- 1Norwegian Polar Institute, Fram Centre, Tromsø, Norway
- 2Centre d’Etudes Biologiques de Chizé (CEBC), UMR 7372 CNRS-La Rochelle Université, Villiers-en-Bois, France
- 3Akvaplan-niva AS, Tromsø, Norway
- 4Norwegian Institute for Nature Research, Trondheim, Norway
- 5Institut de Ciències del Mar (ICMCSIC), Department of Renewable Marine Resources, Barcelona, Spain
- 6Laboratoire de génétique de la Conservation, Institut de Botanique, Liège, Belgium
The Arctic experiences a rapid retreat of sea-ice, particularly in spring and summer, which may dramatically affect pagophilic species. In recent years, the decline of many Arctic seabird populations has raised concerns about the potential role of sea-ice habitats on their demography. Spring sea-ice drives the dynamics of phytoplankton blooms, the basis of Arctic food webs, and changes in spring sea-ice have the potential to affect the demographic parameters of seabirds through bottom-up processes. To better understand the effects of spring sea-ice on Arctic seabirds, we investigated the influence of spring sea-ice concentration on the survival and breeding success of three seabird species with contrasted foraging strategies in two Svalbard fjords in the high Arctic. We examined these relationships using long-term demographic data (2005–2021) from black-legged kittiwakes (Rissa tridactyla), Brünnich guillemots (Uria lomvia), and little auks (Alle alle). Spring sea-ice concentration was positively related to both the survival and breeding success of little auks, suggesting a higher sensitivity of this species to spring sea-ice. By contrast, the two other species were not particularly sensitive to changes in spring sea-ice, even though a potentially spurious negative effect on the breeding success of black-legged kittiwakes was observed. Overall, the study suggests that spring sea-ice may be involved in the demography of Arctic seabirds, but probably does not play a major role.
Introduction
The Arctic is warming at a higher rate than any other region in the world (Jansen et al., 2020). This resulted in a dramatic decrease in the concentration, extent, volume, and duration of the sea-ice season (Zhang, 2005; Serreze and Barry, 2011). Sea-ice extent has been decreasing in all seasons, but especially in the summer (Cai et al., 2021). Multi-year ice is being replaced by first-year ice and summer sea-ice is expected to disappear completely in the Arctic by mid-century (Stocker et al., 2013). The global contraction of sea-ice alters the habitats of ice-dependent organisms (e.g., Perrette et al., 2011; Will et al., 2020; Florko et al., 2021) and may lead to changes in primary production (Van Leeuwe et al., 2018), in the timing of biological events (e.g., plankton blooms; Arrigo et al., 2008; MacNeil et al., 2010; Gibson et al., 2020), and in the distribution and abundance of multiple species such as extension ranges of southern organisms (e.g., Wassmann et al., 2011; Fossheim et al., 2015; Vihtakari et al., 2018; Węsławski et al., 2018; Tarling et al., 2022). All these changes threaten the viability of ice-dependent meso- and top-predator populations, including seabirds and marine mammals, which may not be able to adapt quickly enough to these environmental changes (e.g., Divoky et al., 2021).
Since the last decades to more recent years, several Arctic seabird populations have shown a marked decline (e.g., Gilchrist and Mallory, 2005; Descamps et al., 2017; Strøm et al., 2020; Descamps and Strøm, 2021), and climate change is often considered to be an important driver of these population trajectories. However, few studies have looked at the impact of sea-ice on these populations, and especially on their demographic parameters, although this appears crucial to determine the mechanisms involved in these declines (but see, Moe et al., 2009; Ramírez et al., 2017; Amélineau et al., 2019; Gutowsky et al., 2022). In addition, demographic responses to variations in sea-ice conditions can be species-specific (Descamps and Ramírez, 2021), as different seabird species exhibit a wide range of food preferences or foraging behaviors that can lead to greater or lesser sensitivity to environmental changes (Burr et al., 2016; Poloczanska et al., 2016; Descamps et al., 2019). Seabird populations can be affected by changes in sea-ice, in particular through a variety of bottom-up processes that may influence their survival, breeding probability, or breeding success (Ramírez et al., 2017; Descamps and Ramírez, 2021). In that context, spring sea-ice plays a key role in resource availability for seabirds during the breeding season by driving the dynamics of phytoplankton blooms, the basis of Arctic food webs (Leu et al., 2011).
In long-lived species, such as seabirds, adult survival has a strong influence on population growth rate, and a small change in survival can strongly affect the overall population dynamics (Caswell, 2000; Sæther and Bakke, 2000). In this context, the demographic buffer (or canalization) hypothesis predicts that adult survival should be characterized by a small temporal variance (Gaillard et al., 2000; Hilde et al., 2020). This has led to the expectation that survival is “protected” from environmental stochasticity (Morris and Doak, 2004), and that only the worst conditions may have a significant impact on survival. The winter season is generally characterized by harsh weather conditions and limited food availability, and has been suggested to be the period during which most deaths occur in seabirds (Lack, 1954; Barbraud and Weimerskirch, 2003; Gilchrist and Mallory, 2005; Frederiksen et al., 2008; Sæther et al., 2016). However, winter environmental conditions may not always be the main and only driver of adult survival, and very few studies have investigated the effects of other periods (i.e., post- and pre-breeding migration or breeding period; Franke et al., 2011; Jenouvrier, 2013; Hovinen et al., 2014).
Here we used long-term data (2005–2021) of three of the most common Arctic seabird species, breeding on a high Arctic archipelago, to explore their demographic responses to changes in spring sea-ice concentration. We focused on the Brünnich guillemot Uria lomvia, the little auk Alle alle, and the black-legged kittiwake Rissa tridactyla, which are characterized by contrasted foraging strategies: the Brünnich’s guillemot is a diving species, feeding mainly on fish up to 200 m depths (Mehlum and Gabrielsen, 1993; Falk et al., 2000; Elliott and Gaston, 2008); the little auk also feeds underwater, at depths up to 35 m, and almost exclusively on crustaceans (Karnovsky et al., 2011; Møller et al., 2018); and the black-legged kittiwake (hereafter kittiwake), is a surface feeder, that targets small fish and zooplankton (Vihtakari et al., 2018). In the high Arctic, Brünnich guillemots and kittiwakes forage on ice-associated fish, such as polar cod Boreogadus saida (Mehlum and Gabrielsen, 1993; Vihtakari et al., 2018), and their population dynamics have already shown positive associations with sea-ice extent (Descamps and Ramírez, 2021). The little auk, the only exclusively zooplanktivorous seabird in the North Atlantic which feeds mainly on lipid-rich copepods Calanus glacialis (Lønne and Gabrielsen, 1992; Descamps et al., 2022), has also shown a strong association with sea-ice (e.g., Jakubas et al., 2012; Amélineau et al., 2019). However, we currently lack detailed information on the effects of sea-ice on demographic parameters, like survival and breeding success.
Our primary objectives were to quantify the potential effects of changes in spring sea-ice concentration on adult survival and breeding success in these three species. Since adult survival is a canalized trait that generally exhibits limited temporal variation (Caswell, 1989; Stearns, 1992), we predicted a limited effect of sea-ice concentration on survival, and a more pronounced effect on breeding success. We also expect differences between species according to their dependence on sea-ice. Brünnich’s guillemot and little auk are endemic species exclusively distributed in the Arctic, whereas kittiwakes have a larger distribution range and are also common in northern temperate environments (Descamps and Strøm, 2021). Assuming that these large-scale distributions indicate the extent to which the species is tolerant to less Arctic conditions, and thus to a loss of sea-ice, we predicted that variations in sea-ice concentration would have a stronger effect on Brünnich’s guillemots and little auks when compared to kittiwakes.
Materials and methods
Study site and species
The study was carried out between 2005 and 2021, in Kongsfjorden and Isfjorden, two fjords located in western Spitsbergen, Svalbard (Figure 1). The little auk is a small alcid (ca. 160 g), nesting in areas with talus slopes. The females lay a single egg in early to mid-June, which is incubated for around 30 days; the chick is then reared for around 27 days (Wojczulanis-Jakubas et al., 2009, 2020). The Brünnich’s guillemot is a large alcid (ca. 1,000 g) that breeds on cliffs. The females lay a single egg in late May/early June and incubation lasts 33 days on average, then adults feed their chicks for 15–30 days at the nest site before the young birds depart to sea (Birkhead and Nettleship, 1987). The kittiwake breeds on cliffs, and on Svalbard, females typically lay one or two eggs in early June, incubated for approximately 27 days; the chicks are then reared for around 43 days (Barrett, 1980). In all three species, females and males share the incubation and chick-rearing duties.
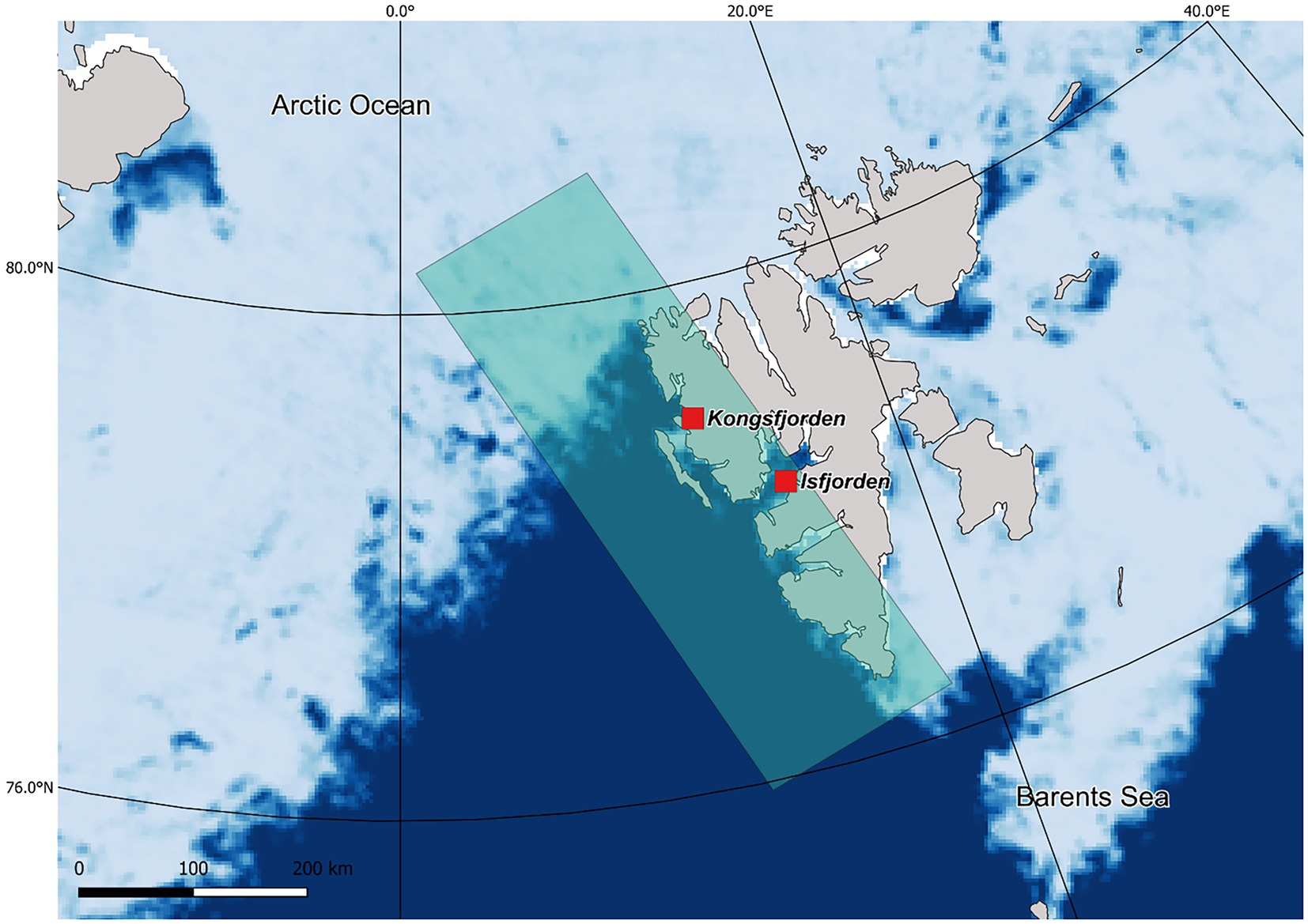
Figure 1. Study area at Spitsbergen (Svalbard, Norway). The red squares indicate the fjord locations. The shaded rectangle on the western coast of Spitsbergen represents the area from which the long-term time-series (1988–2018) of maximum sea-ice concentration data was extracted to evaluate the relationship between sea-ice conditions and seabirds’ population. For illustrative purposes, we include background colors pinpointing the seasonal ice zone. Dark blue indicates open water, the lighter the gradient the higher the concentration of sea-ice. White cells along the coastline indicate no data on sea-ice.
Survival and reproduction monitoring
Capture-mark-recapture data were collected annually during the breeding period (mid -June to late July), in both fjords. Brünnich guillemots and little auks were monitored from 2005 to 2021 in Isfjorden (n = 449 ringed guillemots at the Diabasodden colony and n = 621 ringed little auks at the Bjørndalen colony) and from 2006 to 2021 in Kongsfjorden (n = 253 ringed guillemots at the Ossian sarsfjellet colony and n = 791 ringed little auks at the Feiringfjellet colony). Kittiwakes were monitored from 2008 to 2021, in both Isfjorden and Kongsfjorden (n = 258 and n = 1,317 at the Grumantbyen and Krykkjefjellet colonies, respectively). Guillemots and kittiwakes were captured from their nest during incubation and chick rearing, using a noose pole. Little auks were captured randomly in the colony using passive traps (noose carpets). A few non-breeding birds may have been captured and ringed, but as captured occurred during chick rearing only, we are confident that the vast majority of birds were breeders.
Nest monitoring to obtain breeding success data has been carried out in Isfjorden, from 2005 to 2021, for little auks, and from 2008 to 2021, for kittiwakes, and in Kongsfjorden, from 2011 to 2021, for Brünnich’s guillemots. Annually, a sample of nests (average of 53 ± 12 SD, 39 ± 14 SD, and 47 ± 16 SD for guillemots, little auks, and kittiwakes) have been visited approximately twice a week to assess the nest status and content. Due to logistical constraints, nest monitoring could not have been carried out until the chicks had fledged; we defined successful nests as nests where the chick (or at least one chick for the kittiwakes) had survived until 15 days of age.
Sea-ice data
Daily sea-ice concentration was determined from an AMSR-E and AMSR-2 derived data set provided by the Institute of Environmental Physics, University of Bremen, Germany (Spreen et al., 2008). The data consists of the ice concentration at the highest possible grid resolution of 3.125 km. The maximum daily sea-ice concentration from April to June was selected for each year, from 2003 to 2021. Daily sea-ice concentration data were averaged from a fixed marine area that was assumed to encompass the main foraging areas of the three species, during the pre-breeding and breeding seasons (see Descamps and Ramirez, 2021 for details). Although this area is probably larger than the one used by birds during part of the breeding season like chick rearing (Ramírez et al., 2017; Bertrand et al., 2021), the general dynamics of sea ice changes in this large area is similar to the one in smaller areas centered around the colonies (see Descamps and Ramirez, 2021 for details). Moreover, the maximum spring sea-ice concentration is strongly correlated with average spring sea-ice and the maximum annual sea-ice, and thus provides a good proxy of the general sea-ice conditions (Descamps and Ramirez, 2021). We assessed the temporal trend in maximum spring sea-ice concentration using linear models (lm function in R software version 4.1.2, R Core Team, 2022). This analysis was not intended to provide new evidence for the decline of Arctic sea-ice, a well-known trend, but was a necessary support for further analyses and interpretation of the results.
Survival analysis
General model
First, we constructed single-state capture-mark-recapture models for each species separately, which provided unbiased demographic estimators by taking into account imperfect detectability of marked individuals (Lebreton et al., 1992). Capture histories were coded considering two events corresponding to field observations: 0 = not observed, 1 = observed. The general models were the extended Cormack-Jolly-Seber (CJS) models, in which the survival (Φ) and recapture (p) probabilities were time and colony dependent (i.e., modeled as , where c represents the colony and t the time; Lebreton et al., 1992). This method does not separate permanent emigration from mortality and only estimates apparent survival. However, the three species studied show very high colony fidelity and the assumption that apparent survival reflects actual survival is robust. To test that the data met the model assumptions, we performed goodness of fit (GOF) tests for single state models using the software U-Care (v3.3, Choquet et al., 2009a). The overall GOF test of the CJS models indicated a clear lack of fit for all species, mostly caused by significant transience and trap dependence (significant test components 3.SR and 2.CT; Supplementary Table A1). Test 2.CT tests the hypothesis that there is no difference in the probability of being recaptured at occasion t + 1 between those who were captured at occasion t and those who were not. Test 3.SR tests the hypothesis that there is no difference between previously and newly tagged individuals caught at time t in their probability of being recaptured later. To deal with trap dependence, we used the Pradel and Sanz Aguilar approach, which considers immediate trap-dependence based on a multi-event framework (see details in Pradel and Sanz-Aguilar, 2012). Transience was dealt with by considering two age classes in survival (age being defined as the number of years following the first capture): survival in the year following the first capture, and subsequent survival (i.e., survival of non-transient individuals; Pradel et al., 1997). We then accounted for the remaining heterogeneity by scaling model deviance using an overdispersion parameter ( , computed after discarding the 3.SR and 2.CT components, by dividing the chi-square statistic by its degrees of freedom), and based the model selection on the quasi-likelihood Akaike Information Criterion adjusted for small sample size (QAICc, Anderson and Burnham, 1999).
Second, we performed a model selection (for each species separately) using program E-SURGE (v.2.2.3, Choquet et al., 2009b) to obtain a reference model (also called “a parsimonious model,” see Anderson et al., 1994) to test for the effects of sea-ice on survival. If ΔQAICc (i.e., the difference in QAICc between two models) was <2, the models are deemed to have equal statistical support and in case of nested models, the simplest was preferred. We started to model the recapture probabilities and then the survival probabilities, and tested whether they varied among years and/or colonies. During the selection process we kept the survival of the fist age class (i.e., transient individuals) fully parameterized (i.e., time and colony dependent).
Temporal trend and sea-ice effect on survival
We used the reference model structure obtained in the previous step to test whether the survival in the different species and colonies showed a temporal trend during the study period and whether survival was affected by the inter-annual changes in maximum spring sea-ice concentration. For all three species, the reference models did not include any colony effect (see Supplementary Table A2) indicating that their survival did not differ among fjords. Consequently, we analysed both fjords simultaneously for each species when testing for a trend or sea-ice effects.
To identify the trend (if any) in seabird survival, we only considered a linear trend to avoid making the models too complex. Then, we tested for an effect of spring sea-ice maximum concentration on adult survival. We considered a direct effect of sea-ice as well as a lagged effect with a one-to-three-year lag, depending on the species. Sea-ice may have a delayed effect on seabirds, depending on the life cycle of the prey and the stage at which it is consumed (i.e., larvae, juveniles, or adults). The associations between sea-ice, prey availability, and the foraging activities of ice-associated species are complex and poorly understood. We thus tested for several time lags for each species, to explore several potential mechanisms. For Brünnich guillemots and kittiwakes we considered a time-lag from 1 to 3 years, as they feed preferentially on polar cod between 1 and 3 years of age (Mehlum and Gabrielsen, 1993; Vihtakari et al., 2018). For little auk we considered a time-lag from 1 to 2 years, as the life cycle of C. glacialis extends over 2 years (Kwasniewski et al., 2003). Relationships between the covariates and survival were fitted using a logit link function: where Φ is the (apparent) adult survival between t−1 and t, is the intercept, is the slope, and is the value of the covariate (sea-ice or linear trend) at year t. We then performed an analysis of deviance (ANODEV), (Grosbois et al., 2008), to assess the fit of a covariate model relative to that of the constant and the time-dependent models and to test the significance of each relationship. This ANODEV statistic was calculated as:
The proportion of deviance explained by the covariates (Skalski, 1996) was estimated as:
In case of co-occurrence of a temporal trend in both survival and sea-ice, a significant relationship between these two parameters could be spurious and not represent a real causal relationship. We thus tested whether sea-ice explained a significant fraction of the inter-annual variation in survival independently of any potential linear trend (i.e., by keeping a linear trend in the model).
Breeding success analysis
Temporal trend and sea-ice effect on breeding success
In a first step, we assessed the temporal trend in breeding success for each species separately, using a generalized linear mixed model (GLMM), with the nest as a random factor to take into account the lack of independence (i.e., repeated measures) at the nest level (glmer function, lm4 package). Our breeding success proxy was binary (0: failure, 1: success), thus we used a binomial error distribution and a logit link function. As for the survival analysis, we considered only linear effects. Details about residuals’ distribution for the binomial regression model (using the deviance residuals for model checking) are available in Supplementary material (Figures A1, A2, and A3). In a second step, we analysed the effects of sea-ice, with and without a time-lag, on breeding success using GLMMs (glmer function, lm4 package) with the nest as a random factor to take into account the lack of independence at the nest level (for kittiwake and guillemot), and with year as continuous predictor to take into account the potential temporal trend. To assess the effect of the trend and sea-ice covariates, we compared the different models using AICc (Burnham and Anderson, 2002). If the difference in AICc values between two models was <2, the models had equal statistical support, and in the case of nested models, the simplest model was preferred. All breeding success analyses were performed in R (version 4.1.2, R Core Team 2022).
Results
Trends in maximum spring sea-ice concentration and demographic parameters
The maximum spring sea-ice concentration in West Spitsbergen showed significant inter-annual fluctuations and declined significantly from about 45 to 30% in our study area between 2003 and 2021 (Figure 2G).
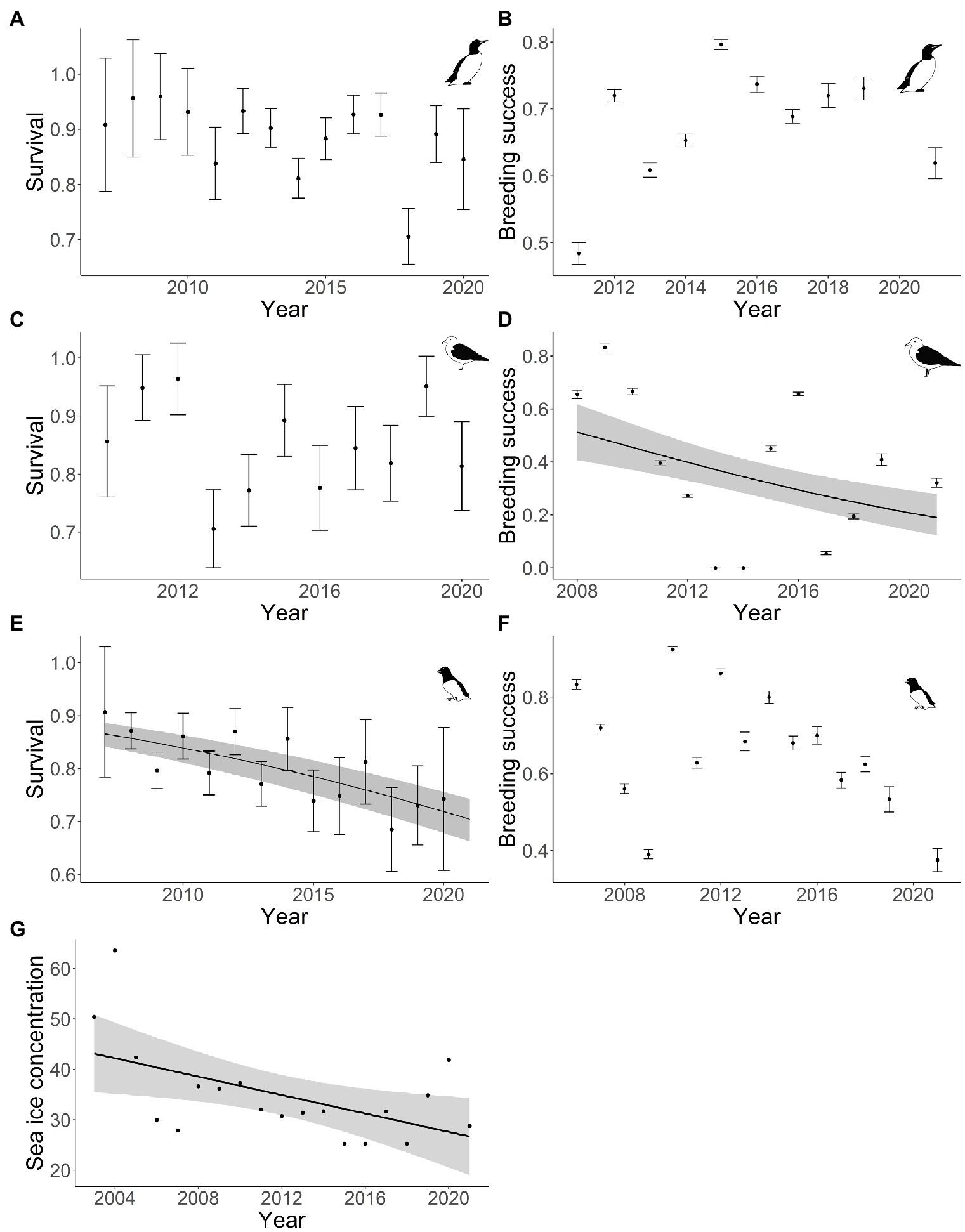
Figure 2. Long-term trends of demographic parameters of Brünnich guillemot (A,B), black legged kittiwake (C,D), little auk (E,F) and maximum sea-ice concentration (G) in West Spitsbergen (Svalbard archipelago, Norway). Survival was estimated from a time-dependent model with standard error. Plain line and shaded areas represent the estimated trajectory from the significant regression selected and its associated 95% confidence interval.
The survival of kittiwakes did not show any significant linear trend (average of 0.83 ± 0.01 SE; Supplementary Table A3, Figure 2C). Even though Brünnich guillemots showed lower survival in recent years (0.80 ± 0.08 SE on average in the last 4 years) compared to earlier years (0.90 ± 0.04 SE on average), a model including a linear trend was not preferred over a model with constant survival (Supplementary Table A3, Figure 2A). Survival of little auks showed a significant linear decline from 0.86 (±0.02 SE) to 0.70 (±0.04 SE) over about 15 years (2005–2021; Figure 2E).
Kittiwakes’ breeding success showed a linear trend over time with a decline from 0.66 (±0.02) to 0.32 (±0.02; Supplementary Table A4, Figure 2D). Little auk and Brünnich guillemots’ breeding success did not show any significant linear trend during our study period (Supplementary Table A4, Figures 2B,F).
Effect of maximum spring sea-ice concentration on demographic parameters
The effects of maximum sea-ice concentration, with and without time-lags, on survival are summarized for each species in Table 1. We did not find any effect of maximum spring sea-ice (with and without lag) on guillemot and kittiwake survival (Table 1, Supplementary Figure A5). In little auks, however, adult survival was positively and significantly associated with the maximum spring sea-ice concentration with no lag (slope: 0.24 ± 0.09, Table 1, Figure 3, Supplementary A5). This effect shows that a 15% decrease in sea-ice concentration was associated with a 10% decrease in survival. However, this apparent sea-ice effect on little auk survival might be the result of the co-occurrence of a linear trend in both parameters. After adjusting the survival for a linear trend in the model, the effect of sea-ice was indeed no longer significant (ANODEV: F-statistic = 0.52, value of p = 0.482).
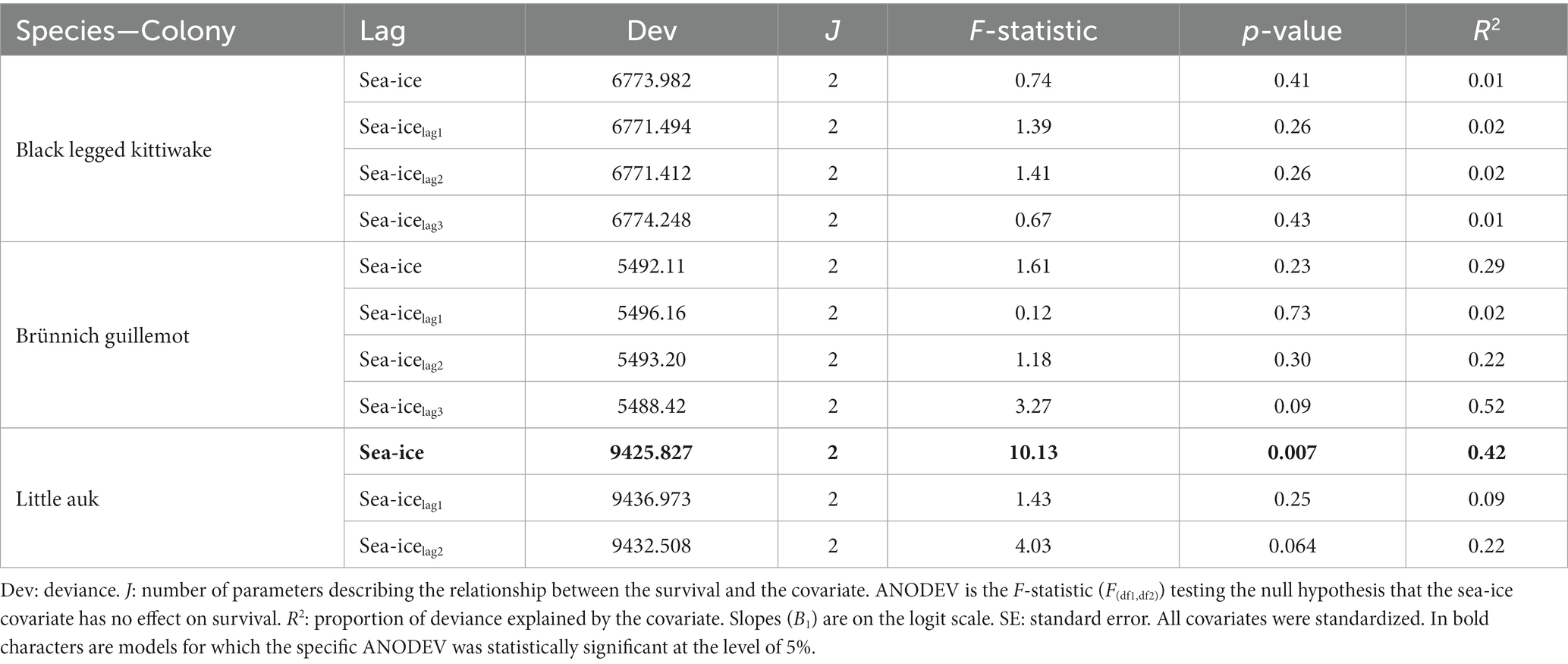
Table 1. Results of the ANODEV from the models testing for the effects of maximum sea-ice concentration with and without time-lag on survival probability of Brünnich guillemots, little auk, and black legged kittiwake breeding in west Svalbard, over the study periods.
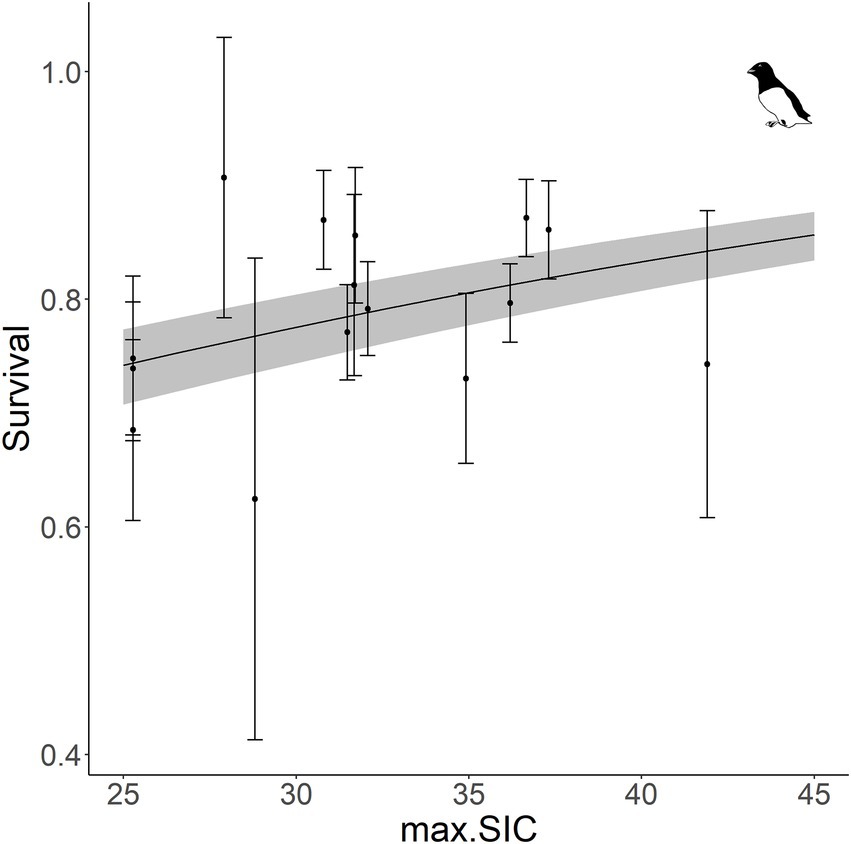
Figure 3. Survival of little auk modeled as a function of maximum sea-ice concentration (max. SIC, plain line) with 95% confidence intervals and annual estimates obtained from the time dependent model (filled circles) with standard error.
The effects of maximum sea-ice concentration, with and without time-lags, on breeding success are summarized for all three species in Table 2. In kittiwakes, breeding success was negatively affected by the maximum spring sea-ice (without a lag, and with a three-year lag; Table 2, Figures 4A,B, Supplementary A6). These models showed a decrease in breeding success from about 0.74 for a 25% sea-ice concentration to 0.60 for a 40% sea-ice concentration. In little auks, we observed a positive association between maximum sea-ice concentration with a two-year lag and breeding success (Table 2, Figure 4C, Supplementary A6). These models showed an increase in breeding success from about 0.60 for a 25% sea-ice concentration to 0.90 for a 60% sea-ice concentration. In Brünnich guillemots, we found no effect of the maximum sea-ice concentration with or without lag on breeding success (Table 2, Supplementary Figure A6).
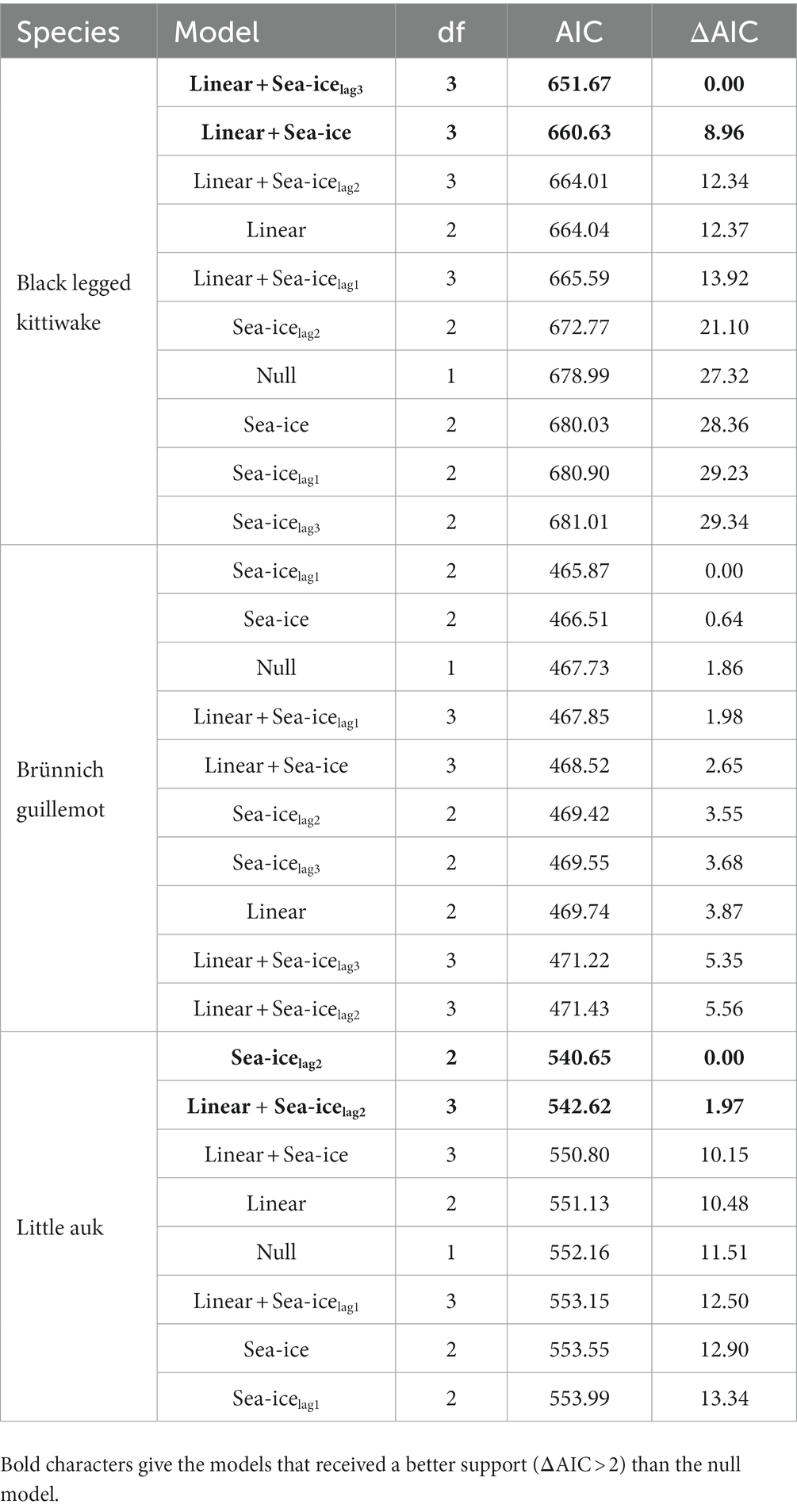
Table 2. Results of the model testing for the effect of sea-ice concentration with and without time-lag on breeding success of Brünnich guillemot, black legged kittiwake, and little auk breeding in west Svalbard, over the study period.
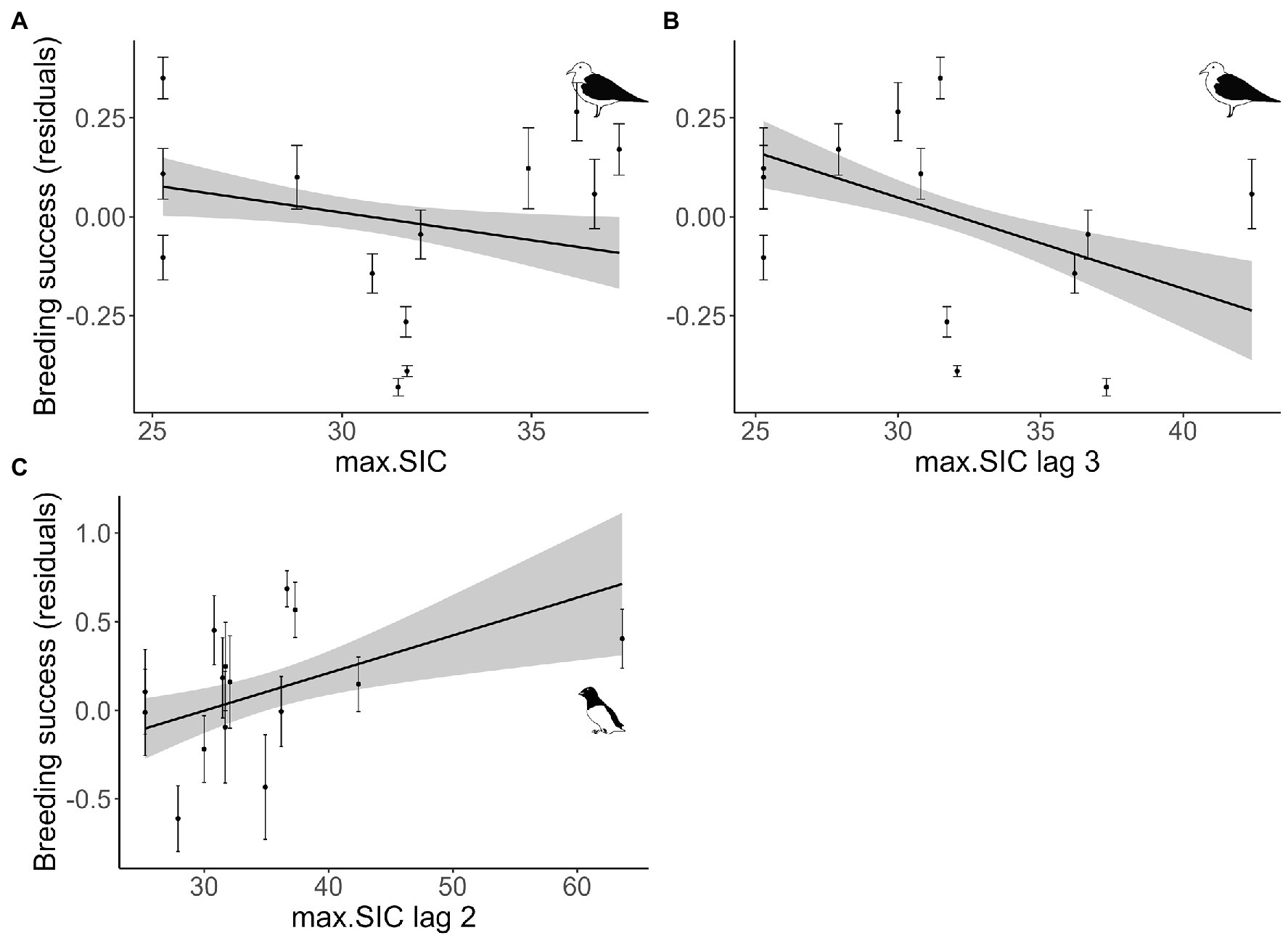
Figure 4. Relationship between maximum sea-ice concentration (max. SIC) with or without a lag (lag 2 = 2 years, lag 3 = 3 years) and breeding success probability of kittiwakes (A,B) and little auk (C) in Isfjorden (west Svalbard). Plain lines correspond to estimated relationships from a linear model considering the detrended time-series with associated 95% confidence intervals. Dots correspond to residuals from the time-series adjusted for their trends with standard error.
Discussion
In this study we provide elements to decipher the role of spring sea-ice on seabird demography through two vital rates (adult survival and breeding success) and thus provide new insights on the role of sea-ice in driving the trajectories of high Arctic seabird populations. Our results showed that both adult survival and breeding success of little auks were positively associated with the maximum spring sea-ice concentration. In kittiwakes, we did not detect any effect of sea-ice on adult survival, but breeding success was apparently negatively associated to sea-ice. In Brünnich guillemots, we did not detect any effect of sea-ice on adult survival and breeding success.
Potential mechanisms linking sea-ice changes and seabird vital rates
The mechanisms driving the relationship between sea-ice and seabird vital rates are likely related to bottom-up processes (Jenouvrier, 2013; Descamps et al., 2017). Sea-ice characteristics (e.g., concentration, extent, and thickness) are largely affected by wind and temperature (Stammerjohn et al., 2008; Persson and Vihma, 2017). These sea-ice characteristics, together with other factors such as water column stratification, determine the availability of nutrients and light for primary producers (Pabi et al., 2008). In turn, primary producers affect the abundance of Arctic fish and crustaceans, the main prey of Arctic seabirds. This simplified causal pathway provides an overall understanding of the relationship between sea-ice and seabirds. However, these relationships are likely much more complex, they can be delayed in time due to the life cycles of the various prey species, which can extend over several years and lead to time-lags between changes in environmental conditions and their consequences at upper trophic levels. They can also have non-linear effects (e.g., Doak and Morris, 2010; Desprez et al., 2018). For example, Iles et al. (2020) tested the hypothesis of optimal sea-ice conditions on the dynamics of several Adélie penguin (Pygoscelis adeliae) populations at the scale of the Antarctic continent. Their analysis confirmed that Adélie penguin habitat is strongly determined by sea-ice conditions, with average penguin population growth being a non-linear function of average winter sea-ice concentration. Longer time-series for Arctic seabird populations would be needed to assess such potential non-linear effects of sea-ice on seabird demography, which stresses the importance of maintaining existing monitoring programs in the Arctic.
Our study partly supports the hypothesis that bottom-up processes may be involved in the relationship between sea-ice and Arctic seabird demography. We did not detect any effect on Brünnich’s guillemot survival or breeding success, but we found a positive effect of sea-ice with a lag of 2 years on the breeding success of little auks. In Svalbard, the little auk feeds mainly on large, energy-rich copepods (C. glacialis, stages CV; Descamps et al., 2022) whose life-history is affected by sea-ice (Ershova et al., 2021). Annual sea-ice concentration affects the abundance of early life stages of copepods (CI-CII) which are present in spring (May–June; Ershova et al., 2021). Thus, after a two-year lag, the abundance of the CV stage of C. glacialis (the main prey of the little auk) could also be affected. Our results, showing a positive effect of sea-ice with a two-year lag on little auk breeding success, are consistent with this mechanism. However, the positive effect of sea-ice with no lag observed on little auk survival is harder to interpret and might suggest that other mechanisms are involved. This result could also be spurious and originate from the co-occurrence of a linear decline in little auk survival and spring sea-ice. In kittiwakes, the observed negative relationship between sea-ice and breeding success seems mostly affected by 2 years (2013 and 2014) where breeding success was extremely low. This low breeding success in 2013 and 2014 was probably the consequence of a high predation rate by glaucous gulls (Larus hyperboreus) as well as many nests falling off the ledges (S. Descamps, unpubl. data). The negative association between kittiwake breeding success and spring sea-ice may thus very likely be spurious. Longer time-series would be needed to confirm, or deny, such relationship.
Overall our study was based on relatively short time-series (i.e., 2011–2021 for guillemots breeding success), which may not provide the statistical power needed to assess the effect of sea-ice on seabirds’ adult survival and breeding success. Our interpretations should therefore be taken with caution. Except for the positive effect of sea-ice on little auk breeding success, we found no clear evidence that sea-ice plays a major role in the demography of these species. In our study, sea-ice explains, at best, only a small proportion of the observed inter-annual changes in seabird demography. This is consistent with earlier results linking sea-ice and seabird population dynamics (Descamps and Ramirez, 2021). This study showed that the effect of sea-ice on guillemot and kittiwake population size, although significant, explained only a very small proportion of the changes. Sea-ice may therefore be important (see Gaston et al., 2012; Smith and Gaston, 2012), but it is clear that other factors more important than maximum sea-ice in spring appear to be at play here. For example, the availability of food resources in west Spitsbergen also depends on the advection of Atlantic water (Hop et al., 2019). Indeed, such Atlantic water inflow in west Spitsbergen fjords strongly affects both the amount and composition of the prey available to seabirds (e.g., Vihtakari et al., 2018; Descamps et al., 2022). Productivity in the fjords can also be affected by other processes like glacier discharge (Lydersen et al., 2014). Indeed, freshwater discharge from tidewater glacier fronts can affect the local prey availability and create foraging hotspots for seabirds (Bertrand et al., 2021).
Implication
As expected by the demographic buffer hypothesis, our analyses did not detect any strong effect of spring sea-ice concentration on the survival of Brünnich guillemots and kittiwakes. In contrast, little auk survival may be affected by changes in spring sea-ice (assuming that the detected effect is not spurious). This would be coherent with previous results found in the same population suggesting that little auk survival was affected by summer environmental conditions (Hovinen et al., 2014; Descamps et al., 2022). Indeed, Hovinen et al. (2014) suggested an effect of sea surface temperatures during the breeding season of the preceding year on little auk survival, even though the time-series used was relatively short (8 years) and the results mainly driven by 1 year with high SST values. Descamps et al. (2022) found that wintering in the same area was not sufficient to synchronize adult survival among different little auk populations, suggesting that survival was also affected by the conditions encountered during the rest of the year. Our results may support this conclusion by showing a relationship between spring sea-ice and little auk survival, thus highlighting the effects of environmental conditions during the breeding season.
Our result about seabird survival can also be interpreted in the context of the “tap/tub hypothesis” (Sæther et al., 2004). The “tub” hypothesis considers that fluctuations in population size are closely related to climate variation during the non-breeding season because the climatic conditions of this season determine the number of birds that survive. The “tap” hypothesis predicts that the annual variation in population size is related to climatic conditions during the breeding season, as this will influence the influx of new recruits into the population the following year. In seabirds, both effects may be relevant, but the “tap” effect seems more common (Sandvik et al., 2012). However, the hypothesis initially defined does not mention any effect of climatic conditions during the breeding period on survival. Our results could refine this hypothesis by providing an empirical example of an environmental variable acting during the breeding season and affecting adult survival.
Conclusion
This study provides a new understanding of the effects of sea-ice on Arctic seabirds breeding in Svalbard, a region where sea-ice changes profoundly alter ecosystems (Descamps et al., 2017) and where some seabird populations are in steep decline (Strøm et al., 2020; Descamps and Strøm, 2021).
Our results indicate a potential higher sensitivity to spring sea-ice in little auks with observed effects on breeding success and perhaps survival, while no effect is shown in guillemots, and the relationships observed in kittiwakes is probably spurious. However, to better understand the mechanisms involved and to confirm or invalidate these results, further studies with longer data sets focusing on the effect of sea-ice changes on the main prey of Arctic seabirds may be needed. Furthermore, including other demographic parameters (i.e., breeding probability, juvenile recruitment) would greatly improve our understanding of the effects of climate-related variables on population dynamics, but data on such parameters are difficult to obtain. Overall, based on our current knowledge, our results suggest that spring sea-ice may be involved in the demography of Arctic seabirds, but does not play a major role.
Data availability statement
The raw data supporting the conclusions of this article will be made available by the authors, without undue reservation.
Ethics statement
The animal study was reviewed and approved by the animal study was reviewed and approved by Mattilsynet (Norwegian Food Safety Anthority, in charge of animal welfare).
Author contributions
CS and SD contributed to conception and design of the study. FA, PB, OC, GG, WJ, AK, BM, ST, and SD collected and processed the data. CS performed the statistical analysis. CS: writing—original draft, and visualization. FR, FA, PB, OC, GG, WJ, AK, ST, and SD: writing—review and editing. All authors contributed to the article and approved the submitted version.
Funding
This study was funded by the project FACE-IT (The Future of Arctic Coastal Ecosystems—Identifying Transitions in Fjord Systems and Adjacent Coastal Areas) and the programs SEAPOP (www.seapop.no) and MOSJ (www.mosj.no). FACE-IT has received funding from the European Union’s Horizon 2020 research and innovation program under grant agreement No 869154. The long-term study of kittiwakes in the Kongsfjord was supported by Institut Polaire Français (IPEV project no. 330) and the BNP Paribas Foundation (project SENSEI).
Acknowledgments
The authors also thank K. Sagerup, S. Nilsen, A. Ask, T. Nordstad, A. Goutte, C. Clément-Chastel, and the AWIPEV staff for their help in the fieldwork. The authors thank H. Steen for initiating some of the monitoring of Svalbard seabirds in 2005 and all the field assistants who helped collecting these data every summer.
Conflict of interest
Author PB was employed by Akvaplan-niva AS.
The remaining authors declare that the research was conducted in the absence of any commercial or financial relationships that could be construed as a potential conflict of interest.
Publisher’s note
All claims expressed in this article are solely those of the authors and do not necessarily represent those of their affiliated organizations, or those of the publisher, the editors and the reviewers. Any product that may be evaluated in this article, or claim that may be made by its manufacturer, is not guaranteed or endorsed by the publisher.
Supplementary material
The Supplementary material for this article can be found online at: https://www.frontiersin.org/articles/10.3389/fevo.2023.1107992/full#supplementary-material
References
Amélineau, F., Grémillet, D., Harding, A. M. A., Walkusz, W., Choquet, R., and Fort, J. (2019). Arctic climate change and pollution impact little auk foraging and fitness across a decade. Sci. Rep. 9:1014. doi: 10.1038/s41598-018-38042-z
Anderson, D. R., and Burnham, K. P. (1999). Understanding information criteria for selection among capture-recapture or ring recovery models. Bird Stud. 46, S14–S21. doi: 10.1080/00063659909477227
Anderson, D. R., Burnham, K. P., and White, G. C. (1994). AIC Model Selection in Overdispersed Capture-Recapture Data. Ecology 75, 1780–1793. doi: 10.2307/1939637
Arrigo, K. R., van Dijken, G., and Pabi, S. (2008). Impact of a shrinking Arctic ice cover on marine primary production. Geophys. Res. Lett. 35. doi: 10.1029/2008GL035028
Barbraud, C., and Weimerskirch, H. (2003). Climate and density shape population dynamics of a marine top predator. Proc. R. Soc. Lond. Ser. B Biol. Sci. 270, 2111–2116. doi: 10.1098/rspb.2003.2488
Barrett, R. T. (1980). Temperature of kittiwake Rissa tridactyla eggs and nests during incubation. Ornis Scand. 11, 50–59. doi: 10.2307/3676265
Bertrand, P., Strøm, H., Bêty, J., Steen, H., Kohler, J., Vihtakari, M., et al. (2021). Feeding at the front line: interannual variation in the use of glacier fronts by foraging black-legged kittiwakes. Mar. Ecol. Prog. Ser. 677, 197–208. doi: 10.3354/meps13869
Birkhead, T. R., and Nettleship, D. N. (1987). Ecological relationships between common murres, Uria aalge, and thick-billed murres, Uria lomvia, at the Gannet Islands, Labrador. I. Morphometrics and timing of breeding. Can. J. Zool. 65, 1621–1629. doi: 10.1139/z87-251
Burnham, K. P., and Anderson, D. R. (2002). A practical information-theoretic approach. Model Select. Multimodel Inf. 2, 70–71. doi: 10.2307/3803155
Burr, Z. M., Varpe, Ø., Anker-Nilssen, T., Erikstad, K. E., Descamps, S., Barrett, R. T., et al. (2016). Later at higher latitudes: large-scale variability in seabird breeding timing and synchronicity. Ecosphere 7:e01283. doi: 10.1002/ecs2.1283
Cai, Q., Wang, J., Beletsky, D., Overland, J., Ikeda, M., and Wan, L. (2021). Accelerated decline of summer Arctic Sea ice during 1850–2017 and the amplified Arctic warming during the recent decades. Environ. Res. Lett. 16:034015. doi: 10.1088/1748-9326/abdb5f
Caswell, H. (1989). Analysis of life table response experiments I. decomposition of effects on population growth rate. Ecol. Model. 46, 221–237. doi: 10.1016/0304-3800(89)90019-7
Caswell, H. (2000). Matrix Population Models: Construction, Analysis, and Interpretation. 2nd Ed. Oxford, New York: Oxford University Press.
Choquet, R., Lebreton, J.-D., Gimenez, O., Reboulet, A.-M., and Pradel, R. (2009a). U-CARE: utilities for performing goodness of fit tests and manipulating CApture–REcapture data. Ecography 32, 1071–1074. doi: 10.1111/j.1600-0587.2009.05968.x
Choquet, R., Rouan, L., and Pradel, R. (2009b). “Program E-surge: A software application for fitting multievent models” in Modeling demographic processes in marked populations environmental and ecological statistics. eds. D. L. Thomson, E. G. Cooch, and M. J. Conroy (Boston, MA: Springer US), 845–865.
Descamps, S., Aars, J., Fuglei, E., Kovacs, K. M., Lydersen, C., Pavlova, O., et al. (2017). Climate change impacts on wildlife in a high Arctic archipelago - Svalbard, Norway. Glob. Chang. Biol. 23, 490–502. doi: 10.1111/gcb.13381
Descamps, S., and Ramírez, F. (2021). Species and spatial variation in the effects of sea ice on Arctic seabird populations. Divers. Distrib. 27, 2204–2217. doi: 10.1111/ddi.13389
Descamps, S., Ramírez, F., Benjaminsen, S., Anker-Nilssen, T., Barrett, R. T., Burr, Z., et al. (2019). Diverging phenological responses of Arctic seabirds to an earlier spring. Glob. Chang. Biol. 25, 4081–4091. doi: 10.1111/gcb.14780
Descamps, S., and Strøm, H. (2021). As the Arctic becomes boreal: ongoing shifts in a high-Arctic seabird community. Ecology 102:e03485. doi: 10.1002/ecy.3485
Descamps, S., Wojczulanis-Jakubas, K., Jakubas, D., Vihtakari, M., Steen, H., Welcker, J., et al. (2022). Consequences of Atlantification on a zooplanktivorous Arctic seabird. Front. Mar. Sci. 9:878746. doi: 10.3389/fmars.2022.878746
Desprez, M., Jenouvrier, S., Barbraud, C., Delord, K., and Weimerskirch, H. (2018). Linking oceanographic conditions, migratory schedules and foraging behaviour during the non-breeding season to reproductive performance in a long-lived seabird. Funct. Ecol. 32, 2040–2053. doi: 10.1111/1365-2435.13117
Divoky, G. J., Brown, E., and Elliott, K. H. (2021). Reduced seasonal sea ice and increased sea surface temperature change prey and foraging behaviour in an ice-obligate Arctic seabird, Mandt’s black guillemot (Cepphus grylle mandtii). Polar Biol. 44, 701–715. doi: 10.1007/s00300-021-02826-3
Doak, D. F., and Morris, W. F. (2010). Demographic compensation and tipping points in climate-induced range shifts. Nature 467, 959–962. doi: 10.1038/nature09439
Elliott, K. H., and Gaston, A. J. (2008). Mass-length relationships and energy content of fishes and invertebrates delivered to nestling thick-billed murres Uria lomvia in the Canadian Arctic, 1981-2007. Mar. Ornithol. 36, 25–34.
Ershova, E. A., Kosobokova, K. N., Banas, N. S., Ellingsen, I., Niehoff, B., Hildebrandt, N., et al. (2021). Sea ice decline drives biogeographical shifts of key Calanus species in the Central Arctic Ocean. Glob. Chang. Biol. 27, 2128–2143. doi: 10.1111/gcb.15562
Falk, K., Benvenuti, S., Dall’antonia, L., Kampp, K., and Ribolini, A. (2000). Time allocation and foraging behaviour of chick-rearing Brünnich’s guillemots Uria lomvia in high-arctic Greenland. Ibis 142, 82–92. doi: 10.1111/j.1474-919X.2000.tb07687.x
Florko, K. R. N., Thiemann, G. W., Bromaghin, J. F., and Richardson, E. S. (2021). Diet composition and body condition of polar bears (Ursus maritimus) in relation to sea ice habitat in the Canadian high Arctic. Polar Biol. 44, 1445–1456. doi: 10.1007/s00300-021-02891-8
Fossheim, M., Primicerio, R., Johannesen, E., Ingvaldsen, R. B., Aschan, M. M., and Dolgov, A. V. (2015). Recent warming leads to a rapid borealization of fish communities in the Arctic. Nat. Clim. Chang. 5, 673–677. doi: 10.1038/nclimate2647
Franke, A., Therrien, J.-F., Descamps, S., and Bêty, J. (2011). Climatic conditions during outward migration affect apparent survival of an arctic top predator, the peregrine falcon Falco peregrinus. J. Avian Biol. 42, 544–551. doi: 10.1111/j.1600-048X.2011.05466.x
Frederiksen, M., Daunt, F., Harris, M. P., and Wanless, S. (2008). The demographic impact of extreme events: stochastic weather drives survival and population dynamics in a long-lived seabird. J. Anim. Ecol. 77, 1020–1029. doi: 10.1111/j.1365-2656.2008.01422.x
Gaillard, J.-M., Festa-Bianchet, M., Yoccoz, N. G., Loison, A., and Toigo, C. (2000). Temporal variation in fitness components and population dynamics of large herbivores. Annu. Rev. Ecol. Syst. 31, 367–393. doi: 10.1146/annurev.ecolsys.31.1.367
Gaston, A. J., Smith, P. A., and Provencher, J. F. (2012). Discontinuous change in ice cover in Hudson Bay in the 1990s and some consequences for marine birds and their prey. ICES J. Mar. Sci. 69, 1218–1225. doi: 10.1093/icesjms/fss040
Gibson, G., Weijer, W., Jeffery, N., and Wang, S. (2020). Relative impact of sea ice and temperature changes on Arctic marine production. J. Geophys. Res. Biogeosci. 125:e2019JG005343. doi: 10.1029/2019JG005343
Gilchrist, H., and Mallory, M. L. (2005). Declines in abundance and distribution of the ivory gull (Pagophila eburnea) in Arctic Canada. Biol. Conserv. 121, 303–309. doi: 10.1016/j.biocon.2004.04.021
Grosbois, V., Gimenez, O., Gaillard, J.-M., Pradel, R., Barbraud, C., Clobert, J., et al. (2008). Assessing the impact of climate variation on survival in vertebrate populations. Biol. Rev. 83, 357–399. doi: 10.1111/j.1469-185X.2008.00047.x
Gutowsky, S. E., Baak, J. E., Gaston, A. J., and Mallory, M. L. (2022). Sea ice extent and phenology influence breeding of high-Arctic seabirds: 4 decades of monitoring in Nunavut. Can. Oecologia. 198, 393–406. doi: 10.1007/s00442-022-05117-8
Hilde, C. H., Gamelon, M., Sæther, B.-E., Gaillard, J.-M., Yoccoz, N. G., and Pélabon, C. (2020). The demographic buffering hypothesis: evidence and challenges. Trends Ecol. Evol. 35, 523–538. doi: 10.1016/j.tree.2020.02.004
Hop, H., Wold, A., Vihtakari, M., Daase, M., Kwasniewski, S., Gluchowska, M., et al. (2019). “Zooplankton in Kongsfjorden (1996–2016) in relation to climate change,” in The ecosystem of kongsfjorden, svalbard advances in polar ecology. eds. H. Hop and C. Wiencke (Cham: Springer International Publishing), 229–300.
Hovinen, J. E. H., Welcker, J., Descamps, S., Strøm, H., Jerstad, K., Berge, J., et al. (2014). Climate warming decreases the survival of the little auk (Alle alle), a high Arctic avian predator. Ecol. Evol. 4, 3127–3138. doi: 10.1002/ece3.1160
Iles, D. T., Lynch, H., Ji, R., Barbraud, C., Delord, K., and Jenouvrier, S. (2020). Sea ice predicts long-term trends in Adélie penguin population growth, but not annual fluctuations: results from a range-wide multiscale analysis. Glob. Chang. Biol. 26, 3788–3798. doi: 10.1111/gcb.15085
Jakubas, D., Iliszko, L., Wojczulanis-Jakubas, K., and Stempniewicz, L. (2012). Foraging by little auks in the distant marginal sea ice zone during the chick-rearing period. Polar Biol. 35, 73–81. doi: 10.1007/s00300-011-1034-x
Jansen, E., Christensen, J. H., Dokken, T., Nisancioglu, K. H., Vinther, B. M., Capron, E., et al. (2020). Past perspectives on the present era of abrupt Arctic climate change. Nat. Clim. Chang. 10, 714–721. doi: 10.1038/s41558-020-0860-7
Jenouvrier, S. (2013). Impacts of climate change on avian populations. Glob. Chang. Biol. 19, 2036–2057. doi: 10.1111/gcb.12195
Karnovsky, N., Brown, Z., Welcker, J., Harding, A., Walkusz, W., Cavalcanti, A., et al. (2011). Inter-colony comparison of diving behavior of an Arctic top predator: implications for warming in the Greenland Sea. Mar. Ecol. Prog. Ser. 440, 229–240. doi: 10.3354/meps09351
Kwasniewski, S., Hop, H., Falk-Petersen, S., and Pedersen, G. (2003). Distribution of Calanus species in Kongsfjorden, a glacial fjord in Svalbard. J. Plankton Res. 25, 1–20. doi: 10.1093/plankt/25.1.1
Lebreton, J.-D., Burnham, K. P., Clobert, J., and Anderson, D. R. (1992). Modeling survival and testing biological hypotheses using marked animals: a unified approach with case studies. Ecol. Monogr. 62, 67–118. doi: 10.2307/2937171
Leu, E., Søreide, J. E., Hessen, D. O., Falk-Petersen, S., and Berge, J. (2011). Consequences of changing sea-ice cover for primary and secondary producers in the European Arctic shelf seas: timing, quantity, and quality. Prog. Oceanogr. 90, 18–32. doi: 10.1016/j.pocean.2011.02.004
Lønne, O. J., and Gabrielsen, G. W. (1992). Summer diet of seabirds feeding in sea-ice-covered waters near Svalbard. Polar Biol. 12, 685–692. doi: 10.1007/BF00238868
Lydersen, C., Assmy, P., Falk-Petersen, S., Kohler, J., Kovacs, K. M., Reigstad, M., et al. (2014). The importance of tidewater glaciers for marine mammals and seabirds in Svalbard, Norway. J. Mar. Syst. 129, 452–471. doi: 10.1016/j.jmarsys.2013.09.006
MacNeil, M. A., Graham, N. A. J., Cinner, J. E., Dulvy, N. K., Loring, P. A., Jennings, S., et al. (2010). Transitional states in marine fisheries: adapting to predicted global change. Philos. Trans. Royal Soc. B: Biol. Sci. 365, 3753–3763. doi: 10.1098/rstb.2010.0289
Mehlum, F., and Gabrielsen, G. W. (1993). The diet of high-Arctic seabirds in coastal and ice-covered, pelagic areas near the Svalbard archipelago. Polar Res. 12, 1–20. doi: 10.1111/j.1751-8369.1993.tb00417.x
Moe, B., Stempniewicz, L., Jakubas, D., Angelier, F., Chastel, O., Dinessen, F., et al. (2009). Climate change and phenological responses of two seabird species breeding in the high-Arctic. Mar. Ecol. Prog. Ser. 393, 235–246. doi: 10.3354/meps08222
Møller, E. F., Johansen, K. L., Agersted, M. D., Rigét, F., Clausen, D. S., Larsen, J., et al. (2018). Zooplankton phenology may explain the north water polynya’s importance as a breeding area for little auks. Mar. Ecol. Prog. Ser. 605, 207–223. doi: 10.3354/meps12745
Morris, W. F., and Doak, D. F. (2004). Buffering of life histories against environmental stochasticity: accounting for a spurious correlation between the variabilities of vital rates and their contributions to fitness. Am. Nat. 163, 579–590. doi: 10.1086/382550
Pabi, S., van Dijken, G. L., and Arrigo, K. R. (2008). Primary production in the Arctic Ocean, 1998–2006. J. Geophys. Res. 113:C08005. doi: 10.1029/2007JC004578
Perrette, M., Yool, A., Quartly, G. D., and Popova, E. E. (2011). Near-ubiquity of ice-edge blooms in the Arctic. Biogeosciences 8, 515–524. doi: 10.5194/bg-8-515-2011
Persson, O., and Vihma, T. (2017). “The atmosphere over sea ice” in Sea Ice (John Wiley & Sons Ltd.), 160–196.
Poloczanska, E. S., Burrows, M. T., Brown, C. J., García Molinos, J., Halpern, B. S., Hoegh-Guldberg, O., et al. (2016). Responses of marine organisms to climate change across oceans. Front. Mar. Sci. 3:62. doi: 10.3389/fmars.2016.00062
Pradel, R., Hines, J. E., Lebreton, J.-D., and Nichols, J. D. (1997). Capture-recapture survival models taking account of transients. Biometrics 53, 60–72. doi: 10.2307/2533097
Pradel, R., and Sanz-Aguilar, A. (2012). Modeling trap-awareness and related phenomena in capture-recapture studies. PLoS One 7:e32666. doi: 10.1371/annotation/e240f425-0375-4c32-b0a7-85fa586d0f40
Ramírez, F., Tarroux, A., Hovinen, J., Navarro, J., Afán, I., Forero, M. G., et al. (2017). Sea ice phenology and primary productivity pulses shape breeding success in Arctic seabirds. Sci. Rep. 7:4500. doi: 10.1038/s41598-017-04775-6
Sæther, B.-E., and Bakke, Ø. (2000). Avian life history variation and contribution of demographic traits to the population growth rate. Ecology 81, 642–653. doi: 10.1890/0012-9658(2000)081[0642:ALHVAC]2.0.CO;2
Sæther, B.-E., Grøtan, V., Engen, S., Coulson, T., Grant, P. R., Visser, M. E., et al. (2016). Demographic routes to variability and regulation in bird populations. Nat. Commun. 7, 1–8. doi: 10.1038/ncomms12001
Sæther, B.-E., Sutherland, W. J., and Engen, S. (2004). Climate influences on avian population dynamics. Adv. Ecol. Res. 35, 185–209. doi: 10.1016/S0065-2504(04)35009-9
Sandvik, H., Erikstad, K. E., and Sæther, B.-E. (2012). Climate affects seabird population dynamics both via reproduction and adult survival. Mar. Ecol. Prog. Ser. 454, 273–284. doi: 10.3354/meps09558
Serreze, M. C., and Barry, R. G. (2011). Processes and impacts of Arctic amplification: a research synthesis. Glob. Planet. Chang. 77, 85–96. doi: 10.1016/j.gloplacha.2011.03.004
Skalski, J. R. (1996). Regression of abundance estimates from mark–recapture surveys against environmental covariates. Can. J. Fish. Aquat. Sci. 53, 196–204. doi: 10.1139/f95-169
Smith, P., and Gaston, A. (2012). Environmental variation and the demography and diet of thick-billed murres. Mar. Ecol. Prog. Ser. 454, 237–249. doi: 10.3354/meps09589
Spreen, G., Kaleschke, L., and Heygster, G. (2008). Sea ice remote sensing using AMSR-E 89-GHz channels. J. Geophys. Res. Oceans 113. doi: 10.1029/2005JC003384
Stammerjohn, S. E., Martinson, D. G., Smith, R. C., and Iannuzzi, R. A. (2008). Sea ice in the western Antarctic peninsula region: Spatio-temporal variability from ecological and climate change perspectives. Deep-Sea Res. II Top. Stud. Oceanogr. 55, 2041–2058. doi: 10.1016/j.dsr2.2008.04.026
Stocker, T. F., Qin, D., Plattner, G.-K., Tignor, M. M., Allen, S. K., Boschung, J., et al. (2013). “Climate change 2013: the physical science basis,’’ in Contribution of working group I to the fifth assessment report of IPCC the intergovernmental panel on climate change. (Cambridge: Cambridge University Press).
Strøm, H., Bakken, V., Skoglund, A., Descamps, S., Fjeldheim, V., and Steen, H. (2020). Population status and trend of the threatened ivory gull Pagophila eburnea in Svalbard. Endanger. Species Res. 43, 435–445. doi: 10.3354/esr01081
Tarling, G. A., Freer, J. J., Banas, N. S., Belcher, A., Blackwell, M., Castellani, C., et al. (2022). Can a key boreal Calanus copepod species now complete its life-cycle in the Arctic? Evidence and implications for Arctic food-webs. Ambio 51, 333–344. doi: 10.1007/s13280-021-01667-y
Van Leeuwe, M. A., Tedesco, L., Arrigo, K. R., Assmy, P., Campbell, K., Meiners, K. M., et al. (2018). Microalgal community structure and primary production in Arctic and Antarctic Sea ice: a synthesis. Elem.: Sci. Anthrop. 6:4. doi: 10.1525/elementa.267
Vihtakari, M., Welcker, J., Moe, B., Chastel, O., Tartu, S., Hop, H., et al. (2018). Black-legged kittiwakes as messengers of Atlantification in the Arctic. Sci. Rep. 8:1178. doi: 10.1038/s41598-017-19118-8
Wassmann, P., Duarte, C. M., Agustí, S., and Sejr, M. K. (2011). Footprints of climate change in the Arctic marine ecosystem. Glob. Chang. Biol. 17, 1235–1249. doi: 10.1111/j.1365-2486.2010.02311.x
Węsławski, J. M., Dragańska-Deja, K., Legeżyńska, J., and Walczowski, W. (2018). Range extension of a boreal amphipod Gammarus oceanicus in the warming Arctic. Ecol. Evol. 8, 7624–7632. doi: 10.1002/ece3.4281
Will, A., Takahashi, A., Thiebot, J.-B., Martinez, A., Kitaiskaia, E., Britt, L., et al. (2020). The breeding seabird community reveals that recent sea ice loss in the Pacific Arctic does not benefit piscivores and is detrimental to planktivores. Deep-Sea Res. II Top. Stud. Oceanogr. 181-182:104902. doi: 10.1016/j.dsr2.2020.104902
Wojczulanis-Jakubas, K., Jakubas, D., and Stempniewicz, L. (2009). Sex-specific parental care by incubating little auks (Alle alle). Ornis Fenn 86, 140–148.
Wojczulanis-Jakubas, K., Jiménez-Muñoz, M., Jakubas, D., Kidawa, D., Karnovsky, N., Cole, D., et al. (2020). Duration of female parental care and their survival in the little auk Alle alle - are these two traits linked? Behav. Ecol. Sociobiol. 74:82. doi: 10.1007/s00265-020-02862-9
Keywords: Rissa tridactyla, Uria lomvia, Alle alle, survival, breeding success, sea-ice concentration, Svalbard
Citation: Sauser C, Angelier F, Blévin P, Chastel O, Gabrielsen GW, Jouanneau W, Kato A, Moe B, Ramírez F, Tartu S and Descamps S (2023) Demographic responses of Arctic seabirds to spring sea-ice variations. Front. Ecol. Evol. 11:1107992. doi: 10.3389/fevo.2023.1107992
Edited by:
Yan Jiao, Virginia Tech, United StatesReviewed by:
Lech Stempniewicz, University of Gdansk, PolandRujia Bi, University of Wisconsin-Madison, United States
Copyright © 2023 Sauser, Angelier, Blévin, Chastel, Gabrielsen, Jouanneau, Kato, Moe, Ramírez, Tartu and Descamps. This is an open-access article distributed under the terms of the Creative Commons Attribution License (CC BY). The use, distribution or reproduction in other forums is permitted, provided the original author(s) and the copyright owner(s) are credited and that the original publication in this journal is cited, in accordance with accepted academic practice. No use, distribution or reproduction is permitted which does not comply with these terms.
*Correspondence: C. Sauser, Y2hyaXN0b3BoZS5zYXVzZXJAZ21haWwuY29t