- 1Department of Animal and Biomedical Sciences, School of Life Science, Lanzhou University, Lanzhou, China
- 2School of Biological and Pharmaceutical Engineering, Lanzhou Jiaotong University, Lanzhou, China
Understanding how animals living in highland adapt to extreme conditions is critical to evolutionary biology. In contrast to birds and mammals, little information was available on the adaptation mechanisms for O2 transport in high-altitude ectothermic vertebrates. Here we report for the first time on hematological parameters, amino acid sequences of α and β chains of hemoglobin (Hb), O2 affinity of purified hemoglobins (Hbs) and their sensitivities to anion allosteric effector (H+, Cl−, ATP) and temperature in the high-altitude (2,292 m) black-spotted frogs (Pelophylax nigromaculatus) from the Qinghai-Tibet plateau (QTP) compared with the low-altitude (135 m) population. Our results showed that high-altitude black-spotted frogs exhibit significantly increased relative lung mass, hematocrit, and hemoglobin concentration, but significantly decreased body mass and erythrocyte volume, which could improve the blood O2 carrying capability. Compared with the low-altitude population, the purified Hbs of high-altitude black-spotted frogs possessed significantly higher intrinsic Hb-O2 affinity, similar low anion allosteric effector sensitivities, Bohr effects and temperature sensitivities. The elevated Hb-O2 affinity of highland frogs could maximize the O2 extraction from the lungs. Molecular dynamics simulations showed that the Gln123Glu substitution on α2 chain in highland frogs could form a hydrogen bond with 127Lys on α2 chain, resulting in the elimination of a hydrogen bond between 127Lys on α2 chain and 141Arg on α1 chain. This could weaken the interaction between two semirigid dimers (α1β1 and α2β2) and then lead to the high intrinsic Hb-O2 affinity in high-altitude black-spotted frogs.
Introduction
High-altitude environment was characterized by hypobaric hypoxia, high ultraviolet radiation and cold. Previous studies indicated that both ambient oxygen partial pressure () and temperature decreased with the increase in elevation (McClelland and Scott, 2019). Thus, a primary concern for high-altitude animals is how to keep life events under these great stresses. Extensive researches have shown that animals have adapted to high altitude in many aspects including gene (mutation, evolution and expression), protein (structural and function), hematological parameters, and organs (lung, eardrum, vomerine tooth among others) (Simonson et al., 2010; Storz et al., 2010; McClelland and Scott, 2019). Adaptive changes to enhance the ability of O2 transport in hypobaric hypoxia for high-altitude animals may occur in hematological parameters and oxygenation properties of hemoglobin.
Hemoglobin, the major component of red blood cells, plays an indispensable role in oxygen transport in most vertebrates. The structure of vertebrate hemoglobin is a heterotetramer composed of two α chains (α1 and α2) and two β chains (β1 and β2). α1 and α2 chains have the same amino acid sequence, and β1 and β2 chains have the same amino acid sequence. They were numbered to facilitate the exploration of spatial structural properties of Hb. Heme is located in a hydrophobic pocket on each chain (α1, α2, β1, β2). During O2 binding, the two semi-rigid dimers (α1β1 and α2β2) glide to each other, resulting in the transition of the spatial conformation of Hb from the tense state (T state, low O2 affinity) to the relaxed state (R state, high O2 affinity). Many ectothermic vertebrates express multiple α- and β-type globin genes, resulting in multiple or heterogeneous Hbs (isoHbs). Hb heterogeneity and ‘polymorphism’ (intraspecific differences in Hbs) provide a basis for a division of labor among component Hbs under different conditions (Weber and Jensen, 1988).
Animals adapted to high altitudes have successfully overcome the harsh environment, providing an important opportunity to look for insight into the molecular and physiological adaptation of hemoglobin. Many studies found that high-altitude natives evolved elevated Hb-O2 affinity (Simonson et al., 2010; Qiu et al., 2012; Qu et al., 2013; Lorenzo et al., 2014). For example, the significantly increased intrinsic Hb-O2 affinity of the bar-headed goose (Anser indicus) is largely caused by the α-119Pro → Ala mutation which weakens the interaction within the semi-rigid dimer (Jessen et al., 1991; Weber et al., 1993; Jendroszek et al., 2018; Natarajan et al., 2018). Weber et al. (2002) found that Andean frog (Telmatobius peruvianus) at 3,800 m exhibited higher O2 affinity than low-altitude population which could be attributed to the acetylation modification of NH2-terminal Val at α-chain and the amino acid substitution of α131Thr → Ala. Pu et al. (2019) found that plateau zokor (Eospalax baileyi) Hbs possessed high intrinsic Hb-O2 affinity compared to mice which might be related to the substitution of Ser by Asn at position 131 on the α2-chain. The adaptive adjustments of hematological traits and increased Hb-O2 affinity were also found in high-altitude Asiatic toads (Bufo gargarizans) which could improve O2 carrying capacity under hypoxia environment (Pu et al., 2021).
The evolved elevated Hb-O2 affinity of high-altitude species could enhance the O2 extraction capacity from the lungs, on the other hand, it could compromise O2 unloading to the tissues in the systemic capillaries. The allosteric effects of organic phosphate, chloride, protons, and CO2 on Hb-O2 affinity could compensate for the side effect, which binds to specific sites and stabilize the low-affinity Hbs (T state). The main organic phosphates were 2,3-diphos-phoglycerate (DPG) for mammals, inositol pentaphosphate (IP5) for birds, and adenosine triphosphate (ATP) for reptiles. Due to the exothermic nature of hemoglobin oxygenation, an increase in temperature could decrease the Hb-O2 affinity and favor O2 unloading to warm exercising tissues and organs. However, this temperature effect would hinder the O2 loading in the cold limbs for animal living in polar or alpine environments. As we know ectotherms lack the ability of temperature regulation, whether the low temperature sensitivities of Hbs founded in arctic or alpine mammals is true in alpine reptiles remains to be investigated (Weber and Campbell, 2011). However, the low temperature sensitivity of Hbs was reported in Asiatic toad (Pu et al., 2021).
Black-spotted frog (Pelophylax nigromaculatus) belonging to the genus Pelophylax in the family of Ranidae has a wide distribution in East Asia (Gong et al., 2013). Due to their widespread, rapid propagation and easily collected, black-spotted frogs have extensive value in academic, edible and medical aspects. Current research on the black-spotted frog mainly focus on toxicology, conservation biology, and immunology (Yu et al., 2020). Few studies were available on the high-altitude adaptation of this specie despite they can expand to the Qinghai-Tibetan Plateau (QTP).
This study aimed to explore whether blood O2 carrying capability of high-altitude black-spotted frog undergoes adaptive adjustments compared with low-altitude population. We compared their hematological traits and oxygenation properties of purified Hbs, sequenced the subunit gene of hemoglobin and analyzed the structure properties of Hb tetramers. We hypothesized that there are obvious changes in hematological traits between high- and low-altitude population. Moreover, Hbs of high-altitude black-spotted frog may exhibit the genetic-based higher Hb-O2 affinity to obtaining adequate O2 under hypoxia environment compared with low-altitude population.
Materials and methods
Animal and samples
High-altitude black-spotted frogs (N = 6) were captured at Guide County, Qinghai Province, China (36°10′87″N, 101°53′43″E, 2292 m). Low-altitude black-spotted frogs (N = 12) were captured at Ningyang County, Shandong Province, China (35°46′8″N, 116°48′12″E, 135 m). High- and low-altitude black-spotted frogs were collected in April and June 2019, respectively. Our experiment received ethics approval from the Ethics Committee of Animal Experiments at Lanzhou University and following guidelines from the China Council on Animal Care. The climate data of sampling site is available in the supplementary attachment (Supplementary Table S1). The climate data of sampling site comes from the national data center of the meteorological discipline in China.1
Ether was used for anesthetizing all frogs. Blood was drawn from the ventricle by heparinized syringe after exposing heart. A portion of fresh blood was used to measure hematological parameters, another portion was washed three times with a threefold excess of 0.67% NaCl. Then the packed washed cells were dispensed (100 μL per tube) and immediately frozen in liquid nitrogen and stored at −80°C for subsequently purifying.
Relative lung mass and hematological parameters
Relative lung mass was calculated as lung weights/body mass in each sample. The hemiglobincyanide (HiCN) method was used for assessing hemoglobin concentration ([Hb]), which measured the absorbance of a mixture of 10 μL fresh blood and 2.5 mL Van Kampen-Zijlstra solution at the wavelength of 540 nm (Moharram et al., 2006). Red blood cell count (RBC) was measured using a hemocytometer with the mixture of 10 μL blood and 1.99 mL RBC diluent under microscope (Motic, China). Hematocrit (Hct) was measured as the proportion of red blood cells in whole blood by centrifuging fresh blood at 3,000 g for 10 min in microhematocrit capillaries. Mean corpuscular hemoglobin concentration (MCHC) was calculated as ([Hb]/Hct)*100, mean corpuscular volume (MCV) was calculated as Hct/RBC, and mean corpuscular hemoglobin (MCH) was calculated as [Hb]/RBC. The long and short diameters (a and b) of erythrocytes fixed on the dried blood smears were determined by the ocular micrometer on a microscope (SOPTOP, China). At least 20 erythrocytes were measured for each sample. Then, erythrocyte volume was calculated using formula of (4/3)πab2.
Hb purification and O2 equilibrium curves
The frozen red blood cells were redissolved with the threefold volume of ice-cold 10 mmol/L Hepes (pH 7.8) and lysed on ice for 20 min. Then the obtained hemolysate was centrifuged at 4°C (9,000 g, 10 min) to remove membranes and cellular debris, and supernatants were injected into an IexCap Q 6FF 5-mL column (Smartlifesciences, China) on the Äkta Pure Chromatography System (GE Healthcare Life Sciences). After equilibrated with 20 mmol/L Tris⋅HCl (pH 8.4, 0.5 mmol/L EDTA), the supernatant was eluted with a linear gradient of 0–400 mmol/L NaCl at a flow rate of 1 mL/min to get the mixture of hemoglobin isoforms (Hbs) and remove residual endogenous phosphates (Rollema and Bauer, 1979; Bonaventura et al., 1999). The absorbance of the eluate was monitored at the wavelength of 280 nm. The collected Hbs were further desalted by dialysis against three changes of 200-fold volume of 10 mmol/L Hepes buffer (pH 7.6, 0.5 mmol/L EDTA) at 4°C. The final purified Hbs were concentrated to the concentration of ~1.3 mmol/L heme by ultrafiltration using Amicon® Ultra-4 Centrifugal Filter Unit fitted with a 10-kDa cut-off filter (Millipore, Ireland) and stored at −80°C for subsequent experiments.
We measured the O2 equilibrium curves of purified Hbs from high- and low-altitude Black-spotted frogs using a home-made modified diffusion chamber based on the method as described by Weber (1981, 1992), Weber et al. (1993), and Damsgaard et al. (2013). The purified Hbs samples were dissolved in 0.2 mol/L Hepes buffers with pH of 7.0 and 7.5 in the absence (stripped) and presence of Cl− (adding to 0.1 mol/L KCl) and/or ATP (100-fold molar excess tetrameric Hbs). Then the absorbances of the prepared assay solution (3–5 μL) were measured at 436 nm to calculate the fractional O2 saturations under corresponding O2 tension (, mmHg) which was stepwise increased by mixing ultrapure N2 and atmospheric air. The fractional saturation of O2 () was calculated by = (A−A0)/(A100−A0), where A0 and A100 were the absorbances of 0 and 100% O2 saturation that was obtained by equilibrating with ultrapure N2 and atmospheric air, respectively. SevenCompact pH/Ion Meter S220 (Mettler Toledo, Switzerland) equipped with an InLab micro pH electrode (Mettler Toledo, Switzerland) was used for measuring pH values of the prepared assay solution. Before each measurement, the electrode was calibrated by standard pH buffers and the temperature was measured using an ATC temperature probe (Mettler Toledo, Switzerland). Each condition was repeated six times at least.
Since the binding of O2 to hemoglobin conforms to the Hill’s sigmoidal equation, 5–10 saturation data (O2 saturation range≈0.1–0.9) were used to fit this equation by nonlinear sigmoidal Hill fitting (r2 > 0.99) on the GraphPad Prism 9 (GraphPad Software, United States) and yielded P50 and n50 (means±SE) for each condition. P50 is the when of hemoglobin is 50%, and n50 is the Hill cooperativity coefficient. The Bohr effect (Riggs, 1988) describes the change in Hb-O2 affinity that results from a change in H+ concentration and is often expressed numerically as ΔlogP50/ΔpH (Bohr factor Φ), where pH refers to that of the Hb solution. The Bohr factors were measured in conditions of 0.1 mol/L Hepes buffers in pH 7.0 and pH 7.5 and equal to the slope of linear plots of logP50 as a function of pH. The enthalpy of oxygenation (ΔH, kJ/mol O2), that is the heat liberated upon oxygenation of Hbs, was calculated by the van’s Hoff equation ΔH = 2.303R(ΔlogP50/Δ(1/T)). T is the absolute temperature in Kelvin and R is the gas constant [8.314*J/(K mol O2)]. The anionic sensitivities were calculated by different in logP50 in the presence of Cl− and/or ATP and stripped condition.
Globin sequencing, Hb homology modeling, and molecular dynamic simulation
Considering close phylogenetic relationship, the gene sequences of α and β globin of the common frog [Rana temporaria, LOCUS: α (XM_040357009.1), β (NC_053494.1)] were used for designing primers to amplified the coding sequence of α and β goblins of high- and low-altitude black-spotted frogs. For each population, samples (N = 5) were collected from liver and used for total DNA extraction (Rapid Animal Genomic DNA Isolation kit, Sangon Biotech, China) and RNA extraction [Steadypure Quick RNA Extraction Kit, Accurate Biotechnology (Hunan) Co., Ltd., Changsha, China], followed by cDNA reverse transcription [Evo M-MLV Kit, Accurate Biotechnology (Hunan) Co., Ltd., Changsha, China]. Using PCR protocol (Taq polymerase, Fermentas, Burlington, ON, Canada) with one pair primer, we had successfully amplified the sequence of mRNA α and β, but the mRNA sequence of β gene was not complete. Since it is difficult to design suitable primer, we amplified β gene of black spotted frog from total DNA with two pair segmented primers (Table 1) and then β gene sequence assembly and translation was performed with software (DNAMAN 6.0.3.40, United States). PCR protocol: 1 cycle of 5 min at 94°C, 35 cycles of 30 s at 94°C, 30 s at annealing temperature, and 60 s at 72°C, and one final cycle of 10 min at 72°C. The obtained sequence of β gene identical matches both the partial CDS sequence that we obtained from cDNA and the mRNA sequence identified in the published liver transcriptome data of black spotted frogs (GSE132282_unigene: TRINITY_DN76909_c0_g1). However, another type of β gene identified in the common frog cannot be cloned from mRNA (cDNA) in the liver of black-spotted frogs, and it was also not identified in the liver transcriptome data of black-spotted frogs. So, we had successfully amplified the CDS sequence of one type of α and β gene, respectively, and speculated that there might be only one type of α and β gene expressed in the black spotted frog.
The sequencing data have been uploaded to GenBank (high-altitude α: OQ079690; high-altitude β: OQ079692; low-altitude α: OQ079691; low-altitude β: OQ079693). We obtained the total length of these genes. Since the methionine (Met), encoded by the start codon, is not involved in the formation of the final polypeptide, the amino acid sequences of α and β chains are numbered from the first amino acid after Met. Then, SWISS-MODLE online software2 was used to construct the tetramer structures of deoxy Hb for high- and low-altitude frogs using the Side-necked turtle Hb structure (PDB ID: 3at5) in the T state as the template. The amino acid sequence of the template was 55.24% similar to the Hb of both high- and low-altitude black-spotted frogs. Molecular dynamics simulations (MD) were further performed to explore the quaternary structural properties of deoxy Hb under physiological conditions using the NAMD and VMD software (Humphrey et al., 1996; Phillips et al., 2005). The psfgen plugins of VMD were used for adding missing hydrogen atoms to homology models, then, which were immersed in equilibrated TIP3P water boxes with physiological salt concentrations (0.12 mol/L NaCl). NAMD was performed for energy minimization and molecular dynamics simulations using CHARMM version c35b2 with the all-atom 27 protein force field. And there were some constraint conditions in each simulation: p = 1 atm, t = 310 K and PME (Particle-Mesh-Ewald) method for calculating the electrostatic interactions. After 50 ns MD simulation, when the root mean square deviation (RMSD) of the structure showed good convergence, we analyzed the structural features of tetrameric Hb using VMD and its plugin.
Statistical analyses
Data were tested for normality and homogeneity of the variance using SPSS v16.0 (SPSS Corp., IL, United States). The significance of each result between high- and low-altitude frogs was analyzed by ANOVA. Data were presented as means ± standard error (SE) and statistical significance was accepted at p < 0.05.
Results
Relative lung mass and hematological parameters
The results of relative lung mass, body mass and hematological parameters of high-altitude and low-altitude black-spotted frogs are presented in Table 2. High-altitude black-spotted frogs exhibited significantly higher values in ML (relative lung mass), Hct, [Hb], MCH, and MCHC (p < 0.05), but significantly lower value in erythrocyte volume (p < 0.05) and body mass (p < 0.001) compared with low-altitude population.
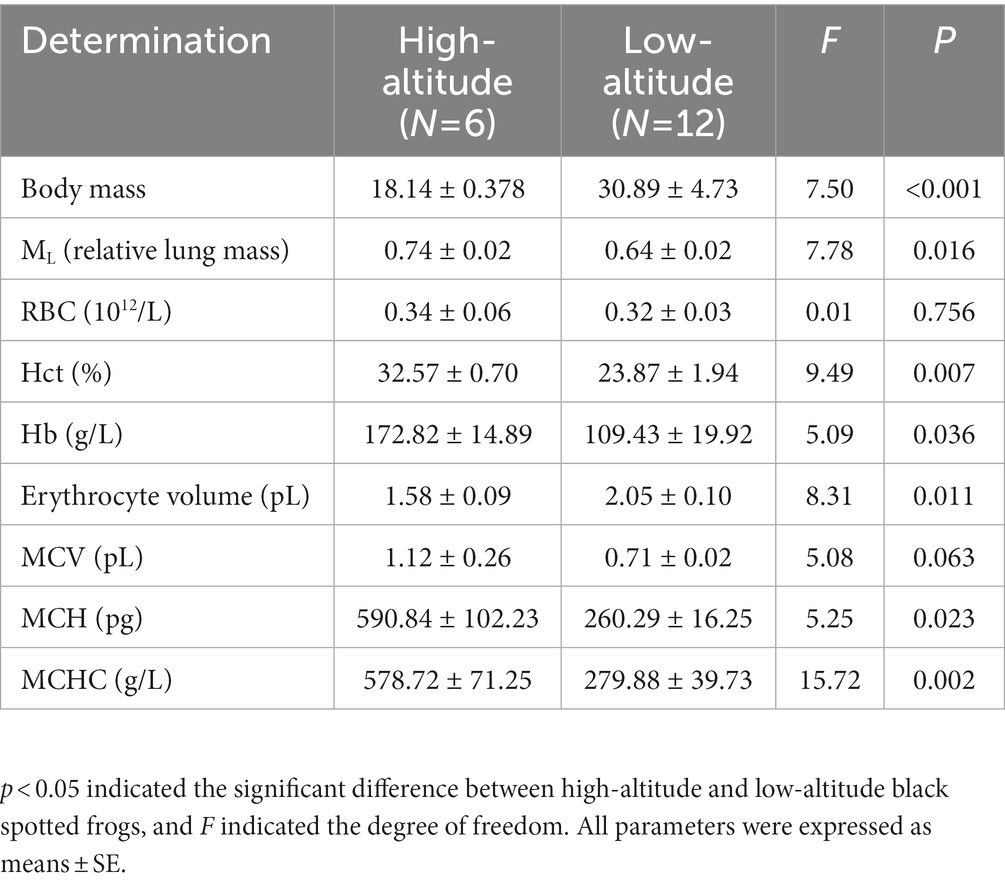
Table 2. Hematological parameters and relative lung mass of high- and low-altitude black spotted frogs.
Oxygenation properties of frog Hbs
Purified Hbs of high-altitude black-spotted frogs exhibited significantly higher overall and intrinsic O2 affinity compared with the low-altitude population as the P50 values of the high-altitude frog exhibited significantly lower values than the low-altitude population under every same experimental condition (Table 3). The n50 values of Hbs in both high- and low-altitude populations were higher than 2.49 in different experimental conditions, which means that O2 binding was positively cooperative (Table 3).
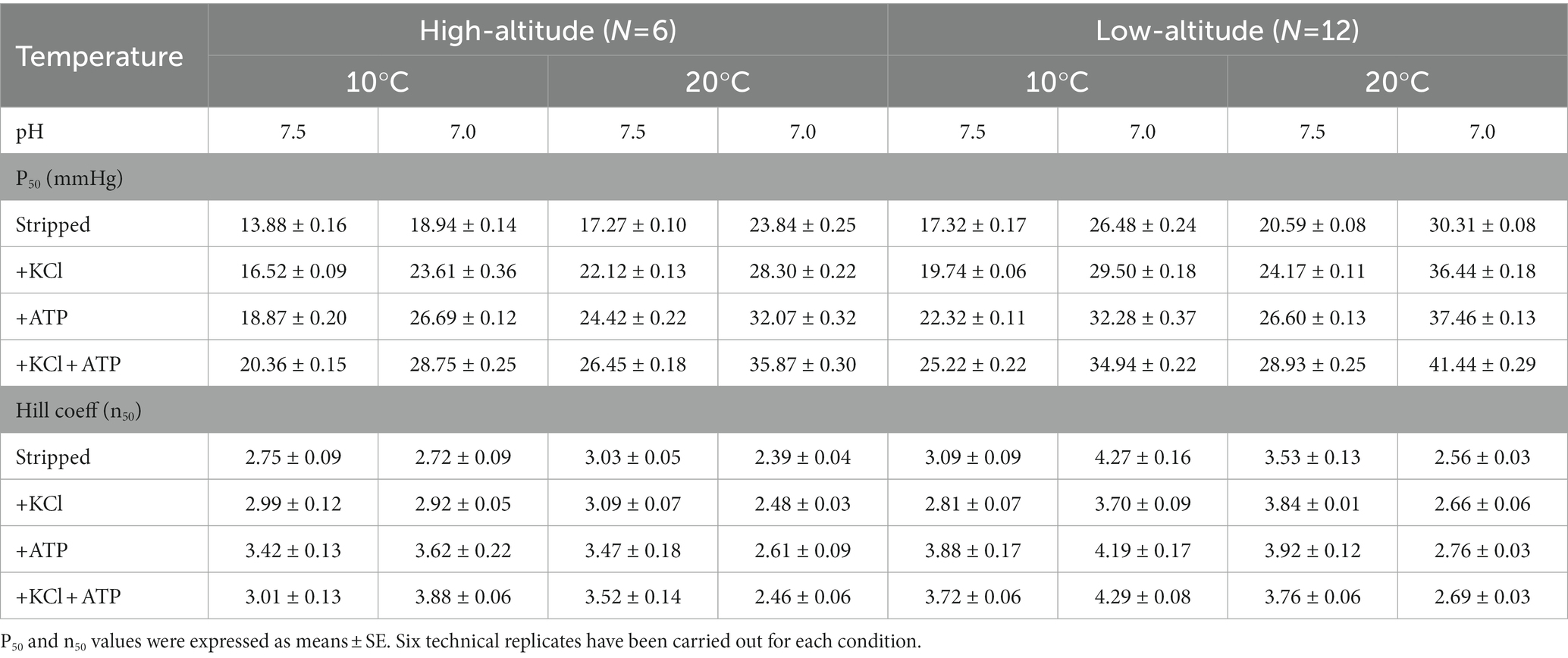
Table 3. Oxygen affinity (P50) and Hill cooperativity coefficient (n50) for high- and low-altitude black spotted frogs Hbs measured in different experimental conditions.
Sensitivities of ATP and/or Cl− of purified Hbs were indexed by the difference between the logP50 in the absence (stripped) and the presence of ATP and/or Cl− in both high- and low-altitude black-spotted frogs (Table 4). Compared with the stripped state, O2 equilibrium curves shifted to right and the Hb-O2 affinities were significantly reduced in the presence of Cl− and/or ATP for both high- and low-altitude populations. Furthermore, the effect of ATP on Hb-O2 affinities was greater than Cl− in both high- and low-altitude frogs, and the reduced impact reached the maximum when ATP and Cl− exist simultaneously. Sensitivities of ATP and/or Cl− of purified Hbs in high-altitude population were slightly greater than low-altitude populations with one exception (at 20°C, pH 7.0, Table 4).
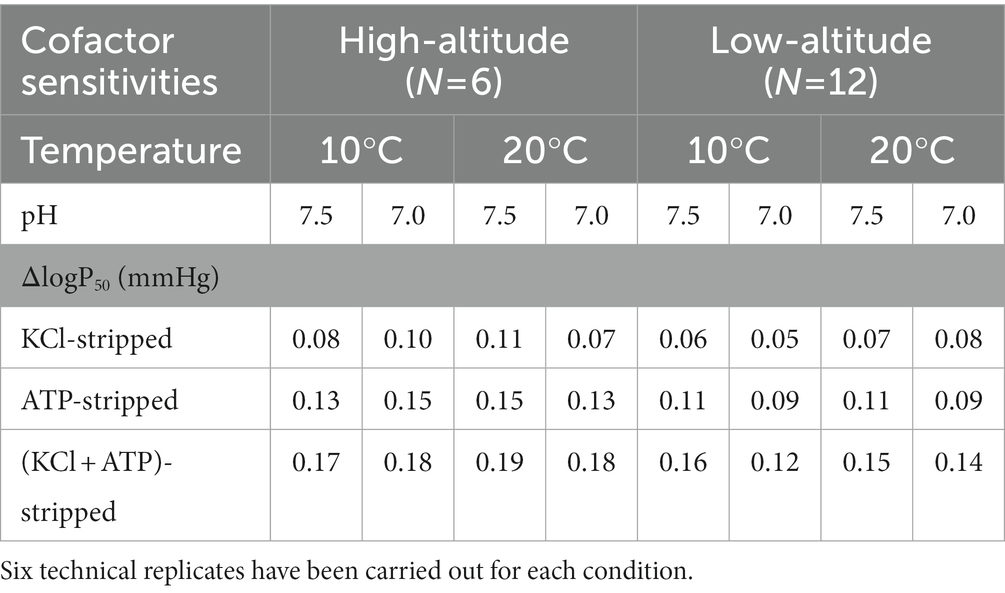
Table 4. Anionic allosteric cofactor sensitivities of high- and low-altitude black spotted frogs Hbs under different experimental conditions.
Hb-O2 affinity was increased and the O2 equilibrium curves move to right in both high- and low-altitude frogs when the pH value increased from 7.0 to 7.5 as the P50 values significantly decreased (Figure 1A; Table 3). Bohr factor (Φ = ΔlogP50/ΔpH) is equal to the slope of linear curve of logP50 versus pH in the range of pH 7.0–7.5 (Figures 1C,D; Table 5). Under all conditions, high- and low-altitude black-spotted frog Hbs exhibited roughly similar Bohr factors. Furthermore, when the temperature raised from 10°C to 20°C, the O2 equilibrium curves move to right and Hb-O2 affinity was reduced in both high- and low-altitude frogs (Figure 1B). Compared with low-altitude black-spotted frog Hbs, the high-altitude black-spotted frog Hbs exhibited little difference in temperature sensitivity (enthalpy of oxygenation, ΔH) under the same conditions (Table 5).
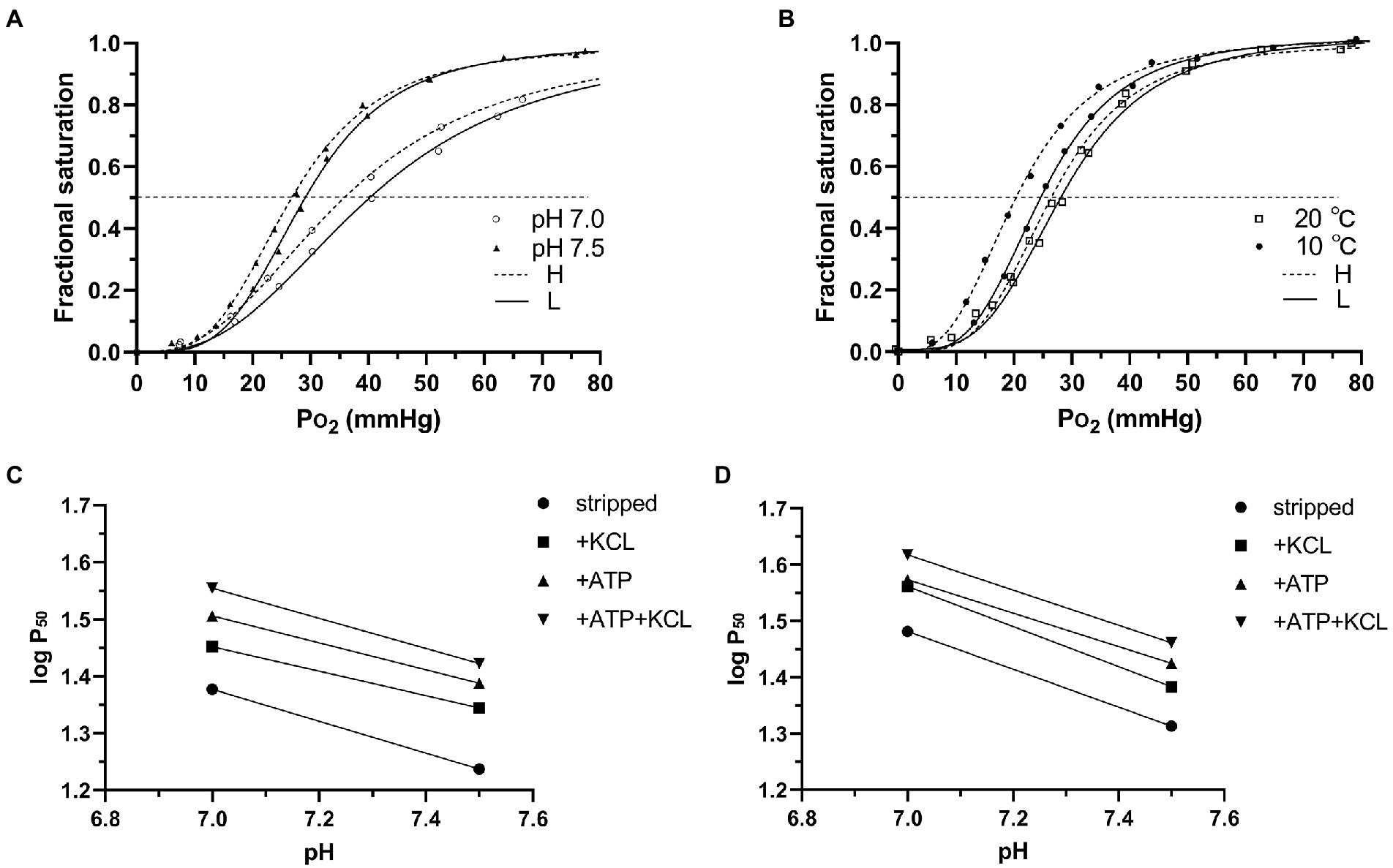
Figure 1. Representative O2 equilibrium curves of high- and low-altitude black spotted frogs Hbs in pH7.0 and pH7.5 at 20°C (A), and at 10°C and 20°C in pH 7.0 (B) in the presence of Cl− and ATP. Bohr plots [logP50 vs. pH] of high-altitude (C) and low-altitude (D) black-spotted frogs Hbs in the absence (stripped) or/and presence of Cl− and ATP at 20°C. The slope of each of these linear plots is Bohr factor (Φ) reported in Table 4. The sample sizes of high- and low-altitude black spotted frogs are 6 and 12, respectively. Each experiment has been carried out six times.
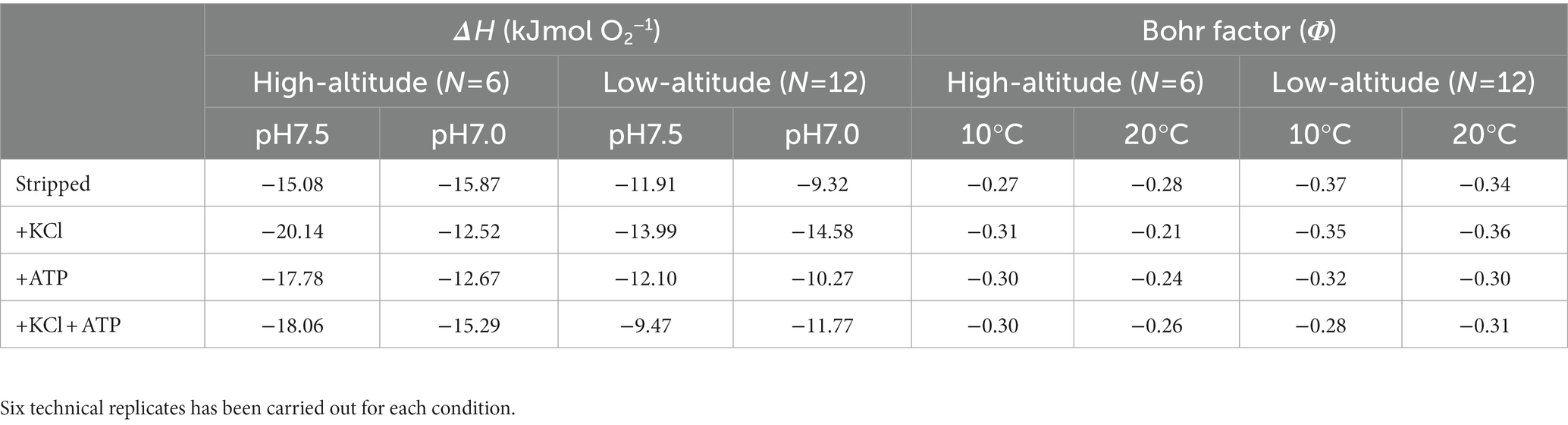
Table 5. Temperature effect (indexed as enthalpy of oxygenation (ΔH)) and Bohr effect (indexed as Bohr factor) on oxygen affinity of high- and low-altitude black spotted frogs Hbs under different experimental conditions.
Amino acid sequences alignment and molecular dynamic simulation
We had successfully obtained one type of CDS sequence of α and β gene. After translation, the amino acid sequences of α and β chains were presented in Figure 2A. There are two amino acid substitutions of Hb on α chain (Met63Val and Gln123Glu) and one amino acid substitution on β chain (Gln76His) between high- and low-altitude black-spotted frogs (Figure 2A). The backbone RMSD ≤0.1 Å between the template and the constructed Hb model was considered usable. After evaluating the accuracies of constructed Hb models for high- and low-altitude black-spotted frogs, we performed the molecular dynamic simulation to predict the stable conformations of the Hb models. Our results of the molecular dynamic simulation showed that the RMSD of the backbone atom exhibited excellent convergence (about 3.0–4.0 Å) after simulating 30 ns (Supplementary Figure S1). Then we analyzed the interaction between semirigid dimers in the final 20 ns of the trajectories. The mutation was changed from amide group (-CONH2) to carboxyl (-COOH) at the site of 123 on α2 chain and the properties of amino acid were changed from uncharged polar to negatively charged. It causes an extra hydrogen bond formation between α2-123-Glu (oxygen atom of side-chain carboxyl group) and α2-127-Lys (hydrogen atom of side-chain amino group) in high-altitude black-spotted frogs Hb (Figure 2B). This hydrogen bond causes the change of the spatial orientation of α2-127-Lys side chain, increase the distance between α2-127-Lys and α1-141-Arg and decrease the likelihood of hydrogen bond formation. Thus, it found that an additional hydrogen bond between α1-141-Arg (oxygen atom of carboxyl-terminus) and α2-127-Lys (hydrogen atom of side-chain amino group) presented in low-altitude black-spotted frogs Hb (Figure 2C), but did not exist in high-altitude black-spotted frogs Hb (Figure 2B). In addition, the substitutions of Met63Val on α chain and Gln76His on β chain in the high-altitude frogs do not affect hydrogen bond interaction between dimers based on our analysis of MD results.
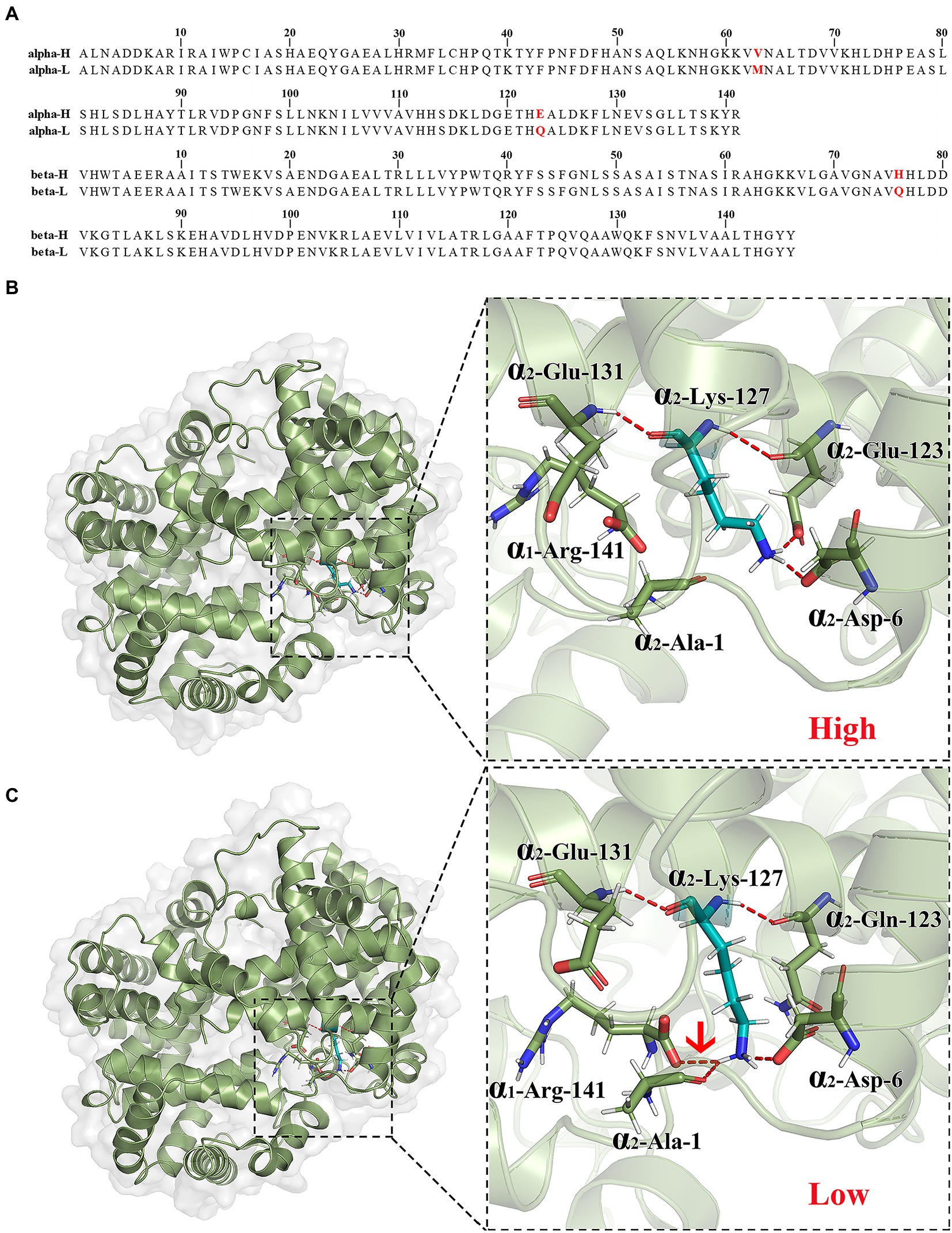
Figure 2. Amino acid substitution of Gln123Glu on α2 chain affects the spatial structure of Hb of black-spotted frog. Amino acid sequence alignments of α- and β-chains in hemoglobin of high- and low-altitude black spotted frog (A). The constructed spatial structure of Hb in high-altitude black-spotted frog (B). The constructed spatial structure of Hb in low-altitude black-spotted frog (C). Substitution of Gln to Glu at position 123 on α2 chain of high-altitude frog could form an additional hydrogen bond with 127Lys on the same chain, resulting in the change of Hb conformation and the loss of hydrogen bond interaction between α1 and α2 chain compared with the low-altitude frog. Red dashed lines indicated the hydrogen bonds formed between 127Lys on α2 chain and 141Arg on α1 chain in the Hb of low-altitude frog. Each experiment has been carried out three times.
Discussion
The adaptive adjustments in the O2 transport system of high-altitude natives occurred in several steps including O2 utilization in mitochondria, intrinsic Hb-O2 affinity, cardiorespiratory system and O2 diffusion and circulation in tissue (McClelland and Scott, 2019). Our research investigated the adaptation mechanisms in the O2 transport system of high-altitude black-spotted frogs dwelling on the QTP from two aspects: phenotypic plasticity and genetic change.
In terms of phenotypic plasticity, we found that ML (relative lung mass), Hct, [Hb], MCH and MCHC increased, but erythrocyte volume and body mass decreased in high-altitude black-spotted frogs (Table 2). The higher [Hb] of high-altitude black-spotted frogs could improve the O2 carrying capacity of the blood. Similar phenomenon was also found in high-altitude Asiatic toads (Bufo gargarizans) and lake titicaca frogs (Telmatobius culeus) (Hutchison et al., 1976; Pu et al., 2021). The Hct of high-altitude black-spotted frog was higher than low-altitude black-spotted frog. The moderate increase in the Hct could allow the blood to transport more O2 to aerobically tissues (Guyton and Richardson, 1961). Similar high Hct were also found in Bufo spinulosus limensis (39.53 ± 8.92%), high-altitude Asiatic toads (41.87 ± 0.92%), male Bufo bufo (42.4 ± 1.2%) (Ostojic et al., 2000; Donmez et al., 2009; Pu et al., 2021). On the other hand, high-altitude black-spotted frogs had smaller erythrocyte volume, which was presumed to increase the relative surface area of RBC and minimize the adverse effects of increased blood viscosity (Dunlap, 2006). There was a contradiction between MCV and erythrocyte volume in our results. This may be attributed to the low plasma volume of high-altitude frogs. Lower plasma could lead to the higher Hct, consequently, lead to the higher MCV of the high-altitude frogs although its measured erythrocyte volume was lower than the low-altitude frogs. The speculated low plasma volume of high-altitude frogs may be related to the lower humidity at Guide compared to Ningyang (Supplementary Table S1). However, more experiments about the plasma volume is needed to prove these deductions. Body mass is inversely proportional to surface-area-to-volume ratio, which constrains transport rates for water and respiratory gases across the skin. The smaller frogs have larger contact area with the air and are expected to obtain a higher proportion of oxygen through the skin. Hence, the lower body mass of the high-altitude black-spotted frog may be a strategy for high-altitude adaptation (Toledo and Jared, 1993).
Furthermore, our results showed that there was no significant difference in RBC between high and low-altitude black-spotted frogs. This was inconsistent with previous studies. High-altitude Asiatic toads, Lizards (Phrynocephalus erythrurus), and domesticated yaks (Bos grunniens) possess higher RBC than low-altitude populations (Ding et al., 2014; Lu et al., 2015; Pu et al., 2021). So, we caution against the over interpretation of this finding possibly due to our small sample size.
The O2 equilibrium results revealed that the P50 value of purified Hbs of high-altitude black-spotted frogs was significantly lower than that of low-altitude black-spotted frogs under the same conditions, indicating that high-altitude black-spotted frogs Hbs have significantly elevated O2 affinity (Table 3). The increase in O2 affinity of high-altitude natives is generally thought to be beneficial for safeguarding the tissue O2 supply under the reduced O2 availability at high-altitude or other conditions. Similar results were also found in other species, such as bar-headed goose (Anser indicus), Asiatic toad (Bufo gargarizans), Andean frog (Telmatobius peruvianus), plateau zoker (Eospalax baileyi) (Weber et al., 2002; Jendroszek et al., 2018; Pu et al., 2019, 2021).
The O2 affinity of Hbs is influenced by temperature and several allosteric effectors (H+, Cl−, ATP, and/or DPG). In our study, high-altitude black-spotted frogs exhibit slightly higher anionic cofactor sensitivities than low-altitude population under the same experimental conditions except for Cl− sensitivities at 20°C, pH 7.0 (Table 4). However, Cl− and/or ATP sensitivities (ΔlogP50 = 0.06–0.19) of black-spotted frogs were dramatically low compared with other species, such as Asiatic toad, T. peruvianus and X. laevis (Weber et al., 2002, 2017; Pu et al., 2021). The Bohr effect, quantified by the Bohr factor (Φ), could facilitate O2 unloading to the metabolizing tissue, such as exercise muscle. However, there were hardly noticeable difference in Bohr factor between the high- and low-altitude black-spotted frogs Hbs under approximately physiological conditions (both ATP and Cl− added) (Table 5). This indicated that the hinder effect of the high Hb-O2 affinity of Hbs in high-altitude black-spotted frog was not compensated by the elevated Bohr effect, which was found in other amphibians, such as Asiatic toad and bufo spinulosus flavolineatus (Ostojic et al., 2000; Pu et al., 2021). Moreover, there is very little difference in the enthalpy of oxygenation (ΔH) between high- and low-altitude black-spotted frogs Hb, and the ΔH was largely unaffected by the presence of allosteric effectors (ATP, Cl−, and H+) (Table 5). ΔH values of the high- and low-altitude black-spotted frogs were lower than that of Asiatic toad which was deemed as the low temperature sensitivity (Pu et al., 2019) after exclude the heat of solution of O2. Although significant temperature effects of Hbs favor the unloading of O2 in warm exercise muscles, it also impairs O2 delivery to the cold limbs of mammals living in cold environments. Hb with reduced temperature sensitivity can compensate for this adverse effect and may have adaptive significance for animals living in polar or alpine environments. As an ectotherm, the body temperature of black-spotted frogs is determined by ambient temperature. Thus, the low temperature sensitivity of black-spotted frogs could reduce the impact of temperature on Hb-O2 affinity during the process of O2 delivery (Weber and Campbell, 2011).
Considering the similar allosteric effectors (ATP, Cl−, and H+) and low temperature sensitivities and Bohr effects of the high- and low-altitude black-spotted frogs Hbs, we speculate that the higher O2 affinity of high-altitude black-spotted frogs should be attributed to its increased intrinsic Hb-O2 affinity. It has been demonstrated in numbers of high-altitude natives that elevated intrinsic Hb-O2 affinity was attributed to the substitutions in amino acid sequence α and/or β globin, as these substitutions could change the spatial structure of tetramer Hb (Storz and Moriyama, 2008; Storz et al., 2009; Jendroszek et al., 2018; Signore and Storz, 2020). Only one kind of α-type and one kind of β-type globins were successfully sequenced in this study, respectively. After sequence alignment, there were found two amino acid substitutions of Hb on α chain (Met63Val and Gln123Glu) and one amino acid substitution on β chain (Gln76His) between high- and low-altitude black-spotted frogs (Figure 2A). The predicted Hb structure of high-altitude black-spotted frogs consists of four chains (α1, α2, β1, and β2). α1 and α2 are the same peptide in the Hb. β1 and β2 are the same peptide in the Hb. ‘alpha-H’ and ‘alpha-L’ are the amino acid sequence of α chain (α1 and α2) in hemoglobin of high- and low-altitude black-spotted frogs, respectively. ‘beta-H’ and ‘beta-L’ are the amino acid sequence of β chain (β1 and β2) in hemoglobin of high- and low-altitude black-spotted frogs, respectively. Our molecular dynamic simulation results showed that hydrogen bond interaction was changed between Gln123Glu substation with spatially adjacent resides on the α2 chain (Figures 2B,C). At position 123 on α2 chain of Hb structure model of high-altitude black-spotted frogs, polar uncharged Gln was replaced by negatively charged Glu, which lead to the interaction of hydrogen bonds between 123Glu with positively charged 127Lys on the same α2 chain compared to Hb of low-altitude frogs (Figures 2B,C). This affects the orientation of side chains of 127Lys and increases the distance between the 127Lys on α2 chain and the 141Arg on α1 chain, resulting in the loss of the hydrogen bond between them (Figures 2B,C). Then, the steric hindrance could be reduced in transition from the T state to the R state when O2 binding with Hb in the high-altitude black-spotted frogs. Therefore, the reduced interaction between α1β1 and α2β2 semirigid dimer interface of the T-state Hb could be the structural mechanism of the high intrinsic Hb-O2 affinity of high-altitude black-spotted frogs Hbs. Similar structure mechanisms had been found in other high-altitude animals. The higher intrinsic Hb-O2 affinity of plateau zokor might be attributed to the substitution of the 131 Ser → Asn on α2 chain which could weaken the interaction between α1 and β2 subunits (Pu et al., 2019). The significantly high intrinsic Hb-O2 affinity of the bar-headed goose (Anser indicus) compared with gray goose has been proved due to the 119 Pro→Ala mutation on α chain which leads to the elimination of interaction between α and β chains inside the semi-rigid dimer (Jessen et al., 1991; Weber et al., 1993; Jendroszek et al., 2018; Natarajan et al., 2018).
Through our preliminary results, we can conclude that high-altitude black-spotted frogs have an efficient and effective oxygen transport system. The significantly increased ML (relative lung mass), Hct, [Hb], MCH, MCHC and Hbs-O2 affinity in high-altitude black-spotted frogs could elevate the capability of O2 extraction, carrying and transporting. Moreover, the significantly decreased erythrocyte volume could reduce the blood viscosity to some extent and decreased body mass could help obtain more O2 in high-altitude black-spotted frogs. The significantly high O2 affinity of purified Hbs in high-altitude black-spotted frogs could help the pulmonary O2 uploading under hypoxia. The corresponding structural mechanisms could be the weakening of the interaction between two semirigid dimers due to the Gln123Glu amino acid substitution on the α2 chain. The low temperature sensitivities of high- and low-altitude black-spotted frogs for interspecies comparison could provide a more stable O2 carrying and transporting system by reducing the effect of temperature on Hb-O2 affinity. Our results provide fundamental insight into the adaptation mechanism of black-spotted frogs to high-altitude environments, and it is a valuable reference for adaptive study in other high-altitude ectothermic amphibians.
Data availability statement
The original contributions presented in the study are included in the article/Supplementary material, further inquiries can be directed to the corresponding author.
Ethics statement
The animal study was reviewed and approved by the Ethics Committee of Animal Experiments at Lanzhou University.
Author contributions
QC, PP, and MM: conceptualization. QC, PP, MM, and ZN: methodology. MM: formal analysis and writing—original draft. QC, PP, ZN, TZ, XT, and JW: investigation and supervision. All authors contributed to the article and approved the submitted version.
Funding
The authors were supported by funding from the National Natural Science Foundation of China (No. 31971416 to QC) and the Fundamental Research Funds for the Central Universities (lzujbky-2021-it23 to ZN and lzujbky-2022-55 to XT).
Acknowledgments
We thank Jinzhou Wang and Wangjie Cao for their assistance in the acquisition of experimental animals. We thank Fei Meng and Lu Xi for their helps in laboratory experiments. We thank the Supercomputing Center of Lanzhou University for supporting MD calculations and the Core Facility of the School of Life Science, Lanzhou University for technical assistance.
Conflict of interest
The authors declare that the research was conducted in the absence of any commercial or financial relationships that could be construed as a potential conflict of interest.
Publisher’s note
All claims expressed in this article are solely those of the authors and do not necessarily represent those of their affiliated organizations, or those of the publisher, the editors and the reviewers. Any product that may be evaluated in this article, or claim that may be made by its manufacturer, is not guaranteed or endorsed by the publisher.
Supplementary material
The Supplementary material for this article can be found online at: https://www.frontiersin.org/articles/10.3389/fevo.2023.1103406/full#supplementary-material
Abbreviations
QTP, Qinghai Tibetan Plateau; Hbs, Hemoglobins; Hb, Hemoglobin; ATP, Adenosine triphosphate; DPG, 2,3-diphos-phoglycerate; IP5, Inositol pentaphosphate; [Hb], Hemoglobin concentration; RBC, Red blood cell count; Hct, Hematocrit; MCHC, Mean corpuscular hemoglobin concentration; MCV, Mean corpuscular volume; MCH, Mean corpuscular hemoglobin; RMSD, Root mean square deviation; MD, Molecular dynamics simulations.
Footnotes
References
Bonaventura, C., Ferruzzi, G., Tesh, S., and Stevens, R. D. (1999). Effects of S-nitrosation on oxygen binding by normal and sickle cell hemoglobin. J. Biol. Chem. 274, 24742–24748. doi: 10.1074/jbc.274.35.24742
Damsgaard, C., Storz, J. F., Hoffmann, F. G., and Fago, A. (2013). Hemoglobin isoform differentiation and allosteric regulation of oxygen binding in the turtle, Trachemys scripta. Am. J. Phys. Regul. Integr. Comp. Phys. 305, R961–R967. doi: 10.1152/ajpregu.00284.2013
Ding, X. Z., Liang, C. N., Guo, X., Wu, X. Y., Wang, H. B., Johnson, K. A., et al. (2014). Physiological insight into the high-altitude adaptations in domesticated yaks (Bos grunniens) along the Qinghai-Tibetan plateau altitudinal gradient. Livest. Sci. 162, 233–239. doi: 10.1016/j.livsci.2014.01.012
Donmez, F., Tosunoglu, M., and Gul, C. (2009). Hematological values in hermaphrodite, Bufo bufo (Linnaeus, 1758). North-Western J. Zool. 5, 97–103.
Dunlap, K. D. (2006). Ontogeny and scaling of hematocrit and blood viscosity in Western fence lizards, Sceloporus occidentalis. Copeia 3, 535–538. doi: 10.1643/0045-8511(2006)2006[535:OASOHA]2.0.CO;2
Gong, J., Sun, Q. P., Xue, F., Fang, S. G., and Wan, Q. H. (2013). Molecular characterization of the major histocompatibility complex class Ia gene in the black-spotted frog, Pelophylax nigromaculata. Biochem. Genet. 51, 876–888. doi: 10.1007/s10528-013-9614-9
Guyton, A. C., and Richardson, T. Q. (1961). Effect of hematocrit on venous return. Circ. Res. 9, 157–164. doi: 10.1161/01.res.9.1.157
Humphrey, W., Dalke, A., and Schulten, K. (1996). VMD: visual molecular dynamics. J. Mol. Graph. 14, 33–38. doi: 10.1016/0263-7855(96)00018-5
Hutchison, V. H., Haines, H. B., and Engbretson, G. (1976). Aquatic life at high altitude: respiratory adaptations in the Lake Titicaca frog, Telmatobius culeus. Respir. Physiol. 27, 115–129. doi: 10.1016/0034-5687(76)90022-0
Jendroszek, A., Malte, H., Overgaard, C. B., Beedholm, K., Natarajan, C., Weber, R. E., et al. (2018). Allosteric mechanisms underlying the adaptive increase in hemoglobin-oxygen affinity of the bar-headed goose. J. Exp. Biol. 221:jeb185470. doi: 10.1242/jeb.185470
Jessen, T. H., Weber, R. E., Fermi, G., Tame, J., and Braunitzer, G. (1991). Adaptation of bird hemoglobins to high-altitudes - demonstration of molecular mechanism by protein engineering. Proc. Natl. Acad. Sci. U. S. A. 88, 6519–6522. doi: 10.1073/pnas.88.15.6519
Lorenzo, F. R., Huff, C., Myllymaki, M., Olenchock, B., Swierczek, S., Tashi, T., et al. (2014). A genetic mechanism for Tibetan high-altitude adaptation. Nat. Genet. 46, 951–956. doi: 10.1038/ng.3067
Lu, S. S., Xin, Y., Tang, X. L., Yue, F., Wang, H. H., Bai, Y. C., et al. (2015). Differences in hematological traits between high- and low-altitude lizards (genus Phrynocephalus). PLoS One 10:e0125751. doi: 10.1371/journal.pone.0125751
McClelland, G. B., and Scott, G. R. (2019). Evolved mechanisms of aerobic performance and hypoxia resistance in high-altitude natives. Annu. Rev. Physiol. 81, 561–583. doi: 10.1146/annurev-physiol-021317-121527
Moharram, N. M. M., El Aouad, R., Al Busaidy, S., Fabricius, A., Heller, S., Wood, W. G., et al. (2006). International collaborative assessment study of the AHD(575) method for the measurement of blood haemoglobin. East Mediterr. Health J. 12, 722–734.
Natarajan, C., Jendroszek, A., Kumar, A., Weber, R. E., Tame, J. R. H., Fago, A., et al. (2018). Molecular basis of hemoglobin adaptation in the high-flying bar-headed goose. PLoS Genet. 14:e1007331. doi: 10.1371/journal.pgen.1007331
Ostojic, H., Monge-C, C., and Cifuentes, V. (2000). Hemoglobin affinity for oxygen in three subspecies of toads (Bufo sp.) living at different altitudes. Biol. Res. 33, 5–10. doi: 10.4067/S0716-97602000000100007
Phillips, J. C., Braun, R., Wang, W., Gumbart, J., Tajkhorshid, E., Villa, E., et al. (2005). Scalable molecular dynamics with NAMD. J. Comput. Chem. 26, 1781–1802. doi: 10.1002/jcc.20289
Pu, P., Lu, S. S., Niu, Z. Y., Zhang, T., Zhao, Y. F., Yang, X. W., et al. (2019). Oxygenation properties and underlying molecular mechanisms of hemoglobins in plateau zokor (Eospalax baileyi). Am. J. Phys. Regul. Integr. Comp. Phys. 317, R696–R708. doi: 10.1152/ajpregu.00335.2018
Pu, P., Zhao, Y., Niu, Z. Y., Cao, W. J., Zhang, T., He, J., et al. (2021). Comparison of hematological traits and oxygenation properties of hemoglobins from highland and lowland Asiatic toad (Bufo gargarizans). J. Comp. Physiol. B Biochem. Syst. Environ. Physiol. 191, 1019–1029. doi: 10.1007/s00360-021-01368-8
Qiu, Q., Zhang, G. J., Ma, T., Qian, W. B., Wang, J. Y., Ye, Z. Q., et al. (2012). The yak genome and adaptation to life at high altitude. Nat. Genet. 44, 946–949. doi: 10.1038/ng.2343
Qu, Y. H., Zhao, H. W., Han, N. J., Zhou, G. Y., Song, G., Gao, B., et al. (2013). Ground tit genome reveals avian adaptation to living at high altitudes in the Tibetan plateau. Nat. Commun. 4:2071. doi: 10.1038/ncomms3071
Riggs, A. F. (1988). The Bohr effect. Annu. Rev. Physiol. 50, 181–204. doi: 10.1146/annurev.ph.50.030188.001145
Rollema, H. S., and Bauer, C. (1979). The interaction of inositol pentaphosphate with the hemoglobins of highland and lowland geese. J. Biol. Chem. 254, 12038–12043. doi: 10.1016/S0021-9258(19)86424-4
Signore, A. V., and Storz, J. F. (2020). Biochemical pedomorphosis and genetic assimilation in the hypoxia adaptation of Tibetan antelope. Sci. Adv. 6:eabb5447. doi: 10.1126/sciadv.abb5447
Simonson, T. S., Yang, Y. Z., Huff, C. D., Yun, H. X., Qin, G., Witherspoon, D. J., et al. (2010). Genetic evidence for high-altitude adaptation in Tibet. Science 329, 72–75. doi: 10.1126/science.1189406
Storz, J. F., and Moriyama, H. (2008). Mechanisms of hemoglobin adaptation to high altitude hypoxia. High Alt. Med. Biol. 9, 148–157. doi: 10.1089/ham.2007.1079
Storz, J. F., Runck, A. M., Sabatino, S. J., Kelly, J. K., Ferrand, N., Moriyama, H., et al. (2009). Evolutionary and functional insights into the mechanism underlying high-altitude adaptation of deer mouse hemoglobin. Proc. Natl. Acad. Sci. U. S. A. 106, 14450–14455. doi: 10.1073/pnas.0905224106
Storz, J. F., Scott, G. R., and Cheviron, Z. A. (2010). Phenotypic plasticity and genetic adaptation to high-altitude hypoxia in vertebrates. J. Exp. Biol. 213, 4125–4136. doi: 10.1242/jeb.048181
Toledo, R. C., and Jared, C. (1993). Cutaneous adaptations to water-balance in amphibians. Comp. Biochem. Physiol. A Physiol. 105, 593–608. doi: 10.1016/0300-9629(93)90259-7
Weber, R. E. (1981). Cationic control of O2 affinity in lugworm erythrocruorin. Nature 292, 386–387. doi: 10.1038/292386a0
Weber, R. E. (1992). Use of ionic and Zwitterionic (Tris Bistris and Hepes) buffers in studies on hemoglobin-function. J. Appl. Physiol. 72, 1611–1615. doi: 10.1152/jappl.1992.72.4.1611
Weber, R. E., and Campbell, K. L. (2011). Temperature dependence of haemoglobin-oxygen affinity in heterothermic vertebrates: mechanisms and biological significance. Acta Physiol (Oxf.) 202, 549–562. doi: 10.1111/j.1748-1716.2010.02204.x
Weber, R. E., Jarvis, J. U. M., Fago, A., and Bennett, N. C. (2017). O2 binding and CO2 sensitivity in haemoglobins of subterranean African mole rats. J. Exp. Biol. 220, 3939–3948. doi: 10.1242/jeb.160457
Weber, R. E., and Jensen, F. B. (1988). Functional adaptations in hemoglobins from ectothermic vertebrates. Annu. Rev. Physiol. 50, 161–179. doi: 10.1146/annurev.ph.50.030188.001113
Weber, R. E., Jessen, T. H., Malte, H., and Tame, J. (1993). Mutant hemoglobins (alpha(119)-ala and Beta(55)-Ser)–functions related to high-altitude respiration in geese. J. Appl. Physiol. 75, 2646–2655. doi: 10.1152/jappl.1993.75.6.2646
Weber, R. E., Ostojic, H., Fago, A., Dewilde, S., Van Hauwaert, M. L., Moens, L., et al. (2002). Novel mechanism for high-altitude adaptation in hemoglobin of the Andean frog Telmatobius peruvianus. Am. J. Phys. Regul. Integr. Comp. Phys. 283, R1052–R1060. doi: 10.1152/ajpregu.00292.2002
Keywords: black-spotted frog, high altitude, hematological traits, temperature, Bohr effect, Hb-O2 affinity
Citation: Ma M, Pu P, Niu Z, Zhang T, Wu J, Tang X and Chen Q (2023) A novel mechanism for high-altitude adaptation in hemoglobin of black-spotted frog (Pelophylax nigromaculatus). Front. Ecol. Evol. 11:1103406. doi: 10.3389/fevo.2023.1103406
Edited by:
Liming Chang, Chengdu Institute of Biology (CAS), ChinaReviewed by:
Kevin Campbell, Faculty of Science, University of Manitoba, CanadaLusha Liu, Huazhong Agricultural University, China
Copyright © 2023 Ma, Pu, Niu, Zhang, Wu, Tang and Chen. This is an open-access article distributed under the terms of the Creative Commons Attribution License (CC BY). The use, distribution or reproduction in other forums is permitted, provided the original author(s) and the copyright owner(s) are credited and that the original publication in this journal is cited, in accordance with accepted academic practice. No use, distribution or reproduction is permitted which does not comply with these terms.
*Correspondence: Qiang Chen, Y2hlbnFAbHp1LmVkdS5jbg==
†These authors have contributed equally to this work