Corrigendum: Sequential biomolecular, macrofossil, and microfossil extraction from coprolites for reconstructing past behavior and environments
- 1Environmental Archaeology Research Laboratory, Department of Anthropology, Washington State University, Pullman, WA, United States
- 2School of History, Classics and Archaeology, Newcastle University, Newcastle upon Tyne, United Kingdom
- 3Organic Geochemistry Unit, School of Chemistry, University of Bristol, Bristol, United Kingdom
The study of macroscopic, microscopic, and biomolecular remains recovered from coprolites can provide a wide range of information on past human and animal behavior and environments. In earlier studies, research tended to focus on one or two proxies, but multiproxy approaches combining data from all remains within coprolites are becoming more common. Multiproxy analyses have demonstrated value for strengthening our understanding of the past and reducing equifinality. Here we present a sequential biomolecular, macrofossil, and microfossil extraction protocol that separates all different coprolite components and is intended as a best-practice guideline for coprolite analysis. We demonstrate the effectiveness of this method by applying it to an assemblage of coprolites from the Paisley Caves, Oregon, USA. By combining a wide range of proxies, this study provides important information on the taxonomy and behavior of organisms in the past as well as the paleoecological context of behavior.
1. Introduction
Coprolites (desiccated or mineralized feces) are increasingly recognized as important archives of paleoecological, paleontological, and archeological data (Reinhard and Bryant, 1992; Shillito et al., 2020a; Blong and Shillito, 2021). Coprolites are unique archives in that they represent a short-term record of an individual organism’s diet and environment. Studying coprolites from successive occupations at a site(s) can provide fine-grained perspectives on trends in organism behavior and environmental change over long timescales. These data can illuminate the long-term impacts of climate change on organism behavior and many aspects of past human societies including settlement patterns, food systems, social organization, and political systems (for example, Reitz et al., 2008). The long-term perspective afforded by the study of human- and non-human animal-environment interactions is a crucial part of any interdisciplinary solutions to current environmental issues (de Noort, 2011). Therefore, these data obtained from coprolites are important for understanding our past, but also have the potential to provide information relevant to current and future social and environmental challenges (Rick and Sandweiss, 2020; Rockman and Hritz, 2020).
Coprolites typically contain a range of organic and inorganic biomarkers, macrofossils, and microfossils that survive the digestive process. Compared to other lines of evidence, coprolites provide a unique high-resolution snapshot of an organism’s diet and local environment over a period of 1–2 days. Traditional coprolite studies have focused on identifying plant and animal macrofossils and microfossils recovered from coprolites [see reviews in Bryant and Reinhard (2012) and Shillito et al. (2020a)]. Recent research has also shown the utility of biomolecular methods (e.g., lipids, aDNA) for providing important additional information in multi-proxy studies of human diet and paleoenvironments (Lin and Connor, 2001; Shillito et al., 2011; Sistiaga et al., 2014; Battillo and Fisher, 2015). Biomolecular methods have an added advantage of providing an unequivocal species identification, which is especially important in cases where coprolite morphology is ambiguous. By combining multiple lines of evidence, we can provide a fuller picture of past human-environment interactions. The use of multi-proxy methodologies is fast becoming the standard approach for archeological analysis; however, in practice, multiproxy analysis can be challenging. The nature of archeology as a practice means that individual analysts have expertise in different components contained within coprolites, and it can be challenging to assemble a team of experts that cover the wide range of potential materials that can be recovered from coprolites. This often leads to studies that focus on only a few proxies, rather than the entire assemblage of material contained within a coprolite.
Another issue is that coprolite studies are limited by the small number and size of coprolites recovered from archeological contexts (Hunt et al., 2012). Depending on the environment and burial conditions, coprolites can also undergo post-depositional transformation, and the quantity and quality of these small packages of information is further reduced over time (Schiffer, 1983). Given the destructive nature of sampling for most analyses, once a coprolite has been processed for one proxy, it is not always possible to go back and extract a different proxy from the same sample. Because coprolites are often a limited resource, it is essential to develop best-practice methods to maximize the amount of information that can be extracted and maintain an adequate archive of material that can be investigated with potential future methodological advances.
Existing coprolite processing protocols largely focus on extracting a select few types of materials, and the literature is lacking a comprehensive procedure for sequential extraction of biomolecules, macrofossils, pollen, phytoliths, starch grains, and parasite eggs. This paper describes recent methodological studies developed using coprolites from the Paisley Caves, south-central Oregon (Figure 1). The Paisley Caves have produced a large assemblage of human and non-human coprolites from terminal Pleistocene through late Holocene contexts, offering an important resource for the diachronic study of human behavior and the environment. Our current research program is focused on analysis of plant macrofossils, pollen, phytoliths, bone, insects, and fecal biomarkers from the Paisley coprolites, but we are also subsampling material for future parasite and starch grain analyses. In addition, our approach prioritizes archiving coprolite material for future research, for example aDNA analyses. As such, our study is ideal for establishing best-practice guidelines for multi-proxy coprolite analysis. There are two goals to this paper: (1) present a new method for sequential extraction of biomarkers, macrofossils, and microfossils, and (2) review the use of coprolite data for reconstructing human-environment interactions and paleoenvironments.
1.1. Review of previous coprolite processing methods
The goal of coprolite processing is to extract all preserved organic remains so that they can be individually analyzed by specialists (Pearsall, 2015; Shillito et al., 2020a). This review focuses on processing methods for desiccated coprolites recovered as discrete morphological units. Mineralized coprolites typically require a different suite of processing procedures (e.g., disaggregation in hydrochloric acid or analysis through thin section) (Chin, 2021). These methods are not covered in this protocol. However, the protocol detailed here can be adapted to mineralized coprolites (see Section “4. Discussion” below). Fecal material that has been disaggregated and incorporated into sediments (e.g., latrine soils) requires different processing methods (e.g., Warnock and Reinhard, 1992), and are also not covered here.
There was very little methodological standardization in early (pre-1960’s) coprolite research. However, many early coprolite studies used some variation of a “dry” processing approach primarily focused on extracting macroscopic (visible with the unaided eye) plant and animal material from the coprolite matrix [see reviews in Fry (1976):1–2 and Bryant and Dean (2006)]. The dry approach typically consisted of dissecting a desiccated coprolite using tweezers or similar implements, with the goal of extracting obvious macroscopic remains such as plant seeds, animal bones, and insect remains. Subsequent processing methods applied a rehydration approach consisting of soaking individual coprolites in a 0.5% solution of trisodium phosphate (Na3PO4) for 72+ h to rehydrate and soften desiccated coprolites (Callen, 1963). This approach made it easier to disaggregate the constituents of the coprolite, thereby preserving the structure of individual components for analysis. The trisodium phosphate rehydration approach has been widely adopted in coprolite analysis and is used in most studies today.
Early coprolite studies focused primarily on macroscopic remains; however, microscopic remains were often noted. For example Callen (1967) describes calcium oxalate crystals and starch grains preserved in plant cellular material recovered from human coprolites. Bryant and Williams-Dean (1975) also observed calcium oxalate crystals in coprolites that they link to consumption of Opuntia sp. (prickly pear cactus). A significant advancement for the formal analysis of microscopic remains in coprolites came with the extraction and analysis of pollen from human coprolites (Martin and Sharrock, 1964). In this study, the outside of the coprolite was not sampled to avoid potentially recovering ambient pollen that adhered to the exterior surface of the coprolite after it was deposited. Extraction was achieved by taking 1 cm3 from the interior of a desiccated coprolite, boiling in potassium hydroxide, applying “bleach” or acetolysis to remove organic material, and treatment with hydrofluoric acid to remove silicates (Martin and Sharrock, 1964:171).
The pioneering research by Martin and Sharrock was followed by studies refining methods for extracting and analyzing microfossils from coprolites. Bryant (1974b) developed a sequential method for extracting macroscopic plant remains and pollen grains from coprolite material. In Bryant’s method, a coprolite is cut along the long axis and one half is collected for analysis, ensuring that the analyst collects a subsample of the entire diet represented by the coprolite. The coprolite subsample is rehydrated in trisodium phosphate until fully reconstituted. The disaggregated coprolite and solution are then sieved through 841-micron and 210-micron mesh screen to separate macroscopic and microscopic fractions. The macroscopic fraction is dried and analyzed. The liquid fraction (<210 microns) is further treated to remove non-pollen material, including removal of carbonates using hydrochloric acid, removal of silicates using hydrofluoric acid, and removal of organic matter (primarily cellulose) through acetolysis. The acetolysis process consists of heating a pollen sample in a 9:1 mixture of acetic anhydride and sulfuric acid; a 9:1 ratio is appropriate for coprolites due to increased cellulose material (Erdtman, 1960; Faegri and Iversen, 1989). This method was one of the first to provide a formal sequence of steps to extract both macrofossils and microfossils from the same coprolite.
In the decades after Bryant’s method was introduced two basic approaches to multiproxy coprolite analysis emerged. One method followed Bryant and extracted proxy records sequentially from the same sample, and the other method used discrete samples that were collected and processed individually for a particular proxy record (Pearsall, 2015). Studies applying a discrete sampling method typically subsample a small amount of coprolite material from a particular place on a coprolite, or break the coprolite into separate segments and process each for a particular proxy (e.g., Wood and Wilmshurst, 2016). Studies have shown that separate samples collected from different locations on the same coprolite can provide statistically different pollen frequencies (Martin and Sharrock, 1964; Kelso and Solomon, 2006; Beck et al., 2019), and different macrofossil assemblages (Reinhard and Hevly, 1991), presumably representing different meals. While this has not been tested for other microfossils, it is assumed that the same holds true for phytoliths and starch (Beck et al., 2019). Therefore, a sequential method starting with a single sample collected along the length of the coprolite is recommended to avoid this issue; otherwise, the bias needs to be accounted for when interpreting the resulting data.
Pearsall (2015 and references therein) provides a sequential processing method for extracting pollen, parasite eggs, phytoliths, and non-silica crystals from fecal-related samples including latrine sediments and coprolites. The first step is to sieve the sample to separate microscopic remains from the microfossil solution. Pearsall recommends splitting the sieved microfossil solution into two subsamples (1 and 2) by shaking and pouring half of the liquid into another container. Subsample 1 is processed for pollen and parasites through (1) HF treatment to dissolve silicates, (2) sonication to disaggregate microfossils, and (3) heavy-density flotation to separate out microfossils based on their differing densities. Subsample 1 is split into two subsamples (1a and 1b) again following the procedure described above. Subsample 1a is treated with potassium hydroxide (KOH) to remove humic acids, then acetolysis; this subsample is analyzed for pollen. Subsample 1b is analyzed for parasite eggs and starch grains. Subsample 2 is treated with (1) hydrogen peroxide (H2O2) alone or in combination with potassium dichromate to dissolve organic material, (2) heavy density flotation to separate phytoliths and non-silica crystals, and (3) concentration by centrifugation. Splitting the liquid microfossil fraction into subsamples is necessary because microfossils can’t all be treated with the same chemical and physical processes; for example, hydrofluoric acid will dissolve silica phytoliths (Coil et al., 2003), and acetolysis can damage or destroy parasite eggs and cause starch grains to shrink (Warnock and Reinhard, 1992; Dozier et al., 2021). As Pearsall (2015:219) notes, it does not seem possible to recover organic and inorganic microfossils in a simultaneous procedure (i.e., without splitting into subsamples with different treatments). Because of this, most coprolite research today uses a variation of the sequential approach originally developed by Bryant and expanded upon by Pearsall and others (Blong and Shillito, 2021).
Biomolecular (e.g., DNA, lipids, and protein) analyses have fundamentally changed the field of coprolite analysis [see review in Shillito et al. (2020a)]. Biomolecular extraction techniques vary depending on the biomolecules being targeted. There are comprehensive reviews detailing the extraction and analysis of ancient DNA (Green and Speller, 2017) and proteins (Warinner et al., 2022). This study focuses on extraction of fecal lipid biomarkers specifically for the identification of the organism that deposited the coprolite; however, biomarkers resulting from consumption of plant and animal foods can also be analyzed from the same extraction. Previous research largely relied on qualitative approaches to identifying the organism that deposited a coprolite. For example, coprolites rehydrated in trisodium phosphate tend to color the trisodium phosphate solution (likely related to organism diet), and some researchers have used the color of the solution to estimate the origin of the coprolite (Bryant, 1974b); however, this is not always seen as a reliable indicator for coprolite origin (Fry, 1976; Blong et al., 2020). Similarly, the odor of the trisodium phosphate solution has been used as an indicator of coprolite origin (Callen, 1967; Bryant, 1974b). Odor is a subjective measure that is likely to be recorded differently from one researcher to the next, although recent research indicates that there are significant differences in the composition of volatile compounds in coprolites deposited by humans, carnivores, and herbivores, lending support to qualitative inferences of coprolite origin based on smell (Zhao et al., 2022).
Fecal biomarkers comprise lipids that derive from both dietary input and the digestive system of particular organisms and are therefore useful markers for determining both fecal origin and diet (Bull et al., 2002; Prost et al., 2017). The two classes of compounds utilized to determine fecal origin, 5β-stanols and bile acids, are produced within the digestive track and are thus fecal-specific (Murtaugh and Bunch, 1967; Hofmann, 1999). The relative proportions of 5β-stanols are indicative of the producer such that omnivores, herbivores and carnivores can be distinguished (Bull et al., 2002; Prost et al., 2017; Harrault et al., 2019). Characteristic suites of bile acids, which are species-specific, can further be used to distinguish between omnivores and confirm species identification (Simpson et al., 1999; Hagey et al., 2010). Analytical techniques generally follow protocols consisting of solvent extraction of the total lipid extract, followed by compound class separation using column chromatography, and derivatization prior to instrument analysis (Elhmmali et al., 2000; Bull et al., 2002). Analysis applies a combination of techniques which are capable of achieving compound level resolution; for example, gas chromatography (GC) and GC-mass spectrometry (GC-MS) (Evershed et al., 1990).
2. Materials and equipment
Basic laboratory equipment required to perform this protocol includes a fumehood, drying oven, muffle furnace capable of reaching 500°C, precision balance (0.01 g), centrifuge with capacity for 50-, 15-, and 1.5-mL centrifuge tubes and tubes in these sizes, heating block with capacity for 15-mL centrifuge tubes, vortex mixer, magnetic stirrer, Munsell soil color chart, 250 and 200 μm sieves, pestle and mortar, ceramic crucibles, and other standard lab materials as described below. Equipment required for GC-MS analysis includes microwave assisted extraction system (or equivalent extraction system, such as Soxhlet), nitrogen blow down system (or equivalent such as rotary evaporator), water purifier, GC column non-polar (Rtx-1, 50 m × 0.32 mm × 0.17 μm) or equivalent, gas chromatograph, and gas chromatograph-mass spectrometer. Chemical solutions and standards required for this protocol are listed in Table 1.
3. Sequential extraction method for coprolites
The sequential extraction protocol presented here was developed for coprolites from the Paisley Caves, Oregon, USA that were subsampled in a museum setting (Shillito et al., 2018, 2020b; Blong et al., 2020). However, it can be applied to any coprolite material that maintains morphological integrity. When working with coprolites, researchers should adopt safe working practices including wearing personal protective equipment such as a lab coat, powder-free latex gloves, and safety glasses. We also advise wearing a mask (e.g., N95 respirator) when documenting and subsampling coprolites to avoid breathing in any potentially hazardous dry particulate matter loosened during handling or cutting.
3.1. Documenting coprolites prior to sampling
We photographed two sides of each coprolite and documented the sample number, provenience, maximum length, width, and thickness to 0.01 mm, and weight to 0.1 g. We recorded physical characteristics following the standardized method presented in Jouy-Avantin et al. (2003), including state of preservation, volume, number of constrictions, shape of extremities, taphonomic modifications, inclusions, and hardness. These data are important for documenting all aspects of a coprolite prior to destructive analysis and can also provide insight into the organism that produced the coprolite.
Micro-computed tomography X-ray (μCT) imaging has been shown to be useful for documenting coprolite morphology (Bravo-Cuevas et al., 2017; Wang et al., 2018; Romaniuk et al., 2020). μCT data can be rendered using specialized software to produce a 3D image of a coprolite showing the overall morphological shape as well as the spatial location of material within the coprolite (Romaniuk et al., 2020). This technique can be used to preserve a record of a coprolite prior to destructive analysis and can also be added as a first step to guide subsampling location (e.g., targeting specific inclusions visible in the 3D image). Recent research indicates that μCT imaging can be used to identify faunal element and taxonomy in intact coprolites (Bravo-Cuevas et al., 2017; Romaniuk et al., 2020). This same approach may also be useful for identifying plant material in coprolites (e.g., large or distinctive seeds).
3.2. Subsampling and processing coprolites
After thoroughly documenting the physical characteristics of a coprolite, we cut the coprolite in half to collect our subsample. The goal of this procedure is to capture the entire sequence of meals that produced the coprolite by sampling along the long axis, thereby capturing the beginning though the end of the defecation episode. If the coprolite is cylindrical or conical (following Jouy-Avantin et al., 2003), it is typically straightforward to identify the long axis of the excreted feces and cut the coprolite along this axis. However, if the coprolite is spherical or flat, it can sometime be difficult to identify the long axis. In this case, the analyst can look for extremity features (e.g., rounded or sharp-pointed) to identify the beginning or end of the coprolite and subsample along the temporal axis. In some cases, it may not be possible to determine the beginning or end of a spherical or flat coprolite; in these instances, the analyst can use an arbitrary axis to cut the coprolite.
We recorded the weight of the subsample to the 0.1 g, and the remaining half of the coprolite was archived for future analysis. When possible, we scraped the exterior layer of our subsample to remove potential contaminants prior to processing. The coprolite is subdivided in the initial stage of processing: our protocol subsamples 1 g of coprolite for fecal biomarker analysis then proceeds with sequential extraction of plant and animal macrofossils, pollen, phytoliths, starch, and parasites (Figure 2). The fecal biomarker subsample should be collected from the interior of the coprolite to avoid any potential external contaminants. The fecal biomarker analysis presented here assumes that fecal biomarker signals are consistent throughout the coprolite, so this subsample can be collected from a single location inside the coprolite. The coprolite subsample is not homogenized prior to collecting the fecal biomarker sample to ensure that the appropriate analytical procedures are followed to reduce potential contamination. Biomarker preparation includes crushing and sieving to remove the coarse fraction, microwave extraction of lipids, hydrolyzation of lipid extract, and isolation of fractions by compound class. Fractions containing target biomarkers are analyzed by GC and GC-MS. Blanks are run with each batch of samples to check for contamination. Macro/microfossil preparation consists of rehydration in 0.5% trisodium phosphate and sieving to separate microfossils from microfossils. Microfossil processing includes deflocculation using 5% sodium hexametaphosphate, then subdividing microfossils into thirds for pollen, phytolith, and starch/parasite processing. Pollen processing includes dissolving organic compounds using acetolysis, heavy density separation using sodium polytungstate calibrated to 1.9 g/cm3, and staining/mounting extracted pollen. Phytolith processing includes removal of organic matter through combustion, heavy density separation using sodium polytungstate calibrated to 2.3 g/cm3 and mounting extracted phytoliths. At each step we viewed a subsample of waste material on a glass slide under a transmitted and polarized light microscope to ensure no microfossils were lost. A detailed step-by-step protocol for this procedure is provided in the Supplementary material.
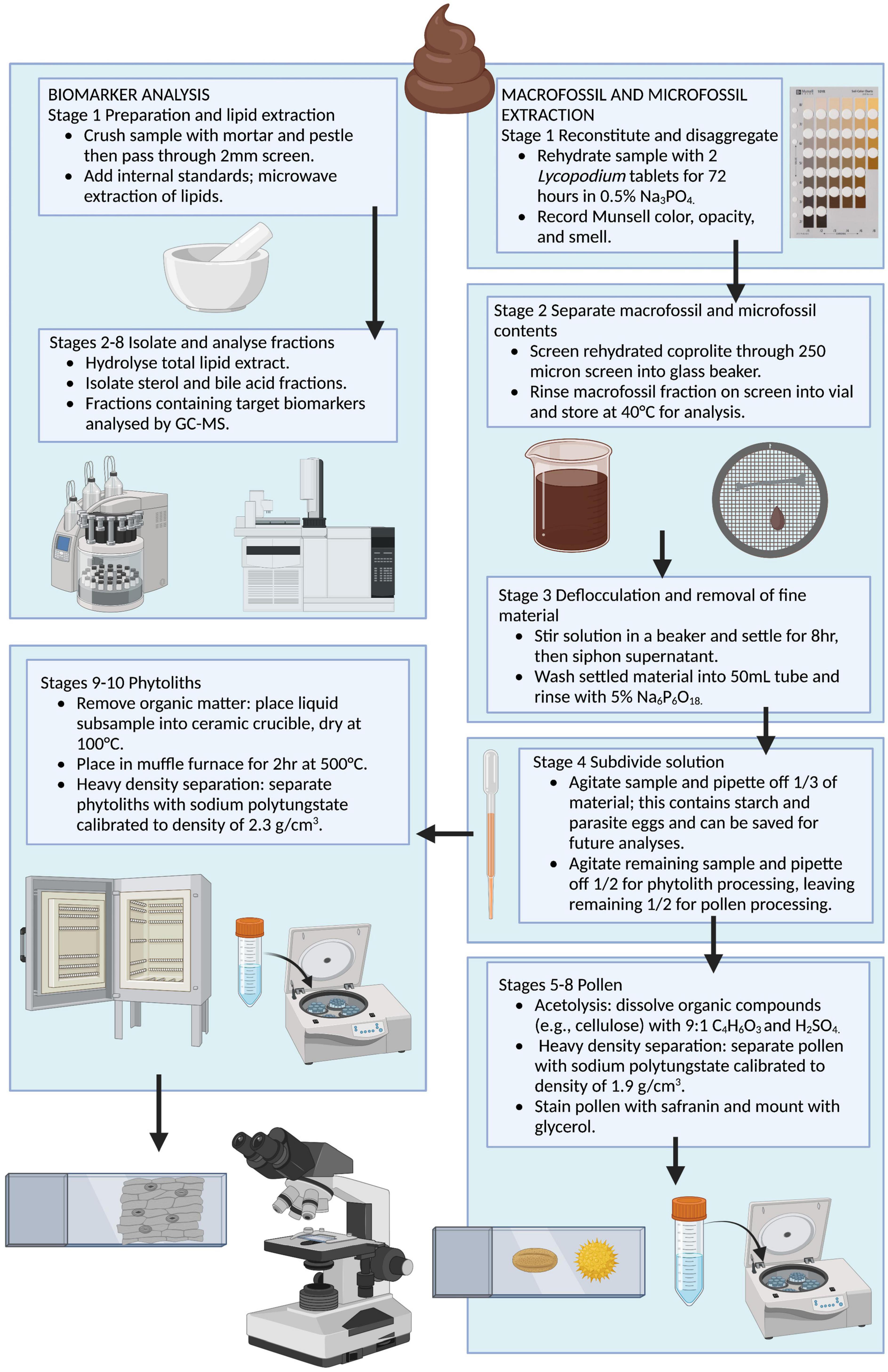
Figure 2. Flow chart illustrating the sequential biomolecular, macrofossil, and microfossil extraction method for coprolites. Created with Biorender.com.
4. Discussion
4.1. New protocol for sequential extraction from coprolites
The broader significance for this sequential extraction protocol is that it enables maximum data extraction from coprolites which are typically a limited resource in archeological assemblages. The sequential biomolecular, macrofossil, and microfossil extraction protocol presented here improves on previously published procedures (e.g., Wood and Wilmshurst, 2016) by extracting macro- and microfossils from a homogenized section of the entire length of the coprolite, thereby sampling remains of meals distributed throughout the coprolite. This is important because studies demonstrate that discrete sections collected from different locations on the same coprolite can provide significantly different dietary signals leading to different interpretations (Martin and Sharrock, 1964; Reinhard and Hevly, 1991; Beck et al., 2019). If coprolites need to be preserved in relatively intact form, we recommend taking a series of small (1 cm3) samples along the long axis of the coprolite, thereby preserving the original morphology of the coprolite, but still sampling the entire length. Our sampling strategy for biomarker analysis relies on a discrete 1 g subsample. This has proven sufficient in most cases to determine the organism that deposited the coprolite through fecal biomarker analysis (Shillito et al., 2020b). However, future research should focus on studying variability in fecal biomarker signatures in different locations of the coprolites and assessing whether this can impact resulting interpretations.
Our protocol also improves on previous procedures by excluding several corrosive and caustic chemicals typically used in microfossil processing with the goal of reducing workplace risks. Pearsall (2015) recommends treatment of the pollen subsample with potassium hydroxide (KOH) to remove organic matter, but this is typically only necessary for latrine soils. We did not observe a significant amount of residual organic matter in our samples after eliminating this step. Similarly, Pearsall (2015) recommends treating the phytolith subsample with hydrogen peroxide (H2O2) to remove organic matter. Our protocol eliminates this step by burning off organic matter in a muffle furnace. Hydrofluoric acid treatment is important for extracting pollen from sediments, but coprolites typically have relatively low silica content so this treatment is not needed (Reinhard et al., 2019). As noted above, hydrofluoric acid will destroy silica phytoliths, so removing this treatment preserves phytoliths while enabling the analyst to continue processing the entire sample as one prior to the final phytolith processing steps. Sodium polytungstate (Na6[H2W12O40]) is a non-toxic alternative to more toxic heavy density liquids (e.g., zinc bromide) typically used for heavy density separation (Gregory and Johnston, 1987; Coil et al., 2003), and can be easily recycled (Six et al., 1999).
It is important to note that this protocol was developed for our specific samples and research goals and may need to be adapted in other studies. For example, this study used a 250-micron sieve to separate macrofossils from microfossils; however, this may need to be adjusted to a smaller sieve size depending on the size of macrofossils expected to be recovered. An alternative approach would be to sieve the rehydrated and disaggregated coprolite through nested sieves with 250-micron and 125-micron mesh to ensure recovery of smaller macrofossils. Genetic studies of coprolite material will require more stringent anti-contamination sampling procedures (Wood and Wilmshurst, 2016). Coprolites typically have low carbonate content, so treatment with hydrochloric acid is not needed (Reinhard et al., 2019). However, it may be necessary to treat coprolites if they have been impacted by calcium carbonate mineralization. To avoid destroying calcium oxalate crystals and damaging bone fragments, samples can be treated with glacial acetic acid which will dissolve carbonates but not calcium oxalate crystals (Coil et al., 2003). Once demineralized, the sample can progress through the protocol provided above. Several studies have raised important concerns about controlling starch contamination in ancient starch laboratories (Crowther et al., 2014; Henry, 2020). Of particular concern is evidence for modern starch contamination in common lab consumables such as nylon gloves, pipettes, towels, and even in lab chemicals such as sodium polytungstate (Crowther et al., 2014). Starch contamination is potentially a serious issue and care should be taken to test for contamination and avoid contaminated lab products.
4.2. Coprolites as an archive of human-environment interactions
The method detailed here has been successfully applied to a long-term study of coprolites from the Paisley Caves, Oregon, USA (Figures 3, 4). Gilbert et al. (2008) extracted mitochondrial DNA (mtDNA) corresponding to Native American founding haplogroups from six Paisley Caves coprolites, three of which were radiocarbon dated to between 12,400 ± 60 and 12,140 ± 70 14C years BP (14,850–13,795 cal BP). At the time, this was one of a handful of sites providing well-accepted evidence for human presence in the Americas prior to 13,000 cal BP (Goebel et al., 2008). The mtDNA and radiocarbon dating were criticized in subsequent publications (Goldberg et al., 2009; Poinar et al., 2009). Despite efforts to respond to these critiques (Gilbert et al., 2009; Rasmussen et al., 2009), the evidence for early human presence at the caves continued to be disputed (Fiedel, 2014). Following the processing method outlined here, we determined that 13 of 21 Paisley Caves coprolites with human mtDNA were in fact human, including three of the pre-13,000 cal BP coprolites (Shillito et al., 2018, 2020b). This established that humans occupied the Paisley Caves prior to 13,000 cal BP. We used the sequential extraction method presented here to study plant and animal macrofossil (e.g., seed, bone, and chitin), pollen, and phytolith dietary evidence from the Western Stemmed Tradition (WST) occupation dating to 12,900–9,000 cal BP at the Paisley Caves. Our study provided information on foods consumed during the WST period, season of occupation, and the ecological landscapes people interacted with (Blong et al., 2020).
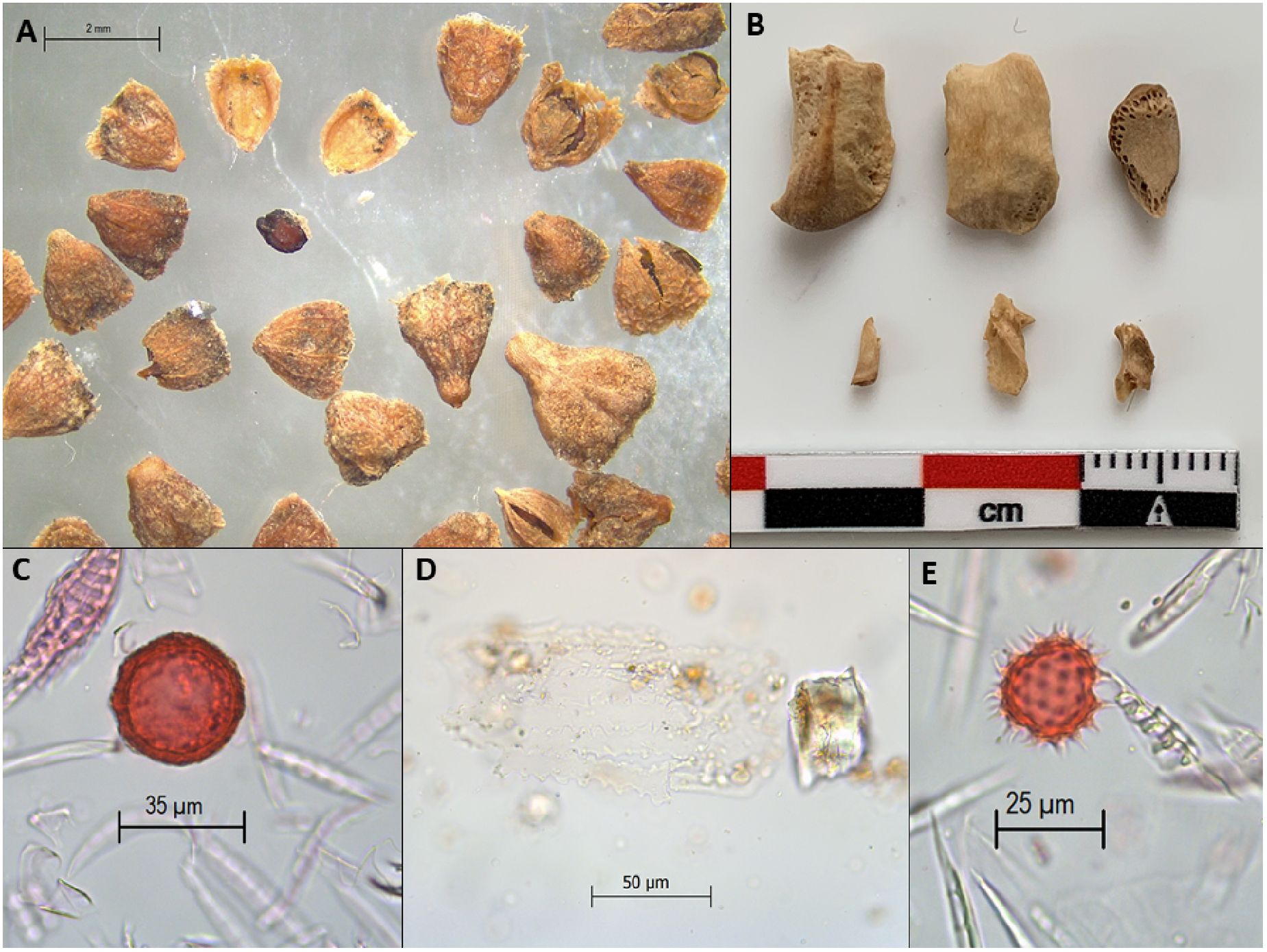
Figure 3. Examples of macroscopic and microscopic plant and animal remains recovered from Paisley Caves coprolites: (A) Amaranthaceae Atriplex sp. seeds, (B) bone fragments, (C) Taeniidae Taenia sp. egg, (D) conjoined phytolith sheet, (E) Asteraceae pollen.
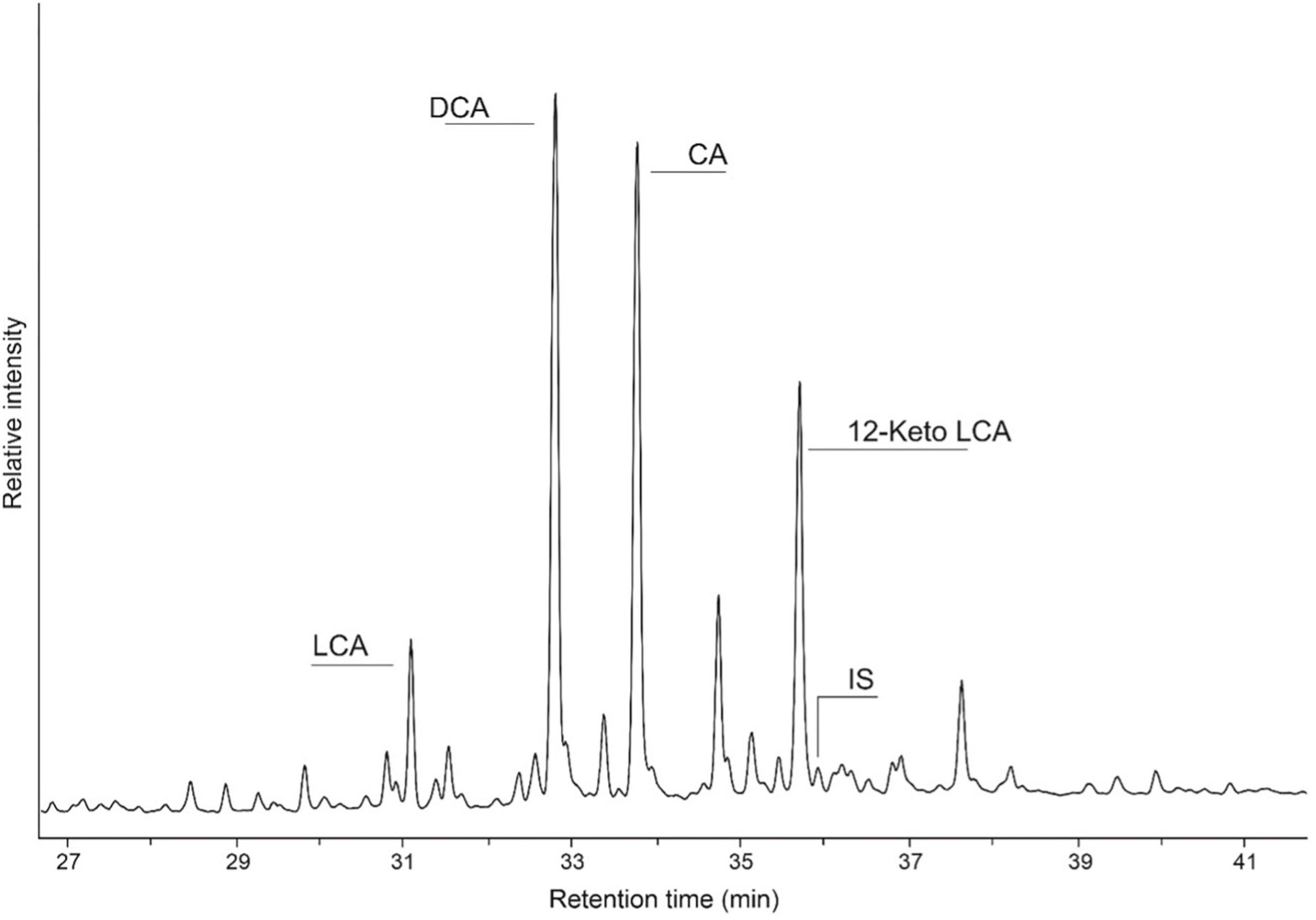
Figure 4. Partial gas chromatogram illustrating the distribution of bile acids in a coprolite identified as human, where LCA is lithocholic acid, DCA is deoxycholic acid, CA is cholic acid (CA), and IS denotes the added internal standard hyocholic acid (HCA).
There are numerous additional studies that use macroscopic, microscopic, and molecular remains extracted from human coprolites to investigate human-environment interactions. McDonough (2019) applies multiproxy analysis of human coprolites from southcentral Oregon, USA to reconstruct season of occupation and foraging landscape in the late Middle Holocene period, a time of cooler, wetter climate. These data indicate that Middle Holocene humans mapped onto productive lowland wetland environments but traveled to seasonally-available resources in higher elevations, a strategy well-suited to the vast lowland wetland systems that characterize this period. Battillo (2019) applies multiproxy analysis of coprolites including ancient DNA (aDNA) analysis to determine that farming populations in the North American Southwest consumed a significant amount of wild plant resources, particularly weeds associated with agricultural fields, to supplement maize agriculture. This study demonstrates that agriculturalist populations relied on traditional ecological knowledge of wild foods even after the adoption of maize agriculture and practiced landscape management strategies that increased overall resource availability.
Other studies have demonstrated the utility of coprolite analysis for the study of the use of different ecological landscapes (Watson and Yarnell, 1966; Callen, 1967; Holden, 1991; Sutton, 1993; Gremillion, 1996; Oeggl et al., 2007; Sobolik, 2008; Sonderman et al., 2019; Zhang et al., 2020a), seasonal use of sites/locations on the landscape (Bryant, 1974a,b; Sutton, 1998; Riley, 2008, 2012; Martínez Tosto et al., 2016; Blong et al., 2020; Zhang et al., 2020b), and use of space in settlements (Shillito et al., 2011). Coprolites from domesticated animals can also provide important information on human land use and settlement patterns in the past (Horrocks et al., 2002; Égüez et al., 2020; Portillo et al., 2020; Romaniuk et al., 2020; Fuks and Dunseth, 2021). These studies demonstrate the strength of coprolites as a means for understanding human behavior in the context of past environments.
4.3. Coprolites and paleoecological reconstruction
Taxonomic identification is important when interpreting past environments from coprolite data because we need to understand the behavior of the organism that deposited the coprolite to evaluate the potential origin of the proxy records represented in the coprolite. For example, Horrocks et al. (2003) suggest that animals such as canids are continuously sniffing the ground and might have a more diverse pollen spectrum in their coprolites because they are ingesting pollen from zoophilous taxa not well represented in atmospheric pollen. Herbivores selectively consume particular plant species whose pollen will be well represented in their feces, and there should be little variability from one individual to the next foraging in the same environment/season (King, 1977). However, the vegetation they consume will also be coated in pollen rain representing regional vegetation (Carrion, 2002). Specific taxonomic identifications can also be used to evaluate geographic distribution and niche occupation of non-human species in the past (Bravo-Cuevas et al., 2017; Wang et al., 2018). Many paleoecological coprolite studies focus on presumed carnivore coprolites, but often this is determined through qualitative methods and the organism that produced the coprolite is often equivocal (e.g., Horrocks et al., 2003; Ochando et al., 2022). Our method can be used to distinguish among human, herbivore, and carnivore coprolites so the researcher knows what data considerations and evaluations of biases must be made.
Macroscopic, microscopic, and molecular remains from coprolites are products of the environment that the organism depositing the coprolite lived in, so these remains have utility for paleoecological reconstruction. Often paleoecological reconstruction is approached through pollen recovered from coprolites. Pollen analysis has a long history of application in paleoecological reconstruction (Faegri and Iversen, 1989; Moore et al., 1991). Palynological paleoecological reconstruction is based on the concept of pollen rain, representing pollen from all flowering plants in a vegetation community carried by prevailing weather patterns and deposited on the landscape. There is typically seasonal variation in the composition of pollen rain linked to flowering times of plants in a vegetation community, and annual changes based on variables such as wind direction during flowering time. However, over several years these variations will even out creating a homogenized pollen rain (Faegri and Iversen, 1989:21). Samples analyzed for paleoecological reconstruction typically represent pollen deposited over many years if not decades, so pollen extracted from sedimentary records are typically thought to be a proxy for vegetation landscapes. There are many complex considerations that must be made when interpreting pollen rain data, but the core concept is that changes in pollen composition over time and space represent changes in vegetation communities on the landscape (Prentice, 1988; Seppä, 2007).
Pollen from coprolites represents pollen ingested within 1–2 days of an organisms life, not the homogenized multi-year signal represented by pollen rain in sediments (Horrocks et al., 2003; Shillito et al., 2020a). This needs to be considered when reconstructing paleoecological conditions from coprolite pollen. Despite this caveat, pollen from non-human coprolites has demonstrated utility for paleoecological reconstruction and has been shown to correlate with local and regional sedimentary pollen records (Horrocks et al., 2003). Pollen records from non-human coprolites have proven to be particularly useful for their representation of local and/or seasonally flowering zoophilous taxa that typically produce less pollen than anemophilous taxa (Carrion, 2002; Velazquez et al., 2017; Velázquez et al., 2020; Ochando et al., 2022). Studies comparing pollen in “fresh” non-human feces (primarily herbivore) to pollen in surface sediments provide evidence that feces contain many zoophilous pollen types that are rare in sediments, and conversely, pollen of anemophilous taxa are underrepresented in feces relative to sediments (King, 1977; Moe, 1983; Carrion, 2002). These studies indicate that pollen in feces is invaluable for identifying zoophilous vegetation with limited environmental distribution and are therefore of use for determining the local foraging environment of the organism that deposited the feces. In this way, pollen from non-human feces can be used to correct for overrepresentation of anemophilous pollen in sedimentary records (Carrion, 2002; Ochando et al., 2022).
Pollen records from non-human coprolites are particularly important in arid regions that do not have well-represented wetland or lacustrine depositional landscapes typically cored for paleoecological reconstruction (Carrion, 2002; Velázquez et al., 2020). These arid settings are often conducive for preservation of coprolites in sheltered locations, providing a source of material for paleoecological reconstruction. Given the proportional underrepresentation of anemophilous taxa in coprolites, care must be taken when interpreting past vegetation landscapes from coprolites alone; however, in arid environments this is sometimes the only paleoecological proxy available (Carrion, 2002).
Pollen extracted from human coprolites is considered less valuable for paleoecological reconstruction because of the selective cultural behaviors often represented in human fecal pollen (Martin and Sharrock, 1964; Bryant, 1974b). Like non-human coprolites, human coprolites can contain relatively high frequencies of zoophilous pollen when compared to natural pollen rain frequencies obtained from sediments/cores; this is typically interpreted to represent intentional ingestion of zoophilous plant taxa. However, environmental pollen representing unintentional ingestion (e.g., inhaled, consumed in drinking water, or settled on food) is also present and can reflect local and/or seasonally flowering vegetation, suggesting that paleoecological reconstruction using pollen from human coprolites is an underutilized approach (Martin and Sharrock, 1964). For example, it has been shown that human coprolites from the same site and temporal context had no detectible differences in anemophilous taxa representing environmental (non-cultural) derived pollen (Reinhard et al., 2006). This suggests that coprolites may have a consistent environmental pollen signal that can be used for paleoecological reconstruction, while also providing seasonal signals for both vegetation reconstruction, and human/animal diet and seasonal movements.
Phytoliths recovered from non-human coprolites have demonstrated utility for paleoecological reconstruction (Bamford et al., 2010; Shillito, 2011; Dunseth et al., 2019; Álvarez-Barra, 2020). The size of phytoliths recovered from coprolites can be used as an indicator of past environments, particularly related to water availability (Shillito, 2011). Phytoliths recovered from herbivore and carnivore coprolites have been used to reconstruct vegetation patterns, for example the distribution of taxa with restricted ecological tolerance, and the ratio of C3 and C4 grasses on the landscape, both of which have a direct relationship to past climate (Bamford et al., 2010; Álvarez-Barra, 2020). One issue with the use of phytoliths as a paleoecological proxy is the often low taxonomic value of most phytolith morphological types (Piperno, 1988). This highlights the need to study multiple coprolite proxy records including phytoliths to provide a suite of data that can overcome the shortcomings of individual proxy records (Dunseth et al., 2019).
Environmental DNA and aDNA extracted from coprolites can be a useful tool for reconstructing foraging environments in the past, particularly when used in tandem with pollen and plant macrofossils extracted from the coprolite (van Geel et al., 2008; Green and Speller, 2017). Stable carbon and nitrogen isotopes and aDNA from domesticated dog coprolites were combined with macrofossil data to reconstruct late Holocene dietary patterns in the American Bottom (Witt et al., 2021). In Witt et al.’s (2021) study, isotopes were used to reconstruct the role of fish and C3/C4 plant foods in dog diets as a way of better understanding human diets during this period. This study was notable for the use of aDNA to reconstruct the microbiome signature of the coprolite, which was then used to confirm the coprolites were deposited by dogs. This mirrors recent research applying aDNA analysis of gut microbiomes in coprolites to identify the organism that deposited the coprolite (Borry et al., 2020) and explore the evolutionary history of human gut microbiota (Wibowo et al., 2021). Stable carbon and nitrogen isotopes in non-human coprolites have been used to characterize the isotopic composition of human food sources (Iacumin et al., 1998), foraging environment (Rawlence et al., 2016), and for food web and associated paleoecological reconstruction (Barrios-de Pedro et al., 2020; Mychajliw et al., 2020). Isotopic analysis has been applied to Cretaceous- and Carboniferous-period coprolites to assess diet and gastrointestinal physiology, demonstrating the utility of this approach for understanding organism-environment interactions millions of year ago (Ghosh et al., 2003; Tripp et al., 2022).
Faunal material recovered from coprolites can also provide paleoecological data. A recent study at the Paisley Caves reconstructed the paleohydrology of the surrounding Chewaucan basin based on changes in the frequency of lake and stream-dwelling fish remains recovered from archeological deposits at the caves, as well as the carbon, oxygen, and strontium isotopes in the fish remains (Hudson et al., 2021). The climate of the Great Basin warmed in the Holocene, and pluvial lakes and wetlands dried up during this time. The frequency of fish remains from the Paisley Caves peak during the wetter Bølling-Allerød interstadial and early part of the Younger Dryas, reflecting more habitat availability. Fish isotopes reflect the habitat that the fish lived in, and Paisley Caves fish bones from the Bølling-Allerød interstadial indicate a deep, open-lake habitat. This contrasts with fish bones representing the early to middle Holocene which have signatures indicating a freshwater wetland habitat close to spring outlets, suggesting contracted wetland and lake environments (Hudson et al., 2021). Coprolites from the Paisley Caves contain bone from fish and other organisms (Blong et al., 2020), so coprolites are potentially an important data source for isotopic studies. The application of these methods to coprolite studies is relatively new but shows promise moving forward (Shillito et al., 2020a).
To date, non-human coprolites recovered from archeological sites have provided the most potential for paleoecological reconstruction, but the increasing focus on multiproxy analyses incorporating new biomolecular techniques indicates that there is potential for paleoecological data recorded in coprolites deposited by any organism, including humans.
5. Conclusion
The sequential multiproxy processing protocol presented here provides a step-by-step approach to extracting biomolecular, macroscopic, and microscopic remains from coprolites. This protocol improves on previous methods by subsampling one half of a coprolite and sequentially extracting a variety of remains while still maximizing the amount of information obtained from valuable coprolite samples. The approach described here also uses relatively safe chemical procedures, thereby reducing workplace risks. This sequential multiproxy protocol has provided important information on the timing and nature of human use of the Paisley Caves, Oregon, but can be adapted to any coprolite studies around the world. The multiproxy records that can be extracted using this method also can be used for paleoecological reconstruction. Multiproxy approaches to investigating past human-environment interactions and paleoenvironments can be challenging but are becoming the standard for environmental archeology studies. These studies have broader implications as we work to understand the potential impacts of current and future social and environmental challenges.
Data availability statement
Publicly available datasets were analyzed in this study. This data can be found here: https://doi.org/10.1007/s12520-020-01160-9 and https://www.science.org/doi/10.1126/sciadv.aba6404.
Author contributions
L-MS and IB conceptualized this research. JB, HW, EA, L-MS, and IB developed the methodology. JB, HW, and EA carried out the investigation. JB and HW wrote the original manuscript draft. EA, L-MS, and IB reviewed and edited the manuscript. All authors contributed to the article and approved the submitted version.
Funding
This research was funded by the UK Natural Environment Research Council NE/P003001/1.
Acknowledgments
We thank to Katelyn McDonough provided valuable feedback on the methods developed for this study. We thank also to Simon Drew assisted with laboratory processing. We appreciate the thoughtful comments by two reviewers that helped to improve this manuscript.
Conflict of interest
The authors declare that the research was conducted in the absence of any commercial or financial relationships that could be construed as a potential conflict of interest.
Publisher’s note
All claims expressed in this article are solely those of the authors and do not necessarily represent those of their affiliated organizations, or those of the publisher, the editors and the reviewers. Any product that may be evaluated in this article, or claim that may be made by its manufacturer, is not guaranteed or endorsed by the publisher.
Supplementary material
The Supplementary Material for this article can be found online at: https://www.frontiersin.org/articles/10.3389/fevo.2023.1131294/full#supplementary-material
References
Álvarez-Barra, V. (2020). “Phytolith analysis from coprolites of Pilauco,” in Pilauco: A late pleistocene Archaeo-paleontological site Osorno, northwestern Patagonia and Chile, eds M. Pino and G. A. Astorga (Switzerland: Springer), 183–193. doi: 10.1007/978-3-030-23918-3_11
Bamford, M. K., Neumann, F. H., Pereira, L. M., Scott, L., Dirks, P. H., and Berger, L. R. (2010). Botanical remains from a coprolite from the Pleistocene hominin site of Malapa, Sterkfontein Valley, South Africa. Palaeontol. Afr. 45, 23–28.
Barrios-de Pedro, S., Rogers, K. M., Alcorlo, P., and Buscalioni, Á. D. (2020). Food web reconstruction through isotopic compositions of fossil faeces: Insights into the ecology of a late Barremian freshwater ecosystem (Las Hoyas, Cuenca, Spain). Cretac. Res. 108:104343. doi: 10.1016/j.cretres.2019.104343
Battillo, J. (2019). Farmers who forage: Interpreting paleofecal evidence of wild resource use by early corn farmers in the North American Southwest. Archaeol. Anthropol. Sci. 11, 5999–6016. doi: 10.1007/s12520-019-00944-y
Battillo, J. M., and Fisher, A. E. (2015). Reconstructing meat consumption through biomarker analyses of paleofeces. Ethnobiol. Lett. 6, 111–113. doi: 10.14237/ebl.6.1.2015.401
Beck, C. W., Bryant, V. M., and McDonough, K. N. (2019). Evidence for non-random distribution of pollen in human coprolites. Archaeol. Anthropol. Sci. 11, 5983–5998. doi: 10.1007/s12520-019-00839-y
Blong, J. C., Adams, M. E., Sanchez, G. M., Jenkins, D. L., Bull, I. D., and Shillito, L. (2020). Younger Dryas and early Holocene subsistence in the Northern Great Basin: Multiproxy analysis of coprolites from the Paisley Caves, Oregon, USA. Archaeol. Anthropol. Sci. 12, 1–29. doi: 10.1007/s12520-020-01160-9
Blong, J. C., and Shillito, L.-M. (2021). Coprolite research: Archaeological and paleoenvironmental potentials. Archaeol. Anthropol. Sci. 13:15. doi: 10.1007/s12520-020-01242-8
Borry, M., Cordova, B., Perri, A., Wibowo, M., Honap, T. P., Ko, J., et al. (2020). CoproID predicts the source of coprolites and paleofeces using microbiome composition and host DNA content. PeerJ 2020:e9001. doi: 10.7717/peerj.9001
Bravo-Cuevas, V., Morales-García, N., Barrón-Ortiz, C., Theodor, J., and Cabral-Perdomo, M. (2017). Canid coprolites from the late pleistocene of Hidalgo, Central Mexico: Importance for the carnivore record of North America. Ichnos 24, 239–249. doi: 10.1080/10420940.2016.1270209
Bryant, V. M. (1974b). Prehistoric diet in Southwest Texas: The coprolite evidence. Am. Antiq. 39, 407–420. doi: 10.2307/279430
Bryant, V. M. (1974a). “Pollen analysis of prehistoric human feces from mammoth cave,” in Archaeology of the Mammoth Cave area, ed. P. J. Watson (Cambridge: Academic Press), 203–249.
Bryant, V. M. J., and Williams-Dean, G. (1975). The Coprolites of Man. Sci. Am. 232, 100–109. doi: 10.1038/scientificamerican0175-100
Bryant, V. M., and Dean, G. W. (2006). Archaeological coprolite science: The legacy of Eric O, Callen (1912–1970). Palaeogeogr. Palaeoclimatol. Palaeoecol. 237, 51–66. doi: 10.1016/j.palaeo.2005.11.032
Bryant, V. M., and Reinhard, K. J. (2012). “Coprolites and archaeology: The missing links in understanding human health,” in Vertebrate coprolites ed. A. Hunt (Albuquerque: New Mexico Museum of Natural History and Science), 379–387.
Bull, I., Lockheart, M., Elhmmali, M., Roberts, D., and Evershed, R. (2002). The origin of faeces by means of biomarker detection. Environ. Int. 27, 647–654. doi: 10.1016/S0160-4120(01)00124-6
Callen, E. O. (1963). “Diet as revealed by coprolites,” in Science in archaeology: A comprehensive survey of progress and research, eds D. Brothwell and E. Higgs (Bristol: Thames and Hudson Ltd), 186–194.
Callen, E. O. (1967). “Analysis of the Tehuacan coprolites,” in The prehistory of the Tehuacan valley, Vol. 1: Environment and subsistence, ed. D. S. Byers (Austin: University of Texas Press), 261–289.
Carrion, J. S. (2002). A taphonomic study of modern pollen assemblages from dung and surface sediments in arid environments of Spain. Rev. Palaeobot. Palynol. 120, 217–232. doi: 10.1016/S0034-6667(02)00073-8
Chin, K. (2021). “Gastrointestinal parasites of ancient nonhuman vertebrates: Evidence from coprolites and other materials,” in The evolution and fossil record of parasitism, eds K. De Baets and J. W. Huntley (Switzerland: Springer Nature), 359–375. doi: 10.1007/978-3-030-52233-9_11
Coil, J., Korstanje, M. A., Archer, S., and Hastorf, C. A. (2003). Laboratory goals and considerations for multiple microfossil extraction in archaeology. J. Archaeol. Sci. 30, 991–1008. doi: 10.1016/S0305-4403(02)00285-6
Crowther, A., Haslam, M., Oakden, N., Walde, D., and Mercader, J. (2014). Documenting contamination in ancient starch laboratories. J. Archaeol. Sci. 49, 90–104. doi: 10.1016/j.jas.2014.04.023
de Noort, R. (2011). Conceptualising climate change archaeology. Antiquity 85, 1039–1048. doi: 10.1017/S0003598X00068472
Dozier, C. A., Perrotti, A. G., and Howard, E. V. (2021). Effects of acetolysis on starch granules. J. Archaeol. Sci. Rep. 36, 1–6. doi: 10.1016/j.jasrep.2021.102818
Dunseth, Z. C., Fuks, D., Langgut, D., Weiss, E., Melamed, Y., Butler, D. H., et al. (2019). Archaeobotanical proxies and archaeological interpretation: a comparative study of phytoliths, pollen and seeds in dung pellets and refuse deposits at early Islamic Shivta, Negev, Israel. Quat. Sci. Rev. 211, 166–185. doi: 10.1016/j.quascirev.2019.03.010
Égüez, N., Dal Corso, M., Wieckowska-Lüth, M., Delpino, C., Tarantini, M., and Biagetti, S. (2020). A pilot geo-ethnoarchaeological study of dung deposits from pastoral rock shelters in the Monti Sibillini (central Italy). Archaeol. Anthropol. Sci. 12:114. doi: 10.1007/s12520-020-01076-4
Elhmmali, M. M., Roberts, D. J., and Evershed, R. P. (2000). Combined analysis of bile acids and sterols/stanols from riverine particulates to assess sewage discharges and other fecal sources. Environ. Sci. Technol. 34, 39–46. doi: 10.1021/es990076z
Evershed, R. P., Heron, C., and Goad, L. J. (1990). Analysis of organic residues of archaeological origin by high-temperature gas chromatography and gas chromatography-mass spectrometry. Analyst 115, 1339–1342. doi: 10.1039/an9901501339
Fiedel, S. J. (2014). Did pre-clovis people inhabit the Paisley Caves (and why does it matter)? Hum. Biol. 86, 69–74. doi: 10.3378/027.086.0104
Fry, G. F. (1976). “Analysis of prehistoric coprolites from Utah,” in University of Utah anthropologcial papers, eds J. D. Jennings and L. S. Sweeney (Salt Lake City, UT: University of Utah Press).
Fuks, D., and Dunseth, Z. C. (2021). Dung in the dumps: What we can learn from multi-proxy studies of archaeological dung pellets. Veg. Hist. Archaeobot. 30, 137–153. doi: 10.1007/s00334-020-00806-x
Ghosh, P., Bhattacharya, S. K., Sahni, A., Kar, R., Mohabey, D., and Ambwani, K. (2003). Dinosaur coprolites from the late cretaceous (Maastrichtian) Lameta formation of India: Isotopic and other markers suggesting a C3 plant diet. Cretac. Res. 24, 743–750. doi: 10.1016/j.cretres.2003.08.002
Gilbert, M. T., Jenkins, D. L., Götherstrom, A., Naveran, N., Sanchez, J. J., Hofreiter, M., et al. (2008). DNA from pre-clovis human coprolites in Oregon, North America. Science 320, 786–789. doi: 10.1126/science.1154116
Gilbert, M., Jenkins, D., Higham, T., Rasmussen, M., Malmström, H., Svensson, E., et al. (2009). Response to comment by Poinar et al. on “DNA from pre-clovis human coprolites in Oregon, North America. Science 325:148. doi: 10.1126/science.1168457
Goebel, T., Waters, M. R., and ORourke, D. H. (2008). The late pleistocene dispersal of modern humans in the Americas. Science 319, 1497–1502. doi: 10.1126/science.1153569
Goldberg, P., Berna, F., and Macphail, R. I. (2009). Comment on “DNA from pre-clovis human coprolites in Oregon, North America. Science 325:148. doi: 10.1126/science.1167531
Green, E. J., and Speller, C. F. (2017). Novel substrates as sources of ancient DNA: Prospects and hurdles. Genes 8, 1–26. doi: 10.3390/genes8070180
Gregory, M. R., and Johnston, K. A. (1987). A nontoxic substitute for hazardous heavy liquids-aqueous sodium polytungstate (3Na2WO4.9WO3.H2O) solution. N. Z. J. Geol. Geophys. 30, 317–320. doi: 10.1080/00288306.1987.10552626
Gremillion, K. J. (1996). Early agricultural diet in eastern north America: Evidence from Two Kentucky Rockshelters. Am. Antiq. 61, 520–536. doi: 10.2307/281838
Hagey, L. R., Vidal, N., Hofmann, A. F., and Krasowski, M. D. (2010). Evolutionary diversity of bile salts in reptiles and mammals, including analysis of ancient human and extinct giant ground sloth coprolites. BMC Evol. Biol. 10:133. doi: 10.1186/1471-2148-10-133
Harrault, L., Milek, K., Jardé, E., Jeanneau, L., Derrien, M., and Anderson, D. G. (2019). Faecal biomarkers can distinguish specific mammalian species in modern and past environments. PLoS One 14:e0211119. doi: 10.1371/journal.pone.0211119
Henry, A. G. (2020). “Starch granules as markers of diet and behavior,” in Handbook for the analysis of micro-particles in archaeological samples, ed. A. G. Henry (Switzerland: Springer), 97–116. doi: 10.1007/978-3-030-42622-4_5
Hofmann, A. F. (1999). Bile acids: The good, the bad, and the ugly. News Physiol. Sci. 14, 24–29. doi: 10.1152/physiologyonline.1999.14.1.24
Holden, T. G. (1991). Evidence of prehistoric diet from Northern Chile: Coprolites, gut contents and flotation samples from the Tulan Quebrada. World Archaeol. 22, 320–331. doi: 10.1080/00438243.1991.9980149
Horrocks, M., Irwin, G. J., McGlone, M. S., Nichol, S. L., and Williams, L. J. (2003). Pollen, phytoliths and diatoms in prehistoric coprolites from Kohika, Bay of Plenty, New Zealand. J. Archaeol. Sci. 30, 13–20. doi: 10.1006/jasc.2001.0714
Horrocks, M., Jones, M. D., Beever, R. E., and Sutton, D. G. (2002). Analysis of plant microfossils in prehistoric coprolites from Harataonga Bay, Great Barrier Island, New Zealand. J. R. Soc. N. Z. 32, 617–628. doi: 10.1080/03014223.2002.9517712
Hudson, A. M., Emery-Wetherell, M. M., Lubinski, P. M., Butler, V. L., Grimstead, D. N., and Jenkins, D. L. (2021). Reconstructing paleohydrology in the northwest Great Basin since the last deglaciation using Paisley Caves fish remains (Oregon, U.S.A.). Quat. Sci. Rev. 262:106936. doi: 10.1016/j.quascirev.2021.106936
Hunt, A., Lucas, S., Milàn, J., and Spielmann, J. (2012). Vertebrate coprolite studies: Status and prospectus. N. Mexico Mus. Nat. Hist. Sci. Bull. 57, 5–24.
Iacumin, P., Bocherens, H., Chaix, L., and Marioth, A. (1998). Stable carbon and nitrogen isotopes as dietary indicators of ancient Nubian populations (Northern Sudan). J. Archaeol. Sci. 25, 293–301. doi: 10.1006/jasc.1997.0206
Jouy-Avantin, F., Debenath, A., Moigne, A., and Moné, H. (2003). A standardized method for the description and the study of coprolites. J. Archaeol. Sci. 30, 367–372. doi: 10.1006/jasc.2002.0848
Kelso, G. K., and Solomon, A. M. (2006). Applying modern analogs to understand the pollen content of coprolites. Palaeogeogr. Palaeoclimatol. Palaeoecol. 237, 80–91. doi: 10.1016/j.palaeo.2005.11.036
King, F. B. (1977). An evaluation of the pollen contents of coprolites as environmental indicators. J. Ariz. Acad. Sci. 12, 47–52. doi: 10.2307/40021980
Lin, D. S., and Connor, W. E. (2001). Fecal steroids of the coprolite of a Greenland Eskimo mummy, AD 1475: A clue to dietary sterol intake. Am. J. Clin. Nutr. 74, 44–49. doi: 10.1093/ajcn/74.1.44
Martin, P. S., and Sharrock, F. W. (1964). Pollen analysis of prehistoric human feces: A new approach to ethnobotany. Am. Antiq. 30, 168–180. doi: 10.2307/278848
Martínez Tosto, A., Burry, L., Arriaga, M., and Civalero, M. (2016). Archaeobotanical study of Patagonian Holocene coprolites, indicators of diet, cultural practices and space use. J. Archaeol. Sci. 10, 204–211. doi: 10.1016/j.jasrep.2016.09.017
McDonough, K. N. (2019). Middle Holocene menus: Dietary reconstruction from coprolites at the Connley Caves, Oregon, USA. Archaeol. Anthropol. Sci. 11, 5963–5982. doi: 10.1007/s12520-019-00828-1
Moe, D. (1983). Palynology of sheeps faeces: Relationship between pollen content, diet and local pollen rain. Grana 22, 105–113. doi: 10.1080/00173138309431970
Moore, P. D., Webb, J. A., and Collison, M. E. (1991). Pollen analysis. Hoboken, NJ: Blackwell scientific publications.
Murtaugh, J. J., and Bunch, R. L. (1967). Sterols as a measure of fecal pollution. J. Water Pollut. Control Fed. 39, 404–409.
Mychajliw, A. M., Rice, K. A., Tewksbury, L. R., Southon, J. R., and Lindsey, E. L. (2020). Exceptionally preserved asphaltic coprolites expand the spatiotemporal range of a North American paleoecological proxy. Sci. Rep. 10:5069. doi: 10.1038/s41598-020-61996-y
Ochando, J., Carrión, J., Daura, J., Sanz, M., Araújo, A., and Costa, A. (2022). Coprolite palynology from Abrigo do Lagar Velho (Portugal) and a revision of Gravettian vegetation in the Iberian Peninsula. Rev. Palaeobot. Palynol. 299, 1–20. doi: 10.1016/j.revpalbo.2022.104609
Oeggl, K., Kofler, W., Schmidl, A., Dickson, J., Egarter-Vigl, E., and Gaber, O. (2007). The reconstruction of the last itinerary of “Ötzi”, the Neolithic Iceman, by pollen analyses from sequentially sampled gut extracts. Quat. Sci. Rev. 26, 853–861. doi: 10.1016/j.quascirev.2006.12.007
Piperno, D. R. (1988). Phytolith analysis: An archaeological and geological perspective. San Diego, CA: Academic Press.
Poinar, H., Fiedel, S., King, C. E., Devault, A. M., Bos, K., Kuch, M., et al. (2009). Comment on “DNA from pre-clovis human coprolites in Oregon, North America. Science 325:148. doi: 10.1126/science.1168182
Portillo, M., García-Suárez, A., and Matthews, W. (2020). Livestock faecal indicators for animal management, penning, foddering and dung use in early agricultural built environments in the Konya Plain, Central Anatolia. Archaeol. Anthropol. Sci. 12, 1–15. doi: 10.1007/s12520-019-00988-0
Prentice, I. C. (1988). “Records of vegetation in time and space: The principles of pollen analysis,” in Vegetation history, eds B. Huntley and T. I. Webb (Dordrecht: Kluwer Academic Publishers), 17–42. doi: 10.1007/978-94-009-3081-0_2
Prost, K., Birk, J. J., Lehndorff, E., Gerlach, R., and Amelung, W. (2017). Steroid biomarkers revisited; improved source identification of faecal remains in archaeological soil material. PLoS One 12:e0164882. doi: 10.1371/journal.pone.0164882
Rasmussen, M., Cummings, L., Gilbert, T., Bryant, V., Smith, C., Jenkins, D., et al. (2009). Response to comment by Goldberg et al. on “DNA from pre-clovis human coprolites in Oregon, North America. Science 325:148. doi: 10.1126/science.1167672
Rawlence, N. J., Wood, J. R., Bocherens, H., and Rogers, K. M. (2016). Dietary interpretations for extinct megafauna using coprolites, intestinal contents and stable isotopes: Complimentary or contradictory? Quat. Sci. Rev. 142, 173–178. doi: 10.1016/j.quascirev.2016.05.017
Reinhard, K. J., and Bryant, V. M. (1992). Coprolite analysis: A biological perspective on archaeology. Archaeol. Method Theory 4, 245–288.
Reinhard, K. J., and Hevly, R. H. (1991). Dietary and parasitological analysis of coprolites recovered from burial 5, Ventana Cave. Kiva 56, 319–325. doi: 10.1080/00231940.1991.11758174
Reinhard, K. J., Edwards, S., Damon, T. R., and Meier, D. K. (2006). Pollen concentration analysis of Ancestral Pueblo dietary variation. Palaeogeogr. Palaeoclimatol. Palaeoecol. 237, 92–109. doi: 10.1016/j.palaeo.2005.11.030
Reinhard, K., Camacho, M., Geyer, B., Hayek, S., Horn, C., Otterson, K., et al. (2019). Imaging coprolite taphonomy and preservation. Archaeol. Anthropol. Sci. 11, 6017–6035. doi: 10.1007/s12520-019-00946-w
Reitz, E. J., Newsom, L. A., Scudder, S. J., and Scarry, C. M. (2008). “Introduction to environmental archaeology,” in Case studies in environmental archaeology, eds E. J. Reitz, C. M. Scarry, and S. J. Scudder (New York, NY: Springer), 3–19. doi: 10.1007/978-0-387-71303-8_1
Rick, T. C., and Sandweiss, D. H. (2020). Archaeology, climate, and global change in the Age of Humans. Proc. Natl. Acad. Sci. U.S.A. 117, 8250–8253. doi: 10.1073/pnas.2003612117
Riley, T. (2008). Diet and seasonality in the lower pecos: Evaluating coprolite data sets with cluster analysis. J. Archaeol. Sci. 35, 2726–2741. doi: 10.1016/j.jas.2008.04.022
Riley, T. (2012). Assessing diet and seasonality in the Lower Pecos canyonlands: An evaluation of coprolite specimens as records of individual dietary decisions. J. Archaeol. Sci. 39, 145–162. doi: 10.1016/j.jas.2011.09.016
Rockman, M., and Hritz, C. (2020). Expanding use of archaeology in climate change response by changing its social environment. Proc. Natl. Acad. Sci. U.S.A. 117, 8295–8302. doi: 10.1073/pnas.1914213117
Romaniuk, A., Panciroli, E., Buckley, M., Pal Chowdhury, M., Willars, C., Herman, J. S., et al. (2020). Combined visual and biochemical analyses confirm depositor and diet for Neolithic coprolites from Skara Brae. Archaeol. Anthropol. Sci. 12, 1–15. doi: 10.1007/s12520-020-01225-9
Schiffer, M. B. (1983). Toward the identification of formation processes. Am. Antiq. 48, 675–706. doi: 10.2307/279771
Seppä, H. (2007). “Pollen analysis: Principles,” in Encyclopedia of quaternary science, eds S. A. Elias and C. J. Mock (Amsterdam: Elsevier), 2486–2497. doi: 10.1016/B0-44-452747-8/00175-7
Shillito, L. M. (2011). Simultaneous thin section and phytolith observations of finely stratified deposits from Neolithic Çatalhöyük, Turkey: Implications for paleoeconomy and early holocene paleoenvironment. J. Quat. Sci. 26, 576–588. doi: 10.1002/jqs.1470
Shillito, L., Blong, J. C., Jenkins, D. L., Stafford, T. W., Whelton, H. L., McDonough, K. N., et al. (2018). New research at paisley caves: Applying new integrated analytical approaches to understanding stratigraphy, taphonomy, and site formation processes. PaleoAmerica 4, 82–86. doi: 10.1080/20555563.2017.1396167
Shillito, L., Bull, I., Matthews, W., Almond, M., Williams, J., and Evershed, R. (2011). Biomolecular and micromorphological analysis of suspected faecal deposits at Neolithic Çatalhöyük, Turkey. J. Archaeol. Sci. 38, 1869–1877. doi: 10.1016/j.jas.2011.03.031
Shillito, L.-M., Blong, J. C., Green, E. J., and van Asperen, E. N. (2020a). The what, how and why of archaeological coprolite analysis. Earth Sci. Rev. 207, 1–21. doi: 10.1016/j.earscirev.2020.103196
Shillito, L.-M., Whelton, H. L., Blong, J. C., Jenkins, D. L., Connolly, T. J., and Bull, I. D. (2020b). Pre-Clovis occupation of the Americas identified by human faecal biomarkers in coprolites from Paisley Caves, Oregon. Sci. Adv. 6, 1–8. doi: 10.1126/sciadv.aba6404
Simpson, I. A., van Bergen, P. F., Perret, V., Elhmmali, M. M., Roberts, D. J., and Evershed, R. P. (1999). Lipid biomarkers of manuring practice in relict anthropogenic soils. Holocene 9, 223–229. doi: 10.1191/095968399666898333
Sistiaga, A., Mallol, C., Galván, B., and Summons, R. E. (2014). The neanderthal meal: A new perspective using faecal biomarkers. PLoS One 9:e101045. doi: 10.1371/journal.pone.0101045
Six, J., Schultz, P. A., Jastrow, J. D., and Merckx, R. (1999). Recycling of sodium polytungstate used in soil organic matter studies. Soil Biol. Biochem. 31, 1193–1196. doi: 10.1016/S0038-0717(99)00023-1
Sobolik, K. D. (2008). “Nutritional constraints and mobility patterns of hunter-gatherers in the Northern Chihuahuan desert,” in Case studies in environmental archaeology, eds E. J. Reitz, C. M. Scarry, and S. J. Scudder (New York, NY: Springer), 211–233. doi: 10.1007/978-0-387-71303-8_11
Sonderman, E. M., Dozier, C. A., and Smith, M. F. (2019). Analysis of a coprolite from Conejo Shelter, Texas: Potential ritualistic viperous snake consumption. J. Archaeol. Sci. Rep. 25, 85–93. doi: 10.1016/j.jasrep.2019.03.032
Sutton, M. Q. (1993). Midden and Coprolite derived subsistence evidence: An analysis of data from the La Quinta Site, Salton Basin, California. J. Ethnobiol. 13, 1–15.
Sutton, M. Q. (1998). Cluster analysis of paleofecal data sets: A test of late prehistoric settlement and subsistence patterns in the Northern Coachella Valley, California. Am. Antiq. 63, 86–107. doi: 10.2307/2694778
Tripp, M., Wiemann, J., Brocks, J., Mayer, P., Schwark, L., and Grice, K. (2022). Fossil biomarkers and biosignatures preserved in coprolites reveal carnivorous diets in the carboniferous Mazon Creek ecosystem. Biology 11:1289. doi: 10.3390/biology11091289
van Geel, B., Aptroot, A., Baittinger, C., Birks, H., Bull, I., Cross, H., et al. (2008). The ecological implications of a Yakutian mammoths last meal. Quat. Res. 69, 361–376. doi: 10.1016/j.yqres.2008.02.004
Velazquez, N. J., Burry, L. S., and Fugassa, M. H. (2017). A methodology for the determination of pollen sources in studies of Patagonian camelid coprolites. J. Archaeol. Sci. Rep. 11, 743–749. doi: 10.1016/j.jasrep.2017.01.007
Velázquez, N. J., Burry, L. S., Mancini, M. V., and Fugassa, M. H. (2020). Palynological analysis of the outer parts of camelid coprolites: Implications for vegetation reconstruction. Archaeol. Anthropol. Sci. 12:58. doi: 10.1007/s12520-019-00969-3
Wang, X., White, S., Balisi, M., Biewer, J., Sankey, J., Garber, D., et al. (2018). First bone-cracking dog coprolites provide new insight into bone consumption in Borophagus and their unique ecological niche. Elife 7:e34773. doi: 10.7554/eLife.34773
Warinner, C., Korzow Richter, K., and Collins, M. J. (2022). Paleoproteomics. Chem. Rev. 122, 13401–13446. doi: 10.1021/acs.chemrev.1c00703
Warnock, P. J., and Reinhard, K. J. (1992). Methods for extracting pollen and parasite eggs from latrine soils. J. Archaeol. Sci. 19, 261–264. doi: 10.1016/0305-4403(92)90015-U
Watson, P. J., and Yarnell, R. A. (1966). Archaeological and paleoethnobotanical investigations in salts cave, mammoth cave national park, Kentucky. Am. Antiq. 31, 842–849. doi: 10.2307/2694457
Wibowo, M. C., Yang, Z., Borry, M., Hübner, A., Huang, K. D., Tierney, B. T., et al. (2021). Reconstruction of ancient microbial genomes from the human gut. Nature 594, 234–239. doi: 10.1038/s41586-021-03532-0
Witt, K. E., Yarlagadda, K., Allen, J. M., Bader, A. C., Simon, M. L., Kuehn, S. R., et al. (2021). Integrative analysis of DNA, macroscopic remains and stable isotopes of dog coprolites to reconstruct community diet. Sci. Rep. 11:3113. doi: 10.1038/s41598-021-82362-6
Wood, J. R., and Wilmshurst, J. M. (2016). A protocol for subsampling late quaternary coprolites for multi-proxy analysis. Quat. Sci. Rev. 138, 1–5. doi: 10.1016/j.quascirev.2016.02.018
Zhang, Y., van Geel, B., Gosling, W. D., Sun, G., Qin, L., and Wu, X. (2020a). Typha as a wetland food resource: Evidence from the Tianluoshan site, Lower Yangtze region, China. Veg. Hist. Archaeobot. 29, 51–60. doi: 10.1007/s00334-019-00735-4
Zhang, Y., Zhang, D., Yang, Y., and Wu, X. (2020b). Pollen and lipid analysis of coprolites from Yuhuicun and Houtieying, China: Implications for human habitats and diets. J. Archaeol. Sci. Rep. 29:102135. doi: 10.1016/j.jasrep.2019.102135
Keywords: coprolite, multiproxy, macrofossil, pollen, phytolith, fecal biomarker
Citation: Blong JC, Whelton HL, van Asperen EN, Bull ID and Shillito L-M (2023) Sequential biomolecular, macrofossil, and microfossil extraction from coprolites for reconstructing past behavior and environments. Front. Ecol. Evol. 11:1131294. doi: 10.3389/fevo.2023.1131294
Received: 24 December 2022; Accepted: 11 April 2023;
Published: 27 April 2023.
Edited by:
Angelina Perrotti, University of Wisconsin-Madison, United StatesReviewed by:
Maciej Tomasz Krajcarz, The Polish Academy of Sciences, PolandRachel E. B. Reid, Virginia Tech, United States
Copyright © 2023 Blong, Whelton, van Asperen, Bull and Shillito. This is an open-access article distributed under the terms of the Creative Commons Attribution License (CC BY). The use, distribution or reproduction in other forums is permitted, provided the original author(s) and the copyright owner(s) are credited and that the original publication in this journal is cited, in accordance with accepted academic practice. No use, distribution or reproduction is permitted which does not comply with these terms.
*Correspondence: John C. Blong, am9obi5ibG9uZ0B3c3UuZWR1; Lisa-Marie Shillito, bGlzYS1tYXJpZS5zaGlsbGl0b0BuZXdjYXN0bGUuYWMudWs=