- 1Department of Biology, York University, Toronto, ON, Canada
- 2Department of Mathematics and Statistics, York University, Toronto, ON, Canada
Introduction: The diversity of freshwater fishes is threatened by multiple environmental stressors, including climate change, alterations in land use, and introduction of non-native species. However, the quantification of temporal biodiversity in freshwater communities is limited. Here, we asked: i) how has alpha (species richness), beta (changes in freshwater species composition), and gamma diversity (total species diversity in a landscape) changed over time for lakes over a 50 year period?; and ii) What are the climatic, land use, and lake morphological drivers associated with higher diversity?
Methods: We assembled a database of fish species occurrence from 20 lakes across subalpine and alpine regions in Alberta from 1970-2019, in addition to lake morphological, climatic, and land use characteristics of the watersheds.
Results: We observed an overall increase in alpha, beta, and gamma diversity from the 1970s to 2009s. However, all measures of diversity declined from 2010-2019. We found that more lakes and species assemblages were influenced by species gains, rather than species losses (with the exception of the last decade of sampling).
Discussion: Generally, we found that coolwater species were expanding and coldwater fishes were being lost throughout our study lakes. We highlight temporal heterogeneity in fish biodiversity responses to substantial environmental pressures in this region.
1 Introduction
Freshwater fishes play an important role in ecosystems by influencing levels of water clarity, nutrient loading, as well as the abundance of aquatic vegetation, invertebrates, and other fish species (Eilers et al., 2007; Mutethya and Yongo, 2021). Despite their economic, nutritional, and ecological importance, freshwater fishes are increasingly threatened by a multitude of natural and anthropogenic stressors (Islam and Berkes, 2016). Future climate scenarios predict an average loss of 20% of fish species from all river basins worldwide due to climate change alone (Manjarrés-Hernández et al., 2021). Climate-intensified extreme weather events (Rolls et al., 2017), higher water temperatures (O’Reilly et al., 2015), and reduced water quality (Vogt et al., 2018; Woolway et al., 2020), will fragment and degrade habitat quality and thermal habitat availability, leading to population declines and range contractions of freshwater fishes (Brie et al., 2016; Van Zuiden and Sharma, 2016). These climate-induced environmental stressors coupled with, land use alterations and species introductions(Dudgeon et al., 2006; Reid et al., 2019), may result in the extinction of an additional 53 to 86 freshwater fishes in North America alone by 2050 (Burkhead, 2012), highlighting the need for immediate action.
Alterations in community composition can provide early warnings of environmental stress and subsequent biodiversity loss (Johnson and Angeler, 2014). Quantifying the temporal response of freshwater fish communities in terms of alpha, beta, and gamma diversity can provide insights into how the health of freshwater fish communities are changing over time (Erős et al., 2020). Gamma diversity is the measure of regional diversity, describing the totality of species diversity across the study sites (Whittaker, 1960). Alpha diversity is defined as the species richness within a community (Whittaker, 1960). Beta-diversity is the degree of change in species composition and abundance between two or more communities (Whittaker, 1960; Whittaker, 1972) can be quantified spatially or temporally (Anderson et al., 2011), and calculated in a variety of ways (Legendre and De Cáceres, 2013). Changes in alpha diversity can either increase or decrease beta diversity. If changes in alpha diversity tend to homogenize different regions (e.g., by adding the same set of species or removing unique species), beta diversity will decline. If changes in alpha diversity tend to diversify different regions (e.g., by adding unique species or removing shared species), beta diversity will increase.
Measures of species richness, such as alpha and gamma diversity are insufficient to determine shifts in community composition (Dornelas et al., 2014). For instance, (Kortz and Magurran, 2019) assessed plant assemblages on a spatial scale and found increasing alpha-diversity coupled with decreasing beta-diversity. These results demonstrate that although species introductions can cause an initial increase in local species richness, the widespread introduction of these same species into several communities leads to increased spatial species similarity between localities (Finderup Nielsen et al., 2019). Temporal patterns of beta-diversity are relatively understudied in freshwater fish assemblages. This knowledge gap exists not only because of a paucity of long-term fish community data spanning decades (Magurran et al., 2019) but is compounded by the complex dynamics of species composition (Olden and Poff, 2003). Short-term temporal studies that evaluate communities immediately before and after an environmental disturbance (Richardson et al., 2018) would not be able to capture the changing community compositions because of the time it can take to fully observe complex community responses. These varying results highlight the context-dependent mechanism of species composition and the need for further studies of this phenomenon over longer intervals of time.
Our goal was to identify how biodiversity has changed in Albertan freshwater fish communities over the past five decades in response to previously documented drivers of homogenization: climate change (Magurran et al., 2015) and land use changes (Iacarella et al., 2018). We focused on 20 lakes surveyed over five decades from 1971 to 2019 located in central and southern Alberta, Canada. This alpine region is a good study system to elucidate the impact of anthropogenic activity and climate change on lake fish assemblages owing to its rising temperatures (Kienzle, 2018) and intensive land development (Komers and Stanojevic, 2013). We quantified changes in species composition and populations of functional groups, such as thermal and trophic guilds. Additionally, we identified temporal patterns in biotic homogenization by calculating both temporal gamma, alpha, and beta diversity metrics (Rahel, 2002; Olden and Rooney, 2006). More specifically, we asked: (1) How have gamma, alpha, and beta diversity changed over time? and (2) Which lake morphology, climate, and land use variables are associated with higher diversity?
Examining the potential effects of climate change and land use on Albertan lake fish assemblages over a period of five decades may contribute to filling in gaps in the literature regarding temporal beta diversity as a response to multiple environmental stressors, particularly in relatively understudied alpine systems. We predict that the fish communities in Alberta will become more similar over time with increased non-native species richness and decreased native species richness (Villéger et al., 2011; Moi et al., 2021; Sleezer et al., 2021). We expect higher species richness in larger lakes and in lower elevation regions with warmer temperatures, as these lakes are generally able to support more diversity (Macarthur, 1965; Guo et al., 2015). Finally, we predict that the key drivers of changes in the fish community will be Alberta’s accelerated warming and intensive land use (Magurran et al., 2015; Iacarella et al., 2018; Cazelles et al., 2019).
2 Methods
2.1 Study area
To investigate long term changes in lake fish communities in Alberta, we compared fish assemblages over 5 decades in 20 lakes across Alberta. These lakes sit within a geographic region spanning 49.9°N – 56.6°N, and 110.03°W – 118.6°W. Most of the lakes are found at latitudes north of 53.5°N, except for Lake Newell and Rattlesnake Lake which lie around the 50.0°N parallel (Figure 1). Similarly, while most of the lakes can be characterized as being within the boreal forest biogeographical region, the westernmost lakes, Musreau and Sturgeon are in the eastern slopes of the Rocky Mountains, while the more southerly lakes, Newell and Rattlesnake, are situated in parkland prairies (Alberta-Parks 2015). The surface area of these 20 lakes ranges from 1 km2 to 1186.5 km2 with a mean surface area of 117.6 km2. Mean depth ranges from 1.6 m to 17.8 m with an average mean depth of 10.4 m. As glacial lakes situated near the foothills of the Rocky Mountains in Alberta, the elevation of our study lakes range from 529 m – 868 m above sea level with mean elevation of 636.3m.
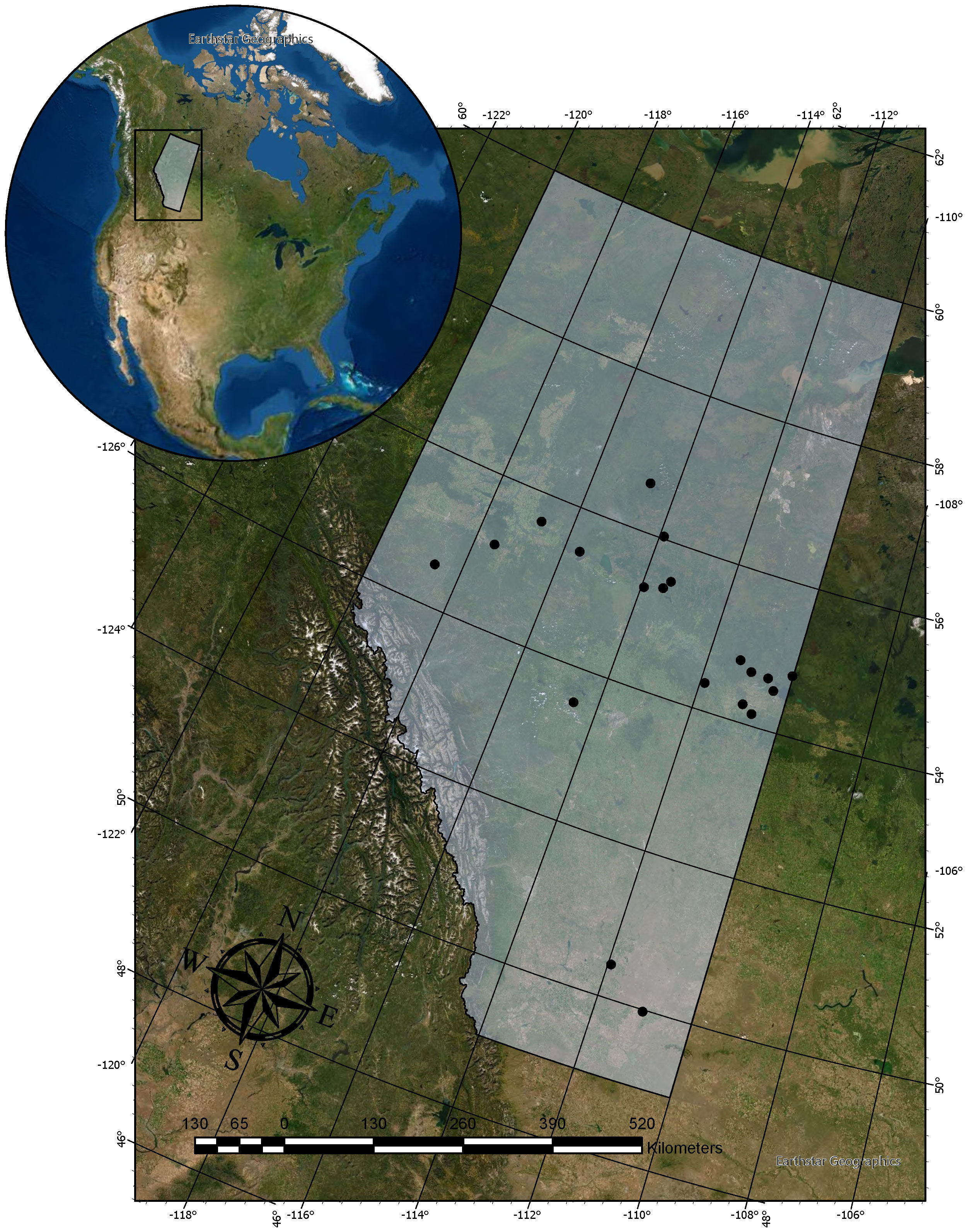
Figure 1 Location of the 20 study lakes found in Alberta, Canada with fish community composition data extending from 1970-2019.
The annual average temperature has increased throughout the province at significant rates from 1950-2010 (Kienzle, 2018). Since the 1950s, the average annual temperatures have increased by a total of 2-4˚C and 1-2˚C in the north and south, respectively (Kienzle, 2018). Northern regions of Alberta appear to be warming more rapidly than the rest of the country, as over the same time period, Canada’s annual mean temperature has increased by 1.5˚C (Vincent et al., 2012). As of 2018, the human footprint is approximately one-third of Alberta’s total land cover (Alberta-Biodiversity-Monitoring-Institute, 2020). The industries that contribute most to the footprint are agriculture, forestry, and energy, including the vast oilsands industry (Alberta-Biodiversity-Monitoring-Institute, 2020). In a 16-year period from 1992 to 2008, Alberta’s boreal forest experienced anthropogenic disturbances and forest fires at a rate of 3156 km2 a year (Komers and Stanojevic, 2013). At current rates, by 2028, all of Alberta’s boreal forest would be within 500 m of a human disturbance (Komers and Stanojevic, 2013). Alberta’s accelerated temperature increases, and intensive land use make the province an ideal study area to assess the effects of multiple stressors on community composition.
2.2 Data acquisition
As we were interested in long-term changes in biological and functional diversity, our goal was to acquire as many lakes with fish community composition going back five decades with resampling approximately every decade. Thus, we assembled a georeferenced time series dataset of 20 lakes in Alberta with fish community data collected between 1971 and 2019. The fish communities comprised fishes from the warmwater, coolwater, and coldwater thermal guilds (Magnuson et al., 1979). Warmwater fishes are defined as those with a final temperature preferendum greater than 25°C, while coolwater fishes are defined as those with a final temperature preferendum between 19 and 25°C, and coldwater fishes have a final temperature preferendum below 19°C (Coker et al., 2001; Supplementary Table 3). Our dataset included 16 coldwater species, 11 coolwater species, and 2 warmwater species. To quantify how changes in diversity in each lake have affected functional traits and groupings, our focal fish species were also classified into aggregated trophic guilds of preyfish, intermediate predators, and predators based on feeding guilds according to FishBase (Froese and Pauly, 2023). More specifically, a species was classified as preyfish if planktivorous, detritivorous or invertivorous and served as prey to larger adult fishes. Intermediate predators were omnivores, feeding on plankton, insects, mollusks, and other adult fish of smaller size, but were also prey to larger adult fishes. Predators were invertivorous or carnivorous, feeding on other fishes, but were themselves never prey to other fishes as adults. Our dataset included 15 preyfish, 9 intermediate predators, and 5 predators. All of our species were native or naturalized.
Fish community data for individual lakes in Alberta were acquired from the Fish and Wildlife Internet Mapping Tool (FWIMT), a public map-based repository of fisheries and wildlife inventory data that allows users to extract spatially referenced data made accessible by the Government of Alberta’s Ministry of Alberta Environment and Parks (AEP). Within this repository, each lake’s datasets were checked for the type of species data recorded (i.e., presence/absence, species abundance, stocking records), and extent of temporal range. We retained only those lakes with presence/absence datasets to limit biases from unequal sampling effort between lakes and records of repeated sampling that occurred for multiple years. However, we must note that there may be limitations in this dataset as a species absence may not always indicate a real absence of a fish, but may simply be a result of insufficient sampling effort. This left us with an aggregated dataset of 20 lakes with presence/absence data for lake fish communities over the past 50 years, from the 1970s to the 2010s. Each of our 20 study lakes had been sampled at least once per decade, but not evenly (i.e., not every 10 years), for a total of 100 data points. For example, Bourque Lake was first sampled in 1979, resampled in 1989, then sampled again in 2000, 2006, and 2016, with the differences between the years being 10, 11, 6, and 10 respectively. The lake that had been sampled earliest was Wabamun Lake in 1971, while the lake last sampled was Musreau Lake in 2019. Each of our 20 study lakes were then cross-referenced by name and coordinates, using Google Maps, the Watershed Dictionary (https://a100.gov.bc.ca/pub/fidq/viewWatershedDictionary.do), and the Canadian Geographical Names Database (CGNDB) (https://www.nrcan.gc.ca/earth-sciences/geography/querying-canadian-geographical-names-database/canadian-geographical-names-database/19870) to clarify the geographical coordinates, while also ensuring that each lake was the same one sampled over time. On average, annual air temperatures for our study lakes statistically significantly increased by 0.88°C over 36.7 years, or 0.02°C/year (τ = 0.16, p = 0.015). The majority of regions warmed over the course of this study (16 sites). Air temperatures warmed fastest around Bourque Lake at 3.29°C over 37 years or 0.08°C/year.
We acquired 47 environmental variables encompassing climate, lake geomorphology, and changes in land cover. Climatic data consisted of monthly averages of precipitation and air temperatures for January to December, as well as derived seasonal and annual averages. Monthly climatic data were acquired from ClimateNA, an MS Windows application containing PRISM (Daly et al., 2008). We extracted gridded monthly climate normal data from 1971-2000 for each lake’s coordinate and elevation data at a resolution of 800m x 800m. Lake geomorphological characteristics were acquired from georeferenced lake records in HydroLAKES, and included latitude, longitude, lake area (km2), shoreline length and shape, volume (mcm), average depth (m), average discharge (m3/s), elevation above sea level (m), and watershed area (km2) (Messager et al. 2016). Changes in land cover were quantified as GIS derived measures of the average urban, cropland, pasture, forest, shrubland, and sparse land covers present in each lake’s watershed area in hectares (ha) during each decade of sampling. Land cover data were acquired from Historic Land Dynamics Assessment(HILDA+) (Winkler et al., 2020).
Fish community data consisted of species occurrence (presence/absence) data for up to 28 species and one hybrid (Coregonus artedi x C. clupeaformis), from 10 families. The fish assemblages were dominated by fish species from the Cyprinidae and Salmonidae families, which collectively accounted for 58% of the species in our dataset, or 31% (9 species) and 27% (8 species) respectively. All other fish families, including the Esocidae, Lotidae, Percopsidae, Cottidae, and Hiodontidae were represented by one species each (Supplementary Table 3). Several notable species of important commercial status were present in our data, including northern pike (Esox lucius), walleye (Sander vitreus), Cisco (Coregonus artedi), yellow perch (Perca flavescens), lake whitefish (Coregonus clupeaformis), rainbow (Oncorhynchus mykiss), and bull trout (Salvelinus confluentus) (Table 1).
2.3 Data analysis
To examine how lake fish communities in Alberta have changed in our study lakes between the 1970s and 2010s, we first calculated gamma diversity in each decade (Figure 2). Gamma diversity was calculated as the sum of unique species across all of our study lakes during each decade. All analyses were performed in R (R Core Team, 2021).
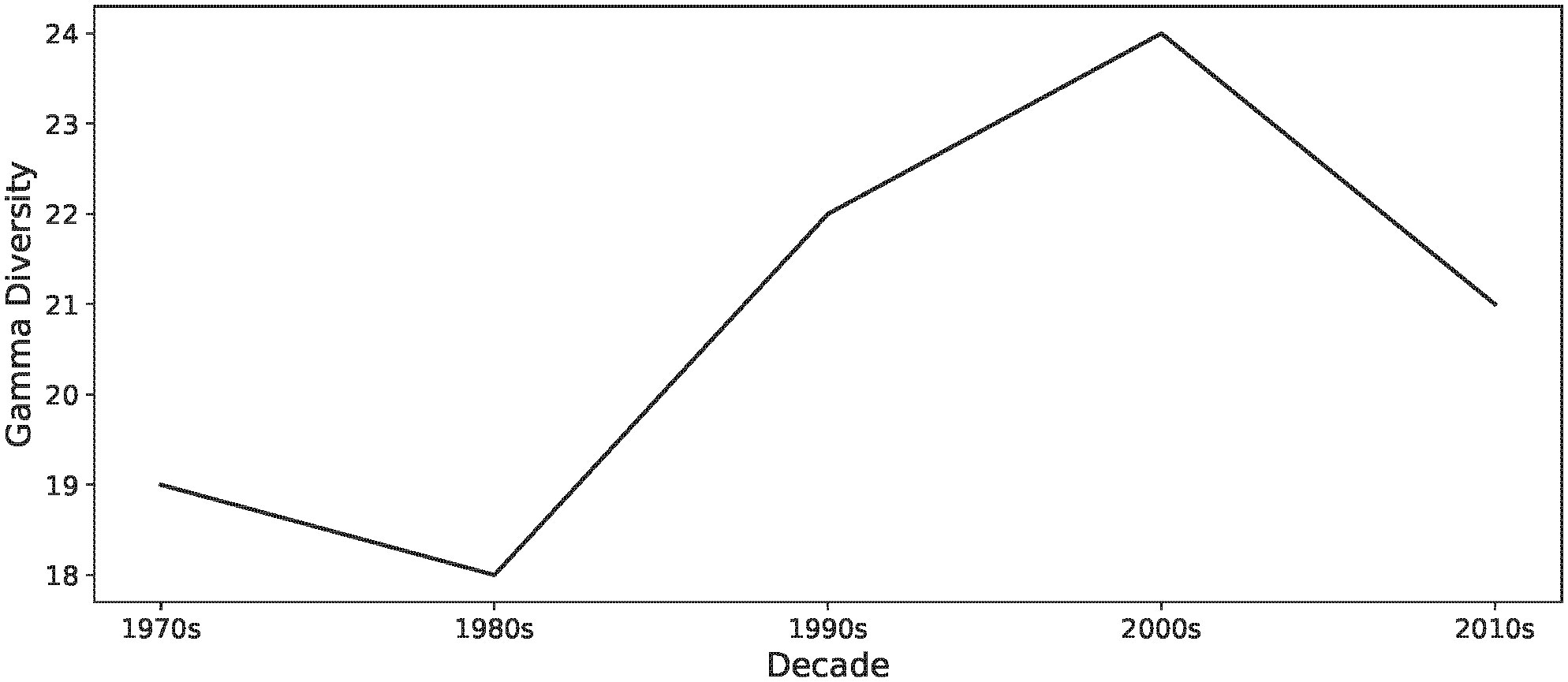
Figure 2 Patterns in gamma diversity in our study lakes between 1970 and 2019. Initial regional diversity consisted of 19 species, but declined to 18 species over the next decade, before increasing to 24 species by the 2000s, and declining again during the 2010s to 21 species.
2.3.1 How has α-diversity changed over time?
We calculated α-diversity as the sum of unique species occurrences (total species richness) in each lake at time of sampling. Next, we examined how α-diversity changed in each lake over time between the 1970 and 2010 decades. Additionally, we calculated average α-diversity values per lake from the 1970s to the 2010s (Supplementary Table 2). To understand how environmental factors are shaping patterns in α-diversity in lake fish communities in Alberta, we calculated Spearman correlations to quantify the significance and strength of the relationships between total species richness and climate, geomorphology, and land cover. Strongly correlated variables based on variance inflation factors greater than 5 were removed from subsequent correlational analyses. Spearman correlations were calculated and plotted using corrplot (Wei and Viliam, 2021).
2.3.2 How has beta diversity changed over time?
We quantified changes in community composition (β-diversity) in each of our lakes over the past five decades by calculating temporal β-diversity indices (TBI). TBI was calculated as a comparison between two decades. Here, we present the comparison between the first and last decades. TBI measures the change in species assemblages between the same site at different time points (Legendre, 2019). TBI values were calculated as dissimilarity (D) values using the Jaccard index and ranged from 0 – 1, with values approaching 1 indicating that the two communities are completely dissimilar (Jaccard, 1908; Legendre, 2019; Magurran et al., 2019). While α-diversity can indicate broad changes in biodiversity such as the pool of species diversity, β-diversity measures calculated as TBI values also provide insight into the losses and gains of species that can indicate changes to community composition even when the number of species present remains the same (Magurran et al., 2019). We also identified which fish species were lost or gained by each lake (Supplementary Table 1), as well as patterns in the losses and gains of species in terms of thermal and trophic guilds. Temporal beta diversity indices were calculated using the adespatial package (Dray et al., 2022).
To understand how environmental factors may be shaping β-Diversity patterns in lake fish communities in Alberta, we conducted a redundancy analysis (RDA) to summarize the variation in the occurrence of fish species explained by time, climatic, lake geomorphological, and land cover environmental factors. We retained the environmental characteristics with variance inflation factor values of less than 5 to reduce multicollinearity between environmental variables. We performed a second redundancy analysis to examine the influence of the environmental factors on average total species richness, net gain, and net loss of species between the 1970s and 2010s amongst fish communities in our set of study lakes. Lastly, we performed an ANOVA to identify the significant variables influencing β-Diversity over the last 50 years. The Redundancy Analysis were run in the vegan package in R (Oksanen et al., 2008).
3 Results
Gamma diversity increased within our set of Albertan lakes until the end of the 2000s and then declined subsequently. In the 1970s, there were 19 species, increasing to 24 species by the 2000s. By the end of the 2010s, there were 21 species in our study lakes (Figure 2).
3.1 How has alpha diversity changed over time?
In general, α-diversity within individual lakes in Alberta remained low across the entire 50-year sampling period between 1971 and 2019, averaging 7.5 species per lake. Each lake rarely hosted more than 10-13 species during any decade out of a total of 30 potential species. The lakes with the highest average species richness over the 50-year period were Wolf and Lesser Slave Lake, with 9.8 species per lake (Supplementary Figure 1).
Despite the low biodiversity, α-diversity in many individual lakes also fluctuated between lakes and across decades. Generally, α-diversity increased from the 1970s to 2000s, followed by a decline in the 2010s. More specifically, during the 1970s, species richness averaged 6.75 across all lakes, ranging from 4 species (Winagami Lake) to 16 species (Primrose Lake) per lake (Figure 3). However, during the next decade in the 1980s, even though species richness averaged 6.7 species, Primrose Lake lost 9 species, thus α-diversity ranged from 4 species (Winagami Lake) to 10 species (Rock Island Lake). During the 1990s, changes in α-diversity within individual lakes continued to fluctuate, with species richness averaging 7.6 and ranging from a minimum of 5 species (Winagami & Bourque Lakes), to a maximum of 11 species at Lake Newell. α-diversity peaked in the 2000s, at an average of 8.6 fishes, with 7 lakes hosting 10 or more species and at a maximum of 13 species per lake (Wolf and Lesser Slave Lakes). However, α-diversity decreased for some lakes, such as Muriel Lake which lost 6 species, reaching an α-diversity value of 3, despite previously experiencing an increase in α-diversity of 9 species during the 1990s. By the 2010s, α-diversity in many lakes decreased, such that only five lakes still had more than ten fish species. The fish communities in this set of Albertan lakes were composed almost exclusively of coldwater (n=16) and coolwater fishes (n=11). However, there have been periodic occurrences of two warmwater prey fishes, Pimephales promelas (fathead minnow) and Chrosomus eos (northern redbelly dace) over the 50-year study period, although these warmwater fishes did not successfully establish. There did not appear to be consistent patterns of diversity changes by functional groups (Table 2). These fishes first appeared in the 1980s, but were no longer present after the subsequent two decades.
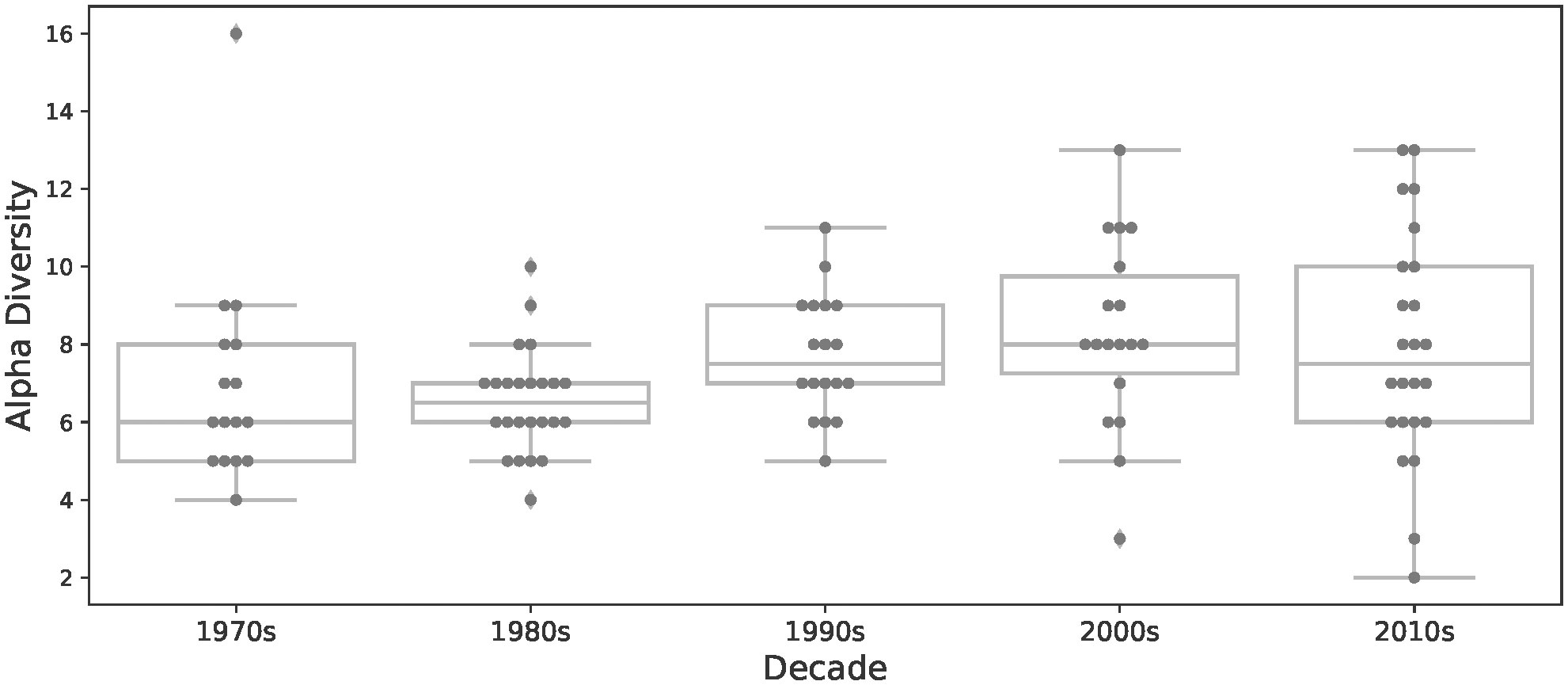
Figure 3 Average α-diversity per decade in our study lakes in Alberta. The data points represent α-diversity for each lake for the specified decade.
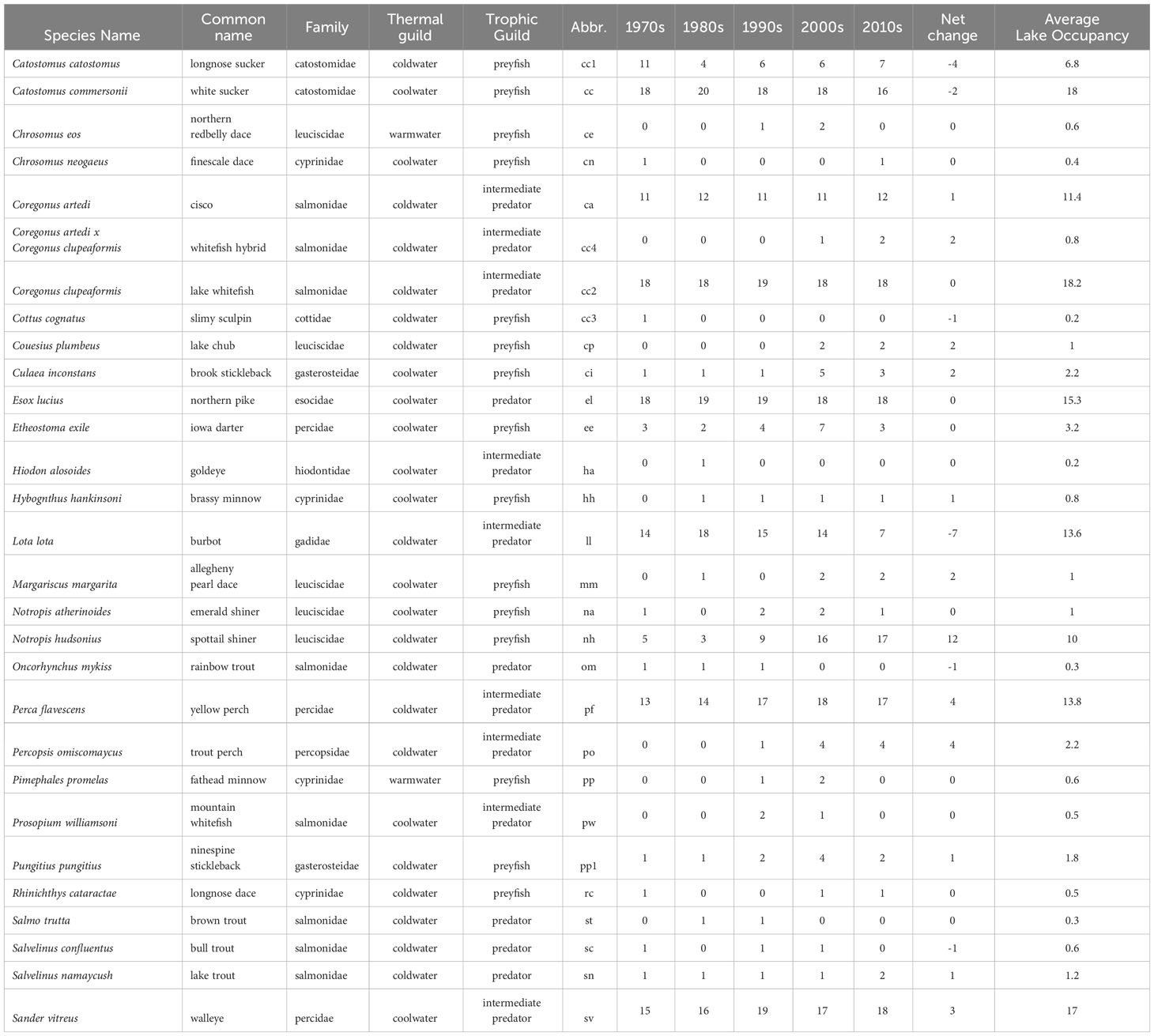
Table 2 Alberta freshwater fish species list with common name, family, thermal (warmwater, coolwater, coldwater) and trophic guilds (preyfish, intermediate predator, predator), and habitat (pelagic, benthopelagic, benthic) and changes in lake occupancy for each of our study fish species and five decades of sampling.
Lower elevation and larger watershed areas were consistently identified as important factors explaining higher total species richness in our study lakes. However, other environmental characteristics were statistically significant depending upon the species richness metric used. For example, when considering total species richness per lake, we found that species richness was higher in deeper lakes (r = 0.23, p < 0.05) with larger watershed areas (r = 0.43, p < 0.05) at lower elevations (r = -0.26, p < 0.05), and greater urban land cover (r = 0.23, p < 0.05) (Figure 4). When considering average total species richness per lake over time, only watershed area (r = 0.74, p < 0.05) was positively correlated with total species richness, whereas elevation (r = -0.45, p < 0.05) was negatively correlated with total species richness (Figure 4). When considering total species richness during the 1970s, the initial decade of sampling, total species richness was positively correlated with watershed area (r = 0.52, p < 0.05), and negatively correlated with elevation (r = -0.61, p < 0.05) and average air temperature (r = -0.57, p < 0.05) (Figure 4). However, during the 2000s, the decade of peak average total species richness, only a larger watershed area was correlated with higher species richness (r = 0.57, p < 0.05) (Figure 4). In the 2010s, the final decade of sampling, the only significant environmental variables with total species richness were watershed area (r = 0.69, p < 0.05), and average air temperatures (r = -0.56, p < 0.05; Figure 4). The lakes with warmer temperatures tended to have lower total species richness, with most lakes with higher species richness in the 0 – 4°C range.
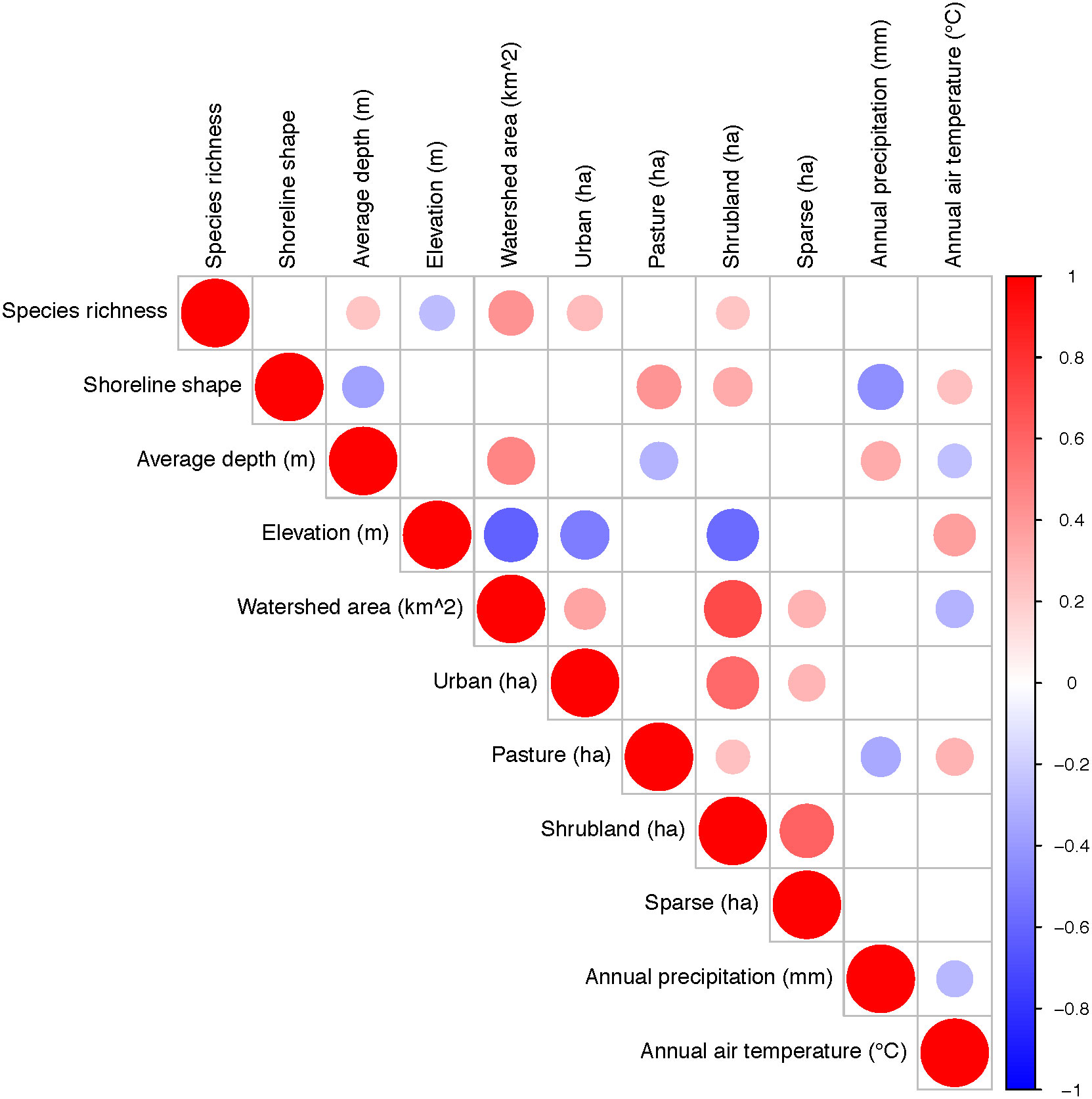
Figure 4 Spearman correlation coefficients between species richness and lake morphological (shoreline length and shape, average depth (m), elevation above sea level (m), and watershed area (km2)), climatic (annual precipitation and air temperature), and land use (urban, pasture, shrubland, sparse) characteristics. The plot only shows significant correlations, that is empty spaces had non-significant correlations (p > 0.05).
3.2 How has beta diversity changed over time?
Between 1970 and 2019, mean temporal beta diversity steadily increased from 0.30 to 0.44 (Figure 5A). Generally, more lakes gained more fish species than lost fish species (Figure 5B). However, in the last decade, there were fewer species gained and more species lost. Over the entire time period, most lakes gained more species overall than lost more species, averaging a net gain of 0.95 species (Figure 5B; Table 3). Thirteen lakes experienced net gains ranging from 1 - 6 species, averaging 2.5 species gained. Five lakes experienced net losses, ranging from 2 to 9 species, or 3.6 species lost. However, this figure is skewed by the 9 species lost by Primrose Lake; otherwise, these five lakes lost 2 - 3 species only, or an average of 1.8 species lost. Overall, each lake experienced different changes in terms of gains or losses in species. Furthermore, fish community composition in our study lakes also changed over time (Table 3).
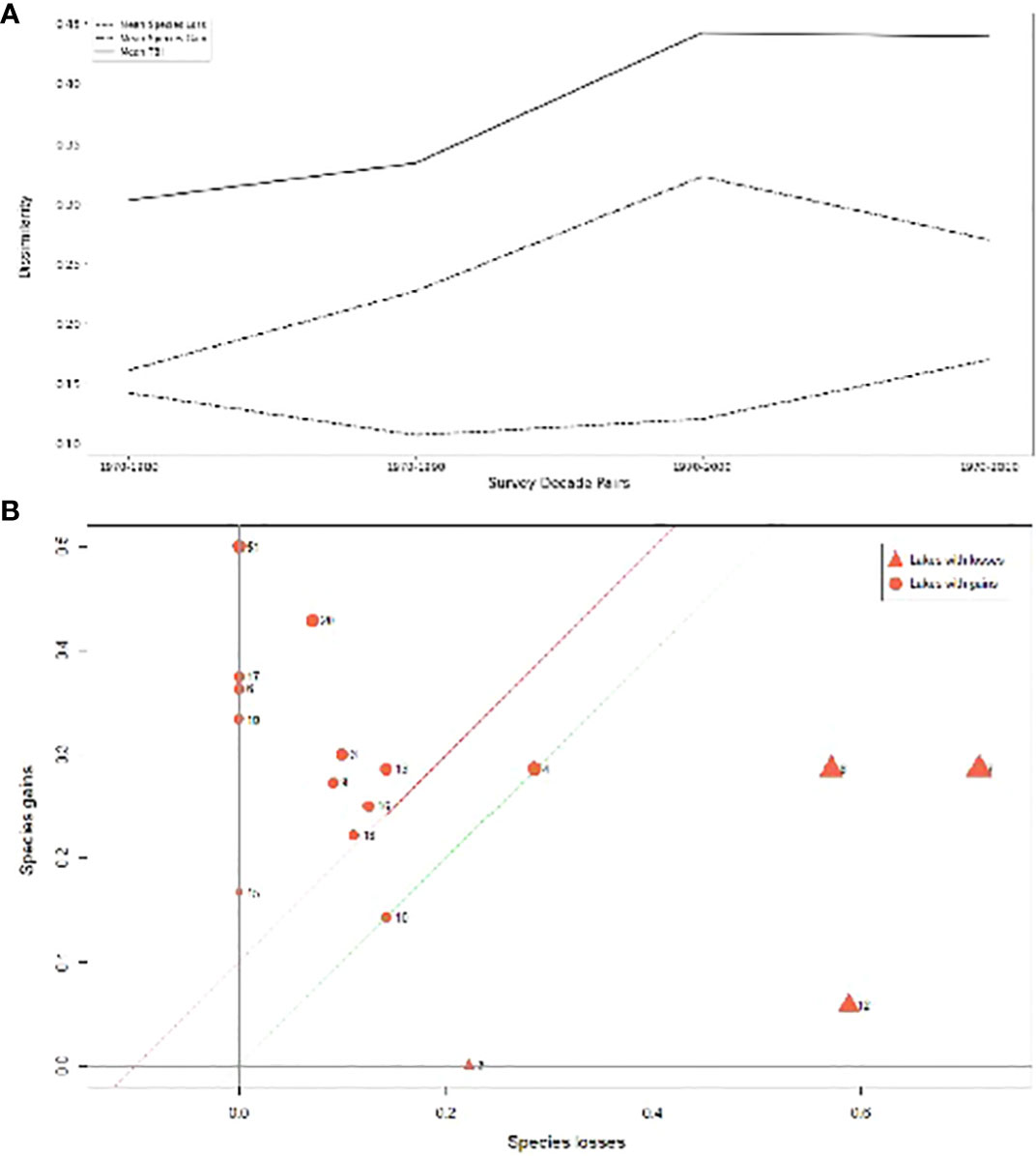
Figure 5 (A) Changes in dissimilarity of fish community composition and its gain and loss components between the 1970s and 2010s; (B) Loss-gain plot summarizing fish species losses and gains from fish community composition for 20 lakes sampled between 1970-2019. The green line indicates the line where species gains equal losses. The position of the red line above the green line indicates that on average, there were more species gains than species losses in our study lakes.
Species composition was associated with elevation, watershed area, average depth, presence of shrubland, and urban land covers, as well as shoreline shape. The redundancy analysis explained 23% of the variation (Figure 6A; R2 = 0.31; R2adj = 0.23). For example, we found that cisco (Coregonus artedi), lake whitefish (Coregonus clupeaformis), northern pike (Esox lucius), and walleye (Sander vitreus) were more likely to occur in lakes experiencing increasing urban land cover over the past 50 years. Burbot (Lota lota) and longnose sucker (Catostomus catostomus), were more likely to occur in deeper lakes with larger watersheds and more shrubland cover. Finally, brassy minnow (Hybognathus hankinsoni), and allegheny pearl dace (Margariscus margarita) were more likely to occur in lakes at higher elevations, or higher levels of annual precipitation and temperature. Additionally, species that occurred in lakes with more pasture and sparse land covers and or lakes at higher elevations or higher levels of annual precipitation and temperature did not generally co-occur with species in lakes with more complex shorelines and a higher quantity of urban land cover.
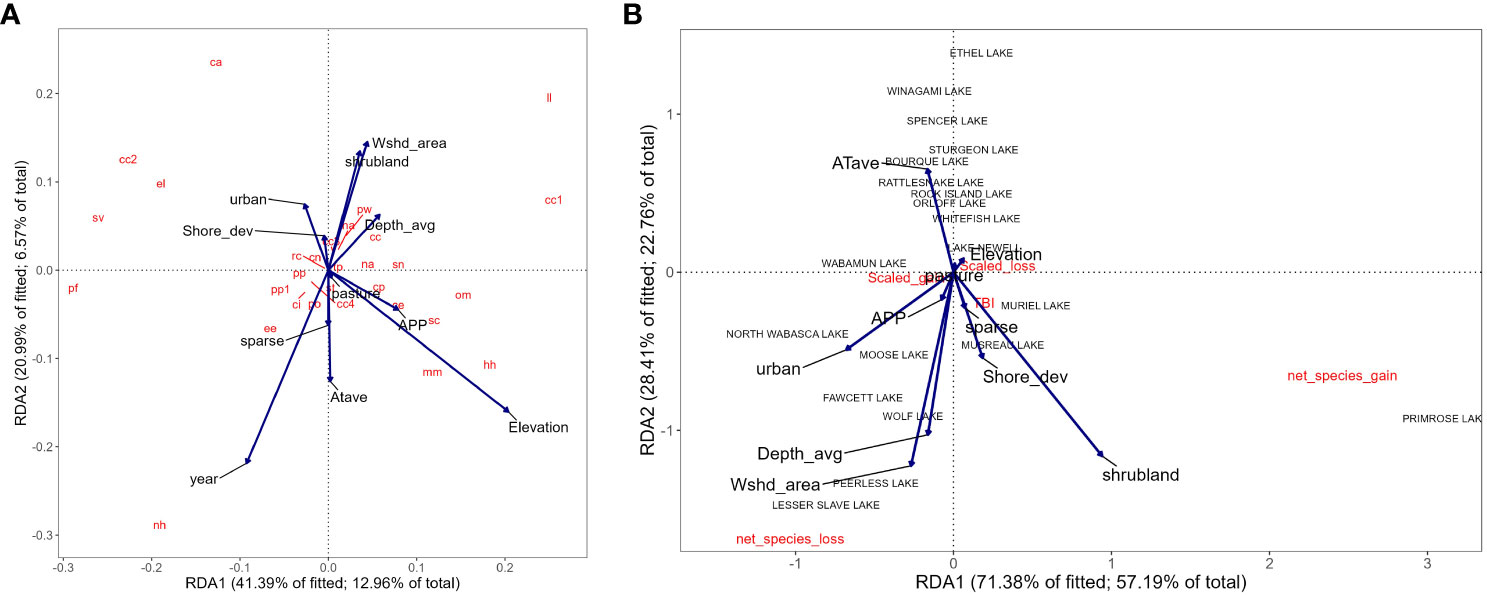
Figure 6 Redundancy analysis of fish community and lake morphological, climatic, and land use characteristics for: (A) species occurrence, and (B) beta diversity metrics. Only the first two RDA axes are shown explaining 23% of the variation for fish species occurrence and 58% of the variation in beta diversity metrics. Abbreviations are used for fish species and the codes are provided in Table 2.
To quantify beta diversity, we calculated net and scaled losses and gains and related them to environmental gradients in our model. The redundancy analysis explained 58% of the variation (Figure 6B; R2adj = 0.58). Lakes with more net losses in species were associated with larger watersheds, and more urban land cover as well as higher precipitation. In contrast, lakes that experienced a net gain in species were associated with more shrubland land covers or had more complex lake shorelines as well as more sparse land cover to a lesser extent. In general, species assemblages appear to have been more strongly influenced by gains in species diversity, rather than species losses. The exceptions to this were Musreau Muriel and Primrose Lakes, where between the 1970s and 2010s, they not only experienced a net gain in species rather than loss, but incoming species also replaced prior assemblages.
4 Discussion
Temporal patterns of biodiversity are relatively understudied, particularly in freshwater fish communities. The paucity of long-term community data spanning decades (Magurran et al., 2019), complex dynamics of biotic homogenization and differentiation (Olden and Poff, 2003), differential responses of environmental stressors on varying communities (Iacarella et al., 2018; Lindholm et al., 2020), and the interacting effects of multiple environmental stressors over space and time (Murdoch et al., 2020a) all contribute to lack of clear evidence of temporal betadiversity across studies. Generally, environmental stressors are expected to increase temporal beta diversity (McGill et al., 2015). Notably, we observed an overall increase in gamma, alpha, and beta diversity from the 1970s-2009, followed by a decrease in all measures of diversity in the 2010s. We highlighted the role of temporal heterogeneity in diversity metrics in our study region. The fish communities in our set of lakes are clearly responding to environmental stressors, including changes in climate and land use. Only time will tell if fish biodiversity within these lakes will continue to decline in response to environmental degradation or rebound back to the highest recorded biodiversity levels of the 2000-2009 decade.
4.1 How has alpha diversity changed over time?
The richness of fish species was relatively low in our set of study lakes found at relatively higher elevations in the province of Alberta, Canada. On average, lakes comprised less than 8 fish species. For both gamma and alpha diversity, we found that between the 1970s and the 2000s, species diversity increased in our alpine study lakes. However, after 2010, species diversity decreased. Overall, we found that many of these lakes gained new fish species until 2010, after which these lakes generally began to lose fish diversity. Notably, only five lakes had more than ten fish species by the end of the sampling period. Even the lake with the highest total species richness at any point during the 50-year sampling period, Primrose Lake, which was found to have 16 species during the 1970s, averaged 8.2 species over the entire temporal range, as by the 1980s, it had only 7 species, and reached a minimum of 5 species by the 2010s. In contrast, the lake with the fewest species at any point during the 50-year sampling period was Muriel Lake during the 2010s, with 2 species, yet it averaged 4.8 species over the entire temporal range.
Despite the low richness of fish species, we observed expected patterns of association between species richness and environmental variables. For example, we found that species richness was higher in deeper lakes with larger watershed areas. The observation that species diversity is higher in larger areas has long been described across ecological studies (Arrhenius, 1921; Williams, 1943; Macarthur, 1965), and lakes are no exception (Barbour and Brown, 1974; Brucet et al., 2013). Larger and deeper lakes tend to have more complex habitats and greater environmental stability than smaller and shallower lakes to host higher species diversity (Irz et al., 2004; Brucet et al., 2013). Further, we found that there were fewer fish species in lakes found at higher elevations. Many studies have shown that higher stress abiotic environments at higher elevations often limits growth, survival, fecundity, and species ranges (Louthan et al., 2015), although climatic warming could increase the invasion risk of alpine systems (Holzapfel and Vinebrooke, 2005; Rahel and Olden, 2008; Pauchard et al., 2016). Finally, there was a weak positive correlation between higher species richness in lakes and urbanized regions. Urbanization has a complex relationship with species diversity across taxa (Luck and Smallbone, 2010). Urbanization may be associated with a higher introduction of species that are desirable to humans. For example, we found more sport and commercially important fishes in urbanized landscapes in Alberta, such as cisco and lake whitefish. Higher urbanization may be associated with a higher influx of nutrients and primary production which have been associated with higher fish species diversity. For example, in a study from 1632 lakes in 11 European countries, primary productivity was the most important determinant of fish density (Brucet et al., 2013). However, as can often occur in fish community composition studies, our redundancy analysis explained only a small fraction of the variation in species occurrence (Figure 6A; R2 = 0.31; R2adj = 0.23) as associated with our environmental variables. In our RDA, we considered elevation, watershed area, average depth, presence of shrubland, and urban land cover. This suggests that factors not considered within our study may be influencing species distributions including other types of land cover (i.e. cropland, pasture, forest etc.), ecological interactions (Soukup et al., 2022), and or habitat structures and measures of habitat complexity (Smokorowski and Pratt, 2007; Wellborn et al., 1996). Furthermore, biases associated with sampling, including the relative ease of catching larger fishes compared to smaller prey, the difficulty of sampling fishes that are found in more complex vegetation, and species that are highly motile, can reduce explained variation in multivariate analyses (Sharma and Jackson, 2007).
4.2 How has beta diversity changed over time?
Many environmental stressors associated with driving the extinction or extirpation of many climate-sensitive freshwater species have intensified over the past 50 years in our study lakes, including non-native species introductions (Eilers et al., 2007), recent increases in urban land cover and their associated water quality impacts, as well as climate pressures from increased annual precipitation and temperature (Reid et al., 2019). However, our study lakes in Alberta may be experiencing an increase in biodiversity. Between 1971 and 2019, mean temporal beta diversity increased from 0.30 to 0.44, with the strongest changes in species assemblages taking place during the 1990 – 2000s, reflecting similar changes in alpha diversity in the same decades. Over the entire time period, most lakes gained more species overall than lost more species. Only two lakes, Lakes Newell, and Orloff lake experienced no net changes in species richness, although they also experienced substitutions by other species. For example, Lake Newell hosted a population of white sucker (Catostomus commersonii) from the 1970s until 2017 and gained a population of Walleye (Sander vitreus) after 1988, and a population of spottail shiner (Notropis hudsonius) in 1997. A population of longose sucker (Catostomus catostomus) was also present in 1974, but not in 1988, present again in 1997, but no longer sampled afterwards.
Fish community compositions in our study lakes have changed over time. The five most common species across the 50-year sampling period were lake whitefish (Coregonus clupeaformis), white sucker, northern pike (Esox lucius), and yellow perch (Perca flavescens). The most common fishes were coolwater fishes, with the exception of lake whitefish, a coldwater species. In contrast, the least common species were the goldeye (Hiodon alosoides), which was found only once in 1986 in Lesser Slave Lake; slimy sculpin (Cottus cognatus), which was found only once in Primrose Lake in 1978; brown trout (Salmo trutta) which was found only twice in Lake Newell in 1988 and 1997; and finescale dace (Chrosomus neogaeus) which was found only twice; in Peerless Lake in 2012 and Wolf lake in 2013. Only two species exhibited rapid and sudden changes in lake occupancy over our 50-year sampling period: spottail shiner and burbot (Lota lota). Spottail shiners are coolwater minnow fish that originally occupied only 5 lakes in Alberta, but eventually expanded into 16 lakes by the 2000s, before being lost from 4 lakes, with a net expansion of 7 lakes. Burbot are a native coldwater and deep-water species that originally occupied 14 lakes and expanded into 18 lakes by the 1980s. However, by the 2010s, burbot was found only in 7 lakes.
Overall, in our study of this set of Albertan lakes, we found that over the entire time period, most lakes gained more species overall than lost species, averaging a net gain of one fish species per lake. Although most lakes lost one or two fish species, many also gained at least two or three more. Although changes to species assemblages were caused by a wide variety of species, only a few species expanded into or were lost from multiple lakes between the 1970s and 2010s. The species that were lost from multiple lakes were mostly coldwater fishes, such as burbot and longnose sucker. Four other species were also lost from one or two lakes, including bull and rainbow trout, slimy sculpin, and white sucker, many of which were already found in few lakes. Coldwater fishes typically require cold, well oxygenated waters to thrive (Scott and Crossman, 1973; Magnuson et al., 1979). In response to water temperature warming, coldwater fishes have been increasingly lost from lakes where water temperatures have begun to exceed the thermal tolerances of coldwater fishes (Sharma et al., 2011; Van Zuiden and Sharma, 2016; Wu et al., 2022). Conversely, coolwater fishes were expanding into our study lakes over the 50-year period. This included important sport and commercial fisheries, such as walleye and yellow perch, but also important coolwater forage fishes, such as spottail shiner. In high northern lakes and rivers, other studies have detected the expansion of coolwater fishes (Lehtonen, 1996; Campana et al., 2020; Murdoch et al. 2020b). For example, in Alaskan streams, climate warming increased fish diversity and abundance, such that coolwater species increased their abundance, productivity, and ranges, whereas coldwater fishes declined (Murdoch et al. 2020b).
5 Conclusions
Interestingly, we found consistent patterns of increasing biodiversity across the study lakes with increasing gamma, alpha, and beta diversity indices in response to changes in climatic stressors and anthropogenic activities from 1970-2009. However, gamma, alpha, and beta diversity began to decline after 2010. The combination of the expansion of coolwater fishes and reduction in coldwater fishes, dissimilar fish assemblages were able to establish across this set of Alberta lakes. A similar case was noticed with fish communities in California, where fish assemblages became progressively dissimilar (Marchetti et al., 2001). The increase in beta diversity may or may not be temporary with continued pressures from accelerated climate warming and intense land use changes, which differentiate the fish assemblages among different sites (Socolar et al., 2016). This highlights the scale dependency of the processes affecting species composition scale (Marchetti et al., 2001).
Much work remains to understand the drivers, mechanisms, and patterns in temporal biodiversity. First and foremost, consistent and systematic long-term ecological monitoring programs, particularly for biotic variables, including species composition, abundance, and life history traits are required (Lindenmayer et al., 2022). Large knowledge gaps remain in quantifying how and why communities are changing over time, the temporal scale at which communities respond to environmental stressors, how environmental stressors affect lakes differently based on the composition of generalists and specialists, non-indigenous species, or varying life history traits, and at which scales biotic data should be gathered to answer these questions. Although there are very few case studies quantifying temporal biodiversity patterns (Winegardner et al., 2017; Zeni et al., 2020; Lindholm et al., 2021), there are not yet clear results on whether biodiversity is increasing or decreasing. For example, we would have hypothesized that fish biodiversity should have decreased in lakes in such close proximity to oilsands and at higher elevations where climate is changing rapidly. For example, both Primrose and Wolf Lakes are relatively close to oilsands. Although Primrose Lake has experienced large declines in fish biodiversity, Wolf Lake has seen gains in fish species.
Nevertheless, we hope that this study can begin to provide a framework to analyze temporal patterns in biodiversity and serve as a guide to advise on conservation efforts to protect endemic species and freshwater ecosystems from intensifying co-occurring environmental threats (Socolar et al., 2016). Specifically, by mapping the temporal changes of alpha diversity, it is possible to identify the biodiversity hotspots that experience major species losses, such as Primrose Lake, which should be prioritized in the conservation context (Fugère et al., 2016). On the other hand, the relationship between environmental stressors and ecological patterns involving local species introductions and extirpations can be investigated by measuring the changes in beta diversity (Socolar et al., 2016; Sarker et al., 2019; Zhang et al., 2022). Long-term studies of biodiversity are integral at providing valuable insights to evaluate the impacts of environmental disturbance on aquatic communities, thereby subsequently allowing us to predict the consequences of global environmental change on our freshwater communities.
Data availability statement
The data supporting the conclusions of this article are available at: https://doi.org/10.6084/m9.figshare.24886569.v1.
Author contributions
TW, TN, MI, JK, and SS contributed to the conception and design of the study. TW, TN, MI acquired and organized data. TW and TN performed the statistical analysis. TW and MI created the figures and tables. TW, TN, and SS wrote the first draft of the manuscript. All authors contributed to the article and approved the submitted version.
Funding
The author(s) declare financial support was received for the research, authorship, and/or publication of this article. Funding for this research was provided by Genomes Canada through the Gen-FISH project, the Natural Sciences and Engineering Research Council Discovery Grant and the York University Research Chair Programme to SS.
Acknowledgments
We thank Shahanaz Akter, Danica Pring, and Aman Basu for their assistance in the early stages of the project with literature reviews and data acquisition. Their efforts are highly appreciated in supporting this study.
Conflict of interest
The authors declare that the research was conducted in the absence of any commercial or financial relationships that could be construed as a potential conflict of interest.
Publisher’s note
All claims expressed in this article are solely those of the authors and do not necessarily represent those of their affiliated organizations, or those of the publisher, the editors and the reviewers. Any product that may be evaluated in this article, or claim that may be made by its manufacturer, is not guaranteed or endorsed by the publisher.
Supplementary material
The Supplementary Material for this article can be found online at: https://www.frontiersin.org/articles/10.3389/fevo.2023.1129356/full#supplementary-material
References
Alberta-Biodiversity-Monitoring-Institute (2020) Alberta parks. Available at: https://abmi.ca/home/reports/2020/human-footprint/details.html?id=1.
Anderson M. J., Crist T. O., Chase J. M., Vellend M., Inouye B. D., Freestone A. L., et al. (2011). Navigating the multiple meanings of β diversity: a roadmap for the practicing ecologist. Ecol. Lett. 14 (1), 19–28. doi: 10.1111/j.1461-0248.2010.01552.x
Barbour C. D., Brown J. H. (1974). Fish species diversity in lakes. Am. Nat. 108 (962), 473–489. doi: 10.1086/282927
Brie A. E., Southee F. M., Jenni L. M. (2016). Using climate and a minimum set of local characteristics to predict the future distributions of freshwater fish in Ontario, Canada, at the lake-scale. Global Ecol. Conserv. 8, 71–84. doi: 10.1016/j.gecco.2016.08.006
Brucet S., Pédron S., Mehner T., Lauridsen T. L., Argillier C., Winfield I. J., et al. (2013). Fish diversity in European lakes: geographical factors dominate over anthropogenic pressures. Freshw. Biol. 58 (9), 1779–1793. doi: 10.1111/fwb.12167
Burkhead N. M. (2012). Extinction rates in North American freshwater fishes 1900–2010. BioScience 62 (9), 798–808. doi: 10.1525/bio.2012.62.9.5
Campana S. E., Casselman J. M., Jones C. M., Black G., Barker O., Evans M., et al. (2020). Arctic freshwater fish productivity and colonization increase with climate warming. Nat. Climate Change 10 (5), 428–433. doi: 10.1038/s41558-020-0744-x
Cazelles K., Bartley T., Guzzo M. M., Brice M.-H., MacDougall A. S., Bennett J. R., et al. (2019). Homogenization of freshwater lakes: Recent compositional shifts in fish communities are explained by gamefish movement and not climate change. Global Change Biol. 25 (12), 4222–4233. doi: 10.1111/gcb.14829
Coker G. A., Portt C. B., Minns C. K. (2001). Morphological and ecological characteristics of Canadian freshwater fishes. Can. Man Rep. Fish. Aquat. Sci. 2554(January), 1–95. Retrieved from http://publications.gc.ca/collections/collection_2007/dfo-mpo/Fs97-4-2554E.pdf.
Daly C., Halbleib M., Smith J. I., Gibson W. P., Doggett M. K., Taylor G. H., et al. (2008). Physiographically sensitive mapping of climatological temperature and precipitation across the conterminous United States. Int. J. Climatology 28 (15), 2031–2064. doi: 10.1002/joc.1688
Dornelas M., Gotelli N. J., McGill B., Shimadzu H., Moyes F., Sievers C., et al. (2014). Assemblage time series reveal biodiversity change but not systematic loss. Science 344 (6181), 296–299. doi: 10.1126/science.1248484
Dray S., Bauman D., Blanchet G., Borcard D., Clappe S., Guénard G., et al. (2022). adespatial: multivariate multiscale spatial analysis. R package version0.3-23. https://CRAN.R-project.org/package=adespatial.
Dudgeon D., Arthington A. H., Gessner M. O., Kawabata Z. I., Knowler D. J., Lévêque C., et al. (2006). Freshwater biodiversity: importance, threats, status and conservation challenges. Biol. Rev. 81 (2), 163–182. doi: 10.1017/S1464793105006950
Eilers J., Dw L., St. Amand A., Vogel A., Ls J., Kann J., et al. (2007). Biological effects of repeated fish introductions in a formerly fishless lake: Diamond Lake, Oregon, USA. Fundam. Appl. Limnology/Archiv für Hydrobiologie 169, 265–277. doi: 10.1127/1863-9135/2007/0169-0265
Erős T., Czeglédi I., Tóth R., Schmera D. (2020). Multiple stressor effects on alpha, beta and zeta diversity of riverine fish. Sci. Total Environ. 748, 141407. doi: 10.1016/j.scitotenv.2020.141407
Finderup Nielsen T., Sand-Jensen K., Dornelas M., Bruun H. H. (2019). More is less: net gain in species richness, but biotic homogenization over 140 years. Ecol. Lett. 22 (10), 1650–1657. doi: 10.1111/ele.13361
Froese R., Pauly D. (2023). FishBase (World Wide Web electronic publication). Available at: www.fishbase.org.
Fugère V., Kasangaki A., Chapman L. J. (2016). Land use changes in an afrotropical biodiversity hotspot affect stream alpha and beta diversity. Ecosphere 7 (6), e01355. doi: 10.1002/ecs2.1355
Guo C., Lek S., Ye S., Li W., Liu J., Chen Y., et al. (2015). Predicting fish species richness and assemblages with climatic, geographic and morphometric factors: A broad-scale study in Chinese lakes. Limnologica 54, 66–74. doi: 10.1016/j.limno.2015.08.002
Holzapfel A. M., Vinebrooke R. D. (2005). Environmental warming increases invasion potential of alpine lake communities by imported species. Global Change Biol. 11 (11), 2009–2015. doi: 10.1111/j.1365-2486.2005.001057.x
Iacarella J. C., Adamczyk E., Bowen D., Chalifour L., Eger A., Heath W., et al. (2018). Anthropogenic disturbance homogenizes seagrass fish communities. Global Change Biol. 24 (5), 1904–1918. doi: 10.1111/gcb.14090
Irz P., Argillier C., Oberdorff T. (2004). Native and introduced fish species richness in french lakes: local and regional influences. Global Ecol. Biogeography 13 (4), 335–344. doi: 10.1111/j.1466-822X.2004.00109.x
Islam D., Berkes F. (2016). Indigenous peoples’ fisheries and food security: a case from northern Canada. Food Secur. 8 (4), 815–826. doi: 10.1007/s12571-016-0594-6
Jaccard P. (1908). Nouvelles recherches sur la distribution florale. Bull. la Societe Vaudoise Des. Sci. Naturelles 44, 223–270. doi: 10.5169/seals-268384
Johnson R. K., Angeler D. G. (2014). Effects of agricultural land use on stream assemblages: Taxon-specific responses of alpha and beta diversity. Ecol. Indic. 45, 386–393. doi: 10.1016/j.ecolind.2014.04.028
Kienzle S. W. (2018). Has it become warmer in Alberta? Mapping temperature changes for the period 1950–2010 across Alberta, Canada. Can. Geographer/Le Géographe canadien 62 (2), 144–162. doi: 10.1111/cag.12432
Komers P. E., Stanojevic Z. (2013). Rates of Disturbance vary by data resolution: implications for conservation schedules using the Alberta Boreal Forest as a case study. Global Change Biol. 19 (9), 2916–2928. doi: 10.1111/gcb.12266
Kortz A. R., Magurran A. E. (2019). Increases in local richness (α-diversity) following invasion are offset by biotic homogenization in a biodiversity hotspot. Biol. Lett. 15 (5), 20190133. doi: 10.1098/rsbl.2019.0133
Legendre P. (2019). A temporal beta-diversity index to identify sites that have changed in exceptional ways in space–time surveys. Ecol. Evol. 9 (6), 3500–3514. doi: 10.1002/ece3.4984
Legendre P., De Cáceres M. (2013). Beta diversity as the variance of community data: dissimilarity coefficients and partitioning. Ecol. Lett. 16 (8), 951–963. doi: 10.1111/ele.12141
Lehtonen H. (1996). Potential effects of global warming on northern European freshwater fish and fisheries. Fisheries Manage. Ecol. 3 (1), 59–71. doi: 10.1111/j.1365-2400.1996.tb00130.x
Lindenmayer D. B., Lavery T., Scheele B. C. (2022). Why we need to invest in large-scale, long-term monitoring programs in landscape ecology and conservation biology. Curr. Landscape Ecol. Rep. 7 (4), 137–146. doi: 10.1007/s40823-022-00079-2
Lindholm M., Alahuhta J., Heino J., Toivonen H. (2020). No biotic homogenisation across decades but consistent effects of landscape position and pH on macrophyte communities in boreal lakes. Ecography 43 (2), 294–305. doi: 10.1111/ecog.04757
Lindholm M., Alahuhta J., Heino J., Toivonen H. (2021). Temporal beta diversity of lake plants is determined by concomitant changes in environmental factors across decades. J. Ecol. 109 (2), 819–832. doi: 10.1111/1365-2745.13508
Louthan A. M., Doak D. F., Angert A. L. (2015). Where and when do species interactions set range limits? Trends Ecol. Evol. 30 (12), 780–792. doi: 10.1016/j.tree.2015.09.011
Luck G. W., Smallbone L. T. (2010). “Species diversity and urbanisation: patterns, drivers and implications,” in Urban ecology. Ed. Gaston K. J. (Cambridge: Cambridge University Press), 88–119.
Macarthur R. H. (1965). PATTERNS OF SPECIES DIVERSITY. Biol. Rev. 40 (4), 510–533. doi: 10.1111/j.1469-185X.1965.tb00815.x
Magnuson J. J., Crowder L. B., Medvick P. A. (1979). Temperature as an ecological resource. Am. Zoologist 19 (1), 331–343. doi: 10.1093/icb/19.1.331
Magurran A. E., Dornelas M., Moyes F., Gotelli N. J., McGill B. (2015). Rapid biotic homogenization of marine fish assemblages. Nat. Commun. 6 (1), 8405. doi: 10.1038/ncomms9405
Magurran A. E., Dornelas M., Moyes F., Henderson P. A. (2019). Temporal β diversity—A macroecological perspective. Global Ecol. Biogeography 28 (12), 1949–1960. doi: 10.1111/geb.13026
Manjarrés-Hernández A., Guisande C., García-Roselló E., Heine J., Pelayo-Villamil P., Pérez-Costas E., et al. (2021). Predicting the effects of climate change on future freshwater fish diversity at global scale. Nat. Conserv. 43, 1–24. doi: 10.3897/natureconservation.43.58997
Marchetti M., Light T., Feliciano J., Armstrong T., Hogan Z., Viers J., et al. (2001). Homogenization of California’s fish fauna through abiotic change. In Biotic Homogenization. (Springer US), 259–278. doi: 10.1007/978-1-4615-1261-5_13
McGill B. J., Dornelas M., Gotelli N. J., Magurran A. E. (2015). Fifteen forms of biodiversity trend in the Anthropocene. Trends Ecol. Evol. 30 (2), 104–113. doi: 10.1016/j.tree.2014.11.006
Messager M., Lehner B., Grill G., Nedeva I., Schmitt O. (2016). Estimating the volume and age of water stored in global lakes using a geo-statistical approach. Nat. Commun. 7, 13603. doi: 10.1038/ncomms13603
Moi D. A., Alves D. C., Figueiredo B. R. S., Antiqueira P. A. P., Teixeira de Mello F., Jeppesen E., et al. (2021). Non-native fishes homogenize native fish communities and reduce ecosystem multifunctionality in tropical lakes over 16 years. Sci. Total Environ. 769, 144524. doi: 10.1016/j.scitotenv.2020.144524
Murdoch A., Mantyka-Pringle C., Sharma S. (2020a). The interactive effects of climate change and land use on boreal stream fish communities. Sci. Total Environ. 700, 134518. doi: 10.1016/j.scitotenv.2019.134518
Murdoch A., Mantyka-Pringle C., Sharma S. (2020b). Impacts of co-occurring environmental changes on Alaskan stream fishes. Freshw. Biol. 65 (10), 1685–1701. doi: 10.1111/fwb.13569
Mutethya E., Yongo E. (2021). A comprehensive review of invasion and ecological impacts of introduced common carp (Cyprinus carpio) in Lake Naivasha, Kenya. Lakes Reservoirs: Science Policy Manage. Sustain. Use 26 (4), e12386. doi: 10.1111/lre.12386
Oksanen J., Legendre P., O’Hara B., Simpson G., Solymos P., Stevens M., et al. (2008). vegan: Community Ecology Package. R package version 2.6-4. https://CRAN.R-project.org/package=vegan.
Olden J. D., Poff N. L. (2003). Toward a mechanistic understanding and prediction of biotic homogenization. Am. Nat. 162 (4), 442–460. doi: 10.1086/378212
Olden J. D., Rooney T. P. (2006). On defining and quantifying biotic homogenization. Global Ecol. Biogeography 15 (2), 113–120. doi: 10.1111/j.1466-822X.2006.00214.x
O’Reilly C. M., Sharma S., Gray D. K., Hampton S. E., Read J. S., Rowley R. J., et al. (2015). Rapid and highly variable warming of lake surface waters around the globe. Geophysical Res. Lett. 42 (24), 10,773–710,781. doi: 10.1002/2015GL066235
Pauchard A., Milbau A., Albihn A., Alexander J., Burgess T., Daehler C., et al. (2016). Non-native and native organisms moving into high elevation and high latitude ecosystems in an era of climate change: new challenges for ecology and conservation. Biol. Invasions 18 (2), 345–353. doi: 10.1007/s10530-015-1025-x
R Core Team (2021). R: A language and environment for statistical computing (Vienna, Austria: R Foundation for Statistical Computing). Available at: https://www.R-project.org/.
Rahel F. J. (2002). Homogenization of freshwater faunas. Annu. Rev. Ecol. Systematics 33 (1), 291–315. doi: 10.1146/annurev.ecolsys.33.010802.150429
Rahel F. J., Olden J. D. (2008). Assessing the effects of climate change on aquatic invasive species. Conserv. Biol. 22 (3), 521–533. doi: 10.1111/j.1523-1739.2008.00950.x
Reid A. J., Carlson A. K., Creed I. F., Eliason E. J., Gell P. A., Johnson P. T. J., et al. (2019). Emerging threats and persistent conservation challenges for freshwater biodiversity. Biol. Rev. Camb Philos. Soc. 94 (3), 849–873. doi: 10.1111/brv.12480
Richardson L. E., Graham N. A. J., Pratchett M. S., Eurich J. G., Hoey A. S. (2018). Mass coral bleaching causes biotic homogenization of reef fish assemblages. Global Change Biol. 24 (7), 3117–3129. doi: 10.1111/gcb.14119
Rolls R. J., Hayden B., Kahilainen K. K. (2017). Conceptualising the interactive effects of climate change and biological invasions on subarctic freshwater fish. Ecol. Evol. 7 (12), 4109–4128. doi: 10.1002/ece3.2982
Sarker S. K., Reeve R., Paul N. K., Matthiopoulos J. (2019). Modelling spatial biodiversity in the world’s largest mangrove ecosystem—The Bangladesh Sundarbans: A baseline for conservation. Diversity Distributions 25 (5), 729–742. doi: 10.1111/ddi.12887
Scott W. B., Crossman E. J. (1973). Freshwater fishes of Canada. Bull. Fisheries Res. Board Canada 184), 966. doi: 10.14430/arctic3062
Sharma S., Vander Zanden M. J., Magnuson J. J., Lyons J. (2011). Comparing climate change and species invasions as drivers of coldwater fish population extirpations. PloS One 6 (8), e22906. doi: 10.1371/journal.pone.0022906
Sharma S., Jackson D. A. (2007). Fish assemblages and environmental conditions in the lower reaches of northeastern lake erie tributaries. J. Great Lakes Res. 33, 15–27.
Sleezer L. J., Angermeier P. L., Frimpong E. A., Brown B. L. (2021). A new composite abundance metric detects stream fish declines and community homogenization during six decades of invasions. Diversity Distributions 27 (11), 2136–2156. doi: 10.1111/ddi.13393
Smokorowski K. E., Pratt T. C. (2007). Effect of a change in physical structure and cover on fish and fish habitat in freshwater ecosystems – a review and meta-analysis. Environ. Rev. 15, 15–41. doi: 10.1139/a06-007
Socolar J. B., Gilroy J. J., Kunin W. E., Edwards D. P. (2016). How should beta-diversity inform biodiversity conservation? Trends Ecol. Evol. 31 (1), 67–80. doi: 10.1016/j.tree.2015.11.005
Soukup P. R., Näslund J., Höjesjö J., Boukal D. S. (2022). From individuals to communities: Habitat complexity affects all levels of organization in aquatic environments (Wiley Interdisciplinary Reviews: Water. John Wiley and Sons Inc). doi: 10.1002/wat2.1575
Van Zuiden T. M., Sharma S. (2016). Examining the effects of climate change and species invasions on Ontario walleye populations: can walleye beat the heat? Diversity Distributions 22 (10), 1069–1079. doi: 10.1111/ddi.12468
Villéger S., Blanchet S., Beauchard O., Oberdorff T., Brosse S. (2011). Homogenization patterns of the world’s freshwater fish faunas. Proc. Natl. Acad. Sci. 108 (44), 18003–18008. doi: 10.1073/pnas.1107614108
Vincent L. A., Wang X. L., Milewska E. J., Wan H., Yang F., Swail V. (2012). A second generation of homogenized Canadian monthly surface air temperature for climate trend analysis. J. Geophysical Research: Atmospheres 117 (D18). doi: 10.1029/2012JD017859
Vogt R. J., Sharma S., Leavitt P. R. (2018). Direct and interactive effects of climate, meteorology, river hydrology, and lake characteristics on water quality in productive lakes of the Canadian Prairies. Can. J. Fisheries Aquat. Sci. 75 (1), 47–59. doi: 10.1139/cjfas-2016-0520
Wei T., Viliam S. (2021). R package ‘corrplot’: visualization of a correlation matrix (Version 0.92). Available from https://github.com/taiyun/corrplot.
Wellborn G. A., Skelly D. K., Werner E. E. (1996). Mechanisms creating community structure across a freshwater habitat gradient. Annu. Rev. Ecol. Systematics 27, 337–363.
Whittaker R. H. (1960). Vegetation of the Siskiyou mountains, Oregon and California. Ecol. Monogr. 30 (3), 279–338. doi: 10.2307/1943563
Whittaker R. H. (1972). EVOLUTION AND MEASUREMENT OF SPECIES DIVERSITY. TAXON 21 (2-3), 213–251. doi: 10.2307/1218190
Williams C. B. (1943). AREA AND NUMBER OF SPECIES. Nature 152 (3853), 264–267. doi: 10.1038/152264a0
Winegardner A. K., Legendre P., Beisner B. E., Gregory-Eaves I. (2017). Diatom diversity patterns over the past c. 150 years across the conterminous United States of America: Identifying mechanisms behind beta diversity. Global Ecol. Biogeography 26 (11), 1303–1315. doi: 10.1111/geb.12640
Winkler K., Fuchs R., Rounsevell M. D. A., Herold M. (2020). HILDA+ Global Land Use Change between 1960 and 2019. PANGAEA. doi: 10.1594/PANGAEA.921846
Woolway R., Kraemer B., Lenters J., Merchant C., Oreilly C., Sharma S. (2020). Global lake responses to climate change. Nat. Rev. Earth Environ. 1. doi: 10.1038/s43017-020-0067-5
Wu T., Imrit M. A., Movahedinia Z., Kong J., Woolway R. I., Sharma S. (2022). Climate tracking by freshwater fishes suggests that fish diversity in temperate lakes may be increasingly threatened by climate warming. Diversity Distributions 29 (2), 300–315. doi: 10.1111/ddi.13664
Zeni J. O., Hoeinghaus D. J., Roa-Fuentes C. A., Casatti L. (2020). Stochastic species loss and dispersal limitation drive patterns of spatial and temporal beta diversity of fish assemblages in tropical agroecosystem streams. Hydrobiologia 847 (18), 3829–3843. doi: 10.1007/s10750-020-04356-1
Keywords: species richness, beta diversity, freshwater fishes, Albertan lakes, biodiversity, climate change, land-use change
Citation: Wu T, Nguyen TN, Imrit MA, Kong J and Sharma S (2024) Increasing fish biodiversity in high elevation Albertan lakes in response to global environmental change over the past 50 years. Front. Ecol. Evol. 11:1129356. doi: 10.3389/fevo.2023.1129356
Received: 21 December 2022; Accepted: 11 December 2023;
Published: 15 January 2024.
Edited by:
Anouschka R. Hof, Wageningen University and Research, NetherlandsReviewed by:
Michael Alister Reid, University of New England, AustraliaAnielly Galego De Oliveira, State University of Maringá, Brazil
Copyright © 2024 Wu, Nguyen, Imrit, Kong and Sharma. This is an open-access article distributed under the terms of the Creative Commons Attribution License (CC BY). The use, distribution or reproduction in other forums is permitted, provided the original author(s) and the copyright owner(s) are credited and that the original publication in this journal is cited, in accordance with accepted academic practice. No use, distribution or reproduction is permitted which does not comply with these terms.
*Correspondence: Sapna Sharma, c2hhcm1hMTFAeW9ya3UuY2E=