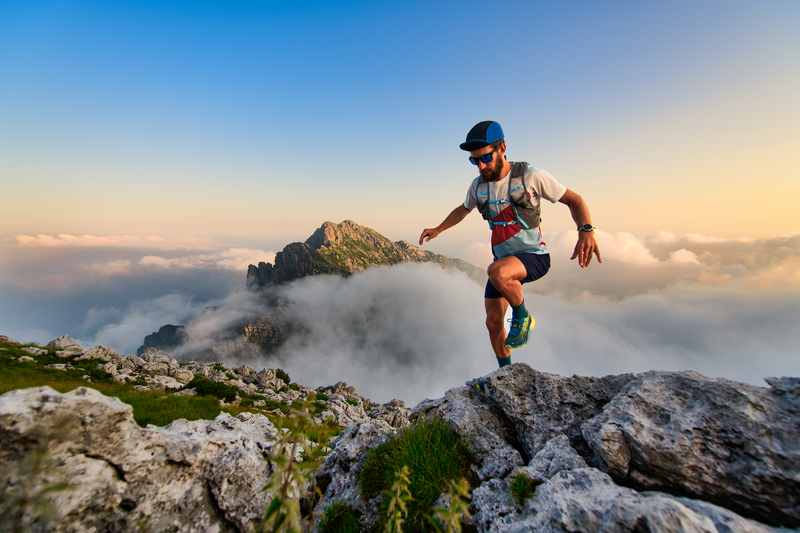
95% of researchers rate our articles as excellent or good
Learn more about the work of our research integrity team to safeguard the quality of each article we publish.
Find out more
ORIGINAL RESEARCH article
Front. Ecol. Evol. , 10 February 2023
Sec. Population, Community, and Ecosystem Dynamics
Volume 11 - 2023 | https://doi.org/10.3389/fevo.2023.1105725
This article is part of the Research Topic Untangling the Complexity: Recent Advances in Understanding Interactions Between Plants, Pathogens and Herbivorous Insects View all 5 articles
Plants are attacked by multiple insect pest species and insect herbivory can alter plant defense mechanisms. The plant defense responses to a specific herbivore may also contribute to the herbivore growth/survival on plants. Feeding by one insect species can modulate the plant defenses, which can either facilitate or hamper the colonization of subsequent incoming insects. However, little is known about the effect of sequential herbivory on sorghum plants. In this study, we demonstrate that a specialist aphid, sugarcane aphid (SCA; Melanaphis sacchari) grows faster on sorghum than a generalist aphid species, greenbug (GB; Schizaphis graminum). We also determined how the pre-infestation of SCA on sorghum affected the invasion of GB and vice-versa. Our sequential herbivory experiments revealed that SCA reproduction was lower on GB-primed sorghum plants, however, the reverse was not true. To assess the differences in plant defenses induced by specialist vs. generalist aphids, we monitored the expression of salicylic acid (SA) and jasmonic acid (JA) marker genes, and flavonoid biosynthetic pathway genes after 48 h of aphid infestation. The results indicated that GB infestation induced higher expression of SA and JA-related genes, and flavonoid pathway genes (DFR, FNR, and FNSII) compared to SCA infestation. Overall, our results suggested that GB-infested plants activate the plant defenses via phytohormones and flavonoids at early time points and hampers the colonization of incoming SCA, as well as explain the reproductive success of SCA compared to GB.
Sorghum (Sorghum bicolor) is one of the most important monocot crops grown for food, feed, and/or fuel. In 2022, it was grown on 41.10 million hectares worldwide, with an annual projected production of 62.67 million metric tons, and in the United States, it was planted on 2.23 million hectares with an annual projected production of 9.68 million metric tons.1 Sorghum possesses an innate ability to tolerate heat and moisture stress, making its cultivation more viable in dry areas (Balakrishna et al., 2020). Additionally, sorghum grains serve as a rich source of bioactive nutrients (Przybylska-Balcerek et al., 2019). The presence of antioxidant phenolic compounds in sorghum offers health benefits such as anti-microbial, anti-cancer, and anti-inflammatory activities (Rao et al., 2018). However, at least 150 insect species are known to infest sorghum crop (Guo et al., 2011) and cause significant yield losses. Some of the important sorghum insects that attack sorghum include sugarcane aphid (SCA; Melanaphis sacchari), greenbug (GB; Schizaphis graminum), chinch bug (Blissus leucopterus leucopterus), corn leaf aphid (Rhopalosiphum maidis), fall armyworm (Spodoptera frugiperda), corn earworm (Helicoverpa zea), true armyworm (Pseudaletia unipuncta), and sorghum midge (Stenodiplosis sorghicola).
GB and SCA are among the most important and serious sap-sucking pests of sorghum ever since they were recognized as pests on sorghum in North America in 1968 and 2013, respectively (Porter et al., 1997; Bowling et al., 2016). GB can feed on over 70 graminaceous plant species whereas SCA prefers sorghum and Johnson grass (Michels, 1986; Armstrong et al., 2015). GB is distributed globally in regions like Asia, southern Europe, Africa, the Middle East, and North and South America (Royer et al., 2015). SCA is present in nearly 30 countries around the world where sugarcane (Saccharum officinarum) and sorghum are commonly cultivated (Singh et al., 2004). GB appears in the field on seedlings, booting, and heading stage (Cronholm et al., 2007) and SCA can infest the plants starting from emergence until harvesting (Zapata et al., 2018). Similar to other aphids, both GB and SCA are phloem-feeding insects equipped with piercing and sucking type mouthparts causing minimal visible injury to plant tissue but ingest the phloem sap using their stylets (Ma et al., 1990; Singh et al., 2004). Plant damage occurs due to direct loss of nutrients, leaf chlorosis, and reduced photosynthetic ability following the development of secondary sooty mold on honeydew excreted by the aphids (Singh et al., 2004). During feeding, GB injects toxic enzymes into the leaf tissue like pectinases, which causes tissue necrosis and cell wall degradation (Ma et al., 1990). They are also known to cause indirect damage by vectoring different plant viral diseases (Berger et al., 1987; Akbar et al., 2010). Around 20 biotypes of GB were identified on different cereal crops such as wheat, barley, and sorghum; however, biotypes C, E, I, and K are known to be more harmful to the crops (Harris-Shultz et al., 2019). SCAs are documented as “superclones” due to their ability to reproduce asexually and have high reproduction rates and, are spread over a large geographical range in the United States (Harris-Shultz et al., 2017). In sorghum fields, SCA population can increase exponentially within 2 weeks of flowering. The outbreak of SCA has significantly caused yield losses in sorghum-producing areas (Szczepaniec, 2018; Zapata et al., 2018).
As herbivore initiates feeding on plant, it disturbs the integrity of plant tissues and mechanical damage alone can trigger defense responses in plants (Bricchi et al., 2010). Plants also recognize compounds released by herbivores during feeding. Numerous studies have shown that the oral secretions, saliva, or digestive waste products (e.g., frass, honey dew) of insects, have the ability to enhance the defense response of plants (Turlings et al., 1993; Musser et al., 2002; Schwartzberg and Tumilison, 2013). Upon recognition of herbivore-associated cues, plant activates a cascade of defense responses. As a counter-defense mechanism, herbivores have also evolved the capability to suppress plant defenses through its effector molecules (Musser et al., 2002; Basu et al., 2018; Nalam et al., 2019). Plant defense against insect pests is mediated by numerous signaling pathways that are regulated by phytohormones, for example, jasmonic acid (JA) and salicylic acid (SA; Robert-Seilaniantz et al., 2011). Plants exhibit distinct kinds of defense responses to piercing and chewing insects (De Vos et al., 2005; Bidart-Bouzat and Kliebenstein, 2011). Phloem-feeding insects mostly induce SA-mediated defense responses (Zhang et al., 2013; Nalam et al., 2019). The SA pathway regulates a wide range of defense genes such as Pathogenesis-related Protein (PR) encoding genes. For example, potato aphid (Macrosiphum euphorbiae) feeding on tomato plants can stimulate the expression of PR proteins regulated by SA pathway (Martinez De Ilarduya et al., 2003). On the contrary, chewing insects induce plant defense by JA signaling. JA is synthesized through the oxylipin pathway and wounding/herbivory induces the expression of JA-defense-responsive genes like protease inhibitors (PIN) and wound-induced proteins (WIP; Wang and Wu, 2013). However, JA is also documented in providing resistance against piercing and sucking types of insects like aphids and whiteflies (Zhu-Salzman et al., 2004; Sun et al., 2017).
Plants synthesize a variety of secondary metabolites as defensive traits against insects. These metabolites can be constitutively produced in the plants or induced upon insect attack (War et al., 2012). They may negatively affect the behavior of the insects by displaying antixenosis, which deters the insect to feed, oviposit and shelter on plants (non-preference; Kogan and Ortman, 1978; Smith and Clement, 2012; Padmaja, 2016) or adversely affect the biology of the insect when the insect feeds on the plant, also known as antibiosis (Painter, 1951; Padmaja, 2016). One of the important secondary metabolites includes the flavonoids, which are synthesized through phenylpropanoid pathway (Falcone Ferreyra et al., 2012). Different classes of flavonoids include flavone, flavanones, isoflavones, flavanols, anthocyanins, leucoanthocyanins, and proanthocyanins (Mierziak et al., 2014; Singh et al., 2021). Flavonoids are known to provide resistance against sap-feeding insects. For example, pisatin, a known flavonoid in pea (Pisum sativum) provides resistance against pea aphid (Acyrthosiphon pisum; Morkunas et al., 2016). Similarly, it was recently demonstrated that flavonoid 3-deoxyanthocyanidin is responsible for conferring resistance against R. maidis in sorghum (Kariyat et al., 2019).
Most research on host-plant interactions uses pairwise comparisons with a single host and pest species, limiting the ability to mimic field conditions where crops are not only attacked by a single insect, but by diverse insect pests. Since the herbivore-associated molecular patterns present in the oral secretions or saliva of herbivores, herbivore-associated endosymbionts, and recently studied insect frass play a major role in regulating plant defense response, mode of herbivore feeding is a key factor in understanding plant–insect interactions (Hogenhout and Bos, 2011; Basu et al., 2018; Chen and Mao, 2020). When plants are attacked by multiple insects, the outcome of plant-mediated interactions between herbivores is influenced by several factors, including the identity of the attacking herbivore, host plant species, and importantly, the sequence of the herbivore incidence (Erb et al., 2011; Stam et al., 2014; Mertens et al., 2021; de Bobadilla et al., 2022). For example, green peach aphid (Myzus persicae) infested potato (Solanum tuberosum) plants attracted more M. euphorbiae as compared to uninfested plants (Brunissen et al., 2009). Similarly, cabbage (Brassica oleracea L.) plants pre-infested with cabbage aphids, Brevicoryne brassicae, supported faster growth of cabbage butterfly caterpillars (Pieris brassicae; Soler et al., 2012). In maize (Zea mays), it has been shown that the arrival of leaf feeder (S. frugiperda) impacts colonization of root feeder (Diabrotica virgifera virgifera) adversely, if leaf feeder arrives before root feeder (Erb et al., 2011; Huang et al., 2017). Consequently, the plants attacked by S. frugiperda are not accepted by D. v. virgifera larvae to initiate the feeding (Huang et al., 2017). However, only a few studies have reported about the changes in plant resistance with successive herbivory by different insect species in sorghum (Bayoumy et al., 2016; Michaud et al., 2017). Previously, it has been shown that SCA feeding can improve the suitability of sorghum as a host plant for the bird cherry-oat aphid (Michaud et al., 2017). In a separate study, GBs have been shown to benefit from co-infestation with SCA on the susceptible cultivar (Bayoumy et al., 2016). However, the sequential herbivory effects on GB and SCA growth are unknown. The objective of this study was to determine the effects of sequential aphid attack on sorghum plants and to understand the differential defense responses in sorghum following SCA and GB attacks, during the early infestation period. Since plant defense response is mediated by the JA/SA signaling pathway, we also examined possible cross-talk of SA and JA signaling and their response to different aphid pests.
Sorghum genotype RTx430, an elite reference line (Bouchet et al., 2017) was used for this study. Plants were grown in Cone-tainers (Ray Leach SC10; 427 Stuewe & Sons, Inc., Tangent, OR) filled with soil mixture containing vermiculite and perlite (PRO-MIX BX BIOFUNGICIDE + MYCORRHIZAE, Premier Tech Horticulture Ltd., Canada) at the University of Nebraska-Lincoln greenhouse. The GB and SCA colonies were reared as previously described (for details see Grover et al., 2019; Tetreault et al., 2019) and were maintained on the common susceptible BCK60 sorghum genotype in different growth chambers. Plants and aphid colonies were raised under similar conditions of 16-h-light/8-h-dark photoperiod, 25°C, and 50%–60% relative humidity. Plants were watered regularly and fertigated (N:P:K::20:10:20) once a week. The BCK60 sorghum plants for aphid rearing were grown in the greenhouse until they reached the 7-leaf stage. Older plants were regularly replaced with new plants in the growth chamber on a need basis. Two-week-old plants at the three-leaf stage were used for all the experiments and experiments were performed in the same conditions in which the plants were grown (Vanderlip and Reeves, 1972). Newly emerged adult apterous aphids were used for all the experiments (Grover et al., 2019, 2022a,b).
For aphid reproduction bioassays, two-week-old RTx430 sorghum plants were infested with either 10 SCA or GB and covered with plastic cages (Michaud et al., 2017; Grover et al., 2020). Total number of aphids was counted on each plant after 96 h post-infestation (hpi; n = 18–20). For the sequential herbivory experiment, two-week-old RTx430 sorghum plants were infested with either 10 SCA or GB and covered with plastic cages. Uninfested plants were also caged that served as controls for the respective aphid treatment. After 48 h, aphids were taken off the plants with a paintbrush and all the plants were double-checked to make sure plants are free from aphids. The GB-infested plants were re-infested with 10 adult SCA and vice-versa. Finally, total number of aphids on each plant was counted after 96 hpi (n = 14). The aphid bioassays were repeated twice.
For gene expression studies, the plants were infested with either GB or SCA on the leaf opposite to the whorl and enclosed in clip cages. Aphid uninfested plants served as controls. At 48 hpi, leaf tissue samples around the clip cages were collected from plants in eppendorf tubes containing beads and immediately placed in liquid nitrogen. Samples were homogenized in a 2010 Geno/Grinder (SPEX SamplePrep) for 30 s. RNA extraction was performed using Zymo Research RNA Clean & Concentrator (Research Irvine, CA, United States) following the manufacturer protocol. Total RNA was reverse transcribed to cDNA using a High-Capacity cDNA reverse transcriptase kit (Applied Biosystems, Foster City, CA). RT-qPCR was performed with StepOnePlus Real-Time PCR System (Applied Biosystems, Foster City, CA). Tubulin was used as the internal control due to its stable gene expression in sorghum under different stress conditions (Scully et al., 2016; Funnell-Harris et al., 2019; Huang and Shrestha, 2022; Shrestha and Huang, 2022; Grover et al., 2022c). The list of gene-specific primers is mentioned in Supplementary Table S1. The RT-qPCR thermocycler conditions were as follows: initial denaturation was done at 95°C for 30 s (s) followed by 40 cycles of two steps (i) denaturation at 95°C for 5 s, (ii) annealing at 58°C for 10 s. The melt curve stage was performed at 65°C for 5 s with an increment of 0.5°C till it reached 95°C. A total of four biological replicates and two technical replicates corresponding to each biological replicate were used for relative gene expression analysis. The relative expression was calculated using the 2–ΔΔCT formula (Livak and Schmittgen, 2001).
The aphid no-choice assays data were analyzed using a mixed model and replications were considered random effects (PROC GLIMMIX, SAS 9.3, SAS Institute). The factors were SCA or GB treated plants and the response variable was total aphid numbers counted on each plant. Negative binomial distribution was used to analyze the aphid count data. The gene expression data were analyzed using one-way ANOVA. The calculated 2–ΔΔCT values were log-transformed to perform statistical analyses. Pairwise comparisons between treatments were computed using student’s t-test (p < 0.05).
To compare the reproduction of SCA and GB, aphid numbers (both adults and nymphs) were counted on RTx430 plants at 96 hpi. We found that SCA reproduced significantly more on sorghum RTx430 plants compared to GB (Figure 2, F1,26 = 76.6884; p < 0.0001).
To determine how the level of plant defenses induced by one aphid species can affect the reproduction of other aphid species, we assessed the GB numbers on SCA pre-infested plants and vice-versa after 96 hpi (Figure 1A). Our results demonstrate that GB pre-infested plants provide enhanced resistance to SCA as the plants supported a lower number of SCA (nymphs and adults) compared to uninfested control plants (Figure 1B, F1,55 = 4.3085; p < 0.0426). However, SCA pre-infested plants support similar numbers of GB as SCA uninfested control plants (Figure 1C, F1,41 = 0.5858; p < 0.4484). Collectively, our results suggest that GB pre-infested plants imposed stronger antibiotic effects on SCA, whereas SCA pre-infested plants did not affect GB reproduction.
Figure 1. Mean number of aphids counted on RTx430 at 96 h post infestation (n = 18–20). Different letters above the bars indicate significant difference between the mean number of aphids found (p < 0.05). Error bars represent mean ± SE.
Figure 2. Bioassay setup for comparing greenbug (GB) and sugarcane aphid (SCA) reproduction (A) (i) One set of plants was infested with 10 GB, which were removed after 48 h and replaced with 10 SCA. The other set served as control that was also infested with 10 SCA after 48 h (n = 14). SCA were counted on both sets of plants after 96 h post-SCA infestation. (ii) One set of plants was infested with 10 SCA, which were removed after 48 h and replaced with 10 GB. Control plants were infested with 10 GB after 48 h (n = 14). GB were counted on both sets of plants 96 h post-GB infestation. (B) Mean number of SCA were counted on GB-uninfested control plants and GB pre-infested plants at 96 hpi (n = 14). (C) Mean number of GB were counted on SCA-uninfested control plants and SCA pre-infested plants at 96 hpi (n = 11). Different letters above the bars indicate significant difference between the mean number of aphids found (p < 0.05). Error bars represent mean ± SE.
To investigate if the GB/SCA-infested plants induce the plant defenses via SA, we measured the relative expression of SA-responsive marker genes, PATHOGENESIS-RELATED PROTEIN 1 (PR1) and PR10. At 48 hpi, GB-infested plants induced significantly higher expression levels of PR1 and PR10 compared to SCA-infested and control plants (Figure 3A, F2,9 = 52.7063; p < 0.0002; Figure 3B, F2,9 = 36.1633; p < 0.0001). Expression of PR10 in SCA-infested plants was also significantly higher as compared to control plants (Figure 3B). To determine if sorghum activates jasmonate-mediated defense response in response to GB/SCA, we measured the expression of two genes, PROTEASE INHIBITOR 2 (PIN2) and WOUND-INDUCED PROTEIN (WIP). Expression levels of PIN2 and WIP were significantly higher in GB-infested plants compared to SCA-infested and control plants (Figure 4A, F2,9 = 4.5625; p = 0.0428; Figure 4B, F2,9 = 7.0843; p = 0.0142). However, the expression levels of both genes in SCA-infested plants did not significantly differ from control plants (Figure 4). Overall, the results showed that GB induced higher expression levels of SA and JA-related genes in sorghum as compared to SCA.
Figure 3. Relative expression levels of salicylic acid-related defense marker genes (A) Pathogenesis-Related Protein 1 (PR1), (B) Pathogenesis-Related Protein 10 (PR10) after 48 h of infestation of sorghum RTx430 plants (n = 3–4). Different letters above the bars indicate values that are significantly different from each other (p < 0.05). Error bars represent ± SE.
Figure 4. Relative expression levels of jasmonic acid defense responsive marker genes (A) Protease Inhibitor 2 (PIN2), and (B) Wound-Induced Protein (WIP) after 48 h of infestation of sorghum RTx430 plants (n = 4). Different letters above the bars indicate values that are significantly different from each other (p < 0.05). Error bars represent ± SE.
We also measured the expression of three key genes of the flavonoid biosynthesis pathway, dihydroflavonol 4-reductase (DFR), flavanone 4-reductase (FNR), and flavonoid 3—hydroxylase (FNSII). We found that GB infestation led to a significant increase in the expression of DFR, FNR, and FNSII compared to SCA-infested and control plants (Figure 5A, F2,6 = 18.6914; p = 0.0026; Figure 5B, F2,6 = 3.7611; p = 0.0874; and Figure 5C, F2,6 = 3.1326; p < 0.0007). These results collectively demonstrated that plants induce flavonoid pathway genes against GB attack, but they did not induce those genes against SCA at 48 hpi.
Figure 5. Relative expression of flavonoid pathway genes (A) Dihydroflavonol 4-reductase (DFR3), (B) Flavanone 4-reductase (FNR), and (C) Flavonoid 3-hydroxylase (FNSII) after 48 h of infestation of sorghum RTx430 plants (n = 3). Different letters above the bars indicate values that are significantly different from each other (p < 0.05). Error bars represent ± SE.
Under natural settings, plants are attacked by multiple insect pests. Interspecific competition among plants influences the structure, function, and stability of natural and agricultural ecosystems. For herbivorous insects, interspecific competition can occur through direct interference or plant-mediated indirect effects. The sequence of arrival of different insect herbivore species on a plant has been proposed as an important factor shaping the outcome of plant-mediated interactions among them. SCA and GB are two key piercing-sucking key pests of sorghum, while SCA acts as a specialist, GB acts more as a generalist pest based on their host range. Several reports showed that there are sorghum genotypes which show resistance to both GB and SCA (Armstrong et al., 2015; Grover et al., 2019, 2022b). However, no information is available on the sequential herbivory of GB and SCA on sorghum and its defense response. In the current study, we studied the effects of sequential herbivory of GB and SCA and plant defense responses to their attack. We found that sorghum plants support fewer GB as compared to SCA (Figure 2). Furthermore, our study revealed that pre-infestation of sorghum plants with GB initiate strong defense responses that affect the reproduction of subsequent arriving insect, i.e., SCA, however, the reverse was not true (Figures 1B,C). Similar results were observed when sorghum plants preconditioned with bird cherry-oat aphids (R. padi) negatively affected the SCA survival, whereas SCA preconditioned plants benefitted the bird cherry-oat aphids (Michaud et al., 2017). During the co-infestation of GB and SCA on sorghum plants, SCA had a positive effect on the developmental and reproductive rates of GB (Bayoumy et al., 2016). To identify the differences in plant defense response to two different aphids, we analyzed the expression of genes related to plant defense upon GB and SCA infestation. Our results showed that GB infestation induced a higher expression of PR protein-encoding genes, PR1 and PR10, compared to SCA infestation at 48 hpi (Figures 3A,B). Flavonoid biosynthetic pathway genes were also found induced in sorghum after GB infestation, but not after SCA infestation. Overall, our results suggested that sorghum plants have the ability to defend better against GB as compared to SCA, which highly likely could result in higher reproduction of SCA compared to GB.
Previously, we have reported that SCA feeding suppresses and upregulates the PR proteins at 1 and 7 dpi, respectively (Grover et al., 2022a). Our current results align with our proteomic data as the expression of the PR1 gene was downregulated compared to control plants at an early stage of aphid feeding. SA is well known for providing defense predominantly against sap-sucking insects by inducing the generation of PR proteins (Li et al., 2006; Zarate et al., 2007). NON-EXPRESSOR OF PATHOGENESIS-RELATED GENE 1 (NPR1) is a receptor protein of SA involved in plant defenses whose expression was increased after SCA feeding on the resistant sorghum genotype (Kiani and Szczepaniec, 2018). Previously, we have also shown that SCA feeding induces SA levels at 7 dpi in sorghum (Grover et al., 2022b). Other hemipterans, for example, the Russian wheat aphid, Diuraphis noxia (Mordvilko), also induces the production of SA in wheat providing resistance to aphids (Mohase and Van Der Westhuizen, 2002). Aphid feeding can also impact the nutritional quality of the plants by increasing the amino acid contents, which may also regulate the plant immunity (Sandström et al., 2000; Zeier, 2013). For example, phenylalanine is the major amino acid involved in the biosynthesis of SA that further regulate the plant defenses (Shah, 2003). Arabidopsis thaliana ALD1 (AGD2-LIKE DEFENSE RESPONSE PROTEIN1) gene is important for PR gene expression and SA production and is an aminotransferase having a high activity toward lysine (Jong et al., 2004). The ald1 had attenuated levels of SA upon pathogen attack, which suggested that plant defenses were regulated through amino acid via SA signaling (Jong et al., 2004). In addition to SA, JA has also been shown to provide deterrence/defense against sap-sucking insects in few instances (Zhu-Salzman et al., 2004; Xu et al., 2021). Global transcriptomic analysis of SCA-resistant sorghum genotype revealed that the expression of genes encoding JA signaling transcription factors was increased at 5 dpi compared to the susceptible genotype (Tetreault et al., 2019). Our results also revealed that JA-related defense genes PIN2 and WIP had higher expression levels in GB-infested plants (Figures 4A,B). Transcriptomic and metabolomic results of wheat plants infested with either GB or grain aphid (Sitobion avenae) had shown that GB induced a stronger defense response on wheat by increasing the levels of SA and also the expression of several PR and PIN genes as compared to S. avenae infested plants (Zhang et al., 2019a,b). Heightened expression of SA and JA-related genes after GB infestations have provided evidence that SA and JA are involved in priming the sorghum plants to provide enhanced resistance to subsequent SCA attack.
Flavonoids are among the major secondary metabolites, which are important for plant growth and have properties to defend the plant against herbivory (Makoi et al., 2010; Singh et al., 2021). Cowpea (Vigna unguiculata) plants grown from the seeds, which have higher concentrations of flavonoids and anthocyanins, were less attacked by aphids, thrips and pod-sucking bugs (Makoi et al., 2010). Our data showed that GB infestation induced the expression of three flavonoid genes DFR, FNR, and FNSII but expression of these genes was decreased or not altered in SCA-infested plants compared to control plants (Figures 5A–C). Similarly, transcriptomic analysis of SCA-infested sorghum-resistant line displayed the downregulation of DFR gene (Tetreault et al., 2019). This depicts that feeding by GB initiates the defense response in sorghum via flavonoid biosynthesis, which eventually impacts the performance of SCA on GB pre-infested plants. Similarly in barley, the toxicity of secondary metabolites such as phenolics and flavonoids have collectively provided resistance to GB (Todd et al., 1971). Several flavonoid related genes in cucumber (Cucumis sativus L.) had increased gene expression after 2 days post infestation of Aphis gossypii but later their expression decreased, suggesting that plants rapidly activates defense via flavonoids upon aphid herbivory (Liang et al., 2015). These studies also display that the induction of plant defenses depends on the insect species attacking the plants. Flavonoids have been shown to damage the peritrophic matrix, which provides protection to the caterpillar midgut (Chatterjee et al., 2022). However, flavonoids are known to affect aphid feeding behavior (Goławska and Łukasik, 2012). But the exact mechanism of flavonoids affecting aphid growth is not fully understood.
Feeding by insects belonging to similar guild generally induce similar kind of plant defense response at molecular level due to the same feeding habit (Erb et al., 2012). However this is not true in all the cases (Bidart-Bouzat and Kliebenstein, 2011). Variability in the defense response levels could occur depending on the insect species. For example, even though tobacco hornworm (Manduca sexta) and beet armyworm (S. exigua) are lepidopteran pests, the tobacco (Nicotiana attenuata) plants infested with these pests separately showed contrasting phytohormonal signaling (Diezel et al., 2009). Also, insects belonging to different guilds may induce differential plant defenses and affect the incoming pests. S. exigua larvae performance was negatively impacted on plants previously fed by either aphids or S. exigua or both (Rodriguez-Saona et al., 2010). Furthermore, simultaneous herbivory by two insects can have negative impact on each other and on the subsequent pest invasion. For example, dual herbivory by caterpillars (Plutella xylostella) and aphids (B. brassicae) negatively affected each other’s performance and also the impacted the growth of subsequent incoming pest, cabbage moth (Mamestra brassicae; Kroes et al., 2016). The carmine spider mite (Tetranychus cinnabarinus) pre-infested cucumber plants were more attractive to whitefly Bemisia tabaci as compared to the mealybug (Phenacoccus solenopsis) pre-infested plants. This was explained by the induction of JA by mites, which could help the survival of whiteflies on the plants (Lin et al., 2019).
To conclude, our study has shown that sorghum defenses induced by GB infestation have negatively impacted subsequent SCA colonization on sorghum plants. Therefore, the sequence of insects arriving on the plant portrays the establishment of later arriving insects. Most of the studies are focused on assessing the defense response to insects of different guilds, but this study is based on the insects of the similar guilds. Uncovering the differences in plant defense response to aphid feeding may also require an understanding of differences in SCA and GB salivary components. It is highly likely that SCA saliva has a strong ability to suppress the sorghum defenses while GB does not, which may also explain the specialist nature of SCA along with its higher reproductive success. Future studies are needed to identify the potential SCA salivary factors that are involved in regulating sorghum defenses. Additionally, there is a need to further explore the other underlying molecular and biochemical defense mechanisms related to sequential herbivory, which may help us to tease apart the complex intraguild interactions occurring in the field. The information from this study can potentially aid in developing aphid resistant sorghum varieties supporting the fact that GB resistant sorghum varieties may also be used against SCA. Deployment of resistant varieties will also lead to reducing the use of chemicals and offers sustainable sorghum pest management.
The original contributions presented in the study are included in the article/Supplementary material, further inquiries can be directed to the corresponding author.
SG conceived the concept and designed the experiments. JA, EI, IR, and HP conducted the experiments and collected data. JA, HP, and SG analyzed the data. RK, JL, and SG provided critical feedback and shaped the research and analysis. The manuscript was written by HP and revised by JL, RK, and SG. All authors contributed to the article and approved the submitted version.
This work was supported by the National Science Foundation CAREER grant (IOS-1845588) awarded to JL.
We would like to thank John Toy for supplying the seeds for the experiments.
The authors declare that the research was conducted in the absence of any commercial or financial relationships that could be construed as a potential conflict of interest.
All claims expressed in this article are solely those of the authors and do not necessarily represent those of their affiliated organizations, or those of the publisher, the editors and the reviewers. Any product that may be evaluated in this article, or claim that may be made by its manufacturer, is not guaranteed or endorsed by the publisher.
The Supplementary material for this article can be found online at: https://www.frontiersin.org/articles/10.3389/fevo.2023.1105725/full#supplementary-material
Armstrong, J. S., Rooney, W. L., Peterson, G. C., Villenueva, R. T., Brewer, M. J., and and Sekula-Ortiz, D. (2015). Sugarcane aphid (Hemiptera: Aphididae): host range and sorghum resistance including cross-resistance from greenbug sources. J. Econ. Entomol. 108, 576–582. doi: 10.1093/jee/tou065
Akbar, W., Showler, A. T., Reagan, T. E., and White, W. H. (2010). Categorizing sugarcane cultivar resistance to the sugarcane aphid and yellow sugarcane aphid (Hemiptera: Aphididae). J. Econ. Entomol. 103, 1431–1437. doi: 10.1603/EC09336
Balakrishna, D., Singode, A., Bhat, B. V., and Tonapi, V. A. (2020). Sorghum improvement through efficient breeding technologies. Accel. Plant Breeding 1, 411–435. doi: 10.1007/978-3-030-41866-3_16
Basu, S., Varsani, S., and Louis, J. (2018). Altering plant defenses: herbivore-associated molecular patterns and effector arsenal of chewing herbivores. Mol. Plant Microbe Interact. 31, 13–21. doi: 10.1094/MPMI-07-17-0183-FI
Bayoumy, M. H., Perumal, R., and Michaud, J. P. (2016). Comparative life histories of greenbugs and sugarcane aphids (Hemiptera: Aphididae) coinfesting susceptible and resistant sorghums. J. Econ. Entomol. 109, 385–391. doi: 10.1093/jee/tov271
Berger, P. H., Zeyen, R. J., and And Groth, J. V. (1987). Aphid retention of maize dwarf mosaic virus (potyvirus): epidemiological implications. Ann. Appl. Biol. 111, 337–344. doi: 10.1111/J.1744-7348.1987.TB01460.X
Bidart-Bouzat, M. G., and Kliebenstein, D. (2011). An ecological genomic approach challenging the paradigm of differential plant responses to specialist versus generalist insect herbivores. Oecologia 167, 677–689. doi: 10.1007/s00442-011-2015-z
Bouchet, S., Olatoye, M. O., Marla, S. R., Perumal, R., Tesso, T., Yu, J., et al. (2017). Increased power to dissect adaptive traits in global sorghum diversity using a nested association mapping population. Genetics 206, 573–585. doi: 10.1534/GENETICS.116.198499/-/DC1
Bowling, R. D., Brewer, M. J., Kerns, D. L., Gordy, J., Seiter, N., Elliott, N. E., et al. (2016). Sugarcane aphid (Hemiptera: Aphididae): a new pest on sorghum in North America. J. Integr. Pest Manag. 7, 12–13. doi: 10.1093/jipm/pmw011
Bricchi, I., Leitner, M., Foti, M., Mithöfer, A., Boland, W., and Maffei, M. E. (2010). Robotic mechanical wounding (MecWorm) versus herbivore-induced responses: early signaling and volatile emission in lima bean (Phaseolus lunatus L.). Planta 232, 719–729. doi: 10.1007/s00425-010-1203-0
Brunissen, L., Cherqui, A., Pelletier, Y., Vincent, C., and Giordanengo, P. (2009). Host-plant mediated interactions between two aphid species. Entomol. Exp. Appl. 132, 30–38. doi: 10.1111/j.1570-7458.2009.00862.x
Chatterjee, D., Lesko, T., Peiffer, M., Elango, D., Beuzelin, J., Felton, G. W., et al. (2022). Sorghum and maize flavonoids are detrimental to growth and survival of fall armyworm Spodoptera frugiperda. J. Pest. Sci. 1–17. doi: 10.1007/s10340-022-01535-y
Chen, C. Y., and Mao, Y. B. (2020). Research advances in plant–insect molecular interaction. F1000Res 9:198. doi: 10.12688/F1000RESEARCH.21502.1
Cronholm, G. B., Knutson, A. E., Parker, R. D., and Pendleton, B. (2007). Managing insects and mite pests of Texas sorghum. Texas Farmer Collection. Texas A&M AgriLife Extension Service, USA.
de Bobadilla, M. F., Van Wiechen, R., Gort, G., and Poelman, E. H. (2022). Plasticity in induced resistance to sequential attack by multiple herbivores in Brassica nigra. Oecologia 198, 11–20. doi: 10.1007/S00442-021-05043-1
De Vos, M., Van Oosten, V. R., Van Poecke, R. M. P., Van Pelt, J. A., Pozo, M. J., Mueller, M. J., et al. (2005). Signal signature and transcriptome changes of Arabidopsis during pathogen and insect attack. Mol. Plant Microbe Interact. 18, 923–937. doi: 10.1094/MPMI-18-0923
Diezel, C., von Dahl, C. C., Gaquerel, E., and Baldwin, I. T. (2009). Different lepidopteran elicitors account for cross-talk in herbivory-induced phytohormone signaling. Plant Physiol. 150, 1576–1586. doi: 10.1104/pp.109.139550
Erb, M., Meldau, S., and Howe, G. A. (2012). Role of phytohormones in insect-specific plant reactions. Trends Plant Sci. 17, 250–259. doi: 10.1016/j.tplants.2012.01.003
Erb, M., Robert, C. A. M., Hibbard, B. E., and Turlings, T. C. J. (2011). Sequence of arrival determines plant-mediated interactions between herbivores. J. Ecol. 99, 7–15. doi: 10.1111/J.1365-2745.2010.01757.X
Falcone Ferreyra, M. L., Rius, S. P., and Casati, P. (2012). Flavonoids: biosynthesis, biological functions, and biotechnological applications. Front. Plant Sci. 3:222. doi: 10.3389/FPLS.2012.00222
Funnell-Harris, D. L., Sattler, S. E., O’Neill, P. M., Gries, T., Tetreault, H. M., and Clemente, T. E. (2019). Response of sorghum enhanced in monolignol biosynthesis to stalk rot pathogens. Plant Dis. 103, 2277–2287. doi: 10.1094/PDIS-09-18-1622-RE
Goławska, S., and Łukasik, I. (2012). Antifeedant activity of luteolin and genistein against the pea aphid, Acyrthosiphon pisum. J. Pest. Sci. 85, 443–450. doi: 10.1007/s10340-012-0452-z
Grover, S., Agpawa, E., Sarath, G., Sattler, S. E., and Louis, J. (2020). Interplay of phytohormones facilitate sorghum tolerance to aphids. Plant Mol. Biol. 109, 639–650. doi: 10.1007/S11103-020-01083-Y
Grover, S., Cardona, J. B., Zogli, P., Alvarez, S., Naldrett, M. J., Sattler, S. E., et al. (2022a). Reprogramming of sorghum proteome in response to sugarcane aphid infestation. Plant Sci. 320:111289. doi: 10.1016/J.PLANTSCI.2022.111289
Grover, S., Puri, H., Xin, Z., Sattler, S., and Louis, J. (2022b). Dichotomous role of jasmonic acid in modulating sorghum defense against aphids. Mol. Plant Microbe Interact. 35, 755–767. doi: 10.1094/MPMI-01-22-0005-R
Grover, S., Shinde, S., Puri, H., Palmer, N., Sarath, G., Sattler, S. E., et al. (2022c). Dynamic regulation of phenylpropanoid pathway metabolites in modulating sorghum defense against fall armyworm. Front. Plant Sci. 13, 1–14. doi: 10.3389/fpls.2022.1019266
Grover, S., Wojahn, B., Varsani, S., Sattler, S. E., and Louis, J. (2019). Resistance to greenbugs in the sorghum nested association mapping population. Arthropod Plant Interact. 13, 261–269. doi: 10.1007/s11829-019-09679-y
Guo, C., Cui, W., Feng, X., Zhao, J., and Lu, G. (2011). Sorghum insect problems and management. J. Integr. Plant Biol. 53, 178–192. doi: 10.1111/J.1744-7909.2010.01019.X
Harris-Shultz, K., Armstrong, S., and Jacobson, A. (2019). Invasive cereal aphids of North America: biotypes, genetic variation, management, and lessons learned. Trends Entomol. 15, 99–122.
Harris-Shultz, K., Ni, X., Wadl, P., Wang, X., Wang, H., Huang, F., et al. (2017). Microsatellite markers reveal a predominant sugarcane aphid (Homoptera: Aphididae) clone is found on sorghum in seven states and one territory of the USA. Wiley Online Libr. 57, 2064–2072. doi: 10.2135/cropsci2016.12.1010
Hogenhout, S. A., and Bos, J. I. B. (2011). Effector proteins that modulate plant-insect interactions. Curr. Opin. Plant Biol. 14, 422–428. doi: 10.1016/j.pbi.2011.05.003
Huang, W., Robert, C. A. M., Hervé, M. R., Hu, L., Bont, Z., and Erb, M. (2017). A mechanism for sequence specificity in plant-mediated interactions between herbivores. New Phytol. 214, 169–179. doi: 10.1111/NPH.14328
Huang, J., and Shrestha, K. (2022). Revealing differential expression of phytohormones in sorghum in response to aphid attack using the metabolomics approach. Int. J. Mol. Sci. 23:13782. doi: 10.3390/ijms232213782
Jong, T. S., Lu, H., McDowell, J. M., and Greenberg, J. T. (2004). A key role for ALD1 in activation of local and systemic defenses in Arabidopsis. Plant J. 40, 200–212. doi: 10.1111/j.1365-313x.2004.02200.x
Kariyat, R. R., Gaffoor, I., Sattar, S., Dixon, C. W., Frock, N., Moen, J., et al. (2019). Sorghum 3-deoxyanthocyanidin flavonoids confer resistance against corn leaf aphid. J. Chem. Ecol. 45, 502–514. doi: 10.1007/S10886-019-01062-8
Kiani, M., and Szczepaniec, A. (2018). Effects of sugarcane aphid herbivory on transcriptional responses of resistant and susceptible sorghum. BMC Genomics 19, 1–18. doi: 10.1186/S12864-018-5095-X
Kogan, M., and Ortman, E. F. (1978). Antixenosis–a new term proposed to define painter’s “nonpreference” modality of resistance. Bull. Entomol. Soc. Am. 24, 175–176. doi: 10.1093/BESA/24.2.175
Kroes, A., Stam, J. M., David, A., Boland, W., van Loon, J. J. A., Dicke, M., et al. (2016). Plant-mediated interactions between two herbivores differentially affect a subsequently arriving third herbivore in populations of wild cabbage. Plant Biol. 18, 981–991. doi: 10.1111/plb.12490
Li, Q., Xie, Q. G., Smith-Becker, J., Navarre, D. A., and Kaloshian, I. (2006). Mi-1-mediated aphid resistance involves salicylic acid and mitogen-activated protein kinase signaling cascades. Mol. Plant Microbe Interact. 19, 655–664. doi: 10.1094/MPMI-19-0655
Liang, D., Liu, M., Hu, Q., He, M., Qi, X., Xu, Q., et al. (2015). Identification of differentially expressed genes related to aphid resistance in cucumber (Cucumis sativus L.). Sci. Rep. 5, 1–10. doi: 10.1038/srep09645
Lin, D., Xu, Y., Wu, H., Liu, X., Zhang, L., Wang, J., et al. (2019). Plant defense responses induced by two herbivores and consequences for whitefly Bemisia tabaci. Front. Physiol. 10, 1–9. doi: 10.3389/fphys.2019.00346
Livak, K. J., and Schmittgen, T. D. (2001). Analysis of relative gene expression data using real-time quantitative PCR and the 2-ΔΔCT method. Methods 25, 402–408. doi: 10.1006/meth.2001.1262
Ma, R., Reese, J. C., Black, W. C. IV, and Bramel-Cox, P. (1990). Detection of pectinesterase and polygalacturonase from salivary secretions of living greenbugs, Schizaphis graminum (Homoptera: Aphididae). J. Insect Physiol. 36, 507–512. doi: 10.1016/0022-1910(90)90102-L
Makoi, J. H. J. R., Belane, A. K., Chimphango, S. B. M., and Dakora, F. D. (2010). Seed flavonoids and anthocyanins as markers of enhanced plant defence in nodulated cowpea (Vigna unguiculata L. Walp.). F. Crop. Res. 118, 21–27. doi: 10.1016/j.fcr.2010.03.012
Martinez De Ilarduya, O., Xie, Q. G., and Kaloshian, I. (2003). Aphid-induced defense responses in Mi-1-mediated compatible and incompatible tomato interactions. Mol. Plant Microbe Interact. 16, 699–708. doi: 10.1094/MPMI.2003.16.8.699
Mertens, D., Fernández de Bobadilla, M., Rusman, Q., Bloem, J., Douma, J. C., and Poelman, E. H. (2021). Plant defence to sequential attack is adapted to prevalent herbivores. Nat. Plants 7, 1347–1353. doi: 10.1038/s41477-021-00999-7
Michaud, J. P., Zhang, Y., and Bain, C. (2017). Feeding by Melanaphis sacchari (Hemiptera: Aphididae) facilitates use of sorghum by Rhopalosiphum padi (Hemiptera: Aphididae), but reciprocal effects are negative. Environ. Entomol. 46, 268–273. doi: 10.1093/EE/NVW167
Michels, G. J. Jr (1986). Graminaceous North American host plants of the greenbug with notes on biotypes. Southwest. Entomol. 11, 55–66.
Mierziak, J., Kostyn, K., and Kulma, A. (2014). Flavonoids as important molecules of plant interactions with the environment. Molecules 19, 16240–16265. doi: 10.3390/MOLECULES191016240
Mohase, L., and Van Der Westhuizen, A. J. (2002). Salicylic acid is involved in resistance responses in the Russian wheat aphid-wheat interaction. J. Plant Physiol. 159, 585–590. doi: 10.1078/0176-1617-0633
Morkunas, I., Woźniak, A., Formela, M., Mai, V. C., Marczak, Ł., Narożna, D., et al. (2016). Pea aphid infestation induces changes in flavonoids, antioxidative defence, soluble sugars and sugar transporter expression in leaves of pea seedlings. Protoplasma 253, 1063–1079. doi: 10.1007/S00709-015-0865-7
Musser, R. O., Hum-Musser, S. M., Eichenseer, H., Peiffer, M., Ervin, G., Murphy, J. B., et al. (2002). Herbivory: caterpillar saliva beats plant defences. Nature 416, 599–600. doi: 10.1038/416599A
Nalam, V., Louis, J., and Shah, J. (2019). Plant defense against aphids, the pest extraordinaire. Plant Sci. 279, 96–107. doi: 10.1016/j.plantsci.2018.04.027
Padmaja, P. G. (2016). “Insect pest resistance in sorghum” in Biotic stress resistance in millets (UK: Elsevier Academic Press), 105–145.
Porter, D. R., Burd, J. D., Shufran, K. A., Webster, J. A., and Teetes, G. L. (1997). Greenbug (Homoptera: Aphididae) biotypes: selected by resistant cultivars or preadapted opportunists? J. Eeon. Entomol 90, 1055–1065. doi: 10.1093/JEE/90.5.1055
Przybylska-Balcerek, A., Frankowski, J., and Stuper-Szablewska, K. (2019). Bioactive compounds in sorghum. Eur. Food Res. Technol. 245, 1075–1080. doi: 10.1007/S00217-018-3207-0
Rao, S., Santhakumar, A. B., Chinkwo, K. A., Wu, G., Johnson, S. K., and Blanchard, C. L. (2018). Characterization of phenolic compounds and antioxidant activity in sorghum grains. J. Cereal Sci. 84, 103–111. doi: 10.1016/J.JCS.2018.07.013
Robert-Seilaniantz, A., Grant, M., and Jones, J. D. G. (2011). Hormone crosstalk in plant disease and defense: more than just jasmonate-salicylate antagonism. Annu. Rev. Phytopathol. 49, 317–343. doi: 10.1146/ANNUREV-PHYTO-073009-114447
Rodriguez-Saona, C. R., Musser, R. O., Vogel, H., Hum-Musser, S. M., and Thaler, J. S. (2010). Molecular, biochemical, and organismal analyses of tomato plants simultaneously attacked by herbivores from two feeding guilds. J. Chem. Ecol. 36, 1043–1057. doi: 10.1007/s10886-010-9854-7
Royer, T. A., Pendleton, B. B., Elliott, N. C., and Giles, K. L. (2015). Greenbug (Hemiptera: Aphididae) biology, ecology, and management in wheat and sorghum. J. Integr. Pest Manag. 6, 1–10. doi: 10.1093/jipm/pmv018
Sandström, J., Telang, A., and Moran, N. A. (2000). Nutritional enhancement of host plants by aphids—a comparison of three aphid species on grasses. J. Insect Physiol. 46, 33–40. doi: 10.1016/S0022-1910(99)00098-0
Schwartzberg, E., and Tumilison, J. H. (2013). Aphid honeydew alters plant defence responses. Funct. Ecol. 28, 386–394. doi: 10.1111/1365-2435.12182
Scully, E. D., Gries, T., Sarath, G., Palmer, N. A., Baird, L., Serapiglia, M. J., et al. (2016). Overexpression of SbMyb60 impacts phenylpropanoid biosynthesis and alters secondary cell wall composition in Sorghum bicolor. Plant J. 85, 378–395. doi: 10.1111/tpj.13112
Shah, J. (2003). The salicylic acid loop in plant defense. Curr. Opin. Plant Biol. 6, 365–371. doi: 10.1016/S1369-5266(03)00058-X
Shrestha, K., and Huang, Y. (2022). Genome-wide characterization of the sorghum JAZ gene family and their responses to phytohormone treatments and aphid infestation. Sci. Rep. 12:3238. doi: 10.1038/s41598-022-07181-9
Singh, S., Kaur, I., and Kariyat, R. (2021). The multifunctional roles of polyphenols in plant-herbivore interactions. Int. J. Mol. Sci. 22:1442. doi: 10.3390/IJMS22031442
Singh, B. U., Padmaja, P. G., and Seetharama, N. (2004). Biology and management of the sugarcane aphid, Melanaphis sacchari (Zehntner) (Homoptera: Aphididae), in sorghum: a review. Crop Prot. 23, 739–755. doi: 10.1016/j.cropro.2004.01.004
Smith, C., and Clement, S. L. (2012). Molecular bases of plant resistance to arthropods. Annu. Rev. Entomol. 57, 309–328. doi: 10.1146/annurev-ento-120710-100642
Soler, R., Badenes-Pérez, F. R., Broekgaarden, C., Zheng, S. J., David, A., Boland, W., et al. (2012). Plant-mediated facilitation between a leaf-feeding and a phloem-feeding insect in a brassicaceous plant: from insect performance to gene transcription. Funct. Ecol. 26, 156–166. doi: 10.1111/j.1365-2435.2011.01902.x
Stam, J., Kroes, A., Li, Y., Gols, R., and Van Loon, J. (2014). Plant interactions with multiple insect herbivores: from community to genes. Annu. Rev. Plant Biol. 65, 689–713. doi: 10.1146/annurev-arplant-050213-035937
Sun, Y. C., Pan, L. L., Ying, F. Z., Li, P., Wang, X. W., and Liu, S. S. (2017). Jasmonic acid-related resistance in tomato mediates interactions between whitefly and whitefly-transmitted virus. Sci. Rep. 7:566. doi: 10.1038/s41598-017-00692-w
Szczepaniec, A. (2018). Interactive effects of crop variety, insecticide seed treatment, and planting date on population dynamics of sugarcane aphid (Melanaphis sacchari) and their predators in late-colonized sorghum. Crop Prot. 109, 72–79. doi: 10.1016/j.cropro.2018.03.002
Tetreault, H. M., Grover, S., Scully, E. D., Gries, T., Palmer, N. A., Sarath, G., et al. (2019). Global responses of resistant and susceptible sorghum (Sorghum bicolor) to sugarcane aphid (Melanaphis sacchari). Front. Plant Sci. 10:145. doi: 10.3389/FPLS.2019.00145
Todd, G. W., Getahun, A., and Cress, D. C. (1971). Resistance in barley to the greenbug, Schizaphis graminum. 1. Toxicity of phenolic and flavonoid compounds and related substances. Ann. Entomol. Soc. Am. 64, 718–722. doi: 10.1093/AESA/64.3.718
Turlings, T. C. J., McCall, P. J., Alborn, H. T., and Tumlinson, J. H. (1993). An elicitor in caterpillar oral secretions that induces corn seedlings to emit chemical signals attractive to parasitic wasps. J. Chem. Ecol. 19, 411–425. doi: 10.1007/BF00994314
Vanderlip, R. L., and Reeves, H. E. (1972). Growth stages of sorghum [Sorghum bicolor, (L.) Moench.]1. Agron. J. 64, 13–16. doi: 10.2134/AGRONJ1972.00021962006400010005X
Wang, L., and Wu, J. (2013). The essential role of jasmonic acid in plant–herbivore interactions – using the wild tobacco Nicotiana attenuata as a model. J. Genet. Genomics 40, 597–606. doi: 10.1016/J.JGG.2013.10.001
War, A. R., Paulraj, M. G., Ahmad, T., Buhroo, A. A., Hussain, B., Ignacimuthu, S., et al. (2012). Mechanisms of plant defense against insect herbivores. Plant Signal. Behav. 7, 1306–1320. doi: 10.4161/PSB.21663
Xu, J., Wang, X., Zu, H., Zeng, X., Baldwin, I. T., Lou, Y., et al. (2021). Molecular dissection of rice phytohormone signaling involved in resistance to a piercing-sucking herbivore. New Phytol. 230, 1639–1652. doi: 10.1111/NPH.17251
Zapata, S. D., Dudensing, R., Sekula, D., Esparza-DÍaz, G., and Villanueva, R. (2018). Economic impact of the sugarcane aphid outbreak in South Texas. J. Agric. Appl. Econ. 50, 104–128. doi: 10.1017/aae.2017.24
Zarate, S. I., Kempema, L. A., and Walling, L. L. (2007). Silverleaf whitefly induces salicylic acid defenses and suppresses effectual jasmonic acid defenses. Plant Physiol. 143, 866–875. doi: 10.1104/PP.106.090035
Zeier, J. (2013). New insights into the regulation of plant immunity by amino acid metabolic pathways. Plant Cell Environ. 36, 2085–2103. doi: 10.1111/pce.12122
Zhang, P. J., Di Li, W., Huang, F., Zhang, J. M., Xu, F. C., and Lu, Y. B. (2013). Feeding by whiteflies suppresses downstream jasmonic acid signaling by eliciting salicylic acid signaling. J. Chem. Ecol. 39, 612–619. doi: 10.1007/S10886-013-0283-2
Zhang, Y., Fan, J., Fu, Y., Francis, F., and Chen, J. (2019a). Plant-mediated interactions between two cereal aphid species: promotion of aphid performance and attraction of more parasitoids by infestation of wheat with phytotoxic aphid Schizaphis graminum. J. Agric. Food Chem. 67, 2763–2773. doi: 10.1021/acs.jafc.8b06150
Zhang, Y., Fu, Y., Fan, J., Li, Q., Francis, F., and Chen, J. (2019b). Comparative transcriptome and histological analyses of wheat in response to phytotoxic aphid Schizaphis graminum and non-phytotoxic aphid Sitobion avenae feeding. BMC Plant Biol. 19, 547–518. doi: 10.1186/s12870-019-2148-5
Keywords: sequential herbivory, plant resistance, sorghum, greenbug (Schizaphis graminum), sugarcane aphid (Melanaphis sacchari), jasmonic acid, salicylic acid
Citation: Puri H, Ikuze E, Ayala J, Rodriguez I, Kariyat R, Louis J and Grover S (2023) Greenbug feeding-induced resistance to sugarcane aphids in sorghum. Front. Ecol. Evol. 11:1105725. doi: 10.3389/fevo.2023.1105725
Received: 23 November 2022; Accepted: 25 January 2023;
Published: 10 February 2023.
Edited by:
Chad Nihranz, Cornell University, United StatesReviewed by:
Julian Chen, Institute of Plant Protection (CAAS), ChinaCopyright © 2023 Puri, Ikuze, Ayala, Rodriguez, Kariyat, Louis and Grover. This is an open-access article distributed under the terms of the Creative Commons Attribution License (CC BY). The use, distribution or reproduction in other forums is permitted, provided the original author(s) and the copyright owner(s) are credited and that the original publication in this journal is cited, in accordance with accepted academic practice. No use, distribution or reproduction is permitted which does not comply with these terms.
*Correspondence: Sajjan Grover, ✉ c2FqamFuLmdyb3ZlckBodXNrZXJzLnVubC5lZHU=
†ORCID: Heena Puri, https://orcid.org/0000-0002-0696-8974
Rupesh Kariyat, https://orcid.org/0000-0002-6565-6276
Joe Louis, https://orcid.org/0000-0001-7137-8797
Sajjan Grover, https://orcid.org/0000-0003-4391-0584
Disclaimer: All claims expressed in this article are solely those of the authors and do not necessarily represent those of their affiliated organizations, or those of the publisher, the editors and the reviewers. Any product that may be evaluated in this article or claim that may be made by its manufacturer is not guaranteed or endorsed by the publisher.
Research integrity at Frontiers
Learn more about the work of our research integrity team to safeguard the quality of each article we publish.