- 1Horn Point Laboratory, University of Maryland Center for Environmental Science, Cambridge, MD, United States
- 2Department of Geography, University of California, Santa Barbara, CA, United States
- 3Wye Research and Education Center, University of Maryland, Queenstown, MD, United States
- 4Department of Plant Pathology and Ecology, The Connecticut Agricultural Experiment Station, New Haven, CT, United States
Tidal marshes are important sites of silicon (Si) transformation, where dissolved Si (DSi) taken up by macrophytic vegetation and algal species is converted to biogenic silica (BSi), which can accumulate in the soil, be recycled within the marsh, or be exported to adjacent coastal waters. The role of restored and created tidal marshes in these processes is not well understood, nor is the impact of nutrient enrichment at either the plant or ecosystem level. Here, Si fluxes were examined to develop a Si mass balance in a nitrogen (N)-enriched marsh created with fine-grained dredged material from the Chesapeake Bay, United States. In addition, the effectiveness of Si soil amendments to ameliorate the negative effects of excess nitrogen on Spartina alterniflora was examined through laboratory and field experiments. Silicon was exported to the estuary as DSi (49 g m−2 y−1) and BSi (35 g m−2y−1) in stoichiometric excess of nitrogen and phosphorus. Rapid recycling of Si within both marsh and the tidal creeks appeared to be important in the transformation of Si and export from the marsh. Enhanced macrophyte SiO2 tissue concentrations were observed in the field experiment, with end-of-season mean values of 2.20–2.69% SiO2 in controls and 2.49–3.24% SiO2 in amended plots, among the highest reported for S. alterniflora; however, improved plant fitness was not detected in either experiment. Thus, tidal marshes created with a fine-grained, N-rich dredged material appear to function as a rich source of Si to the restored marsh and local estuarine environment, an overlooked ecosystem service. Soil Si amendments, however, did not appear likely to alleviate N-induced stress in S. alterniflora.
1. Introduction
Silicon (Si) is important for vascular plant metabolism and growth (Datnoff et al., 2001) and is essential for marine diatoms (protists), which often form the basis of marine food webs (Ragueneau et al., 2006). It is transported to coastal waters from terrestrial systems via riverine and groundwater inputs (Tréguer et al., 1995; Conley, 1997), as particulate and dissolved Si (DSi) derived from the weathering of terrigenous rock, and as biogenic Si (BSi) from terrestrial vegetation. As a link between terrestrial and coastal ecosystems, tidal marshes are positioned to play a key role in regulating Si fluxes.
In terrestrial ecosystems, amorphous Si (ASi) largely consists of BSi derived primarily from Si deposited in plant tissue in the form of phytoliths and other biological sources, combined with mineral (non-biological) non-crystalline forms, and can persist in the soil indefinitely (Conley, 1998). Occluded carbon (C) within phytoliths can contribute to C sequestration through organic matter burial (Parr and Sullivan, 2005; Li et al., 2013). In coastal areas, tidal flooding and groundwater flow promote soil ASi dissolution (Hackney et al., 2000). ASi has a solubility several orders of magnitude greater than crystalline mineral silicates (Van Cappellen, 2003), and regular flooding in salt marshes further promotes ASi dissolution due to higher pH and the catalytic effect of cations in seawater (Loucaides et al., 2008). Higher fluxes of DSi from tidal salt marshes compared with tidal freshwater marshes (Struyf and Conley, 2009) can result in Si leached soils in some mature salt marshes (Müller et al., 2013). This leads to export of ecologically significant amounts of DSi to adjacent subtidal ecosystems (Vieillard et al., 2011; Schoelynck et al., 2014; Carey and Fulweiler, 2014a).
Coastal eutrophication has disrupted the historically diatom-based food web in many coastal ecosystems, including Chesapeake Bay, stimulating diatom growth and resulting in DSi depletion early in the growing season (Officer and Ryther, 1980; Conley and Malone, 1992). Despite its relatively high abundance in terrestrial and many aquatic ecosystems, seasonal Si depletion in eutrophic estuaries has led to shifts in the dominance of algal species in summer and is hypothesized to promote the proliferation of harmful algal blooms in some coastal areas (Cooper and Brush, 1991; Humborg et al., 2000).
The extent to which tidal marshes may buffer the impact of eutrophication on Si depletion in estuarine and coastal areas remains unclear, with a wide range of reported estimates of Si exchange rates (Norris and Hackney, 1999; Struyf et al., 2006; Vieillard et al., 2011; Carey and Fulweiler, 2013; Müller et al., 2013). Despite increased tidal marsh restoration and creation, there are few estimates of Si fluxes from new or restored marshes (Jacobs et al., 2008) and their importance in local Si cycling is not well understood. Fluxes may differ from natural marshes due to substrate differences, e.g., lower organic matter content, and different rates of macrophytic productivity and biogeochemical cycling. Studies of Si fluxes from restored and created marshes are needed to enhance our understanding of how these practices may affect estuarine and coastal Si dynamics (Giblin et al., 2021).
Eutrophication can also have profound impacts on plant growth and morphology, leading to physical changes in tidal marsh soils and geomorphology. The response of Spartina alterniflora, the dominant species growing in many coastal marshes, to eutrophication includes enhanced annual biomass production (Mendelssohn and Morr, 2002; Darby and Turner, 2008), a shift in biomass allocation (root:shoot ratio, RSR) in favor of shoot growth (Darby and Turner, 2008) and lodging (collapse of excessively tall culms; Deegan et al., 2012). Nitrogen enrichment of plant tissue can also lead to increased predation by grazers and susceptibility to pathogens, likely due to changes in lignin content, tissue SiO2 concentrations, and other anatomical and biochemical changes (Marschner, 2012). Long-term exposure to high levels of N and phosphorus (P) may ultimately undermine the structural integrity of salt marshes, leading to peat collapse and increasing vulnerability to sea level rise (Turner, 2011; Deegan et al., 2012).
Although Si has not historically been considered essential for growth of most plants, Epstein (1999) considered it “quasi-essential” due to its important role in mitigating biotic and abiotic stress. Silicon is taken up by plants primarily as silicic acid (H4SiO4), the hydrated form of SiO2. It promotes survival of many taxa, including the Bryophyta (bryophytes), the Lycopsida (club mosses), Equisetopsida (horsetails), Pteridophyta (ferns), and, among the angiosperms, the Cyperaceae (sedges) and the Poaceae (grasses; Hodson et al., 2005). Species within the Poaceae are particularly susceptible to Si deficiency under conditions of high fertility, and often benefit from a Si soil amendment to reduce the effects of N enrichment (Epstein, 2001; Ma et al., 2001). For example, in the cultivation of rice (Oryza sativa), a wetland graminoid, Si leaching from paddy soils due to continuous flooding depletes Si availability. The application of soil Si amendments increases Si availability, raises plant tissue SiO2 concentrations and improves resistance to both biotic and abiotic stresses, resulting in increased yields (Ma and Takahashi, 2002). Several other crop species, including sugarcane (Sacharinum sacharinum), turfgrass (Poa spp., Agrostis spp., Zoysia spp.), and banana (Musa spp.) are treated with Si soil amendments to increase yield by counteracting the negative effects of N fertilization while minimizing the use of harmful chemicals (Datnoff et al., 2001; Korndorfer and Lepsch, 2001; Ma et al., 2001; Nanayakkara et al., 2009).
In earlier work on native North American marsh grasses, parallels were drawn with cultivated rice by Lanning and Eleuterius (1981, 1983), who speculated that coastal eutrophication could impose N stress on marsh grasses such as S. alterniflora. In a survey to provide a baseline of Si plant tissue concentrations in coastal areas that were still considered pristine, S. alterniflora was one of the higher Si accumulating species (Lanning and Eleuterius, 1981). Spartina alterniflora tissue concentrations are considered intermediate for grasses (Ma et al., 2001), ranging from 0.26–2.28% SiO2 (Giblin et al., 2021). However, the relationships between tissue concentrations and levels of abiotic stress (Querne et al., 2012) and soil Si availability (de Bakker et al., 1999) remain unclear.
While early Si studies in tidal marshes focused on tissue SiO2 concentrations, more recent studies have emphasized the Si pools and exchanges of DSi and BSi with adjacent waters (Struyf et al., 2006; Struyf and Conley, 2009; Vieillard et al., 2011; Querne et al., 2012; Carey and Fulweiler, 2013). The created marshes at the Paul S. Sarbanes Ecosystem Restoration Project at Poplar Island (hereafter Poplar Island), in Chesapeake Bay (Figure 1), provide an opportunity to examine Si cycling and fluxes in a mesohaline, N-rich created marsh, and to determine the potential benefit of soil Si amendments to improve S. alterniflora fitness under N-enriched conditions. These tidal marshes are being constructed with material dredged from navigation channels in upper-Chesapeake Bay, which has experienced significant ecosystem changes resulting from anthropogenic nutrient enrichment (Kemp et al., 2005), including seasonal changes in Si availability and trophic shifts (Conley and Malone, 1992). The fine-grained substrate is largely of terrigenous origin (Cornwell and Owens, 2011), and is therefore a potentially rich source of Si. Yet it is also highly enriched in N (Cornwell et al., 2020), resulting in very high porewater ammonium (NH4+) concentrations in the created tidal marshes, the N form preferred by S. alterniflora (Mendelssohn, 1979). It has resulted in S. alterniflora that shows distinctive N induced stress responses (Figure 2), including low root:shoot ratios (Staver et al., 2020), high rates of fungal infection and intense grazing pressure (unpublished data), and lodging. These symptoms may contribute to sudden S. alterniflora dieback (Elmer et al., 2012), which has also been observed in these marshes. The site differs from many historical marsh restoration projects in the use of fine-grained, nutrient rich dredged material, in contrast to the sandy substrates with low to moderate nutrient availability typically used in marsh restoration projects (Craft et al., 1999). Similar substrates are increasingly being used, however, in other eutrophic estuaries, e.g., the Gulf Coast of Louisiana (Costa-Pierce and Weinstein, 2002), and are likely to increase with the beneficial use of dredged material for coastal restoration.
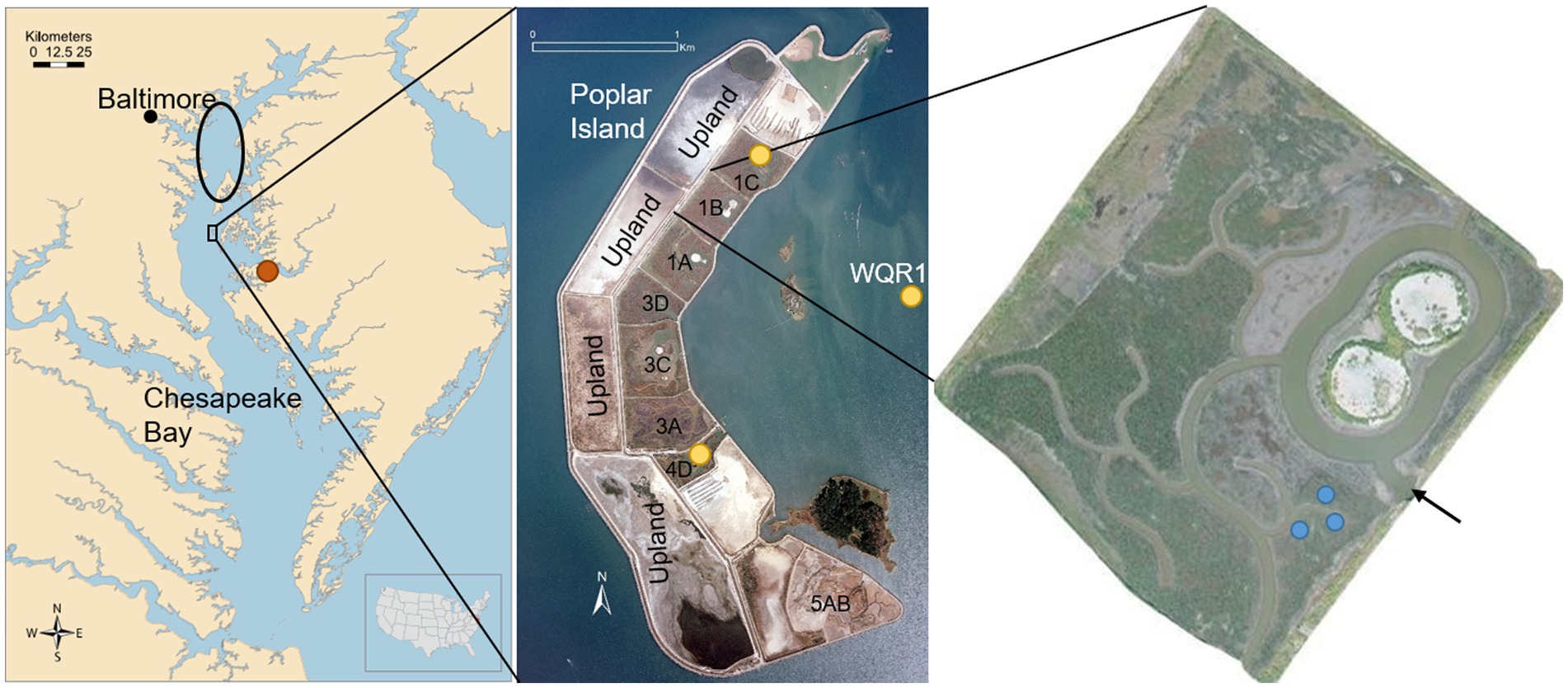
Figure 1. Location of study site in Chesapeake Bay, United States (left), showing the source of dredged material (black oval), the natural reference marsh at Horn Point Laboratory (HPL, orange dot), and Poplar Island (black rectangle). Middle inset shows island configuration, with yellow dots indicating dissolved Si monitoring sites (Cells 4D, 1C and Poplar Harbor, WQR1). Right inset is Cell 1B, the site of tidal flux and soil amendment studies, showing the tidal inlet (black arrow) and soil amendment plots (blue dots). Map credit (left),Tracey Saxby, Kate Boicourt, Integration and Application Network (ian.umces.edu/media-library); photo credit (middle and right), U.S. Army Corps of Engineers.
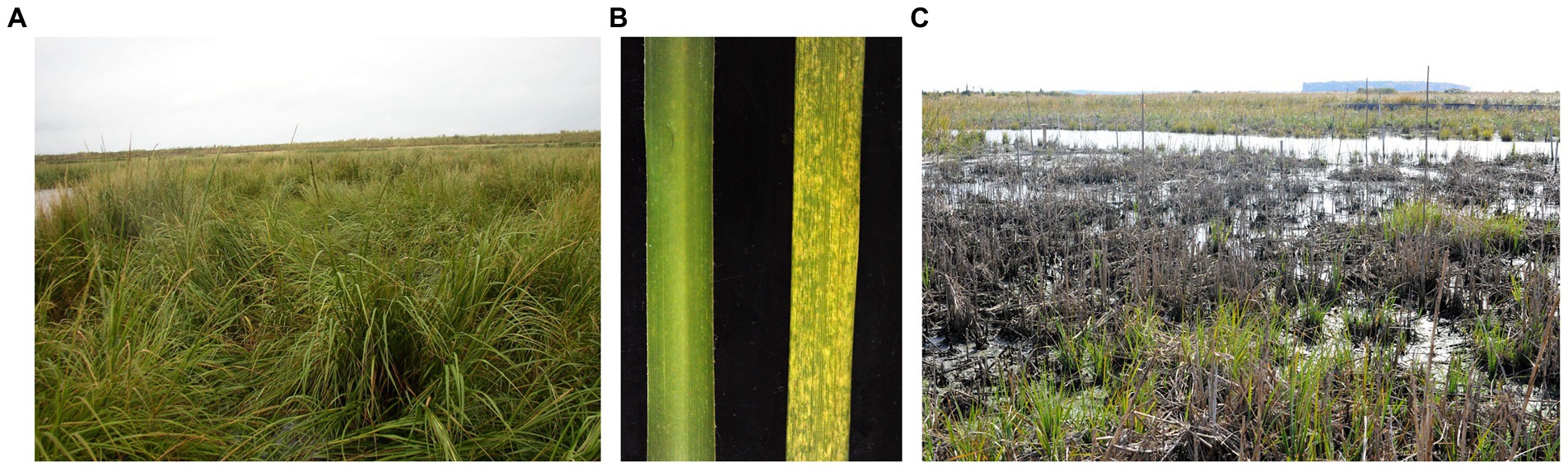
Figure 2. Spartina alterniflora characteristics observed at Poplar Island that may be related to nitrogen enrichment include (A) lodging; (B) increased insect pressure (right blade) compared with natural marsh (left blade); and (C) sudden vegetation dieback.
Marsh export of Si to local waters to sustain diatom growth and contribute to secondary production, including commercially important fisheries, may be more important in a eutrophic system like Chesapeake Bay than in a more pristine coastal system. In addition, the potential for soil Si amendments to improve S. alterniflora plant fitness in this N-enriched environment by reducing the incidence of N-induced symptoms and dieback, and contributing to greater resilience of the marshes to sea-level rise (SLR), has not been examined. We addressed some of these concerns in the present study using (1) data collected as part of the Poplar Island monitoring program to develop a Si mass balance for one newly constructed marsh to examine Si pools and exchanges with the adjacent estuary; and (2) targeted experiments to test the potential for a Si soil amendment to increase tissue Si concentrations and alleviate the observed symptoms of N stress in S. alterniflora at Poplar Island.
2. Methods
2.1. Site description
Poplar Island is located in mid-Chesapeake Bay, 3.2 km northwest of Tilghman in Talbot County, Maryland, United States (Figure 1). The site experiences a mean diurnal tide range of 0.47 m (NOAA Tides and Currents, station ID 8572271), and a mean surface salinity range (1985–2018) of 10.1–12.5 (US EPA CBP 2019). The study was conducted primarily in one marsh subunit (cell) on the site, Cell 1B, a 12.41 ha tidal marsh completed in 2012. The marsh platform was designed as 80% low marsh (LM, 6.92 ha) and 20% high marsh (HM, 2.51 ha), planted with S. alterniflora and S. patens, respectively, and 1.79 ha of tidal creeks. The cell also includes a 1.19 ha upland habitat island, which was excluded from the marsh mass balance estimates. The marsh substrate (to a depth of approximately 2 m) is fine-grained dredged material resulting from maintenance dredging of the navigation channels in upper Chesapeake Bay (Cornwell and Owens, 2011), providing a high fertility substrate for the restored tidal marshes (Cornwell et al., 2020). Each marsh is surrounded by sand dikes extending approximately 3 m above mean sea level, and tidal exchange occurs through side-by-side 1.83 m2 concrete box culverts, simplifying flow estimates and making the site well suited to a tidal flux study. The marsh is connected to adjacent marshes (Cells 1A and 1C; Figure 1) via shallow lateral channels designed to facilitate fish passage, but exchange through these channels is less than 5% of tidal exchange at the inlet (Fleri et al., 2019). Upland drainage into the marsh is limited to the area of the surrounding dikes, and the consolidated dredged material substrate limits transport within the sediment profile primarily to diffusion.
2.2. Silicon mass balance
A Si mass balance developed for the Cell 1B marsh (11.22 ha, excluding the upland habitat island) identified potential inputs as atmospheric deposition, tidal exchange with the estuary, and upward diffusion from the sediment below the root zone. Potential outputs were tidal exchange and burial. Measurements of site-specific atmospheric deposition were beyond the scope of our study, so an estimate based on literature values was used. Upward diffusion of DSi from the sediment below the root zone was not estimated and internal cycling, while important, was not quantified in this study with the exception of remineralization from macrophytic vegetation. All other pools and fluxes were based on Si analysis of samples collected as part of the long-term monitoring program at Poplar Island, or as part of targeted experiments from 2012 to 2014.
The pool of Si in marsh vegetation was estimated from 2014 end-of-season biomass measurements (Staver et al., 2020), combined with mean end-of-season tissue SiO2 concentrations from live S. alterniflora shoots (2.37%) and roots/rhizomes (1.06%) from the control subplots in the Si amendment experiment (described below), and standing dead material collected for a decomposition experiment (2.00%) in 2012–2013 (Supplementary Table S1). Tissue SiO2 concentrations were not determined for S. patens, but Giblin et al. (2021) reported a range of 0.29–2.19% SiO2, similar to that for S. alterniflora, so the same tissue concentrations were used to estimate the high marsh vegetation pool. Annual shoot and root production in Cell 1B were estimated from clipped plots (0.25 m2) and sediment cores (42 cm2 × 20 cm) harvested in October 2014 (Staver et al., 2020, 2021).
Porewater and solid phase soil concentrations were combined to estimate the total sediment Si pool within the root zone (15 cm depth). Porewater samples were obtained using porewater equilibrators (Hesslein, 1976), deployed and retrieved following Cornwell et al. (2020). Dissolved Si concentrations were determined as SiO2 using the molybdate blue methodology (Strickland and Parsons, 1968), modified for a Technicon AutoAnalyzer II (Technicon Industrial Method 186-72 W-Modified), with ascorbic acid as the reductant. The sediment porewater DSi pool was estimated from concentrations measured in the control subplots of the Si amendment experiment described below, and assumed a pore space volume of 50%. A representative value from these profiles of 200 μmol L−1 was used to calculate the root zone DSi pool. Sediment solid phase concentrations were determined as BSi for 18 solid phase samples collected in 2011, prior to planting the marsh, using the base digestion technique (Demaster, 1981) modified by Saccone et al. (2006) followed by silica (SiO2) analysis. The BSi range among those samples was 2.34–3.45% dry weight. The mean value, 2.96% (± 0.07 se, n = 18), and a bulk density of 1.1 g cm−3 (Staver et al., 2021) was used to calculate the root zone BSi pool.
An average value for the Si pool in tidal creeks (68.28 μmol L−1) was estimated by using the mean annual ebb concentrations of DSi and BSi (41.96 and 26.46 μmol L−1, respectively) in the tidal exchange study, combined with the tidal creek area (1.79 ha) and an average depth of 1 m.
The Si pool in edaphic algae was not quantified here, although it is recognized that benthic diatoms are often present on the marsh surface and are likely important in Si cycling within the marsh seasonally (Ragueneau et al., 2006). Staver et al. (2020) reported seasonally high rates of carbon fixation in unvegetated sediment cores, but respiration always exceeded carbon (C) fixation. Thus, while the benthic algal community is active, fixed carbon is rapidly recycled and stocks are low. In our study, although it is recognized that diatoms are a part of the benthic algal community and may be important in Si processing, it is assumed that, like C, the Si pool is comparatively small and recycles rapidly.
Annual tidal fluxes of DSi and BSi were estimated from seasonal measurements made during 2014. In addition, dissolved inorganic N (DIN), soluble reactive phosphorus (SRP), total suspended solids (TSS) and chlorophyll a (Chl a) concentrations were measured to allow examination of correlations with particulates and the stoichiometry of Si and essential plant nutrients N and P. A full description of tidal water velocity measurements and constituent flux calculations can be found in Staver et al. (2020). In brief, seasonal (February, May, July, and November) tidal water velocity measurements were made with a Nortek Aquadopp acoustic Doppler profiler (ADP), mounted on a weighted PVC plate and placed in the center of one culvert, equidistant from the open ends and sidewalls.
Concurrent with the water velocity measurements, an ISCO automatic water sampler was deployed for a 24-h period (two full tidal cycles) in the same culvert at the mouth of Cell 1B. Discreet water samples were collected at hourly intervals from 30 to 40 cm off the bottom of the culvert. The samples were chilled with ice in the ISCO sampler, returned to the lab at the conclusion of the sample period and kept refrigerated during processing. Samples were subsampled for DSi within 24 h, usually immediately upon return from the field (within 2 h). For BSi analysis, 30 ml subsamples were filtered through 0.45 μm polycarbonate filters, which were analyzed according to Saccone et al. (2006). For validation, the DSi concentrations from the tidal flux study were compared with ambient concentrations measured monthly in 2014 at three long term water quality monitoring stations at Poplar Island. Two stations were located in tidal creeks within the interior of Poplar Island marshes Cell 4D (sand substrate, completed 2003) and Cell 1C (fine-grained dredged material, completed 2005), and a third was located just offshore in Poplar Harbor (WQR1; Figure 1).
Burial rates were determined in a litter bag study described in detail in Staver et al. (2020). In brief, litter bags of S. alterniflora aboveground (AG) biomass were deployed in the low marsh in three fine-grained dredged material marshes spanning a range of age, Cells 3D, 1A and 1C (constructed in 2005, 2009 and 2011, respectively), and Cell 4D, the low nutrient sand substrate marsh (constructed in 2003) from February 2012 to December 2013. Decomposition rates were not determined separately for S. patens or for the high marsh; so for the annual burial estimate the low marsh, the S. alterniflora rate was also applied to the high marsh. For this study, litter bag contents were analyzed for BSi as described above (Saccone et al., 2006). Based on the results of this study, which showed minimal dry mass loss during the second year, it was assumed that a steady state in decomposition is reached after 2 years (Staver et al., 2020). This is likely not the case in the high marsh, which constitutes just 20% of the marsh platform, potentially introducing a small error in the marsh estimate. The burial rate was estimated as where AP is the area-weighted mean annual biomass production of the high and low marsh (2,131 g dry weight; Supplementary Table S1); % DM is the mean percent of original dry mass of S. alterniflora litter remaining in litter bags; and % SiO2 is the SiO2 concentration in litter at the conclusion of the decomposition study in the fine-grained dredged material cells (Cells 1A, 1C, 3D).
In a review of global atmospheric Si transport, Tegen and Kohfeld (2006 in The Silicon Cycle) estimate that the mid-Atlantic coast receives between 0.1–1.0 g Si m−2 y−1. For atmospheric deposition, we used the estimate of Anderson and Downing (2006) for Iowa, where agriculture is a dominant land use, similar to much of the region surrounding our study site. Atmospheric deposition averaged 0.61 g Si m−2 y−1 (equivalent to 1.30 g SiO2 m−2 y−1), with highest deposition rates occurring in spring when agricultural activity causes dust to become airborne. While agricultural activity is absent on Poplar Island, it was assumed that dust produced by the use of heavy equipment as part of on-going dredged material management activities is analogous to that produced by agricultural activity, meriting a mid to upper level estimate within the range of Tegen and Kohfeld (2006).
2.3. Silicon amendments
2.3.1. Phytotron experiment
To test Si soil amendment effects on S. alterniflora tissue SiO2 concentrations, a small-scale experiment was conducted in a climate controlled environmental chamber (“phytotron,” Environmental Growth Chambers, Chagrin Falls, OH) at Horn Point Laboratory (HPL), Cambridge, MD. Dredged material was collected from Cell 1B in September 2010 prior to planting or tidal exchange. The material was dry sieved (1 cm mesh) to remove large plant debris and soil aggregates. For comparison of the fine-grained dredged material with sand, which is commonly used in marsh restoration, we included an unamended sand treatment using commercially available construction grade (course) sand from a local source.
Containers made of 30 cm sections of 10 cm ID PVC pipe, covered at one end with 1 mm mesh screen, were filled to a depth of 20 cm with either fine-grained dredged material (2,100 g) or sand (2,865 g). The substrate filled containers were conditioned in filtered (2 μm) ambient Choptank River water at HPL (salinity 6.8, 12 μM SiO2) for approximately 24 h, draining them, and repeating twice. Ten replicate containers per treatment received either no soil amendment (dredged material control and sand control), or a calcium meta-silicate soil amendment (Cal-Sil Corporation, Columbia, TN) which was mixed into the top 10 cm of the substrate in each container by hand, at rates to simulate 1, 2, 4, and 8 Mg ha−1. Porewater samplers (Rhizon MOM 10 cm model 19.21.21, Rhizosphere Research Products) were inserted into the top of the soil in 5 of the 10 replicates of each treatment. One 2.5 cm nursery grown S. alterniflora stock plant grown from wild collected seed (Environmental Concern, St. Michaels, MD) was placed in each container after rinsing the nursery substrate from the root mass. Each container was placed in a 19 l bucket, filled to 15 cm depth with filtered ambient Choptank River water. The experiment was conducted under a 15-h light, 26°C, 60% humidity/9-h dark, 22°C, 80% humidity cycle for 93 days. Soil pH (measured at 5 cm depth) was monitored approximately every 2–3 weeks with a Corning model 315 pH meter equipped with a Sensoret model S175CD spear tip electrode.
At the conclusion of the experiment (93 days), stem height was measured, flowering was noted, and AG and belowground (BG) biomass were harvested. AG biomass was rinsed in deionized water and dried to constant weight in a forced draft oven (Grieve model 343). BG biomass was rinsed free of sediment over a 1 mm mesh sieve and similarly dried and weighed. Dried plant samples were ground in a Wiley Mill (1 mm mesh screen) prior to analysis for BSi using the modified wet alkaline digestion technique (Saccone et al., 2006).
2.3.2. Field experiment
In 2012, triplicate experimental plots were established parallel to a tidal creek in the low marsh zone in Cell 1B (Figure 1). Each plot was divided into three 5 × 5 meter subplots (split-plot experimental design), which were assigned to receive either a soil Si amendment, broadcast on the sediment surface at a rate of 6 Mg ha−1 and roto-tilled into the top 5 cm using a small rototiller attachment on a Stihl Model 115R string trimmer; tillage without the soil amendment (procedural control); or neither amendment nor tillage (control). The soil amendment was a granular form of calcium meta-silicate (Cal-Sil Corporation, Columbia, TN).
Porewater DSi concentration profiles were measured using 45 cm PVC sediment porewater equilibrators (Hesslein, 1976) on July 24, 2012. Due to limited availability, equilibrators were deployed only in control and amended subplots, but not in the tillage control plots. Porewater DSi measurements were not made in the experimental plots after 2012, but measurements elsewhere in Cell 1B in 2013 (n = 3) and 2014 (n = 5) were made to assess spatial variation and change over time. DSi concentrations were determined as silicate using the molybdate blue methodology as described above. Although solid phase soil analysis was not conducted on samples from the experimental plots, BSi concentrations from 18 sites in Cell 1B collected in fall 2011 were assumed representative of the substrate in the experimental plots.
Vegetation in the experimental plots was sampled monthly for S. alterniflora tissue SiO2 concentrations. Three representative whole stems were collected from each subplot and analyzed for SiO2 as described above. For comparison purposes, samples were also collected from the Poplar Island marsh Cell 4D (sandy substrate) and a natural reference marsh (HPL) near Horn Point Laboratory (38°35′32.25″N, 76°7′49.90″W), approximately 29 km from Poplar Island. The subplots were inspected monthly during the growing season (April–September) through 2012–2013 for lodging and muskrat (Ondatra zibethicus) grazing, and sampled once toward the end of the 2012 and 2013 growing seasons for fungal infection. Fungal infection rates were determined for fresh S. alterniflora stems in the amended and control (not the tillage control) plots in 2012 (n = 20 stem sections per treatment plot) and 2013 (n = 50 sections per plot). Stem sections were incubated on Peptone-PCNB agar (selective for Fusarium species; Elmer and Marra, 2011). Five to 7 days later stem pieces with fungal colonies were counted. Single spores from colonies were sub-cultured on Carnation Leaf agar and held for 10 days at 22°C on laboratory benches. Cultures were examined under 100 × and 400 × magnification for identification based on fungal morphology (Elmer and Marra, 2011).
2.4. Statistical analyses
Pearson Product Moment Correlations were performed to determine relationships between tidal flux components. For the phytotron experiment, statistical analyses included Kruskal–Wallis one-way analysis of variance on ranks (ANOVA) to test for significant effects of a soil amendment on shoot growth and root growth. One-way ANOVA was performed on tissue SiO2 concentrations, followed by pairwise comparisons based on rank sums (Tukey test). For the field experiment, significant differences in soil porewater DSi profiles between Control and Si amended plots were tested with a split-plot ANOVA, with plot, treatment, and depth as variables. Differences between amended and control treatments in tissue SiO2 concentrations throughout the growing season in each year (2012 and 2013) were tested with a split-plot ANOVA, with plot, treatment and date as variables. Statistical analyses for the phytotron, tidal flux and fungal (field experiment) data were performed in SigmaPlot 13.0 at p = 0.05; analyses for the soil porewater and S. alterniflora tissue SiO2 concentrations (field experiment) were performed in R using the Agricolae package for split-plot experimental designs.
3. Results
3.1. Silicon mass balance
3.1.1. Pools
Sediment BSi is overwhelmingly the largest Si pool in the marsh, followed by the vegetation pool (Table 1). The sediment DSi pool is comparatively small, on the same scale as the water column (tidal creek) pool, which represents a combination of DSi and BSi in the tidal creeks.
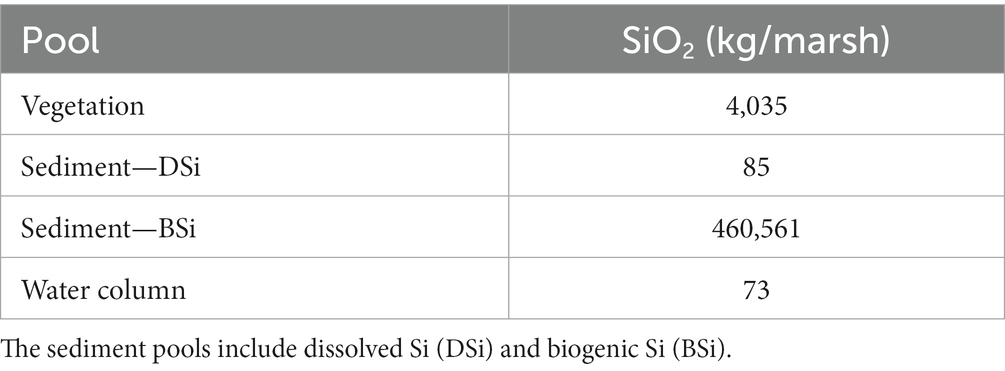
Table 1. Estimated Si pools for the Cell 1B tidal marsh (11.22 ha) at Poplar Island, Chesapeake Bay, including macrophyte vegetation, sediment root zone (top 15 cm), and tidal creek water volume in 2014.
3.1.2. Burial
There was a net decrease in dry mass (Figure 3A) in the three marshes with fine-grained substrate, with the most rapid losses occurring in the first 6 months followed by little change in the subsequent 16 months. Initial BSi litter concentrations varied widely (Figure 3B), with the older Poplar Island marshes, Cells 4D (sand substrate, constructed 2003) and 3D (constructed 2005), having much lower concentrations than the two younger marshes, Cells 1A and 1C during the growing season. Tissue BSi concentrations decreased rapidly in litter with higher initial BSi concentrations (Cells 1A and 1C), but there was little change in litter with lower initial concentrations (Cells 3D and 4D). Using the area-weighted mean biomass (2,131 g m−2), mean remaining dry mass (24%) and SiO2 concentration (0.51%) for the dredged material marshes, annual burial was estimated at 246 kg SiO2 for the marsh platform (9.43 ha).
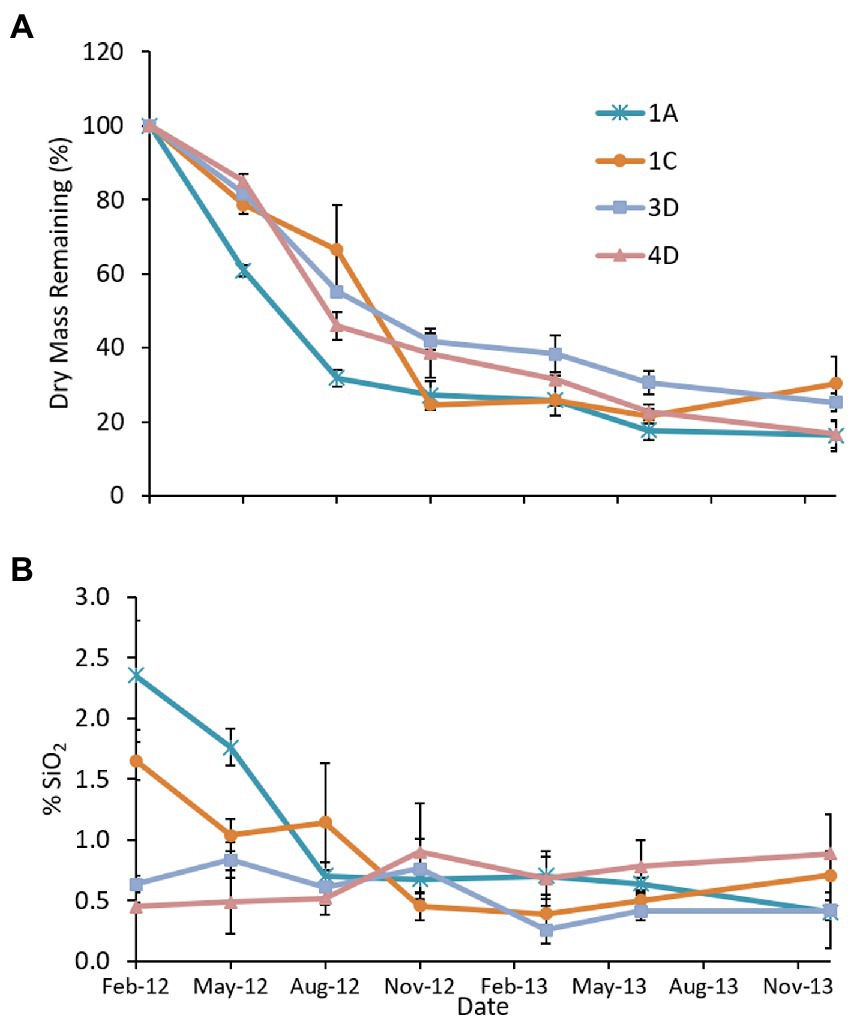
Figure 3. Results of the 2012–2013 Spartina alterniflora litter decomposition study showing (A) mean (n = 3 litter bags) percent remaining dry weight, and (B) litter SiO2 concentration in four marshes at Poplar Island. Cells 3D, 1A and 1C are dredged material marshes; in Cell 4D the substrate is sand. Error bars represent standard error.
3.1.3. Tidal exchange
Ebb concentrations of DSi, BSi and TSS exceeded flood concentrations during all sampling periods (Table 2; Supplementary Table S2). Concentrations of DIN, SRP, and Chl a were more variable throughout the year, with DIN ebb exceeding flood in the colder months, but the reverse in the warmer months; SRP ebb exceeding flood except in May; and Chl a ebb exceeding flood except in May. The highest concentrations of BSi, DSi, TSS and Chl a occurred in July, when nutrient concentrations were lowest.
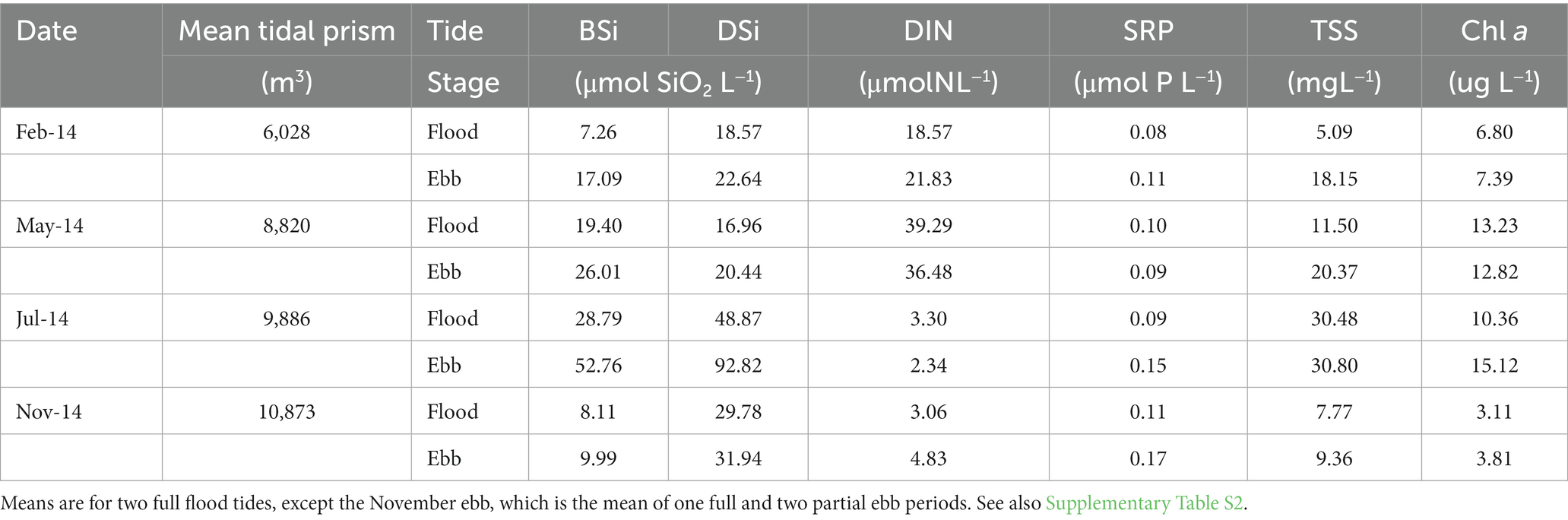
Table 2. Mean (n = 2 tidal cycles) tidal prism and flood and ebb tide concentrations of water column dissolved Si (DSi), biogenic Si (BSi), dissolved inorganic nitrogen (DIN) and soluble reactive phosphorus (SRP), total suspended solids (TSS) and chlorophyll a (Chl a) at the Cell 1B inlet, Poplar Island, Chesapeake Bay in 2014.
Estimated net fluxes of both DSi and BSi were negative (export) during all monitoring periods, with the largest net export of both constituents in July (Table 3; Supplementary Table S3). Extrapolating these values to estimate exchange on an annual basis resulted in net export of 3,980 and 5,468 kg y−1 of BSi and DSi, respectively, as SiO2 (Table 4). Biogenic Si dominated exports during the first two quarters of the year, while DSi dominated exports during the latter half of the year.
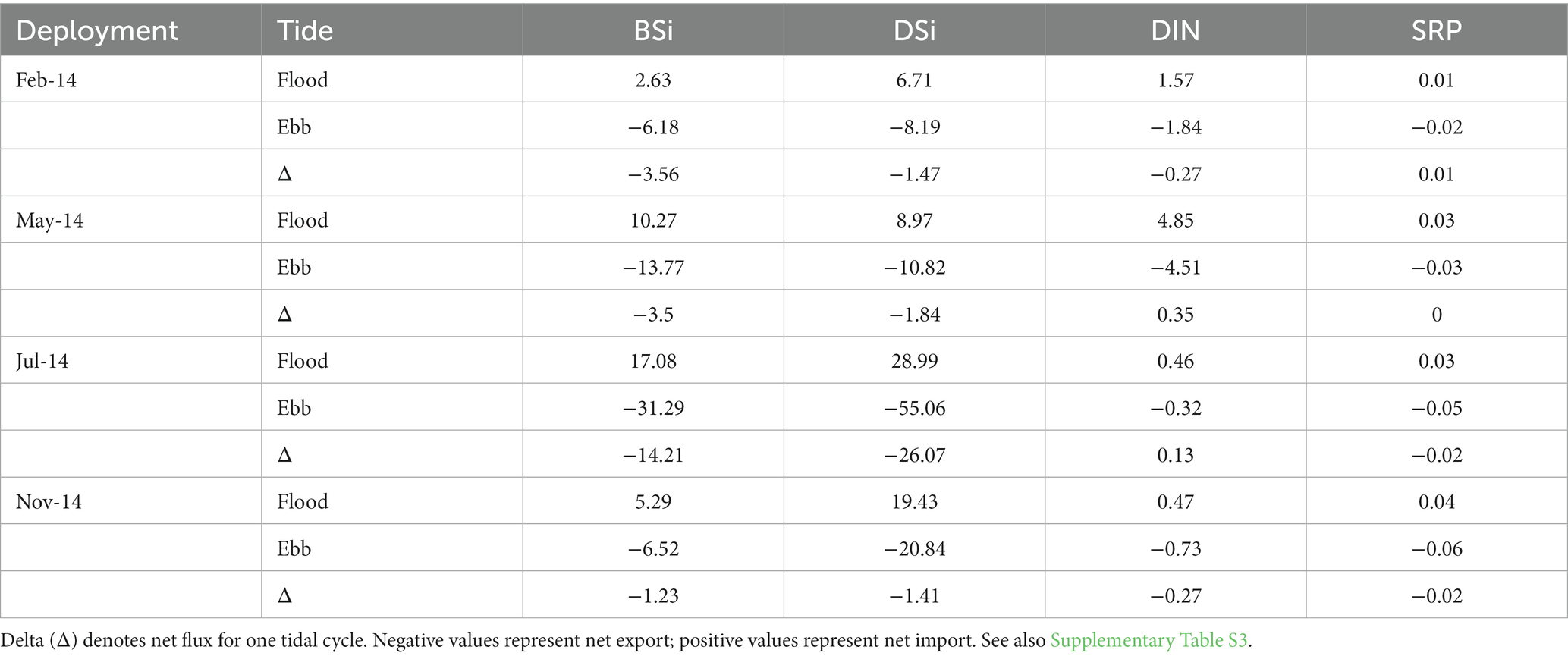
Table 3. Estimated mean (n = 2 tidal cycles) tidal fluxes of dissolved Si (DSi) and biogenic Si (BSi; kg SiO2), dissolved inorganic nitrogen (DIN, kg N) and soluble reactive phosphorus (SRP, kg P) during flood and ebb tides in 2014 at the Cell 1B tidal inlet.
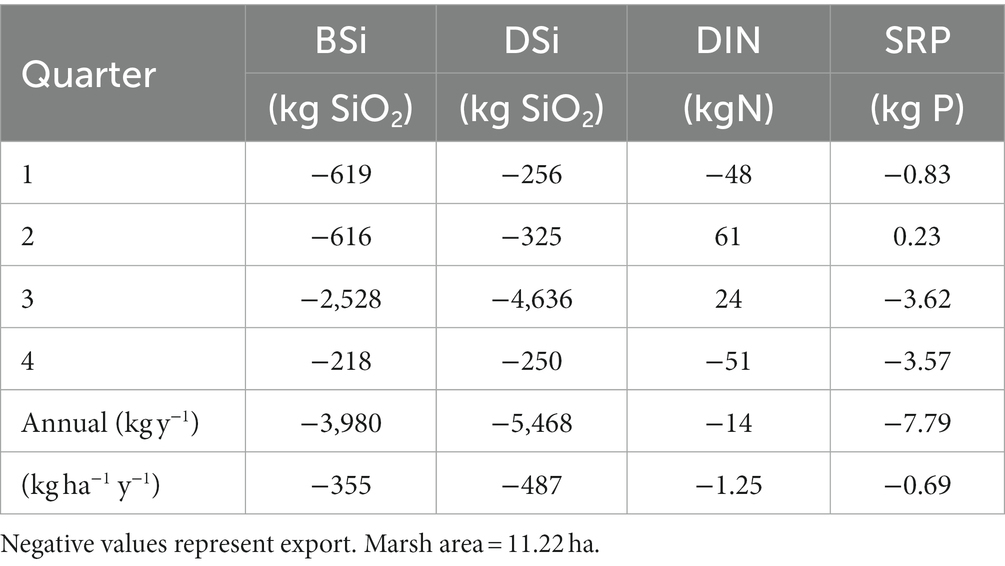
Table 4. Estimates of net quarterly and annual fluxes of dissolved Si (DSi) and biogenic Si (BSi) in kg SiO2, total dissolved nitrogen (TDN, kg N) and soluble reactive phosphorus (SRP, kg P) at the Cell 1B inlet, Poplar Island, Chesapeake Bay, in 2014.
There were strong correlations between mean BSi and TSS (correlation coefficient = 0.88, p = 0.0036; Figure 4A), and between mean BSi and Chl a (correlation coefficient = 0.82, p = 0.012; Figure 4B). The relationship between mean DSi and Chl a was much weaker (correlation coefficient = 0.43, p = 0.285; Figure 4B).
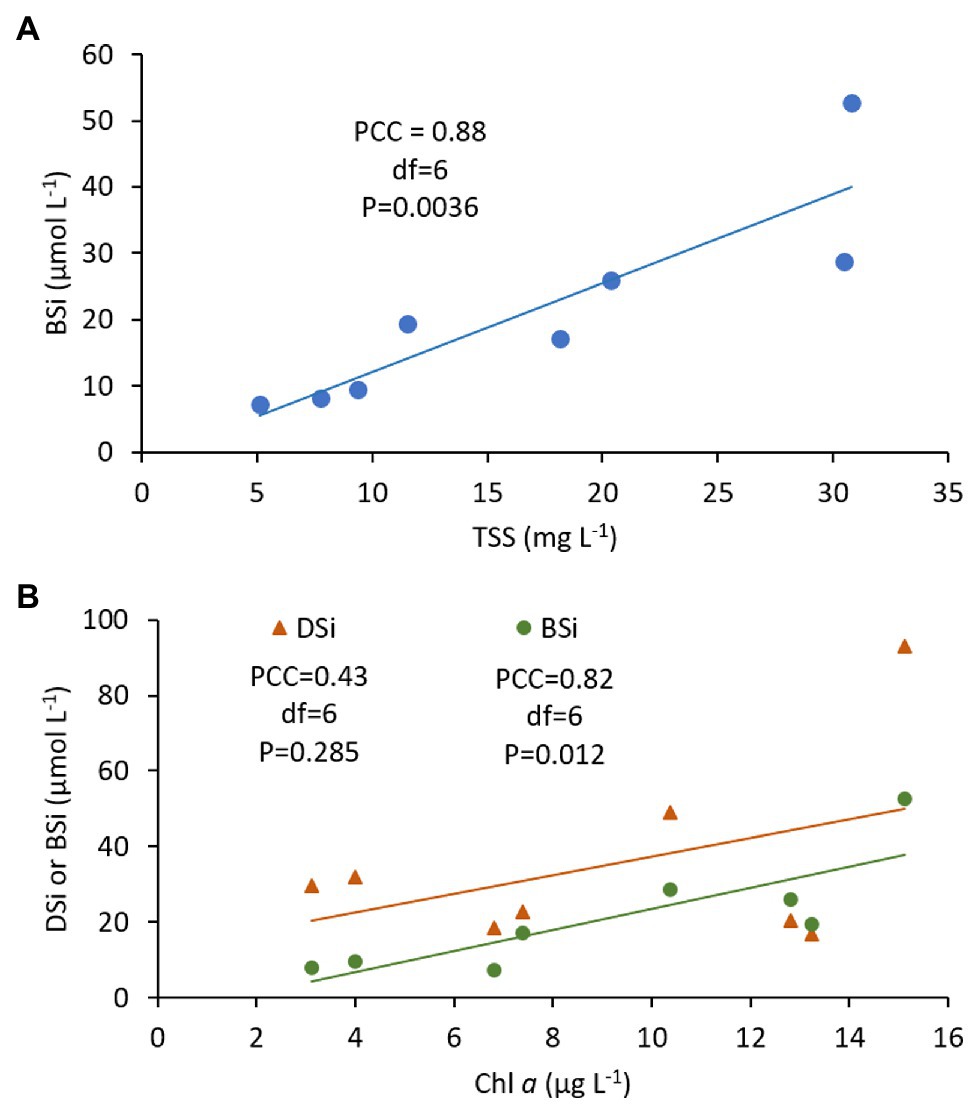
Figure 4. Pearson’s correlation analysis of volume-weighted mean concentrations of (A) biogenic Si (BSi) with total suspended solids (TSS) and, (B) dissolved Si (DSi) and biogenic Si (BSi) with chlorophyll a (Chl a), showing Pearson’s correlation coefficient (PCC), degrees of freedom (df), p value and trend line. Data are from the 2014 tidal flux study at the Cell 1B inlet, Poplar Island, Chesapeake Bay (Table 2).
Dissolved SiO2 concentrations in samples collected in the tidal creeks in Cells 4D and 1C, and in Poplar Harbor varied by a factor of four throughout 2014 (Figure 5). Minimum concentrations were observed in April at all stations, increased during the warmer months and declined after September. Concentrations were consistently highest in Cell 1C, the dredged material marsh, where they were at least double the other stations on all but one sampling date and similar to concentrations measured during the tidal flux study in Cell 1B. Concentrations at the inlet to Cell 4D, the sand marsh, were close to ambient Poplar Harbor concentrations and showed a similar pattern.
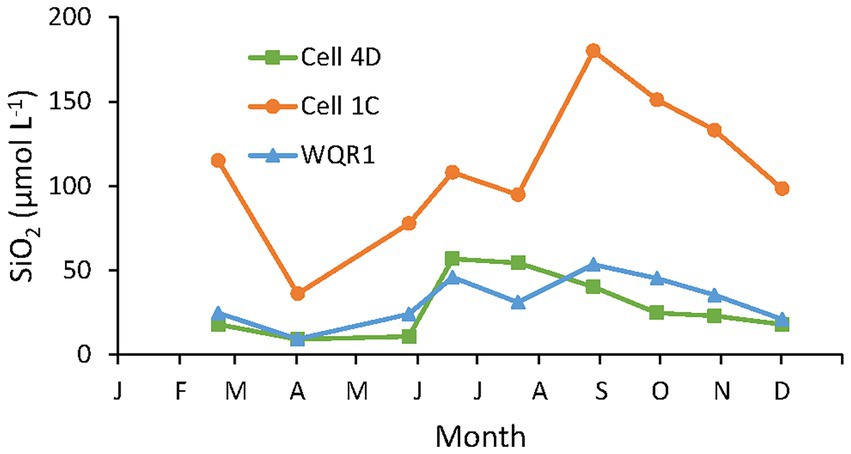
Figure 5. Ambient 2014 water column concentrations of DSi in Cell 4D (sand substrate), Cell 1C (dredged material substrate) tidal creeks and a subtidal site in Poplar Harbor (WQR1; Figure 1).
The mass balance for the Cell 1B marsh indicates that this young marsh is a net exporter of Si as both DSi and BSi on an annual basis (Figure 6). The fine-grained dredged material substrate is the primary source of Si, with vegetation mining about half of the exported Si from the root zone.
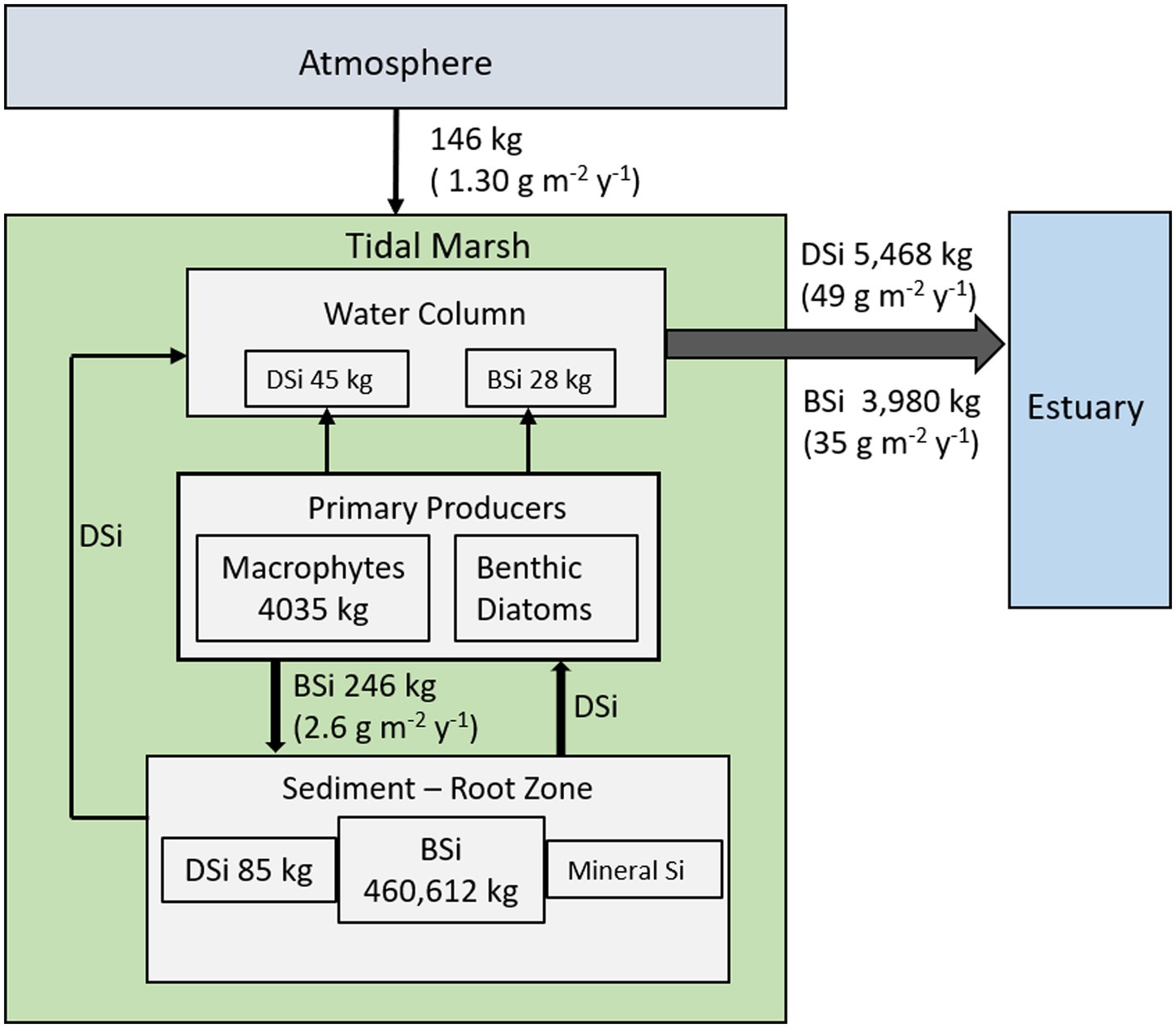
Figure 6. Conceptual model of annual SiO2 mass balance for the Cell 1B tidal marsh at Poplar Island. All fluxes for the marsh are expressed as SiO2 (annual, kg SiO2 y−1; area-based fluxes in parentheses, g SiO2 m−2 y−1).
3.2. Silicon amendment experiments
3.2.1. Phytotron experiment
In the 2012 laboratory experiment, porewater DSi concentrations were similar at all levels of Si amendment (Figure 7A), but somewhat lower on the beginning and ending sampling dates in the sand treatment (no amendment) due to the low solubility of mineral Si in sand. Soil pH increased with application rate, due to the alkalinity introduced by the calcium meta-silicate amendment (Lacroix et al., 2014), and the differences persisted throughout the duration of the experiment (Figure 7B). Shoot biomass at the termination of the experiment was similar across all dredged material treatments, while biomass in the sand treatment was only 5.5% of the mean of dredged material treatments (Table 5). Root biomass was variable across the dredged material treatments, but, again, the sand treatment had only 5.6% of the root biomass of dredged material treatments (Table 5). Analysis (Supplementary Table S4) showed significant differences between treatments in both AG and BG biomass (AG biomass, p < 0.001; BG biomass p < 0.001). There were no significant differences in tissue SiO2 concentrations at the termination of the experiment across all treatments (p = 0.084).
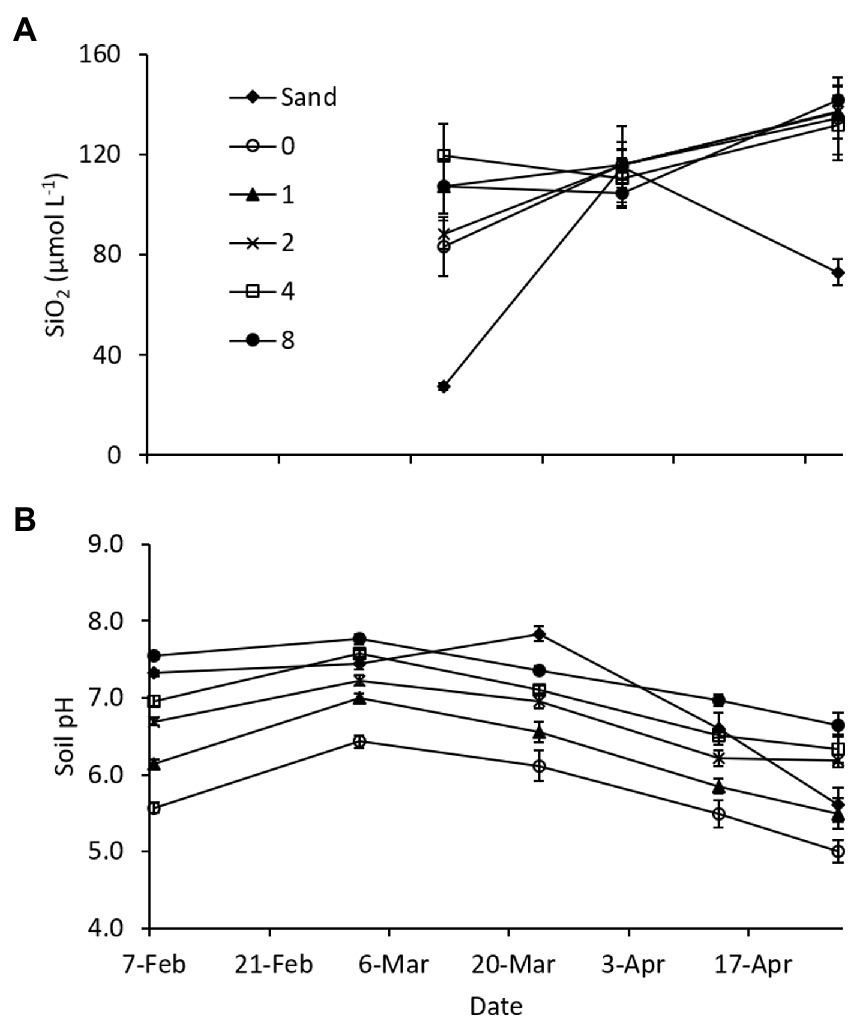
Figure 7. Mean (A) soil porewater dissolved Si (DSi) concentrations (n = 5), and (B) soil pH (n = 10) in the 2012 Si amendment laboratory (phytotron) experiment. Sand and 0 treatments received no soil amendment; other treatments received a calcium meta-silicate amendment at the rates indicated (Mg ha−1). Error bars represent standard error.
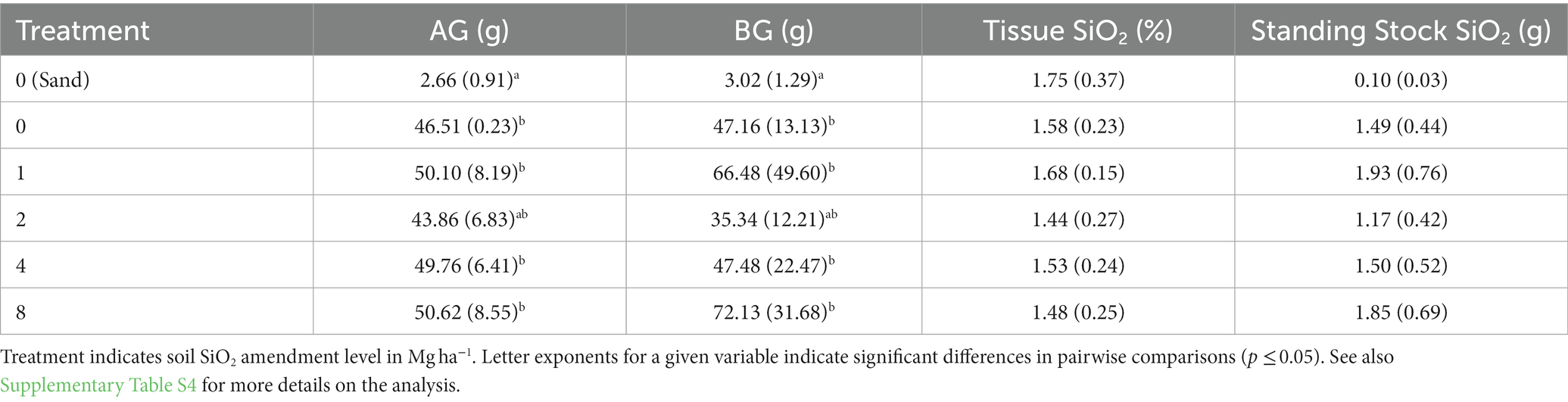
Table 5. Results at the conclusion of the 2012 laboratory (phytotron) experiment showing mean (± standard deviation, n = 10 containers) aboveground (AG) and belowground (BG) Spartina alterniflora biomass (g per 2.4 l container), S. alterniflora tissue SiO2 concentration (%) and SiO2 standing stock (g).
3.2.2. Field experiment
Background soil BSi concentrations in Cell 1B at the start of the Si amendment field trial ranged from 2.34–3.45% SiO2, somewhat lower than observations in mesohaline in situ Chesapeake Bay channel sediments (3.0–7.0%; Cornwell et al., 1996). In November 2012, at the end of the first growing season, mean soil pore water DSi concentrations ranged from 169 to 788 μmol L−1 and 302–1,035 μmol L−1 in the control and Si amended plots, respectively (Figure 8A). There were significant differences with Plot*Depth (p < 0.001) and Treatment (p = 0.003), but not by Treatment*Depth (p = 0.962; Supplementary Table S5). Concentrations in the same range were observed in Cell 1B monitoring locations outside the experimental plots in 2013 and 2014 (Figure 8B).
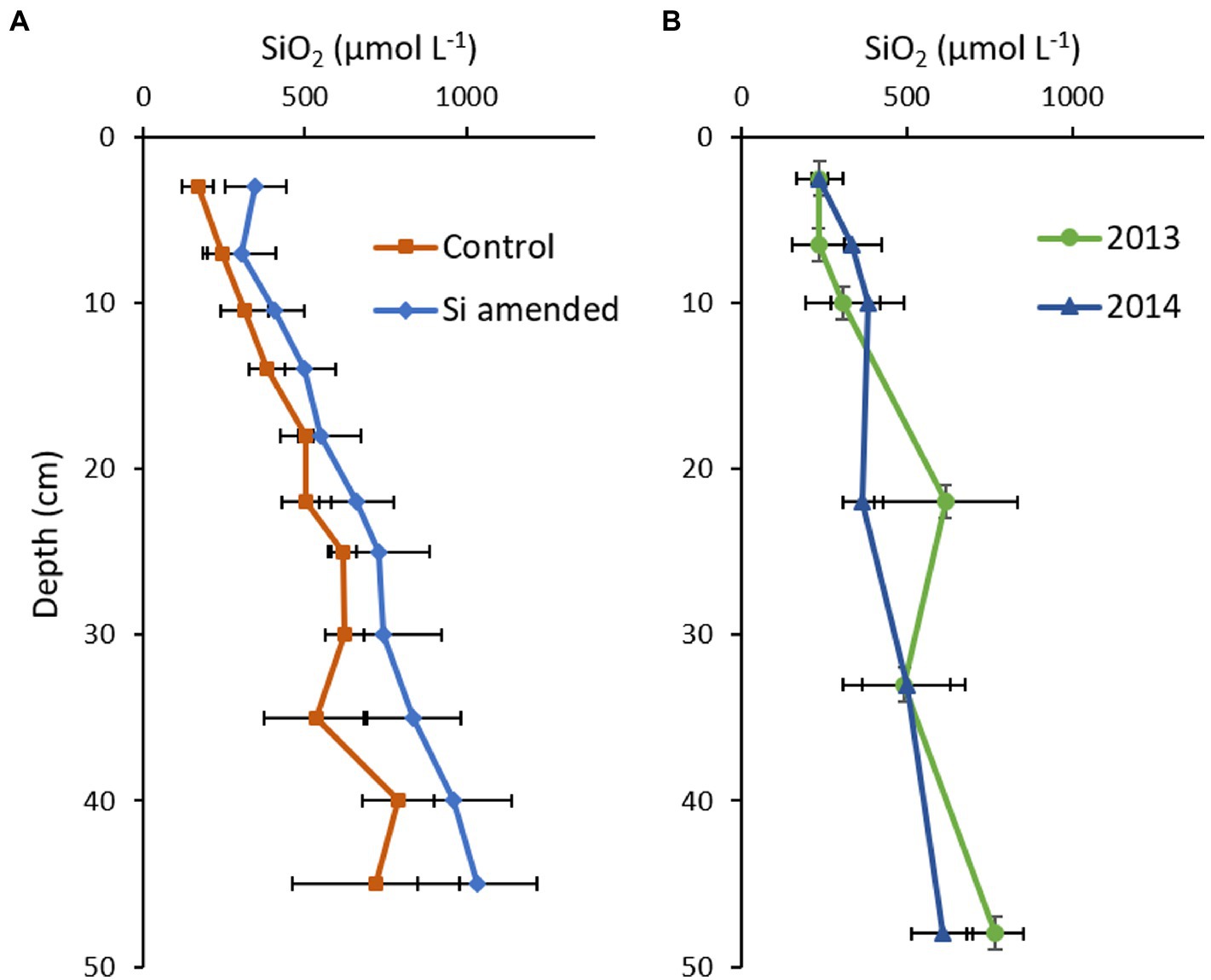
Figure 8. Mean porewater dissolved Si (DSi) concentration depth profiles measured in (A) 2012 in the Cell 1B experimental Si amendment (orange, n = 3) and unamended (blue, n = 3) plots; and (B) elsewhere in Cell 1B in 2013 (green, n = 3) and 2014 (blue, n = 5). Error bars represent standard deviation.
Plant tissue SiO2 concentrations in the experimental Si amendment field plots at Poplar Island were similar at the beginning of the growing season in both 2012 and 2013, but diverged as the season progressed each year, with an overall increase through the growing season (Figure 9). Differences between treatments were modest. In 2012, there were significant differences within plots over time (Plot*Date, p < 0.001) and due to Treatment (p = 0.002), but not Treatment*Date (p = 0.671). Similar results were obtained in 2013, with significant differences with Time (Plot*Date, p < 0.001), Treatment (p < 0.001), and Treatment*Date (p = 0.00147; Supplementary Table S6).
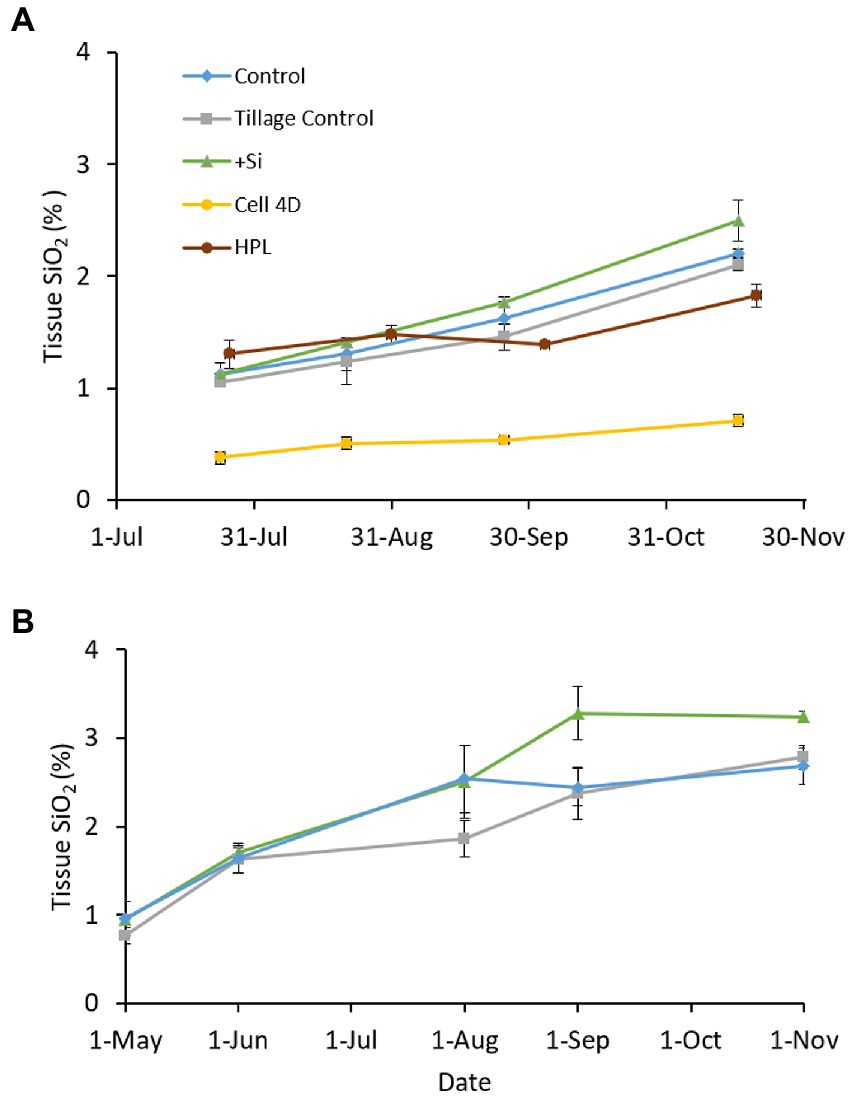
Figure 9. Mean (± standard error) Spartina alterniflora tissue SiO2 concentrations in the Si amended and control plots through (A) 2012 and (B) 2013. Data from the reference marshes, Horn Point Laboratory (HPL) and Cell 4D, were excluded from statistical analyses.
In both years, mean percent fungal incidence was higher in the control plots than in the Si amended plots (Supplementary Table S7), but the differences were not significant in either year (Supplementary Table S8; 2012, p = 0.264; 2013, p = 0.335). In 2012, however, there were five different Fusarium species found in the samples from control plots (F. palustre, F. proliferatum, F. solani, F. incarnatum, and F. oxysporum), while only two were found in samples from the Si amended plots (F. oxysporum and F. incarnatum; Supplementary Table S7). Notably, different Fusarium species were dominant in the treated and control plots in both years. Fusarium palustre, a species unique to S. alterniflora (Elmer and Marra, 2009) that has been associated with S. alterniflora sudden vegetation dieback (Elmer and Marra, 2011), was found only in control plots in both years and was dominant in 2 of the 3 control plots in 2013, while F. proliferatum dominated amended plots. Both species have been reported from natural S. alterniflora stands on the east coast of the United States and are considered pathogenic only when plants are stressed by other factors, e.g., drought (Elmer and Marra, 2011).
No signs of muskrat grazing or lodging were observed in any of the experimental plots in either year. An infestation of stem boring insect larvae in 2013, which appeared to kill the meristem in a high percentage of stems, may have reduced the propensity for lodging by limiting stem height. These stem borers persisted through 2014, based on an informal summer survey.
4. Discussion
4.1. Silicon mass balance
Silicon cycling in the created tidal marsh (Cell 1B, Poplar Island) in Chesapeake Bay is notable for the large net annual tidal export of combined dissolved and biogenic SiO2. The export of DSi is consistent with Si fluxes reported for other marshes (Struyf et al., 2006; Jacobs et al., 2008; Vieillard et al., 2011). Unlike other marshes, which import BSi with TSS, especially in summer (Struyf et al., 2007), there was a constant export of BSi from Cell 1B. The estimated annual uptake of DSi by macrophytes (4,034 kg) is of similar magnitude as the estimated net annual export of DSi from the marsh (5,468 kg), and is about half the total tidal export (DSi + BSi, 9,448 kg). Combined with the estimate that approximately 6% of the SiO2 mobilized from the sediment through macrophyte uptake is re-buried with organic matter, the mass balance suggests there is a significant flux of DSi out of the sediment in addition to macrophyte uptake.
The dredged material substrate is the primary source of N for macrophytic growth and the driver of net N export in the Cell 1B marsh (Staver et al., 2021). Similarly, it appears to be the primary source of SiO2 for uptake by macrophytic vegetation and both benthic and pelagic diatoms, as well as direct fluxes to the water column during flood tides. While direct measurements of sediment DSi fluxes were not made as part of this study, sediment porewater profiles show depletion in the root zone (≤ 15 cm depth) resulting from plant uptake and, likely, direct fluxes when the marsh is flooded, since porewater DSi concentrations greatly exceed those in overlying water. Concentrations below the root zone remain among the highest reported (Müller et al., 2013), 200–1,000 μmol L−1 in 2012, exceeding concentrations reported for a natural marsh on Plum Island, MA, 0–300 μmol L−1 (Vieillard et al., 2011; Carey and Fulweiler, 2013, 2014a), and a Delaware marsh, 100–900 μmol L−1 (Scudlark and Church, 1989). The high concentrations of both BSi and DSi in the dredged sediment likely result from the accumulation of fine-grained particles of terrestrial origin combined with diatomaceous BSi in the deep navigation channels. As the dredged material ages, concentrations will likely decline due to export. There was little change over the course of this study (Figure 9), however, suggesting the large volume of dredged material beneath the root zone will provide a long-term source.
Potential areas of uncertainty in the Si mass balance include heterogeneity in macrophyte biomass production (Wehrhan et al., 2021), and the estimate of tidal fluxes. Biomass is monitored annually in the Poplar Island marshes, and the 2014 data used here is consistent with average production in these marshes. In some years, however, sudden vegetation dieback (Elmer et al., 2012) dramatically reduces S. alterniflora production and likely affects Si uptake, recycling and exchange with the estuary. In addition to the influence of macrophyte production, estimates of tidal exchange are constrained by limited sampling. Hourly water sample collection over two tidal cycles was used to estimate seasonal fluxes, and storm events were not represented in our study. The ADPs were deployed for a minimum of 1 week in each season, to ensure the tidal prisms during water collection were representative of that period, but extrapolating to a full three-month season undoubtedly introduces some error. This could be constrained in future efforts through more frequent sampling, e.g., monthly, combined with storm sampling to characterize fluxes associated with extraordinary tides. A smaller error is likely due to an overestimate of burial, for which low marsh decomposition rates were applied to the high marsh where decomposition can be slower due to differences in vegetation and less frequent tidal inundation (Frasco and Good, 1982). Since only 20% of the marsh platform is high marsh, a more accurate burial estimate would have a minimal impact on the mass balance.
In our study, the peak export of DSi and BSi occurred in July when soil temperatures and sediment flux rates are reported to be highest (Scudlark and Church, 1989). However, SiO2 loss from S. alterniflora detritus was most rapid during the spring (Figure 3) prior to peak DSi and BSi export from the marsh (Table 3). The delay may be due to assimilation by benthic diatoms in spring, followed by release as diatoms are shaded by macrophytes or replaced by other algal species during the warmer months.
In Danish marshes, the summer export of DSi is considered a valuable DSi source to coastal waters when ambient DSi concentrations are at a minimum (Struyf et al., 2006; Jacobs et al., 2008). In mid-Chesapeake Bay, the annual minimum occurs in early summer (Conley and Malone, 1992), prior to the peak export by the Poplar Island marshes (Table 3 and Figure 5). Rather, it coincides with a summer peak in ambient DSi resulting from remineralization of diatom BSi following the spring diatom bloom (Conley and Malone, 1992). In the tidal flux study presented here, the positive relationships between BSi and TSS, and BSi and Chl a (Figure 4) suggest that much of the BSi export from the marsh is in the form of pelagic diatoms. If this is the case, it suggests that diatoms remain abundant in the marsh creeks despite the high inorganic N concentrations due to the availability of DSi. The Si:N ratio in diatoms is ~ 1 (Sterner and Elser, 2002), while the molar Si:N ratio of exports from Cell 1B is approximately 6.6 for dissolved forms of Si and N, suggesting that overall the marsh is exporting a surplus of Si relative to N and diatom production in marsh channels is not Si limited. Together, these observations support the hypothesis that DSi depletion in eutrophic estuaries is responsible for the shift in species composition toward non-diatomaceous species, including those which produce harmful algal blooms (Giblin et al., 2021), and may have implications for higher trophic levels. A characterization of temporal changes in algal species composition in the Poplar Island marsh creeks could resolve this question.
The retention of macrophyte detritus within Cell 1B due to the limited tidal exchange appears to contribute to robust rates of vertical accretion (Staver et al., 2020), although it is not clear what effect it may have on Si burial rates. Struyf et al. (2007) report that young Dutch marshes have higher deposition and burial rates than mature marshes, with rates of both declining after the marshes reach an elevation in equilibrium with sea level. The resulting recycling efficiency ranges from 15% in young marshes (85% buried) to 60% in mature marshes (40% buried). The young marshes at Poplar Island also have higher rates of vertical accretion and may follow a similar trajectory. However, upward diffusion of sediment N and DSi from below the root zone likely persists for years to decades and may result in persistently higher recycling rates and lower burial rates for a longer period than in the Dutch marshes.
Although the marshes studied by Struyf et al. (2007) were formed on former agricultural land, they did not consider the effects of soil N on Si recycling or burial. This was examined in a study of two Rhode Island marshes exposed to different levels of N (Carey and Fulweiler, 2013). Nitrogen enrichment was associated with higher sediment BSi and DSi concentrations, as well as higher plant tissue concentrations. Increased Si availability due to high TSS deposition and high rates of recycling induced by N enrichment were thought responsible for the elevated sediment and plant concentrations, although there was likely also a difference in Si inputs. The results presented here suggest that at the ecosystem scale, increased primary production resulting from N enrichment enhances sediment SiO2 mobilization, remineralization and export. Thus, through continuous export of DSi these marshes may help mitigate the negative effects of eutrophication on spring and summer diatom populations in the surrounding estuary, potentially enhancing their role in support of fisheries (Baker et al., 2020).
4.2. Silicon amendments
At the plant scale, there has long been speculation that S. alterniflora could develop a growth induced Si deficiency in response to N enrichment (Lanning and Eleuterius, 1981, 1983), and several studies have examined the interactions of marsh macrophytes, stress (biotic and abiotic) and Si availability. Results of amendment studies have been mixed, with some species showing enhanced tissue concentrations in response to Si enrichment (Schaller et al., 2012; Mateos-Naranjo et al., 2013), while others did not (de Bakker et al., 1999). The role of tissue Si concentrations in regulating S. alterniflora growth and resistance to pathogens, grazing and abiotic stressors also remains unclear (Querne et al., 2012; Bazzano and Elmer, 2017).
In our study, it was hypothesized that N availability in the Poplar Island marshes, combined with the numerous indications of N stress in S. alterniflora, made this an ideal site to examine whether growth induced Si deficiency can be mitigated by soil Si amendments, as in some crop species. The results of our small-scale phytotron experiments growing S. alterniflora in dredged material enriched with calcium meta-silicate were, however, inconclusive. While Si amendments had a pronounced effect on soil pH, there was not a large difference in plant growth or tissue Si concentrations with increasing Si amendment. Plants grown in sand were stunted compared with those grown in fine-grained dredged material, likely due to N deficiency, but they contained similar tissue Si concentrations (Figure 9). The experiment was only 12 weeks long, and since DSi is taken up passively by S. alterniflora, this experiment may not have reflected the results that would be obtained in a longer growing season. However, these data highlight the effect of N enrichment on soil Si mobilization by macrophytic vegetation through increased biomass production. This likely contributes to an increase in DSi availability within the marsh and to the large net total SiO2 export from the Cell 1B marsh.
In the two-year field experiment, tissue concentrations were similar in Si-amended and control plots early in each growing season, but differences developed and increased as the season progressed. In contrast to the phytotron experiment, it appeared that soil SiO2 amendment can help mitigate root zone depletion and produce a modest effect on tissue concentrations. This effect persisted through year two following soil amendment. Thus, it appears that in an N-rich environment, SiO2 uptake potential can exceed supply even where availability is high.
Also noteworthy in the field experiment was that tissue Si concentrations of S. alterniflora plants from Cell 4D, which has a sand (low nutrient) substrate, were substantially lower than plants from the dredged material plots, while plants from the HPL reference marsh, with higher soil porewater NH4+ concentrations (unpublished) were closer to the dredged material marsh controls. These results align with the results of Carey and Fulweiler (2013) that plants exposed to higher N availability contain higher tissue Si concentrations. What is not clear is whether this is due to differences in N availability and plant growth, or differences in Si availability or uptake. Nitrogen enrichment delays senescence, and passive Si uptake persists as long as the plants are transpiring. A prolonged growing period for N enriched plants could result in higher tissue Si concentrations at the end of the growing season through passive uptake in the absence of increasing biomass. Active uptake has also been reported for S. alterniflora (Carey and Fulweiler, 2014b), but the steady increase in tissue SiO2 throughout the growing season in the field experiment suggest that passive uptake is the primary mode of Si acquisition in the Poplar Island marsh.
The question of whether increased tissue concentrations confer resistance to stress in S. alterniflora was not resolved by these studies. Neither lodging nor grazing by O. zibethicus were observed in or near the experimental plots during the course of the field experiment. Fungal analysis suggested that there could be a protective mechanism conveyed by Si enrichment, at least with respect to the more pathogenic strains found in S. alterniflora. Bazzano and Elmer (2017) found Si amended S. alterniflora in greenhouse trials did not, however, differ in susceptibility to F. palustre or in herbivory by marsh crabs when compared to untreated plots.
Although some increases in S. alterniflora tissue Si concentrations were observed in response to amendments in this study, associated improvements in fitness and production were not observed even under extreme N enrichment, as has been reported for O. sativa and some other crop species. Both species are members of the same family, Poacea, known to have higher tissue concentrations than most other vascular plants. S. alterniflora, however, has been designated an intermediate Si user accumulating Si primarily through passive uptake, with tissue concentrations of 0.5–1.0% dry weight (Querne et al., 2012), whereas O. sativa is considered an accumulator, showing both passive and active uptake and tissue concentrations > 1.0% (Ma and Takahashi, 2002). Notably, tissue concentrations in our study were within the range considered to be characteristic of accumulators, but we hypothesize that this results from N-enrichment, which can delay senescence, allowing Si uptake to continue later in the growing season (Marschner, 2012). The reasons for generally lower tissue Si concentrations in S. alterniflora compared with O. sativa are unclear, but may be related to Si availability in brackish to saltwater versus freshwater environments, or to physiological requirements related to perennial (S. alterniflora) versus annual (O. sativa) life cycles.
4.3. Conclusion
The present study has demonstrated that newly constructed fine-grained dredged material marshes can provide a source of both dissolved and biogenic Si to local waters in stoichiometric excess (relative to algal requirements) of exported N and P, an overlooked ecosystem service. High nutrient availability drives high rates of macrophyte production, which appear to enhance mobilization of sediment DSi and remineralization. The tidal creeks also appear to be important in the transformation and export of Si. Effects on the composition and shifts of the local benthic and pelagic algal community are unknown, but may be important locally for higher trophic levels, a potential area for future study.
Soil amendment with calcium meta-silicate resulted in slightly enhanced tissue concentrations in S. alterniflora, but improved plant fitness was not detected in this study, even in this N rich environment where symptoms of N stress were abundant. The accumulation of evidence from this and other studies appears to cast doubt on the concerns of Lanning and Eleuterius (1981, 1983) that N stress in S. alterniflora results from a Si deficiency.
Data availability statement
The original contributions presented in the study are included in the article/Supplementary material, further inquiries can be directed to the corresponding author.
Author contributions
LS, JS, and NN conceived and designed the tidal flux study. LS, JS, JC and WE designed the experiments. LS and NN performed the tidal flux study and LS performed the experiments. LS, MO, and WE performed sample analysis. LS, NN and KS performed data analysis. LS wrote the manuscript. All authors contributed to the article and approved the submitted version.
Funding
This research was funded by the Maryland Department of Transportation Maryland. Port Administration (MDOT MPA) with project management by the Maryland Environmental Service (MES), grant number 14-07-16.
Acknowledgments
We wish to thank Maryland Environmental Service (MES) for logistical support for field work. We thank S. Lyubchich and S. Malkin for assistance with statistical analyses. We are grateful to two reviewers and special issue editor Myriam Barbeau for reviews of the manuscript and many helpful comments. We thank D. Stevenson and R. Long for field assistance, and J. Charest for editorial assistance. This is contribution #6288 from the University of Maryland Center for Environmental Science.
Conflict of interest
The authors declare that the research was conducted in the absence of any commercial or financial relationships that could be construed as a potential conflict of interest.
Publisher’s note
All claims expressed in this article are solely those of the authors and do not necessarily represent those of their affiliated organizations, or those of the publisher, the editors and the reviewers. Any product that may be evaluated in this article, or claim that may be made by its manufacturer, is not guaranteed or endorsed by the publisher.
Supplementary material
The Supplementary material for this article can be found online at: https://www.frontiersin.org/articles/10.3389/fevo.2023.1097380/full#supplementary-material
References
Anderson, K. A., and Downing, J. A. (2006). Dry and wet atmospheric deposition of nitrogen, phosphorus and silicon in an agricultural region. Water Air Soil Pollut. 176, 351–374. doi: 10.1007/s11270-006-9172-4
Baker, R., Taylor, M. D., Able, K. W., Beck, M. W., Cebrian, J., Colombano, D. D., et al. (2020). Fisheries rely on threatened salt marshes. Science 370, 670–671. doi: 10.1126/science.370.6517.670-b
Bazzano, M., and Elmer, W. (2017). Interactions and consequences of silicon, nitrogen, and Fusarium palustre on herbivory and DMSP levels of Spartina alterniflora. Estuar. Coast. Shelf Sci. 198, 106–113. doi: 10.1016/j.ecss.2017.08.046
Carey, J. C., and Fulweiler, R. W. (2013). Nitrogen enrichment increases net silica accumulation in a temperate salt marsh. Limnol. Oceanogr. 58, 99–111. doi: 10.4319/lo.2013.58.1.0099
Carey, J. C., and Fulweiler, R. W. (2014a). Salt marsh tidal exchange increases residence time of silica in estuaries. Limnol. Oceanogr. 59, 1203–1212. doi: 10.4319/lo.2014.59.4.1203
Carey, J. C., and Fulweiler, R. W. (2014b). Silica uptake by Spartina—evidence of multiple modes of accumulation from salt marshes around the world. Front. Plant Sci. 5, 1–11. doi: 10.3389/fpls.2014.00186
Conley, D. J. (1997). Riverine contribution of biogenic silica to the oceanic silica budget. Limnol. Oceanogr. 42, 774–777. doi: 10.2307/2839124
Conley, D. J. (1998). An interlaboratory comparison for the measurement of biogenic silica in sediments. Mar. Chem. 63, 39–48. doi: 10.1016/S0304-4203(98)00049-8
Conley, D. J., and Malone, T. C. (1992). Annual cycle of dissolved silicate in Chesapeake Bay; implications for the production and fate of phytoplankton biomass. Mar. Ecol. Prog. Ser. 81, 121–128. doi: 10.3354/meps081121
Cooper, S. R., and Brush, G. S. (1991). Long-term history of Chesapeake Bay anoxia. Science 254, 992–996. doi: 10.2307/2879708
Cornwell, J. C., Conley, D. J., Owens, M., and Stevenson, J. (1996). A sediment chronology of the eutrophication of Chesapeake Bay. Estuaries 19, 488–499. doi: 10.2307/1352465
Cornwell, J. C., and Owens, M. S. (2011). Quantifying sediment nitrogen releases associated with estuarine dredging. Aquat. Geochem. 17, 499–517. doi: 10.1007/s10498-011-9139-y
Cornwell, J. C., Owens, M. S., Staver, L. W., and Stevenson, J. C. (2020). Tidal marsh restoration at Poplar Island I: transformation of estuarine sediments into marsh soils. Wetlands 40, 1673–1686. doi: 10.1007/s13157-020-01294-5
Costa-Pierce, B. A., and Weinstein, M. P. (2002). Use of dredge materials for coastal restoration. Ecol. Eng. 19, 181–186. doi: 10.1016/S0925-8574(02)00076-9
Craft, C., Reader, J., Sacco, J. N., and Broome, S. W. (1999). Twenty-five years of ecosystem development of constructed Spartina alterniflora (Loisel) marshes. Ecol. Appl. 9, 1405–1419. doi: 10.1890/1051-0761(1999)009[1405:TFYOED]2.0.CO;2
Darby, F. A., and Turner, R. E. (2008). Below-and aboveground biomass of Spartina alterniflora: response to nutrient addition in a Louisiana salt marsh. Estuar. Coasts 31, 326–334. doi: 10.1007/s12237-008-9037-8
Datnoff, L. E., Snyder, G. E., and Korndorfer, G. H. (eds.). (2001). Silicon in Agriculture. Amsterdam: Elsevier.
de Bakker, N. V. J., Hemminga, M. A., and Van Soelen, J. (1999). The relationship between silicon availability, and growth and silicon concentration of the salt marsh halophyte Spartina anglica. Plant Soil 215, 19–27. doi: 10.1023/A:1004751902074
Deegan, L. A., Johnson, D. S., Warren, R. S., Peterson, B. J., Fleeger, J. W., Fagherazzi, S., et al. (2012). Coastal eutrophication as a driver of salt marsh loss. Nature 490, 388–392. doi: 10.1038/nature11533
Demaster, D. J. (1981). The supply and accumulation of silica in the marine environment. Geochim. Cosmochim. Acta 45, 1715–1732. doi: 10.1016/0016-7037(81)90006-5
Elmer, W. H., and Marra, R. E. (2009). Discovery of a new species of fusarium from Spartina alterniflora and the influence of drought on its ability to cause plant mortality. Phytopathology 99:S192. doi: 10.1094/PHYTO.2009.99.6.S191
Elmer, W. H., and Marra, R. E. (2011). New species of fusarium associated with dieback of Spartina alterniflora in Atlantic salt marshes. Mycologia 103, 806–819. doi: 10.3852/10-155
Elmer, W. H., Useman, S., Schneider, R. W., Marra, R. E., LaMondia, J. A., Mendelssohn, I. A., et al. (2012). Sudden vegetation dieback in Atlantic and Gulf Coast salt marshes. Plant Dis. 97, 436–445. doi: 10.1094/PDIS-09-12-0871-FE
Epstein, E. (1999). Silicon. Annu. Rev. Plant Biol. 50, 641–664. doi: 10.1146/annurev.arplant.50.1.641
Epstein, E. (2001). “Silicon in plants: facts vs. concepts” in Silicon in Agriculture. eds. L. E. Datnoff, G. E. Snyder, and G. H. Korndorfer (Amsterdam: Elsevier), 1–15.
Fleri, J. R., Lera, S., Gerevini, A., Staver, L., and Nardin, W. (2019). Empirical observations and numerical modelling of tides, channel morphology, and vegetative effects on accretion in a restored tidal marsh. Earth Surf. Process. Landf. 44, 2223–2235. doi: 10.1002/esp.4646
Frasco, B. A., and Good, R. E. (1982). Decomposition dynamics of Spartina alterniflora and Spartina patens in a New Jersey salt marsh. Am. J. Bot. 69, 402–406. doi: 10.1002/j.1537-2197.1982.tb13273.x
Giblin, A. E., Fulweiler, R. W., and Hopkinson, C. S. (2021). “The role of marshes in coastal nutrient dynamics and loss” in Salt Marshes: Function, Dynamics, and Stresses. eds. D. M. FitzGerald and Z. J. Hughes (Cambridge: Cambridge University Press), 113–154.
Hackney, C. T., Cahoon, L. B., Preziosi, C., and Norris, A. (2000). “Silicon is the link between tidal marshes and estuarine fisheries: a new paradigm” in Concepts and Controversies in Tidal Marsh Ecology. eds. M. P. Weinstein and D. A. Kreeger (Dordrecht, Netherlands: Kluwer Academic Publishers), 543–552.
Hesslein, R. H. (1976). An in situ sampler for close interval pore water studies. Limnol. Oceanogr. 21, 912–914. doi: 10.4319/lo.1976.21.6.0912
Hodson, M. J., White, P. J., Mead, A., and Broadley, M. R. (2005). Phylogenetic variation in the silicon composition of plants. Ann. Bot. 96, 1027–1046. doi: 10.1093/aob/mci255
Humborg, C., Conley, D. J., Rahm, L., Wulff, F., Cociasu, A., and Ittekkot, V. (2000). Silicon retention in river basins: far-reaching effects on biogeochemistry and aquatic food webs in coastal marine environments. AMBIO: a journal of the human. Environment 29, 45–50. doi: 10.1579/0044-7447-29.1.45
Jacobs, S., Struyf, E., Maris, T., and Meire, P. (2008). Spatiotemporal aspects of silica buffering in restored tidal marshes. Estuar. Coast. Shelf Sci. 80, 42–52. doi: 10.1016/j.ecss.2008.07.003
Kemp, W. M., Boynton, W. R., Adolf, J. E., Boesch, D. F., Boicourt, W. C., Brush, G., et al. (2005). Eutrophication of Chesapeake Bay: historical trends and ecological interactions. Mar. Ecol. Prog. Ser. 303, 1–29. doi: 10.3354/meps303001
Korndorfer, G. H., and Lepsch, I. (2001). “Effect of silicon on plant growth and crop yield” in Silicon in Agriculture. eds. L. E. Datnoff, G. E. Snyder, and G. H. Korndorfer (Amsterdam: Elsevier), 133–158.
Lacroix, E., Brovelli, A., Barry, D. A., and Holliger, C. (2014). Use of silicate minerals for pH control during reductive dechlorination of chloroethenes in batch cultures of different microbial consortia. Appl. Environ. Microbiol. 80, 3858–3867. doi: 10.1128/aem.00493-14
Lanning, F. C., and Eleuterius, L. N. (1981). Silica and ash in several marsh plants. Gulf Res. Rep. 7, 47–52. doi: 10.18785/grr.0701.07
Lanning, F. C., and Eleuterius, L. N. (1983). Silica and ash in tissues of some coastal plants. Ann. Bot. 51, 835–850. doi: 10.1093/oxfordjournals.aob.a086534
Li, Z., Song, Z., Parr, J. F., and Wang, H. (2013). Occluded C in rice phytoliths: implications to biogeochemical carbon sequestration. Plant Soil 370, 615–623. doi: 10.1007/s11104-013-1661-9
Loucaides, S., Van Cappellen, P., and Behrends, T. (2008). Dissolution of biogenic silica from land to ocean: role of salinity and pH. Limnol. Oceanogr. 53, 1614–1621. doi: 10.4319/lo.2008.53.4.1614
Ma, J. F., Miyake, Y., and Takahashi, E. (2001). “Silicon as a beneficial element for crop plants” in Silicon in Agriculture. eds. L. E. Datnoff, G. E. Snyder, and G. H. Korndorfer (Amsterdam: Elsevier), 17–39.
Ma, J. F., and Takahashi, E. (2002). Soil, Fertilizer, and Plant Silicon Research in Japan. Amsterdam: Elsevier.
Mateos-Naranjo, E., Andrades-Moreno, L., and Davy, A. J. (2013). Silicon alleviates deleterious effects of high salinity on the halophytic grass Spartina densiflora. Plant Physiol. Biochem. 63, 115–121. doi: 10.1016/j.plaphy.2012.11.015
Mendelssohn, I. (1979). The influence of nitrogen level, form, and application method on the growth response of <i>Spartina alterniflora in North Carolina. Estuar. Coasts 2, 106–112. doi: 10.2307/1351634
Mendelssohn, I. A., and Morris, J. T. (2002). “Eco-physiological controls on the productivity of Spartina alterniflora Loisel” in Concepts and Controversies in Tidal Marsh Ecology. eds. M. P. Weinstein and D. A. Kreeger (Dordrecht, The Netherlands: Kluwer Academic Publishers), 59–80.
Müller, F., Struyf, E., Hartmann, J., Wanner, A., and Jensen, K. (2013). A comprehensive study of silica pools and fluxes in Wadden Sea salt marshes. Estuar. Coasts 36, 1150–1164. doi: 10.1007/s12237-013-9621-4
Nanayakkara, U. N., Uddin, W., and Datnoff, L. E. (2009). Soil silicon amendment for managing gray leaf spot of perennial ryegrass turf on golf courses in Pennsylvania. Can. J. Plant Pathol. 31, 415–426. doi: 10.1080/07060660909507616
Norris, A. R., and Hackney, C. T. (1999). Silica content of a mesohaline tidal marsh in North Carolina. Estuar. Coast. Shelf Sci. 49, 597–605. doi: 10.1006/ecss.1999.0506
Officer, C. B., and Ryther, J. H. (1980). The possible importance of silicon in marine eutrophication. Mar. Ecol. Prog. Ser. 3, 83–91. doi: 10.3354/meps003083
Parr, J. F., and Sullivan, L. A. (2005). Soil carbon sequestration in phytoliths. Soil Biol. Biochem. 37, 117–124. doi: 10.1016/j.soilbio.2004.06.013
Querne, J., Ragueneau, O., and Poupart, N. (2012). In situ biogenic silica variations in the invasive salt marsh plant, Spartina alterniflora: a possible link with environmental stress. Plant Soil 352, 157–171. doi: 10.1007/s11104-011-0986-5
Ragueneau, O., Conley, D. J., Leynaert, A., Longphuirt, S. N., and Slomp, C. P. (2006). “Role of diatoms in silicon cycling and coastal marine food webs” in The Silicon Cycle, Human Perturbations and Impacts on Aquatic Systems. eds. V. Ittekkot, D. Unger, C. Humborg, and N. T. An (Washington, DC: Island Press), 163–195.
Saccone, L., Conley, D. J., and Sauer, D. (2006). Methodologies for amorphous silica analysis. J. Geochem. Explor. 88, 235–238. doi: 10.1016/j.gexplo.2005.08.045
Schaller, J., Brackhage, C., Gessner, M. O., Bauker, E., and Gert Dudel, E. (2012). Silicon supply modifies C:N:P stoichiometry and growth of Phragmites australis. Plant Biol. (Stuttg.) 14, 392–396. doi: 10.1111/j.1438-8677.2011.00537.x
Schoelynck, J., Müller, F., Vandevenne, F., Bal, K., Barão, L., Smis, A., et al. (2014). Silicon–vegetation interaction in multiple ecosystems: a review. J. Veg. Sci. 25, 301–313. doi: 10.1111/jvs.12055
Scudlark, J. R., and Church, T. M. (1989). The sedimentary flux of nutrients at a Delaware salt marsh site: a geochemical perspective. Biogeochemistry 7, 55–75. doi: 10.2307/1468528
Staver, L. W., Cornwell, J. C., Nidzieko, N. J., Staver, K. W., Stevenson, J. C., Owens, M., et al. (2021). The fate of nitrogen in dredged material used for tidal marsh restoration. J. Mar. Sci. Eng. 9:849. doi: 10.3390/jmse9080849
Staver, L., Stevenson, J., Cornwell, J., Nidzieko, N., Staver, K., Owens, M., et al. (2020). Tidal marsh restoration at Poplar Island: II. Elevation trends, vegetation development, and carbon dynamics. Wetlands 40, 1687–1701. doi: 10.1007/s13157-020-01295-410.1007/s13157-020-01295-4
Sterner, R. W., and Elser, J. J. (2002). Ecological Stoichiometry, the Biology of Elements From Molecules to the Biosphere. Princeton, NJ: Princeton University Press.
Strickland, J., and Parsons, T. (1968). A Practical Handbook of Seawater Analysis. Ottawa: Queen’s Printer.
Struyf, E., and Conley, D. J. (2009). Silica: an essential nutrient in wetland biogeochemistry. Front. Ecol. Environ. 7, 88–94. doi: 10.1890/070126
Struyf, E., Dausse, A., Damme, S. V., Bal, K., Gribsholt, B., Boschker, H. T. S., et al. (2006). Tidal marshes and biogenic silica recycling at the land-sea interface. Limnol. Oceanogr. 51, 838–846. doi: 10.2307/3841092
Struyf, E., Temmerman, S., and Meire, P. (2007). Dynamics of biogenic Si in freshwater tidal marshes: Si regeneration and retention in marsh sediments (Scheldt estuary). Biogeochemistry 82, 41–53. doi: 10.2307/20456434
Tegen, I., and Kohfeld, K. E. (2006). “Atmospheric transport of silicon” in The Silicon Cycle. eds. V. Ittekkot, D. Unger, C. Humborg, and N. T. An (Washington, DC: Island Press), 81–91.
Tréguer, P., Nelson, D. M., Bennekom, A. J. V., DeMaster, D. J., Leynaert, A., and Quéguiner, B. (1995). The silica balance in the world ocean: a reestimate. Science 268, 375–379. doi: 10.2307/2886587
Turner, R. E. (2011). Beneath the salt marsh canopy: loss of soil strength with increasing nutrient loads. Estuar. Coasts 34, 1084–1093. doi: 10.1007/s12237-010-9341-y
Van Cappellen, P. (2003). Biomineralization and global biogeochemical cycles. Rev. Mineral Geochem. 54, 357–381. doi: 10.2113/0540357
Vieillard, A. M., Fulweiler, R. W., Hughes, Z. J., and Carey, J. C. (2011). The ebb and flood of silica: quantifying dissolved and biogenic silica fluxes from a temperate salt marsh. Estuar. Coast. Shelf Sci. 95, 415–423. doi: 10.1016/j.ecss.2011.10.012
Keywords: dredged material, beneficial use, nutrients, Poplar Island, ecosystem services, Spartina alterniflora
Citation: Staver LW, Stevenson JC, Cornwell JC, Nidzieko NJ, Staver KW, Owens MS and Elmer WH (2023) Silicon pools, fluxes and the potential benefits of a silicon soil amendment in a nitrogen-enriched tidal marsh restoration. Front. Ecol. Evol. 11:1097380. doi: 10.3389/fevo.2023.1097380
Edited by:
Myriam A. Barbeau, University of New Brunswick Fredericton, CanadaReviewed by:
Robinson W. Fulweiler, Boston University, United StatesDaniel Puppe, Leibniz Center for Agricultural Landscape Research (ZALF), Germany
Copyright © 2023 Staver, Stevenson, Cornwell, Nidzieko, Staver, Owens and Elmer. This is an open-access article distributed under the terms of the Creative Commons Attribution License (CC BY). The use, distribution or reproduction in other forums is permitted, provided the original author(s) and the copyright owner(s) are credited and that the original publication in this journal is cited, in accordance with accepted academic practice. No use, distribution or reproduction is permitted which does not comply with these terms.
*Correspondence: L. W. Staver, bHN0YXZlckB1bWNlcy5lZHU=