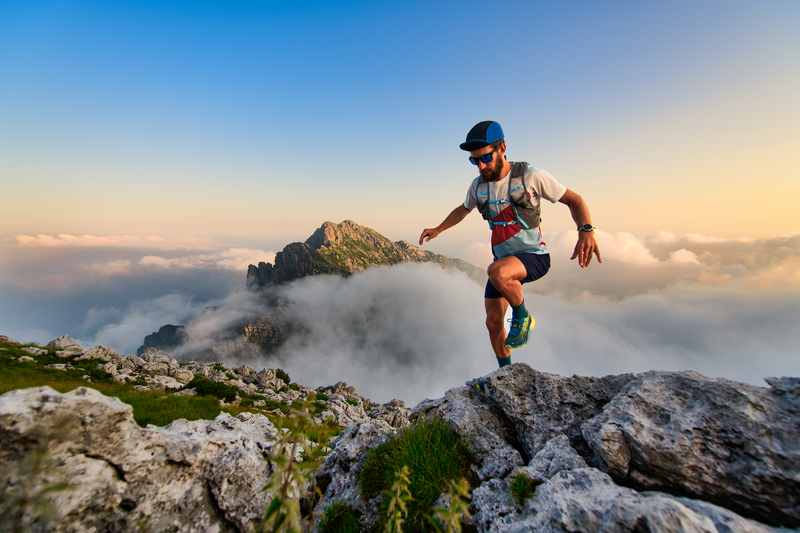
95% of researchers rate our articles as excellent or good
Learn more about the work of our research integrity team to safeguard the quality of each article we publish.
Find out more
ORIGINAL RESEARCH article
Front. Ecol. Evol. , 17 April 2023
Sec. Population, Community, and Ecosystem Dynamics
Volume 11 - 2023 | https://doi.org/10.3389/fevo.2023.1102457
This article is part of the Research Topic Insect Pollinators in the Anthropocene: How Multiple Environmental Stressors Are Shaping Pollinator Health View all 11 articles
Varroa destructor is a major threat for apiculture worldwide. A successful approach to control this parasite must include the application of effective treatments at the correct time. To understand the effect that treatment timing has on Varroa populations at different seasons, we conducted an experiment using a dataset comprising two separate field trials over multiple years, both trials containing four apiary sites composed of 20 honey bee colonies across an area representative of north central Florida environments. Before the start of the season, colonies were treated with two acaricides simultaneously to bring the Varroa populations to ∼0.25 mites/100 bees. Following treatment, we monitored the mite populations monthly via alcohol washes. Our results show that the temporal efficacy of Varroa treatments varies across seasons. We observed that it takes about 4–5 months after treatment in winter and spring for mite populations to return to the standard economical threshold (3 mites/100 bees). Nevertheless, there is a steeper increase in mite populations (<3 months to exceed the economic threshold) after treating colonies in summer and fall. The level of infestation that leads to colony collapse and the rate of colony decline also varied by season. To our knowledge, this is the first study evaluating seasonal effects on Varroa population growth and the first model of Varroa population growth in Florida, USA. Our results serve as a foundation for Varroa treatment models, aiding beekeepers in the future as a part of a holistic approach to control this devastating honey bee parasite.
For decades, beekeepers around the world have struggled to control Varroa destructor, an ectoparasitic mite, in their honey bee (Apis mellifera L.) colonies (Rosenkranz et al., 2010). The pest now has a worldwide distribution and can be found nearly everywhere honey bee colonies are managed (Boncristiani et al., 2021). Varroa’s impact is primarily linked to its ability to vector and transmit a large number of viruses (Genersch and Aubert, 2010; Traynor et al., 2020), severely weakening honey bee colony strength (Budge et al., 2015), and resulting in widespread colony losses (Brutscher et al., 2016; Gray et al., 2020).
Different non-chemical and chemical treatments are used by beekeepers to reduce V. destructor populations below the economic threshold, which is typically considered as 3 mites/100 adult bees (Jack and Ellis, 2021). The more time a colony spends above the economic threshold, the more likely it is to experience both increased viral infection and colony mortality (Kulhanek et al., 2021). Each year, beekeepers must decide which V. destructor treatment regimens are best to use in their management setting, this being based on several factors (Thoms et al., 2019; Underwood et al., 2019; Steinhauer et al., 2020). The most obvious factor is the efficacy of the treatment, which can vary under specific circumstances (Jack and Ellis, 2021). Weather conditions, particularly temperature and precipitation, have been observed to play a significant role in the efficacy of several V. destructor treatments (Beyer et al., 2018; Steube et al., 2021). Natural chemical treatments are particularly responsive to ambient temperature conditions, as volatile chemicals release gases based on the temperature where they are placed (Imdorf et al., 1995; Gracia et al., 2017). Even honey bee behaviors, such as grooming behaviors to remove V. destructor, can be affected by weather (Currie and Tahmasbi, 2008).
Beekeepers have historically relied heavily on synthetic chemical treatments to control V. destructor (Roth et al., 2020), but the efficacy of these treatments has become limited due to resistance issues (Haber et al., 2019). Some have observed treatment efficacy to vary according to location and season in which it is applied (Currie and Gatien, 2006; Gracia et al., 2017). As V. destructor can only reproduce within the capped brood cells containing honey bee pupae, the mites spend a significant amount of their lives inside these cells (Rosenkranz et al., 2010). Thus, the timing of chemical treatments is also essential for sustaining V. destructor control (Delaplane and Hood, 1997; Gatien and Currie, 2003), as the effectiveness of some treatments is considerably reduced if mites are hidden within the capped brood cells at time of application (Kraus and Berg, 1994; Rosenkranz et al., 2010; Al Toufailia and Ratnieks, 2018).
Another factor beekeepers must consider when attempting to control V. destructor is the amount of time required to apply the treatment. Some non-chemical treatments can be effective (Ellis et al., 2001; Wantuch and Tarpy, 2009; Kablau et al., 2020), but often require too much time. This makes such treatments unpopular among commercial beekeepers (Underwood et al., 2019). The length of time the treatment must remain in the hive is also an important factor to consider, as most chemical treatments are not labeled for use while honey supers are present on the hive (Honey Bee Health Coalition, 2018). To be successful with sustainable V. destructor control, beekeepers must consider all these variables in relation to beekeeping activities such as honey production, queen rearing, package bee production, and commercial pollination. It would be extremely valuable to beekeepers to have a decision tool to aid them in their selection of V. destructor treatments according to their own location and beekeeping situation.
Before a V. destructor control decision tool could be created, researchers need to understand the relationship between V. destructor population growth and the individual mite treatments. Many researchers have explored the complex dynamics of V. destructor population growth (reviewed by Fries et al., 1994; DeGrandi-Hoffman and Curry, 2004; Coffey et al., 2010; Ratti et al., 2012; DeGrandi-Hoffman et al., 2016). Some have created elaborate growth models which include several factors such as honey bee brood rearing, acaricidal efficacy, mite reproductive rates, and the total number of foragers with mites to name a few (reviewed by Fries et al., 1994; Wilkinson and Smith, 2002; DeGrandi-Hoffman and Curry, 2004; DeGrandi-Hoffman et al., 2016). Nevertheless, after thorough research, an extensive study evaluating the effect of season has not been conducted to our knowledge. By knowing the seasonal growth rate of V. destructor populations, one may be able to predict how long after treatment it takes mite populations to return to pretreatment levels.
It is necessary to create a model of natural V. destructor population growth by season as a first step toward the creation of a decision tool. Herein, we observed natural V. destructor population growth rates in north central Florida throughout multiple years and compared the growth rates by season. We hypothesized that V. destructor population growth would vary by season, with the fastest rate of growth happening in the summer and fall seasons, as that is when the greatest amount of capped brood is present in colonies. We made this hypothesis based on the knowledge that the population dynamics of V. destructor and honey bees are interwoven, as the mites can only reproduce within capped brood cells (Rosenkranz et al., 2010).
Two separate trials were conducted over multiple years. In both trials, groups of honey bee colonies were maintained in four different apiaries in north central Florida, all within 32 km of the University of Florida’s Honey Bee Research and Extension Laboratory (HBREL), Gainesville, FL (29°37′38″ N 82°21′23″ W). During each trial, a specific apiary was assigned to a designated calendar season. The apiary sites were as follows: (1) North Gainesville, FL (29°44′01″ N 82°16′31″ W), (2) Citra, FL (29°24′36″ N 82°08′48″ W), (3) Hawthorne, FL (29°35′24″ N 82°08′36″ W), and (4) HBREL (29°37′38″ N 82°21′23″ W). Trial 1 was initiated in January 2018 and trial 2 was initiated in April 2020. A map of the apiary locations assigned to each season for both trials is shown in Figure 1.
Figure 1. Satellite image of apiary locations used in both trials (image by Google Earth). Figure legend indicates the assigned season and trial used in each location.
At the start of each trial, apiaries contained 20 healthy honey bee colonies of European-derived honey bee stock. The genetic lineage of honey bees used in this study likely derived primarily from Apis mellifera ligustica stock, though we made no effort to use a specific stock. Honey bee stocks used in the U.S. are usually mixed-race (Schiff and Sheppard, 1995; Delaney et al., 2009). The bees used in this study were of the same genetic origins for both trial 1 and trial 2. Colonies were maintained in 10-frame Langstroth hives consisting of a single deep hive body and a solid bottom board. Brood combs were all a standard size and contained Plasticell foundation (Dadant & Sons, Inc., Hamilton, IL, USA). Colonies were equalized prior to the start of the experiment to ensure that each colony was of similar size and strength (approximately nine frames of bees and six frames of brood).
Prior to the start of each season, colonies within the assigned apiary were treated with acaricides to bring the V. destructor populations to an average of 0.25 mites/100 bees (high of 0.6 mites/100 bees). All colonies were treated with amitraz via Apivar® strips (Véto-pharma, New York, NY, USA) for 3 weeks instead of the recommended 6-week period to maintain appropriate timing of the seasonal groups. However, we do not believe that this reduced treatment period negatively affected the reduction of mites, as colonies were also simultaneously treated with 4 g of oxalic acid (OA) dihydrate (Sigma Aldrich, St. Louis, MO, USA) via vaporization. We used the commercially available ProVap 110® vaporizer (OxaVap LLC, Manning, SC, USA) to vaporize the OA, sealing the hive entrance and all cracks around the nest to limit the escape of the vapor as per Jack et al. (2021). Colonies received OA treatment once per week, for up to 3 consecutive weeks. This process was repeated for the colonies in each apiary prior to the beginning of their respective seasons. Once the experimental colonies’ V. destructor populations were ∼0.25 mites/100 bees, no further treatments were administered. All experimental colonies were managed according to best management practices that are common for this region (feeding bees when necessary, swarm control, etc.), with the exception of applications of additional miticides to control growing V. destructor populations. To maintain the integrity of the study, no brood combs were shared between colonies, even within the same seasonal cohort. Furthermore, small hive beetle (Aethina tumida) traps were added to all colonies to reduce the effects of beetle damage. All experimental colonies were treated with oxytetracycline (Tetra-B Mix 2X®, Dadant & Sons, Inc., Hamilton, IL, USA) to minimize the potential of foulbrood outbreaks. The number of surviving colonies was recorded each month, given there was some colony mortality. Colonies were considered dead once there were no more adult bees to sample or if the health of the colony would have been significantly impacted by the sampling. As colony populations began to decline, they were fed sugar syrup when needed and entrance reducers were placed on hive entrances to limit robbing. We believe that the colony mortality observed in this study was a result of the high V. destructor populations and associated virus loads rather than from the treatment regimen, as few colonies in this study died within 2–3 months after treatment.
Varroa destructor population growth was monitored for every colony monthly using alcohol washes according to the technique described in Dietemann et al. (2013). Each month, 200–300 bees were collected from the brood area and a ratio of # mites/100 bees was calculated for each sample. Monitoring of V. destructor continued for each group until the mite population peaked and then declined for 2 consecutive months, or until all of the colonies within a cohort died. A decline in V. destructor populations indicates that the colonies with severe infestations are collapsing and the remaining colonies are in similar danger. Ending the study after 2 months of consecutive mite population decline allowed us to rescue the remaining colonies before their collapse.
All analyses were performed using the glmer function from the package lme4 (Bates et al., 2015) implemented in the R platform (R Core Team, 2022). Models for each analysis are described below. Graphical visualizations were obtained using ggplot2 (Wickham, 2016).
The main interest with our present research was to observe the effect of season on V. destructor infestation. For that, the following generalized linear mixed model was used (i.e., model 1):
where, is the responsible variable (i.e., # mites/100 bees), μ is overall mean, t is the effect of time since last treatment, se is the effect of last season treated, t × se is the interaction effect between time since last treatment and the last season treated, h is the effect of each hive evaluated (hiveID), and e the vector for the residual error. X1, X2, X3, and Z1 are the incidence matrices for time since last treatment, last season treated, the interaction between time since last treatment and last season treated, and hiveID, respectively. Only hiveID was not considered as a fixed effect, to account for the independent variances of each hive evaluated. The analysis was implemented using the package lme4 (Bates et al., 2015). Autocorrelation regarding the repeated measures was accounted in the model and a Poisson distribution was assumed, given the nature of the data and its skewed distribution. As colony mortality began to be significantly impacted after 6 months, creating significantly unbalanced data, seasonal V. destructor population growth was only analyzed considering the first 7 months of data. Preliminary analysis was performed to select the best model to be used in this analysis (i.e., model 1). For this, nested generalized linear mixed models were tested and comparisons were made considering model fit parameters (AIC, number of parameters, significance in the ANOVA model comparison; data not shown). From these results, we identified that V. destructor population growth per season was not dependent upon trial (P = 0.998) and that the addition of trial effect turned the model singular, which could be indicative of overfitting. Thus, trial effect was not considered in model 1.
The following generalized linear mixed model was used to infer the effect of last season treated on hive lifespan.
In this model, is the responsible variable (i.e., hive’s lifespan), μ is overall mean, T is the effect of trial, se is the effect of last season treated, T × se is the interaction effect between trial and the last season treated, T:h is the effect of each hiveID nested on trial, and e the vector for the residual error. X1, X2, X3, and Z1 are the incidence matrices for trial, last season treated, the interaction between trial and last season treated, and hiveID, respectively. As in the previous model, only hiveID was considered as a random effect, accounting for the independent variances of each hive evaluated. Given the nature of the responsible variable (i.e., counting data), a Poisson distribution was assumed for the analysis.
In order to verify significance between the factors tested in each analysis, post hoc tests assuming Sidak correction for multiple comparisons were performed (σ = 0.05), using functions implemented in the package emmeans v. 1.7.5 (Lenth, 2022).
Varroa destructor population growth per season was not dependent upon the trial (P = 0.998), so data from both trials were combined for subsequent analyses. V. destructor population growth data became unbalanced 6 months post-treatment for all seasons due to colony mortality (Figure 2 and Supplementary Figure 1). Thus, we only included the first 6 months post-treatment in our analyses. There were no significant differences in starting V. destructor levels each season after treatments were administered (P > 0.05). Yet, there was strong evidence to support the observation that V. destructor population growth varies depending on which season the treatment was applied (Figure 2 and Supplementary Table 1).
Figure 2. Average Varroa destructor population growth expressed as number of mites/100 bees after 6 months for each season. These results combine data from both trials 1 and 2. The dashed red line represents the standard economical threshold of 3 mites/100 bees. There was a significant impact of season treatment was administered on the mites/100 bees (P ≤ 0.05). Significant differences between means are indicated with different letters (α = 0.05; a > b > c).
There are some notable observations when examining V. destructor population growth by season. First, when mite populations are reduced in the winter and spring seasons, V. destructor population growth rates remain below the economic threshold (3 mites/100 bees) for 4–5 months, respectively (Figure 2). Second, V. destructor populations rapidly increased after the summer and fall seasons’ treatments, extending beyond the economic threshold less than 3 months after treatment (Figure 2). Third, mite populations peak only 4 months after a summer treatment before significant colony mortality is observed, causing a decline in average V. destructor populations. Meanwhile V. destructor populations may be sustained at higher levels 5–6 months after a fall treatment, likely due to a buildup of bees during the spring (Figure 2).
There was a significant interaction between colony lifespan per season and trial effect (P < 0.05, Supplementary Table 2); therefore, data from each trial were analyzed separately, and interpretations were made separately within each trial. The colony lifespan during trial 2 was generally shorter than that during trial 1 (Figure 3 and Supplementary Table 2). In trial 1, colonies treated during summer had the shortest of all colony lifespans, averaging 7.5 months of survival post-treatment. Regardless, there is no evidence that survival after fall treatment was different than that after summer at 8.5 months survival post-treatment. Colonies treated in the winter season had significantly longer survival post-treatment during trial 1 than did any other group, with an average colony surviving 11.5 months. In trial 2, treatment in the summer and winter seasons ultimately led to the quickest mortality, with colonies averaging only 6.9 and 7.6 months of survival post treatment, respectively. The colonies that survived the longest during trial 2 were treated in the fall and spring seasons, surviving 8.9- and 8-months post-treatment, respectively.
Figure 3. Box plots illustrating the assumed lifespan distribution for colonies (in months) in the season in which treatment were applied for both trials. The × indicates the mean survival for colonies within that seasonal cohort. There was a significant impact of season the mite treatment was administered on colony lifespan within trial (P ≤ 0.05). Post-hoc tests were performed within trial and significant differences between means are indicated with different letters (α = 0.05; a > b > c).
We evaluated an extensive and robust dataset composed of 160 honey bee colonies to understand V. destructor population growth seasonally. Our main goal was to generate information that can guide beekeepers as a part of a holistic approach to control this devastating honey bee parasite. Our results not only confirm the importance of seasonal effects on the efficacy of treatments for the mite, but they can also be used as a natural model of V. destructor population growth for north central Florida, and possibly colonies kept in similar climates. Additionally, we believe that the results of this study demonstrate the importance of regular V. destructor monitoring and treatment by beekeepers, as colony survival is often less than 1 year for untreated colonies in Florida. We anticipate that the benefits and information described in our study can be applied to optimize treatment and control for V. destructor in Florida and can help guide studies and research for the control of this parasite worldwide.
Although much has been written related to V. destructor population growth, to our knowledge, this is the first attempt to determine V. destructor population growth seasonally after treatment. As V. destructor population growth is closely tied to honey bee brood rearing (Wilkinson and Smith, 2002), it is not surprising that mite levels were able to rebound rapidly after treatment in the summer and fall seasons when honey bee brood is plentiful in Florida (Figure 2). However, somewhat surprising is the significantly slower V. destructor population growth after treatment in the spring when compared to that in winter (Figure 2). One might predict that in colonies where mites were reduced to near-zero in the winter, V. destructor populations would be delayed in growth due to the lack of honey bee brood required for reproduction. Interestingly, mite levels were still significantly lower in the spring than in all other seasons 3 months after treatment, making this the longest period during which mite populations were maintained below the economic threshold (Figure 2). Dolezal et al. (2016) demonstrated how the environment in which colonies are placed can influence the nutritional physiology of the colony, thus directly affecting V. destructor presence in a given environment. As honey bee colonies in the spring season have access to more floral resources, this nutritional advantage may have allowed the bees to defend or guard against V. destructor reproduction or reinfestation better than presumably nutrition-deficient colonies treated in winter.
Information regarding seasonal V. destructor population growth is applicable to beekeepers who struggle to maintain mite populations below economic thresholds (Jack and Ellis, 2021; Brodschneider et al., 2022). It appears that reducing V. destructor populations in the spring season is important for long lasting mite control. An effective reduction of mite populations in the spring could provide beekeepers sufficient coverage through the spring and summer months, effectively reducing the likelihood of necessary treatments during the major nectar flows for most temperate regions. Winter is also an effective season to treat for V. destructor, providing beekeepers with coverage through the spring season. However, even after an effective winter treatment, mite populations could still return to economic thresholds by the spring season. In this case, the beekeeper has a difficult decision; either they interrupt their colonies’ honey production during a major nectar flow or they delay treatment until after honey supers have been removed. If beekeepers are not able to reduce the V. destructor population below the economic threshold of 3 mites/100 bees, their colonies are likely to succumb to viral infection (Kulhanek et al., 2021).
While reducing V. destructor populations in summer and fall seasons may be important, the benefits of doing so could be very short-lived. A reduction of mite populations in summer only resulted in about 2 months of coverage for the beekeeper, meaning that another treatment in fall would be necessary. Unfortunately, reducing mite levels in fall again only provides 2 months of coverage, requiring another treatment in winter. Thus, it appears that multiple treatments are likely necessary if mite populations reach economic thresholds in the summer and fall seasons. Frequent treatments such as these can, increase the likelihood that the mites develop resistance to chemical treatments and the cost of controlling V. destructor to beekeepers. However, the need to reduce V. destructor populations in the summer and fall months is crucial, as viral titers tend to be at their highest and colonies are most severely impacted during these seasons (Highfield et al., 2009; Dainat et al., 2012; Traynor et al., 2016).
Varroa destructor treatments can vary widely in cost, with some treatments, for instance oxalic acid, costing less than others, such as the synthetic compound Apivar™. Thus, optimizing treatment efficacy and reducing the frequency of treatments could provide the beekeeper with considerable savings. Based on the V. destructor population growth data presented in Figure 2, we created Table 1 to provide a better understanding of when a beekeeper could expect to apply miticides for the control of the pest. Depending upon when the treatment regime is started, a beekeeper may need to apply one additional treatment over the period of 2 years. Perhaps for a hobbyist beekeeper, that would not equate to significant savings, but it might for a large commercial operation. However, we created Table 1 to be used as a reference for when colonies may need treatment, as treatment efficacy can be region-specific and not all treatments can be applied at all times of the year (Jack and Ellis, 2021). Thus, beekeepers should not stay on a strict treatment regimen but should closely monitor V. destructor populations to determine treatment timing.
Table 1. This table provides a proposed treatment (trt) schedule based on the month (January–December) that beekeepers apply their first treatment (light green).
The difference in colony lifespan post-treatment between the two trials was stark (Figure 3). For instance, survival was greatest in trial one after treatment in the winter. In trial 2, the winter treatment survival was similar to that of the summer group, with both being low. It is possible that these two winter treatments were affected differently by their location, as colonies receiving the winter treatment in trial one were located in the northern Gainesville apiary and while in trial 2, they were located at the Hawthorne apiary. Both apiary sites are ∼24 km apart, yet the floral resources available at the Hawthorne apiary are more plentiful than those at the northern Gainesville apiary during the late spring and summer months. Nutritionally, colonies in the Hawthorne apiary may have been better able to handle V. destructor after a winter treatment than were those at the Gainesville apiary. It is also possible that viral titers differed between colonies at the two sites, leading to the different responses of mite populations to winter treatments at both apiaries. While we believe that the bees used in this study were of the same genetic origins for both trial 1 and trial 2, slight genetic differences may have existed between the two populations. It is possible that these genetic differences of the bees used in both trials could have led to varying rates of death after exposure to elevated V. destructor levels. Weather differences, or other environmental parameters could have played a role. Unfortunately, we cannot determine the impact of location, genetics, virus load or weather on colony survival as we were only able to conduct two trials for this experiment and did not collect all the data necessary to make these determinations.
There are other variables that would likely impact V. destructor populations and warrant additional exploration. These variables could include temperature, frost-free days, rainy/dry seasons, nectar flows, and growing seasons. As mite population growth is closely tied to honey bee brood rearing (Wilkinson and Smith, 2002), the same climatic conditions that increase brood rearing likely also increase V. destructor populations. Ultimately, we can only use our results to predict the V. destructor population outcomes in north central Florida. However, beekeepers managing hives in areas with similar rates of brood rearing could use our work to assist with predictions of their own colonies’ V. destructor population growth. Therefore, regional or countrywide honey bee brood surveillance would be helpful for predicting mite population growth with greater resolution. Such a surveillance program for the southeastern USA is currently underway (G. Williams, personal communication, University of Auburn).
Beekeepers are in desperate need of effective controls to use against V. destructor. As the development of new controls can take many years, it is essential that beekeepers utilize existing treatments more efficiently. We believe that the research presented herein helps us better understand the seasonal efficacy of V. destructor treatments and could potentially aid in the development of a mite control decision tool for beekeepers. More effective timing of treatments could reduce the frequency of treatments, thereby reducing the likelihood of V. destructor development of resistance to a given miticide. Additional research related to V. destructor population predictions and modeling is essential for long-term, sustainable management of this devastating honey bee parasite.
The raw data supporting the conclusions of this article will be made available by the authors, without undue reservation.
CJ and JE: study conception and design, and interpretation of results. CJ: data collection. CK and IB: data analysis and interpretation of results. CJ, CK, IB, and JE: draft manuscript preparation. All authors reviewed the results, contributed to the article, and approved the submitted version.
This research was supported by a scholarship to CJ from the Costco-Apis Scholarship Program, USDA National Institute of Food and Agriculture Multistate Projects (1005822 and 1019945), and USDA Animal and Plant Health Inspection Service (APHIS) cooperative agreements (AP19PPQS&T00C051 and AP21PPQS&T00C049). The funding sources had no involvement in any aspects of the study.
We gratefully thank Walter Taylor, Kaylin Kleckner, Jon Elmquist, Steven Keith, and Branden Stanford for their help managing the experimental honey bee colonies. We also thank Christopher Oster, Michelle Weschler, Ava Bruner, and Zion Needham for their help collecting mite wash data during the experiment.
The authors declare that the research was conducted in the absence of any commercial or financial relationships that could be construed as a potential conflict of interest.
All claims expressed in this article are solely those of the authors and do not necessarily represent those of their affiliated organizations, or those of the publisher, the editors and the reviewers. Any product that may be evaluated in this article, or claim that may be made by its manufacturer, is not guaranteed or endorsed by the publisher.
The Supplementary Material for this article can be found online at: https://www.frontiersin.org/articles/10.3389/fevo.2023.1102457/full#supplementary-material
Al Toufailia, H., and Ratnieks, F. (2018). Towards integrated control of varroa: 5) monitoring honey bee brood rearing in winter, and the proportion of varroa in small patches of sealed brood cells. J. Apicul. Res. 57, 444–451. doi: 10.1080/00218839.2018.1460907
Bates, D., Maechler, M., Bolker, B., and Walker, S. (2015). Fitting linear mixed-effects models using lme4. J. Stat. Soft. 67, 1–48. doi: 10.18637/jss.v067.i01
Beyer, M., Junk, J., Eickermann, M., Clermont, A., Kraus, F., Georges, C., et al. (2018). Winter honey bee colony losses, Varroa destructor control strategies, and the role of weather conditions: Results from a survey among beekeepers. Res. Vet. Sci. 118, 52–60. doi: 10.1016/j.rvsc.2018.01.012
Boncristiani, H., Ellis, J., Bustamante, T., Graham, J., Jack, C., Kimmel, C., et al. (2021). World honey bee health: The global distribution of western honey bee (Apis mellifera L.) pests and pathogens. Bee World 98, 2–6. doi: 10.1080/0005772X.2020.1800330
Brodschneider, R., Schlagbauer, J., Arakelyan, I., Ballis, A., Brus, J., Brusbardis, V., et al. (2022). Spatial clusters of Varroa destructor control strategies in Europe. J. Pest. Sci. 96, 759–783. doi: 10.1007/s10340-022-01523-2
Brutscher, L., McMenamin, A., and Flenniken, M. (2016). The buzz about honey bee viruses. PLoS Pathog. 12:e1005757. doi: 10.1371/journal.ppat.1005757
Budge, G., Pietravalle, S., Brown, M., Laurenson, L., Jones, B., Tomkies, V., et al. (2015). Pathogens as predictors of honey bee colony strength in England and Wales. PLoS One 10:e0133228. doi: 10.1371/journal.pone.0133228
Coffey, M., Breen, J., Brown, M., and McMullan, J. (2010). Brood-cell size has no influence on the population dynamics of Varroa destructor mites in the native western honey bee Apis mellifera mellifera. Apidologie 41, 522–530. doi: 10.1051/apido/2010003
Currie, R. W., and Tahmasbi, G. H. (2008). The ability of high- and low-grooming lines of honey bees to remove the parasitic mite Varroa destructor is affected by environmental conditions. Can. J. Zool. 86, 1059–1067. doi: 10.1139/Z08-083
Currie, R., and Gatien, P. (2006). Timing acaricide treatments to prevent Varroa destructor (Acari: Varroidae) from causing economic damage to honey bee colonies. Can. Entomol. 138, 238–252. doi: 10.4039/n05-024
Dainat, B., Evans, J. D., Chen, Y. P., Gauthier, L., and Neumann, P. (2012). Predictive markers of honey bee colony collapse. PLoS One 7:e32151. doi: 10.1371/journal.pone.0032151
DeGrandi-Hoffman, G., Ahumada, F., Zazueta, V., Chambers, M., Hidalgo, G., and DeJong, E. (2016). Population growth of Varroa destructor (Acari: Varroidae) in honey bee colonies is affected by the number of foragers with mites. Exp. App. Acarol. 69, 21–34. doi: 10.1007/s10493-016-0022-9
DeGrandi-Hoffman, G., and Curry, R. (2004). A mathematical model of varroa mite (Varroa destructor Anderson and Trueman) and honeybee (Apis mellifera L.) population dynamics. Int. J. Acarol. 30, 259–274. doi: 10.1080/01647950408684393
Delaney, D. A., Meixner, M. D., Schiff, N. M., and Sheppard, W. S. (2009). Genetic characterization of commercial honey bee (Hymenoptera: Apidae) populations in the United States by using mitochondrial and microsatellite markers. Ann. Entomol. Soc. Am. 102, 666–673. doi: 10.1603/008.102.0411
Delaplane, K., and Hood, W. (1997). Effects of delayed acaricide treatment in honey bee colonies parasitized by Varroa jacobsoni and a lateseason treatment threshold for the southeastern USA. J. Apicult. Res. 36, 125–132. doi: 10.1080/00218839.1997.11100938
Dietemann, V., Nazzi, F., Martin, S., Anderson, D., Locke, B., Delaplane, K., et al. (2013). Standard methods for varroa research. J. Apicult. Res. 52, 1–54. doi: 10.3896/IBRA.1.52.1.09
Dolezal, A. G., Carrillo-Tripp, J., Miller, W. A., Bonning, B. C., and Toth, A. L. (2016). Intensively cultivated landscape and Varroa mite infestation are associated with reduced honey bee nutritional state. PLoS One 11:e0153531. doi: 10.1371/journal.pone.0153531
Ellis, J., Delaplane, K., and Hood, W. (2001). Efficacy of a bottom screen device, Apistan™, and Apilife VAR™, in controlling Varroa destructor. Am. Bee J. 141, 813–816.
Fries, I., Camazine, S., and Sneyd, J. (1994). Population dynamics of Varroa jacobsoni: A model and a review. Bee World 75, 5–28. doi: 10.1080/0005772X.1994.11099190
Gatien, P., and Currie, R. (2003). Timing of acaracide treatments for control of low-level populations of Varroa destructor (Acari : Varroidae) and implications for colony performance of honey bees. Can. Ent. 135, 749–763. doi: 10.4039/n02-086
Genersch, E., and Aubert, M. (2010). Emerging and re-emerging viruses of the honey bee (Apis mellifera L.). Vet. Res. 41:54. doi: 10.1051/vetres/2010027
Gracia, M., Moreno, C., Ferrer, M., Sanz, A., Peribáñez, M., and Estrada, R. (2017). Field efficacy of acaricides against Varroa destructor. PLoS One 12:e0171633. doi: 10.1371/journal.pone.0171633
Gray, A., Adjlane, N., Arab, A., Ballis, A., Brusbardis, V., Charrière, J., et al. (2020). Honey bee colony winter loss rates for 35 countries participating in the COLOSS survey for winter 2018–2019, and the effects of a new queen on the risk of colony winter loss. J. Apicult. Res. 59, 744–751. doi: 10.1080/00218839.2020.1797272
Haber, A., Steinhauer, N., and vanEngelsdorp, D. (2019). Use of chemical and nonchemical methods for the control of Varroa destructor (Acari: Varroidae) and associated winter colony losses in U.S. beekeeping operations. J. Econ. Entomol. 112, 1509–1525. doi: 10.1093/jee/toz088
Highfield, A. C., Nagar, A. E., Mackinder, L. C., Noël, L. M., Hall, M. J., Martin, S. J., et al. (2009). Deformed wing virus implicated in overwintering honeybee colony losses. Appl. Environ. Microbiol. 75, 7212–7220. doi: 10.1128/AEM.02227-09
Honey Bee Health Coalition (2018). Tools for varroa management a guide to effective varroa sampling & control. Available online at: https://honeybeehealthcoalition.org/wp-content/uploads/2018/06/HBHC-Guide_Varroa_Interactive_7thEdition_June2018.pdf (accessed November 7, 2022).
Imdorf, A., Bogdanov, S., Kilchenmann, V., and Maquelin, C. (1995). Apilife var: A new varroacide with thymol as the main ingredient. Bee World 76, 77–83. doi: 10.1080/0005772X.1995.11099245
Jack, C., and Ellis, J. (2021). Integrated pest management control of Varroa destructor (Acari: Varroidae), the most damaging pest of (Apis mellifera L. (Hymenoptera: Apidae)) colonies. J. Insect Sci. 21, 1–32. doi: 10.1093/jisesa/ieab058
Jack, C., van Santen, E., and Ellis, J. (2021). Determining the dose of oxalic acid applied via vaporization needed for the control of the honey bee (Apis mellifera) pest Varroa destructor. J. Apicul. Res. 60, 414–420. doi: 10.1080/00218839.2021.1877447
Kablau, A., Berg, S., Härtel, S., and Scheiner, R. (2020). Hyperthermia treatment can kill immature and adult Varroa destructor mites without reducing drone fertility. Apidologie 51, 307–315. doi: 10.1007/s13592-019-00715-7
Kraus, B., and Berg, S. (1994). Effect of lactic acid treatment during winter in temperate climate upon Varroa jacobsoni Oud. and the bee (Apis mellifera L.) colony. Exp. Appl. Acarol. 18, 459–468. doi: 10.1007/BF00051468
Kulhanek, K., Steinhauer, N., Wilkes, J., Wilson, M., Spivak, M., Sagili, R., et al. (2021). Survey-derived best management practices for backyard beekeepers improve colony health and reduce mortality. PLoS One 16:e0245490. doi: 10.1371/journal.pone.0245490
Lenth, R. (2022). Emmeans: Estimated marginal means, aka least-squares means R package version 1.7.5.
R Core Team (2022). R: A language and environment for statistical computing. Vienna: R Foundation for Statistical Computing.
Ratti, V., Kevan, P., and Eberl, H. (2012). A mathematical model for population dynamics in honeybee colonies infested with Varroa destructor and the Acute Bee Paralysis Virus. Can. Appl. Math. Quart. 21, 63–93. doi: 10.1007/s11538-017-0281-6
Rosenkranz, P., Aumeier, P., and Ziegelmann, B. (2010). Biology and control of Varroa destructor. J. Invertebr. Pathol. 103, S96–S119. doi: 10.1016/j.jip.2009.07.016
Roth, M., Wilson, J., Taylor, K., and Gross, A. (2020). Biology and management of Varroa destructor (Mesostigmata: Varroidae) in Apis mellifera (Hymenoptera: Apidae) colonies. J. Integr. Pest. Manag. 11, 1–8. doi: 10.1093/jipm/pmz036
Schiff, N. M., and Sheppard, W. S. (1995). Genetic analysis of commercial honey bees (Hymenoptera: Apidae) from the southeastern United States. J. Econ. Entomol. 88, 1216–1220. doi: 10.1093/jee/88.5.1216
Steinhauer, N., vanEngelsdorp, D., and Saegerman, C. (2020). Prioritizing changes in management practices associated with reduced winter honey bee colony losses for US beekeepers. Sci. Total Environ. 753:141629. doi: 10.1016/j.scitotenv.2020.141629
Steube, X., Beinert, P., and Kirchner, W. H. (2021). Efficacy and temperature dependence of 60% and 85% formic acid treatment against Varroa destructor. Apidologie 52, 720–729. doi: 10.1007/s13592-021-00859-5
Thoms, C., Nelson, K., Kubas, A., Steinhauer, N., Wilson, M., and vanEngelsdorp, D. (2019). Beekeeper stewardship, colony loss, and Varroa destructor management. Ambio 48, 1209–1218. doi: 10.1007/s13280-018-1130-z
Traynor, K. S., Mondet, F., de Miranda, J. R., Techer, M., Kowallik, V., Oddie, M., et al. (2020). Varroa destructor: A complex parasite, crippling bees worldwide. Trends Parasitol. 36, 5–19. doi: 10.20944/preprints202002.0374.v1
Traynor, K. S., Rennich, K., Forsgren, E., Rose, R., Pettis, J., Kunkel, G., et al. (2016). Multiyear survey targeting disease incidence in US honey bees. Apidologie 47, 325–347. doi: 10.1007/s13592-016-0431-0
Underwood, R., Traver, B., and López-Uribe, M. (2019). Beekeeping management practices are associated with operation size and beekeepers’ philosophy towards in-hive chemicals. Insects 10:10. doi: 10.3390/insects10010010
Wantuch, H., and Tarpy, D. (2009). Removal of drone brood from Apis mellifera (Hymenoptera: Apidae) colonies to control Varroa destructor (Acari: Varroidae) and retain adult drones. J. Econ. Entomol. 102, 2033–2040. doi: 10.1603/029.102.0603
Wickham, H. (2016). Ggplot2: Elegant graphics for data analysis. New York, NY: Springer-Verlag. doi: 10.1007/978-3-319-24277-4
Keywords: Apis mellifera, Varroa, population, honey bee, infestation, season, survival
Citation: Jack CJ, de Bem Oliveira I, Kimmel CB and Ellis JD (2023) Seasonal differences in Varroa destructor population growth in western honey bee (Apis mellifera) colonies. Front. Ecol. Evol. 11:1102457. doi: 10.3389/fevo.2023.1102457
Received: 18 November 2022; Accepted: 28 March 2023;
Published: 17 April 2023.
Edited by:
Lars Straub, University of Bern, SwitzerlandReviewed by:
Alexis Beaurepaire, University of Bern, SwitzerlandCopyright © 2023 Jack, de Bem Oliveira, Kimmel and Ellis. This is an open-access article distributed under the terms of the Creative Commons Attribution License (CC BY). The use, distribution or reproduction in other forums is permitted, provided the original author(s) and the copyright owner(s) are credited and that the original publication in this journal is cited, in accordance with accepted academic practice. No use, distribution or reproduction is permitted which does not comply with these terms.
*Correspondence: Cameron J. Jack, Y2phY2tAdWZsLmVkdQ==
Disclaimer: All claims expressed in this article are solely those of the authors and do not necessarily represent those of their affiliated organizations, or those of the publisher, the editors and the reviewers. Any product that may be evaluated in this article or claim that may be made by its manufacturer is not guaranteed or endorsed by the publisher.
Research integrity at Frontiers
Learn more about the work of our research integrity team to safeguard the quality of each article we publish.