- 1Section for Ecology and Evolution, Department of Biology, University of Copenhagen, Copenhagen, Denmark
- 2Unité de Recherche en Ecologie et Biodiversité, UFR des Sciences de la Nature, Université Nangui Abrogoua, Abidjan, Côte d’Ivoire
- 3Station de Recherche en Ecologie du Parc National de la Comoé, Abidjan, Côte d’Ivoire
- 4Section for Organismal Biology, Department of Plant and Environmental Sciences, University of Copenhagen, Copenhagen, Denmark
Fungus-farming termite colonies maintain monoculture fungus combs in underground chambers without apparent problems with diseases. Multiple lines of defense contribute to the suppression or removal of antagonists of the symbiosis, but the role of the termite-manipulated environment within mounds has yet to be tested. Specifically, termite mounds have extremely high levels of CO2 compared to atmospheric levels. We tested the effect of 5% CO2 on the growth of fungal crops from Macrotermes bellicosus colonies, generalist fungi that could challenge the symbiosis, as well as a specialist stowaway fungus, Pseudoxylaria. For sporulating fungi, we also quantified the effects on conidia production. We found that elevated CO2 significantly reduces mycelial growth and conidia production of the generalist fungi Aspergillus sp., Beauveria bassiana, and Metarhizium brunneum, whereas it overall had a net positive effect on the growth of the fungal crop Termitomyces and Pseudoxylaria; albeit, with variation between fungal strains within genera. Our findings point to elevated CO2 being of adaptive significance to the fungus-farming termite symbiosis as an additional layer of defense that helps keep termite fungus gardens free from fungal infections. The mound-building activities that make termites ecosystem engineers may thus also generate environmental conditions that impact the fate of fungi inhabiting the extended phenotypes that massive termite mounds represent.
Introduction
Colony constructs in social insects allow for radically different internal environments compared to the outside, allowing essential homeostasis despite fluctuating external conditions. The mounds built by fungus-farming termite species of the subfamily Macrotermitinae (Blattodea; Termitidae) are prominent features in African and Southeast Asian ecosystems (Figure 1A). Obligate mutualism with the fungal genus Termitomyces (Basidiomycota; Lyophyllaceae) allows these termites to obtain near-complete degradation of diverse plant substrates (da Costa et al., 2019). The termites maintain their monoculture fungal crop on structures of partially digested plant material (fungus comb Figure 1D), and the fungus provides nutrition for the termite hosts (da Costa et al., 2019). The termites, in turn, provide the fungus with plant substrate, protection, and optimal growth conditions (Korb, 2003; Korb, 2011). Monoculture farming should attract antagonists that could spread fast in the genetically homogeneous crop, but individual colonies can persist for decades (Wisselink et al., 2020) and do not appear to be challenged with diseases (Otani et al., 2019). Furthermore, non-Termitomyces fungi comprise only a minute proportion of the comb biomass (Moriya et al., 2015; Otani et al., 2019), and weeds are most likely accidentally brought in by foraging termite workers (Thomas, 1987; Guedegbe et al., 2009; Bos et al., 2021). It is only when the termites are removed or the colony is dying, that antagonists and stowaway fungi, such as members of the subgenus Pseudoxylaria (Ascomycota; Xylariaceae), appear (Visser et al., 2011).
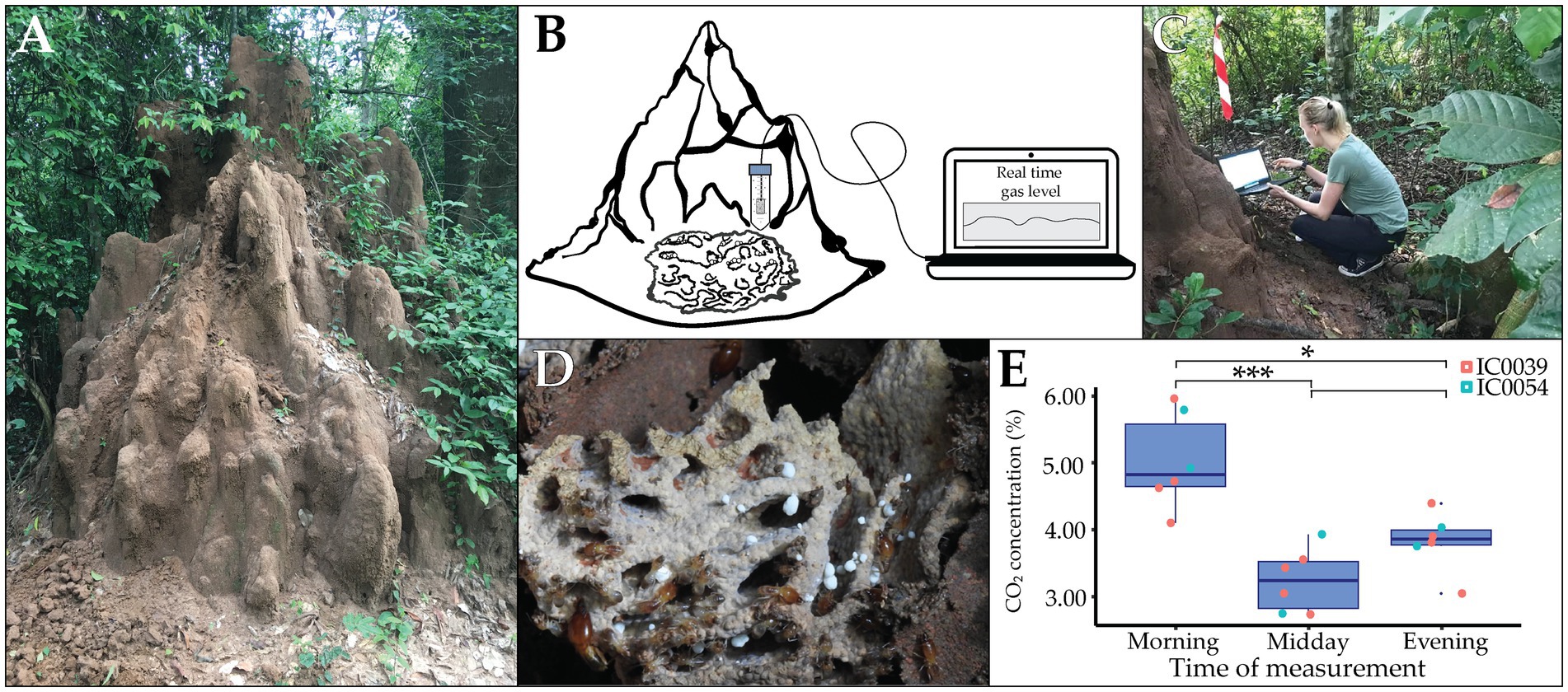
Figure 1. In situ CO2 measurements in Côte d’Ivoire. Macrotermes termites construct elaborate mounds to ensure optimal microclimate for cultivation of Termitomyces. (A) A termite mount of Macrotermes bellicosus colony IC0039 in Côte d’Ivoire. (B) Schematic depiction of the setup for in situ measurements of CO2 levels within termite mounds. (C) Field measurement. (D) Belowground comb structure comprised of plant and fungus biomass. (E) Box plot of CO2 levels in the morning (left), at midday (middle) and in the evening (right) with colors represents different termite colonies. Whiskers extend to 1.5 * Interquartile Range (IQR) (n = 6; significant difference are shown as *p < 0.05; ***p < 0.0001).
The termites protect Termitomyces through a series of complementary defenses that collectively provide very robust protection. The termites monitor and weed the fungus comb (Katariya et al., 2017), avoid substrates that contain mycopathogens (Bodawatta et al., 2019), remove and bury Pseudoxylaria to generate hypoxia that kills the stowaway (Katariya et al., 2018), and utilize antimicrobial compounds of their own (Lamberty et al., 2001) or symbiont origins (Um et al., 2013; Schmidt et al., 2022; Murphy et al., 2023). Recent work has also identified volatile terpenes within the headspace surrounding the fungus comb that could be antimicrobial (Burkhardt et al., 2019; Kreuzenbeck et al., 2022). The comb headspace can play a role in defense as the termites ensure an enclosed, homeostatic comb environment, generated through elaborate ventilation structures that maintain consistent temperature and humidity (Korb, 2003; Murphy et al., 2023).
The tightly controlled comb environment, together with the high metabolism of the termites and Termitomyces and the processing of large quantities of decaying plant material, leads to high amounts of CO2 (Darlington et al., 1997; Konate et al., 2003; Murphy et al., 2023). This leads to the accumulation of CO2 that can be up to 5% in the central part of the mound in Macrotermes carbonarius (Matsumoto, 1978) and ~ 1.2% in the ventilation turrets of Macrotermes bellicosus (Korb and Linsenmair, 1999; Korb and Linsenmair, 2000; Turner, 2001). This is vastly higher than the ~0.04% CO2 in the surrounding atmosphere and a product of limited gas exchange combined with high metabolism of termites and their fungal crop (Matsumoto, 1978), which are adapted to and benefit from these conditions (Luescher, 1961; Matsumoto, 1978; Korb, 2003). High CO2 negatively affects the growth of most organisms, including negative effects on the respiration of the fungal crop of leaf-cutting ants (Kleineidam and Roces, 2000; Romer et al., 2017). However, Termitomyces does not appear to be affected (Katariya et al., 2018). Elevated CO2 could thus aid in the selective suppression of competitor or pathogenic fungi, such as the specialist Pseudoxylaria. This was first proposed by Batra and Batra (1966). However, beyond recent investigations of the impact of hypoxia in low and high CO2 conditions (Katariya et al., 2018), the role of elevated CO2 has not been tested.
To test this hypothesis, we evaluated the effect of elevated CO2 on the growth of Termitomyces, Pseudoxylaria, and other ecologically relevant fungi associated with fungus-farming termites. After confirming high CO2 levels within Macrotermes bellicosus colonies, we subsequently quantified growth of 15 fungal strains from six genera under ambient (0.04%) and elevated (5.00%) CO2 concentrations in vitro. We predicted that Termitomyces and Pseudoxylaria, which are specialized to life in these conditions, would be unaffected or benefit from elevated CO2, while opportunistic fungi should exhibit reduced growth or conidia production when exposed to mound CO2 concentrations.
Materials and methods
In situ measurements of CO2 concentration, humidity, and temperature
We identified two Macrotermes bellicosus colonies (IC0039 and IC0054) close to the Lamto Ecological Research Station1 in Côte d’Ivoire (Supplementary Table 1) and confirmed termite species with PCR amplification of the COXII gene (Zaman et al., 2022). We measured CO2 concentrations using a S8 Miniature 5% CO2 sensor.2 The sensor was placed in a falcon tube with holes to protect the probe from the termites while ensuring airflow. We placed the CO2 probe as close to the central fungus chamber as possible via a small hole in the mound wall and avoided the probe touching anything that would agitate the termites (Figure 1B). We closed the hole with mound soil and left the termites to seal their mound properly and re-establish comb headspace conditions. The following day, we started CO2 measurements in real-time in the morning (07:45–08:15), mid-day (13:30–14:00), and in the evening (19:30–20:00) by connecting the USB to the computer using Gaslab® (Figures 1B,C). We simultaneously measured temperature and humidity using iButtons (Hygrochron iButton®), which were placed in the falcon tube with the CO2 sensor. For colony IC0039, it was possible to register CO2 for four consecutive days; however, the termites in colony IC0054 covered the falcon tube within a few days, restricting the airflow to the CO2 probe, which left us with only 2 days of measurements (Supplementary Table 2).
Fungal isolations and barcoding
We tested the impact of elevated CO2 in vitro on 15 fungal strains from six genera (Supplementary Table 1). We obtained Termitomyces isolates from six colonies (hosting two Termitomyces species; see Results) in Côte d’Ivoire by aseptically placing nodules (asexual fruiting structures) on potato dextrose agar (PDA; VWR, 39 g/L) and subculturing until pure. We also obtained strains from three species of the stow-away fungus Pseudoxylaria from South Africa (X802) and Côte d’Ivoire (IC0040-PS and IC0057-PS). These were isolated by leaving fungus comb in moist conditions for 1–3 days without termites present, transferring emerging Pseudoxylaria mycelium to PDA, and subculturing until pure. Strains from two locations were tested as Pseudoxylaria species appear to be generalists of fungus-farming termites (Visser et al., 2009). We also included three Aspergillus strains (three species) isolated from dead Macrotermes natalensis, M. bellicosus and Trinervitermes sp. termites in South Africa or Côte d’Ivoire two entomopathogenic fungi (Metarhizium brunneum and Beauveria bassiana) that were originally isolated from Cydia pomonella larvae (Lepidoptera: Tortricidae) in Austria and an adult of Anthocoris nemorum (Hemiptera: Anthocoridae) in Denmark (Meyling et al., 2009), respectively and finally a mycopathogen, Trichoderma harzianum T22, originally produced by the fusion of T. harzianum strains T12 and T95 (Ahmad and Baker, 1988; Sivan and Harman, 1991).
Strain genotyping
We verified species identities of the fungal strains by sequencing the Internal Transcribed Spacer (ITS) region of the nuclear ribosomal DNA (Schoch et al., 2012). For DNA isolation, we used a Chelex protocol as described in Conlon et al. (2022). We used either the basidiomycete-specific primers ITS1F and ITS4B (Gardes and Bruns, 1993) or the ascomycete-specific primers ITS5F and ITS4B (White et al., 1990). PCR reactions were run with the following conditions: 94°C for 4 min followed by 35 cycles of 94°C for 30s, 58°C for 30s, and 72°C for 30s with a final extension step at 72°C for 4 min. PCR products were checked using agarose gel electrophoresis and purified using ExoSAP-IT™ (Affymetrix Inc., United States). Purified PCR products were sent to Eurofins MWG Operon (Ebersberg, Germany) for sanger sequencing. Sequences were identified using NCBI BLAST to estimate the number of species used for each fungal genus, with species delimitation based on a similarity threshold of >97% between ITS sequences, as this has been shown to be suitable for estimating the number of species within most fungal genera (Blaalid et al., 2013).
In vitro effects of colony CO2 condition on fungal growth and sporulation
All fungi were grown on PDA supplemented with agar (10 g/L, VWR). First, plates of each fungus were grown until harvestable. Then, a ~ 2 cm2 area of fungal biomass was scraped off the agar and deposited in a 150 mL solution of 0.05 g/L Tween 80 (MERCK) and 0.05 g/L Agar (Bie & Berntsen). After vortexing, a sterile inoculation needle was dipped in the solution, and then poked onto the middle of a 90 mm petri dish with PDA. We did 10 biological replicates for each fungal strain and each CO2 treatment, considering a single plate per condition a replicate. This was repeated twice on separate dates, resulting in 20 replicates (with the exception of strain M17-03 (M. brunneum), for which we only measured growth once). Plates were incubated at 30°C and 70% relative humidity at either ambient CO2 in a Digital incubator (INCU-Line®, VWR, Denmark) or at 5.00% CO2 in a Midi 40 CO2 Incubator (Thermo Scientific, Germany). Due to differences in growth rate, the strains were incubated for either 9 days (Termitomyces and Pseudoxylaria), 4 days (M. brunneum, B. bassiana and Aspergillus), or 2 days (T. harzianum). The difference in incubation time was set to ensure that the fungus would not overgrow the Petri dish and thus prevent correct measurements, while ensuring that there was enough growth to measure area. To compare growth across strains, we obtained the mycelial growth area by measuring the area in ImageJ (Rasband, 2023). If the colony was circular, we measured the diameter twice and used the average of the two measurements to calculate the growth area. If the colony was not circular, we obtained the growth area by manually drawing around the colony. Due to a systematic effect of date, we standardized the measurements by subtracting the mean area of all replicates from the individual replicate area and then divided with the standard deviation of all replicates. We verified that CO2 concentrations within Petri dishes were consistently at ambient or 5.00% using the S8 Miniature 5% CO2 sensor. All individual measurements and calculations are available in Supplementary Table 3.
For Aspergillus strains N1IC19 and DT1W, and for M. brunneum M17-03, asexual spores (conidia) could be harvested after incubation, so we counted conidia production per plate after 4 days of growth for both CO2 concentrations. We did this by depositing 10 mL 0.05% Triton-X (Sigma) on each fungal plate and rubbed the culture with a Drigalsky spatula to obtain a suspension of conidia, which was washed twice in 0.05% Triton-X by centrifugation and then discarding of the supernatant. We subsequently did a dilution series and counted conidia using a hemocytometer (Fuchs-Rosenthal). To test if prior exposure to elevated CO2 affected sporulation even under ambient CO2 levels, we pipetted 100 μL of a 1,000x dilution from plates grown at 0.04% or 5.00% CO2 onto new PDA plates, spread conidia using a Drigalsky spatula, and counted the number of live (germinated) vs. dead (not germinated) conidia after overnight incubation. We did this for at least two replicates per strain. Neither Termitomyces or Pseudoxylaria produce conidia in our lab conditions, precluding testing of the impact of CO2 on conidia production.
Statistical analyses
All analyses were performed in R 3.6.3 (RCoreTeam, 2021). The in situ CO2 measurements of termite mounds were compared with a two-way ANOVA testing for colony and time of day effects. Subsequently we determined effect sizes by using Cohen’s f and then conducted pairwise comparisons of time points with TukeyHSD post hoc testing. The effect of CO2 level on fungal growth was analyzed using a linear model with the standardized growth area as the dependent variable and CO2 treatment and replicate as fixed effects. Replicate was added as a fixed effect instead of a random effect, as there were fewer than five levels, which can make estimates of random effects unreliable. Post hoc pairwise comparisons between the two CO2 conditions (0.04 and 5.00%) and fungal strains were conducted using the emmeans R package (Lenth et al., 2022). We tested the effect of CO2 treatment on conidia production using a linear model on the log spore count as the dependent variable and CO2 treatment and replicate as fixed effects. A two-way ANOVA of the regression model was used to test for significance of strain and CO2 level. Conidia viability was tested by fitting a generalized linear model using a binomial family and a logit link function to assess the association between dead/alive spores and CO2 concentration. A Pearson’s χ2-test was used to test if fungal strain affected the results, followed by a one-way ANOVA to test the significance of CO2 concentration on spore viability.
Results
In situ measurements of CO2 concentration in Côte d’Ivoire
Mound CO2 concentrations were more than 100-fold higher (Mean ± SD: 4.15% ± 1.00%) than the external atmosphere (0.04 ± 0.00%). CO2 concentrations were highest in the morning (5.21 ± 0.74%), declined during the day (3.22 ± 0.47%), and increased again in the evening (3.82 ± 0.39%) (Figure 1E and Supplementary Table 2). The CO2 sensor we used is designed to measure within the range of 0–5% CO2 with a ± 0.02% accuracy, implying that accuracy for the measurements exceeding 5% is not known. However, these represented only a few samples and, even if accuracy was lower than within the 0–5% range, it would not impact the overall pattern. Colonies were not significantly different in CO2 level (two-way ANOVA; F1 = 0.7150, p = 0.4142), while time of day did have an effect (F2 = 13.74, p = 0.0008). Cohen’s f indicated the effect size to be 1.51. Pairwise comparisons with TukeyHSD showed that CO2 levels varied across different times of day; morning vs. mid-day: adj-p = 0.0007, morning vs. evening: adj-p = 0.0122, mid-day vs. evening: adj-p = 0.2510. The average temperature within mounds was 26.6°C (SD ± 0.98°C) and relative humidity was 98.2% (SD ± 1.14%). The temperature decreased approximately 0.5°C from 8:00–11:00 and again from 18:00–23:00.
Fungal growth in different CO2 conditions
Combining the six Termitomyces strains, we found a significant positive effect of elevated CO2 on growth (post hoc paired t-ratio test; t = −2.339; df = 211; p = 0.0200), with an overall medium effect of CO2 (Cohen’s f = 0.407). However, when evaluated individually, three strains exhibited significantly increased growth, two were unaffected, and one strain exhibited significantly decreased growth when exposed to elevated CO2 (Figure 2A and Table 1). The positive effect of growth was most significant on the Termitomyces species associated with colonies IC0010 and IC0034, whereas we observed more variable effects of elevated CO2 on the species associated with IC0027 and IC0033 (unaffected), IC0032 (negative), and IC0031 (positive). The three Pseudoxylaria species were overall positively affected by elevated CO2 (t = −0.741; df = 89; p = 0.0318) with a medium effect (Cohen’s f = 0.397), driven by a significant positive effect on one of the strains (Figure 2B and Table 1). Two of the three Aspergillus species were negatively affected by elevated CO2 (N1IC19: t = 2.806; df = 27; p = 0.0052; GF10t1: t = 3.459; df = 23; p = 0.0006), as was B. bassiana (t = 5.319; df = 43; p < 0.0001) and M. brunneum (t = 3.360; df = 17; p = 0.0008), but not T. harzianum (t = −0.5614; df = 63; p = 0.5749) (Figure 2C and Table 1). The individual p-values and effect sizes are given in Table 1.
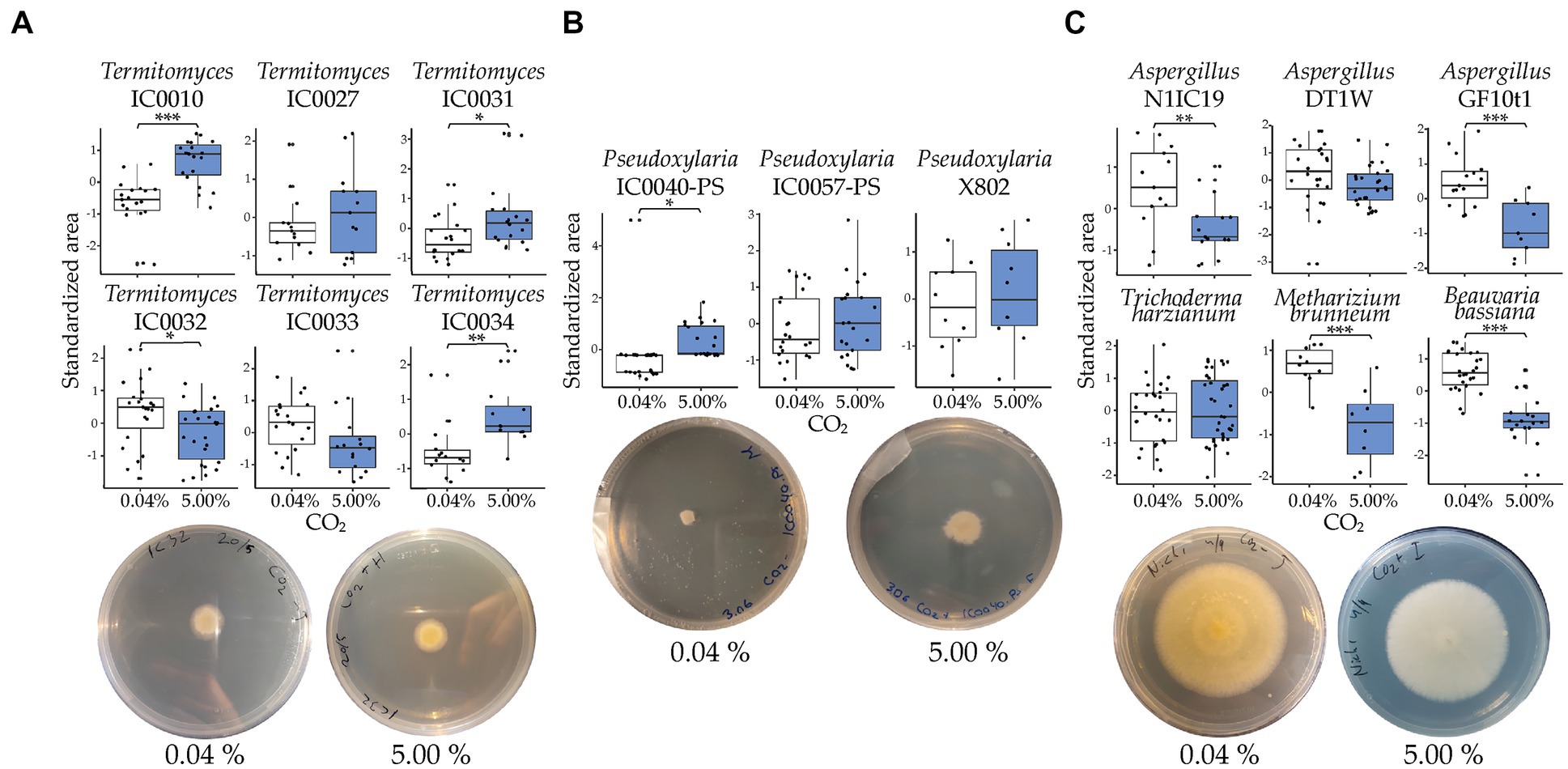
Figure 2. Standardized growth of fungal strains at ambient (0.04%) and elevated (5.00%) levels of CO2. White boxplots represent growth under ambient CO2 and blue boxplots represent growth in 5% CO2. (A) Results for each of the six Termitomyces strains. (B) Results for each of the three Pseudoxylaria strains. (C) Results for each of the six generalist strains. The significance of treatments was assessed with a post hoc pairwise comparison between the two CO2 conditions for each fungal strain. Whiskers extend to 1.5*IQR. Below each boxplot are selected plates of a strain showing how they grew in the different CO2 conditions: (A) Termitomyces, IC0032, (B) Pseudoxylaria, IC0040-PS, and (C) Aspergillus (N1IC19). See Supplementary Table 1 for strain information, Supplementary Table 3 for the raw data, and Table 1 for the full results of the statistical analyses. Significant differences are shown as *p < 0.05; **p < 0.01; ***p < 0.001.
Conidia production and viability
Our evaluation of the impact of elevated CO2 on conidia production and viability revealed that the two Aspergillus strains (N1IC19 and DT1W) produced fewer conidia after incubation at elevated CO2 (post hoc paired t-ratio test; DT1W, t = 2.967; df = 15; p = 0.0053; N1IC19, t = 13.45; df = 15; p < 0.0001), but this was not the case for M. brunneum (t = 1.457; df = 9; p = 0.1538) (Figures 3A,B). Fungal strain did not significantly affect the number of viable conidia (Pearson χ2 = 11.63; df = 6; p = 0.0709). We therefore pooled all results, and a subsequent test of the pooled results did not reveal an impact on the proportion of live vs. dead conidia under subsequent CO2 conditions of strains originally grown at ambient vs. elevated CO2 concentrations (one-way ANOVA; F3 = 0.4652; p = 0.7074) (Figure 3C).
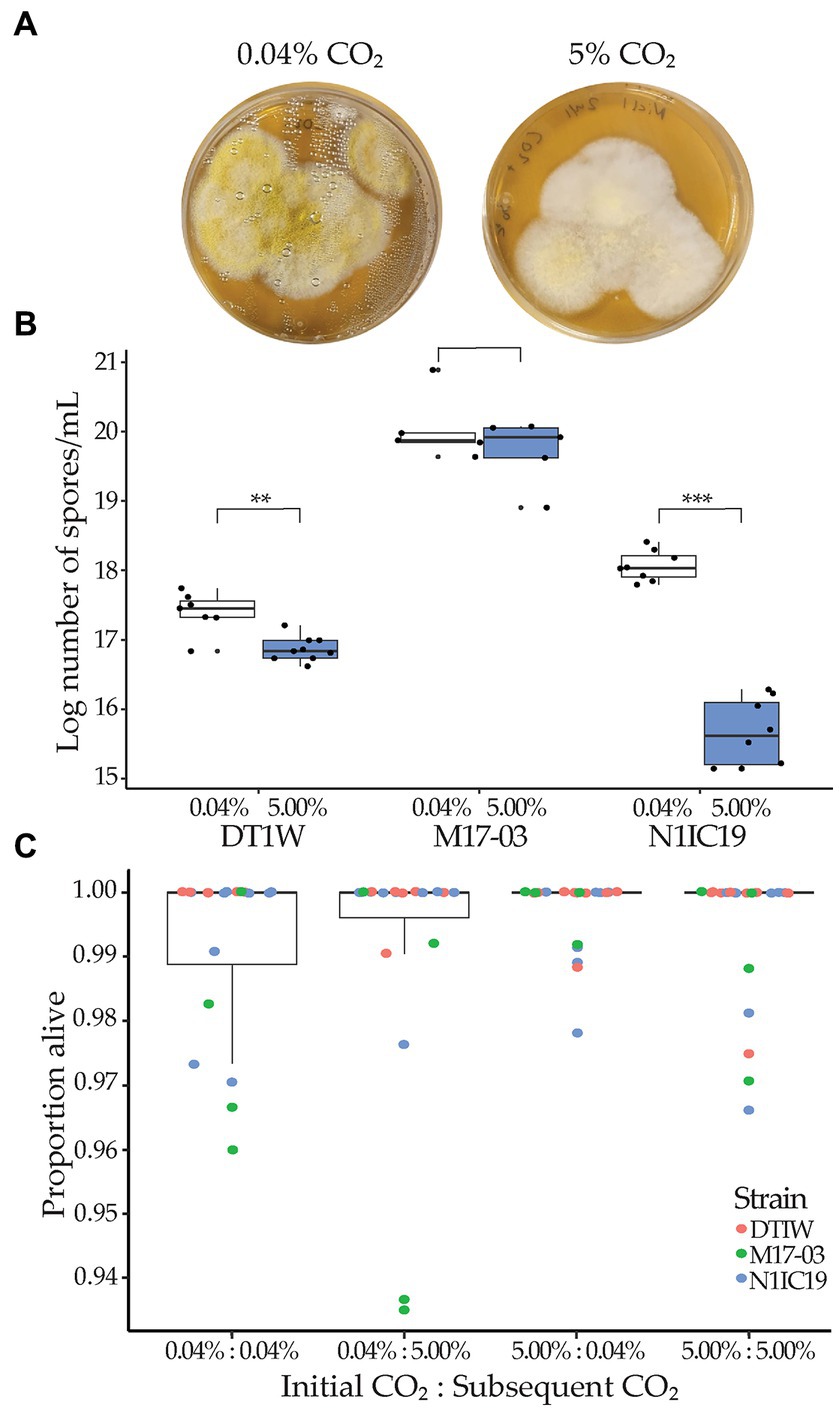
Figure 3. Impact of elevated CO2 level on sporulation. (A) Representative images of Aspergillus sp. (N1IC19) at 0.04 and 5.00% CO2 after 4 days of growth, showing marked reduction in conidia production, as signified by the color reduction. (B) Average (log)number of conidia per ml after incubation in either 0.04% (ambient; white boxplots) or 5.00% (mound level; blue boxplots) CO2 for Aspergillus strains (DT1W and N1IC19) and M. brunneum (M17-03). Significant difference are shown as **p < 0.01; ***p < 0.001. (C) After incubation, conidia from each CO2 treatment were replated and incubated in another round of CO2 treatment to establish viability. The combined proportion of live vs. dead conidia across all three fungal strains is given on the y-axis. Colors indicate individual results for Aspergillus (DT1W = red, N1IC19 = blue) and M. brunneum (M17-03 = green) strains. The x-axis label “Initial CO2: Subsequent CO2” indicates the initial incubation CO2 concentration for the fungus and the CO2 concentration once the spores were replated. CO2 treatment had no significant effect on conidia viability.
Discussion
We confirmed extraordinarily high CO2 concentrations within the mounds of Macrotermes bellicosus, and found consistent patterns between colonies, which allowed us to set an appropriate CO2 level for our in vitro studies. These measurement align with previous findings in conspecifics (Matsumoto, 1978) and underline how specialists of the symbioses are adapted to these relatively unique growth conditions. As expected, specialists of the symbiosis were generally either unaffected or positively affected by the high CO2 levels, while generalists exhibited reduced growth and/or conidia production. Notably, we also observed substantial variation between replicates of the same fungal strains, which likely emerges from variation in inoculum size and from variation in the proportion of inocula that successfully establishes on plates. The CO2 environment within mounds may thus not merely be a result of a trade-off between ventilation and protection from desiccation but also be of adaptive significance to the fungus-farming termite symbiosis as a potential additional layer of defense to help keep termite fungus gardens free from infections.
As expected for specialists of the symbioses, five of the six Termitomyces strains and all Pseudoxylaria strains were either unaffected or exhibited increased growth with elevated CO2, with some variability. The remaining strain of Termitomyces showed reduced growth under elevated CO2, however only with a low to medium effect size. The mound microclimate may thus influence growth differently across species of specialists. This general overall effect underlines how these fungal genera are adapted to comb conditions and suggests that mound CO2 conditions do not aid in the normal suppression of the stowaway fungus. In line with this, the absence of increased Pseudoxylaria growth at ambient (compared to elevated) CO2 concentrations indicates that decreased CO2 concentration when mound wall integrity is compromised is unlikely to be the signal that triggers rampant growth of Pseudoxylaria (Visser et al., 2011). Other defenses must therefore be more important to suppress this fungus, such as antifungals (Visser et al., 2012; Um et al., 2013; reviewed by Schmidt et al., 2022), termite weeding and grooming of the comb (Katariya et al., 2017; Murphy et al., 2023), or the competitive advantage that Termitomyces obtains through its dense inoculation during comb formation (Badertscher et al., 1983; Leuthold et al., 1989).
Elevated CO2 suppressed the growth of several of the entomopathogens and generalist contaminants that may enter mounds with the plant substrates (Bos et al., 2021) and persist in trace amounts within fungus combs (Otani et al., 2019). Aspergillus spp. are ubiquitous fungi found in a variety of environments and substrates, such as soil, air, and decaying plant material, where they thrive as saprophytes (Mousavi et al., 2016). The Aspergillus strains exhibited reduced growth and conidia production for the two strains for which this could be quantified (Figures 2, 3). This implies that although the fungi may enter combs, they are unlikely to be adapted to the internal nest conditions. This suggests that tradeoffs exist that preclude adaptations to the environment within termite mounds. The two generalist entomopathogens also exhibited reduced growth, implying that elevated CO2 may help prevent their spread between termite hosts within colonies. The discrepancy in the impact on conidia production on the fungal genera may imply that mechanisms affecting sporulation differ, which should be further explored, ideally across more strains and species per genus. In contrast to entomopathogens, the mycopathogen T. harzianum was unaffected by elevated CO2. Trichoderma spp. are found in nearly all soil types world-wide, infect termite fungal combs (Mathew et al., 2012), and respond in species-specific ways to CO2 conditions (Danielson and Davey, 1973). Since the mycopathogen was not impacted by elevated CO2, it is likely critical that workers avoid plant material containing the fungus (Bodawatta et al., 2019), and that combinations of defenses collectively allow the termites to maintain robust defenses toward diverse fungi with different susceptibilities to environmental conditions.
Our findings do not determine the mechanism that causes growth suppression, but elevated CO2 may reduce comb pH as CO2 reacts with H2O to form carbonic acid (Wang et al., 2016). This is consistent with comb pH levels being 4–5 in Macrotermes and Odontotermes spp. compared to 6–7 in the surrounding soil (Muwawa et al., 2014). Lower pH causes weak acid stress in Saccharomyces cerevisiae (Piper et al., 2001), while alkaline conditions have been shown to increase fungal virulence (Selvig and Alspaugh, 2011; Vylkova, 2017). The elevated CO2 inside mounds could thereby not only affect the growth but also the virulence of potential pathogens. Species of Metarhizium prefer alkaline conditions and can increase surrounding pH (Lovett and St. Leger, 2015) to optimize growth and conidia production (Gao et al., 2009). Lowered pH could thus limit proliferation of certain fungi, but as many fungi inhabit mildly acidic environments, such as soils and plant and animal surfaces (Vylkova, 2017), low pH in itself is most likely not enough to rid unwanted fungi. Increased CO2 concentration also stimulates the cAMP/Protein kinase A pathway, which can regulate fungal growth, development, and conidia and secondary metabolite production (Azzam et al., 2010; Sun et al., 2022). We did not evaluate whether elevated CO2 affected this pathway, but it could explain the observed effects. Irrespective of the causal effects of elevated CO2 on growth and reproduction, our findings imply that comb CO2 should impact fungal proliferation and ultimately reproduction, potentially in species-specific ways. The variation we observe implies that more elaborate comparisons are needed to establish how variable responses are to elevated CO2 levels, and that different optima perhaps exist for specialists in the symbiosis vs. generalist pathogens and saprotrophs. Once established, a natural next step would be manipulation experiments to test the impacts of compromised CO2 environments on colony health to establish the adaptive benefits of CO2 in defense.
We guided our experiments based on CO2 concentrations in the massive mounds of M. bellicosus, which were comparable to levels in another species that also builds large mounds, such as M. carbonarius (Matsumoto, 1978). CO2 concentrations in species with comparably large colonies and ventilation turrets, i.e., members of the genera Macrotermes and Odontotermes that represent ~33% of the known species within the Macrotermitinae (Bánki et al., 2022) are likely similar. However, ventilation and the propensity for CO2 to play a role in defense is conceivably different in termite genera with different colony structures. For example, species of the genera Microtermes and Ancistrotermes build small interconnected fungal comb chambers within the soil (Jouquet et al., 2003; van de Peppel and Aanen, 2020). Insights into CO2 levels here are absent, and future work should take a comparative approach to establish in detail the environment within fungal combs as well as the potential for (or limitations of) CO2 to act in defense.
The extended phenotype of the termite mound is a cornerstone in termite ecology. Our findings point to an additional adaptive role emerging from generating a comb environment that aids in the suppression of alien fungi. This speaks to the need to view disease suppression in a more holistic view than previously considered. It also implies that other complementary parameters may play roles in defense, such as reduced oxygen availability or volatile compounds present within the comb headspace. Preliminary work on the latter suggests that this is indeed plausible (Kreuzenbeck et al., 2022, 2023). In addition, the ability to tolerate the extreme environment within mounds is likely an adaptive homeostatic trait that the termite can fine-tune to provide not only a defensive barrier but also optimal growth conditions for their specific crop species (and strains). Furthering our understanding of how termite farmers manage this complex environment for optimal farming of their fungal crop for food, while effectively suppressing antagonists, should thus integrate comb abiotic conditions.
Data availability statement
The datasets presented in this study can be found in online repositories. The names of the repository/repositories and accession number(s) can be found in the article/Supplementary material.
Author contributions
SS, NB, and MP: conceptualization and writing—original draft. SS, NB, KS, NK, and NM: data curation. SS, NB, MP, NM, and RM: methodology. SS, NB, and RM: formal analysis and visualization. SS, NB, RM, NM, KS, NK, and MP: writing—review and editing. MP and NK: supervision. MP: funding acquisition. All authors contributed to the article and approved the submitted version.
Funding
This research was funded by the European Research Council Consolidator (Grant No. ERC-CoG-771349) to MP.
Acknowledgments
We thank members of the Social and Symbiotic Evolution group at the University of Copenhagen for comments on a previous version of the manuscript.
Conflict of interest
The authors declare that the research was conducted in the absence of any commercial or financial relationships that could be construed as a potential conflict of interest.
Publisher’s note
All claims expressed in this article are solely those of the authors and do not necessarily represent those of their affiliated organizations, or those of the publisher, the editors and the reviewers. Any product that may be evaluated in this article, or claim that may be made by its manufacturer, is not guaranteed or endorsed by the publisher.
Supplementary material
The Supplementary material for this article can be found online at: https://www.frontiersin.org/articles/10.3389/fevo.2023.1134492/full#supplementary-material
Footnotes
References
Ahmad, J. S., and Baker, R. (1988). Implications of rhizosphere competence of Trichoderma harzianum. Can. J. Microbiol. 34, 229–234. doi: 10.1139/m88-043
Azzam, Z. S., Sharabi, K., Guetta, J., Bank, E.M, and Gruenbaum, Y. (2010). The physiological and molecular effects of elevated CO2 levels. Cell Cycle 9, 1528–1532. doi: 10.4161/cc.9.8.11196
Badertscher, S., Gerber, C., and Leuthold, R. H. (1983). Polyethism in food-supply and processing in termite colonies of Macrotermes subhyalinus (Isoptera). Behav. Ecol. Sociobiol. 12, 115–119. doi: 10.1007/Bf00343201
Bánki, O., Roskov, Y., Döring, M., Ower, G., Vandepitte, L., Hobern, D., et al. (2022). Catalogue of life checklist (version 2022-09-25). Catalogue of life. doi: 10.48580/dfqz
Batra, L. R., and Batra, S. W. T. (1966). Fungus-growing termites of tropical India and associated fungi. J. Kansas Entomol. Soc. 39, 725–738.
Blaalid, R., Kumar, S., Nilsson, R. H., Abarenkov, K., Kirk, P. M., and Kauserud, H. (2013). ITS1 versus ITS2 as DNA metabarcodes for fungi. Mol. Ecol. Resour. 13, 218–224. doi: 10.1111/1755-0998.12065
Bodawatta, K. H., Poulsen, M., and Bos, N. (2019). Foraging Macrotermes natalensis fungus-growing termites avoid a mycopathogen but not an entomopathogen. Insects 10:185. doi: 10.3390/insects10070185
Bos, N., Guimaraes, L., Palenzuela, R., Renelies-Hamilton, J., Maccario, L., Silue, S. K., et al. (2021). You don’t have the guts: a diverse set of fungi survive passage through Macrotermes bellicosus termite guts. BMC Ecol. Evol. 21:163. doi: 10.1186/s12862-021-01744-6
Burkhardt, I., Kreuzenbeck, N. B., Beemelmanns, C., and Dickschat, J. S. (2019). Mechanistic characterization of three sesquiterpene synthases from the termite-associated fungus Termitomyces. Org. Biomol. Chem. 17, 3348–3355. doi: 10.1039/c8ob02744g
Conlon, B. H., Schmidt, S., Poulsen, M., and Shik, J. Z. (2022). Orthogonal protocols for DNA extraction from filamentous fungi. STAR Protocol 3:101126. doi: 10.1016/j.xpro.2022.101126
da Costa, R. R., Hu, H. F., Li, H. J., and Poulsen, M. (2019). Symbiotic plant biomass decomposition in fungus-growing termites. Insects 10:87. doi: 10.3390/insects10040087
Danielson, R. M., and Davey, C. B. (1973). Non nutritional factors affecting the growth of Trichoderma in culture. Soil Biol. Biochem. 5, 495–504. doi: 10.1016/0038-0717(73)90039-4
Darlington, J. P. E. C., Zimmerman, P. R., Greenberg, J., Westberg, C., and Bakwin, P. (1997). Production of metabolic gases by nests of the termite Macrotermes jeanneli in Kenya. J. Trop. Ecol. 13, 491–510. doi: 10.1017/S0266467400010671
Gao, L., Liu, X. Z., Sun, M. H., Li, S. D., and Wang, J. L. (2009). Use of a novel two-stage cultivation method to determine the effects of environmental factors on the growth and sporulation of several biocontrol fungi. Mycoscience 50, 317–321. doi: 10.1007/s10267-009-0483-3
Gardes, M., and Bruns, T. D. (1993). Its primers with enhanced specificity for basidiomycetes - application to the identification of mycorrhizae and rusts. Mol. Ecol. 2, 113–118. doi: 10.1111/j.1365-294X.1993.tb00005.x
Guedegbe, H. J., Miambi, E., Pando, A., Roman, J., Houngnandan, P., and Rouland-Lefevre, C. (2009). Occurrence of fungi in combs of fungus-growing termites (Isoptera: Termitidae, Macrotermitinae). Mycol. Res. 113, 1039–1045. doi: 10.1016/j.mycres.2009.06.008
Jouquet, P., Mery, T., Rouland, C., and Lepage, M. (2003). Modulated effect of the termite Ancistrotermes cavithorax (Isoptera, Macrotermitinae) on soil properties according to the structures built. Sociobiology 42, 403–412.
Katariya, L., Ramesh, P. B., and Borges, R. M. (2018). Dynamic environments of fungus-farming termite mounds exert growth-modulating effects on fungal crop parasites. Environ. Microbiol. 20, 971–979. doi: 10.1111/1462-2920.14026
Katariya, L., Ramesh, P. B., Gopalappa, T., Desireddy, S., Bessiere, J. M., and Borges, R. M. (2017). Fungus-farming termites selectively bury weedy fungi that smell different from crop fungi. J. Chem. Ecol. 43, 986–995. doi: 10.1007/s10886-017-0902-4
Kleineidam, C., and Roces, F. (2000). Carbon dioxide concentrations and nest ventilation in nests of the leaf-cutting ant Atta vollenweideri. Insect. Soc. 47, 241–248. doi: 10.1007/Pl00001710
Konate, S., Le Roux, X., Verdier, B., and Lepage, M. (2003). Effect of underground fungus-growing termites on carbon dioxide emission at the point- and landscape-scales in an African savanna. Funct. Ecol. 17, 305–314. doi: 10.1046/j.1365-2435.2003.00727.x
Korb, J. (2003). Thermoregulation and ventilation of termite mounds. Naturwissenschaften 90, 212–219. doi: 10.1007/s00114-002-0401-4
Korb, J. (2011). “Termite mound architecture, from function to construction” in Biology of termites: a modern synthesis. eds. D. E. Bignell, Y. Roisin, and N. Lo (Dordrecht: Springer Netherlands), 349–373.
Korb, J., and Linsenmair, K. E. (1999). The architecture of termite mounds: a result of a trade-off between thermoregulation and gas exchange? Behav. Ecol. 10, 312–316. doi: 10.1093/beheco/10.3.312
Korb, J., and Linsenmair, K. E. (2000). Ventilation of termite mounds: new results require a new model. Behav. Ecol. 11, 486–494. doi: 10.1093/beheco/11.5.486
Kreuzenbeck, N., Dhiman, S., Roman, D., Burkhardt, I., Conlon, B. H., Fricke, J., et al. (2023). Isolation, (bio)synthetic studies and evaluation of antimicrobial properties of drimenol-type sesquiterpenes of Termitomyces fungi. Commun. Chem. 6:79. doi: 10.1038/s42004-023-00871-z
Kreuzenbeck, N. B., Seibel, E., Schwitalla, J. W., Fricke, J., Conlon, B. H., Schmidt, S., et al. (2022). Comparative genomic and Metabolomic analysis of Termitomyces species provides insights into the Terpenome of the fungal cultivar and the characteristic odor of the fungus garden of Macrotermes natalensis termites. Msystems 7:e0121421. doi: 10.1128/msystems.01214-21
Lamberty, M., Zachary, D., Lanot, R., Bordereau, C., Robert, A., Hoffmann, J. A., et al. (2001). Insect immunity - constitutive expression of a cysteine-rich antifungal and a linear antibacterial peptide in a termite insect. J. Biol. Chem. 276, 4085–4092. doi: 10.1074/jbc.M002998200
Lenth, R. V., Buerkner, P., Herve, M., Jung, M., Love, J., Miguez, F., et al. (2022). emmeans: estimated marginal means, aka least-squares means. R package version 1.8.1. Available at: https://cran.r-project.org/web/packages/emmeans/index.html
Leuthold, R. H., Badertscher, S., and Imboden, H. (1989). The inoculation of newly formed fungus comb with Termitomyces in Macrotermes colonies (Isoptera, Macrotermitinae). Insect. Soc. 36, 328–338. doi: 10.1007/Bf02224884
Lovett, B., and St. Leger, R. J. (2015). Stress is the rule rather than the exception for Metarhizium. Curr. Genet. 61, 253–261. doi: 10.1007/s00294-014-0447-9
Luescher, M. (1961). Air-conditioned termite nests. Sci. Am. 205:138. doi: 10.1038/scientificamerican0761-138
Mathew, G. M., Ju, Y. M., Lai, C. Y., Mathew, D. C., and Huang, C. C. (2012). Microbial community analysis in the termite gut and fungus comb of Odontotermes formosanus: the implication of Bacillus as mutualists. FEMS Microbiol. Ecol. 79, 504–517. doi: 10.1111/j.1574-6941.2011.01232.x
Matsumoto, T. (1978). Population density, biomass, nitrogen and carbon content, energy value and respiration rate of four species of termites in Pasoh Forest Reserve. Malay. Nat. J. 2, 335–351.
Meyling, N. V., Lubeck, M., Buckley, E. P., Eilenberg, J., and Rehner, S. A. (2009). Community composition, host range and genetic structure of the fungal entomopathogen Beauveria in adjoining agricultural and seminatural habitats. Mol. Ecol. 18, 1282–1293. doi: 10.1111/j.1365-294X.2009.04095.x
Moriya, S., Inoue, T., Ohkuma, M., Yaovapa, T., Johjima, T., Suwanarit, P., et al. (2015). Fungal community analysis of fungus gardens in termite nests. Microbes Environ. 20, 243–252. doi: 10.1264/jsme2.20.243
Mousavi, B., Hedayati, M. T., Hedayati, N., Ilkit, M., and Syedmousavi, S. (2016). Aspergillus species in indoor environments and their possible occupational and public health hazards. Curr. Med. Mycol. 2, 36–42. doi: 10.18869/acadpub.cmm.2.1.36
Murphy, R., Sinotte, V. M., Schmidt, S., Li, G., Renelies-Hamilton, J., Koné, N., et al. (2023). “Impact of microbial symbionts on fungal-farming termites and their derived ecosystem functions” in Assessing the microbiological health of ecosystems. ed. C. J. Hurst (John Wiley & Son Inc), 185–203.
Muwawa, E. M., Makonde, H. M., Budambula, N. L. M., Osiemo, Z. L., and Boga, H. I. (2014). Chemical properties associated with guts, soil and nest materials of Odontotermes and Macrotermes species from Kenya. J. Biol. Environ. Sci. 4, 253–263.
Otani, S., Challinor, V. L., Kreuzenbeck, N. B., Kildgaard, S., Krath Christensen, S., Larsen, L. L. M., et al. (2019). Disease-free monoculture farming by fungus-growing termites. Sci. Rep. 9:8819. doi: 10.1038/s41598-019-45364-z
Piper, P., Calderon, C. O., Hatzixanthis, K., and Mollapour, M. (2001). Weak acid adaptation: the stress response that confers yeasts with resistance to organic acid food preservatives. Microbiology 147, 2635–2642. doi: 10.1099/00221287-147-10-2635
Rasband, W. S. (2023). ImageJ. U. S. National Institutes of Health, Bethesda, Maryland, USA. https://imagej.nih.gov/ij/
RCoreTeam (2021). R: a language and environment for statistical computing. R Foundation for Statistical Computing, Vienna.
Romer, D., Bollazzi, M., and Roces, F. (2017). Carbon dioxide sensing in an obligate insect-fungus symbiosis: CO2 preferences of leaf-cutting ants to rear their mutualistic fungus. PLoS One 12:e0174597. doi: 10.1371/journal.pone.0174597
Schmidt, S., Kildgaard, S., Guo, H., Beemelmanns, C., and Poulsen, M. (2022). The chemical ecology of the fungus-farming termite symbiosis. Nat. Prod. Rep. 39, 231–248. doi: 10.1039/d1np00022e
Schoch, C. L., Seifert, K. A., Huhndorf, S., Robert, V., Spouge, J. L., Levesque, C. A., et al. (2012). Nuclear ribosomal internal transcribed spacer (ITS) region as a universal DNA barcode marker for Fungi. Proc. Natl. Acad. Sci. U. S. A. 109, 6241–6246. doi: 10.1073/pnas.1117018109
Selvig, K., and Alspaugh, J. A. (2011). pH response pathways in fungi: adapting to host-derived and environmental signals. Mycobiology 39, 249–256. doi: 10.5941/MYCO.2011.39.4.249
Sivan, A., and Harman, G. E. (1991). Improved rhizosphere competence in a protoplast fusion progeny of Trichoderma harzianum. J. Gen. Microbiol. 137, 23–29. doi: 10.1099/00221287-137-1-23
Sun, Z. B., Yu, S. F., Wang, C. L., and Wang, L. (2022). cAMP signalling pathway in biocontrol fungi. Curr. Issues Mol. Biol. 44, 2622–2634. doi: 10.3390/cimb44060179
Thomas, R. J. (1987). Distribution of Termitomyces and other fungi in the nests and major workers of Several Nigerian macrotermitinae. Soil Biol. Biochem. 19, 335–341. doi: 10.1016/0038-0717(87)90019-8
Turner, J. S. (2001). On the mound of Macrotermes michaelseni as an organ of respiratory gas exchange. Physiol. Biochem. Zool. 74, 798–822. doi: 10.1086/323990
Um, S., Fraimout, A., Sapountzis, P., Oh, D. C., and Poulsen, M. (2013). The fungus-growing termite Macrotermes natalensis harbors bacillaene-producing Bacillus sp. that inhibit potentially antagonistic fungi. Sci. Rep. 3:3250. doi: 10.1038/srep03250
van de Peppel, L. J. J., and Aanen, D. K. (2020). High diversity and low host-specificity of Termitomyces symbionts cultivated by Microtermes spp. indicate frequent symbiont exchange. Fungal Ecol. 45:100917. doi: 10.1016/j.funeco.2020.100917
Visser, A. A., Kooij, P. W., Debets, A. J. M., Kuyper, T. W., and Aanen, D. K. (2011). Pseudoxylaria as stowaway of the fungus-growing termite nest: interaction asymmetry between Pseudoxylaria, Termitomyces and free-living relatives. Fungal Ecol. 4, 322–332. doi: 10.1016/j.funeco.2011.05.003
Visser, A. A., Nobre, T., Currie, C. R., Aanen, D. K., and Poulsen, M. (2012). Exploring the potential for actinobacteria as defensive symbionts in fungus-growing termites. Microb. Ecol. 63, 975–985. doi: 10.1007/s00248-011-9987-4
Visser, A. A., Ros, V. I., De Beer, Z. W., Debets, A. J., Hartog, E., Kuyper, T. W., et al. (2009). Levels of specificity of Xylaria species associated with fungus-growing termites: a phylogenetic approach. Mol. Ecol. 18, 553–567. doi: 10.1111/j.1365-294X.2008.04036.x
Vylkova, S. (2017). Environmental pH modulation by pathogenic fungi as a strategy to conquer the host. PLoS Pathog. 13:e1006149. doi: 10.1371/journal.ppat.1006149
Wang, H. B., Zeuschner, J., Eremets, M., Troyan, I., and Willams, J. (2016). Stable solid and aqueous H2CO3 from CO2 and H2O at high pressure and high temperature. Sci. Rep. 6:19902. doi: 10.1038/srep19902
White, T. J., Bruns, T., Lee, S., and Taylor, J. (1990). “Amplification and direct sequencing of fungal ribosomal RNA genes for phylogenetics” in PCR protocols: a guide to methods and applications. eds. M. A. Innis, D. H. Gelfand, J. J. Sninsky, and T. J. White (New York, NY: Academic Press), 315–322.
Wisselink, M., Aanen, D. K., and van’t Padje, A. (2020). The longevity of colonies of fungus-growing termites and the stability of the symbiosis. Insects 11:527. doi: 10.3390/insects11080527
Keywords: Termitomyces, Macrotermitinae, Macrotermes bellicosus, extended phenotype, defense, Pseudoxylaria
Citation: Schmidt S, Bos N, Murphy R, Koné NA, Silué KS, Meyling NV and Poulsen M (2023) Make the environment protect you from disease: elevated CO2 inhibits antagonists of the fungus-farming termite symbiosis. Front. Ecol. Evol. 11:1134492. doi: 10.3389/fevo.2023.1134492
Edited by:
Philippe Vandenkoornhuyse, University of Rennes 1, FranceReviewed by:
Jos Kramer, ETH Zürich, SwitzerlandJie Hu, Netherlands Institute of Ecology (NIOO-KNAW), Netherlands
Copyright © 2023 Schmidt, Bos, Murphy, Koné, Silué, Meyling and Poulsen. This is an open-access article distributed under the terms of the Creative Commons Attribution License (CC BY). The use, distribution or reproduction in other forums is permitted, provided the original author(s) and the copyright owner(s) are credited and that the original publication in this journal is cited, in accordance with accepted academic practice. No use, distribution or reproduction is permitted which does not comply with these terms.
*Correspondence: Suzanne Schmidt, U3V6YW5uZS5TY2htaWR0QGJpby5rdS5kaw==
†Present address
Nick Bos, Radiometer Medical ApS, Copenhagen, Denmark