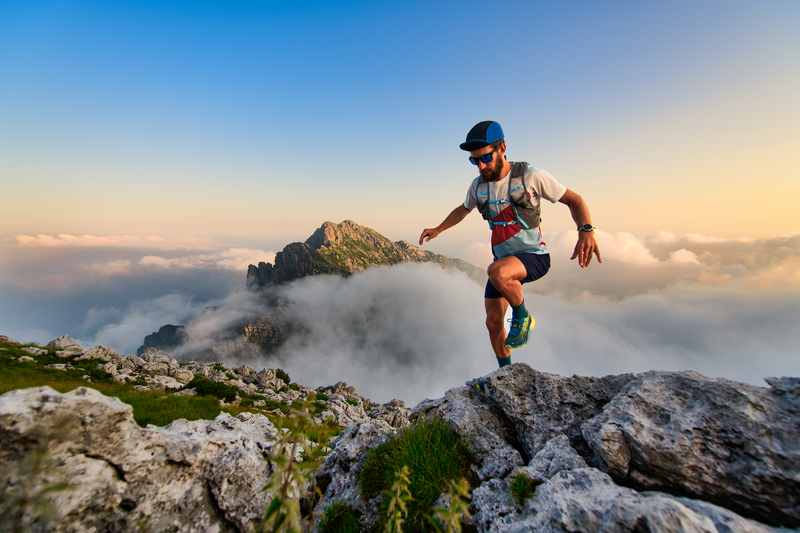
95% of researchers rate our articles as excellent or good
Learn more about the work of our research integrity team to safeguard the quality of each article we publish.
Find out more
ORIGINAL RESEARCH article
Front. Ecol. Evol. , 21 June 2023
Sec. Ecophysiology
Volume 11 - 2023 | https://doi.org/10.3389/fevo.2023.1113357
This article is part of the Research Topic Marine Omics in a Changing Ocean: Modelling Molecular Pathways and Networks to Understand Species Acclimation and Adaptation View all 10 articles
The symbiotic relationship between coral and its endosymbiotic algae, Symbiodiniaceae, greatly influences the hosts’ potential to withstand environmental stress. To date, the effects of climate change on this relationship has primarily focused on adult corals. Uncovering the effects of environmental stress on the establishment and development of this symbiosis in early life stages is critical for predicting how corals may respond to climate change. To determine the impacts of future climate projections on the establishment of symbionts in juvenile corals, ITS2 amplicon sequencing of single coral juveniles was applied to Goniastrea retiformis and Acropora millepora before and after exposure to three climate conditions of varying temperature and pCO2 levels (current and RCP8.5 in 2050 and 2100). Compared to ambient conditions, juvenile corals experienced shuffling in the relative abundance of Cladocopium (C1m, decrease) to Durusdinium (D1 and D1a, increase) over time. We calculated a novel risk metric incorporating functional redundancy and likelihood of impact on host physiology to identify the loss of D1a as a “low risk” to the coral compared to the loss of “higher risk” taxa like D1 and C1m. Although the increase in stress tolerant Durusdinium under future warming was encouraging for A. millepora, by 2100, G. retiformis communities displayed signs of symbiosis de-regulation, suggesting this acclimatory mechanism may have species-specific thresholds. Whilst this study cannot specifically disentangle the individual effects of temperature and pCO2, it does provide valuable insights into the impacts of both stressors combined. These results emphasize the need for understanding of long-term effects of climate change induced stress on coral juveniles, and their potential for increased acclimation to heat tolerance through changes in symbiosis.
Increasing global temperatures due to anthropogenic climate change is currently the greatest threat to coral survival and has resulted in three global mass bleaching events since the 1980s (1998, 2010, 2014–2017), with bleaching becoming ever more prevalent and extreme (Hughes et al., 2017b). Coral bleaching occurs when endosymbiotic dinoflagellates (family Symbiodiniaceae) are lost from the host coral tissue. This stress response is seen as a loss of the endosymbiont due to a breakdown of the symbiotic relationship (Brown, 1997). As Symbiodiniaceae support their host’s energy demands through the provisioning of photosynthates, the prolonged loss of this relationship may cause starvation, and lead to coral mortality (Brown, 1997; Suggett et al., 2017).
Known stressors of coral symbioses include, but are not limited to, increased sea surface temperatures, and increased partial pressure of carbon dioxide (pCO2). Elevated temperatures are linked to damage of the photosystems of coral endosymbionts, shifting the energetic demands and outputs of the symbiont (Fitt et al., 2001; Robison and Warner, 2006). This can take on a similar form to “parasitism” (sensu Baker et al., 2018), as it reduces the photosynthates translocated to the host (Baker et al., 2018). As a result, the host physiology suffers as the coral works to make up for a lack of energy by reaching into other energy storages. While the coral host can turn to heterotrophy to meet some energy demands, there is variation across coral species as to the level to which it is able to accommodate symbiont loss (Anthony et al., 2009). In addition, the increase in pCO2 into the oceans has been predicted to impact various physiological responses in both the coral host and Symbiodiniaceae (Cohen and Holcomb, 2009; Schoepf et al., 2013). While decreased calcification rates have been well-studied, other responses such as changes in respiration, and metabolism of the host and symbiont may also be impacted by increases in pCO2 similar, to other phenotypic measures when examined under predicted future climate scenarios (Anthony et al., 2008).
Both stressors have grave implications for coral early life history stages, where energy demands are potentially high during skeletal development and growth as well as during symbiosis establishment. For example, calcification may also be more critical and at-risk during this stage when a coral juvenile is first recruiting and secreting skeletal structures (Foster et al., 2016). The high rates of ocean warming and pCO2 coupled with the high incidence of bleaching events may be too rapid to allow for natural adaptation to occur (Hoegh-Guldberg et al., 2007; Schoepf et al., 2013; Hughes et al., 2017a). Understanding the effects of climate change on coral–Symbiodiniaceae interactions in early life stages is therefore critical for understanding growth and recovery potentials on reefs, and predicting coral reef resilience to future climate.
Due to the critical role of the endosymbiotic Symbiodiniaceae in energy translocation within the coral host, there has been an emphasis on increased taxonomic resolution of the Symbiodiniaceae present in the host using genetic markers such as the internal transcribed spacer 2 (ITS2) region (Pochon et al., 2014; LaJeunesse et al., 2018; Davies et al., 2022). Symbiodiniaceae are comprised of 9 currently identified genera (formerly clades), each of which is subset into species (formerly “types”) (LaJeunesse et al., 2018). Host environmental sensitivity often varies according to the predominant algal symbiont or community (Rowan et al., 1997). For example, colonies with different dominant Symbiodiniaceae communities experienced different bleaching patterns in locations that correlated to predicted limits of symbiont distributions (Rowan et al., 1997; Quigley et al., 2017; Claar et al., 2020; Quigley et al., 2022). These predicted limits of coral symbionts are based on observations where some Symbiodiniaceae taxa are shown to out-perform others, e.g. Durusdinium showing greater thermal tolerance than Cladocopium. In one example, adults of Acropora millepora exhibited changes in Symbiodiniaceae relative abundance towards Durusdinium that corresponded to an increase in host heat tolerance by 1–1.5 °C (Berkelmans and van Oppen, 2006). Despite few studies assessing the independent role of pCO2 on Symbiodiniaceae communities, there have been indications of the physiological effects to coral hosts which may be attributed to differences in symbiont taxa and community composition (Howe-Kerr et al., 2020). Finally, the dominance of specific Symbiodiniaceae genera within the coral host can also promote greater resilience under environmental stressors like increased sea surface temperatures or to other stressors present in the environment (Baker et al., 2004; Berkelmans and van Oppen, 2006; Stat and Gates, 2008; Stat et al., 2008; Oliver and Palumbi, 2011).
While the change in relative abundances (termed shuffling) of specific symbiont taxa may improve adult coral tolerance to environmental stress, it is less understood if symbiont shuffling is pervasive in coral early life-history stages (Reich et al., 2017; Quigley et al., 2019). Coral juveniles generally establish their initial endosymbiont community either through direct transmission from the maternal colony (vertical transmission), or via environmental acquisition (horizontal transmission) (Baird et al., 2009) or a combination of the two (mixed-mode transmission) (Quigley et al., 2018b). Vertical symbiont establishment provides the opportunity for larvae and subsequent juveniles to acquire a potentially advantageous community of endosymbionts best suited for the local, maternal environment through parental adaptation (Padilla-Gamiño et al., 2012; Quigley et al., 2016; Poland and Coffroth, 2017). Conversely, horizontal transmission can occur through other environmental sources, and it allows for more flexibility to acquire novel symbionts that could be advantageous under changing environmental conditions or after large dispersal distances (Lewis and Coffroth, 2004; Yuyama et al., 2016). Horizontal transmission can occur through the water column, sediment, or other environmental means (McIlroy and Coffroth, 2017; Ali et al., 2019; Umeki et al., 2020). Some research has indicated that coral larvae are capable of selecting and integrating new symbionts from their environment into their endosymbiotic community (LaJeunesse, 2002; Rohwer et al., 2002; Coffroth et al., 2006; LaJeunesse et al., 2009) which may promote greater rates of symbiont acquisition in early life stages (Nitschke et al., 2015; Poland and Coffroth, 2017; Quigley et al., 2017). This ability of symbiont selection via environmental uptake allows for the transfer of beneficial symbiotic traits, and the improvement of coral tolerance to environmental stressors (Baker et al., 2004; Adams et al., 2009; Poland and Coffroth, 2017). Different Symbiodiniaceae taxa also provide the host with distinct benefits. For example, Durusdinium may provide increased survival to juveniles under simulated bleaching events (Quigley et al., 2020b; Quigley et al., 2020c). Further, growth rates vary in juveniles between those dominated by either Cladocopium or Durusdinium (Cantin et al., 2009; Yuyama and Higuchi, 2014; Quigley et al., 2020c), linked to differences in carbon translocation between genera (Cantin et al., 2009). Physiological differences also exist at finer taxonomic scales, for example, within Durusdinium “D1a” compared to “D1” might promote slightly greater photosystem II activity (Suggett et al., 2015).
Symbiont diversity varies across coral early life-history stages, in which the diversity of the symbiont community during coral juvenile development may also be important for growth, and survival. For example, there is some evidence that the acquisition of diverse communities of endosymbionts may promote higher growth rates, and increase survival (Yuyama and Higuchi, 2014). Compared to adult corals, early life-history stages (within 1 month of settlement) generally have more diverse endosymbiont communities (Quigley et al., 2017), which eventually winnow to a more stable, lower diversity community (Abrego et al., 2009; Lee et al., 2016; Rouzé et al., 2019). In some cases, symbiont communities in juvenile corals may be impacted by environmental factors. This includes the combined but not necessarily additive effects of temperature and pCO2, as well as increased temperature, and light (Abrego et al., 2012) or completely halted by increased temperatures with minimal impacts of pCO2 (Sun et al., 2020). Moreover, the acquisition of symbionts during coral early life-history stages has been assessed only for a limited number of coral species (Cantin et al., 2009; Yorifuji et al., 2017; Quigley et al., 2019; Quigley et al., 2020a), and the effects of elevated temperature and pCO2 on this process are poorly understood. Combined, these results highlight the importance of Symbiodiniaceae community diversity for the fitness of coral adult and early life-history stages, and highlight an increased need for a better understanding of fine-scale host–symbiont interactions.
To assess changes in Symbiodiniaceae acquisition during coral developmental stages under future climate scenarios, two horizontally transmitting coral species, Goniastrea retiformis and Acropora millepora, were exposed to varying levels of temperature and pCO2 predicted for the years 2050 (+1°C offset, pCO2 685 ± 60ppm) and 2100 (+2°C offset, pCO2 940 ± 60ppm) under the IPCC RCP 8.5 pathway for projected greenhouse gas concentrations, and compared to present day levels (28.5°C, pCO2 400 ± 60ppm). These coral species represent different known stress tolerance levels of both stressors, as G. retiformis is recognized as being thermally tolerant compared to stress-sensitive A. millepora and so were selected to better understand how different coral species may respond to future climate conditions. Juveniles were sampled and the ITS2 region of the symbionts was targeted using amplicon sequencing. The dynamics of symbiont community changes (prevalence, relative abundance, and diversity) were assessed under exposure to these multiple climate treatments for these coral juveniles and further assessed over time (0, 10 days and 4 weeks) for stress-tolerant G. retiformis or only at the final timepoint for A. millepora.
In October 2017, gravid colonies of G. retiformis and A. millepora were collected from Geoffrey Bay at Magnetic Island (S 19°09.326′, E 146°51.861′; Great Barrier Reef Marine Park Authority Permit Number: G13/36318.1), and transported to holding outdoor mesocosm tanks at the National Sea Simulator (SeaSim) at the Australian Institute of Marine Science as per details outlined in (Botté et al., 2020; Uthicke et al., 2020). Corals were acclimated to the following conditions (~27°C, pCO2 400 ± 60ppm) until spawning (G. retiformis: 8th of November 2017; A. millepora: 12th December 2017). Multiple colonies were collected, spawned during the spawning night, and mixed in bulk cultures (exact numbers per culture were not recorded). Following spawning, gametes were collected, mixed for fertilization, and larvae were reared in mass culture tanks for 3–4 weeks. Larval culturing followed established methods (Quigley et al., 2016; Pollock et al., 2017) after fertilization success was checked by visually inspecting embryos every hour for up to three hours under the magnification of a benchtop microscope. Larvae were maintained in 500 L flow-through culture tanks at a density of 1–1.2 larva mL−1, filled with 0.2 μm filtered seawater (Pollock et al., 2017).
Larvae (n = 20 per well, 60 per plate) were then distributed to sterile, 6-well plates filled with 0.2 μm filtered seawater containing autoclaved crustose coralline algae (CCA) to induce larval settlement. These plates were not exposed to flow-through conditions, instead they were filled with water, larvae added, and then lid replaced, and left overnight in the dark for larvae to settle. At the first sampling time point of newly settled recruits (T0), individuals were sampled using a sterile scalpel, preserved in absolute ethanol in Eppendorf tubes with minimal water transfer to ensure adequate preservation, and minimize any chance of transfer of symbionts in the water (n = 3 individuals per tube). Following initial sampling, the 6-well plates were placed in outdoor mesocosm tanks (n = 3 tank replicates per treatment; 2 × 6-well plates per tank for each species) representing ambient (present day ~28.5°C, pCO2 400 ± 60ppm), 2050 (+1°C offset, pCO2 685 ± 60ppm), and 2100 (+2°C offset, pCO2 940 ± 60ppm) conditions forecast under RCP 8.5 (Meinshausen et al., 2011; Collins et al., 2013) (Supplementary Table 1). As outlined in previous studies (Botté et al., 2020; Uthicke et al., 2020), these mesocosm tanks had a range of flora and fauna, including fish, corals, seagrasses, and benthic sediments. Sampling was repeated across each tank after 10 days for both of the coral species in this study, at T1 (G. retiformis, A. millepora), and 4 weeks (G. retiformis) or 5 weeks (A. millepora) (T2) of exposure to simulated climate conditions. All samples were stored at −80°C prior to DNA extraction and ITS2 amplicon sequencing of Symbiodiniaceae communities. However, due to lack of gel electrophoresis bands present in T0 and T1 A. millepora samples following PCR amplification, these time points were not sequenced (see “DNA extraction and sequencing” section). No other samples (e.g. water or sediment samples) were collected for sequencing. Juvenile survival was not monitored in this study.
The ambient treatment in this experiment represented average reef conditions over the past ~20 years at a well-studied central Great Barrier Reef site (Davies Reef), allowing for comparisons of symbiont acquisition under elevated temperatures and pCO2 expected by 2050, and 2100 under RCP 8.5. Corals generally live close to their thermal maximums, and +1 and +2 degrees of temperature increases impact coral offspring physiology and that of their symbionts (Abrego et al., 2012) suggesting that these treatments represent a “stress” for coral offspring.
Genomic DNA was extracted from G. retiformis and A. millepora samples across three sampling times (T0, T1 and T2), and treatments (ambient, 2050, 2100). Note that the T0 sampling timepoint occurred immediately before juveniles were placed into the three experimental treatments. DNA extractions were performed on individual coral juveniles using a KOH-EDTA method following (Sun et al., 2014), with increased incubation time (15 minutes at 70°C) to enhance cellular lysis.
Polymerase Chain Reaction (PCR) amplification of the ITS-2 locus were conducted using MyTaq DNA Polymerase (Bioline) following manufacturer’s protocols with an addition of 1µl 50mM magnesium. The ITS-2 locus was amplified using ITS2alg-F (5’-TCGTCGGCAGCGTCAGATGTGTATAAGAG ACAGGTGAATTGCAGAACTCCGTG) and ITS2alg-R(3’-TTCGTATATTCATTCGCCTCCGACAGAGAATATGTGTAGAGGCTCGGGTGCTCTG-5’) primers. PCR was performed in 25µl reactions (2µL template) per sample. PCR conditions were the following: initial denaturation at 95°C for 10 minutes, 32 cycles at 95°C for 30 sec, 59°C for 60 sec, 72°C for 30 sec, and a final elongation at 72°C for 7 minutes. PCR amplification products were delivered to the Ramaciotti Centre for Genomics (UNSW, Sydney) for Miseq amplicon sequencing of the 300 bp ITS-2 region (LaJeunesse, 2001; LaJeunesse, 2002). No bands were retrieved during PCR amplification in A. millepora samples at T0 and T1 although optimizations were attempted. This was likely due to the extremely low densities of symbionts in these first days of symbiosis establishment, as low density has been demonstrated in other studies of A. millepora symbiosis at 40 days (Quigley et al., 2018a). Sequencing was therefore only conducted on A. millepora samples for T2.
A total of 135 individual juvenile samples were successfully sequenced (some samples were removed if PCRs did not result in bands). Of these successfully sequenced juveniles, 27 samples belonged to A. millepora, and 110 belonged to G. retiformis (see Supplementary Table 2).
Symbiodiniaceae taxonomy is currently undergoing an extensive revision (LaJeunesse et al., 2018) with substantial effort to understand how sequence diversity links with species, genus, and family designations, and most importantly, the functional significance of the symbiont within the coral. Presently, there are generally two analysis pipelines. The first groups sequences within genera (Symportal; Hume et al., 2019), and is generally good for high-abundance taxa and making broad inferences across treatments. The other is based on characterizing individual sequence variants using DADA2 (ASVs; Callahan et al., 2016; Quigley et al., 2019), and provides a higher resolution at the sequence level for exploration of potential diversity at both high and low abundances. The choice of method depends on the question being asked, where both will not render species – or functional – level designations with a formal taxonomic description. Here we chose to use the DADA2 method given our questions about the earliest uptake of symbiont cells and acknowledge that some of the ASVs discovered may be spurious sequence variants and may not represent actual Symbiodiniaceae “species” per-se. The pros and cons of each method and the justification for their use are outlined more fully in (Davies et al., 2022), and a formal comparison of the methods can be found in (Quigley et al., 2022). The DADA2 pipeline generates amplicon sequence variants based on ITS2 sequencing libraries. The ITS2 gene is highly replicated in Symbiodiniaceae and has high levels of intragenomic variation (see Quigley et al., 2014 for full discussion). The calling of ASVs therefore results in the recovery of a diversity of unique ASVs from each Symbiodiniaceae species, resulting in potentially artificially inflated diversity data (discussed in Davies et al., 2022), but offers a higher resolution approach compared to Symportal. Symportal (by design) collapses relevant species diversity when many congeneric Symbiodiniaceae species co-occur within many samples. This therefore represents an overall conservative approach, especially where we are principally focused on low-abundance, highly rare diversity given the life-stage and experimental question. However, to minimize the impact of spurious sequences, most of the analysis collapses diversity to higher levels and primarily focusses on the ten most abundant Symbiodiniaceae ASVs (>4.2% relative abundance cut-off). Neither the authors of this work nor the workshop participants formally endorse either pipeline. The full pipeline and scripts are described in (Quigley et al., 2019) and links therein. Briefly, fastq files were first filtered to remove any reads with retained sequencing adapters using BBDuk (BBMap, package 38.63 http://sourceforge.net/projects/bbmap/) (Bushnell, 2017) in R (R Core Team, 2013). Based on quality filtering using BBDuk, reads were then assessed for low quality were trimmed by removing reads without an overlap of 30 bp and one expected error. Dereplication of reads grouped unique sequences, which eliminates any redundant comparisons in the pipeline. Culling spurious sequence variants by merging denoised forward and reverse reads and removing none-overlapping paired reads further helps to remove spurious sequences. Error rates for forward and reverse reads are learnt, both ends are merged and used as input into the DADA2 naive RDP’s Bayesian classifier to assign amplicon sequence variants (hereafter ASVs). After chimera removal of 2 samples (135 remaining), the assigned taxonomy function within DADA2 was used to classify ASVs to known Symbiodiniaceae sequences from the updated GeoSymbio ITS2 database (Franklin et al., 2012). In essence, this procedure groupings are based on sequence similarity, with names derived from the literature as represented in the updated GeoSymbio database, but do not correspond with strict taxonomic designations, i.e. no binomial species name. Bootstrapping at a threshold of 50 allowed for maximum retention of sequences while minimizing low quality matches. Here we refer to ASVs when discussing the sequence level, and “types” when discussing multiple ASVs that are assigned to the same Symbiodiniaceae taxa (i.e “ASVs” ASV1_C1 and ASV2_C1 are collapsed into “type” C1). On average, 100,801.90 ± 4,212.15 sequences were retained in each sample after quality filtering.
Percent relative abundances of each ASV given the total number of cleaned reads were calculated per sample using the abundance (compositional) function in the package “microbiome” (v. 1.10.0) (Lahti et al., 2017). Alpha and beta diversity metrics were calculated using the relative abundance data in “Phyloseq” (v. 1.30.0, McMurdie and Holmes, 2013). Alpha diversity was measured using the Shannon index, and linear mixed models were performed to test differences in diversity across time, treatments and species using the R package lmerTest (Kuznetsova et al., 2017). The assumptions of normality, homogeneity of variance and linearity were tested using the DHARMA (Hartig and Hartig, 2021). To test for differences in diversity across time (T0, T1, T2) and treatments (ambient, 2050, 2100) in G. retiformis, a linear mixed model that included time and treatment as fixed effects (and their interactions) and tank as a random effect were performed. For A. millepora, the effect of climate treatment on diversity was tested using a linear mixed model using treatment as fixed factor and tank as random factor on square-root transformed data. To test for differences in alpha diversity between species at T2, a linear mixed model with species and treatment (and their interaction) as fixed factors and tank as random factor was run. Post-hoc multiple comparisons adjusted by the False Discovery Rate (Benjamini and Hochberg, 1995) were run in case of significant interactions (or factors) using the R package multcomp (Hothorn et al., 2008). To test for significant differences in the relative abundances of each ASV across treatments, the packages DESeq (v. 1.39.0) and DESeq2 (v. 1.26.0) (Love et al., 2014) were used. Generalized linear models with time (T0, T1, T2) and treatment (ambient, 2050, 2100) as factors were run for 30 iterations, where then non-converging models were filtered prior to multiple test correcting. Significantly differential abundant ASVs were then aligned using Clustal Omega (Sievers et al., 2011).
A risk metric was developed to better understand how the loss or gain of different Symbiodiniaceae taxa could impact host functioning. Taxa of interest were selected based on their total average abundance, overall function profile that included significant shifts between timepoints (ranked redundancy value) and heat tolerance using a “thermal sensitivity” rating (Rk value) determined by (Swain et al., 2016). First, 16 most abundant ASVs were selected. Abundance was determined for each by taking the average sequence reads across the dataset and then selecting the highest average 16 scores.
The ranked redundancy profile was determined by first assigning a function profile for each of the 16 most abundant taxa. To do this, a review of the literature was performed for those selected 16 ASVs to determine a functional redundancy ranking. This literature review rendered health-associated measurements derived from 17 published experimental studies. Source of stress were calculated for each type based on measurements from the literature from these 17 publications (Supplementary Table 3). These health-associated measurements included: Fv/Fm, chlorophyll content, lipid assessment, Photosystem I function relative to photosystem II function, dark oxygen consumption, ratio of photosynthesis to respiration, DMSP concentration, DMSO activity, glutanthione activity, catalase-like activity, superoxide dismutase activity, bleaching response, light pressure response, rate of photosynthesis, experiment type, maximum excitation pressure, and growth. For those publications which measured a value both before and after a stress treatment, the average change across each measurement for each taxon was calculated to assign a rank value. For example, this rendered the difference in Fv/Fm values for D1a before and after a stress treatment. If the study associated this difference with a positive outcome for the symbiont or the host, this change in value was ranked positive (“healthy” or “resilient” host–symbiont interaction). Therefore, each measurement was associated with an average change value, where a change of −0.1 (the smallest change value calculated) was ranked as “1st” (less change) and a change of −0.51 (the largest change value calculated) was ranked as “13th” (more change). Therefore, we define a positive experimental outcome with a small change, compared to a large change, to indicate a greater resilience to stress. For publications with single measurements (only a before or after measurement), ranks were based on the value measurements only. For example, chlorophyll content (Chl a + c2) was ranked from high to low values. To address differences in the number of observations for each measurement and for each taxon, a “point” was given per observation and then the total number of observations for each measurement was divided by the total number of observations to render a relative point score. Taking the example of Fv/Fm and D1a above, this rendered a final relative score of 0.333. This resulted in a ranked redundancy score that included the functional profile, the relative change in measurements that make up the functional profile, and a positive or negative outcome associated with that change. We assume that if the experimental outcome was positive, a higher change is representative of a shift towards greater tolerance.
Thermal sensitivity scores (Rk values) were derived from Swain et al. (2016). The Borda Rank method (Borda, 1781) was used to calculate the final ranking of Rk values and averaged to result in a relative ranking per function per taxa (from “1” being an important taxon in terms of function to “21”, being a less important taxa for function) (Supplementary Tables 4, 5). Finally, with these two derived metrics (ranked redundancy and Rk), taxa were categorized into 4 risk categories: High Function, High Sensitivity (High Risk, red points), Low Function, High Sensitivity or High Function (Medium Risk, green points), Low Sensitivity (Medium Risk, green points), and Low Function, Low Sensitivity (Low Risk, blue points). Therefore, a taxon that is considered high risk of loss (lower left) are those taxa that contribute to a large number of functions that are unique (low redundancy) in the coral and that also tend to undergo a large change in thermal sensitivity under heat stress. If lost from the coral, these taxa would potentially have the greatest impact on host function due to their low redundancy and high functional importance. Taxa colored in black were ranked based on only one value for the calculation of the Rk value.
Adult G. retiformis are typically dominated by Symbiodiniaceae of the genus Cladocopium with the lowered abundance of Durusdinium as “background” taxa (Leveque et al., 2019). However, studies conducted on other coral species suggest that under elevated temperatures, an increase in more thermally tolerant Durusdinium could occur (Baker et al., 2004; Claar et al., 2020; Quigley et al., 2022). Like conspecific adults, G. retiformis juveniles in this study were typically dominated by Cladocopium taxa, but only at T0, although the cumulative abundance of all Cladocopium taxa remained higher in juveniles through time under ambient conditions – compared to the higher temperature and pCO2 2050 and 2100 treatments (Figures 1, 2). At T1 and T2, Durusdinium (averaged across all taxa in that genus) were found at higher relative abundance (20–23%, Table 1), especially in the 2050 treatment, which was accompanied by a decrease in Cladocopium relative abundance (from 56% to as low as 2%, Table 1). This pattern was evident in both the T1 and T2 timepoints, which may be due to the extended duration of stress at both high temperature and pCO2 or juvenile ontogeny and winnowing of symbiont communities.
Figure 1 Effects of elevated temperature and pCO2 on Symbiodiniaceae communities in coral juveniles of Acropora millepora and Goniastrea retiformis. Corals were sampled for ITS2 amplicon sequencing at 14 days post-settlement (T0; before exposure to treatment conditions), 10 days (T1) and four (G. retiformis) or five (A. millepora) weeks (T2) of exposure to 2050 (+1°C offset, pCO2 685 ± 60ppm) and 2100 (+2°C offset, pCO2 940 ± 60ppm) conditions. For A. millepora, only samples collected at T2 were sequenced, samples of A. millepora were not sequenced (NS) at T0 or T1 (see detailed information in Materials and Methods). The ten most abundant Symbiodiniaceae taxa (>4.2% relative abundance cut-off) across juveniles are shown here.
Figure 2 Relative abundance of the dominant Symbiodiniaceae taxa in Goniastrea retiformis juveniles over time and across climate treatments. (A) Mean relative abundance of the top Symbiodiniaceae taxa at T0 (before exposure to treatment conditions), T1 (10-day exposure), T2 (4-week exposure) under ambient, 2050 and 2100 conditions. (B) Proportion of coral juveniles associated with each dominant Symbiodiniaceae taxa under climate treatments over time (T0, T1, T2).
Table 1 Relative abundance of the dominant Cladocopium and Durusdinium taxa across both species, timepoints and stress exposures (note no data was available for A. millepora for T0 and T1).
As the average relative abundance of Cladocopium decreased through time (from 56% to as low as 2%, Table 1), this genus was replaced by Durusdinium or other ASV-identified taxa (e.g., B1g, I4) in the 2050 and 2100 scenarios at T2 (Figure 1). This could suggest that G. retiformis may shuffle their symbiont communities towards more heat or high pCO2 resistant taxa in the “shorter” term (up to 2050), taking on the classic shuffling pattern of Cladocopium to Durusdinium dominance. However, the future temperature and pCO2 conditions projected for 2100 may represent a threshold in which this acclimatory shuffling mechanism breaks down, manifesting as the lowered relative abundances of Cladocopium and Durusdinium and increases in potentially “non-symbiotic” taxa (Figures 1, 2). We also cannot exclude that the distinct Symbiodiniaceae communities observed at T0 (i.e. before recruits were exposed to treatment conditions) across treatments may have influenced the symbiont community dynamics under 2050 and 2100 conditions at the later time points. Further, a limitation to this study is the lack of survival data collected. It is therefore not known with certainty if juveniles with sub-optimal symbiont communities experienced mortality before sampling. Therefore, we cannot conclusively say that the 2050 or 2100 treatment caused any impact or mortality over our sampling period. However, given the vast literature on the impacts of heat and acidification on coral juvenile survival as well as the impacts of these treatments specifically on other reef organisms (Botté et al., 2020; Uthicke et al., 2020), it is likely that the 2050 and 2100 conditions had an impact on growth and survival in this study. Some of the changes seen here, which we ascribed to potential shuffling, could be due to juveniles with sub-optimal symbiont communities dying. Regardless, we were interested in characterizing climate change impacts on the symbiont communities across time and species, even if it was not the result of differential mortality. Additional assessment of survival could help in indicating at what stress levels corals experience shuffling or if the observed shifts were a result of survival of the fittest.
G. retiformis juveniles sampled from the ambient treatment at T0 (i.e. before recruits were exposed to treatment conditions) were dominated by Cladocopium (as discussed above), but more specifically, by C3k and C1f and background ASVs like C1m (mean relative of <1% of samples) (Figure 2). Interestingly, these abundances varied across the three treatments despite the T0 sampling occurring before treatment exposure. This may be due to high flexibility in early life stages (Coffroth et al., 2001; Little et al., 2004) in combination with a smaller sample size at T0 (see Supplementary Material). These may represent potential symbiotic partnerships and may be relevant to the changes seen at the later time points.
In the ambient treatment, there were also changes in Symbiodiniaceae relative abundances over time, including an increase in Durusdinium (from 0 to 11–23%, Table 1). The shuffling (i.e. change in the relative abundance) of specific Symbiodiniaceae were also observed in the ambient treatment, including D1, D1a, and C1m (present in >50% of samples), as well as in background ASVs (C50, C66, and B1g), observed from T0 to T1 (Figures 1, 2). After four weeks (T2) under ambient conditions, juveniles were dominated by D1, D1a and C1m, with background ASVs including C50, C66, and I4 (Figures 1, 2). At T0, before exposure to 2050 and 2100 conditions, juveniles were dominated by C50, D1, and C3k in 2050 treatments, and C3k and D1 in 2100 treatments, with background ASVs of D1a (2050), and C1m, C50, C1f, and C3.14 (2100) (Figure 1). After 10 days (T1) under 2050 and 2100 and 4 weeks (T2) at the 2050 treatment, Cladocopium relative abundances dropped and were replaced by Durusdinium ASVs, which were present across >75% of samples (Figure 2). By T2 in the 2100 treatment, more background types were represented in higher relative abundance, including D1.1, B1g, B20, I4, and F3.1a (Figure 1). This change toward Durusdinium-dominance may have been driven by juvenile age (or duration of temperature and pCO2 stress exposure), although climate treatment was the main driver of the change in individual Amplicon Sequence Variants (ASVs). For example, a total of 70 ASVs were differentially abundant in relation to treatment or treatment*species (Figure 3). Significant changes by ASVs and time were only related to changes in ASV abundance when the climate treatment interaction was included. This suggests that while juvenile age and duration of climate exposure may result in changes in the relative abundance of ASVs within the Symbiodiniaceae communities, climate treatments likely represent the main driver of symbiont community changes in our study.
Figure 3 Venn diagram incorporating only ASVs that significantly changed in relative abundance between treatments, time and species. A generalized linear model was run to determine which factors (time, treatment, species, or their interactions) explained the greatest variation in ASV abundance using the package DESeq and DESeq2 (v.1.26.0). Specifically, species*time corresponds to: A. millepora (T2) and in G. retiformis (T0, T1, T2). There were no ASVs that were significantly associated with the time*treatment interaction and are not illustrated in the figure.
Acropora millepora juveniles (sequenced only at five weeks, T2, see Materials and Methods), were dominated by Durusdinium (D1 and D1a) across climate treatments (Figures 1, 4). ASVs associated with Cladocopium decreased in relative abundances with increasing climate stress (from 64% to as low as 0% in the T1 2100 treatment, Table 1, Figures 1, 4). For example, the average relative abundance of Cladocopium C1m decreased with increasing stress in the 2050 and 2100 treatments (from as high as 25% at T1 ambient to close to 0% in other treatments, Figure 4). Likewise, other background ASVs were more abundant in the ambient treatment juveniles compared to those under 2050 and 2100 conditions, with 2100 samples comprising very few background ASVs.
Figure 4 Relative abundance of the dominant Symbiodiniaceae taxa in Acropora millepora juveniles following 5-week exposure to climate treatments. (A) Mean relative abundance of the most abundant Symbiodiniaceae taxa under ambient, 2050 and 2100 conditions. Dark blue colours represent values >45%. (B) Proportion of coral juveniles associated with each dominant Symbiodiniaceae taxa under climate treatments.
Our study examined the combined impacts of temperature and pCO2. However, our results parallel studies that looked at these stressors singularly. For example, the dominance of Durusdinium in our study from the combined climate stressors was consistent with other studies conducted on A. millepora juveniles, which showed a change towards Durusdinium-dominance at higher temperatures (Abrego et al., 2012). Similar patterns were also reported in adult corals of the same species, with an increased abundance of Durusdinium observed in corals exposed to elevated sea surface temperatures and/or human disturbances (Oliver and Palumbi, 2011; Sully et al., 2019; Claar et al., 2020; Quigley and van Oppen, 2022). It is important to note that although community changes towards Durusdinium may increase host thermal tolerance or a more generalized stress tolerance, it may concomitantly decrease growth rates (Little et al., 2004). Hence, there may be limited benefits of hosting Durusdinium for the coral A. millepora. Finally, the increased relative abundance of D1 and D1a in conjunction with the decreased abundance of background types in both the high temperature and high pCO2 2050 and 2100 treatments (for example, at T2, from <40% to >60%) suggests that A. millepora juveniles may have the ability to acclimate to these combined future climate conditions for at least short experimental periods. This demonstrated potential, if applicable in the wild, may promote greater survival under future climate scenarios, thereby increasing the opportunity for some coral species to survive through disturbance, like mass bleaching events combined with the impacts of acidification, to then go on to grow and reproduce and contribute to reef recovery.
Changes in the symbiont community in coral early life history stages have been observed in both field and lab-based experiments (Abrego et al., 2009; Quigley et al., 2017; Quigley et al., 2020a), in which the overall symbiont community diversity and composition can strongly influence host performance such as growth, survival and thermotolerance (Mieog et al., 2009). Endosymbiotic community diversity may also vary in response to environmental conditions, including temperature and nutrients (Gong et al., 2018). In our study, climate treatments did not impact diversity (as measured by the Shannon diversity index) within each coral species (p > 0.001; Figure 5). However, diversity in G. retiformis juveniles changed significantly over time, with an overall lower diversity at T1 compared to T2 (Lmer: F2,97 = 11.2, p < 0.01), though there were no significant differences between T0, and the later life stages. When comparing diversity between species at T2, mean diversity was higher in A. millepora compared to G. retiformis in juveniles under 2100 conditions (Lmer, species: F1,54 = 6.0, p = 0.02; species*treatment: F2,54 = 3.4, p = 0.04; G. retiformis 2100 – A. millepora 2100: p = 0.03). Taken together, these results suggest that at least at the final timepoint under 2100 conditions, Symbiodiniaceae communities in A. millepora were more diverse and more evenly distributed across individual juveniles compared to within G. retiformis, and importantly, they were shuffling towards a community of Durusdinium dominance.
Figure 5 Shannon diversity index in A. millepora (circle) and G. retiformis (square) juveniles across temperature and pCO2 treatments (ambient, 2050, 2100) and time (T0, T1, T2). The Shannon diversity metric was calculated using normalized ASVs abundances calculated for each individual juvenile using the R package Phyloseq. Samples of A. millepora were not sequenced (NS) at T0 and T1 for this species. Shannon diversity index differed significantly within each coral species (p > 0.001) and between species*conditions (species*treatment: p = 0.04) and over time for G. retiformis juveniles between T1 to T2 (p < 0.01). At T2 for 2100 conditions, A. millepora was significantly more diverse compared to G. retiformis (p = 0.03).
Durusdinium are of particular ecological interest due to their relative tolerance to stress compared to other symbionts (Morikawa and Palumbi, 2019). In our study, an increased abundance of Durusdinium (generally from 13% to 20–23%, Table 1) was observed through time (G. retiformis) and under climate stress (G. retiformis and A. millepora), and Durusdinium ASVs were the taxa most likely to change across climate treatments, including D. glynnii (15 ASVs to D1), and D. trenchii (9 ASVs to D1a). These shifts towards a Durusdinium-dominated community may be a consequence of the decrease in Cladocopium relative abundance, leaving available niches for Durusdinium through time or via competitive exclusion. Cladocopium ASVs also changed in abundance and included a total of 12 Cladocopium ASVs across our treatment conditions (Figure 4). The most common taxa that changed included C50 (4 ASVs), C1 (3 ASVs), and C1m (3 ASVs), mostly driven by climate treatment effects (Figure 3, Supplementary Figure 1). Interestingly, communities within the G. retiformis juveniles sampled from the 2100 treatment group changed over time from C50 (eight ASVs in T0 and T1) to C66 dominance (one ASV in T2 detected through outlier analysis). However, changes in C50 were more directly related to treatment effects whilst C66 changed by treatment*species interaction (Figure 3). While Cladocopium C66 has not been previously identified in Goniastrea adults (Leveque et al., 2019), it has been found at low abundance in corals (LaJeunesse et al., 2003). However, C66 has been reported from A. tenuis juveniles, with an increase of C66 over 71 days in the wild (Quigley et al., 2020a). This suggests that our T2 treatment may represent the initial establishment of a Cladocopium taxon containing the C66 marker and not an experimental artifact.
Further to consider is how different ASVs matching to Cladocopium species responded to different stress treatments (see Supplementary Figure 1). For instance, of the 4 ASVs designated C50, two reflected an increase in the relative abundances in response to increased thermal and pCO2 stress in A. millepora, with increases in G. retiformis corresponding more to time (up to ~12.5%, Figure 1). This could be a factor of variable host–symbiont interactions between species, however this would require sequencing of A. millepora at earlier timepoints, which was not deemed feasible due to a lack of PCR bands. In addition, Fugacium sp. (formerly clade F) is recognized as being a potentially important thermally tolerant taxon (Cunning et al., 2015). While only one ASV matching Fugacium (F5.2) was identified as significantly changing in response to treatment factors, this change was seen in the species*treatment factor (p = 0.025). F5.2 was present in the greatest relative abundance in G. retiformis juveniles under ambient conditions and were alternatively at the lowest relative abundances in ambient A. millepora. This finding demonstrates the species-specific nature of host–symbiont relationships and further emphasizes the need to investigate symbiosis establishment across multiple coral taxa.
Symbiodiniaceae taxa are functionally diverse (Suggett et al., 2015; Swain et al., 2016) with different taxa performing diverse functions within the coral host (Muscatine, 1990). The loss or gain of particular taxa could, as a result, cause physiological changes in host functioning. This change of function may include changes to nutrient transfer (e.g. carbon translocation) or the increase or decrease in tolerance of the host to a stressor. To infer potential consequences on host functioning due to the taxonomic changes measured here, we developed a risk metric that incorporated 16 of the most highly abundant symbiont ASVs in our coral juveniles and their functional roles from the literature, to calculate a “risk” metric that would result from the loss of taxa relative to the redundancy of their functional roles (Figure 6). This metric also incorporates the metric Rk (sensu Swain et al., 2016, Supplementary Table 5), where a higher Rk value is indicative of increased thermal tolerance. This metric shows that Cladocopium ASVs vary in their sensitivity to high temperatures. For example, Cladocopium goreaui (C1) are more thermally-sensitive (Rk = 21.72) compared to C40 (Rk = 55.32), which are more tolerant. This was combined with relative abundance data from this study, for example, in ASVs like C40 that experienced dramatic changes in relative abundance between ambient and climate stress treatments (Rk shift = 55). Redundancy within juveniles for this taxon was also relatively low (redundancy rank = 6). Combined, this resulted in the assignment of this taxon to a “medium risk” category if lost (green points, Figure 6). This suggests that given C40’s relatively high heat tolerance and potential ability to provide the coral host with greater stress resilience, the loss of this symbiont could be detrimental to host health given its low functional redundancy.
Figure 6 Risk metric quantifying the potential risk associated with the breakdown of host functioning due to Symbiodiniaceae loss. The metric included the 16 most highly abundant Symbiodiniaceae ASVs and included rankings of the likelihood of shifts (x-axis; Rk score) and functional redundancy of each taxon (y-axis). Rk values are from Swain et al. (2016) which measure the thermo-sensitivity of taxa. Redundancy rankings were calculated based on multiple health-associated measurements (e.g. Fv/Fm, chlorophyll content, lipid assessment) derived from 17 published experimental studies. The 16 ASVs were selected because they were the most abundant and were the ASVs which overlapped in both our study and those assessed for ranking. For each taxon, measurements were averaged across all studies per trait, with each function per taxa ranked from smallest to largest change, where a small change is indicative of greater redundancy and potential resilience of the host against stress. Final rankings were scored using a Borda Rank method and averaged to result in a relative ranking per function per taxa (from 1: important taxa for function – 21: less important taxa for function). Based on these two metrics, taxa were broken up into High (red points), Medium (green points), and Low (blue points) risk categories. For example, taxa in the High risk (lower left quadrat) identifies taxa which lost would have the greatest risk to impact host functioning due to their low redundancy and high functional importance. Taxa placed along the axis and colored in black only have one Rk value.
This same analysis was repeated for each of the 16 most highly abundant symbiont ASVs. Durusdinium trenchii (D1a), also generally known as a stress tolerant symbiont, had a correspondingly high Rk (37.03) and experienced relatively large changes in relative abundance between treatments. However, functional redundancy was high (~13), suggesting that there is a diminished risk to host health if this taxon is lost. Other ASVs like C1, C1b, and D4 have low thermal tolerance, experienced large changes in relative abundance, and had low functional redundancy, suggesting the loss of these ASVs are of greater risk to the host. Combined, this novel risk framework suggests that the loss or gain of different symbionts may not be functionally equivalent across these simulated future climate treatments and that each loss of particular symbionts may impact the host through downstream impacts on host function.
These outcomes may also be species-dependent, where some coral species may rely more heavily upon specific Symbiodiniaceae taxa to fulfill certain functions. It is also important to note that the majority of data for the Rk metric used in this risk assessment is derived from coral adults. Given we focus here on juveniles, we underscore those functions may be different across life-stages. Despite this, the use of the risk metric to understand the potential implications of loss and gain of symbiont taxa under future climate scenarios opens up the opportunity to better predict coral reef function in the future. The loss of high-functioning and low redundancy taxa, in particular, highlight the need to better protect reefs in order to minimize loss of these critical symbionts at critical early life stages.
As alluded to in the Materials and Methods, Symbiodiniaceae taxonomy is challenging given the Symbiodiniaceae ITS2 gene is multicopy with high intragenomic variation (see Quigley et al., 2014 for full discussion). The choice of the DADA2 pipeline may therefore overinflate diversity but minimizes the chance of false-negatives that would be possible from the Symportal pipeline. To address these challenges, a workshop was convened to discuss strategies to move forward amongst experts in the field (Davies et al., 2022). In this work, the pros and cons of the ASV vs. DIV methods were presented, including how the methodology relates to how sequence and species diversity are being treated. Other work has undertaken formal comparisons of these two methods (during the review Quigley et al., 2019; published Quigley et al., 2022), and in both, we confirmed that the results are largely consistent between the ASV and DIV methods. However, and most importantly, we specifically chose the ASV method here for its superior ability to detect novel, low-abundance sequences (Davies et al., 2022).
Overall, we observed an increase in relative abundance of Durusdinium over time in relation to juvenile age and duration of climate stress exposure. In addition, we found a significant capacity for coral juveniles to take up D1 and D1a symbionts at treatments with increasing temperature and pCO2, which generally corresponded with a decrease in Cladocopium. These results support the idea of “shuffling” algal symbiont communities for increased acclimation to stress, as seen in studies of adult corals. Longer-term observations of coral juveniles are required to understand the ontogenic consequences of temperature and pCO2 on symbiosis as it may provide coral offspring with an increased capacity for heat tolerance and survival.
The datasets presented in this study can be found in online repositories. The names of the repository/repositories and accession number(s) can be found in the article/Supplementary Material.
AT and KQ wrote manuscript. AT performed the lab work. KQ and NW developed the research idea; KQ and EM designed the experiment and sampling strategy and performed the experiment. AT ran the data analysis. NW and KQ provided funding. All authors contributed to the article and approved the submitted version.
Funding was provided by: AIMS@JCU, James Cook University, the Australian Institute of Marine Science.
This study was part of the Evolution21 project funded by AIMS and we are grateful to the AIMS SeaSim staff and volunteers who helped to collect the colonies, spawn the corals, and carry out the husbandry and experimental set-up and maintenance. The authors acknowledge the Gurambilbarra Wulgurukaba Traditional Owners of Magnetic Island, and pay our respects to their Elders, past, present, and emerging.
The authors declare that the research was conducted in the absence of any commercial or financial relationships that could be construed as a potential conflict of interest.
All claims expressed in this article are solely those of the authors and do not necessarily represent those of their affiliated organizations, or those of the publisher, the editors and the reviewers. Any product that may be evaluated in this article, or claim that may be made by its manufacturer, is not guaranteed or endorsed by the publisher.
The Supplementary Material for this article can be found online at: https://www.frontiersin.org/articles/10.3389/fevo.2023.1113357/full#supplementary-material
Abrego D., van Oppen M. J. H., Willis B. L. (2009). Onset of algal endosymbiont specificity varies among closely related species of acropora corals during early ontogeny. Mol. Ecol. 18, 3532–3543. doi: 10.1111/j.1365-294X.2009.04276.x
Abrego D., Willis B. L., van Oppen M. J. H. (2012). Impact of light and temperature on the uptake of algal symbionts by coral juveniles. PLoS One 7, e50311. doi: 10.1371/journal.pone.0050311
Adams L. M., Cumbo V., Takabayashi M. (2009). Exposure to sediment enhances primary acquisition of Symbiodinium by asymbiotic coral larvae. Mar. Ecol. Prog. Ser. 377, 156–179. doi: 10.3354/meps07834
Ali A., Kriefall N. G., Emery L. E., Kenkel C. D., Matz M. V., Davies S. W. (2019). Recruit symbiosis establishment and symbiodiniaceae composition influenced by adult corals and reef sediment. Coral Reefs 38, 405–415. doi: 10.1007/s00338-019-01790-z
Anthony K., Hoogenboom M. O., Maynard J. A., Grottoli A. G., Middlebrook R. (2009). Energetics approach to predicting mortality risk from environmental stress: a case study of coral bleaching. Funct. Ecol. 23, 539–550. doi: 10.1111/j.1365-2435.2008.01531.x
Anthony K. R. N., Kline D. I., Diaz-Pulido G., Dove S., Hoegh-Guldberg O. (2008). Ocean acidification causes bleaching and productivity loss in coral reef builders. Proc. Natl. Acad. Sci. 105, 17442–17446. doi: 10.1073/pnas.0804478105
Baird A. H., Bhagooli R., Ralph P. J., Takahashi S. (2009). Coral bleaching: the role of the host. Trends Ecol. Evol. 24, 16–20. doi: 10.1016/j.tree.2008.09.005
Baker D. M., Freeman C. J., Wong J. C. Y., Fogel M. L., Knowlton N. (2018). Climate change promotes parasitism in a coral symbiosis. ISME J. 12, 921. doi: 10.1038/s41396-018-0046-8
Baker A. C., Starger C. J., McClanahan T. R., Glynn P. W. (2004). Corals’ adaptive response to climate change. Nature 430, 741. doi: 10.1038/430741a
Benjamini Y., Hochberg Y. (1995). Controlling the false discovery rate: a practical and powerful approach to multiple testing. J. R. Stat. society. Ser. B (Methodological) 57 (1), 289–300. doi: 10.1111/j.2517-6161.1995.tb02031.x
Berkelmans R., van Oppen M. J. H. (2006). The role of zooxanthellae in the thermal tolerance of corals: a “nugget of hope” for coral reefs in an era of climate change. Proc. R. Soc. B: Biol. Sci. 273, 2305–2312. doi: 10.1098/rspb.2006.3567
Botté E. S., Luter H. M., Marangon E., Patel F., Uthicke S., Webster N. S. (2020). Simulated future conditions of ocean warming and acidification disrupt the microbiome of the calcifying foraminifera marginopora vertebralis across life stages. Environ. Microbiol. Rep. 12, 693–701. doi: 10.1111/1758-2229.12900
Brown B. E. (1997). Coral bleaching: causes and consequences. Coral Reefs 16, S129–S138. doi: 10.1007/s003380050249
Callahan B. J., McMurdie P. J., Rosen M. J., Han A. W., Johnson A. J. A., Holmes S. P. (2016). DADA2: high-resolution sample inference from illumina amplicon data. Nat. Methods 13, 581–583. doi: 10.1038/nmeth.3869
Cantin N., van Oppen M., Willis B., Mieog J., Negri A. (2009). Juvenile corals can acquire more carbon from high-performance algal symbionts. Coral Reefs 28, 405–414. doi: 10.1007/s00338-009-0478-8
Claar D. C., Starko S., Tietjen K. L., Epstein H. E., Cunning R., Cobb K. M., et al. (2020). Dynamic symbioses reveal pathways to coral survival through prolonged heatwaves. Nat. Commun. 11, 1–10. doi: 10.1038/s41467-020-19169-y
Coffroth M. A., Lewis C. F., Santos S. R., Weaver J. L. (2006). Environmental populations of symbiotic dinoflagellates in the genus Symbiodinium can initiate symbioses with reef cnidarians. Curr. Biol. 16, R985–R987. doi: 10.1016/j.cub.2006.10.049
Coffroth M. A., Santos S., Goulet T. (2001). Early ontogenetic expression of specificity in a cnidarian-algal symbiosis. Mar. Ecol. Prog. Ser. 222, 85–96. doi: 10.3354/meps222085
Cohen A. L., Holcomb M. (2009). Why corals care about ocean acidification: uncovering the mechanism. Oceanography 22, 118–127. doi: 10.5670/oceanog.2009.102
Collins M., Knutti R., Arblaster J., Dufresne J.-L., Fichefet T., Friedlingstein P., et al. (2013). “Long-term climate change: projections, commitments and irreversibility,” in Climate change 2013-the physical science basis: contribution of working group I to the fifth assessment report of the intergovernmental panel on climate change (Cambridge, UK and New York, USA: Cambridge University Press), 1029–1136.
Cunning R., Silverstein R. N., Baker A. C. (2015). Investigating the causes and consequences of symbiont shuffling in a multi-partner reef coral symbiosis under environmental change. Proc. R. Soc B 282, 20141725. doi: 10.1098/rspb.2014.1725
Davies S., Gamache M. H., Howe-Kerr L. I., Kriefall N. G., Baker A. C., Banaszak A. T., et al. (2022). Building consensus around the assessment and interpretation of symbiodiniaceae diversity. PeerJ 11, e15023. doi: 10.7717/peerj.15023
Fitt W. K., Brown B. E., Warner M. E., Dunne R. P. (2001). Coral bleaching: interpretation of thermal tolerance limits and thermal thresholds in tropical corals. Coral Reefs 20, 51–65. doi: 10.1007/s003380100146
Foster T., Falter J. L., McCulloch M. T., Clode P. L. (2016). Ocean acidification causes structural deformities in juvenile coral skeletons. Sci. Adv. 2, e1501130. doi: 10.1126/sciadv.1501130
Franklin E. C., Stat M., Pochon X., Putnam H. M., Gates R. D. (2012). GeoSymbio: a hybrid, cloud-based web application of global geospatial bioinformatics and ecoinformatics for Symbiodinium-host symbioses. Mol. Ecol. Resour 12, 369–373. doi: 10.1111/j.1755-0998.2011.03081.x
Gong S., Chai G., Xiao Y., Xu L., Yu K., Li J., et al. (2018). Flexible symbiotic associations of symbiodinium with five typical coral species in tropical and subtropical reef regions of the northern south China Sea. Front. Microbiol. 9, 2485. doi: 10.3389/fmicb.2018.02485
Hoegh-Guldberg O., Mumby P. J., Hooten a J., Steneck R. S., Greenfield P., Gomez E., et al. (2007). Coral reefs under rapid climate change and ocean acidification. Science 318, 1737–1742. doi: 10.1126/science.1152509
Hothorn T., Bretz F., Westfall P., Heiberger R. M. (2008) Multcomp: simultaneous inference for general linear hypotheses 2008. Available at: http://CRAN.R-project.org/package=multcomp.
Howe-Kerr L. I., Bachelot B., Wright R. M., Kenkel C. D., Bay L. K., Correa A. M. S. (2020). Symbiont community diversity is more variable in corals that respond poorly to stress. Glob Chang Biol. 26, 2220–2234. doi: 10.1111/gcb.14999
Hughes T. P., Barnes M. L., Bellwood D. R., Cinner J. E., Cumming G. S., Jackson J. B. C., et al. (2017a). Coral reefs in the anthropocene. Nature 546, 82–90. doi: 10.1038/nature22901
Hughes T. P., Kerry J. T., Álvarez-Noriega M., Álvarez-Romero J. G., Anderson K. D., Baird A. H., et al. (2017b). Global warming and recurrent mass bleaching of corals. Nature 543, 373–377. doi: 10.1038/nature21707
Hume B. C. C., Smith E. G., Ziegler M., Warrington H. J. M., Burt J. A., LaJeunesse T. C., et al. (2019). SymPortal: a novel analytical framework and platform for coral algal symbiont next-generation sequencing ITS 2 profiling. Mol. Ecol. Resour 19 (4), 1063–1080. doi: 10.1111/1755-0998.13004
Kuznetsova A., Brockhoff P. B., Christensen R. H. B. (2017). lmerTest package: tests in linear mixed effects models. J. Stat. Softw 82, 1–26. doi: 10.18637/jss.v082.i13
Lahti L., Shetty S., Blake T., Salojarvi J. (2017). Tools for microbiome analysis in r. version, Vol. 1. 28.
LaJeunesse T. C. (2001). Investigating the biodiversity, ecology, and phylogeny of endosymbiont dinoflagellates in the genus Symbiodinium using the ITS region: in search of a “species” level marker. J. Phycol 37, 866–880. doi: 10.1046/j.1529-8817.2001.01031.x
LaJeunesse T. L. (2002). Diversity and community structure of symbiotic dinoflagellates from Caribbean coral reefs. Mar. Biol. 141, 387–400. doi: 10.1007/s00227-002-0829-2
LaJeunesse T. C., Loh W. K. W., Woesik R., Hoegh-Guldberg O., Schmidt G. W., Fitt W. K. (2003). Low symbiont diversity in southern great barrier reef corals, relative to those of the Caribbean. Limnol Oceanogr 48, 2046–2054. doi: 10.4319/lo.2003.48.5.2046
LaJeunesse T. C., Parkinson J. E., Gabrielson P. W., Jeong H. J., Reimer J. D., Voolstra C. R., et al. (2018). Systematic revision of symbiodiniaceae highlights the antiquity and diversity of coral endosymbionts. Curr. Biol. 28, 2570–2580. doi: 10.1016/j.cub.2018.07.008
LaJeunesse T. C., Smith R. T., Finney J., Oxenford H. (2009). Outbreak and persistence of opportunistic symbiotic dinoflagellates during the 2005 Caribbean mass coral “bleaching” event. Proc. R. Soc. B: Biol. Sci. 276, 4139–4148. doi: 10.1098/rspb.2009.1405
Lee M. J., Jeong H. J., Jang S. H., Lee S. Y., Kang N. S., Lee K. H., et al. (2016). Most low-abundance “background’’ symbiodinium spp. are transitory and have minimal functional significance for symbiotic corals. Microb. Ecol. 71, 771–783. doi: 10.1007/s00248-015-0724-2
Leveque S., Afiq-Rosli L., Ip Y. C. A., Jain S. S., Huang D. (2019). Searching for phylogenetic patterns of symbiodiniaceae community structure among indo-pacific merulinidae corals. PeerJ 7, e7669. doi: 10.7717/peerj.7669
Lewis C. L., Coffroth M. A. (2004). The acquisition of exogenous algal symbionts by an octocoral after bleaching. Science 304, 1490–1492. doi: 10.1126/science.1097323
Little A. F., van Oppen M. J. H., Willis B. L. (2004). Flexibility in algal endosymbioses shapes growth in reef corals. Science 304, 1492–1494. doi: 10.1126/science.1095733
Love M., Anders S., Huber W. (2014). Differential analysis of count data–the DESeq2 package. Genome Biol. 15, 550. doi: 10.1186/s13059-014-0550-8
McIlroy S. E., Coffroth M. A. (2017). Coral ontogeny affects early symbiont acquisition in laboratory-reared recruits. Coral Reefs 36, 927–932. doi: 10.1007/s00338-017-1584-7
McMurdie P. J., Holmes S. (2013). Phyloseq: an r package for reproducible interactive analysis and graphics of microbiome census data. PloS One 8, e61217. doi: 10.1371/journal.pone.0061217
Meinshausen M., Smith S. J., Calvin K., Daniel J. S., Kainuma M. L. T., Lamarque J.-F., et al. (2011). The RCP greenhouse gas concentrations and their extensions from 1765 to 2300. Clim Change 109, 213–241. doi: 10.1007/s10584-011-0156-z
Mieog J., van Oppen M., Berkelmans R., Stam W., Olsen J. (2009). Quantification of algal endosymbionts (Symbiodinium) in coral tissue using real-time PCR. Mol. Ecol. Resour 9, 74–82. doi: 10.1111/j.1755-0998.2008.02222.x
Morikawa M. K., Palumbi S. R. (2019). Using naturally occurring climate resilient corals to construct bleaching-resistant nurseries. Proc. Natl. Acad. Sci. U.S.A. 116, 10586–10591. doi: 10.1073/pnas.1721415116
Muscatine L. (1990). The role of symbiotic algae in carbon and energy flux in reef corals. Ecosyst. World: Coral Reefs 25, 75–87.
Nitschke M. R., Davy S. K., Ward S. (2016). Horizontal transmission of Symbiodinium cells between adult and juvenile corals is aided by benthic sediment. Coral Reefs 35, 335–344. doi: 10.1007/s00338-015-1349-0
Oliver T., Palumbi S. (2011). Do fluctuating temperature environments elevate coral thermal tolerance? Coral Reefs 30, 429–440. doi: 10.1007/s00338-011-0721-y
Padilla-Gamiño J. L., Pochon X., Bird C., Concepcion G. T., Gates R. D. (2012). From parent to gamete: vertical transmission of Symbiodinium (Dinophyceae) ITS2 sequence assemblages in the reef building coral Montipora capitata. PLoS One 7, e38440. doi: 10.1371/journal.pone.0038440
Pochon X., Gates R. D., Vik D., Edmunds P. J. (2014). Molecular characterization of symbiotic algae (Symbiodinium spp.) in soritid foraminifera (Sorites orbiculus) and a scleractinian coral (Orbicella annularis) from St John, US virgin islands. Mar. Biol. 161, 2307–2318. doi: 10.1007/s00227-014-2507-6
Poland D. M., Coffroth M. A. (2017). Trans-generational specificity within a cnidarian–algal symbiosis. Coral Reefs 36 (1), 119–129. doi: 10.1007/s00338-016-1514-0
Pollock F. J., Katz S. M., van de Water J. A. J. M., Davies S. W., Hein M., Torda G., et al. (2017). Coral larvae for restoration and research: a large-scale method for rearing Acropora millepora larvae, inducing settlement, and establishing symbiosis. PeerJ 5, e3732. doi: 10.7717/peerj.3732
Quigley K. M., Bay L. K., van Oppen M. J. H. (2020b). Genome-wide SNP analysis reveals an increase in adaptive genetic variation through selective breeding of coral. Mol. Ecol 29 (12), 2176–2188. doi: 10.22541/au.158921584.47783210
Quigley K. M., Bay L. K., Willis B. L. (2017). Temperature and water quality-related patterns in sediment-associated symbiodinium communities impact symbiont uptake and fitness of juveniles in the genus acropora. Front. Mar. Sci. 4. doi: 10.3389/fmars.2017.00401
Quigley K. M., Davies S. W., Kenkel C. D., Willis B. L., Matz M. V., Bay L. K. (2014). Deep-sequencing method for quantifying background abundances of Symbiodinium types: exploring the rare symbiodinium biosphere in reef-building corals. PLoS One 9 (4), e94297. doi: 10.1371/journal.pone.0094297
Quigley K., Ramsby B., Laffy P., Harris J., Mocellin V., Bay L. (2022). Symbioses are restructed by repeated mass coral bleaching. Sci. Adv. 8 (49), eabq8349. doi: 10.1126/sciadv.abq8349
Quigley K. M., Randall C. J., van Oppen M. J. H., Bay L. K. (2020c). Assessing the role of historical temperature regime and algal symbionts on the heat tolerance of coral juveniles. Biol. Open 9 (1), bio047316. doi: 10.1242/bio.047316
Quigley K. M., Strader M. E., Matz M. V. (2018a). Relationship between acropora millepora juvenile fluorescence and composition of newly established symbiodiniumassemblage. PeerJ 6, e5022. doi: 10.7717/peerj.5022
Quigley A. R. C., Torda G., Bourne D., Willis B. (2020a). Co-Dynamics of symbiodiniaceae and bacterial populations during the first year of symbiosis with acropora tenuis juveniles. Microbiologyopen 9, e959. doi: 10.1002/mbo3.959
Quigley K. M., van Oppen M. J. H. (2022). Predictive models for the selection of thermally tolerant corals based on offspring survival. Nat. Commun. 13, 1–13. doi: 10.1038/s41467-022-28956-8
Quigley K. M., Warner P. A., Bay L. K., Willis B. L. (2018b). Unexpected mixed-mode transmission and moderate genetic regulation of symbiodinium communities in a brooding coral. Heredity 121 (6), 524–536. doi: 10.1101/173591
Quigley K. M., Willis B. L., Bay L. K. (2016). Maternal effects and symbiodinium community composition drive differential patterns in juvenile survival in the coral acropora tenuis. R Soc. Open Sci. 3 (10), 160471. doi: 10.1098/rsos.160471
Quigley K. M., Willis B. L., Kenkel C. D. (2019). Transgenerational inheritance of shuffled symbiont communities in the coral montipora digitata. Sci. Rep. 9 (1), 13328. doi: 10.1038/s41598-019-50045-y
R Core Team (2013). R core team. 2013. r: a language and environment for statistical computing (Vienna, Austria: R Foundation for Statistical Computing).
Reich H. G., Robertson D. L., Goodbody-Gringley G. (2017). Do the shuffle: changes in Symbiodinium consortia throughout juvenile coral development. PloS One 12, e0171768. doi: 10.1371/journal.pone.0171768
Robison J. D., Warner M. E. (2006). Differential impacts of photoacclimation and thermal stress on the photobiology of four different phylotypes of symbiodinium (Pyrrhophyta). J. Phycol 42, 568–579. doi: 10.1111/j.1529-8817.2006.00232.x
Rohwer F., Seguritan V., Azam F., Knowlton N. (2002). Diversity and distribution of coral-associated bacteria. Mar. Ecol. Prog. Ser. 243, 1–10. doi: 10.3354/meps243001
Rouzé H., Lecellier G., Pochon X., Torda G., Berteaux-Lecellier V. (2019). Unique quantitative symbiodiniaceae signature of coral colonies revealed through spatio-temporal survey in moorea. Sci. Rep. 9, 7921. doi: 10.1038/s41598-019-44017-5
Rowan R., Knowlton N., Baker A., Jara J. (1997). Landscape ecology of algal symbionts creates variation in episodes of coral bleaching. Nature 388, 265–269. doi: 10.1038/40843
Schoepf V., Grottoli A. G., Warner M. E., Cai W.-J., Melman T. F., Hoadley K. D., et al. (2013). Coral energy reserves and calcification in a high-CO2 world at two temperatures. PLoS One 8, e75049. doi: 10.1371/journal.pone.0075049
Sievers F., Wilm A., Dineen D., Gibson T. J., Karplus K., Li W., et al. (2011). Fast, scalable generation of high-quality protein multiple sequence alignments using clustal omega. Mol. Syst. Biol. 7. doi: 10.1038/msb.2011.75
Sievers K. T., McClure E. C., Abesamis R. A., Russ G. R.. (2020). Non‐reef habitats in a tropical seascape affect density and biomass of fishes on coral reefs. Ecol Evol 10 (24), 13673–13686.
Stat M., Gates R. D. (2008). Vectored introductions of marine endosymbiotic dinoflagellates into Hawaii. Biol. Invasions 10, 579–583. doi: 10.1007/s10530-007-9167-0
Stat M., Morris E., Gates R. D. (2008). Functional diversity in coral–dinoflagellate symbiosis. Proc. Natl. Acad. Sci. 27, 9256–9261. doi: 10.1073/pnas.0801328105
Suggett D. J., Goyen S., Evenhuis C., Szabó M., Pettay D. T., Warner M. E., et al. (2015). Functional diversity of photobiological traits within the genus Symbiodinium appears to be governed by the interaction of cell size with cladal designation. New Phytol. 208, 370–381. doi: 10.1111/nph.13483
Suggett D. J., Warner M. E., Leggat W. (2017). Symbiotic dinoflagellate functional diversity mediates coral survival under ecological crisis. Trends Ecol. Evol. 32, 735–745. doi: 10.1016/j.tree.2017.07.013
Sully S., Burkepile D. E., Donovan M. K., Hodgson G., van Woesik R. (2019). A global analysis of coral bleaching over the past two decades. Nat. Commun. 10, 1264. doi: 10.1038/s41467-019-09238-2
Sun Z., Huang Y., Wang Y., Zhao Y., Cui Z. (2014). Potassium hydroxide-ethylene diamine tetraacetic acid method for the rapid preparation of small-scale PCR template DNA from actinobacteria. Mol. Genetics Microbiol. Virol. 29, 42–46. doi: 10.3103/S089141681401008X
Sun Y., Jiang L., Gong S., Guo M., Yuan X., Zhou G., et al. (2020). Impact of ocean warming and acidification on symbiosis establishment and gene expression profiles in recruits of reef coral acropora intermedia. Front. Microbiol. 11, 532447. doi: 10.3389/fmicb.2020.532447
Swain T. D., Chandler J., Backman V., Marcelino L. (2016). Coral bleaching response index: a new tool to standardize and compare susceptibility to thermal bleaching. Glob. Chang. Biol. 22 (7), 2475–2488. doi: 10.1111/gcb.13276
Umeki M., Yamashita H., Suzuki G., Sato T., Ohara S., Koike K. (2020). Fecal pellets of giant clams as a route for transporting symbiodiniaceae to corals. PLoS One 15, e0243087. doi: 10.1371/journal.pone.0243087
Uthicke S., Patel F., Karelitz S., Luter H. M., Webster N. S., Lamare M. (2020). Key biological responses over two generations of the sea urchin echinometra sp. a under future ocean conditions. Mar. Ecol. Prog. Ser. 637, 87–101. doi: 10.3354/meps13236
Yorifuji M., Harii S., Nakamura R., Fudo M. (2017). Shift of symbiont communities in Acropora tenuis juveniles under heat stress. PeerJ 5, e4055. doi: 10.7717/peerj.4055
Yuyama I., Higuchi T. (2014). Comparing the effects of symbiotic algae (Symbiodinium) clades C1 and D on early growth stages of Acropora tenuis. PLoS One 9, e98999. doi: 10.1371/journal.pone.0098999
Keywords: coral reefs, acclimation, symbiosis, Symbiodiniaceae, recruit, warming, acidification, bleaching
Citation: Terrell AP, Marangon E, Webster NS, Cooke I and Quigley KM (2023) The promotion of stress tolerant Symbiodiniaceae dominance in juveniles of two coral species under simulated future conditions of ocean warming and acidification. Front. Ecol. Evol. 11:1113357. doi: 10.3389/fevo.2023.1113357
Received: 01 December 2022; Accepted: 02 June 2023;
Published: 21 June 2023.
Edited by:
Carolina Madeira, NOVA University Lisbon, PortugalReviewed by:
Alison Gould, California Academy of Sciences, United StatesCopyright © 2023 Terrell, Marangon, Webster, Cooke and Quigley. This is an open-access article distributed under the terms of the Creative Commons Attribution License (CC BY). The use, distribution or reproduction in other forums is permitted, provided the original author(s) and the copyright owner(s) are credited and that the original publication in this journal is cited, in accordance with accepted academic practice. No use, distribution or reproduction is permitted which does not comply with these terms.
*Correspondence: Kate M. Quigley, a2F0ZW1hcmllLnF1aWdsZXlAbXkuamN1LmVkdS5hdQ==
Disclaimer: All claims expressed in this article are solely those of the authors and do not necessarily represent those of their affiliated organizations, or those of the publisher, the editors and the reviewers. Any product that may be evaluated in this article or claim that may be made by its manufacturer is not guaranteed or endorsed by the publisher.
Research integrity at Frontiers
Learn more about the work of our research integrity team to safeguard the quality of each article we publish.