- 1School of Life and Environmental Sciences, University of Sydney, Camperdown, NSW, Australia
- 2Health and Biosecurity, CSIRO, Acton, ACT, Australia
- 3Land and Water, CSIRO, Berrimah, NT, Australia
Roaming domestic cats (Felis catus) are recognised as a threat to wildlife globally. Yet management of pet cats in urbanised areas is not regularly mandated, and management of feral cats in urbanised areas is rarely implemented. Mounting evidence emphasises the value of urban environments as hot spots of wildlife activity, which as the human population continues to grow may become the best or only habitats available to some wildlife species. Wildlife in urban environments must navigate introduced stressors that can compound with natural stressors. Additional, often novel, predators such as free-roaming pet and feral cats that are prevalent in urban environments could have high nonconsumptive fear/stress impacts on urban wildlife that influence their activity and adversely affect their health and reproduction capabilities, possibly more so than direct predation effects do. Cat roaming activity, particularly that of pet cats, could be managed with the support of the community, though motivation needs to be ensured. Understanding if roaming cat activity influences urban wildlife activity via perceived fear/stress impacts will help to build community motivation for the need for domestic cat management in urbanised areas. Using infrared motion sensor cameras positioned in both yards and green space edge habitats, we observed whether the presence and times active of native and introduced small mammals, and native birds, were impacted by domestic cat activity within a 24-h period and by their activity in the prior-24-h period. We found evidence of cat roaming activity during the hours of most wildlife activity, and show that wildlife navigated “landscapes of fear” relative to cat activity, as wildlife observed across a 24-h period increased their activity in the absence of cats in the same 24-h period and in the previous 24-h period. We also tested if cat activity was relative to previous cat activity, or disturbances, and found that cats reduced activity in response to each, but were still consistently present. Our results provide justification for the need to increase management of domestic cats in urbanised areas and offer fear/stress impacts as a novel approach to engender community support of such management.
Introduction
Roaming domestic cats (Felis catus) are recognised as a threat to wildlife globally (Trouwborst et al., 2020). They inhabit all continents but Antarctica, persist on numerous remote islands (Courchamp et al., 2003), and are one of the most popular domestic pets in many countries (Crowley et al., 2020). In many situations domestic cats are estimated to occur in much higher numbers than all species of wild cats combined (Hunter, 2015). As a relatively fecund species able to persist under varied climate, habitat, and resource conditions (Lowe et al., 2000; Legge et al., 2017), and which is also regularly given food subsidies by people (Toukhsati et al., 2007; Davey et al., 2019), the domestic cat has some advantage over native predators and prey. Domestic cats (hereafter “cats”) impact wildlife via predation, fear effects, competition, and as a vector of diseases and parasites (Beckerman et al., 2007; Dubey and Jones, 2008; Medina et al., 2011; Doherty et al., 2016). Some attention is being directed at mitigating feral cat populations in natural environments (Dorph and Ballard, 2022), yet management of pet cats in urbanised areas is largely suggestive and not regularly mandated (Legge et al., 2020a,b), with management of feral cats in urbanised areas being implemented even more rarely (Dorph and Ballard, 2022). This situation is problematic, as mounting evidence emphasises the value of urban environments as hot spots of wildlife activity, globally (Cincotta et al., 2000; Seto et al., 2012; Ives et al., 2016; Wintle et al., 2019).
As the human population continues to grow, urban habitats may become the best or only habitats available to some wildlife species (Hobbs et al., 2009). Wildlife species in natural habitats are facing increased threats, many of which are difficult to mitigate (Sattar et al., 2021). However, mitigation of urban-specific threats to “urban exploiter” wildlife – that is those species that make full use of urban resources (McKinney, 2006), may well be more achievable if suitable habitat is available. The conservation of extant wildlife across green space habitats that serve as rich islands between human developments (Dearborn and Kark, 2010; Gaston et al., 2013), is therefore, of growing importance (Soanes and Lentini, 2019). Native wildlife that persists in urban environments (e.g., Łopucki and Kitowski, 2017; Rebolo-Ifrán et al., 2017; Maclagan et al., 2018; Singh et al., 2021) must navigate the attendant introduced stressors that can compound with natural stressors, to receive the rewards from living in proximity to high human activity (Fardell et al., 2020). These stressors include the presence of non-native flora and fauna (Vitousek et al., 1997; Gaertner et al., 2017), and disturbances to biogeochemical cycles, hydrology, soil composition, and climate (Grimm et al., 2008; Kowarik, 2011). The rewards are opportunities to gain food, water, and shelter (Pickett et al., 2001; Gaston et al., 2005; Hobbs et al., 2009). Should the stressors outweigh the rewards then there can be fatal impacts (Fardell et al., 2020). Free-roaming pet and feral cats that are prevalent in urban environments (Legge et al., 2017, 2020a), could exacerbate stress impacts on urban wildlife through influencing their activity and adversely affecting their health and reproduction capabilities (Preisser et al., 2005), as well as the more obvious direct predation impacts (Murphy et al., 2019).
Fear or stress can have a greater effect on populations than direct predation (Preisser et al., 2005). Nonconsumptive effects of cats reduce reproduction in birds, and thus abundance, by 95% compared to only a 1% impact from predation (Beckerman et al., 2007). The visual cue of a taxidermied cat reduced parental care by blackbirds owing to increased alarm calls and agitation behaviours, which resulted in higher rates of nest predation by other predators (Bonnington et al., 2013). Use by birds of avian feeders in urban environments has also been observed to be reduced when cats are present (Tryjanowski et al., 2015; Freeberg et al., 2016). Adopting an effective coping response to a stressor is beneficial to the fitness of stressed animals (McEwen and Wingfield, 2003), with behaviours such as increased detection of approaching predators/stressors, increased escape responses, reduced interaction with threats, or self-soothing, all reducing the stress response (Lima and Dill, 1990; Brown, 1999; Koolhaas et al., 1999; Herberholz and Marquart, 2012; Estanislau et al., 2019). In most predator–prey systems, the species share a coevolutionary history that allows both predator and prey to coexist (Marrow et al., 1992; Abrams, 2000). If interactions between predator and prey are altered or novel, such as in urban ecosystems, there can be deleterious impacts to the prey species (Bridges, 1999; Carthey and Blumstein, 2018), that can cascade through the ecosystem (Brown et al., 2015), at least for an initial period. With time, repeat encounters with a stressor, such as a novel predator, can incite an adaptive response (West et al., 2018; Bytheway and Banks, 2019; Tay et al., 2021). However, when predation pressures are strong and persistent, as with numerous different predators in a novel system, they can deplete prey species’ energy budgets for reproduction and health management either via energy exhaustion from repeat stress (i.e., the predation stress hypothesis: Moberg, 1991; Boonstra et al., 1998; Clinchy et al., 2004; Romero, 2004), or via reduced nutrition from foraging compromises (i.e., the predator-sensitive food hypothesis: Sinclair and Arcese, 1995; Brown and Kotler, 2004).
In “landscapes of fear,” where wildlife navigate their landscape according to high and low perceived predation threats (Laundré et al., 2001, 2010, 2014), a prey species’ use of the topography, refuges, and escape substrates can indicate its perceived predation risk (Brown and Kotler, 2004; Shrader et al., 2008; van der Merwe and Brown, 2008). The landscapes of fear model (Laundré et al., 2001, 2010) has been assessed extensively to provide a plausible explanation for shifts in population dynamics (Laundré et al., 2014). This confirms the need to understand the direct and indirect impacts of fear imposed on prey by predators, in order to gain a deeper insight into the factors that shape and maintain ecological network stability. The concept implies an understanding of the responses of prey species to potential predator cues, like olfactory stimuli, as well as their presence and direct impacts (Laundré et al., 2001; Takahashi, 2014). Olfactory information can be used to evaluate predation risk, as predators and prey both secrete body odours (Davis et al., 2013) that reflect their dietary activity and metabolism (Ferkin et al., 1997). These odours may also provide clues to animal health, sex, age and behaviour via glandular secretions, such as steroid hormones that may indicate stress (Zamaratskaia and Squires, 2009). It follows then that the presence or absence of olfactory cues can indirectly affect other species within food webs and, as individuals receive messages intended for them or eavesdrop on each other (Banks et al., 2016; Jones et al., 2016), a landscape of fear may be created. This could arise from recent (over the same 24-h period) or prior (within previous 24-h periods) predator activity in the area.
Olfaction drives the “fear” response in many landscapes (Soso et al., 2014; Banks et al., 2016; Jones et al., 2016; Parsons et al., 2017). Prey responses to predator olfactory cues from their prior activity in the area vary from no response or mild inhibitory/repellent behaviours (e.g., Herman and Valone, 2000; Orrock and Danielson, 2009) to strong avoidance and modified activity patterns and habitat use (e.g., Fenn and Macdonald, 1995; Shrader et al., 2008; Willems and Hill, 2009; Parsons and Blumstein, 2010; Anson and Dickman, 2013), which could negatively impact their fitness (Loss and Marra, 2017). Indeed, cat olfactory cues have been observed to incite modified foraging behaviour responses by small mammals in disturbed habitats (Apfelbach et al., 2005), which could stem from diurnal or nocturnal cat roaming. Cat roaming activity, particularly of cats that are pets, could be managed with the support of the community, although motivation needs to be ensured (Legge et al., 2020a; van Eeden et al., 2021). Understanding whether roaming cat activity does influence urban wildlife activity via perceived landscapes of fear would contribute to the growing knowledge base of cat impacts on urban wildlife. This poorly understood interaction could better help to build motivation for the need for cat management in urbanised areas.
The compounding additional predator pressures in urban ecosystems (Fardell et al., 2020, 2021b, 2022a), from pet cats and dogs (Young et al., 2011; Legge et al., 2020a), wild predators attracted to the supplementary resources that people provide (Newsome et al., 2015; Reshamwala et al., 2018), and the predator-like stress that human activity can cause (Frid and Dill, 2002; Rehnus et al., 2014; Patten and Burger, 2018), are likely to create strong and persistent predator pressure that could cause nonconsumptive fear or stress effects, including landscapes of fear. Management of pet cats is often stymied by a lack of evidence of direct predation and whether this impacts wildlife populations. Offering evidence for the negative nonconsumptive effects of roaming cats could support the need for more consideration in management to be given to the fear and stress impacts that cat presence alone can have on wildlife populations. We, therefore, investigated the fear/stress impacts on wildlife imposed by cat roaming activity, at all hours, in a patchy urban environment in Australia, where pet and feral cats are prevalent across all areas (Legge et al., 2017, 2020b), to determine if landscapes of fear were evident. To do so, we used infrared motion sensor cameras to observe rates and times of activity of native and introduced wildlife, compared to roaming cat activity within a 24-h period in both yard and green space edge habitats. To further test the fear/stress responses of wildlife we also examined their rates and times of activity compared to cat activity within the previous 24-h period. To understand how roaming cat activity may be influenced we also examined cat rates and times of activity relative to previous cat activity and to disturbance events from people, dogs, horses, and chickens. We predicted that wildlife would show some evidence for a landscape of fear response to the novel predator and would reduce their activity in local areas relative to cat presence both within the same 24-h period and within the previous 24-h period. We also predicted that responses would be similar across different (yard and green space) habitats.
Methods
Study area
As described and depicted in Fardell et al. (2021a,b, 2022a,b), this research was conducted in the connected suburban areas of Whitebridge and Dudley, in the Lake Macquarie district of New South Wales, Australia. The combined area is a patchy urban environment, interspersed with many green spaces and corridors, most of which contain remnant vegetation that pre-dates European disturbance [Department of Environment, Climate Change and Water (DECCW), 2010; Bell, 2016] and eventually links up to the larger Glenrock State Conservation Area (GSCA) or Awabakal Nature Reserve. This area is biodiverse [Department of Environment, Climate Change and Water (DECCW), 2010], with wildlife frequently active near to houses/human activity (Fardell et al., 2021b, 2022a,b). At the time of research, the only legislative requirement for pet cat ownership in this area was that cats be registered; no containment or time restrictions on roaming were in place. Similarly, no feral cat control occurred in the area.
Survey methods
The monitoring of wildlife was conducted under animal ethics approval from the University of Sydney (2017/1275), and a New South Wales Scientific License (SL102024). Data were collected for wildlife responses to cats in yard habitats as published in Fardell et al. (2022b), and for green space edge habitats as published in (Fardell et al. 2021b, 2022a), using cameras to assess wildlife/cat/disturbance presence across time. In brief, within the yards of 21 volunteer households, four motion-sensor infrared Reconyx Hyperfire PC800 cameras were set to take a quick-burst of 10 images continually across 24-h periods for a minimum of three consecutive days and nights, between May and June 2019 (Fardell et al., 2022b). Cameras were positioned ~20 cm from the ground at a 10° downward angle, targeting small–medium-sized terrestrial wildlife (Fleming et al., 2014), with two cameras in more “open” areas of yards and the remaining two positioned in more “vegetated” or covered areas of yards (Fardell et al., 2022b). Each camera had a scent lure of fish-oil-soaked sponges in sealed PVC pipes with air holes that were secured ~1.5–2 m in front of them on the ground, and a bait of mixed peanut butter, honey, and oats scattered in this area. Volunteer households that owned pets were encouraged to let their pets move around the property as they would normally, despite the cameras.
Within the area between the volunteer yards a “green space edge” habitat was also surveyed. The green space consisted of a patch of largely remnant dry sclerophyll forest that surrounded a heavily used grass sports oval; the outer edge of the vegetated patch was surrounded by a wide grass fire trail/corridor that connected to the larger GSCA (via a road) and bordering this were houses, all intersected by walking/cycle tracks (see Figure 1 of Fardell et al., 2021b and associated description). Six motion-sensor infrared Reconyx Hyperfire PC800 cameras were set in this area at the edges of the forest on human-made tracks or narrower animal runs, covering an area of ~20,717 m2 within a ~ 660 m perimeter, spaced ~60–80 m apart dependent upon habitat suitability. Cameras were fixed to trees ~1 m above ground at a 10° downward angle to capture a wider area (Fardell et al., 2021b, 2022a). Cameras were set to take a quick-burst of 10 images continually across both day and night and had a scent and bait lure of fish oil and a peanut butter-honey-oat mix scattered directly amongst leaf litter on the ground ~2–3 m away (to account for the higher camera position for a wider area), which was reapplied after 3 days (Fardell et al., 2021b, 2022a). Cameras were set for eight consecutive nights, for four repeated survey sessions between January and September of 2019.
To account for the effect that high illumination moon-phase can have on small mammal foraging behaviours (Navarro-Castilla and Barja, 2014), all camera surveys were conducted when the moon was <70% full (Fardell et al., 2021b, 2022a,b). Adverse weather events were also avoided (Fardell et al., 2021b, 2022a,b). For ease of assessment in both datasets (i.e., yard and green space edge), records of native birds were pooled considering the small number of visits per species, commonalities in their times active, habitats used, and means of escape by flight. Introduced rodents—brown and black rats (Rattus norvegicus and R. rattus) and, in the case of the green space edge habitat, house mouse (Mus musculus)—were also grouped together for assessment as there were few observations of the latter two species and all exhibit common urban behaviours (Feng and Himsworth, 2014). All images captured were included in analyses, and combined per species/group to give a total for each 24-h period. In this way the data were an indicator of not only presence, with a zero-result recorded if a species/group was not observed in a 24-h period, but also the amount of activity within the area.
Statistical methods
We ran generalised linear mixed models that allowed for zero inflation and dispersion parameters, using the package glmmTMB (Brooks et al., 2017) in the statistical software R (R Core Team, 2022), to test the effect of cat activity on wildlife and disturbances on cat presence in the yard and edge habitats. This method was selected to account for the high number of true zeros observed in the wildlife/cat activity dataset. Within each model, the first section tests the conditional mean to determine the influence of the predictors on the response, the second section assesses which predictors increase the probability of a zero, and the third section tests for dispersion effects and the balance of them relative to the mean (Brooks et al., 2017). To accommodate these processes, log link functions were used on the first and third sections, and a logit link function on the second section of each model (Brooks et al., 2017). The odds ratio and percentage change were exponentiated for presentation and ease of interpretation.
Models were run to independently test the effects on wildlife from cat presence in (1) the same 24-h period, and (2) the previous 24-h period, using the total number of cat images observed in each period as a fixed effect in (a) yard, and (b) edge habitats. The response in each of these models was the total number of wildlife images captured, modelled as individual species, or grouped orders, per 24-h period. To test the effect of disturbances on cats, models were also run using the total number of disturbances (yards: people, dogs, horses, chickens; and edge: people, dogs) observed in a 24-h period as the fixed effect on the response of the total number of cats observed in the 24-h period in (a) yard, and (b) edge habitats. To test the effect of cat presence on cat presence, models were run using the total number of cat images observed in the previous 24-h period as the fixed effect on the response of the total number of cat images observed in the 24-h period in (a) yard, and (b) edge habitats. Within the yard habitat models, the “open” and “vegetated” camera data were grouped together to represent the typical variation in yard microhabitats. Camera/yard location, survey day, survey session (green space models only), and the total number of animal/disturbance types in a 24-h period (only when needed to balance the model) were each used as random effects to account for repeated measures. The total number of images recorded per 24-h period was used as an offset to relativise the image counts by accounting for the uneven number of images captured each 24-h period. If there were explanatory and/or random variables that showed variance in a response, then they were included in the relative model’s dispersion formula. Alternatively, a single inflation parameter was applied to the combination of all fixed and random effects (i.e., ~1), where it best fitted. Explanatory and/or random variables that showed the highest deviation in a zero-response, or the standard dispersion given the family modelled, were included in the model’s zero inflation formula relative to the best-fit model. Model fit was assessed via Akaike Information Criterion comparisons (Burnham and Anderson, 2004), and successful testing and observations on the residuals, using the package DHARMa (Hartig, 2021).
Overlap coefficients for activity times between each species/group and cats across the yard and green space edge habitats were calculated from the camera data and are presented as the percentage overlap in times active. These comparisons were made and plotted using the R package overlap that uses radians to fit kernel density functions to times of observations of animals (Ridout and Linkie, 2009).
Results
Camera trapping
Across the four cameras in each of the 21 volunteer properties for 3–4 × 24-h survey periods, a total of 12,892 images was captured within the yard habitats. The majority (9,605: 74.5%) of these images were of wildlife, being: 5,467 (42.4%) images of common brushtail possums (Trichosurus vulpecula), 1,722 (13.4%) images of combined introduced rodents, 1,029 (8%) images of northern brown bandicoots (Isoodon macrourus), 985 (7.6%) images of brown antechinuses (Antechinus stuartii), 400 (3.1%) images of combined native birds (see species below), and 2 (0.01%) images of red foxes (Vulpes vulpes). Disturbances were also prevalent in the images (2,855: 22.1%), including: 2,077 (16.1%) images of dogs (Canis familiaris), 534 (4.1%) images of people (Homo sapiens), 232 (1.8%) images of chickens (Gallus domesticus), and 12 (0.09%) images of horses (Equus caballus). Cats were also present in the yard images, totalling 432 (3.4%) images. Seven individual cats were observed, half of which visited the cameras twice at a single property, but none of these were observed to visit multiple close-by properties. One cat was observed wearing a collar.
Across the six cameras positioned along the edge of the green space remnant habitat for 4 survey sessions of 8 nights each, a total of 34,419 images was captured within the green space edge habitats. The majority (30,861: 89.6%) of these images were of wildlife, being: 11,111 (32.3%) images of introduced rodents, 11,075 (32.2%) images of northern brown bandicoots, 7,652 (22.2%) images of common brushtail possums, 631 (1.8%) images of red foxes, 283 (0.9%) images of combined native birds, 60 (0.2%) images of brown antechinuses, and 49 (0.1%) images of common ringtail possums (Pseudocheirus peregrinus). Disturbances were also prevalent in the images (2,365: 6.9%), including: 1,735 (5%) images of dogs, and 630 (1.8%) images of people. Cats were also ubiquitous in the green space edge images, totalling 1,193 (3.5%) images. Six individual cats were observed, all of which were captured on multiple separate events that totalled 58, which comprised of: 16 for cat #1, 13 for cat #2, 2 for cat #3, 16 for cat #4, 8 for cat #5, and 3 for cat #6. One cat was observed with a collar. One successful predation event was caught on camera with cat #1 leaving the dense vegetation with a small mammal in its mouth, though only the long tail and foot were visible. Stalking by cat #1 on a young northern brown bandicoot was also observed at the edge of the dense vegetation and the corridor, although the result occurred off camera after the cat pounced and the bandicoot ran with the cat chasing after it (Figure 1).
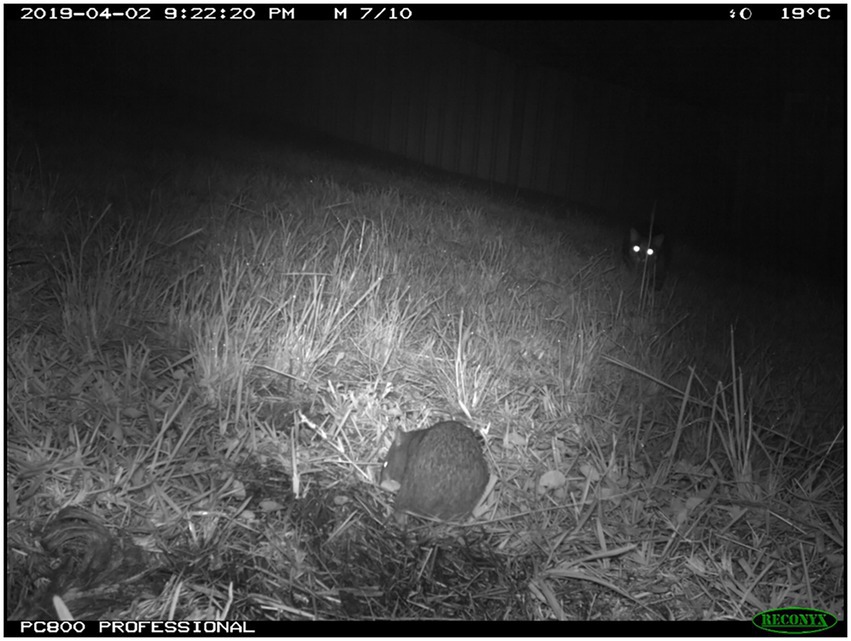
Figure 1. Northern brown bandicoot (Isoodon macrourus) and domestic cat (Felis catus). Photo captured on Reconyx sensor camera by L. Fardell at the green space edge habitat.
Owing to the smaller number of brown antechinuses observed, to enable analyses, these were grouped together with northern brown bandicoot data for each location. Considering that both are native marsupials that would likely be foraging for terrestrial invertebrates when captured on camera, and that each has a unique escape method (spiral arboreal climbing for the brown antechinus, Dickman, 1991; and a loping run for the northern brown bandicoot, Bennett and Garden, 2004), we felt that this approach was justified. Similarly, owing to the small number of common ringtail possums observed at the green space edge habitat only, records were grouped with common brushtail possum data to enable analyses. This was justified as these possums are ecologically similar native arboreal marsupials, capable of the same movement and behaviours. The bird species grouped together for the yard habitats included: laughing kookaburra (Dacelo novaeguineae) – 146 images, Australian magpie (Gymnorhina tibicen) – 125 images, crested pigeon (Ocyphaps lophotes) – 50 images, buff-banded rail (Gallirallus phillippensis) – 41 images, brush turkey (Alectura lathami) – 20 images, and noisy miner (Manorina melanocephala) – 19 images. The bird species grouped together for the green space edge habitats included: Australian magpie – 200 images, satin bower bird (Ptilonorhynchus violaceus) – 47 images, noisy miner – 28 images, and eastern whip bird (Psophodes olivaceus) – 8 images.
Temporal effects of cats on wildlife in yard and green space edge habitats
The times that cats were most active varied between the yard and green space edge habitats (Table 1). Within the yard habitat, cats were only active during six hourly periods, with most activity observed around 10–11 pm, then 7 pm, 4 am, and 10 am, followed by 3 am, and 11 am. Within the green space edge habitat, cats were active during all but 3 h (from 6 to 8 am), with most activity observed during the pre-dawn period of 5 am, followed by 3 am and 8 pm; moderate activity was also evident at midday from 11 am – 2 pm as well as at 6 pm and 9 pm, with low activity in the other hours. Overlaps in times that both wildlife and cats were active varied between the yards and green space edge habitats too (Table 2). Within the yards habitat, the largest overlap was evident between cats and common brushtail possums, then brown antechinuses, northern brown bandicoots, introduced rodents, followed by pet dogs, people, and native birds (Figure 2). Within the green space edge habitat, the largest overlap was evident between cats and introduced rodents, then northern brown bandicoots, brown antechinuses, and common brushtail possums, followed by people, native birds, red foxes, pet dogs, and common ringtail possums (Figure 3). The periods of most overlap, however, were common across the yards and green space edge habitats, being largely pre-dawn and post-dusk for mammals, dawn and middle of the day for native birds, and day to 6–9 pm for pet dogs and people (Figures 2, 3).
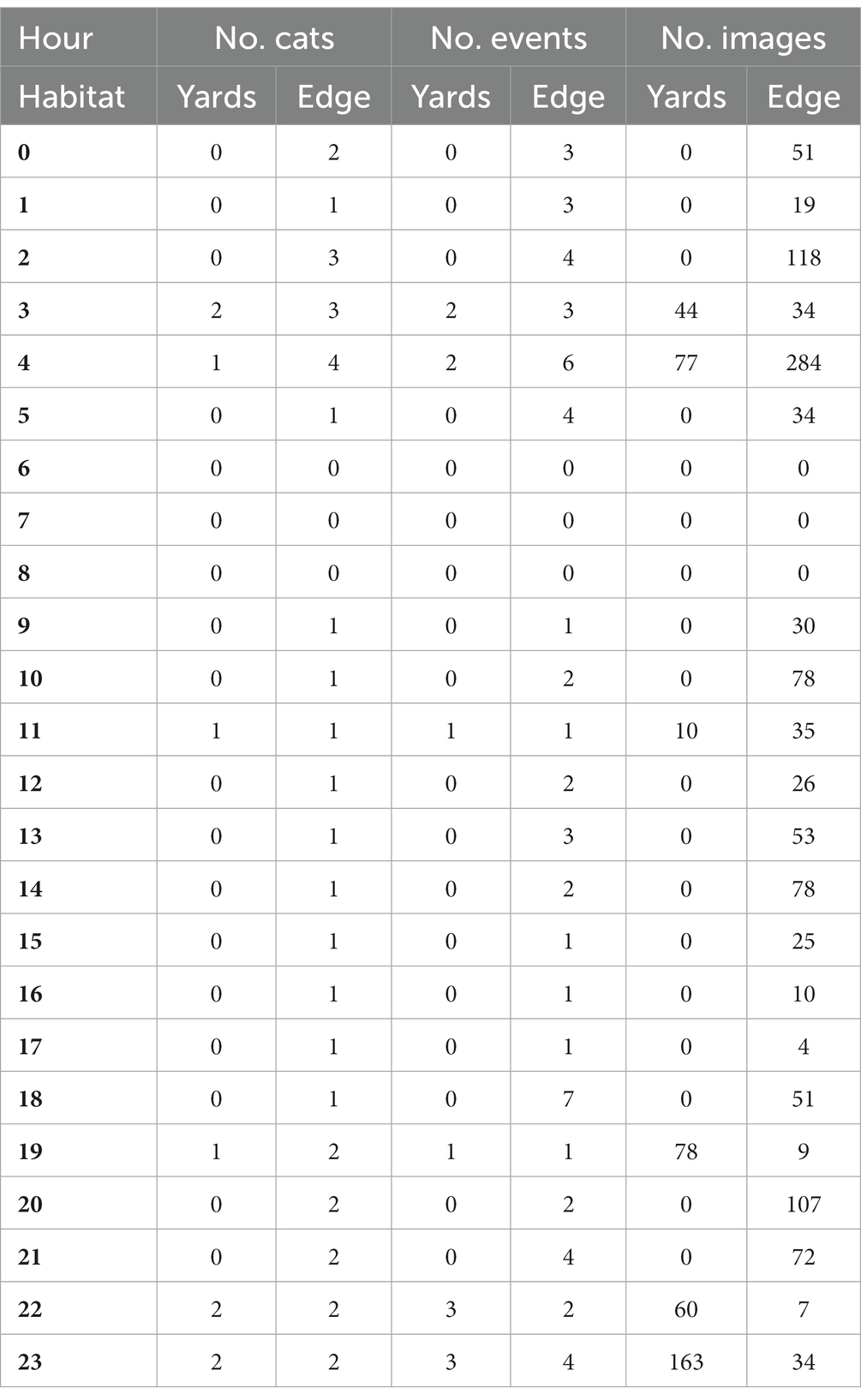
Table 1. The total number of individual domestic cats (No. Cats), events (No. Events) of cat activity – considering consecutive images, and number of images (No. Images) per hour (24 h), in the collective study periods, across yard and green space edge habitats.
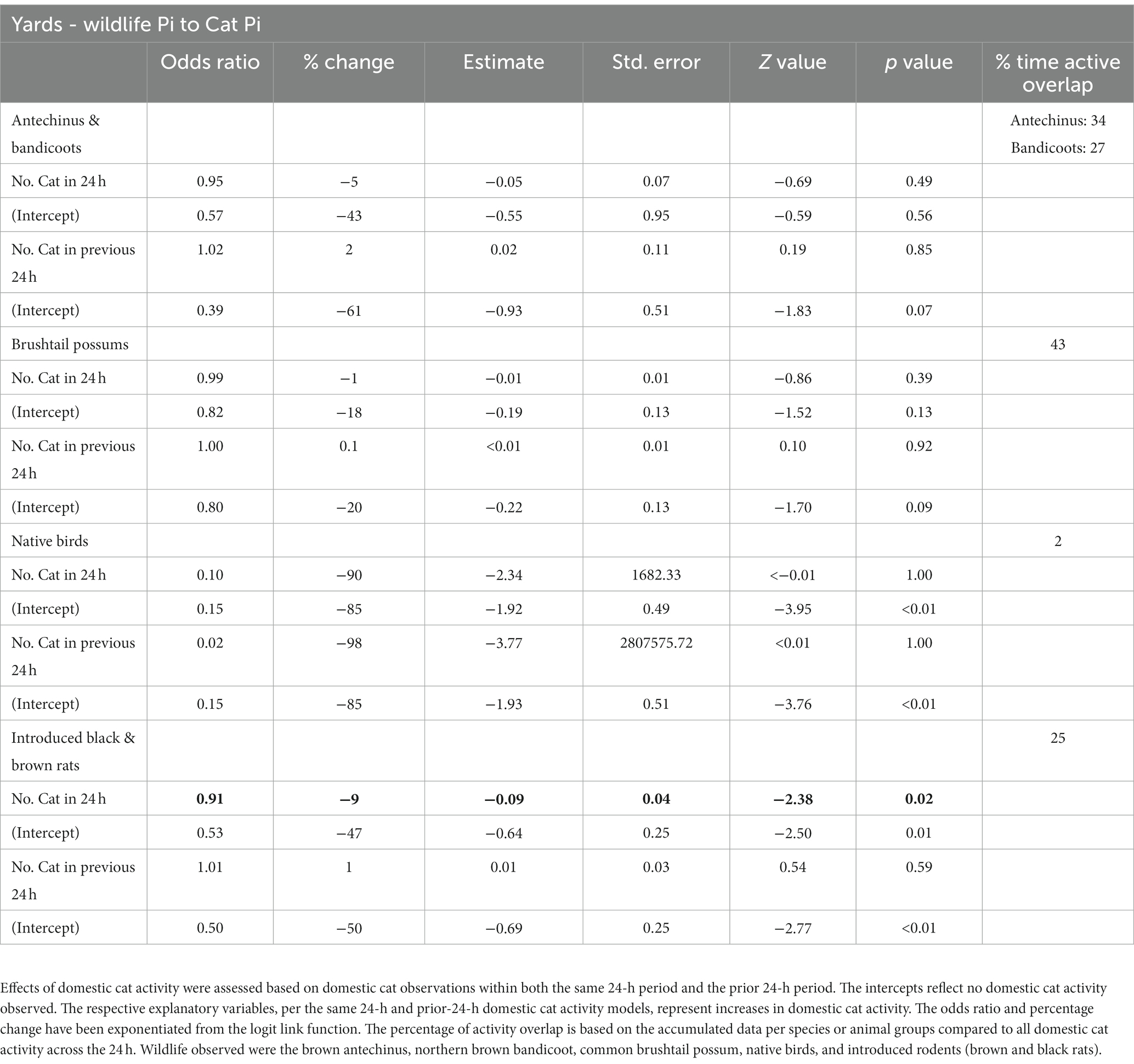
Table 2. Summary of the generalised linear mixed model output testing the effect of domestic cat activity on wildlife activity in the yard habitats of 21 volunteer yards, based on four infrared scent and bait lured cameras in each yard for a duration of three-four consecutive 24-h periods.
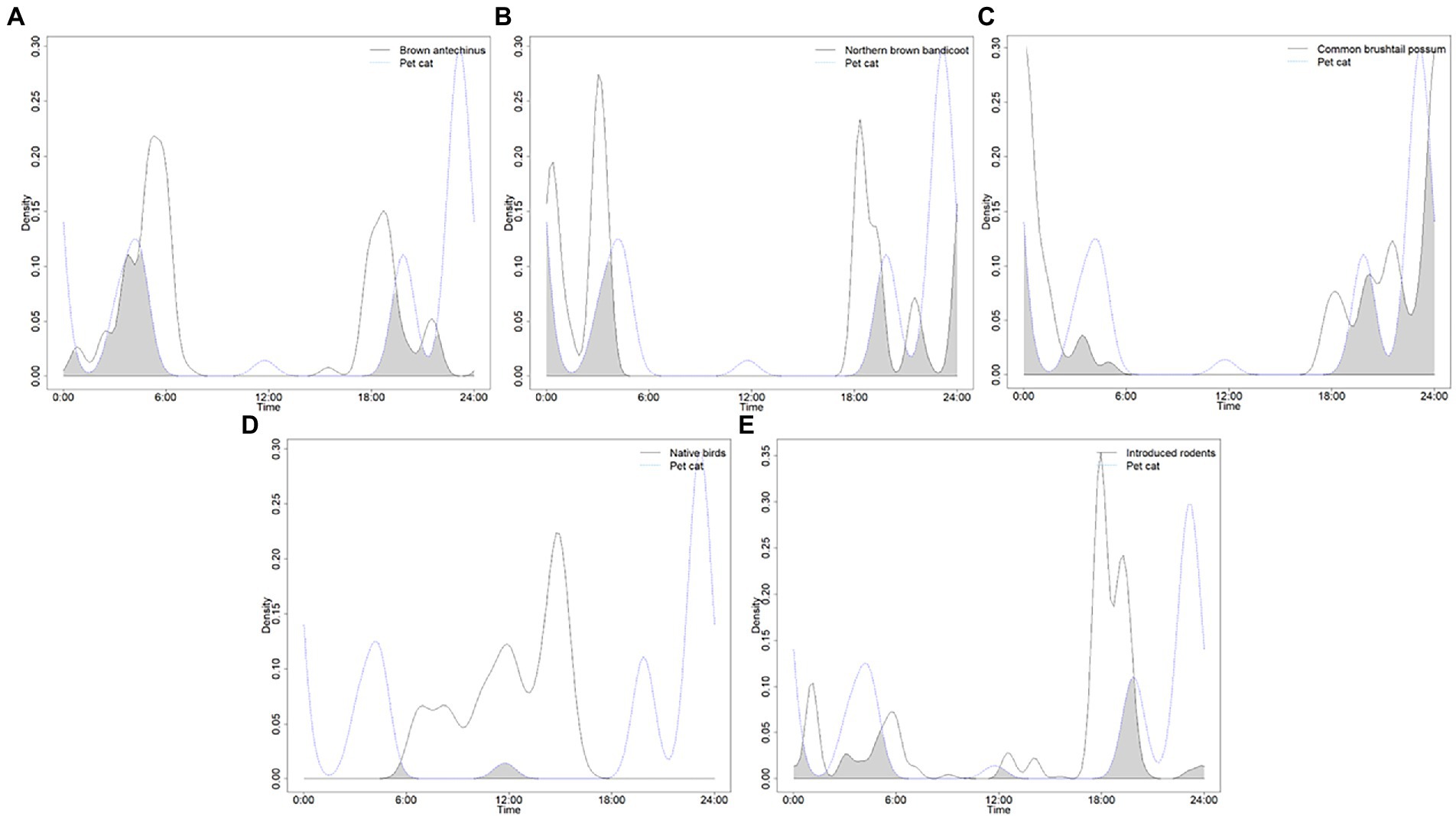
Figure 2. Density pair plots for the overlap coefficients for wildlife hours active compared to the hours that domestic cats were active in the yard habitats for: (A) brown antechinus, (B) northern brown bandicoot, (C) common brushtail possum, (D) native birds, and (E) introduced rodents (brown and black rats). Results recorded by four infrared scent and bait lured cameras positioned in 21 volunteer yards for the duration of three-four consecutive 24-h periods.
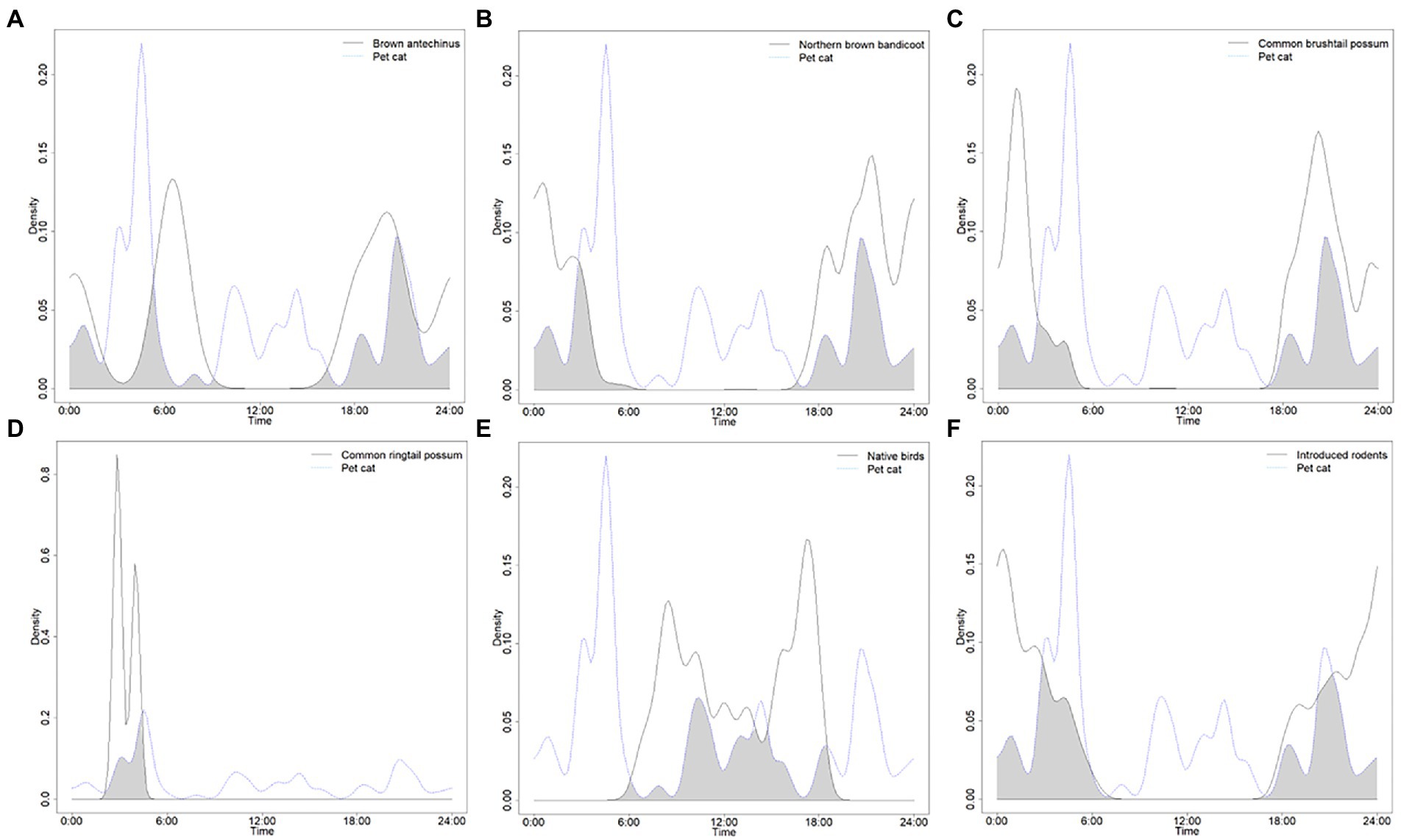
Figure 3. Density pair plots for the overlap coefficients for wildlife hours active compared to the hours that domestic cats were active in the green space edge habitat for: (A) brown antechinus, (B) northern brown bandicoot, (C) common brushtail possum, (D) common ringtail possum, (E) native birds, and (F) introduced rodents (brown and black rats, and house mouse). Results recorded by six infrared scent and bait lured cameras positioned throughout a green space edge habitat for the duration of eight consecutive 24-h periods, for four repeated survey sessions.
Effects of cats on wildlife in yard habitats
Within the yard habitats, wildlife largely responded negatively to the presence of cats compared to their absence within each 24 h-period, with brown antechinuses and northern brown bandicoots, common brushtail possums, native birds, and introduced rodents all increasing when no cats were observed (odds ratios <1, intercept results: Table 2, Figure 4). Inverse responses to no cat observations were most evident in native birds, followed by introduced rodents, then brown antechinuses and northern brown bandicoots, and finally common brushtail possums (intercept results: Table 2). However, the large standard errors associated with native birds suggest that these results are less reliable (Table 2). Increases in cat observations within the 24-h period had a small to minimal negative effect on brown antechinuses and northern brown bandicoots, and common brushtail possums (odds ratios ~1), a small but significant negative effect on introduced rats, and a large negative effect on native birds, but with large error margins (Table 2).
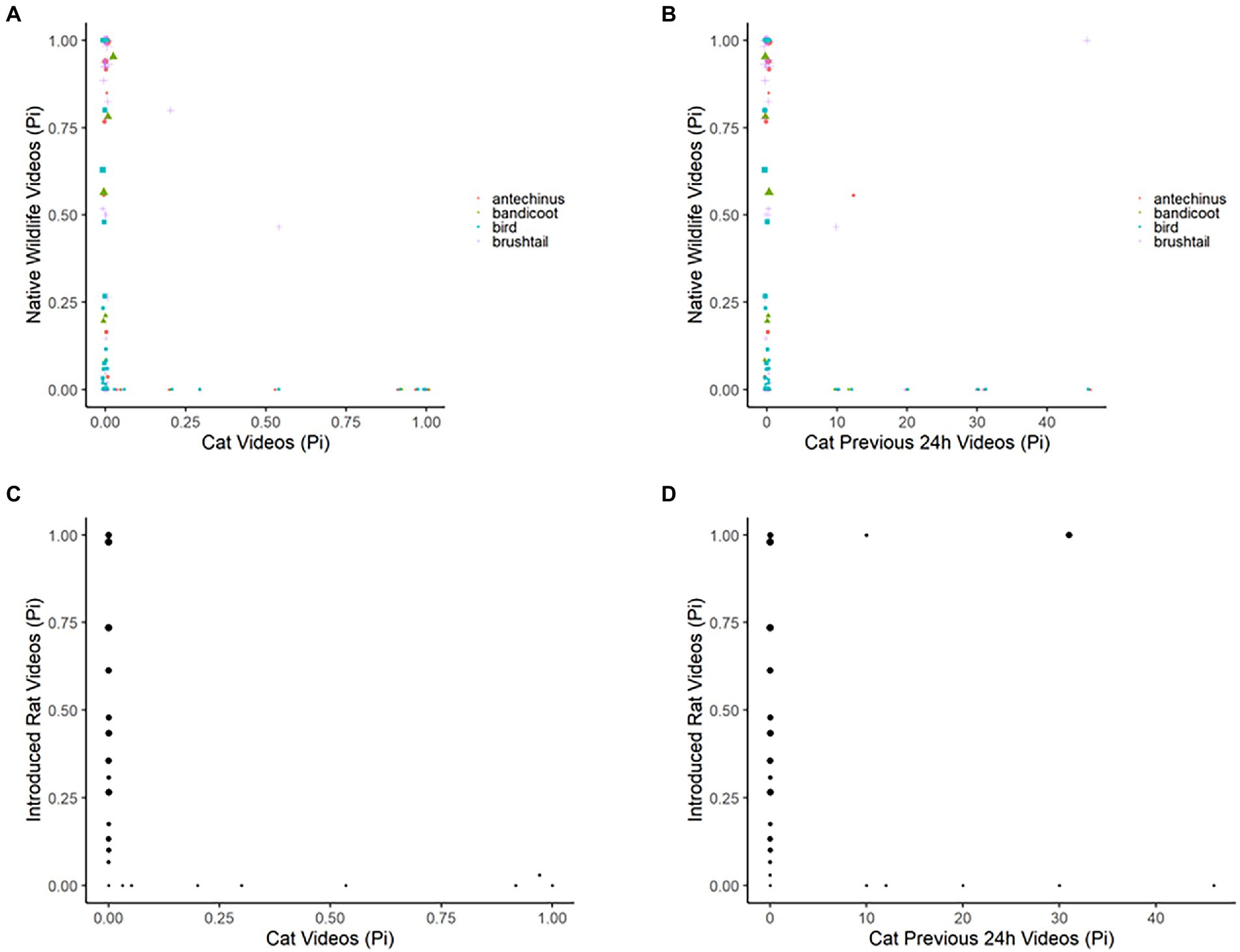
Figure 4. Plots depicting the raw data for the proportion of images observed per 24-h period for each species or animal group compared to the proportion of images that were of domestic cats, for wildlife (brown antechinus, northern brown bandicoot, native birds, and common brushtail possum) and introduced rats (brown and black rats) in the yard habitats; as: (A) wildlife compared to domestic cats across the same 24-h period, (B) wildlife compared to domestic cats across the prior-24-h period, (C) introduced rodents compared to domestic cats across the same 24-h period, and (D) introduced rodents compared to domestic cats across the prior-24-h period. Results recorded by four infrared scent and bait lured cameras positioned in 21 volunteer yards for the duration of three-four consecutive 24-h periods.
Wildlife responses in yard habitats to increases in cat observations within the previous 24-h period had zero to minimal positive effect on all mammals (odds ratios ~1), but observations of native birds reduced even more than if a cat was observed within the same 24-h period (Table 2). When no cats had been observed in the previous 24-h period, wildlife observations increased with the largest ratio of change evident in native birds, followed by brown antechinuses and northern brown bandicoots, then introduced rats, and finally common brushtail possums (intercept results: Table 2, Figure 4). However, each result for native birds is less reliable considering the associated large error margins (Table 2).
Cat responses to increases in disturbance observations of people, dogs, horses, or chickens, showed nil to very minimal negative effects (odds ratios ~1). However, when no disturbances were observed then cat observations increased (Table 3, Figure 5). Cat observations in response to cat observations in the previous 24-h period showed nil to minimal positive effect (odds ratios ~1), but if there had been no cats observed in the prior period then observations of cats increased (odds ratios <1; Table 3; Figure 5).
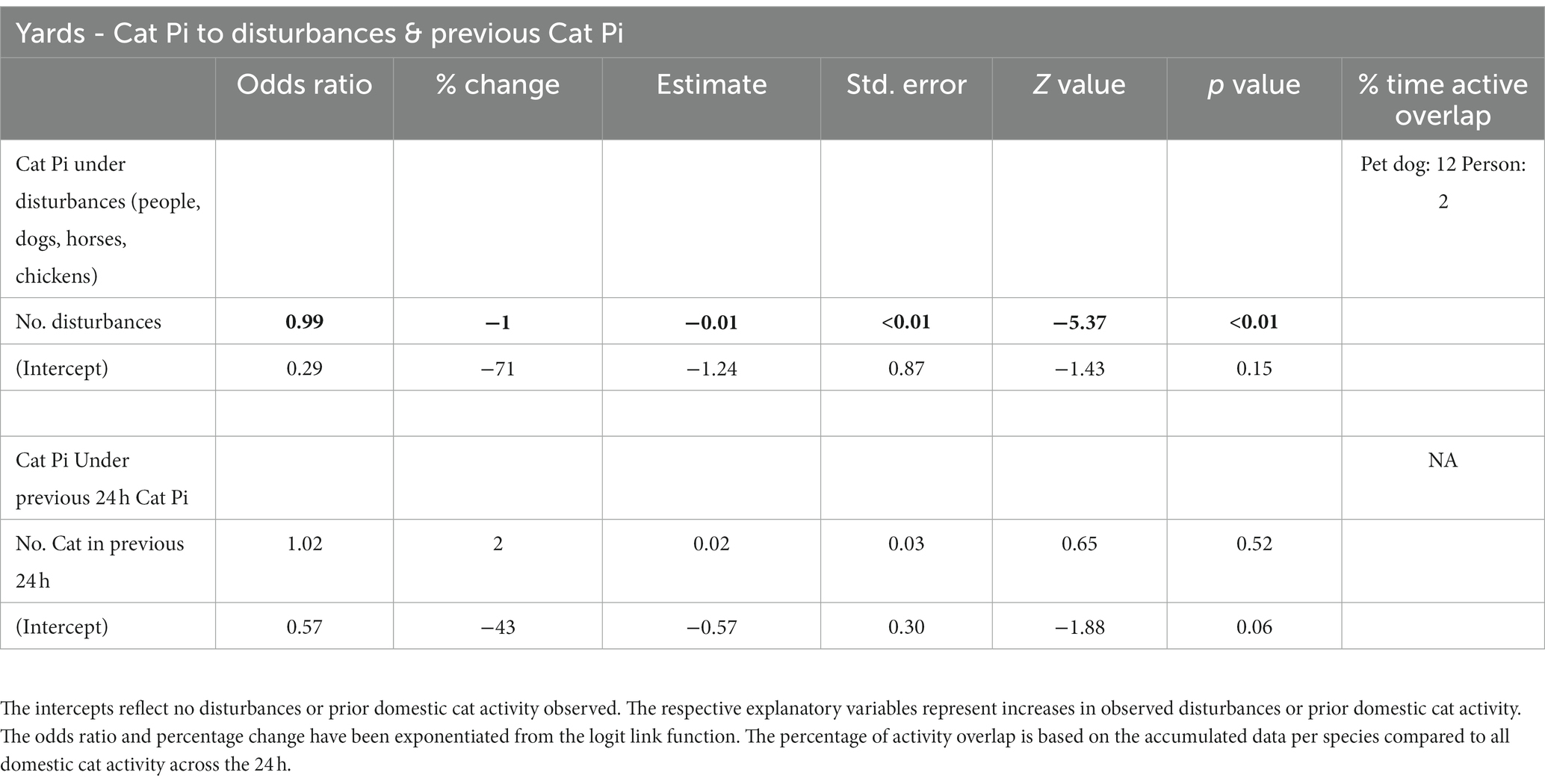
Table 3. Summary of the generalised linear mixed model output testing the effect of disturbances (people, dogs, horses, chickens) and prior domestic cat activity on domestic cat activity in the yard habitat of 21 volunteer yards, based on four infrared scent and bait lured cameras in each yard for a duration of three-four consecutive 24-h periods.
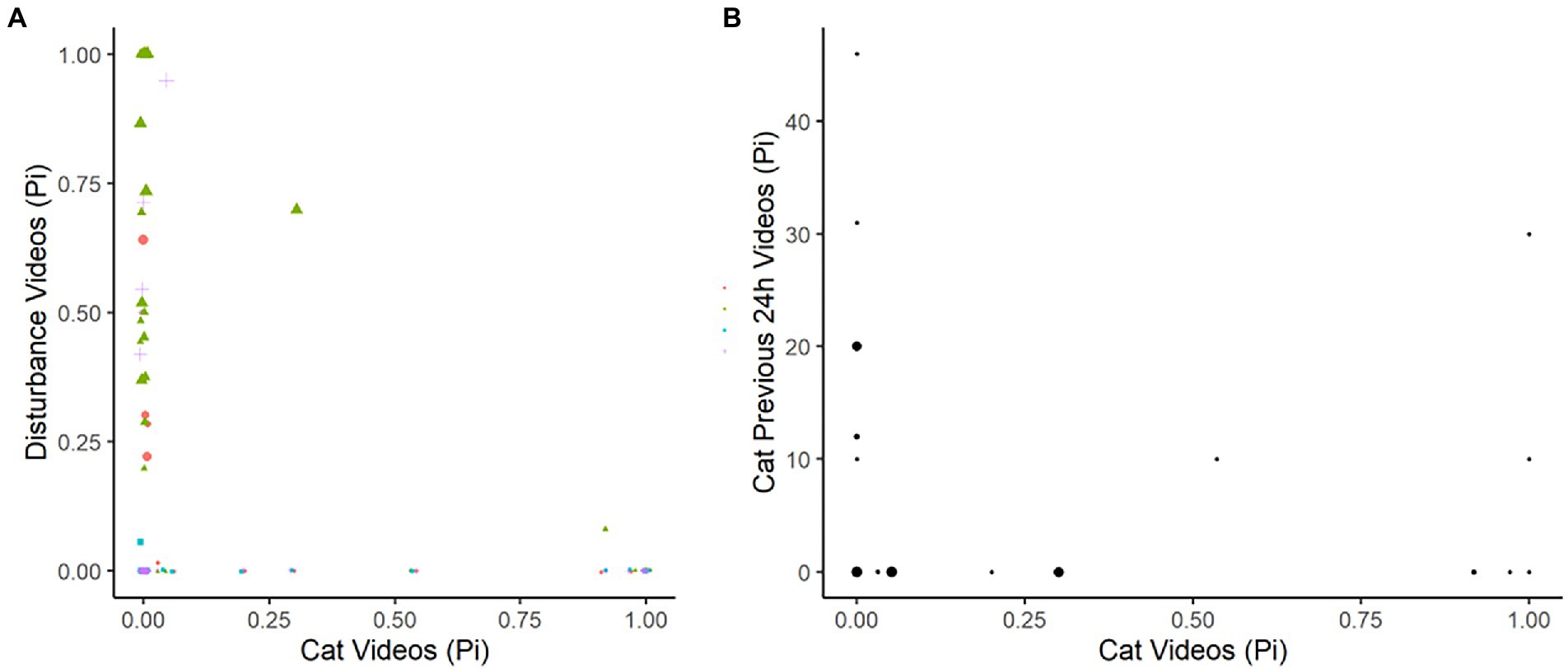
Figure 5. Plots depicting the raw data for the proportion of images observed per 24-h period for domestic cats in the yard habitats, compared to (A) the proportion of images that were disturbances (chickens, dogs, horses, people), and (B) the proportion of images that were of prior-24-h domestic cat observations. Results recorded by four infrared scent and bait lured cameras positioned in 21 volunteer yards for the duration of three-four consecutive 24-h periods.
Factors affecting data dispersion and frequency of zero wildlife observations in yard habitats
For all yard habitat models, except those for common brushtail possums and cat observations relative to previous cat observations, the probability of producing a structural zero, that is, not generated by the conditional model, was equal for all fixed and random effects modelled (i.e., ziformula = ~1, in the best fit models; Brooks et al., 2017). Positive zero-inflation estimates suggest a high chance of a zero-result given an increase in all factors modelled together (Brooks et al., 2017), which was significant for each model (Supplementary material; Table 1). For common brushtail possums’ responses to cats in the 24-h period model, both the day and number of animals observed in a day were used as zero inflation parameters in the best fit models (Supplementary material; Table 1). The effect of all days and number of animals in a day, except for the number of animals on the final survey day, showed a reduced probability of a zero-result compared to day 1 and 1 animal, and the number of animals on the final day showed an increased probability of a zero-result compared to day 1 and 1 animal, although they were not significant (Supplementary material; Table 1). The same result was evident in the model for common brushtail possums’ responses to cats in the previous 24 h. However, the number of cats observed the previous day was also used as a zero-inflation parameter in the best fit model, with an increased probability of a zero result as this measure increased, which was not significant (Supplementary material; Table 1). For the cats’ responses to observations of cats in the previous 24 h model, both the day and number of cats observed in the previous 24 h were used as zero inflation parameters in the best fit models (Supplementary material; Table 1). Each day increased the probability of a zero-result compared to day 1, and as the number of cats observed in the previous 24 h increased, it decreased the probability of a zero result, though none was significant (Supplementary material; Table 1). For all the best-fit yard habitat models, the dispersion parameter was set as the standard dispersion variance given the negative binomial 2 family distribution (Brooks et al., 2017).
Effects of cats on wildlife in green space edge habitats
Within the green space edge habitats, wildlife responses to increases in cat observations within the 24-h period had no to minimal negative effect (odds ratios ~1, Table 4). However, when there had been no cats observed in the 24-h period, wildlife observations increased, with the largest ratio of inverse change evident in red foxes, native birds, then brown antechinuses and northern brown bandicoots, common brushtail and ringtail possums, and finally, introduced rodents (odds ratios <1, intercept results: Table 4; Figure 6).
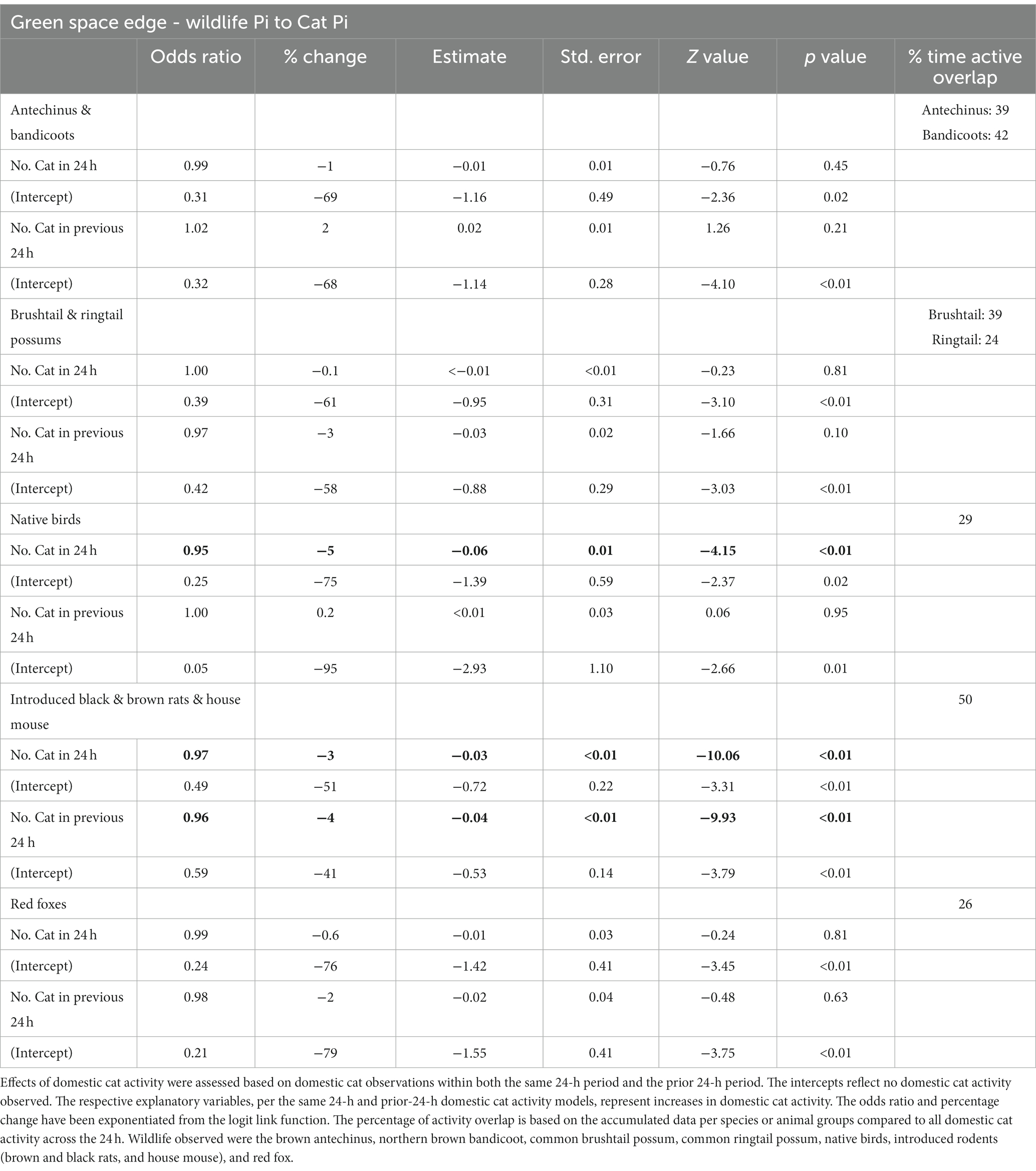
Table 4. Summary of the generalised linear mixed model output testing the effect of domestic cat activity on wildlife activity in the green space edge habitat, based on six infrared scent and bait lured cameras positioned throughout the habitat for the duration of eight consecutive 24-h periods, for four repeated survey sessions.
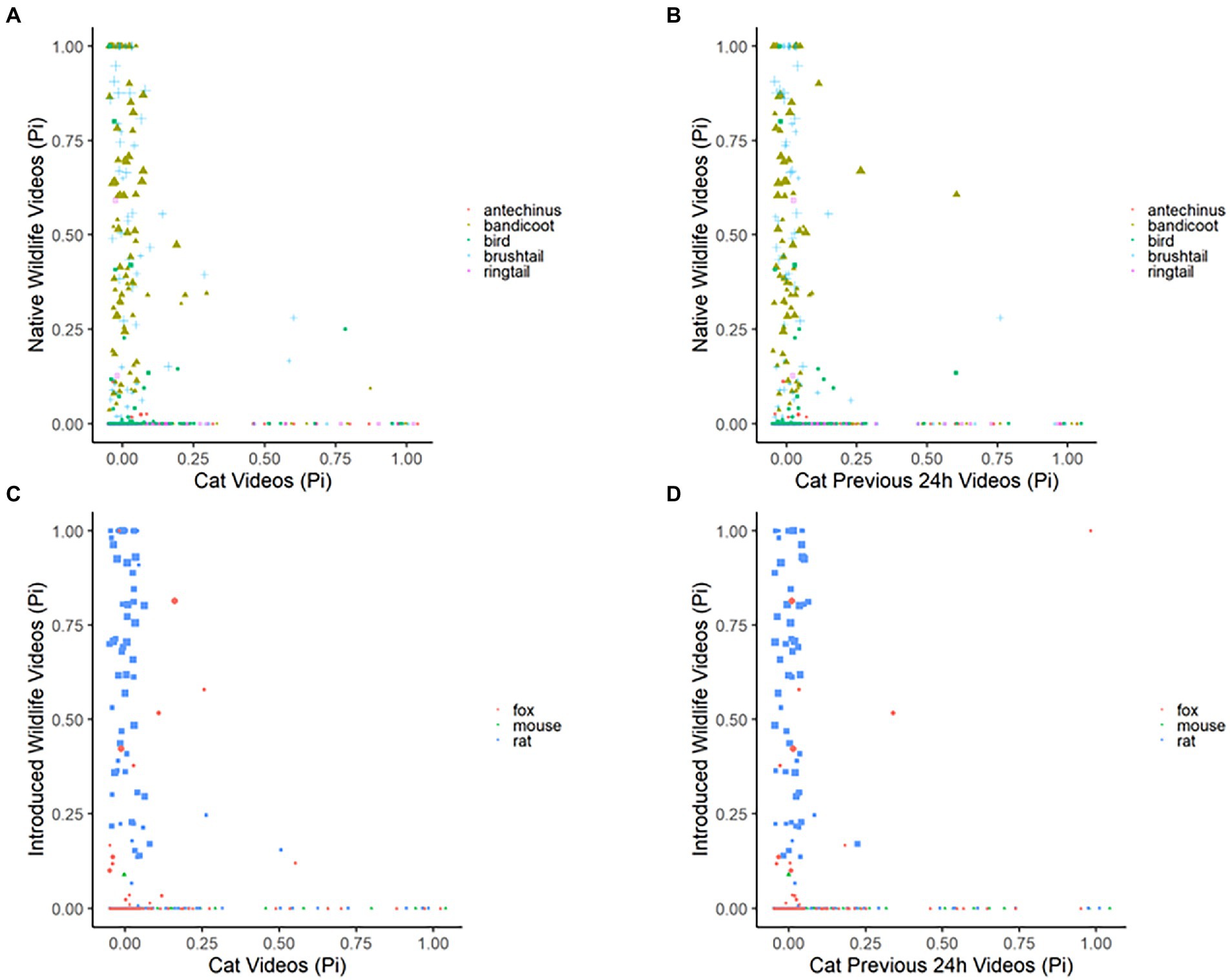
Figure 6. Plots depicting the raw data for the proportion of images observed per 24-h period for each species or animal group compared to the proportion of images that were of domestic cats in the green space edge habitat, for native wildlife (brown antechinus, northern brown bandicoot, native birds, common brushtail possum, and common ringtail possum) and introduced wildlife (red fox, brown and black rats, and house mouse); as: (A) native wildlife compared to domestic cats across the same 24-h period, (B) native wildlife compared to domestic cats across the prior-24-h period, (C) introduced wildlife compared to domestic cats across the same 24-h period, and (D) introduced wildlife compared to domestic cats across the prior-24-h period. Results recorded by six infrared scent and bait lured cameras positioned throughout a green space edge habitat for the duration of eight consecutive 24-h periods, for four repeated survey sessions.
Wildlife responses in green space edge habitats to increases in cat observations within the previous 24-h period had zero to small negative effects on common brushtail and ringtail possums, introduced rodents, and red foxes, but zero or minimal positive effect on brown antechinuses and northern brown bandicoots, and native birds (odds ratios ~1, Table 4). However, when there had been no cats observed in the previous 24-h period, all wildlife observations increased, with the largest ratio of inverse change evident in native birds, red foxes, then brown antechinuses and northern brown bandicoots, common brushtail and ringtail possums, and finally, introduced rodents (odds ratios <1, intercept results: Table 4, Figure 6).
Cat responses within the green space edge habitats to increases in disturbance observations of people and dogs were negative but minimal (odds ratios ~1). However, when no disturbances were observed there was a large increase in cat observations (odds ratios <1; Table 5, Figure 7). The same pattern was also evident for cat observations in response to cat observations in the previous 24-h period, showing a very minimal negative effect with increases in previous cat observations (odds ratios ~1) but large increases in observations of cats if there had been none observed in the prior period (odds ratios <1; Table 5; Figure 7).
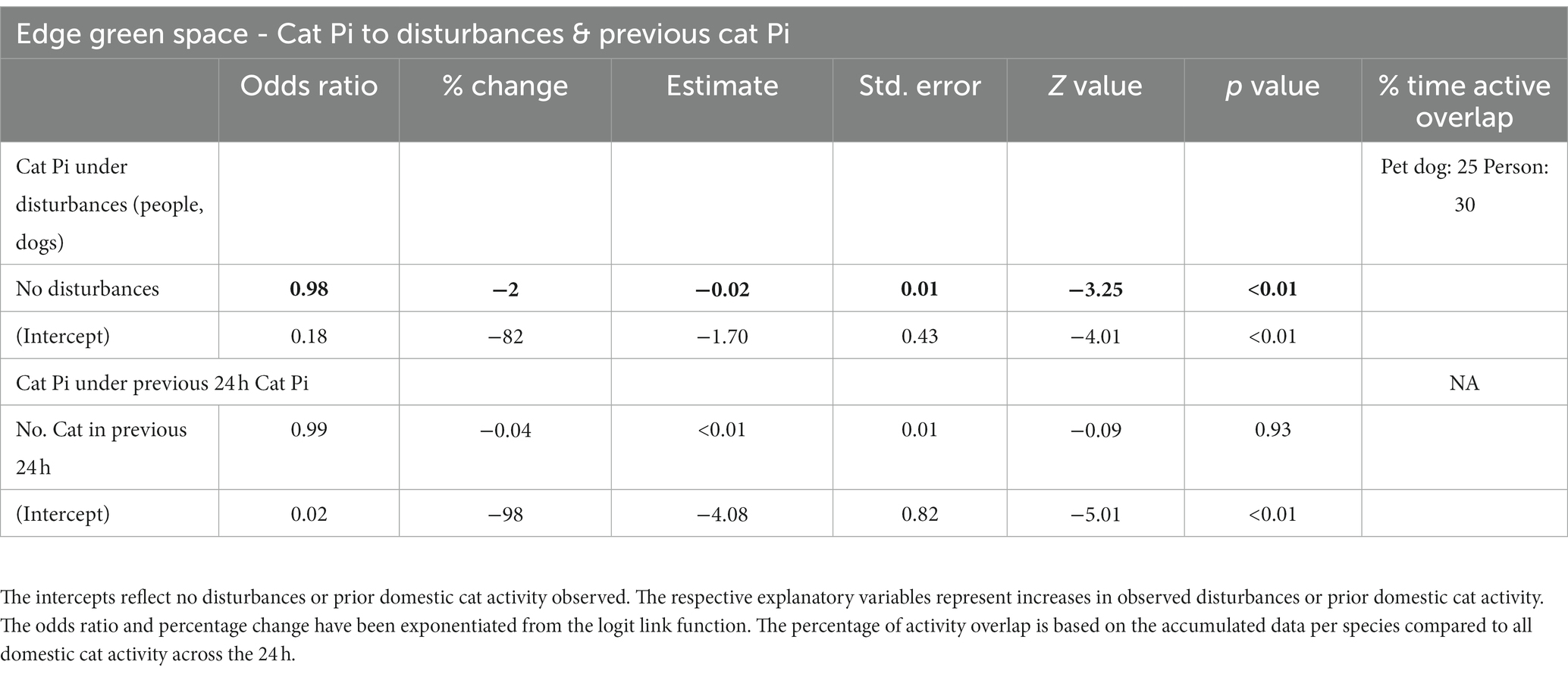
Table 5. Summary of the generalised linear mixed model output testing the effect of disturbances (people, dogs) and prior domestic cat activity on domestic cat activity in the green space edge habitat, based on six infrared scent and bait lured cameras positioned throughout the habitat for the duration of eight consecutive 24-h periods, for four repeated survey sessions.
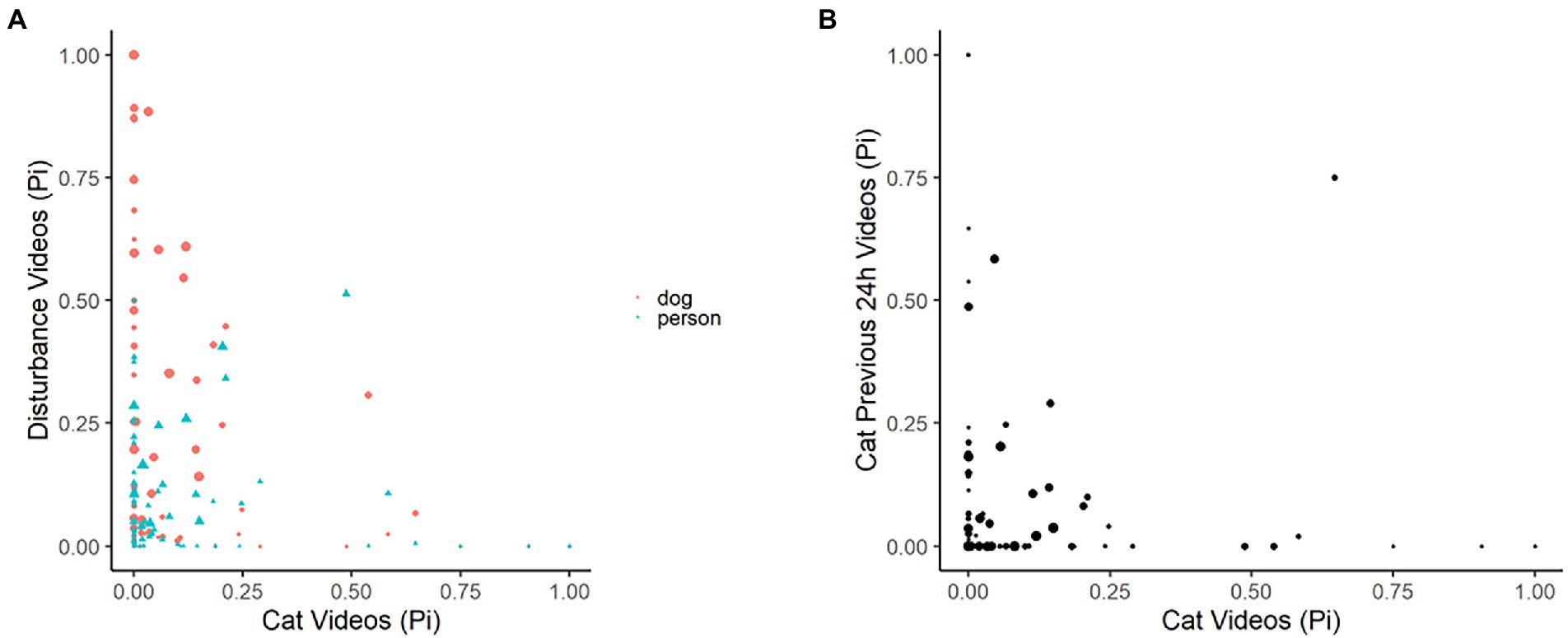
Figure 7. Plots depicting the raw data for the proportion of images observed per 24-h period for domestic cats in the green space edge habitat, compared to (A) the proportion of images that were disturbances (dogs, person), and (B) the proportion of images that were of prior-24-h domestic cat observations. Results recorded by six infrared scent and bait lured cameras positioned throughout a green space edge habitat for the duration of eight consecutive 24-h periods, for four repeated survey sessions.
Factors affecting data dispersion and frequency of zero wildlife observations in green space edge habitats
Dispersion and zero inflation parameters mostly differed for each of the best-fit green space edge habitat models (Supplementary material; Table 2). In each model below the variances are relative to the base of 1 (i.e., day 1, session 1, camera location 1, 1 type of animal observed). Responses to cats were present in most models’ variance parameters and had varied effects on the probability of a zero result and dispersion. For the native wildlife models of brown antechinuses and northern brown bandicoots, and native birds, the number of cats observed in the relative period (24-h, previous 24-h) had an inverse effect and increased the probability of a zero result as the number of cat observations decreased, without significance. For brown antechinuses and northern brown bandicoots this also had a significantly positive effect on dispersion. However, for the common brushtail and ringtail possum models the number of cats increased the probability of a zero result, as cat observations increased, significantly so under the cats observed in the previous 24-h model. Responses varied for the introduced mammals too, with the total number of cats increasing the probability of a zero result for introduced rodents, significantly in the previous 24-h model, but significantly decreasing the probability of a zero result in all red fox response models.
For all common brushtail and ringtail possum models and for the native birds model in response to cats in the 24-h period only, the dispersion parameter was set as the standard dispersion variance given the negative binomial 2 family distribution (Brooks et al., 2017), and the zero-inflation parameters consisted of the same variables. The camera location, survey session, and total number of animal types either increased or decreased the probability of a zero-result, with the decreases in location 4, session 4, and 2 types of animals observed in the possum models, and 4 and 5 types of animals observed for the native birds model each being significant. In the best fit model for native bird responses to cats observed in the previous 24 h, however, zero inflation was set to equal for all fixed and random effects modelled combined, which significantly increased the probability of a zero-result. Similarly, for each of the best-fit brown antechinus and northern brown bandicoot response models, survey session decreased the probability of a zero-result and camera location increased the probability of a zero-result, with locations 3 and 4 significant for the 24-h cat response models and locations 3, 4, and 5 significant for the previous 24-h cat response models. Survey session also had a positive effect on dispersion for all sessions but session 4 under the response to cats observed in the 24-h period model.
For each of the best-fit introduced rodent response models, the total number of animal types observed in the period, and day, decreased the probability of a zero-result, with the exception of day 8 that increased the probability. Each decreased probability was significant for both models, except for the observations of 6 types of animals in the response to cats observed in the previous 24-h model. In the 24-h period model only, camera location increased the probability of a zero-result, with location 3 being significant, and survey session decreased or increased the probability of a zero-result, but with no significance. Dispersion parameters were not fitted to any of the introduced rodent response models, considering the Poisson family distribution required for the model fit. Similarly, for the best-fit introduced red fox response to cats observed in the previous 24-h model, survey session increased the probability of a zero-result, without any significance. The dispersion parameter for both red fox response models was the total number of cats observed in the periods relative to each model (24 h, previous 24 h), which had a negative effect and was significant for the response to cats observed in the previous 24-h period model.
For the best-fit cat response to disturbances model, the total number of cats observed in the 24-h period significantly decreased the probability of a zero-result, and the dispersion parameter of total number of disturbance events in the 24-h period had a significantly inverse effect. For the best-fit cat response to the previously observed cats model, the total number of cats observed in the previous 24-h period decreased the probability of a zero-result, and the dispersion parameter of total number of cats observed in the previous 24-h period had an inverse effect, neither of which were significant.
Discussion
Urban environments are increasingly being found to have considerable conservation value to wildlife (Cincotta et al., 2000; Seto et al., 2012; Ives et al., 2016; Wintle et al., 2019). However, roaming cat activity is a common threat to wildlife persistence globally (Trouwborst et al., 2020), including within urban environments. Fear and stress effects can be just as detrimental to wildlife population persistence, if not more, than direct predation (Preisser et al., 2005; Beckerman et al., 2007; Loss and Marra, 2017), and can occur in response to predator cues alone (Apfelbach et al., 2005; Preisser et al., 2005, 2007). As such, we sought to investigate the possible fear/stress responses of wildlife, including native and introduced mammals and native birds, in urban yard and green space edge habitats in response to cat activity within the same 24-h and previous 24-h periods. We found that cat activity—both in the immediate and previous 24-h periods—reduced wildlife activity in a landscape of fear response, likely due to fear aversion behaviours redirecting activity from areas of cat activity. Although responses were more evident at the green space edge habitats, possibly due to the protection that yard enclosures and structures may offer wildlife (Fardell et al., 2022b), there was measurable native small mammal and bird activity in both the yard and green space edge habitats. These observations support the need for increased cat management to conserve urban biodiversity.
Of the combined 13 individual cats observed in both the yard and green space edge habitats, only two wore collars, one in each habitat. However, given the healthy condition of the cats and that few pet cats wear a collar due to perceived hazards they may cause (Harrod et al., 2016), with only 53% of Australian cat owners reported to use collars (Calver et al., 2007), it is likely that the cats we observed were pets or benefited from human-supplied resources. From our observations, cats were active largely at night in the yard habitats and across all hours except 6–8 am in the green space edge habitats. The activity overlap between wildlife and cats was between a quarter and half of the diel period, with higher overlap apparent in the green space edge habitats. There was clear evidence of cat activity during the hours of most wildlife activity, in both the yard and green space edge habitats. This may be little known to pet cat owners, as previous studies have determined that whilst owners thought they were containing their cat at night, 39% of their cats were actually roaming free at night (Roetman et al., 2017). Alternatively, owners may not be motivated to contain their cats’ roaming activity, believing instead that their cats’ impacts are minimal (Bruce Lauber et al., 2007). Interestingly, across both locations, we found no cat roaming activity between 6.00 and 8.00 am, which may be indicative of when they are being fed/getting human attention before the workday starts, which further supports our proposition that the observed cats were pets. Similarly, but to a lesser extent, we also observed reduced cat activity between 3.00 and 5.00 pm, when they may be fed/receive human attention at the end of the workday. This aligns with previous findings on times of cat roaming activity in an urban area in Australia, where activity was reduced when cats were likely to gain human attention (Meek, 2003).
Cat roaming across the hours common to wildlife activity affected the rates and areas of wildlife activity. In both the yard and green space edge habitats, wildlife reduced their activity in locations of cat activity in both the 24-h and previous 24-h periods, with the effect relative to cat presence and not changing with increasing cat activity. This indicates that any level of cat activity within an environment adversely affects wildlife behaviours as they respond to the direct or stressor cues from previous cat activity. Consistently changed behaviours from stress responses, such as predator aversion, can have acute effects that decrease reproduction and nutritional gain via increased cerebral activity and glucose release (Sapolsky et al., 2000). If stress responses are prolonged and persistent, then chronic effects can ensue and lead to allostatic overload (Dantzer et al., 2014), impairing the ability to maintain homeostasis (McEwen and Wingfield, 2003), leading in turn to reduced/impaired reproduction, and suppressed immune function (Dhabhar and McEwen, 1999; McEwen and Wingfield, 2003; Romero, 2004; Travers et al., 2010). Chronic stress responses can induce extended behavioural changes and reduce an animal’s ability to persist against future stressors (Mineur et al., 2006). Whilst we did not measure stress, we believe that aversion responses such as those observed warrant support of a fear/stress response to the stressor of cat (predator) activity in the area. Anxiety-based behaviours of retreat, increased vigilance, reduced grooming and movement, and elevated blood pressure have certainly been observed in rodent species in response to cat olfactory cues (Dielenberg and McGregor, 2001). Such responses to cat odour occur via the same neural pathways that are engaged in direct response to the presence of a live cat, which further confirms that predator cues can be just as stress-inducing as predation activity (Canteras and Goto, 1999; Dielenberg et al., 2001).
Maintaining health in the natural environment, however, requires some learnt and moderated responses to stressors if they are persistent, as we have observed in wild introduced rodents in response to cat olfactory cues, as they became more active over the night to meet foraging requirements, without any additional predator cues present (Fardell et al., 2021c). However, coping with a persistent stressor may well not reduce all stress effects and can still leave animals susceptible to additional compounding stressors, considering at the least the energy that coping responses, such as increased vigilance or redirected foraging, may need (Lima and Dill, 1990; Lima, 1998; Preisser et al., 2005, 2007; Doherty et al., 2015; Wirsing et al., 2021). For example, in support of this, Mahlaba et al. (2017) found that cats or dogs alone did not elicit an obvious aversion response but when their presence was combined there was a clear reduction in rodent activity. This may have population-level impacts as the presence of multiple stressors can have detrimental impacts on animals’ physiological systems (Arlettaz et al., 2015; Geary et al., 2019; Legge et al., 2019). Our results align with previous findings of reduced activity by wildlife in response to cat cues (Dielenberg and McGregor, 2001; Beckerman et al., 2007; Freeberg et al., 2016). Combined, our results suggest that landscape of fear effects are indeed evident for urban wildlife in response to cat roaming activity, and thus, the nonconsumptive effects of cats could be promoted to garner better support for urban cat management. This is important as the nonconsumptive or stress effects of cats can be stronger than those of actively hunting predators; especially as cats’ sit-and-wait stalking behaviour means there is a particularly high chance of their olfactory cue being related to a predation risk (Preisser et al., 2007).
As our placements of cameras in yards and green space edge habitats that bordered vegetated areas were near urban disturbances, it is possible that the wildlife we observed had bold personalities. High stress/predation on these individuals could, therefore, affect selection by disproportionately impacting the bold personality type, in turn negatively influencing the populations’ ability to persist; personality differences among individuals in a population are important for evolutionary processes (Wolf and Weissing, 2012). Such impacts have been found in fish populations relative to fishing, which can select for and remove bold and fast-growing genotypes from the population (Biro and Post, 2008). This is of concern considering that despite the trend of more wildlife activity when no cats were observed, when cat activity increased, the probability of any additional zero brown antechinus and northern brown bandicoot observations decreased. Perhaps this is indicative of a higher level of bold personalities in these species, or of the cross-over in foraging areas with cats increasing the reward relative to the risk, as cats can prey on invertebrates and small reptiles that these species could eat too. This could make such native species even more susceptible to predation pressures by cats and higher stress from increased interaction rates. The same inverse effect was evident for foxes, with the probability of zero observations decreasing with increasing cat observations, despite the trend of less activity in the presence of cats. This may indicate some mesopredator niche cross-over between these two introduced predators and the benefits to following each other to areas of prey activity (Molsher, 1999), which may compound their introduced predation and stressor pressures (Fardell et al., 2021b, 2022a). The opposite, however, was observed for the common brushtail and ringtail possums and the introduced rodents that had an increased probability of zero observations as cat observations increased. This may reflect stronger aversive behaviours of increased vigilance that we have observed previously in these species, in this area, in response to introduced predators and human disturbances (Fardell et al., 2022a). This may better equip them to be relatively more synurban (Feng and Himsworth, 2014; Wat, 2019).
There was some evidence of cat activity reducing in response to disturbances (people, dogs, horses, chickens), but cat activity was still observed consistently and at levels comparable to the native wildlife. The 13 cats observed in this study could indeed have detrimental impacts on wildlife in the area from predation alone, following the conclusion that unowned cats in highly modified landscapes can kill/consume ~158–255 small mammals per year, per individual cat, and that pet cats may kill/consume ~46.4 small mammals per year per individual cat (Murphy et al., 2019). That could equate to between ~603 and 3,315 small mammals removed annually from our combined study environments, with many more impacted by fear/stress responses to cat cues and their direct presence, as all but one camera location in the green space edge habitat recorded multiple cat observations, as did seven of the yards. Interestingly, cat observations increased if there had been no cat observations in the previous 24 h, which could suggest that cats traverse wider areas for entertainment/predation events, alternating between areas. This may be evident in differences between core and 100–95% roaming ranges (e.g., Hanmer et al., 2017; Fardell et al., 2021a). Such behaviour would spread cat olfactory cues and resulting stress responses in wildlife, and lead to larger areas within wildlife species’ home ranges being influenced by cats.
Conservation management value
Management of cats is challenging owing to them being identified as both feral/pest and companion/pet animals, which can garner mixed support (Farnworth et al., 2010, 2011). Divided community support can be a limiting factor for the management of cats in urban environments (Mameno et al., 2017; Travaglia and Miller, 2018). Cat management in urban areas of Australia, and likely other areas, is largely insufficient for the protection of prey species and biodiversity (Threatened Species Recovery Hub, 2020). Considering the increasing conservation value of urban environments for wildlife persistence (Cincotta et al., 2000; Seto et al., 2012; Ives et al., 2016; Wintle et al., 2019), 24-h containment for pet cats and no subsidised resources for feral cats are required (Threatened Species Recovery Hub, 2020). Our research provides evidence that supports this need. The success of urban cat management initiatives is dependent largely upon community support considering that policing them is both timely and costly, and thus often under-funded and under-staffed (Legge et al., 2020a). However, to garner such support more research is required to illustrate the impact of roaming cats across the 24-h period, without focusing on predatory events alone, as beliefs about this impact are divisive owing to lack of evidence that people may encounter directly (MacDonald et al., 2015; van Eeden et al., 2021). Here, we offer such an example, which may be better accepted by the public through appealing to their empathy for fear/stress effects. However, research on pet cat owner and community responses to such an appeal is yet to be studied. Understanding communities’ perceptions and including the community in wildlife management decisions has been proven to increase community support and the success of new initiatives (Bremner and Park, 2007). Pet cat management could aid in conservation success in urban environments by reducing fear and stress impacts on wildlife. With increasing environmental conscience over the years, pet cat management has increased, for example in Australia, where pet cat owner responsibility has slowly intensified via continual small changes (McCarthy, 2005; Toukhsati et al., 2012; McLeod et al., 2015; Hall et al., 2016; Linklater et al., 2019). Each change was implemented following extensive research to support it (Grayson and Calver, 2004; Denny and Dickman, 2010). Therefore, further research into all aspects of how cats may impact wildlife in urban environments, across the full 24-h period, better considering the fear and stress impacts, should be undertaken to better educate the community and help to influence people’s perception and willingness to support cat management in urbanised areas.
Conclusion
In conclusion, we provide evidence that roaming cat activity indeed creates landscapes of fear for urban wildlife. This offers novel justification for the need to increase cat management in urban areas, supporting 24-h confinement and reducing feral cat resource support from the community, by illuminating the fear and stress impacts that cats impose on wildlife from their roaming activity alone. Through these impacts vital behaviours like foraging and reproduction are redirected, which can be detrimental to wildlife health (Moberg, 1991; Preisser et al., 2005, 2007). Aiding the community to consider different perspectives in cat roaming impacts on wildlife may help to reduce any opposition to community responsibility in managing urban cats. Further research into fear and stress effects on wildlife from roaming cat activity in urban environments is required on a diverse range of species to provide further justification and support for changing community behaviour.
Data availability statement
The raw data supporting the conclusions of this article will be made available by the authors, without undue reservation.
Ethics statement
The animal study was reviewed and approved by animal ethics approval from the University of Sydney (2017/1275).
Author contributions
LF, CP, and CD conceived the study. LF performed the field work, statistical analyses, and wrote the initial manuscript draft. All authors contributed to the article and approved the submitted version.
Funding
This research was supported by a Holsworth Wildlife Research Endowment, and a Lake Macquarie Environmental Research Grant funded by Lake Macquarie City Council and other sponsors, which in 2018–2019 included Hunter Water Corporation, Delta Electricity and Origin Energy. LLF was supported by an Australian Government Research Training Program Stipend, and a University of Sydney Merit Award Scholarship.
Acknowledgments
We acknowledge the traditional owners of country throughout Australia and recognise their continuing connection to lands, waters and communities. We pay our respect to Aboriginal and Torres Strait Islander cultures; and to Elders past, present and emerging. We recognise and acknowledge the Aboriginal people, known today as the Awabakal, as the traditional Custodians of the land presently known as the suburb of Whitebridge. We are grateful to Associate Professor John Clulow for stimulating discussions on urban wildlife in the Lake Macquarie area and for his direction to sites of high wildlife activity. We are also grateful to Bobby Tamayo for his endless support and direction both logistically and in creative problem solving for in-field research.
Conflict of interest
The authors declare that the research was conducted in the absence of any commercial or financial relationships that could be construed as a potential conflict of interest.
Publisher’s note
All claims expressed in this article are solely those of the authors and do not necessarily represent those of their affiliated organizations, or those of the publisher, the editors and the reviewers. Any product that may be evaluated in this article, or claim that may be made by its manufacturer, is not guaranteed or endorsed by the publisher.
Supplementary material
The Supplementary material for this article can be found online at: https://www.frontiersin.org/articles/10.3389/fevo.2023.1123355/full#supplementary-material
References
Abrams, P. A. (2000). The evolution of predator-prey interactions: theory and evidence. Annu. Rev. Ecol. Syst. 31, 79–105. doi: 10.1146/annurev.ecolsys.31.1.79
Anson, J. R., and Dickman, C. R. (2013). Behavioral responses of native prey to disparate predators: naiveté and predator recognition. Oecologia 171, 367–377. doi: 10.1007/s00442-012-2424-7
Apfelbach, R., Blanchard, C. D., Blanchard, R. J., Hayes, R. A., and McGregor, I. S. (2005). The effects of predator odors in mammalian prey species: a review of field and laboratory studies. Neurosci. Biobehav. Rev. 29, 1123–1144. doi: 10.1016/j.neubiorev.2005.05.005
Arlettaz, R., Nusslé, S., Baltic, M., Vogel, P., Palme, R., Jenni-Eiermann, S., et al. (2015). Disturbance of wildlife by outdoor winter recreation: allostatic stress response and altered activity–energy budgets. Ecol. Appl. 25, 1197–1212. doi: 10.1890/14-1141.1
Banks, P. B., Daly, A., and Bytheway, J. P. (2016). Predator odours attract other predators, creating an olfactory web of information. Biol. Lett. 12:20151053. doi: 10.1098/rsbl.2015.1053
Beckerman, A. P., Boots, M., and Gaeston, K. J. (2007). Urban bird declines and the fear of cats. Anim. Conserv. 10, 320–325. doi: 10.1111/j.1469-1795.2007.00115.x
Bell, S. A. J. (2016). Volume 2: Vegetation community profiles, Lake Macquarie local government area, East Coast Flora survey, working draft v2. Unpublished report to Lake Macquarie City Council. Available online at: https://www.researchgate.net/publication/299749214_Volume_2_Vegetation_Community_Profiles_Lake_Macquarie_Local_Government_Area_Working_Draft_v2 [Accessed October 5, 2021].
Bennett, M. B., and Garden, J. G. (2004). Locomotion and gaits of the northern brown bandicoot, Isoodon macrourus (Marsupalia: Peramelidae). J. Mammal. 85, 296–301. doi: 10.1644/BWG-123
Biro, P. A., and Post, J. R. (2008). Rapid depletion of genotypes with fast growth and bold personality traits from harvested fish populations. PNAS 105, 2919–2922. doi: 10.1073/pnas.0708159105
Bonnington, C., Gaston, K. J., and Evans, K. L. (2013). Fearing the feline: domestic cats reduce avian fecundity through trait-mediated indirect effects that increase nest predation by other species. J. Appl. Ecol. 50, 15–24. doi: 10.1111/1365-2664.12025
Boonstra, R., Hik, D., Singleton, G. R., and Tinnikov, A. (1998). The impact of predator-induced stress on the snowshoe hare cycle. Ecol. Monogr. 68, 371–394. doi: 10.1890/0012-9615(1998)068[0371:TIOPIS]2.0.CO;2
Bremner, A., and Park, K. (2007). Public attitudes to the management of invasive non-native species in Scotland. Biol. Conserv. 139, 306–314. doi: 10.1016/j.biocon.2007.07.005
Bridges, C. M. (1999). Predator-prey interactions between two amphibian species: effects of insecticide exposure. Aquat. Ecol. 33, 205–211. doi: 10.1023/A:1009922825444
Brooks, M. E., Kristensen, K., van Benthem, K. J., Magnusson, M., Berg, C. W., Nielsen, A., et al. (2017). glmmTMB balances speed and flexibility among packages for zero-inflated generalized linear mixed modeling. R J. 9, 378–400. doi: 10.32614/RJ-2017-066
Brown, J. S. (1999). Vigilance, patch use and habitat selection: foraging under predation risk. Evol. Ecol. Res. 1, 49–71.
Brown, J. S., and Kotler, B. P. (2004). Hazardous duty pay and the foraging cost of predation. Ecol. Lett. 7, 999–1014. doi: 10.1111/j.1461-0248.2004.00661.x
Brown, M. B., Schlacher, T. A., Schoeman, D. S., Weston, M. A., Huijbers, C. M., Olds, A. D., et al. (2015). Invasive carnivores alter ecological function and enhance complementarity in scavenger assemblages on ocean beaches. Ecology 96, 2715–2725. doi: 10.1890/15-0027.1
Bruce Lauber, T., Knuth, B. A., Tantillo, J. A., and Curtis, P. D. (2007). The role of ethical judgments related to wildlife fertility control. Soc. Nat. Resour. 20, 119–133. doi: 10.1080/08941920601052362
Burnham, K., and Anderson, D. (2004). Model selection and multi-model inference: A practical information theoretic approach, 2nd edn Springer-Verlag, New York, p.63.
Bytheway, J. P., and Banks, P. B. (2019). Overcoming prey naiveté: free-living marsupials develop recognition and effective behavioral responses to alien predators in Australia. Glob. Chang. Biol. 25, 1685–1695. doi: 10.1111/gcb.14607
Calver, M., Thomas, S., Bradley, S., and McCutcheon, H. (2007). Reducing the rate of predation on wildlife by pet cats: the efficacy and practicability of collar-mounted pounce protectors. Biol. Conserv. 137, 341–348. doi: 10.1016/j.biocon.2007.02.015
Canteras, N. S., and Goto, M. (1999). Fos-like immunoreactivity in the periaqueductal gray of rats exposed to a natural predator. Neuroreport 10, 413–418. doi: 10.1097/00001756-199902050-00037
Carthey, A. J., and Blumstein, D. T. (2018). Predicting predator recognition in a changing world. Trends Ecol. Evol. 33, 106–115. doi: 10.1016/j.tree.2017.10.009
Cincotta, R. P., Wisnewski, J., and Engelman, R. (2000). Human population in the biodiversity hotspots. Nature 404, 990–992. doi: 10.1038/35010105
Clinchy, M., Zanette, L., Boonstra, R., Wingfield, J. C., and Smith, J. N. (2004). Balancing food and predator pressure induces chronic stress in songbirds. Proc. R. Soc. Lond. B Biol. Sci. 271, 2473–2479. doi: 10.1098/rspb.2004.2913
Courchamp, F., Chapuis, J. L., and Pascal, M. (2003). Mammal invaders on islands: impact, control and control impact. Biol. Rev. 78, 347–383. doi: 10.1017/S1464793102006061
Crowley, S. L., Cecchetti, M., and McDonald, R. A. (2020). Our wild companions: domestic cats in the Anthropocene. Trends Ecol. Evol. 35, 477–483. doi: 10.1016/j.tree.2020.01.008
Dantzer, B., Fletcher, Q. E., Boonstra, R., and Sheriff, M. J. (2014). Measures of physiological stress: a transparent or opaque window into the status, management and conservation of species? Cons. Physiol. 2:cou023. doi: 10.1093/conphys/cou023
Davey, G., Khor, M. M., and Zhao, X. (2019). Key beliefs underlying public feeding of free-roaming cats in Malaysia and management suggestions. Hum. Dimens. Wildl. 24, 1–13. doi: 10.1080/10871209.2018.1522679
Davis, T. S., Crippen, T. L., Hofstetter, R. W., and Tomberlin, J. K. (2013). Microbial volatile emissions as insect semiochemicals. J. Chem. Ecol. 39, 840–859. doi: 10.1007/s10886-013-0306-z
Dearborn, D. C., and Kark, S. (2010). Motivations for conserving urban biodiversity. Conserv. Biol. 24, 432–440. doi: 10.1111/j.1523-1739.2009.01328.x
Denny, E.A., and Dickman, C. (2010). Review of cat ecology and management strategies in Australia. Canberra, Australia: Invasive Animals Cooperative Research Centre.
Department of Environment, Climate Change and Water (DECCW) (2010). Glenrock state conservation area plan of management ; DECCW: Sydney, NSW, Australia.
Dhabhar, F. S., and McEwen, B. S. (1999). Enhancing versus suppressive effects of stress hormones on skin immune function. PNAS 96, 1059–1064. doi: 10.1073/pnas.96.3.1059
Dickman, C. R. (1991). “Use of trees by ground-dwelling mammals: implications for management” in Conservation of Australia's forest fauna. ed. D. Lunney (Sydney: Royal Zoological Society of New South Wales), 125–136. doi: 10.7882/RZSNSW.1991.012
Dielenberg, R. A., Hunt, G. E., and McGregor, I. S. (2001). ‘When a rat smells a cat’: the distribution of Fos immunoreactivity in rat brain following exposure to a predatory odor. Neuroscience 104, 1085–1097. doi: 10.1016/S0306-4522(01)00150-6
Dielenberg, R. A., and McGregor, I. S. (2001). Defensive behavior in rats towards predatory odors: a review. Neurosci. Biobehav. Rev. 25, 597–609. doi: 10.1016/S0149-7634(01)00044-6
Doherty, T. S., Dickman, C. R., Nimmo, D. G., and Ritchie, E. G. (2015). Multiple threats, or multiplying the threats? Interactions between invasive predators and other ecological disturbances. Biol. Conserv. 190, 60–68. doi: 10.1016/j.biocon.2015.05.013
Doherty, T. S., Glen, A. S., Nimmo, D. G., Ritchie, E. G., and Dickman, C. R. (2016). Invasive predators and global biodiversity loss. PNAS 113, 11261–11265. doi: 10.1073/pnas.1602480113
Dorph, A., and Ballard, G. (2022). Current and emerging feral cat management practices in Australia, report to the resilient landscapes hub of the Australian Government’s National Environmental Science Program, University of New England, Armidale.
Dubey, J. P., and Jones, J. L. (2008). Toxoplasma gondii infection in humans and animals in the United States. Int. J. Parasitol. 38, 1257–1278. doi: 10.1016/j.ijpara.2008.03.007
Estanislau, C., Veloso, A. W., Filgueiras, G. B., Maio, T. P., Dal-Cól, M. L., Cunha, D. C., et al. (2019). Rat self-grooming and its relationships with anxiety, dearousal and perseveration: evidence for a self-grooming trait. Physiol. Behav. 209:112585. doi: 10.1016/j.physbeh.2019.112585
Fardell, L. L., Bedoya-Pérez, M. A., Dickman, C. R., Crowther, M. S., Pavey, C. R., and Narayan, E. J. (2021c). Are physiological and behavioural responses to stressors displayed concordantly by wild urban rodents? Sci. Nat. 108, 5–15. doi: 10.1007/s00114-020-01716-8
Fardell, L. L., Nano, C. E. M., Pavey, C. R., and Dickman, C. R. (2021b). Small prey animal habitat use in landscapes of fear: effects of predator presence and human activity along an urban disturbance gradient. Front. Ecol. Evol. 9:750094. doi: 10.3389/fevo.2021.750094
Fardell, L. L., Nano, C. E. M., Pavey, C. R., and Dickman, C. R. (2022a). Small prey animal foraging behaviours in landscapes of fear: effects of predator presence and human activity along an urban disturbance gradient. Front. Ecol. Evol. 10:805891. doi: 10.3389/fevo.2022.805891
Fardell, L. L., Pavey, C. R., and Dickman, C. R. (2020). Fear and stressing in predator–prey ecology: considering the twin stressors of predators and people on mammals. PeerJ 8:e9104. doi: 10.7717/peerj.9104
Fardell, L. L., Pavey, C. R., and Dickman, C. R. (2022b). Backyard biomes: is anyone there? Improving public awareness of urban wildlife activity. Diversity 14:263. doi: 10.3390/d14040263
Fardell, L. L., Young, L. I., Pavey, C. R., and Dickman, C. R. (2021a). Habitat use by wandering pet cats (Felis catus) in a patchy urban environment. J. Urban Ecol. 7:juab019. doi: 10.1093/jue/juab019
Farnworth, M., Campbell, J., and Adams, N. J. (2011). What’s in a name? Perceptions of stray and feral cat welfare and control in Aotearoa, New Zealand. J. Appl. Anim. Welf. Sci. 14, 59–74. doi: 10.1080/10888705.2011.527604
Farnworth, M. J., Dye, N. G., and Keown, N. (2010). The legal status of cats in New Zealand: a perspective on the welfare of companion, stray, and feral domestic cats (Felis catus). J. Appl. Anim. Welf. Sci. 13, 180–188. doi: 10.1080/10888700903584846
Feng, A. Y., and Himsworth, C. G. (2014). The secret life of the city rat: a review of the ecology of urban Norway and black rats (Rattus norvegicus and Rattus rattus). Urban Ecosyst. 17, 149–162. doi: 10.1007/s11252-013-0305-4
Fenn, M. G., and Macdonald, D. W. (1995). Use of middens by red foxes: risk reverses rhythms of rats. J. Mammal. 76, 130–136. doi: 10.2307/1382321
Ferkin, M., Sorokin, E., Johnston, R., and Lee, C. (1997). Attractiveness of scents varies with protein content of the diet in meadow voles. Anim. Behav. 53, 133–141. doi: 10.1006/anbe.1996.0284
Fleming, P., Meek, P., Ballard, G., Banks, P., Calridge, A., Sanderson, J., et al. (2014). Camera trapping: Wildlife management and research ; CSIRO Publishing: Clayton, Australia.
Freeberg, T. M., Book, D. L., and Weiner, R. L. (2016). Foraging and calling behavior of Carolina chickadees (Poecile carolinensis) in response to the head orientation of potential predators. Ethology 122, 10–19. doi: 10.1111/eth.12438
Frid, A., and Dill, L. M. (2002). Human-caused disturbance stimuli as a form of predation risk. Conserv. Ecol. 6:11. doi: 10.5751/ES-00404-060111
Gaertner, M., Wilson, J. R., Cadotte, M. W., MacIvor, J. S., Zenni, R. D., and Richardson, D. M. (2017). Non-native species in urban environments: patterns, processes, impacts and challenges. Biol. Invasions 19, 3461–3469. doi: 10.1007/s10530-017-1598-7
Gaston, K. J., Ávila-Jiménez, M. L., Edmondsonm, J. L., and Jones, J. (2013). Managing urban ecosystems for goods and services. J. Appl. Ecol. 50, 830–840. doi: 10.1111/1365-2664.12087
Gaston, K. J., Warren, P. H., Thompson, K., and Smith, R. M. (2005). Urban domestic gardens (IV): the extent of the resource and its associated features. Biodivers. Conserv. 14, 3327–3349. doi: 10.1007/s10531-004-9513-9
Geary, W. L., Nimmo, D. G., Doherty, T. S., Ritchie, E. G., and Tulloch, A. I. (2019). Threat webs: reframing the co-occurrence and interactions of threats to biodiversity. J. Appl. Ecol. 56, 1992–1997. doi: 10.1111/1365-2664.13427
Grayson, J., and Calver, M. C. (2004). “Regulation of domestic cat ownership to protect urban wildlife: a justification based on the precautionary principle” in Urban wildlife: More than meets the eye. eds. D. Lunney and S. Burgin (Sydney, NSW, Australia: Royal Zoological Society of New South Wales), 169–178.
Grimm, N. B., Faeth, S. H., Golubiewski, N. E., Redman, C. L., Wu, J., Bai, X., et al. (2008). Global change and the ecology of cities. Science 319, 756–760. doi: 10.1126/science.1150195
Hall, C. M., Adams, N. A., Bradley, J. S., Bryant, K. A., Davis, A. A., Dickman, C. R., et al. (2016). Community attitudes and practices of urban residents regarding predation by pet cats on wildlife: an international comparison. PLoS One 11:e0151962. doi: 10.1371/journal.pone.0151962
Hanmer, H. J., Thomas, R. L., and Fellowes, M. D. E. (2017). Urbanisation influences range size of the domestic cat (Felis catus): consequences for conservation. J. Urban Ecol. 3, 1–11. doi: 10.1093/jue/jux014
Harrod, M., Keown, A. J., and Farnworth, M. J. (2016). Use and perception of collars for companion cats in New Zealand. NZVJ 64, 121–124. doi: 10.1080/00480169.2015.1110064
Hartig, F. (2021). DHARMa: Residual diagnostics for hierarchical (multi-level/mixed) regression models. R package version 0.4.1.
Herberholz, J., and Marquart, G. D. (2012). Decision making and behavioral choice during predator avoidance. Front. Neurosci. 6:125. doi: 10.3389/fnins.2012.00125
Herman, C. S., and Valone, T. J. (2000). The effect of mammalian predator scent on the foraging behavior of Dipodomys merriami. Oikos 91, 139–145. doi: 10.1034/j.1600-0706.2000.910113.x
Hobbs, R. J., Higgs, E., and Harris, J. A. (2009). Novel ecosystems: implications for conservation and restoration. Trends Ecol. Evol. 24, 599–605. doi: 10.1016/j.tree.2009.05.012
Ives, C. D., Lentini, P. E., Threlfall, C. G., Ikin, K., Shanahan, D. F., Garrard, G. E., et al. (2016). Cities are hotspots for threatened species. Glob. Ecol. Biogeogr. 25, 117–126. doi: 10.1111/geb.12404
Jones, M. E., Apfelbach, R., Banks, P. B., Cameron, E. Z., Dickman, C. R., Frank, A., et al. (2016). A nose for death: integrating trophic and informational networks for conservation and management. Front. Ecol. Evol. 4:124. doi: 10.3389/fevo.2016.00124
Koolhaas, J. M., Korte, S. M., De Boer, S. F., Van Der Vegt, B. J., Van Reenen, C. G., Hopster, H., et al. (1999). Coping styles in animals: current status in behavior and stress-physiology. Neurosci. Biobehav. Rev. 23, 925–935. doi: 10.1016/S0149-7634(99)00026-3
Kowarik, I. (2011). Novel urban ecosystems, biodiversity, and conservation. Environ. Pollut. 159, 1974–1983. doi: 10.1016/j.envpol.2011.02.022
Laundré, J. W., Hernández, L., and Altendorf, K. B. (2001). Wolves, elk, and bison: re-establishing the "landscape of fear" in Yellowstone National Park, USA. Can. J. Zool. 79, 1401–1409. doi: 10.1139/z01-094
Laundré, J. W., Hernández, L., Medina, P. L., Campanella, A., López-Portillo, J., González-Romero, A., et al. (2014). The landscape of fear: the missing link to understand top-down and bottom-up controls of prey abundance? Ecology 95, 1141–1152. doi: 10.1890/13-1083.1
Laundré, J. W., Hernández, L., and Ripple, W. J. (2010). The landscape of fear: ecological implications of being afraid. Open Ecol. J. 3, 1–7. doi: 10.2174/1874213001003030001
Legge, S., Murphy, B. P., McGregor, H., Woinarski, J. C. Z., Augusteyn, J., Ballard, G., et al. (2017). Enumerating a continental-scale threat: how many feral cats are in Australia? Biol. Conserv. 206, 293–303. doi: 10.1016/j.biocon.2016.11.032
Legge, S., Smith, J. G., James, A., Tuft, K. D., Webb, T., and Woinarski, J. C. Z. (2019). Interactions among threats affect conservation management outcomes: livestock grazing removes the benefits of fire management for small mammals in Australian tropical savannas. Cons. Sci. Pract. 1, e52. doi: 10.1111/csp2.52
Legge, S., Woinarski, J. C. Z., Dickman, C. R., Doherty, T. S., McGregor, H., and Murphy, B. P. (2020b). Cat ecology, impacts and management in Australia. Wildl. Res. 47:i. doi: 10.1071/WRv47n8_ED
Legge, S., Woinarski, J. C. Z., Dickman, C. R., Murphy, B. P., Woolley, L. A., and Calver, M. C. (2020a). We need to worry about Bella and Charlie: the impacts of pet cats on Australian wildlife. Wildl. Res. 47, 523–539. doi: 10.1071/WR19174
Lima, S. L. (1998). Stress and decision-making under the risk of predation: recent developments from behavioral, reproductive, and ecological perspectives. Adv. Study Behav. 27, 215–290. doi: 10.1016/S0065-3454(08)60366-6
Lima, S. L., and Dill, L. M. (1990). Behavioral decisions made under the risk of predation: a review and prospectus. Can. J. Zool. 68, 619–640. doi: 10.1139/z90-092
Linklater, W. L., Farnworth, M. J., van Heezik, Y., Stafford, K. J., and MacDonald, E. A. (2019). Prioritizing cat-owner behaviors for a campaign to reduce wildlife depredation. Conserv. Sci. Pract. 1:e29. doi: 10.1111/csp2.29
Łopucki, R., and Kitowski, I. (2017). How small cities affect the biodiversity of ground-dwelling mammals and the relevance of this knowledge in planning urban land expansion in terms of urban wildlife. Urban Ecosyst. 20, 933–943. doi: 10.1007/s11252-016-0637-y
Loss, S. R., and Marra, P. P. (2017). Population impacts of free-ranging domestic cats on mainland vertebrates. Front. Ecol. Environ. 15, 502–509. doi: 10.1002/fee.1633
Lowe, S., Browne, M., Boudjelas, S., and De Poorter, M. (2000). 100 of the world’s worst invasive alien species: A selection from the global invasive species database. Invasive Specialist Group, Auckland.
MacDonald, E., Milfont, T., and Gavin, M. (2015). What drives cat-owner behaviour? First steps towards limiting domestic-cat impacts on native wildlife. Wildl. Res. 42, 257–265. doi: 10.1071/WR14164
Maclagan, S. J., Coates, T., and Ritchie, E. G. (2018). Don't judge habitat on its novelty: assessing the value of novel habitats for an endangered mammal in a peri-urban landscape. Biol. Conserv. 223, 11–18. doi: 10.1016/j.biocon.2018.04.022
Mahlaba, T. A. A., Monadjem, A., McCleery, R., and Belmain, S. R. (2017). Domestic cats and dogs create a landscape of fear for pest rodents around rural homesteads. PLoS One 12:e0171593. doi: 10.1371/journal.pone.0171593
Mameno, K., Kubo, T., and Suzuki, M. (2017). Social challenges of spatial planning for outdoor cat management in Amami Oshima Island, Japan. GECCO 10, 184–193. doi: 10.1016/j.gecco.2017.03.007
Marrow, P., Law, R., and Cannings, C. (1992). The coevolution of predator—prey interactions: ESSs and red queen dynamics. Proc. R. Soc. Lond. B Biol. Sci. 250, 133–141.
McCarthy, S. (2005). “Managing impacts of domestic cats in peri-urban reserves.” in Urban animal management conference proceedings. Canberra, Australia: Australian Veterinary Association. pp. 103–109.
McEwen, B. S., and Wingfield, J. C. (2003). The concept of allostasis in biology and biomedicine. Horm. Behav. 43, 2–15. doi: 10.1016/S0018-506X(02)00024-7
McKinney, M. L. (2006). Urbanization as a major cause of biotic homogenization. Biol. Conserv. 127, 247–260. doi: 10.1016/j.biocon.2005.09.005
McLeod, L. J., Hine, D. W., and Bengsen, A. J. (2015). Born to roam? Surveying cat owners in Tasmania, Australia, to identify the drivers and barriers to cat containment. Prev. Vet. Med. 122, 339–344. doi: 10.1016/j.prevetmed.2015.11.007
Medina, F. M., Bonnaud, E., Vidal, E., Tershy, B. R., Zavaleta, E. S., Josh Donlan, C., et al. (2011). A global review of the impacts of invasive cats on island endangered vertebrates. Glob. Chang. Biol. 17, 3503–3510. doi: 10.1111/j.1365-2486.2011.02464.x
Meek, P. D. (2003). Home range of house cats Felis catus living within a National Park. Aust. Mammal. 25, 51–60. doi: 10.1071/AM03051
Mineur, Y. S., Belzung, C., and Crusio, W. E. (2006). Effects of unpredictable chronic mild stress on anxiety and depression-like behavior in mice. Behav. Brain Res. 175, 43–50. doi: 10.1016/j.bbr.2006.07.029
Moberg, G. P. (1991). How behavioral stress disrupts the endocrine control of reproduction in domestic animals. J. Dairy Sci. 74, 304–311. doi: 10.3168/jds.S0022-0302(91)78174-5
Molsher, R.L. (1999). The ecology of feral cats, Felis catus, in open forest in New South Wales: Interactions with food resources and foxes. Ph.D thesis, University of Sydney.
Murphy, B. P., Woolley, L. A., Geyle, H. M., Legge, S. M., Palmer, R., Dickman, C. R., et al. (2019). Introduced cats (Felis catus) eating a continental fauna: the number of mammals killed in Australia. Biol. Conserv. 237, 28–40. doi: 10.1016/j.biocon.2019.06.013
Navarro-Castilla, Á, and Barja, I. (2014). Does predation risk, through moonphase and predator cues, modulate food intake, antipredatory and physiological responses in wood mice (Apodemus sylvaticus)? Behav. Ecol. Sociobiol. 68, 1505–1512. doi: 10.1007/s00265-014-1759-y
Newsome, T. M., Dellinger, J. A., Pavey, C. R., Ripple, W. J., Shores, C. R., Wirsing, A. J., et al. (2015). The ecological effects of providing resource subsidies to predators. Glob. Ecol. Biogeogr. 24, 1–11. doi: 10.1111/geb.12236
Orrock, J. L., and Danielson, B. J. (2009). Temperature and cloud cover, but not predator urine, affect winter foraging of mice. Ethology 115, 641–648. doi: 10.1111/j.1439-0310.2009.01654.x
Parsons, M. H., Apfelbach, R., Banks, P. B., Cameron, E. Z., Dickman, C. R., Frank, A. S., et al. (2017). Biologically meaningful scents: a framework for understanding predator–prey research across disciplines. Biol. Rev. 93, 98–114. doi: 10.1111/brv.12334
Parsons, M. H., and Blumstein, D. T. (2010). Familiarity breeds contempt: kangaroos persistently avoid areas with experimentally deployed dingo scents. PLoS One 5: e10403. doi: 10.1371/journal.pone.0010403
Patten, M. A., and Burger, J. C. (2018). Reserves as double-edged sword: avoidance behavior in an urban-adjacent wildland. Biol. Conserv. 218, 233–239. doi: 10.1016/j.biocon.2017.12.033
Pickett, S. T., Cadenasso, M. L., Grove, J. M., Nilon, C. H., Pouyat, R. V., Zipperer, W. C., et al. (2001). Urban ecological systems: linking terrestrial ecological, physical, and socioeconomic components of metropolitan areas. Annu. Rev. Ecol. Syst. 32, 127–157. doi: 10.1146/annurev.ecolsys.32.081501.114012
Preisser, E. L., Bolnick, D. I., and Benard, M. F. (2005). Scared to death? The effects of intimidation and consumption in predator-prey interactions. Ecology 86, 501–509. doi: 10.1890/04-0719
Preisser, E. L., Orrock, J. L., and Schmitz, O. J. (2007). Predator hunting mode and habitat domain alter nonconsumptive effects in predator–prey interactions. Ecology 88, 2744–2751. doi: 10.1890/07-0260.1
R Core Team (2022). R: A language and environment for statistical computing. R Foundation for Statistical Computing, Vienna, Austria.
Rebolo-Ifrán, N., Tella, J. L., and Carrete, M. (2017). Urban conservation hotspots: predation release allows the grassland-specialist burrowing owl to perform better in the city. Sci. Rep. 7, 1–9. doi: 10.1038/s41598-017-03853-z
Rehnus, M., Wehrle, M., and Palme, R. (2014). Mountain hares Lepus timidus and tourism: stress events and reactions. J. Appl. Ecol. 51, 6–12. doi: 10.1111/1365-2664.12174
Reshamwala, H. S., Shrotriya, S., Bora, B., Lyngdoh, S., Dirzo, R., and Habib, B. (2018). Anthropogenic food subsidies change the pattern of red fox diet and occurrence across trans-Himalayas, India. J. Arid Environ. 150, 15–20. doi: 10.1016/j.jaridenv.2017.12.011
Ridout, M., and Linkie, M. (2009). Estimating overlap of daily activity patterns from camera trapdata. J. Agric. Biol. Environ. Stat 14, 322–337.
Roetman, P., Tindle, H., Litchfield, C., Chiera, B., Quinton, G., Kikillus, H., et al. (2017). Cat tracker South Australia: Understanding pet cats through citizen science. Discovery Circle initiative, University of South Australia: Adelaide, SA, Australia.
Romero, L. M. (2004). Physiological stress in ecology: lessons from biomedical research. Trends Ecol. Evol. 19, 249–255. doi: 10.1016/j.tree.2004.03.008
Sapolsky, R. M., Romero, L. M., and Munck, A. U. (2000). How do glucocorticoids influence stress responses? Integrating permissive, suppressive, stimulatory, and preparative actions. Endocr. Rev. 21, 55–89.
Sattar, Q., Maqbool, M. E., Ehsan, R., and Akhtar, S. (2021). Review on climate change and its effect on wildlife and ecosystem. Open J. Environ. Biol. 6, 008–014. doi: 10.17352/ojeb.000021
Seto, K. C., Güneralp, B., and Hutyra, L. R. (2012). Global forecasts of urban expansion to 2030 and direct impacts on biodiversity and carbon pools. PNAS 109, 16083–16088. doi: 10.1073/pnas.1211658109
Shrader, A. M., Brown, J. S., Kerley, G. I., and Kotler, B. P. (2008). Do free-ranging domestic goats show ‘landscapes of fear’? Patch use in response to habitat features and predator cues. J. Arid Environ. 72, 1811–1819. doi: 10.1016/j.jaridenv.2008.05.004
Sinclair, A. R. E., and Arcese, P. (1995). Population consequences of predation-sensitive foraging: the Serengeti wildebeest. Ecology 76, 882–891. doi: 10.2307/1939353
Singh, N., Price, C., and Downs, C. T. (2021). Aspects of the ecology and behaviour of a potential urban exploiter, the southern tree agama, Acanthocercus atricollis. Urban Ecosyst. 24, 905–914. doi: 10.1007/s11252-020-01078-z
Soanes, K., and Lentini, P. E. (2019). When cities are the last chance for saving species. Front. Ecol. Environ. 17, 225–231. doi: 10.1002/fee.2032
Soso, S. B., Koziel, J. A., Johnson, A., Lee, Y. J., and Fairbanks, W. S. (2014). Analytical methods for chemical and sensory characterization of scent-markings in large wild mammals: a review. Sensors 14, 4428–4465. doi: 10.3390/s140304428
Takahashi, L. K. (2014). Olfactory systems and neural circuits that modulate predator odor fear. Front. Behav. Neurosci. 8:72. doi: 10.3389/fnbeh.2014.00072
Tay, N. E., Fleming, P. A., Warburton, N. M., and Moseby, K. E. (2021). Predator exposure enhances the escape behaviour of a small marsupial, the burrowing bettong. Anim. Behav. 175, 45–56. doi: 10.1016/j.anbehav.2021.02.013
Threatened Species Recovery Hub (2020). Australia, house of representatives, standing committee on the environment and energy inquiry into the problem of feral and domestic cats in Australia. Submission 72. Available online at: https://www.aph.gov.au/Parliamentary_Business/Committees/House/Former_Committees/Environment_and_Energy/Feralanddomesticcats/Submissions (#72) [Accessed December 3, 2022].
Toukhsati, S. R., Bennett, P. C., and Coleman, G. J. (2007). Behaviors and attitudes towards semi-owned cats. Anthrozoös 20, 131–142. doi: 10.2752/175303707X207927
Toukhsati, S. R., Young, E., Bennett, P. C., and Coleman, G. J. (2012). Wandering cats: attitudes and behaviors towards cat containment in Australia. Anthrozoös 25, 61–74. doi: 10.2752/175303712X13240472427195
Travaglia, M., and Miller, K. K. (2018). Cats in the Australian environment: what’s your purr-spective? AJEM 25, 153–173. doi: 10.1080/14486563.2017.1369465
Travers, M., Clinchy, M., Zanette, L., Boonstra, R., and Williams, T. D. (2010). Indirect predator effects on clutch size and the cost of egg production. Ecol. Lett. 13, 980–988. doi: 10.1111/j.1461-0248.2010.01488.x
Trouwborst, A., McCormack, P. C., and Martínez Camacho, E. (2020). Domestic cats and their impacts on biodiversity: a blind spot in the application of nature conservation law. Peop. Nat. 2, 235–250. doi: 10.1002/pan3.10073
Tryjanowski, P., Morelli, F., Skórka, P., Goławski, A., Indykiewicz, P., Møller, A. P., et al. (2015). Who started first? Bird species visiting novel bird feeders. Sci. Rep. 5:11858. doi: 10.1038/srep11858
van der Merwe, M., and Brown, J. S. (2008). Mapping the landscape of fear of the cape ground squirrel (Xerus inauris). J. Mammal. 89, 1162–1169. doi: 10.1644/08-MAMM-A-035.1
van Eeden, L. M., Hames, F., Faulkner, R., Geschke, A., Squires, Z. E., and McLeod, E. M. (2021). Putting the cat before the wildlife: exploring cat owners' beliefs about cat containment as predictors of owner behavior. Conserv. Sci. Pract. 3:e502. doi: 10.1111/csp2.502
Vitousek, P. M., D'antonio, C. M., Loope, L. L., Rejmanek, M., and Westbrooks, R. (1997). Introduced species: a significant component of human-caused global change. N. Z. J. Ecol., 21, 1–16.
Wat, K. Y. K. (2019). Linking animal personality to space use and problem-solving by common brushtail possums, an urban exploiter. Ph.D. Thesis. University of Sydney.
West, R., Letnic, M., Blumstein, D. T., and Moseby, K. E. (2018). Predator exposure improves anti-predator responses in a threatened mammal. J. Appl. Ecol. 55, 147–156. doi: 10.1111/1365-2664.12947
Willems, E. P., and Hill, R. A. (2009). Predator-specific landscapes of fear and resource distribution: effects on spatial range use. Ecology 90, 546–555. doi: 10.1890/08-0765.1
Wintle, B. A., Kujala, H., Whitehead, A., Cameron, A., Veloz, S., Kukkala, A., et al. (2019). Global synthesis of conservation studies reveals the importance of small habitat patches for biodiversity. PNAS 116, 909–914. doi: 10.1073/pnas.1813051115
Wirsing, A. J., Heithaus, M. R., Brown, J. S., Kotler, B. P., and Schmitz, O. J. (2021). The context dependence of non-consumptive predator effects. Ecol. Lett. 24, 113–129. doi: 10.1111/ele.13614
Wolf, M., and Weissing, F. J. (2012). Animal personalities: consequences for ecology and evolution. Trends in Ecology & Evolution, 27, 452–461.
Young, J. K., Olson, K. A., Reading, R. P., Amgalanbaatar, S., and Berger, J. (2011). Is wildlife going to the dogs? Impacts of feral and free-roaming dogs on wildlife populations. Bioscience 61, 125–132. doi: 10.1525/bio.2011.61.2.7
Keywords: risk-sensitive foraging, urban biodiversity, landscape of fear, ecology of fear, stress, Felis catus, pest management, urban conservation motivation
Citation: Fardell LL, Pavey CR and Dickman CR (2023) Influences of roaming domestic cats on wildlife activity in patchy urban environments. Front. Ecol. Evol. 11:1123355. doi: 10.3389/fevo.2023.1123355
Edited by:
Seth Magle, Lincoln Park Zoo, United StatesReviewed by:
Bill Bateman, Curtin University, AustraliaJames Stephen Adelman, University of Memphis, United States
Copyright © 2023 Fardell, Pavey and Dickman. This is an open-access article distributed under the terms of the Creative Commons Attribution License (CC BY). The use, distribution or reproduction in other forums is permitted, provided the original author(s) and the copyright owner(s) are credited and that the original publication in this journal is cited, in accordance with accepted academic practice. No use, distribution or reproduction is permitted which does not comply with these terms.
*Correspondence: Loren L. Fardell, ✉ bG9yZW4uZmFyZGVsbEBnbWFpbC5jb20=