- 1School of Geography and Ecotourism, Southwest Forestry University, Kunming, Yunnan, China
- 2Southwest Research Center for Eco-civilization, National Forestry and Grassland Administration, Kunming, Yunnan, China
The losses of biodiversity have impaired functioning and provision of ecosystem services, and the relationship between biodiversity and ecosystem functioning has emerged as a central issue in environmental sciences. However, the majority of relevant studies are conducted in terrestrial ecosystems, and they focus predominantly on the relationship between community diversity and biomass production of terrestrial vegetation. At present, water eutrophication represents an increasingly serious problem worldwide, and the use of aquatic organisms for improving water quality represents a promising approach. However, more focus is placed on the selection of certain aquatic organisms with good performance, but neglects the effects of biodiversity in the process of water purification and the underlying mechanisms. In the present study, five microalgal species commonly found in freshwater ecosystems were used to assembly experimental microcosms with varying microalgal richness and composition. We analyzed the relationship between microalgal diversity and nitrogen removal efficiency based on mixed-effect models, and further explored the underlying mechanism of microalgal diversity in the process of water quality improvement. The results showed that with an increase in microalgal diversity, nitrogen removal efficiency of microalgal communities also increased. A further analysis of the impacts of microalgal diversity showed that the complementarity effect increased while the selection effect decreased with an increase in microalgal diversity. Meanwhile, there was a significantly positive relationship between microalgal diversity and the total abundance of microalgae. On the one hand, the present study clearly demonstrates two positive diversity-ecosystem functioning relationships. On the other hand, the present study also reveals the underlying mechanism by which microalgal diversity influences nitrogen removal efficiency, namely, high-diversity microalgal communities could use limiting nutrients such as nitrogen in a more efficient and complementary manner (e.g., stronger complementarity effect in high-diversity communities), convert them into higher aggregate community properties (e.g., higher total abundance of microalge in high-diversity communities), and thus exhibit higher purification capacity (e.g., higher nitrogen removal efficiency in high-diversity communities). Under the scenario that global ecosystems are experiencing high rates of anthropogenic nutrient inputs, the use of diverse microalgal species with proper management may help provide a promising approach for improving water quality.
1. Introduction
Contemporary biodiversity is rapidly declining worldwide at unprecedented rates. Since biodiversity begets ecosystem functioning (e.g., productivity, nutrient cycling) and ecosystem stability (e.g., temporal invariability of productivity), ongoing biodiversity erosion inevitably leads to a rapid decline in ecosystem functions and services that are vital to the well-being of human societies (Naeem et al., 1999). Consequently, biodiversity loss has received global concerns, and to better understand the consequences of such losses, research on elucidating the relationships between biodiversity and ecosystem functioning (BEF) has been continuously reinforced over the past half century (Hooper et al., 2005; Duffy, 2009; Naeem et al., 2009; Tilman et al., 2014).
Mounting evidence supports significant and positive BEF relationships based on well-designed grassland experiments (Tilman et al., 1997, 2012; Hector et al., 1999; Tilman, 1999; Reich et al., 2012). There are two primary mechanisms that are responsible for the positive effect of biodiversity on ecosystem functioning, namely, the complementarity effect and the selection effect. The complementarity effect means that as biodiversity increases, species could use limiting resources in a more efficient and complementary manner, and thus further enhance ecosystem functioning (Tilman, 1999). The selection effect means that as biodiversity increases, the probability of including species that are numerically dominant or functionally important also increases, and such species could be more influential than other species in determining ecosystem functioning (Loreau and Hector, 2001). The complementarity and selection effects are not mutually exclusive. Rather, they often operate simultaneously to co-affect ecosystem functioning (Fargione et al., 2007).
Freshwater scarcity and pollution represent some major environmental issues. Particularly, freshwater eutrophication is a leading cause of the impairment of freshwater ecosystems worldwide, which occurs mainly due to the discharge of agricultural nutrient surpluses into water bodies. Freshwater eutrophication disrupts natural balance in aquatic ecosystems, degrades freshwater ecosystems services, causes losses in freshwater biodiversity, and threatens human well-being and health of the planet (Khan and Mohammad, 2014). Nitrogen is an essential nutrient that limits the growth and development of aquatic organisms, and serves as a major factor that causes water eutrophication and water quality degradation. Currently, people are actively exploring the potential of using aquatic organisms to help remove excess nitrogen from water bodies and improve water quality. For example, constructed wetlands are widely used to treat excess nutrient contaminants in the wastewater, and related studies often find that macrophytes play an important role in the removal of nutrients from wastewater (Shah et al., 2014; Kurniawan et al., 2021). However, when constructed wetlands are developed, people tend to select certain macrophyte species with good performance (Brisson and Chazarenc, 2009), but neglect the fact that communities consisting of diverse macrophytes are often more efficient in improving water quality than communities dominated by one or two macrophyte species. Even among studies that assembled constructed wetlands with varying macrophyte richness and composition, the mechanisms by which macrophytes diversity affect wastewater treatment efficiency are unclear.
Similar to macrophytes, microalgae (i.e., microscopic single-celled algae) are also common aquatic photosynthetic organisms that play an important role in removing excess nutrients that overload water bodies (Cardinale, 2011). However, they are strongly inhibited by macrophytes due to shading, nutrient competition, and/or allelopathic effects (Körner and Nicklisch, 2002; Mulderij et al., 2007; Ferreira et al., 2018). In other words, there is often an inverse microalgae-macrophyte relationship (Scheffer, 1998), and current studies tend to ignore the important role played by diverse algae in the process of water purification. Actually, microalgae have important ecological and economic values (Weber and Deutsch, 2010; Sharma and Rai, 2011). They form the energy base of the food web for aquatic heterotrophic organisms. Also, they represent important source of oxygen supply through photosynthesis, and serve as a promising tool for carbon sequestration when compared to conventional forestry, agricultural, and aquatic plants due to their simple nutritional requirements and rapid growth rates (Singh and Ahluwalia, 2013; Cuellar-Bermudez et al., 2015). Meanwhile, microalgae have high economic values. They produce amino acids, proteins, lipids, vitamins and carotenoids that are nutritionally beneficial to the health of animals and humans. In particular, lipids produced by microalgae have a high potential for the production of renewable fuels (Alami et al., 2021), and biodiesel production from algal lipids is non-toxic and highly biodegradable (Menetrez, 2012). Obviously, a microalgae-mediated CO2 fixation can be rendered more sustainable by coupling microalgal multi-product production with wastewater treatment. However, current studies of microalgae-based wastewater treatment focus on strain selection (Álvarez-Díaz et al., 2017; Aketo et al., 2020), and thus neglect the capacity of microalgal diversity in enhancing nutrient capture efficiency. Given that, it is not surprising that some essential questions, such as what is the relationship between microalgal diversity and water purifying capacity, and what are the underlying mechanisms that link microalgal diversity to water quality improvement, remain unaddressed.
Due to increasing pressures on water quality, aquatic life, ecosystem services and human health, studies that explore the possibilities of using microalgae to deal with water eutrophication problem have important theoretical and practical significance, especially when key ecological principles, such as the concept of BEF, are applied to provide practical guidance for water quality improvement. In order to better control environmental conditions and reveal the underlying mechanisms of BEF, we assembled freshwater microalgal communities with varying species richness and composition, analyzed the relationship between microalgal richness and nitrogen removal efficiency, and revealed water purification mechanism of microalgal diversity. In the present study, the following hypotheses are specifically tested--H1: as microalgal richness increases, the total number of microalgae, one important component of aggregate community properties, also increases; H2: as microalgal richness increases, nitrogen removal efficiency of microalgal communities also increases; H3: the selection effect decreases while the complementarity effect increases with an increase in microalgal richness.
2. Methods
Five common freshwater microalgal species, including Closterium libellula, Cosmarium sportella, Selenastrum capricornutum, Scenedesmus quadricauda and Actinastrum hantzschii, were used for the assembly of experimental microcosms. These selected microalgal species are morphologically diverse, which makes them easy to distinguish while counting samples. All microalgal species were obtained from collections at the Institute of Hydrobiology, China (FACHB), and they grew well inside a growth chamber at 20°C with a 16:8 h light dark cycle.
The microalgal richness treatment consisted of all possible monocultures of each of the five focal species, 5 randomly chosen two-species mixtures, 5 randomly chosen three-species mixtures, 5 full four-species mixtures, and 1 full five-species mixture. Microalgal species were inoculated according to a replacement-series design, where total microalgal density was held constant at 6000 cells per mL across all levels of species richness, and there were 3 replicates for each richness level. All experimental microcosms were cultivated in 100 mL glass flasks filled with 40 mL standard BG-11 culture medium. All of the cultivated microcosms were manually shaken on a daily basis. Each microcosm was sampled every 7 days up to the final day of the experiment to track microalgal dynamics. On each sampling day, 0.4 mL medium was withdrawn for visual counts microscopically, and the number of individuals of each microalgal species was recorded. 10% of the culture medium in each microcosm was replaced each week with fresh standard medium to support microalgae growth and reduce metabolic waste accumulation.
The alkaline potassium persulfate digestion UV spectrophotometric method (Smart et al., 1981) was applied to measure total nitrogen concentrations in the culture medium at the start and the end of the experiment. Nitrogen removal efficiency was quantified as the difference in total nitrogen concentrations between these two sampling periods divided by initial total nitrogen concentration. Linear mixed-effect models (LMMs) were applied to test the effects of species richness (the fixed factor) and species composition (the random factor) on nitrogen removal efficiency. LMMs were performed using the “lmer” function in the package “lme4” (Bates et al., 2014) in the statistical software R. We further quantified marginal and conditional R2 values to compare the relative importance of species richness and species composition in determining nitrogen removal efficiency, as marginal R2 is the proportion of variance explained by the fixed effects, while conditional R2 is the proportion explained by the full model, including both fixed and random effects (Nakagawa and Schielzeth, 2013). Both marginal and conditional R2 values were obtained using the function “r.squaredGLMM” provided in the package “MuMIn” (Barton, 2009). We used the additive partitioning method (Loreau and Hector, 2001) to quantify the complementarity and selection effects. The complementarity effect (CE) for a specific number of species k was , where is the deviation from expected performance of a given species in the mixture, and is the average monoculture performance. The selection effect (SE) for a specific number of species k was , where the covariance between the monoculture performance of species and their change in relative yield in the mixture, , was multiplied by the number of species, k, in the mixture. The additive partitioning analysis was performed using the package “partitionBEFsp” (Clark et al., 2019).
3. Results
Nitrogen removal efficiency of microalgal communities increased significantly with an increase in microalgal richness (Fixed effects: DF = 1, Mean Squares = 2,039, F = 68.87, p < 0.001; Figure 1). Also, nitrogen removal efficiency of microalgal communities varied with species composition (Random effects: DF = 21, Variance = 3.02, SD = 1.73). The marginal and conditional R2 value was 0.57 and 0.61, respectively, suggesting that the fixed variable, microalgal richness, was more important than the random variable, species composition, in determining nitrogen removal efficiency. The biodiversity effect of microalgal richness was further partitioned into the complementarity effect and the selection effect, and the complementarity effect increased (F1,61 = 56.38, p < 0.001), while the selection effect decreased (F1,61 = 36.93, p < 0.001), with an increase in microalgal richness (Figure 2). A further analysis showed that the total abundance of high-richness microalgal communities was higher than that of low-richness communities, and thus there was a significantly positive relationship between microalgal richness and the total abundance of microalgal communities (F1,61 = 28.96, p < 0.001; Figure 3).
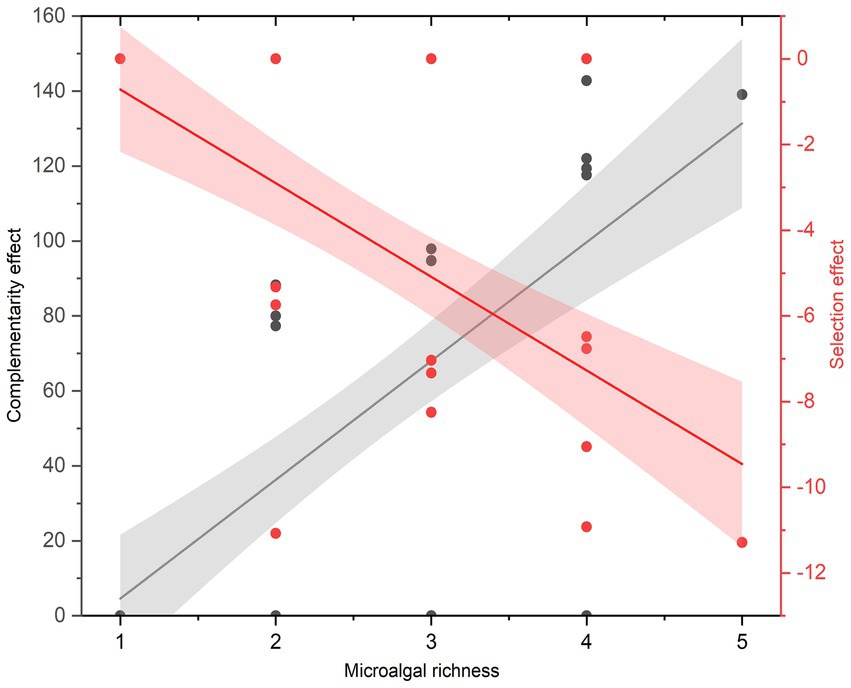
Figure 2. The relationships between microalgal richness, the complementarity effect and the selection effect.
4. Discussion
Our results showed that the total abundance of microalgal communities increased with an increase in microalgal richness, which support the first hypothesis (H1), and corroborate with the findings of many observational and experimental studies that also showed a consistent positive relationship between species richness and community aggregated properties (Hutchinson, 1961; Tilman et al., 2014; Duffy et al., 2017). Our results also showed a positive relationship between microalgal richness and nitrogen removal efficiency, which support the second hypothesis (H2), and are in alignment with previous studies that demonstrated a positive relationship between microalgae richness and nutrient use efficiency, found in both natural (Ptacnik et al., 2008) and artificial (Cardinale, 2011) aquatic systems. Interestingly, a few studies showed that harmful algal blooms could significantly reduce nutrient use efficiency of phytoplankton communities via reducing phytoplankton richness (Zhou et al., 2018; Chai et al., 2020), thus provided clear evidence for the positive relationship between microalgae richness and nutrient use efficiency. Our study, together with some recent research that also aims to test the relationships between biodiversity and multifaceted aspects of ecosystem functions, can help advance our understanding of BEF. We further explored the underlying ecological mechanisms by which microalgal diversity influences nitrogen uptake efficiency. Our findings showed that the selection effect decreased while the complementarity effect increased with an increase in microalgal richness, supporting the third hypothesis (H3). The results suggested that the competitive intensity could increase with increasing microalgal richness, which enforced microalgae to acquire and utilize limiting nutrients in a more complementary manner to coexist through niche partitioning. Although similar conclusions are drawn by some other studies, the vast majority of them are conducted across major terrestrial biomes in general, and within grasslands in specific (Reich et al., 2012; Tilman et al., 2014). Therefore, the present study complements previous studies on elucidating the relationships between biodiversity and ecosystem functioning (BEF).
In the present study, highly controlled laboratory conditions were established in order to better explore water purification mechanisms of microalgal diversity. However, we should be cautious when laboratory-based findings are applied to natural freshwater ecosystems. Although constructed wetlands do not belong to the category of natural aquatic ecosystems, many observational and experimental studies conducted in constructed wetlands showed that macrophytes can impose a strong inhibitory effect on the growth of microalgae (Körner and Nicklisch, 2002; Mulderij et al., 2007). Therefore, in a macrophyte-dominated natural community, water purification capacity of microalgae might be severely constrained. Meanwhile, regardless of the identity of freshwater autotrophs, water purification capacity of freshwater autotrophs follows a dynamic process. In the early stage, autotrophs in a high-richness community can utilize limiting nutrients and convert them into their own biomass in a more complementary and efficient manner. Over time, however, once these autotrophs complete their life cycles, decay from dead materials will release large amounts of nutrients back to the water body, which offsets the strong purification capacity of autotrophs exhibited during their vigorous growth period. Therefore, in order to maintain high levels of water purification efficiency, in addition to the conservation of freshwater autotroph diversity, the biomass of autotrophs should be timely harvested before their senescence to prevent a large amount of nutrients from returning to the water column as autotroph residues decompose. As for microalgal communities, the harvest of their biomass serves not only to help maintain water quality, but also provide raw materials with high economic and ecological values. In additional to their high nutritional quality (e.g., Chlorella sp. for high protein quality; Rasheed et al., 2020), and health benefits (e.g., Spirulina sp. for rich omega-3 fatty acids; Karkos et al., 2011), microalgal biomass is a promising resource for environment friendly applications, which is tightly linked to atmospheric CO2 mitigation (Wang et al., 2008), wastewater treatment (Novoveská et al., 2016), and the integration of CO2 fixation with biofuel production (Harun et al., 2010; Khan et al., 2018).
Our present study tests the relationship between microalgal richness and two ecosystem functions, which provides some extension to traditional BEF studies that are confined to the analysis of richness-biomass relationships, and are predominantly conducted in terrestrial ecosystems. However, since the same ecosystem can provide multiple functions simultaneously, and the effects of biodiversity on ecosystem functioning are stronger for multiple functions than for a single function, the quantification of the relationships between biodiversity and ecosystem multifunctionality will undoubtedly advance the field of BEF research (Gamfeldt et al., 2008; Byrnes et al., 2014; van der Plas, 2019). Meanwhile, biodiversity is often quantified as the number of species within a biological community (i.e., species richness). However, species richness is a relatively insensitive metric with low explanatory power, and may fail to reflect important facets of biodiversity (Loreau et al., 2001; Gaston and Fuller, 2008). For example, a microalgal community with higher functional diversity is expected to have a greater resource niche partitioning and thus resource use efficiency (Ye et al., 2019). Therefore, future BEF research should incorporate a spectrum of biodiversity metrics. In addition, given that a majority of BEF studies concern single trophic levels without accounting for interaction within and between adjacent trophic levels, future BEF research should also be performed across multiple taxa, trophic levels and habitats (Lefcheck et al., 2015).
Microalgae, together with other diverse aquatic microorganisms, represent important components of the food web within aquatic ecosystems (Litchman, 2010), drive major biogeochemical cycles (Falkowski et al., 2008), and play a crucial role in determining water quality and human health (Cardinale, 2011). Although aquatic microorganisms, such as green algae, fungi and bacteria, are capable of removing chemical hazards from water bodies, and thus represent valuable biological resources for bioremediation (Dixit et al., 2015; Zeng et al., 2015; Nguyen et al., 2022), waterborne pathogens and the related diseases they cause are major environmental and human health concerns throughout the world (Magana-Arachchi and Wanigatunge, 2020). Meanwhile, our understanding of the distribution, structure and functions of aquatic microorganisms is still very limited. Therefore, we need to advance our knowledge of aquatic microorganisms and their roles in affecting water quality and human health, take effective measures to manage aquatic resources and improve water quality, and minimize the outbreak risks of waterborne pathogens, which represent some urgent work for humankind, and this is especially true in the context of global changes and anthropogenic disturbances.
Data availability statement
The raw data supporting the conclusions of this article will be made available by the authors, without undue reservation.
Author contributions
WL: conceptualization and resources. SZ, WL, and SH: methodology, validation, and writing–original draft preparation. SZ and WL: formal analysis. WL and SH: writing—review and editing. All authors have read and agreed to the published version of the manuscript.
Funding
This work was supported by National Natural Science Foundation of China (31760175 and 31460158).
Acknowledgments
We would like to thank the editor and reviewers for their constructive comments and suggestions, which helped to improve the quality of the manuscript.
Conflict of interest
The authors declare that the research was conducted in the absence of any commercial or financial relationships that could be construed as a potential conflict of interest.
Publisher’s note
All claims expressed in this article are solely those of the authors and do not necessarily represent those of their affiliated organizations, or those of the publisher, the editors and the reviewers. Any product that may be evaluated in this article, or claim that may be made by its manufacturer, is not guaranteed or endorsed by the publisher.
References
Aketo, T., Hoshikawa, Y., Nojima, D., Yabu, Y., Maeda, Y., Yoshino, T., et al. (2020). Selection and characterization of microalgae with potential for nutrient removal from municipal wastewater and simultaneous lipid production. J. Biosci. Bioeng. 129, 565–572. doi: 10.1016/j.jbiosc.2019.12.004
Alami, A. H., Alasad, S., Ali, M., and Alshamsi, M. (2021). Investigating algae for CO2 capture and accumulation and simultaneous production of biomass for biodiesel production. Sci. Total Environ. 759:143529. doi: 10.1016/j.scitotenv.2020.143529
Álvarez-Díaz, P., Ruiz, J., Arbib, Z., Barragán, J., Garrido-Pérez, M., and Perales, J. (2017). Freshwater microalgae selection for simultaneous wastewater nutrient removal and lipid production. Algal Res. 24, 477–485. doi: 10.1016/j.algal.2017.02.006
Barton, K. (2009). MuMIn: multi-model inference. Available at: http://r-forge.r-project.org/projects/mumin/
Bates, D., Mächler, M., Bolker, B., and Walker, S. (2014). Fitting linear mixed-effects models using lme4. arXiv preprint arXiv. doi: 10.48550/arXiv.1406.5823
Brisson, J., and Chazarenc, F. (2009). Maximizing pollutant removal in constructed wetlands: should we pay more attention to macrophyte species selection? Sci. Total Environ. 407, 3923–3930. doi: 10.1016/j.scitotenv.2008.05.047
Byrnes, J. E., Gamfeldt, L., Isbell, F., Lefcheck, J. S., Griffin, J. N., Hector, A., et al. (2014). Investigating the relationship between biodiversity and ecosystem multifunctionality: challenges and solutions. Methods Ecol. Evol. 5, 111–124. doi: 10.1111/2041-210X.12143
Cardinale, B. J. (2011). Biodiversity improves water quality through niche partitioning. Nature 472, 86–89. doi: 10.1038/nature09904
Chai, Z. Y., Wang, H., Deng, Y., Hu, Z., and Tang, Y. Z. (2020). Harmful algal blooms significantly reduce the resource use efficiency in a coastal plankton community. Sci. Total Environ. 704:135381. doi: 10.1016/j.scitotenv.2019.135381
Clark, A. T., Barry, K. E., Roscher, C., Buchmann, T., Loreau, M., and Harpole, W. S. (2019). How to estimate complementarity and selection effects from an incomplete sample of species. Methods Ecol. Evol. 10, 2141–2152. doi: 10.1111/2041-210X.13285
Cuellar-Bermudez, S. P., Garcia-Perez, J. S., Rittmann, B. E., and Parra-Saldivar, R. (2015). Photosynthetic bioenergy utilizing co2: an approach on flue gases utilization for third generation biofuels. J. Clean. Prod. 98, 53–65. doi: 10.1016/j.jclepro.2014.03.034
Dixit, R., Malaviya, D., Pandiyan, K., Singh, U. B., Sahu, A., Shukla, R., et al. (2015). Bioremediation of heavy metals from soil and aquatic environment: an overview of principles and criteria of fundamental processes. Sustainability 7, 2189–2212. doi: 10.3390/su7022189
Duffy, J. E. (2009). Why biodiversity is important to the functioning of real-world ecosystems. Front. Ecol. Environ. 7, 437–444. doi: 10.2307/25595199
Duffy, J. E., Godwin, C. M., and Cardinale, B. J. (2017). Biodiversity effects in the wild are common and as strong as key drivers of productivity. Nature 549, 261–264. doi: 10.1038/nature23886
Falkowski, P. G., Fenchel, T., and Delong, E. F. (2008). The microbial engines that drive earth's biogeochemical cycles. Science 320, 1034–1039. doi: 10.1126/science.1153213
Fargione, J., Tilman, D., Dybzinski, R., Lambers, J. H. R., Clark, C., Harpole, W. S., et al. (2007). From selection to complementarity: shifts in the causes of biodiversity–productivity relationships in a long-term biodiversity experiment. Proc. R. Soc. B 274, 871–876. doi: 10.1098/rspb.2006.0351
Ferreira, T. F., Crossetti, L. O., Marques, D. M. M., Cardoso, L., Fragoso, C. R. Jr., and van Nes, E. H. (2018). The structuring role of submerged macrophytes in a large subtropical shallow lake: clear effects on water chemistry and phytoplankton structure community along a vegetated-pelagic gradient. Limnologica 69, 142–154. doi: 10.1016/j.limno.2017.12.003
Gamfeldt, L., Hillebrand, H., and Jonsson, P. R. (2008). Multiple functions increase the importance of biodiversity for overall ecosystem functioning. Ecology 89, 1223–1231. doi: 10.1890/06-2091.1
Gaston, K. J., and Fuller, R. A. (2008). Commonness, population depletion and conservation biology. Trends Ecol. Evol. 23, 14–19. doi: 10.1016/j.tree.2007.11.001
Harun, R., Danquah, M. K., and Forde, G. M. (2010). Microalgal biomass as a fermentation feedstock for bioethanol production. J. Chem. Technol. Biotechnol. 85, n/a–203. doi: 10.1002/jctb.2287
Hector, A., Schmid, B., Beierkuhnlein, C., Caldeira, M., Diemer, M., Dimitrakopoulos, P. G., et al. (1999). Plant diversity and productivity experiments in european grasslands. Science 286, 1123–1127. doi: 10.1126/science.286.5442.1123
Hooper, D. U., Chapin, F. S. III, Ewel, J. J., Hector, A., Inchausti, P., Lavorel, S., et al. (2005). Effects of biodiversity on ecosystem functioning: a consensus of current knowledge. Ecol. Monogr. 75, 3–35. doi: 10.2307/4539083
Karkos, P., Leong, S., Karkos, C., Sivaji, N., and Assimakopoulos, D. (2011). Spirulina in clinical practice: evidence-based human applications. Evid. Based Complement. Alternat. Med. 2011:531053. doi: 10.1093/ecam/nen058
Khan, M. N., and Mohammad, F. (2014). Eutrophication: challenges and solutions. Eutrophication: causes. Consequen. Control 2, 1–15. doi: 10.1007/978-94-007-7814-6_1
Khan, M. I., Shin, J. H., and Kim, J. D. (2018). The promising future of microalgae: current status, challenges, and optimization of a sustainable and renewable industry for biofuels, feed, and other products. Microb. Cell Factories 17, 1–21. doi: 10.1186/s12934-018-0879-x
Körner, S., and Nicklisch, A. (2002). Allelopathic growth inhibition of selected phytoplankton species by submerged macrophytes1. J. Phycol. 38, 862–871. doi: 10.1046/j.1529-8817.2002.t01-1-02001.x
Kurniawan, S. B., Ahmad, A., Said, N. S. M., Imron, M. F., Abdullah, S. R. S., Othman, A. R., et al. (2021). Macrophytes as wastewater treatment agents: nutrient uptake and potential of produced biomass utilization toward circular economy initiatives. Sci. Total Environ. 790:148219. doi: 10.1016/j.scitotenv.2021.148219
Lefcheck, J. S., Byrnes, J. E., Isbell, F., Gamfeldt, L., Griffin, J. N., Eisenhauer, N., et al. (2015). Biodiversity enhances ecosystem multifunctionality across trophic levels and habitats. Nat. Commun. 6, 6936–6937. doi: 10.1038/ncomms7936
Litchman, E. (2010). Invisible invaders: non-pathogenic invasive microbes in aquatic and terrestrial ecosystems. Ecol. Lett. 13, 1560–1572. doi: 10.1111/j.1461-0248.2010.01544.x
Loreau, M., and Hector, A. (2001). Partitioning selection and complementarity in biodiversity experiments. Nature 412, 72–76. doi: 10.1038/35083573
Loreau, M., Naeem, S., Inchausti, P., Bengtsson, J., Grime, J. P., Hector, A., et al. (2001). Biodiversity and ecosystem functioning: current knowledge and future challenges. Science 294, 804–808. doi: 10.1126/science.1064088
Magana-Arachchi, D., and Wanigatunge, R. (2020). Ubiquitous Waterborne Pathogens. In: Waterborne Pathogens. eds. Majeti Narasimha Vara Prasad and Anna Grobelak Amsterdam: Elsevier.
Menetrez, M. Y. (2012). An overview of algae biofuel production and potential environmental impact. Environ. Sci. Technol. 46, 7073–7085. doi: 10.1021/es300917r
Mulderij, G., Van Nes, E. H., and Van Donk, E. (2007). Macrophyte–phytoplankton interactions: the relative importance of allelopathy versus other factors. Ecol. Model. 204, 85–92. doi: 10.1016/j.ecolmodel.2006.12.020
Naeem, S., Bunker, D. E., Hector, A., Loreau, M., and Perrings, C. (2009). Biodiversity, Ecosystem Functioning, and Human Wellbeing: An Ecological and Economic Perspective. OUP: Oxford.
Naeem, S., Chapin Iii, F., Costanza, R., Ehrlich, P. R., Golley, F. B., Hooper, D. U., et al. (1999). Biodiversity and ecosystem functioning: maintaining natural life support processes. Issues Ecol 4, 2–11.
Nakagawa, S., and Schielzeth, H. (2013). A general and simple method for obtaining r2 from generalized linear mixed-effects models. Methods Ecol. Evol. 4, 133–142. doi: 10.1111/j.2041-210x.2012.00261.x
Nguyen, L. N., Aditya, L., Vu, H. P., Johir, A. H., Bennar, L., Ralph, P., et al. (2022). Nutrient removal by algae-based wastewater treatment. Curr. Pollut. Rep. 8, 369–383. doi: 10.1007/s40726-022-00230-x
Novoveská, L., Zapata, A. K., Zabolotney, J. B., Atwood, M. C., and Sundstrom, E. R. (2016). Optimizing microalgae cultivation and wastewater treatment in large-scale offshore photobioreactors. Algal Res. 18, 86–94. doi: 10.1016/j.algal.2016.05.033
Ptacnik, R., Solimini, A. G., Andersen, T., Tamminen, T., Brettum, P., Lepistö, L., et al. (2008). Diversity predicts stability and resource use efficiency in natural phytoplankton communities. Proc. Natl. Acad. Sci. 105, 5134–5138. doi: 10.1073/pnas.0708328105
Rasheed, R., Saadaoui, I., Bounnit, T., Cherif, M., Al Ghazal, G., and Al Jabri, H. (2020). Sustainable food production and nutraceutical applications from Qatar desert chlorella sp. (chlorophyceae). Animals 10:81413. doi: 10.3390/ani10081413
Reich, P. B., Tilman, D., Isbell, F., Mueller, K., Hobbie, S. E., and Flynn, D. F. (2012). Impacts of biodiversity loss escalate through time as redundancy fades. Science 336, 589–592. doi: 10.1126/science.1217909
Shah, M., Hashmi, H. N., Ali, A., and Ghumman, A. R. (2014). Performance assessment of aquatic macrophytes for treatment of municipal wastewater. J. Environ. Health Sci. Eng. 12, 1–12. doi: 10.1186/2052-336X-12-106
Sharma, N. K., and Rai, A. K. (2011). Biodiversity and biogeography of microalgae: Progress and pitfalls. Environ. Rev. 19, 1–15. doi: 10.1139/a10-020
Singh, U. B., and Ahluwalia, A. (2013). Microalgae: a promising tool for carbon sequestration. Mitig. Adapt. Strateg. Glob. Chang. 18, 73–95. doi: 10.1007/s11027-012-9393-3
Smart, M. M., Reid, F. A., and Jones, J. R. (1981). A comparison of a persulfate digestion and the kjeldahl procedure for determination of total nitrogen in freshwater samples. Water Res. 15, 919–921. doi: 10.1016/0043-1354(81)90148-2
Tilman, D. (1999). The ecological consequences of changes in biodiversity: a search for general principles. Ecology 80, 1455–1474. doi: 10.2307/176540
Tilman, D., Isbell, F., and Cowles, J. M. (2014). Biodiversity and ecosystem functioning. Annu. Rev. Ecol. Evol. Syst. 45, 471–493. doi: 10.1146/annurev-ecolsys-120213-091917
Tilman, D., Lehman, C. L., and Thomson, K. T. (1997). Plant diversity and ecosystem productivity: theoretical considerations. Proc. Natl. Acad. Sci. 94, 1857–1861. doi: 10.2307/41550
Tilman, D., Reich, P. B., and Isbell, F. (2012). Biodiversity impacts ecosystem productivity as much as resources, disturbance, or herbivory. Proc. Natl. Acad. Sci. 109, 10394–10397. doi: 10.1073/pnas.1208240109
van der Plas, F. (2019). Biodiversity and ecosystem functioning in naturally assembled communities. Biol. Rev. 94, 1220–1245. doi: 10.1111/brv.12499
Wang, B., Li, Y., Wu, N., and Lan, C. Q. (2008). CO2 bio-mitigation using microalgae. Appl. Microbiol. Biotechnol. 79, 707–718. doi: 10.1007/s00253-008-1518-y
Weber, T. S., and Deutsch, C. (2010). Ocean nutrient ratios governed by plankton biogeography. Nature 467, 550–554. doi: 10.1038/nature09403
Ye, L., Chang, C. W., Matsuzaki, S. J. S., Takamura, N., Widdicombe, C. E., and Hsieh, C. H. (2019). Functional diversity promotes phytoplankton resource use efficiency. J. Ecol. 107, 2353–2363. doi: 10.1111/1365-2745.13192
Zeng, X., Guo, X., Su, G., Danquah, M. K., Zhang, S., Lu, Y., et al. (2015). Bioprocess considerations for microalgal-based wastewater treatment and biomass production. Renew. Sust. Energ. Rev. 42, 1385–1392. doi: 10.1016/j.rser.2014.11.033
Keywords: diversity-ecosystem functioning, microalgal microcosms, species richness, species composition, nitrogen removal efficiency
Citation: Zhou S, Li W and He S (2023) Microalgal diversity enhances water purification efficiency in experimental microcosms. Front. Ecol. Evol. 11:1125743. doi: 10.3389/fevo.2023.1125743
Edited by:
Eduardo Jacob-Lopes, Federal University of Santa Maria, BrazilReviewed by:
Tan Lu, Institute of Hydrobiology (CAS), ChinaFernanda Carneiro, State University of Goiás, Brazil
Copyright © 2023 Zhou, Li and He. This is an open-access article distributed under the terms of the Creative Commons Attribution License (CC BY). The use, distribution or reproduction in other forums is permitted, provided the original author(s) and the copyright owner(s) are credited and that the original publication in this journal is cited, in accordance with accepted academic practice. No use, distribution or reproduction is permitted which does not comply with these terms.
*Correspondence: Wei Li, ww0592@gmail.com