for research, conflict mitigation, rehabilitation, or post-mortem examination). Specimens should be archived centrally to maximize sample size and their statistical value. Commercial diagnostic kits for measuring CDV antibodies are designed for use in domestic dogs and should be considered unreliable for use in tigers without validation. Serum neutralization tests are available or have been introduced to several tiger range countries to measure titers of CDV neutralizing antibodies (Techakriengkrai et al., 2019; Mulia et al., 2021; Bodgener et al., 2023). Resulting seroprevelance estimates provide a useful means of validating population viability models based on the demography and structure of local tiger populations, which test the impact of realistic CDV exposure parameters (Gilbert et al., 2014, 2020). These could also be used to assess the impact of more frequent tiger-to-tiger contact that may occur in higher density populations outside Russia. Long-term serological monitoring would also highlight temporal variation in CDV exposure, such as the increase that occurred in Russia from 2000 onwards (Goodrich et al., 2012; Gilbert et al., 2020).
Vaccination is the only means of mitigating CDV risk to tiger populations and theoretically can be used in two ways, depending on the source of infection (Woodroffe, 1999; Haydon et al., 2002). In areas where domestic dogs are the primary source of CDV, vaccination of dogs using conventional modified live vaccines can be very effective at raising herd immunity and reducing CDV transmission to the point of achieving local elimination. However, this approach is ineffective at protecting tigers that acquire infection through contact with a sylvatic reservoir, as vaccines cannot be delivered to wildlife at the scale necessary to eliminate the virus. Under these circumstances, vaccination of tigers is the only option to reduce the threat to population viability (Gilbert et al., 2020). Captive tigers are routinely vaccinated against CDV (Sadler et al., 2016; Georoff et al., 2020), but the delivery of vaccines to free ranging animals presents additional logistical challenges and political controversy (Gilbert et al., 2020). Low coverage vaccination of tigers using injectable vaccines is feasible and would produce substantive reductions in extinction risks (Gilbert et al., 2020). Development of an oral baiting system (Budaszewski et al., 2017), could provide a less costly alternative and similar systems are already in wide use for control of rabies in wildlife.
Unraveling the structure of CDV reservoirs can be a complex undertaking and requires that multiple lines of molecular and immunological evidence be integrated from each of the populations that may be contributing (Viana et al., 2014). Considering the limited options for mitigation, a more realistic approach would attempt to achieve a qualitative assessment of the relative contribution of domestic versus wild sources of virus. Viral sequence data can be used to identify transmission pathways that connect host populations, and every effort should be made to maximize this source of information from all populations. This should include the collection of tissue (a minimum of brain, lung and lymph node) for RT-PCR analysis from every dead tiger that is handled regardless of presumed cause of death, to ensure that underlying conditions or contributory factors are not overlooked. Serology can also be used to investigate tiger exposure risk to dog densities.
3.2. Severe acute respiratory syndrome coronavirus 2
The emergence of SARS-CoV2 in Wuhan, China in 2019 and the global pandemic that ensued has led to millions of human cases and deaths worldwide. In March 2020, the first SARS-CoV2 infections were diagnosed in captive tigers and lions at the Bronx Zoo, New York (McAloose et al., 2020) and numerous other outbreaks have now been reported affecting tigers, lions and snow leopards at other institutions (Fernández-Bellon et al., 2021; Mishra et al., 2021; Mitchell et al., 2021; Grome et al., 2022; Wang et al., 2022). Most cases in Panthera spp. present with mild and transitory respiratory signs (coughing, labored breathing, nasal discharge, malaise) that resolve after a few days or weeks (Bartlett et al., 2021). However, mortalities have been reported, particularly in older animals (Mishra et al., 2021; ProMED Mail, 2021; Madhusoodanan, 2022). Species susceptibility depends on the structure of the angiotensin I converting enzyme 2 (ACE2) that is used by SARS-CoV2 as a receptor for host entry. The amino acid composition of Felid ACE2 predicts a greater susceptibility than that of other carnivores (Damas et al., 2020). To date, in the United States there have been 53 tigers diagnosed with SARS-CoV2 using RT-PCR in 17 states and the district of Columbia (United States Department of Agriculture–Animal and Plant Health Inspection Service, 2022). With a further 52 cases in lions and 13 in snow leopards, more SARS-CoV2 cases have been confirmed in Panthera spp. in the United States than domestic dogs and cats (Felis catus) combined (101 cases). To-date, American zoological institutions have reported the death of one lion and five snow leopards that tested positive for the virus.
With SARS-CoV2 now endemic worldwide it is important to consider the risk to free-living tiger populations. The scales of our understanding of zoonotic disease weigh heavily in favor of pathogen transmission from animals to people, with comparatively little research on those moving in the opposite direction (Fagre et al., 2022). However, there are already signs that SARS-CoV2 is becoming established in free-living mink (Neovison vison) (Aguiló-Gisbert et al., 2021) and the detection in a dead wild leopard in India is a cause for concern (Mahajan et al., 2022). The most alarming ‘spillback’ into wildlife has been that affecting white-tailed deer (Odocoileus virginianus) in North America. Although the mechanism of initial exposure remains unknown, numerous independent human-deer transmission events with subsequent deer-deer transmission has raised the prospect of a possible SARS-CoV2 reservoir in white-tailed deer (Chandler et al., 2021; Hale et al., 2022; Kuchipudi et al., 2022). In Iowa, deer infections peaked at 82.5% (n = 97 deer sampled) during hunting season, soon after a surge in human cases in the state (Kuchipudi et al., 2022). Infected deer do not develop clinical signs, but they do shed virus for prolonged periods (at least 3 weeks) (Palmer et al., 2021) and the accumulation of 76 mutations in a strain detected in Canadian white-tailed deer suggest sustained transmission in deer populations with potential for generating novel variants (Pickering et al., 2022).
The susceptibility of tigers to SARS-CoV2 and its establishment in wild deer populations is concerning, but implications for tiger conservation remain unclear. While swine are poor hosts for SARS-CoV2, the deer that comprise the remainder of tiger diet are likely to be of comparable susceptibility as white-tailed deer (Damas et al., 2020) and therefore a plausible source of infection for wild tigers. However, at the time of writing transmission from deer to other wildlife has yet to be demonstrated. Furthermore, surveillance has yet to identify infection in European deer populations (Holding et al., 2022; Krupińska et al., 2022; Moreira-Soto et al., 2022) and so viral establishment in Asian deer is far from assured. Ecologically, few native deer in tiger range are as gregarious as white-tailed deer, with less extensive human contact, providing fewer opportunities for viral introduction or spread. Only chital (Axis axis) reach comparable densities, yet their populations are concentrated in protected areas with few settlements. Still, opportunities for contact (e.g., through hand feeding by tourists) should be avoided for this and other reasons. The limited prominence of deer hunting in most tiger range states may also hinder transmission, although the role of supplementary feeding or other hunting-related activities remains speculative (Kuchipudi et al., 2022).
If SARS-CoV2 did become endemic in Asian deer populations, the exposure of wild tigers may be unavoidable. However, the population importance of adult mortality related to COVID-19 remains debatable. Although few tigers have died from COVID-19, mortality may be higher in wild settings where animals lack veterinary care and easy meals. Just as for CDV, any wild tiger mortalities would be diagnosed through the introduction of routine post-mortem examination of all dead tigers. Any COVID-19 deaths would only become relevant at a population level if they were additive to other causes of mortality. In strict conservation terms, the loss of old or debilitated tigers would be irrelevant. However, the events since the emergence of the pandemic caution against complacency, with a virus that is adept at generating new variants and exploiting novel hosts. The virulence of SARS-CoV2 in captive settings should be monitored, with importance also placed on surveillance to detect new reservoirs in deer and other species in tiger range countries.
4. Disease that reduces the productivity of breeding tigers
Tigers are late-maturing, slow breeding species, with females producing as few as 4–6 litters in a full reproductive lifespan (Smith and McDougal, 1991; Kerley et al., 2003). Cubs face a difficult life, with 34–47% perishing within their first year from a range of causes, including poaching, starvation (e.g., following poaching of the mother), predation, infanticide and random incidents like fire and flood (Smith and McDougal, 1991; Kerley et al., 2003). Health issues could also contribute to reduced reproductive performance through failure to conceive, carry pregnancy to parturition, or disease of dependent offspring, but are poorly documented in captive or wild tigers. Most of the factors responsible for reproductive failure in domestic cats, such as management or nutritional issues, endocrine disorders, and individual pathologies (Fontbonne et al., 2020), are unlikely to affect free-ranging tigers, particularly at the population level. However, the genetic and chromosomal disorders of domestic cats could have analogs in the inbreeding depression found in small, isolated tiger populations (Smith and McDougal, 1991; Khan et al., 2021; Ning et al., 2022). Infections also have the potential for wider population-level impacts and several viral, bacterial and protozoal pathogens are known to cause reproductive loss in domestic cats (Givens and Marley, 2008; Fontbonne et al., 2020). Most prominent among these are parvoviruses and retroviruses (e.g., feline leukemia virus [FeLV] and FIV), which cause abortions and stillbirths in pregnant cats (Givens and Marley, 2008; Fontbonne et al., 2020), however, FHV, FCV, Toxoplasma gondii (rarely) and a variety of bacteria have also been implicated (Givens and Marley, 2008). Both FIV and FeLV are rare in captive tigers (De la Cruz-Hernández et al., 2016; Liu et al., 2022), and with only one report of FIV in a free-ranging tiger (Naidenko et al., 2018),2 the status of infections in wild populations remains unclear. By contrast, high seroprevalence of parvovirus antibodies (65–73% Table 1) indicates that exposure is common in wild tigers and its significance warrants more detailed investigation.
4.1. Carnivore protoparvovirus 1
The highly contagious, single-stranded DNA virus carnivore protoparvovirus 1 (hereafter parvovirus) can infect a wide range of carnivores and is divided into two main genogroups: the feline parvoviruses (formerly termed feline panleukopenia virus) and canine parvovirus 2 (CPV2) (Allison et al., 2013, 2014). Both genogroups have been diagnosed in captive tigers (Steinel et al., 2000; Wang et al., 2019; Nur-Farahiyah et al., 2021; Huang et al., 2022), and CPV2 has been detected in tissues from a wild tiger in India (Shetty et al., 2020). The parvovirus genome is very small and lacks genes necessary for initiating viral replication, which is achieved through infection of rapidly dividing host cells (e.g., those found in developing fetuses or intestinal mucosa). Infection of pregnant cats can result in abortion, stillbirth, or neurological defects including cerebellar hypoplasia (that causes ‘feline ataxia syndrome’), or in hemorrhagic enteritis, vomiting and leukopenia in older animals (Steinel et al., 2001; Barrs, 2019). Clinical disease is most common in young animals, typically coinciding with the decline of maternal antibodies around 3–4 months of age, or younger for those born to unexposed mothers (Barrs, 2019). Disease in captive tigers has been recorded in cubs from 5 to 10 months (Duarte et al., 2009; Wang et al., 2017).
The conservation significance of parvovirus in tigers remains unclear. The high seroprevalence in adult tigers suggests that many infections may be mild or subclinical, but more severe disease and death may go undetected, occurring during pregnancy or in early life before cubs leave the den (Figure 1). Crucially, it is unclear whether parvovirus mortalities are additive, or mainly affect weaker individuals that are likely to have succumbed to other causes (termed compensatory mortality). Addressing this question is key to understanding the conservation importance of parvovirus but is notoriously difficult to achieve. Approaches require long term datasets of sufficient detail to correlate parvovirus incidence with breeding productivity. Alternatively, interventions (such as domestic dog vaccination) that might reduce sources of tiger infections could act as opportunistic experiments, by relating exposure to reproductive output. Notably, parvovirus exposure of African wild dog (Lycaon pictus) packs increases with proximity to settlements, suggesting a role for domestic dogs as source of CPV2 (Woodroffe et al., 2012). Whether the same applies to tigers and their exposure to both genogroups has yet to be examined.
Several long-term studies have attempted to relate parvovirus exposure to the population ecology of wild carnivores. Packer et al. (1999) were able to demonstrate three discrete outbreaks of parvovirus in Serengeti lions that followed periods of high population density, and occurred during years of average or low cub survival, and average or low female fecundity. However, clear patterns were hard to discern during a period when the population was exposed to waves of several epizootic viruses. A clearer picture emerges in Minnesota, where parvovirus infection in gray wolves (Canis lupus) reduced pup survival by 70%, depressing population growth and constraining recolonization (Mech et al., 2008). Parvovirus was also implicated in the decline of gray wolves in Isle Royale National Park, Michigan, reducing the population to a level from which it was unable to recover (Peterson et al., 1998). By integrating epidemiological and ecological research, these studies go further than simple description of pathogens, instead attempting to interpret their importance, and as such should serve as inspiration for those interested in tiger conservation.
5. Disease that reduces the carrying capacity of tiger habitat
The number of tigers that can be sustained in a landscape is directly proportional to the density of available prey species (Karanth et al., 2004; Hebblewhite et al., 2014; Miller et al., 2014). Tigers prefer large prey that are sufficient to satisfy the energetic needs of hunting and raising cubs (Miller et al., 2014). In most locations, wild boar (Sus scrofa) and large deer (e.g., sambar–Rusa unicolor, red deer–Cervus elaphus, and sika deer–C. nippon) are the most important prey species (Hayward et al., 2012) with banteng (Bos javanicus) and gaur (B. gaurus) replacing boar in Western Thailand (Pakpien et al., 2017). The depletion of prey populations due to unsustainable hunting is one of the primary threats to tiger conservation (Linkie et al., 2003; Aziz et al., 2017; Goodrich et al., 2022). Historically, major epizootics have been equally capable of causing lasting declines in prey populations, with consequent reductions in large carnivores (e.g., the impact of rinderpest in Africa) (Packer et al., 2005; Dures et al., 2019). Over time a reduction in prey density leads to an increase in home range requirements to satisfy energetic needs (Fuller and Sievert, 2001). This reduces available breeding territories, thus limiting the proportion of reproductively active females in the population and raising the age at first breeding. As available biomass declines, females are unable to provision their cubs, increasing juvenile mortality due to malnutrition and predation arising from extended hunting times, as well as reducing recruitment, which limits population size and jeopardizes viability (Fuller and Sievert, 2001; Figure 1).
Domestic livestock are an important source of the pathogens that infect tiger prey species (Supplementary Table S2), and complex global trade networks provide an efficient means for exotic pathogens to gain access to naïve populations. Contemporary examples include lumpy skin disease (LSD) caused by a capripox virus of African origin, which infects cattle and buffalo and is currently spreading across South and Southeast Asia (Namazi and Khodakaram Tafti, 2021). Also, incursions of foot and mouth disease (FMD) occur with some regularity in Southeast Asia, and in 2022 the virus was introduced into Indonesia for the first time in almost 40 years (Qiu et al., 2018; Chen et al., 2022). However, despite having grave implications for agricultural output and global trade, it is unlikely that either LSD or FMD will have a major impact in tiger prey availability. Although cases of LSD have been reported in banteng and gaur (Pruvot et al., 2023), mortality is low in most species (Namazi and Khodakaram Tafti, 2021). Similarly, wildlife mortalities from FMD are relatively uncommon (Gortázar et al., 2021; Ijaz et al., 2022).
Contemporaneous with the spread of FMD and LSD, another exotic pathogen, African swine fever virus (ASFV) that is capable of decimating wild boar populations and has spread throughout tiger range in the space of just 4 years, warranting specific attention.
5.1. African swine fever
The recent and rapid advance of African swine fever (ASF) across tiger range represents an immediate and potentially profound threat to the availability of wild boars as a prey resource for tigers. With mortality of infected domestic pigs (S. domesticus) and wild boar exceeding 90–95% (Penrith and Vosloo, 2009; Sauter-Louis et al., 2021), this viral disease has devastated the Asian pork market, threatens food security of backyard producers, and risks far-reaching impacts on terrestrial ecosystems (Woonwong et al., 2020; Ewers et al., 2021; Luskin et al., 2021). Endemic to Sub-Saharan Africa, ASF (genotype II) was introduced to Georgia in 2007, spreading locally among wild boars and backyard pig producers into neighboring states in the Caucuses and beyond to Ukraine, Belarus and the Russian Federation (Gogin et al., 2013). Following an introduction to the northeast Chinese province of Liaoning in August 2018, the virus spread rapidly across East and Southeast Asia through 2019 (Lu et al., 2020; Mighell and Ward, 2021). Outbreaks in domestic swine reached Myamnar and Northeast India by 2020, Bhutan by 2021 and by the time of writing (December 2022) have reached the northern and southern margins of the key tiger strongholds in the Western Ghats and Central India (Figure 2). Wild boar outbreaks are heavily under-reported (Vergne et al., 2020; Cadenas-Fernández et al., 2022), with concentrations in the Russian Far East, Republic of Korea and Peninsular Malaysia hinting at the scale of wild outbreaks elsewhere (Figure 2). The population implications of ASF for wild boars in tiger habitat remains largely anecdotal, but declines of at least 90% in Sikhote-Alin Biosphere Zapovednik in Russia (Waller et al., 2022) mirrors the situation in Europe (Morelle et al., 2020; Klich et al., 2021; Schulz et al., 2021). If these reports are indicative of the threat to wild boar in other regions, the population and ecological implications could be devastating.
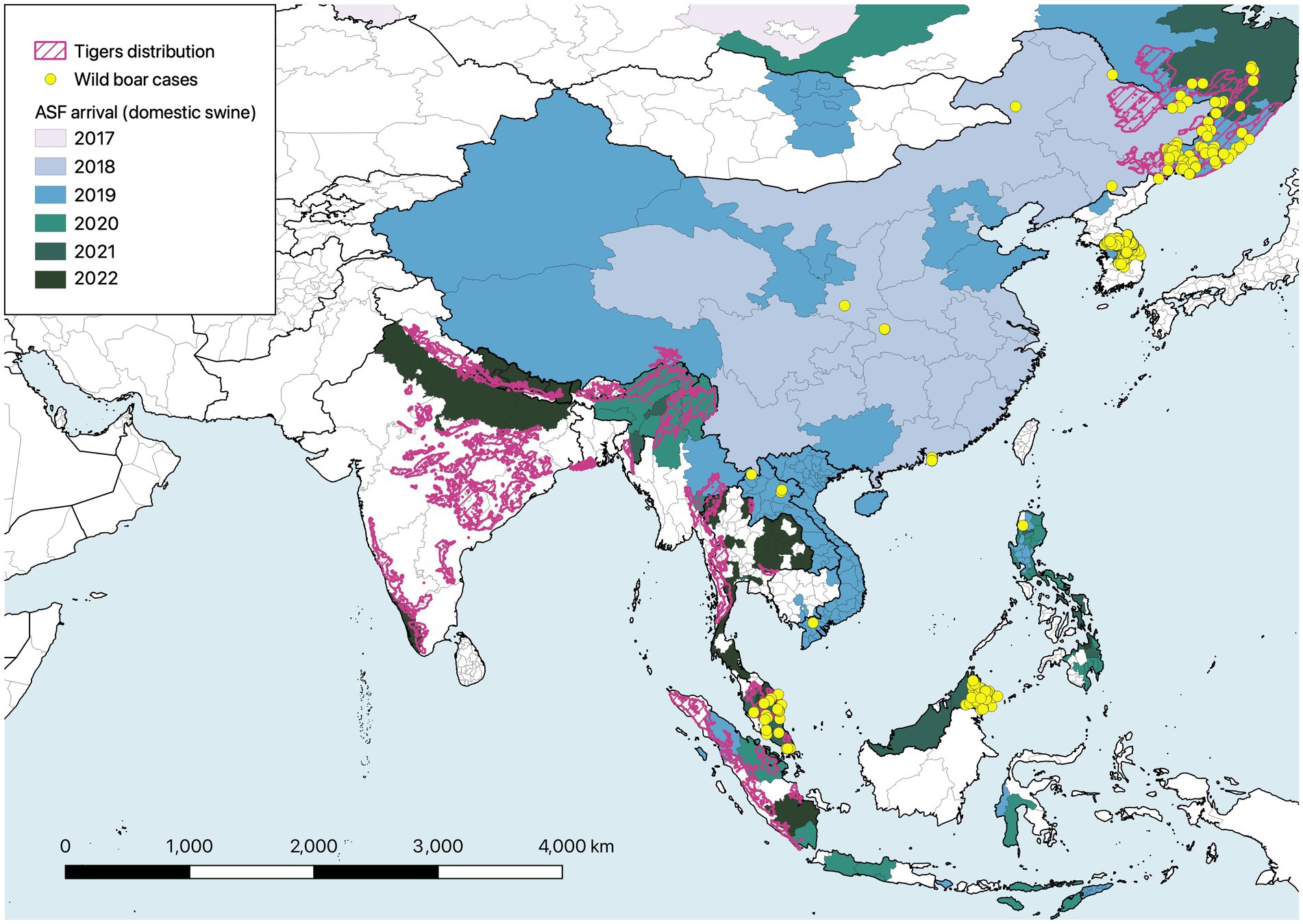
Figure 2. Map illustrating the spread of African swine fever (ASF) across Asia in relation to current tiger distribution (IUCN SSC Cat Specialist Group, 2022). Colors illustrate the year of first detection in domestic pigs at the province level (or equivalent) between August 2018 and December 2022. Location of ASF cases in wild boars are illustrated by yellow points. Source of ASF data: World Organisation for Animal Health - World Animal Health Information System (https://wahis.woah.org/#/event-management), accessed 6 February 2023, and Food and Agriculture Organization of the United Nations - EMPRES-i+ Global Animal Disease Information System (https://empres-i.apps.fao.org/diseases), accessed 3 March 2023.
The disease is caused by a unique, large, double-stranded DNA virus in the family Asfarviridae that evolved to infect warthogs (Phacochoerus africanus) in Eastern and Southern Africa (Penrith et al., 2019). In the natural host, infections are asymptomatic, and the virus is transmitted within burrows by soft-bodied, argasid ticks of the genus Ornithidoros. The virus replicates more efficiently in Sus spp. and can be contracted with or without the involvement of tick vectors (Penrith and Vosloo, 2009). Transmission can occur during direct contact via oro-nasal secretions, or indirectly through oral consumption of contaminated material. The latter is aided due to prolonged environmental stability, with virions remaining viable for weeks or months in blood, tissue, or decomposing remains. Wild boars that investigate infected carcasses promote the gradual spread of local outbreaks, while human transport of contaminated products (feed, meat, offal) and fomites can introduce infection to more remote areas (Guberti et al., 2019; Sauter-Louis et al., 2021). The virus causes a hemorrhagic fever, with infected boar and pigs exhibiting a range of non-specific clinical signs including listless behavior, lack of fear, ataxia, prostration, respiratory distress, vomiting, diarrhea and reddened or hemorrhagic skin lesions (Nurmoja et al., 2017; Guberti et al., 2019; Sauter-Louis et al., 2021). Most animals die within 7–14 days post-infection.
In all likelihood, ASF is now established in all tiger range countries and there is an urgent need to assess its impact on carrying capacity within Tiger Conservation Landscapes (Sanderson et al., 2006). In northern latitudes, ASF has decimated local boar populations (Sauter-Louis et al., 2021), but it may be premature to assume that the same will happen across all of the climatic extremes that tigers occupy. However, if we are to assume a worst-case scenario where ASF becomes endemic and wild boar densities stabilize at much lower levels, there could be serious implications for tiger conservation policy. Wild boar are a key prey resource in almost all of tiger range (Hayward et al., 2012). Elsewhere, another top predator, the gray wolf has been forced to switch to alternative prey species (Klich et al., 2021), but the ability for tigers to follow suit will depend on the diversity and biomass of local ungulates. In some regions, like Peninsular Malaysia and Sumatra, where numbers of large deer have already been heavily depleted (Kawanishi and Sunquist, 2004; Sunquist, 2010), the additional loss of wild boar could restrict tigers to hunting smaller prey that would be energetically unsustainable and constrain reproductive output (Miller et al., 2014). Reduced prey densities could also drive increases in livestock depredation and the risk of retaliatory killings (Soh et al., 2014), increase the frequency of human-tiger encounters, or promote contact with domestic dogs, potentially exacerbating exposure to CDV.
The future implications of ASF for tigers will depend on the capacity of Asian wild boar populations to maintain the virus in the long term. In Eastern Europe wild boar are capable of maintaining ASFV, even at very low population densities, independent from spillover from domestic sources (Kolbasov et al., 2018; Blokhin et al., 2020; Sauter-Louis et al., 2021). However, previously the introduction of ASF into the Iberian Peninsula faded out in wild boar (Pérez et al., 1998), and viral circulation in Sardinia is only maintained due to regular spillover from local farms and free-roaming domestic pigs (Mur et al., 2016; Jurado et al., 2018). The key factor that allows sylvatic transmission cycles to persist in Eastern Europe appears to be the extended stability of ASFV in the environment (O’Neill et al., 2020). In Spain, the rapid consumption of carcasses by vultures and other scavengers reduced environmental contamination (O’Neill et al., 2020). Viral viability is also markedly reduced in the temperate Mediterranean climate. Experiments measuring the viability of ASFV in tissues estimate a half-life of 353–713 days at −20°C, 35–136 days at +4°C, and just 9–17 days at +23°C (Mazur-Panasiuk and Woźniakowski, 2020). For comparative purposes, mean temperatures in Spain vary from 6.3–23.1°C and in Lithuania from −3.3-18.3°C (World Bank, 2022). While other factors such as substrate type and moisture may also influence viral longevity (World Bank, 2022), these findings suggest that the persistence of sylvatic ASF cycles may be less likely in southern tiger range states. In these circumstances wild boar outbreaks would only continue with regular spillover from domestic pigs that are rare or absent in several key locations for tigers (e.g., Bangladesh, Bhutan, Sumatra, Peninsular Malaysia and Central/Southern India) (Gilbert et al., 2018).
The control of ASF in wild boars seeks to disrupt the cycle of viral transmission, which can be achieved in three primary ways:
a. Reduce opportunities for viral introduction – The introduction of ASFV into a landscape can occur either through the gradual progression of wild boar outbreaks from outside the area (an epidemic wave), or introduction from anthropogenic sources, such as spillover from infected pig farms, or movement of contaminated material by people. In Europe, epidemic waves travel at approximately 2–3 km/month (Guberti et al., 2019) and this can only be disrupted by reducing susceptible boar density ahead of the wave (undesirable and impractical for reasons given below). Spillover from domestic sources could be reduced through biosecurity improvements around farms to prevent contact between pigs and boars, as well as measures to discourage movement of contaminated materials from outside the area (from either agricultural or hunting sources). Programs to raise awareness among farmers and hunters are important, with benefits for rural livelihoods as well as wildlife.
b. Reduce densities of susceptible wild boars – This strategy seeks to reduce opportunities for viral transmission, such that the probability of the average infected case successfully passing the virus to a susceptible boar is less than one. Theoretically, vaccination could reduce density of susceptible animals, but currently no ASFV vaccines are available. In Czechia and Belgium local depopulation has been used to prevent the invasion of ASFV following the initial detection of cases (Guberti et al., 2019; Sauter-Louis et al., 2021). This intensive strategy requires a sensitive surveillance system to detect cases early and an efficient means of rapid depopulation to prevent epizootic spread (such as the deployment of sharpshooters). Progression to an epizootic occurs quickly, and once established, the number of infected boars increases very rapidly, and it is no longer possible to eliminate the pathogen using depopulation.
c. Reduce sources of infection – The environmental stability of ASFV is key to its circulation in wild boars. Carcasses and surrounding substrate can remain infective for extended periods and are actively visited by inquisitive boars, thus facilitating transmission (Probst et al., 2017). Early detection and disposal of carcasses is the only way to reduce the amount of virus in a landscape. However, this is a formidable task amid an epizootic when the number of carcasses is high, and many go undetected. Once the initial epizootic subsides, the number of infected carcasses declines and the task of removal becomes more manageable and promotes the likelihood of viral fade out and elimination (Guberti et al., 2019).
Clearly, strategies that rely on depopulation are counterproductive when the objective of disease control is to preserve tiger prey populations. Even in Europe, where disease control measures are motivated by economic and trade considerations, depopulation has only been used under specific circumstances to prevent the establishment of focal outbreaks. Once the virus becomes enzootic, persistence is possible due to the prolonged infectivity of carcasses, as these can continue to seed new cases even once boar densities are heavily depleted.
The establishment of ASF across Asia presents formidable challenges that can only be met through partnerships built on the common interests of the livestock, veterinary and wildlife sectors. No single agency can address pathogens like ASFV, and the wildlife sector has an important role to enhance our understanding of the virus and its management across tiger range. Existing schemes such as WildHealthNet (Pruvot et al., 2023) that take a One Health approach, integrating animal, public health and environmental bodies provide a template for expansion and adaptation in range countries. These initiatives capitalize on the field presence and ecological awareness of rangers in protected areas for the early detection of cases and connects them with veterinary authorities with the necessary diagnostic capacity and expertise in the safe disposal of carcasses. Research on the population ecology of wild boars and other key prey species should be emphasized, with results integrated into epidemiological models that inform control strategies (Bosch et al., 2017; O’Neill et al., 2020; Pepin et al., 2020). Results could also be used to design management interventions to promote the availability of alternative tiger prey as a contingency against declining boar numbers. Other priorities include an assessment of local vector communities to determine whether alternative hosts could contribute to viral spread in new environments (Karalyan et al., 2019).
Ultimately, only time will tell how ASF will affect the tiger prey base throughout the species’ range. Environmental conditions and trends in domestic pig production across the wider landscape may have an important role in determining the eventual outcome for tigers. In the meantime, bleak predictions and the enormity of the challenge should not be used as grounds for complacency, as wildlife professionals have an opportunity to make critically-important contributions to an otherwise intractable One Health issue – one that could have serious implications for the long-term viability of some free-ranging tiger populations.
6. Conclusion
Despite their iconic and endangered status, we still know remarkably little about the health of free-ranging tigers. To some extent this may be explained by the suite of existing tiger conservation challenges, as long-standing politically charged questions of land tenure, wildlife conflict and indigenous rights often dominate discourse. Government officials may be reluctant to look for additional problems, like disease particularly if they are deemed to be convoluted and challenging to resolve. Equally, many established members of the conservation community may be skeptical, perceiving health as an unnecessary distraction that sits uncomfortably outside their own field of expertise. Yet, until we can reverse the declines and isolation of remaining tiger populations, disease threats are only going to become more evident. Despite all of this, there is still room for optimism. Opportunities abound to integrate wildlife health research into existing environmental management programs in ways that enhance their value to the wider community while also meeting conservation objectives.
Opportunistic sampling of tigers could (and should) be readily introduced into existing management activities with only modest financial investment. Sample collection should become part of the routine of tiger immobilizations (serum and whole blood), and all dead tigers should undergo a detailed post-mortem examination (with preservation of representative sets of tissues). While some wildlife management agencies are equipped with laboratories, these are often focused on forensic analysis or molecular research and may lack the capacity to perform the full suite of diagnostic testing required. In these situations, partnership with other agencies, or with national academic institutions should be encouraged to improve laboratory access, while also enhancing national educational opportunities and inter-sectoral communication. Given that most of the pathogens of relevance to tiger conservation are shared with domestic animals and/or humans there are mutual interests for the wildlife sector to partner with their livestock and public health counterparts. Wildlife agencies have access to species and places beyond the reach of veterinary or public health resources and can benefit from specialist assistance in diagnostics or health services, while enhancing surveillance of priority pathogens with One Health implications.
While there are many gaps to fill in our understanding of tiger health, researchers should be encouraged to focus on those issues of the greatest consequences for tigers and their prey. This should include elements of horizon scanning to be ready for the next ASFV or SARS-CoV2, as well as long term efforts to diagnose mortality causes, or changing patterns of pathogen exposure in serological profiles. This should not be viewed as distinct from traditional areas of conservation research. Instead, health research should be integrated into ongoing ecological, demographic investigations, thereby contributing to a more holistic understanding of tiger ecology and conservation. As an example, our ability to monitor and mitigate the effects of ASF will require more detailed understanding of boar ecology that will, in turn, inform our management of prey resources. Ultimately, population health is integral to the ecology of, and future for wild tigers and enhancing capacity in health research greatly enhances our ability to conserve the species.
Data availability statement
The bibliography of citations analyzed for this study can be found in the Cornell University eCommons repository: https://doi.org/10.7298/rpe5-wa81.
Author contributions
MG, ZD-R, and JB conceptualized the study. MG and ZD-R conducted the literature review. MG wrote the first draft of the manuscript. All authors contributed to manuscript revision, read, and approved the submitted version.
Funding
The study was supported by the Cornell Wildlife Health Center and the Cornell Feline Health Center, Cornell University.
Conflict of interest
The authors declare that the research was conducted in the absence of any commercial or financial relationships that could be construed as a potential conflict of interest.
Publisher’s note
All claims expressed in this article are solely those of the authors and do not necessarily represent those of their affiliated organizations, or those of the publisher, the editors and the reviewers. Any product that may be evaluated in this article, or claim that may be made by its manufacturer, is not guaranteed or endorsed by the publisher.
Supplementary material
The Supplementary material for this article can be found online at: https://www.frontiersin.org/articles/10.3389/fevo.2023.1135935/full#supplementary-material
Footnotes
1. ^While extended convalescence may be possible in captivity (Blythe et al., 1983), this is unlikely for tigers in the wild.
2. ^The authors used an ELISA test that has not been validated for use in tigers (Barr, 1996; Hartmann et al., 2007).
References
Acosta-Jamett, G., Chalmers, W. S. K., Cunningham, A. A., Cleaveland, S., Handel, I. G., and deC Bronsvoort, B. M. (2011). Urban domestic dog populations as a source of canine distemper virus for wild carnivores in the Coquimbo region of Chile. Vet. Microbiol. 152, 247–257. doi: 10.1016/j.vetmic.2011.05.008
Aguiló-Gisbert, J., Padilla-Blanco, M., Lizana, V., Maiques, E., Muñoz-Baquero, M., Chillida-Martínez, E., et al. (2021). First description of SARS-CoV-2 infection in two feral American mink (Neovison vison) caught in the wild. Animals 11, 1–13. doi: 10.3390/ani11051422
Allison, A. B., Kohler, D. J., Fox, K. A., Brown, J. D., Gerhold, R. W., Shearn-Bochsler, V. I., et al. (2013). Frequent cross-species transmission of parvoviruses among diverse carnivore hosts. J. Virol. 87, 2342–2347. doi: 10.1128/JVI.02428-12
Allison, A. B., Kohler, D. J., Ortega, A., Hoover, E. A., Grove, D. M., Holmes, E. C., et al. (2014). Host-specific parvovirus evolution in nature is recapitulated by in vitro adaptation to different carnivore species. PLoS Pathog. 10:e1004475. doi: 10.1371/journal.ppat.1004475
Appel, M. J. G., Yates, R. A., Foley, G. L., Bernstein, J. J., Santinelli, S., Spelman, L. H., et al. (1994). Canine distemper epizootic in lions, tigers, and leopards in North America. J. Vet. Diagn. Investig. 6, 277–288. doi: 10.1177/104063879400600301
Aziz, M. A., Tollington, S., Barlow, A., Goodrich, J., Shamsuddoha, M., Islam, M. A., et al. (2017). Investigating patterns of tiger and prey poaching in the Bangladesh Sundarbans: implications for improved management. Glob. Ecol. Conserv. 9, 70–81. doi: 10.1016/j.gecco.2016.12.001
Barr, M. C. (1996). FIV, FeLV, and FIPV: interpretation and misinterpretation of serological test results. Semin. Vet. Med. Surg. 11, 144–153. doi: 10.1016/s1096-2867(96)80026-0
Barrs, V. R. (2019). Feline panleukopenia: A re-emergent disease. Vet. Clin. North Am. Small Anim. Pract. 49, 651–670. doi: 10.1016/j.cvsm.2019.02.006
Bartlett, S. L., Diel, D. G., Wang, L., Zec, S., Laverack, M., Martins, M., et al. (2021). SARS-CoV-2 infection and longitudinal fecal screening in Malayan tigers (Panthera tigris jacksoni), Amur tigers (Panthera tigris altaica), and African lions (Panthera leo krugeri) at the Bronx zoo, New York, USA. J. Zoo Wildl. Med. 51, 733–744. doi: 10.1638/2020-0171
Belsare, A. V., Vanak, A. T., and Gompper, M. E. (2014). Epidemiology of viral pathogens of free-ranging dogs and Indian foxes in a human-dominated landscape in Central India. Transbound. Emerg. Dis. 61, 78–86. doi: 10.1111/tbed.12265
Blokhin, A., Toropova, N., Burova, O., Sevskikh, T., Gogin, A., Debeljak, Z., et al. (2020). Spatio-temporal analysis of the spread of ASF in the Russian Federation in 2017-2019. Acta Vet. Brno 70, 194–206. doi: 10.2478/acve-2020-0014
Blythe, L. L., Schmitz, J. A., Roelke, M., and Skinner, S. (1983). Chronic encephalomyelitis caused by canine-distemper virus in a Bengal tiger. J. Am. Vet. Med. Assoc. 183, 1159–1162.
Bodgener, J., Sadaula, A., Thapa, P. J., Shrestha, B. K., Gairhe, K. P., Subedi, S., et al. (2023). Canine distemper virus in tigers (Panthera tigris) and leopards (P. pardus) in Nepal. Pathogens 12:203. doi: 10.3390/pathogens12020203
Bosch, J., Iglesias, I., Muñoz, M. J., and de la Torre, A. (2017). A cartographic tool for managing African swine fever in Eurasia: mapping wild boar distribution based on the quality of available habitats. Transbound. Emerg. Dis. 64, 1720–1733. doi: 10.1111/tbed.12559
Brown, E. W., Miththapala, S., and Obrien, S. J. (1993). Prevalence of exposure to feline immunodeficiency virus in exotic felid species. J. Zoo Wildl. Med. 24, 357–364.
Budaszewski, R. D. F., Hudacek, A., Sawatsky, B., Krämer, B., Xiangping, Y., Schnell, M. J., et al. (2017). Inactivated recombinant rabies viruses displaying the canine distemper virus glycoproteins induce protective immunity against both pathogens. J. Virol. 91, e02077–e02016. doi: 10.1128/JVI.02077-16
Cadenas-Fernández, E., Ito, S., Aguilar-Vega, C., Sánchez-Vizcaíno, J. M., and Bosch, J. (2022). The role of the wild boar spreading African swine fever virus in Asia: another underestimated problem. Front. Vet. Sci. 9:844209. doi: 10.3389/fvets.2022.844209
Chandler, J. C., Bevins, S. N., Ellis, J. W., Linder, T. J., Tell, R. M., Jenkins-Moore, M., et al. (2021). SARS-CoV-2 exposure in wild white-tailed deer (Odocoileus virginianus). Proc. Natl. Acad. Sci. U. S. A. 118, 1–3. doi: 10.1073/pnas.2114828118
Chapron, G., Miquelle, D. G., Lambert, A., Goodrich, J. M., Legendre, S., and Clobert, J. (2008). The impact on tigers of poaching versus prey depletion. J. Appl. Ecol. 45, 1667–1674. doi: 10.1111/j.1365-2664.2008.01538.x
Chen, R., Gardiner, E., and Quigley, A. (2022). Foot and mouth disease outbreak in Indonesia: summary and implications. Glob. Biosecur. 4, 1–25. doi: 10.31646/gbio.175
Damas, J., Hughes, G. M., Keough, K. C., Painter, C. A., Persky, N. S., Corbo, M., et al. (2020). Broad host range of SARS-CoV-2 predicted by comparative and structural analysis of ACE2 in vertebrates. Proc. Natl. Acad. Sci. U. S. A. 117, 22311–22322. doi: 10.1073/pnas.2010146117
De la Cruz-Hernández, N. I., Merino-Charres, J. O., Salinas-Navarrete, E. M., Garcia, A. E. M., Burnes, J. M., Lucio, J. A. R., et al. (2016). Amyloidosis associated with feline leukemia virus in a white Bengal tiger (Panthera tigris tigris). Thai J. Vet. Med. 46:679.
Duarte, M. D., Barros, S. C., Henriques, M., Fernandes, T. L., Bernardino, R., Monteiro, M., et al. (2009). Fatal infection with feline panleukopenia virus in two captive wild carnivores (Panthera tigris and Panthera leo). J. Zoo Wildl. Med. 40, 354–359. doi: 10.1638/2008-0015.1
Dures, S. G., Carbone, C., Loveridge, A. J., Maude, G., Midlane, N., Aschenborn, O., et al. (2019). A century of decline: loss of genetic diversity in a Southern African lion-conservation stronghold. Divers. Distrib. 25, 870–879. doi: 10.1111/ddi.12905
Ewers, R. M., Nathan, S. K. S. S., and Lee, P. A. K. (2021). African swine fever ravaging Borneo’s wild pigs. Nature 593:37. doi: 10.1038/d41586-021-01189-3
Fagre, A. C., Cohen, L. E., Eskew, E. A., Farrell, M., Glennon, E., Joseph, M. B., et al. (2022). Assessing the risk of human-to-wildlife pathogen transmission for conservation and public health. Ecol. Lett. 25, 1534–1549. doi: 10.1111/ele.14003
Fernández-Bellon, H., Rodon, J., Fernández-Bastit, L., Almagro, V., Padilla-Solé, P., Lorca-Oró, C., et al. (2021). Monitoring natural SARS-CoV-2 infection in lions (Panthera leo) at the Barcelona zoo: viral dynamics and host responses. Viruses 13:1683. doi: 10.3390/v13091683
Fontbonne, A., Prochowska, S., and Niewiadomska, Z. (2020). Infertility in purebred cats–a review of the potential causes. Theriogenology 158, 339–345. doi: 10.1016/j.theriogenology.2020.09.032
Fuller, T. K., and Sievert, P. R. (2001). “Carnivore demography and the consequences of changes in prey availability” in Carnivore Conservation. eds. J. L. Gittleman, S. M. Funk, D. Macdonald, and R. K. Wayne (Cambridge, United Kingdom: Cambridge University Press), 163–178.
Georoff, T. A., Ramsay, E. C., Gyimesi, Z. S., Kilburn, J. J., and Sykes, J. M. (2020). Review of canine distemper vaccination use and safety in north American captive large felids (Panthera spp.) from 2000 to 2017. J. Zoo Wildl. Med. 50, 778–789. doi: 10.1638/2018-0163
Gilbert, M., Miquelle, D. G., Goodrich, J. M., Reeve, R., Cleaveland, S., Matthews, L., et al. (2014). Estimating the potential impact of canine distemper virus on the Amur tiger population (Panthera tigris altaica) in Russia. PLoS One 9:e110811. doi: 10.1371/journal.pone.0110811
Gilbert, M., Nicolas, G., Cinardi, G., Van Boeckel, T. P., Vanwambeke, S. O., Wint, G. R. W., et al. (2018). Global distribution data for cattle, buffaloes, horses, sheep, goats, pigs, chickens and ducks in 2010. Sci. Data 5:180227. doi: 10.1038/sdata.2018.227
Gilbert, M., Soutrina, S., Seryodkin, I., Sulikhan, N., Uphyrkina, O. V., Goncharuk, M., et al. (2015). Canine distemper virus as a threat to wild tigers in Russia and across their range. Integr. Zool. 10, 329–343. doi: 10.1111/1749-4877.12137
Gilbert, M., Sulikhan, N., Uphyrkina, O., Goncharuk, M., Kerley, L., Hernandez Castro, E., et al. (2020). Distemper, extinction, and vaccination of the Amur tiger. Proc. Natl. Acad. Sci. 117, 31954–31962. doi: 10.1073/pnas.2000153117
Givens, M. D., and Marley, M. S. D. (2008). Infectious causes of embryonic and fetal mortality. Theriogenology 70, 270–285. doi: 10.1016/j.theriogenology.2008.04.018
Gogin, A., Gerasimov, V., Malogolovkin, A., and Kolbasov, D. (2013). African swine fever in the North Caucasus region and the Russian Federation in years 2007-2012. Virus Res. 173, 198–203. doi: 10.1016/j.virusres.2012.12.007
Goodrich, J. M., Kerley, L. L., Smirnov, E. N., Miquelle, D. G., McDonald, L., Quigley, H. B., et al. (2008). Survival rates and causes of mortality of Amur tigers on and near the Sikhote-Alin biosphere Zapovednik. J. Zool. 276, 323–329. doi: 10.1111/j.1469-7998.2008.00458.x
Goodrich, J. M., Miquelle, D. G., Smirnov, E. N., Kerley, L. L., Quigley, H. B., and Hornocker, M. G. (2010). Spatial structure of Amur (Siberian) tigers (Panthera tigris altaica) on Sikhote-Alin biosphere Zapovednik, Russia. J. Mammal. 91, 737–748. doi: 10.1644/09-MAMM-A-293.1
Goodrich, J. M., Quigley, K. S., Lewis, J. C. M., Astafiev, A. A., Slabi, E. V., Miquelle, D. G., et al. (2012). Serosurvey of free-ranging Amur tigers in the Russian Far East. J. Wildl. Dis. 48, 186–189. doi: 10.7589/0090-3558-48.1.186
Goodrich, J., Wibisono, H., Miquelle, D., Lynam, A. J., Sanderson, E., Chapman, S., et al. (2022). Panthera tigris. IUCN Red List Threat. Species 2022, e.T15955A214862019.
Gortázar, C., Barroso, P., Nova, R., and Cáceres, G. (2021). The role of wildlife in the epidemiology and control of foot-and-mouth-disease and similar transboundary (FAST) animal diseases: a review. Transbound. Emerg. Dis. 69, 2462–2473. doi: 10.1111/tbed.14235
Grome, H. N., Meyer, B., Read, E., Buchanan, M., Cushing, A., Sawatzki, K., et al. (2022). SARS-CoV-2 outbreak among Malayan tigers and humans, Tennessee, USA, 2020. Emerg. Infect. Dis. 28, 833–836. doi: 10.3201/eid2804.212219
Guberti, V., Khomenko, S., Masiulis, M., and Kerba, S.. (2019). African Swine Fever in Wild Boar Ecology and Biosecurity. FAO Animal Production and Health Manual No. 22. Rome: FAO, OIE and EC.
Hale, V. L., Dennis, P. M., McBride, D. S., Nolting, J. M., Madden, C., Huey, D., et al. (2022). SARS-CoV-2 infection in free-ranging white-tailed deer. Nature 602, 481–486. doi: 10.1038/s41586-021-04353-x
Hartmann, K., Griessmayr, P., Schulz, B., Greene, C. E., Vidyashankar, A. N., Jarrett, O., et al. (2007). Quality of different in-clinic test systems for feline immunodeficiency virus and feline leukaemia virus infection. J. Feline Med. Surg. 9, 439–445. doi: 10.1016/j.jfms.2007.04.003
Haydon, D. T., Laurenson, M. K., and Sillero-Zubiri, C. (2002). Integrating epidemiology into population viability analysis: managing the risk posed by rabies and canine distemper to the Ethiopian wolf. Conserv. Biol. 16, 1372–1385. doi: 10.1046/j.1523-1739.2002.00559.x
Hayward, M. W., Jedrzejewski, W., and Jedrzejewska, B. (2012). Prey preferences of the tiger Panthera tigris. J. Zool. 286, 221–231. doi: 10.1111/j.1469-7998.2011.00871.x
Hebblewhite, M., Miquelle, D. G., Robinson, H., Pikunov, D. G., Dunishenko, Y. M., Aramilev, V. V., et al. (2014). Including biotic interactions with ungulate prey and humans improves habitat conservation modeling for endangered Amur tigers in the Russian Far East. Biol. Conserv. 178, 50–64. doi: 10.1016/j.biocon.2014.07.013
Holding, M., Otter, A. D., Dowall, S., Takumi, K., Hicks, B., Coleman, T., et al. (2022). Screening of wild deer populations for exposure to SARS-CoV-2 in the United Kingdom, 2020–2021. Transbound. Emerg. Dis. 69, e3244–e3249. doi: 10.1111/tbed.14534
Huang, S., Li, X., Xie, W., Guo, L., You, D., Xu, H., et al. (2022). Molecular detection of parvovirus in captive Siberian tigers and lions in northeastern China from 2019 to 2021. Front. Microbiol. 13:898184. doi: 10.3389/fmicb.2022.898184
Ijaz, M., Ali, M. M., Awan, F., Ishaq, M., and Ahmad, A. (2022). FMD virus spillover from domestic livestock caused outbreak in captive wild ungulates: first report from Pakistan. Acta Trop. 231:106439. doi: 10.1016/j.actatropica.2022.106439
IUCN SSC Cat Specialist Group. (2022). The IUCN Red List of Threatened Species. Version 2022–2. Available at https://www.iucnredlist.org Accessed September 22, 2022).
Jurado, C., Fernández-Carrión, E., Mur, L., Rolesu, S., Laddomada, A., and Sánchez-Vizcaíno, J. M. (2018). Why is African swine fever still present in Sardinia? Transbound. Emerg. Dis. 65, 557–566. doi: 10.1111/tbed.12740
Kadam, R. G., Karikalan, M., Siddappa, C. M., Mahendran, K., Srivastava, G., Rajak, K. K., et al. (2022). Molecular and pathological screening of canine distemper virus in Asiatic lions, tigers, leopards, snow leopards, clouded leopards, leopard cats, jungle cats, civet cats, fishing cat, and jaguar of different states, India. Infect. Genet. Evol. 98:105211. doi: 10.1016/j.meegid.2022.105211
Karalyan, Z., Avetisyan, A., Avagyan, H., Ghazaryan, H., Vardanyan, T., Manukyan, A., et al. (2019). Presence and survival of African swine fever virus in leeches. Vet. Microbiol. 237:108421. doi: 10.1016/j.vetmic.2019.108421
Karanth, K. U., Nichols, J. D., Kumar, N. S., Link, W. A., and Hines, J. E. (2004). Tigers and their prey: predicting carnivore densities from prey abundance. Proc. Natl. Acad. Sci. U. S. A. 101, 4854–4858. doi: 10.1073/pnas.0306210101
Kawanishi, K., and Sunquist, M. E. (2004). Conservation status of tigers in a primary rainforest of peninsular Malaysia. Biol. Conserv. 120, 329–344. doi: 10.1016/j.biocon.2004.03.005
Kerley, L. L., Goodrich, J. M., Miquelle, D. G., Smirnov, E. N., Quigley, H. B., Hornocker, M. G., et al. (2003). Reproductive parameters of wild female Amur (Siberian) tigers (Panthera tigris altaica). J. Mammal. 84, 288–298. doi: 10.1644/1545-1542(2003)084<0288:RPOWFA>2.0.CO;2
Khan, A., Patel, K., Shukla, H., Viswanathan, A., van der Valk, T., Borthakur, U., et al. (2021). Genomic evidence for inbreeding depression and purging of deleterious genetic variation in Indian tigers. Proc. Natl. Acad. Sci. 118:e2023018118. doi: 10.1073/pnas.2023018118
Klich, D., Yanuta, G., Sobczuk, M., and Balcerak, M. (2021). Indirect effect of African swine fever on the diet composition of the gray wolf Canis lupus—a case study in Belarus. Animals 11:1758. doi: 10.3390/ani11061758
Kolbasov, D., Titov, I., Tsybanov, S., Gogin, A., and Malogolovkin, A. (2018). African swine fever virus, Siberia, Russia, 2017. Emerg. Infect. Dis. 24, 796–798. doi: 10.3201/eid2404.171238
Krupińska, M., Borkowski, J., Goll, A., Nowicka, J., Baranowicz, K., Bourret, V., et al. (2022). Wild red deer (Cervus elaphus) do not play a role as vectors or reservoirs of SARS-CoV-2 in north-eastern Poland. Viruses 14, 1–7. doi: 10.3390/v14102290
Kuchipudi, S. V., Surendran-Nair, M., Ruden, R. M., Yon, M., Nissly, R. H., Vandegrift, K. J., et al. (2022). Multiple spillovers from humans and onward transmission of SARS-CoV-2 in white-tailed deer. Proc. Natl. Acad. Sci. U. S. A. 119, 1–8. doi: 10.1073/pnas.2121644119
Linhares, M. B., Whiteley, H. E., Samuelson, J. P., Hsiao, S. H., Stern, A. W., Sprandel, I. T., et al. (2021). Sylvatic canine morbillivirus in captive panthera highlights viral promiscuity and the need for better prevention strategies. Pathogens 10:544. doi: 10.3390/pathogens10050544
Linkie, M., Martyr, D. J., Holden, J., Yanuar, A., Hartana, A. T., Sugardjito, J., et al. (2003). Habitat destruction and poaching threaten the Sumatran tiger in Kerinci Seblat National Park, Sumatra. Oryx 37, 41–48. doi: 10.1017/S0030605303000103
Liu, E., Ma, L., Huang, S., You, D., Guo, L., Li, X., et al. (2022). The first feline immunodeficiency virus from Siberian tigers (Panthera tigris altaica) in northeastern China. Arch. Virol. 167, 545–551. doi: 10.1007/s00705-022-05370-5
Lu, G., Pan, J., and Zhang, G. (2020). African swine fever virus in Asia: its rapid spread and potential threat to unaffected countries. J. Infect. 80, 350–371. doi: 10.1016/j.jinf.2019.11.011
Luskin, M. S., Meijaard, E., Surya, S., Sheherazade, W. C., and Linkie, M. (2021). African swine fever threatens Southeast Asia’s 11 endemic wild pig species. Conserv. Lett. 14:e12784. doi: 10.1111/conl.12784
Madhusoodanan, J. (2022). Animal reservoirs–where the next SARS-CoV-2 variant could arise. J. Am. Med. Assoc. 328, 696–698. doi: 10.1001/jama.2022.9789
Mahajan, S., Karikalan, M., Chander, V., Pawde, A. M., Saikumar, G., Semmaran, M., et al. (2022). Detection of SARS-CoV-2 in a free ranging leopard (Panthera pardus fusca) in India. Eur. J. Wildl. Res. 68, 59–55. doi: 10.1007/s10344-022-01608-4
Mazur-Panasiuk, N., and Woźniakowski, G. (2020). Natural inactivation of African swine fever virus in tissues: influence of temperature and environmental conditions on virus survival. Vet. Microbiol. 242:108609. doi: 10.1016/j.vetmic.2020.108609
McAloose, D., Laverack, M., Wang, L., Killian, M. L., Caserta, L. C., Yuan, F., et al. (2020). From people to Panthera: natural SARS-CoV-2 infection in tigers and lions at the Bronx zoo. MBio 11, 1–13. doi: 10.1128/mBio.02220-20
McCauley, D., Stout, V., Gairhe, K. P., Sadaula, A., Dubovi, E., Subedi, S., et al. (2021). Serologic survey of selected pathogens in free-ranging Bengal tigers (Panthera tigris tigris) in Nepal. J. Wildl. Dis. 57, 393–398. doi: 10.7589/JWD-D-20-00046
Mech, L. D., Goyal, S. M., Paul, W. J., and Newton, W. E. (2008). Demographic effects of canine parvovirus on a free-ranging wolf population over 30 years. J. Wildl. Dis. 44, 824–836. doi: 10.7589/0090-3558-44.4.824
Mighell, E., and Ward, M. P. (2021). African swine fever spread across Asia, 2018–2019. Transbound. Emerg. Dis. 68, 2722–2732. doi: 10.1111/tbed.14039
Miller, C. S., Hebblewhite, M., Petrunenko, Y. K., Seryodkin, I. V., Goodrich, J. M., and Miquelle, D. G. (2014). Amur tiger (Panthera tigris altaica) energetic requirements: implications for conserving wild tigers. Biol. Conserv. 170, 120–129. doi: 10.1016/j.biocon.2013.12.012
Mishra, A., Kumar, N., Bhatia, S., Aasdev, A., Kanniappan, S., Sekhar, A. T., et al. (2021). SARS-CoV-2 delta variant among Asiatic lions, India. Emerg. Infect. Dis. 27, 2723–2725. doi: 10.3201/eid2710.211500
Mitchell, P. K., Martins, M., Reilly, T., Caserta, L. C., Anderson, R. R., Cronk, B. D., et al. (2021). SARS-CoV-2 B.1.1.7 variant infection in Malayan tigers, Virginia, USA. Emerg. Infect. Dis. 27, 3171–3173. doi: 10.3201/eid2712.211234
Moreira-Soto, A., Walzer, C., Czirják, G., Richter, M. H., Marino, S. F., Posautz, A., et al. (2022). Serological evidence that SARS-CoV-2 has not emerged in deer in Germany or Austria during the COVID-19 pandemic. Microorganisms 10, 5–10. doi: 10.3390/microorganisms10040748
Morelle, K., Bubnicki, J., Churski, M., Gryz, J., Podgórski, T., and Kuijper, D. P. J. (2020). Disease-induced mortality outweighs hunting in causing wild boar population crash after African swine fever outbreak. Front. Vet. Sci. 7. doi: 10.3389/fvets.2020.00378
Mourya, D. T., Yadav, P. D., Mohandas, S., Kadiwar, R. F., Vala, M. K., Saxena, A. K., et al. (2019). Canine distemper virus in Asiatic lions of Gujarat state, India. Emerg. Infect. Dis. 25, 2128–2130. doi: 10.3201/eid2511.190120
Mulia, B. H., Mariya, S., Bodgener, J., Iskandriati, D., Liwa, S. R., Sumampau, T., et al. (2021). Exposure of wild Sumatran tiger to canine distemper virus. J. Wildl. Dis. 57, 464–466. doi: 10.7589/JWD-D-20-00144
Mur, L., Atzeni, M., Martínez-López, B., Feliziani, F., Rolesu, S., and Sanchez-Vizcaino, J. M. (2016). Thirty-five-year presence of African swine fever in Sardinia: history, evolution and risk factors for disease maintenance. Transbound. Emerg. Dis. 63, e165–e177. doi: 10.1111/tbed.12264
Nagao, Y., Nishio, Y., Shiomoda, H., Tamaru, S., Shimojima, M., Goto, M., et al. (2012). An outbreak of canine distemper virus in tigers (Panthera tigris): possible transmission from wild animals to zoo animals. J. Vet. Med. Sci. 74, 699–705. doi: 10.1292/jvms.11-0509
Naidenko, S. V., Hernandez-Blanco, J. A., Erofeeva, M. N., Litvinov, M. N., and Rozhnov, V. V. (2019). Serum prevalence to non-viral pathogens in wild felids of southern Primorye, Russia. Nat. Conserv. Res. 4, 99–105. doi: 10.24189/ncr.2019.010
Naidenko, S. V., Hernandez-Blanco, J. A., Pavlova, E. V., Erofeeva, M. N., Sorokin, P. A., Litvinov, M. N., et al. (2018). Primary study of seroprevalence to virus pathogens in wild felids of south Primorie, Russia. Can. J. Zool. 96, 839–846. doi: 10.1139/cjz-2017-0192
Namazi, F., and Khodakaram Tafti, A. (2021). Lumpy skin disease, an emerging transboundary viral disease: a review. Vet. Med. Sci. 7, 888–896. doi: 10.1002/vms3.434
Ning, Y., Roberts, N. J., Qi, J., Peng, Z., Long, Z., Zhou, S., et al. (2022). Inbreeding status and implications for Amur tigers. Anim. Conserv. 25, 521–531. doi: 10.1111/acv.12761
Nur-Farahiyah, A. N., Kumar, K., Yasmin, A. R., Omar, A. R., and Camalxaman, S. N. (2021). Isolation and genetic characterization of canine parvovirus in a Malayan tiger. Front. Vet. Sci. 8, 1–12. doi: 10.3389/fvets.2021.660046
Nurmoja, I., Petrov, A., Breidenstein, C., Zani, L., Forth, J. H., Beer, M., et al. (2017). Biological characterization of African swine fever virus genotype II strains from North-Eastern Estonia in European wild boar. Transbound. Emerg. Dis. 64, 2034–2041. doi: 10.1111/tbed.12614
O’Neill, X., White, A., Ruiz-Fons, F., and Gortázar, C. (2020). Modelling the transmission and persistence of African swine fever in wild boar in contrasting European scenarios. Sci. Rep. 10:5895. doi: 10.1038/s41598-020-62736-y
Packer, C., Altizer, S., Appel, M., Brown, E., Martenson, J., O’Brien, S. J., et al. (1999). Viruses of the Serengeti: patterns of infection and mortality in African lions. J. Anim. Ecol. 68, 1161–1178. doi: 10.1046/j.1365-2656.1999.00360.x
Packer, C., Hilborn, R., Mosser, A., Kissui, B., Borner, M., Hopcroft, G., et al. (2005). Ecological change, group territoriality, and population dynamics in Serengeti lions. Science 307, 390–393. doi: 10.1126/science.1105122
Pakpien, S., Simcharoen, A., Duangchantrasiri, S., Chimchome, V., Pongpattannurak, N., and Smith, J. L. D. (2017). Ecological covariates at kill sites influence tiger (Panthera tigris) hunting success in Huai Kha Khaeng wildlife sanctuary, Thailand. Trop. Conserv. Sci. 10:194008291771900. doi: 10.1177/1940082917719000
Palmer, M. V., Martins, M., Falkenberg, S., Buckley, A., Caserta, L. C., Mitchell, P. K., et al. (2021). Susceptibility of white-tailed deer (Odocoileus virginianus) to SARS-CoV-2. J. Virol. 95:e00083. doi: 10.1128/jvi.00083-21
Penrith, M., Beltrán-Alcrudo, D., and Etter, E. M. C. (2019). Epidemiology of African swine fever in Africa today: sylvatic cycle versus socio-economic imperatives. Transbound. Emerg. Dis. 66, 672–686. doi: 10.1111/tbed.13117
Penrith, M. L., and Vosloo, W. (2009). Review of African swine fever: transmission, spread and control. J. S. Afr. Vet. Assoc. 80, 58–62. doi: 10.4102/jsava.v80i2.172
Pepin, K. M., Golnar, A. J., Abdo, Z., and Podgórski, T. (2020). Ecological drivers of African swine fever virus persistence in wild boar populations: insight for control. Ecol. Evol. 10, 2846–2859. doi: 10.1002/ece3.6100
Pérez, J., Fernández, A., Sierra, M. A., Herráez, P., Fernández, A. I., and Martín De Las Mulas, J. (1998). Serological and immunohistochemical study of African swine fever in wild boar in Spain. Vet. Rec. 143, 136–139. doi: 10.1136/vr.143.5.136
Peterson, R. O., Thomas, N. J., Thurber, J. M., Vucetich, J. A., and Waite, T. A. (1998). Population limitation and the wolves of isle Royale. J. Mammal. 79, 828–841. doi: 10.2307/1383091
Pickering, B., Lung, O., Maguire, F., Kruczkiewicz, P., Kotwa, J. D., Buchanan, T., et al. (2022). Divergent SARS-CoV-2 variant emerges in white-tailed deer with deer-to-human transmission. Nat. Microbiol. 7, 2011–2024. doi: 10.1038/s41564-022-01268-9
Probst, C., Globig, A., Knoll, B., Conraths, F. J., and Depner, K. (2017). Behaviour of free ranging wild boar towards their dead fellows: potential implications for the transmission of African swine fever. R. Soc. Open Sci. 4:170054. doi: 10.1098/rsos.170054
ProMED Mail. (2021). Archive Number: 20210125.8134087. PRO/AH/EDR> COVID-19 update (33): Animal, Sweden, Zoo, Tiger, Lion. Available at: https://promedmail.org/promed-post/?id=8134087 (Accessed January 24, 2021).
Pruvot, M., Denstedt, E., Latinne, A., Porco, A., Montecino-Latorre, D., Khammavong, K., et al. (2023). WildHealthNet: supporting the development of sustainable wildlife health surveillance networks in Southeast Asia. Sci. Total Environ. 863:160748. doi: 10.1016/j.scitotenv.2022.160748
Qiu, Y., Abila, R., Rodtian, P., King, D. P., Knowles, N. J., Ngo, L. T., et al. (2018). Emergence of an exotic strain of serotype O foot-and-mouth disease virus O/ME-SA/Ind-2001d in South-East Asia in 2015. Transbound. Emerg. Dis. 65, e104–e112. doi: 10.1111/tbed.12687
Quigley, K. S., Evermann, J. F., Leathers, C. W., Armstrong, D. L., Goodrich, J., Duncan, N. M., et al. (2010). Morbillivirus infection in a wild Siberian tiger in the Russian Far East. J. Wildl. Dis. 46, 1252–1256. doi: 10.7589/0090-3558-46.4.1252
Robinson, H. S., Goodrich, J. M., Miquelle, D. G., Miller, C. S., and Seryodkin, I. V. (2015). Mortality of Amur tigers: the more things change the more they stay the same. Integr. Zool. 10, 344–353. doi: 10.1111/1749-4877.12147
Roelke-Parker, M. E., Munson, L., Packer, C., Kock, R., Cleaveland, S., Carpenter, M., et al. (1996). A canine distemper virus epidemic in Serengeti lions (Panthera leo). Nature 379, 441–445. doi: 10.1038/379441a0
Sadhu, A., Jayam, P. P. C., Qureshi, Q., Shekhawat, R. S., Sharma, S., and Jhala, Y. V. (2017). Demography of a small, isolated tiger (Panthera tigris tigris) population in a semi-arid region of Western India. BMC Zool. 2, 1–13. doi: 10.1186/s40850-017-0025-y
Sadler, R. A., Ramsay, E., McAloose, D., Rush, R., and Wilkes, R. P. (2016). Evaluation of two canine distemper virus vaccines in captive tigers (Panthera tigris). J. Am. Vet. Med. Assoc. 47, 558–563. doi: 10.1638/2015-0223.1
Sanderson, E., Forrest, J., Loucks, C., Ginsberg, J., Dinerstein, E., Seidensticker, J., et al. (2006). Setting Priorities for the Conservation and Recovery of Wild Tigers 2005–2015. A Technical Report. New York, Washington, DC.
Sauter-Louis, C., Conraths, F. J., Probst, C., Blohm, U., Schulz, K., Sehl, J., et al. (2021). African swine fever in wild boar in Europe-a review. Viruses 13:1717. doi: 10.3390/v13091717
Schulz, K., Masiulis, M., Staubach, C., Malakauskas, A., Pridotkas, G., Conraths, F. J., et al. (2021). African swine fever and its epidemiological course in Lithuanian wild boar. Viruses 13:1276. doi: 10.3390/v13071276
Seimon, T. A., Miquelle, D. G., Chang, T. Y., Newton, A. L., Korotkova, I., Ivanchuk, G., et al. (2013). Canine distemper virus: an emerging disease in wild endangered Amur tigers (Panthera tigris altaica). MBio 4, e00410–e00413. doi: 10.1128/mBio.00410-13.Editor
Shetty, B. D., Zachariah, A., Farver, T. B., Smith, B., Goldstein, T., and Mazet, J. A. K. (2020). Carnivore protoparvovirus 1 (parvoviruses) at the domestic-wild carnivore interface in India. J. Zoo Wildl. Med. 50, 1016–1020. doi: 10.1638/2018-0166
Singh, R., Krausman, P. R., Goyal, S. P., and Chauhan, N. S. (2015). Factors contributing to tiger losses in Ranthambhore Tiger Reserve, India. Wildl. Soc. Bull. 39, 670–673. doi: 10.1002/wsb.561
Smith, J. L. D., and McDougal, C. (1991). The contribution of variance in lifetime reproduction to effective population size in tigers. Conserv. Biol. 5, 484–490. doi: 10.1111/j.1523-1739.1991.tb00355.x
Soh, Y. H., Carrasco, L. R., Miquelle, D. G., Jiang, J., Yang, J., Stokes, E. J., et al. (2014). Spatial correlates of livestock depredation by Amur tigers in Hunchun, China: relevance of prey density and implications for protected area management. Biol. Conserv. 169, 117–127. doi: 10.1016/j.biocon.2013.10.011
Steinel, A., Munson, L., van Vuuren, M., and Truyen, U. (2000). Genetic characterization of feline parvovirus sequences from various carnivores. J. Gen. Virol. 81, 345–350. doi: 10.1099/0022-1317-81-2-345
Steinel, A., Parrish, C. R., Bloom, M. E., and Truyen, U. (2001). Parvovirus infections in wild carnivores. J. Wildl. Dis. 37, 594–607. doi: 10.7589/0090-3558-37.3.594
Sunquist, M. (2010). “What is a tiger? Ecology and behaviour” in Tigers of the World, the Science, Politics and Conservation of Panthera tigris. eds. R. Tilson and P. J. Nyhus (London, United Kingdom: Academic Press), 19–51.
Techakriengkrai, N., Bodgener, J., Logan, N., Willett, B. J., Hosie, M. J., and Gilbert, M. (2019). Quantification of canine distemper virus neutralising antibodies in wildlife serum using vesicular stomatitis virus pseudotype-based assay. Thai J. Vet. Med. 49, 34–36.
Ten, D. C. Y., Jani, R., Hashim, N. H., Saaban, S., Hashim, A. K. A., and Abdullah, M. T. (2021). Panthera tigris jacksoni population crash and impending extinction due to environmental perturbation and human-wildlife conflict. Animals 11:1032. doi: 10.3390/ani11041032
Troyer, J. L., Pecon-Slattery, J., Roelke, M. E., Johnson, W., VandeWoude, S., Vazquez-Salat, N., et al. (2005). Seroprevalence and genomic divergence of circulating strains of feline immunodeficiency virus among Felidae and Hyaenidae. J. Virol. 79, 8282–8294. doi: 10.1128/JVI.79.13.8282
United States Department of Agriculture–Animal and Plant Health Inspection Service. (2022). Confirmed cases of SARS-CoV-2 in animals in the United States. Available at: https://www.aphis.usda.gov/aphis/dashboards/tableau/sars-dashboard (Accessed December 5, 2022).
Vergne, T., Guinat, C., and Pfeiffer, D. U. (2020). Undetected circulation of African swine fever in wild boar, Asia. Emerg. Infect. Dis. 26, 2480–2482. doi: 10.3201/eid2610.200608
Viana, M., Mancy, R., Biek, R., Cleaveland, S., Cross, P. C., Lloyd-Smith, J. O., et al. (2014). Assembling evidence for identifying reservoirs of infection. Trends Ecol. Evol. 29, 270–279. doi: 10.1016/j.tree.2014.03.002
Waller, S. J., Brodie, J., Miquelle, D. G., Robinson, H., and Hebblewhite, M.. (2022). Evaluating the Use of Camera Traps to Monitor Prey Populations in the Russian Far East. University of Montana, ScholarWorks at University of Montana
Wang, K., Du, S., Wang, Y., Wang, S., Luo, X., Zhang, Y., et al. (2019). Isolation and identification of tiger parvovirus in captive Siberian tigers and phylogenetic analysis of VP2 gene. Infect. Genet. Evol. 75:103957. doi: 10.1016/j.meegid.2019.103957
Wang, L., Gyimesi, Z. S., Killian, M. L., Torchetti, M., Olmstead, C., Fredrickson, R., et al. (2022). Detection of SARS-CoV-2 clade B.1.2 in three snow leopards. Transbound. Emerg. Dis. 69, e3346–e3351. doi: 10.1111/tbed.14625
Wang, X., Li, T., Liu, H., Du, J., Zhou, F., Dong, Y., et al. (2017). Recombinant feline parvovirus infection of immunized tigers in Central China. Emerg. Microbes Infect. 6, 1–3. doi: 10.1038/emi.2017.25
Wilkes, R. P. (2023). Canine distemper virus in endangered species: species jump, clinical variations, and vaccination. Pathogens 12:57. doi: 10.3390/pathogens12010057
Woodroffe, R. (1999). Managing disease threats to wild mammals. Anim. Conserv. 2, 185–193. doi: 10.1111/j.1469-1795.1999.tb00064.x
Woodroffe, R., Prager, K. C., Munson, L., Conrad, P. A., Dubovi, E. J., and Mazet, J. A. K. (2012). Contact with domestic dogs increases pathogen exposure in endangered African wild dogs (Lycaon pictus). PLoS One 7:e30099. doi: 10.1371/journal.pone.0030099
Woonwong, Y., Do Tien, D., and Thanawongnuwech, R. (2020). The future of the pig industry after the introduction of African swine fever into Asia. Anim. Front. Rev. Mag. Anim. Agric. 10, 30–37. doi: 10.1093/af/vfaa037
World Bank. (2022). Climate Change Knowledge Portal. Available at: https://climateknowledgeportal.worldbank.org/ (Accessed December 22, 2022).
Keywords: Tiger (Panthera tigris), disease, prey, canine distemper virus, African swine fever, health, conservation
Citation: Gilbert M, Dvornicky-Raymond Z and Bodgener J (2023) Disease threats to tigers and their prey. Front. Ecol. Evol. 11:1135935. doi: 10.3389/fevo.2023.1135935
Edited by:
Luke T. B. Hunter, Wildlife Conservation Society (United States), United StatesReviewed by:
Sergio Guerrero Sanchez, City University of Hong Kong, Hong Kong SAR, ChinaKaren Terio, University of Illinois at Urbana-Champaign, United States
Copyright © 2023 Gilbert, Dvornicky-Raymond and Bodgener. This is an open-access article distributed under the terms of the Creative Commons Attribution License (CC BY). The use, distribution or reproduction in other forums is permitted, provided the original author(s) and the copyright owner(s) are credited and that the original publication in this journal is cited, in accordance with accepted academic practice. No use, distribution or reproduction is permitted which does not comply with these terms.
*Correspondence: Martin Gilbert, bS5naWxiZXJ0QGNvcm5lbGwuZWR1