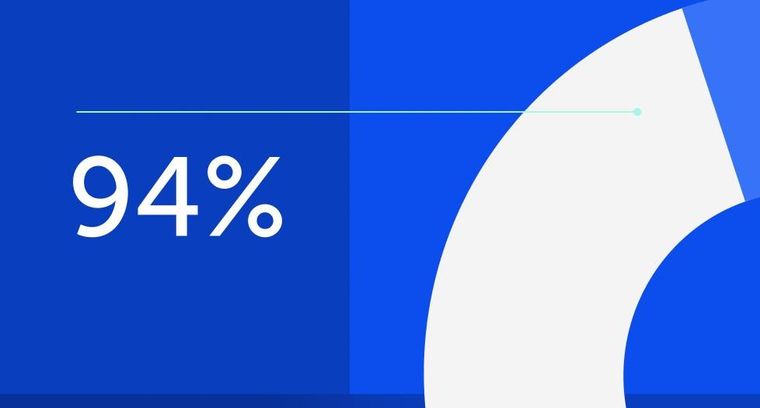
94% of researchers rate our articles as excellent or good
Learn more about the work of our research integrity team to safeguard the quality of each article we publish.
Find out more
ORIGINAL RESEARCH article
Front. Ecol. Evol., 23 February 2023
Sec. Paleoecology
Volume 11 - 2023 | https://doi.org/10.3389/fevo.2023.1087577
Introduction: Islands of the Southwest Pacific are exposed to geologic and climate-related disturbances that occur on a range of timescales and which probably affect, to varying degrees, their terrestrial ecosystems. Over the past ∼1100 years we know of two major events in the region: the Kuwae eruption which is thought to have occurred ∼500 cal. years BP and a shift to drier conditions which began ∼1100 cal. years BP.
Methods: We investigated terrestrial and lacustrine ecosystem responses to these events and also to a changing fire regime, likely human-caused, using a multi-proxy (C/N, charcoal, chironomids, pollen, and tephra) record from Lake Emaotul, Efate, Vanuatu.
Results: Tephra from the Kuwae eruption was found across a 6 cm layer which our age-depth model suggests was deposited 650–510 cal. years BP (95% confidence). Forest and chironomid community turnover increased during the wet-dry shift 1100–1000 cal. years BP; subsequently, chironomid turnover rates decreased again within <135 years and vegetation had partially (but not fully) recovered after ∼80 years. Following Kuwae volcanic tephra deposition, vegetation turnover increased again, reflecting a reduction in small trees and shrubs and an increase in grasses. Subsequently, the forest vegetation did not regain its previous composition, whereas chironomid community composition remained fairly stable before and after tephra deposition. Within the last ∼90 years, enhanced local burning drove another increase in vegetation turnover.
Discussion: Terrestrial and freshwater ecosystems in Efate are sensitive to changes in hydroclimate, volcanism, and anthropogenic fires, although to different degrees; while recent human impacts are often obvious, volcanic eruptions and climatic shifts have also structured Pacific-island ecosystems and will continue to do so.
Islands are often home to high levels of endemism compared with mainland ecosystems, and they also contribute disproportionately by land area to total terrestrial diversity (Kier et al., 2009; Losos and Ricklefs, 2009). Palaeoecological records from islands around the world have shown that human arrival is generally associated with accelerated turnover in floristic diversity (Nogué et al., 2021) and faunal extinctions (Steadman et al., 2002; Bedford and Spriggs, 2014; Gosling et al., 2017). Under such dramatic anthropogenic changes, the impacts of natural disturbances and climate change are challenging to identify, yet they also influence ecosystem dynamics. South Pacific islands are often exposed to natural hazards: cyclones, droughts, landslides, tsunamis, earthquakes, and volcanic eruptions (Siméoni, 2009; Bedford and Spriggs, 2014). Such events would have been the main drivers of change prior to human settlement. High-resolution palaeoecological records, such as the one presented here, provide valuable data on the rates and patterns of recovery of terrestrial ecosystems following natural disturbances.
The January 2022 Hunga Tonga–Hunga Ha’apai eruption was a timely reminder of how Pacific islands can be exposed to volcanic hazards. Responses to eruptions often relate to substantial tephra falls and have been documented previously in palaeovegetation records from both continental and island locations (Supplementary Information). For example, in the tropical Andes of Ecuador, a lake-sediment record from a páramo (alpine grassland) landscape showed a slight increase in herb pollen, a decrease in local and long-distance forest taxa, and a relatively quick (<100 years) recovery following volcanic tephra deposition, whereas in a forested, pre-montane landscape, the forest opened up after tephra deposition and took around 150 years to return to the pre-eruption state (Montoya et al., 2021). On the eastern Atlantic Ocean island of Brava, Cabo Verde, repeated tephra deposition between 1800 and 650 cal. years BP coincided with an expansion of fern-rich, woody scrubland and the decline of previously dominant Urticaceae and Poaceae (Castilla-Beltrán et al., 2021b). In southern Italy, pollen records have shown a decrease in vegetation productivity following eruptions (Allen and Huntley, 2018). Generalisations about recovery rates are difficult to make, as the extent of the impact depends on a range of factors: the volume of ash ejected, thickness of ash (Allen and Huntley, 2018), the height of vegetation, ground surface roughness (Arnalds, 2013), how long ash persists in the catchment (Hickman and Reasoner, 1994), distance from the eruption, the nutrients released, the types of vegetation and propagules that survive (Dale et al., 2005), and local climate conditions (Wilmshurst and Mcglone, 1996).
Lacustrine ecosystems are also impacted by volcanic eruptions. Nutrient enrichment can alter their ecology, but, as with terrestrial ecosystems, aquatic responses to ash falls can vary depending on lake and catchment characteristics. Volcanic ash may deposit elements such as sulphur and decrease soil pH, which in turn may release acid-soluble metals into soil solution (Cronin et al., 1997) as well as to groundwater and lacustrine environments. Low inputs of tephra can also have a fertilising effect on soils (Arnalds, 2013). The 2011 eruption of the Puyehue volcanic complex in Chile ejected large amounts of ash and pumice, and in some lakes, this resulted in increases in suspended solids, light exclusion, increased phosphorus and increased biomass of phytoplankton in relation to the pre-eruption conditions (Modenutti et al., 2013). Also in Chile, at Lake Galletué, palaeoecological reconstructions indicated that tephra deposition in 1957 CE resulted in a transient increase in nutrients, identified through diatom analysis (Urrutia et al., 2007). Montoya et al. (2021) suggested that the vegetation surrounding a lake or within a catchment may be important for creating a barrier against deposition and to reduce tephra runoff into lakes. Laguna Baños, on the Ecuadorian Andean flank, is surrounded by open páramo and thus not protected by a forest barrier, making it susceptible to tephra in-wash. Here, there was also a significant shift in chironomid assemblage composition after ash deposition that may have been caused by shallowing of the lake. According to the results of Matthews-Bird et al. (2017) and Montoya et al. (2021) the chironomid regime shift has still not recovered today, 1500 years later.
With regards to the Pacific islands, hydroclimate changes are another source of environmental variation; they are strongly related to movement of the South Pacific Convergence Zone (SPCZ), a rain band that delivers precipitation to the islands of the South Pacific Ocean (Hassall, 2017; Sear et al., 2020). During El Niño events, the SPCZ moves northwards, which can lead to severe droughts in more southerly islands, including Vanuatu. For example, during the 1994/1995 CE drought in Vanuatu there was no rain for 9 months, and communities experienced water shortages and the loss of livestock and crops (Mcnamara and Prasad, 2014). The impacts of dry periods on South Western Pacific islands in the past may have been severe, and it has been suggested that drought coupled with other societal pressures may have been the impetus for Polynesians to explore the Eastern Pacific islands (Sear et al., 2020). In particular, a drier climate phase has been identified around 1100–500 cal. years BP in Vanuatu, Sāmoa, and Atiu (Cook Islands) (Sear et al., 2020; Maloney et al., 2022).
People settled Vanuatu at least 3000 years ago (Lipson et al., 2020), meaning they have been part of the ecosystem for the duration of this record. An increase in charcoal fragments has been identified in a palaeoecological record from Aneityum island, Southern Vanuatu, and is associated with human arrival (Hope and Spriggs, 1982). Evidence of burning throughout the last 2000 years has also been detected in another palaeoecological record from the island of Erromango (Hope et al., 1999) but the effects of fire on vegetation turnover remain unknown.
Here we investigate a 1600 year record that gives insight into how terrestrial vegetation, and components of the lacustrine system, responded to this past drier climate phase (precipitation record previous published by Maloney et al., 2022), to the Kuwae eruption, and to changes in fire regime.
The nation of Vanuatu in the Southwest Pacific stretches 1000 km from north to south and comprises over 83 islands, which formed as a result of uplift and volcanism associated with the New Hebrides Arc, where eastward subduction of the Indo-Australia Plate beneath the Pacific Plate occurs (Stewart et al., 2010). Around 90% of the islands of Vanuatu are less than 1.8 Ma years old (Mallick, 1975). Here we focus on Efate, Central Province, the location of Port Vila, the capital city of Vanuatu. Efate has a volcanic core (Ash et al., 1978), but the surface geology is dominated by uplifted coral sequences and the Efate Pumice Formation, which form the main topography of the centre of the island (Raos and Mcphie, 2003). A series of now uplifted coral terraces formed within the last 300,000 years along the island coast (Taylor et al., 1987).
The climate of Vanuatu is tropical, with temperatures ranging between 23 and 33°C (Singh et al., 2019). The main climate influences are the position and variability of the West Pacific Warm Pool (WPWP) and SPCZ (Vincent, 1994). Summers are relatively wet and often feature cyclones, while winters are drier. South-westerly trade winds prevail for most of the year. Mean annual rainfall on Efate varies from 3000 mm on the eastern coast of the island to 2400 mm on the western coast (Cillaurren et al., 2001); however, yearly rainfall totals vary in relation to the state of ENSO (the El Niño Southern Oscillation), a pattern of inter-annual climate variability linked to changes in sea-surface temperatures and precipitation (Wyrtki, 1975). During El Niño events the SPCZ is in a more northerly position, and during La Niña events it extends to a more south-easterly position. Typically, during an El Niño phase the climate of Vanuatu is drier than average, while during the opposite La Niña phase it is wetter than average (Wirrmann et al., 2011; Combettes et al., 2015).
The vegetation of Vanuatu varies with human impacts, soil type, leeward or windward locations, and altitude (Mueller-Dombois and Fosberg, 1998). The vegetation can be generally classified as lowland rain forest, montane cloud forest, seasonal forest, scrub, grassland, pioneer species growing on new volcanic surfaces, coastal taxa including mangrove forests, and secondary and cultivated vegetation (see Mueller-Dombois and Fosberg, 1998 for detailed descriptions).
The Kuwae submarine caldera is located between the islands of Epi and Tongoa in Vanuatu (Figure 1B), around 100 km north of Efate (Figure 1). Evidence from multiple ice cores, historical records, and tree rings from the Northern and Southern hemispheres suggested a single eruption of Kuwae in either 1452 or 1453 CE (Gao et al., 2006). However, evidence from ice cores from the Law Dome site, Antarctica, has identified a large sulphate signal dating to ∼1458 CE which may been from the Kuwae eruption (Plummer et al., 2012). Yet, South Pole ice cores containing cryptotephra which was deposited c.1458 CE do not match with Kuwae tephra (Hartman et al., 2019) and Kuwae tephra is yet to be detected within Antarctic ice cores. Locally, the geology of the mainly submarine Kuwae volcano is represented by a complex sequence of andesitic to dacitic pyroclastic deposits covering the islands of Epi, Tongoa, Laika and other nearby islets. Németh et al. (2007) suggest that terrestrial deposits were consistent with pyroclastic flows from median to small eruptions but dismissed the likelihood of a large eruption in the 1400s CE. Using an assumed erupted volume of ∼40 km3 (from Robin et al., 1994), and based on analysis of melt inclusions, Witter and Self (2007) estimated sulphur release of 175–700 Tg from the eruption. If this was during a single event, it amounts to five times the climate-forcing ejection produced by the 1815 CE Tambora eruption (Indonesia) (Cole-Dai et al., 2000). The volume of material ejected is around six times that ejected during the 1991 CE Pinatubo eruption in the Philippines (Pang, 1993). Given the scale of this eruption, we would expect to see evidence of tephra deposition and an effect on vegetation in Efate.
Figure 1. (A) The location of Vanuatu within the Pacific Ocean; (B) the Southern and central islands of Vanuatu, the red triangle indicates the location of the Kuwae submarine caldera; (C) the island of Efate. Yellow circles indicate the location of archaeological sites and the red crosses indicate palaeoecological sites including Lake Emaotul (Wirrmann et al., 2011; Combettes et al., 2015). (D,E) Are photographs taken from the shore of Lake Emaotul in October 2015.
Archaeological and genetic evidence suggests that people first settled Vanuatu c. 3000 years ago (Petchey et al., 2014). Later Mangaasi-style ceramics are identified in numerous coastal and inland sites from 2300–1200 cal. years BP. The 500–510 cal. years BP Kuwae eruption had an important cultural impact since many ni-Vanuatu, the indigenous people of Vanuatu, were forced to migrate away from the affected Sheppard and Epi islands (Hoffmann, 2007); Garanger (1972) has documented long-standing oral traditions of the movement of people from these islands to Efate in the post-eruption period. Furthermore, Bedford et al. (2018) has inferred from a large expanse of agricultural features documented from recent LiDAR aerial surveys, that rapid population expansion on Efate took place within the last 1000 years. This period is associated with Lapita ceramic-ware culture, and these ceramics have been discovered at the coastal Teouma Cemetery, to the west of Emaotfer Swamp on Efate (Figure 1C).
Sediment samples were recovered from Lake Emaotul (sometimes called Duck Lake; 17°43′57.48″S, 168°24′53.58″E) on Efate. Lake Emaotul was selected because it is a confined lake system likely to be sensitive to changes in the precipitation-evaporation balance. It is relatively insulated from marine processes compared with other sites on the island due to its elevation (119 m above sea level). The lake sits within a raised coral reef dated at c. 120,000 BP (Sear et al., 2020). It has a maximum depth of 7.1 m and is meromictic. A chemocline at 6 m results in a suboxic hypolimnion with dissolved oxygen at 5% saturation (Sear et al., 2020).
Hydrologically, the lake is a closed system. It measures 0.3 km2 in area and has a catchment covering 0.98 km2. The lake is fringed by grass-dominated wetlands to the east and west, with the swamp-forest taxa Barringtonia, Hibiscus, and Pandanus. The lake surface is mostly open but has some cover of water lilies, such as Nelumbo nucifera, Nymphaea spp., and Nymphoides spp. The wetland areas are bordered by Phragmites. Beyond the wetland, the lake catchment is covered by secondary tropical forest that supports patches of subsistence gardening and cattle grazing (Sear et al., 2020). Secondary lowland forest taxa include Metroxylon warburgii (Kalfatak and Jaensch, 2014).
The Lake Emaotul cores were retrieved between the 9th and 11th October 2015. The 3.4-m sedimentary sequence of overlapping cores was retrieved using a UWITEC gravity corer and a piston corer (Geo-Core, Columbus, OH, USA) from the deepest part of the lake. Deeper coring of the sediments was impeded by stiff clay. This precluded recovery of sediments that predated human arrival. All six recovered cores were stored in airtight tubes during transportation and subsequently at + 4°C at the School of Geography and Environmental Sciences (SOGES) at the University of Southampton (UK).
Three subsamples of the core that displayed discrete and high magnetic susceptibility were extracted from 173 to 171, 169 to 167, and 166 to 160 cm. To identify the depth range over which tephra was deposited small amounts of sediment were smeared on glass slides and examined under an optical microscope. All samples contained shards; however, particularly high counts of fresh volcanic glass particles occurred between 166 and 160 cm. To obtain the geochemical signature of volcanic glass samples from all three horizons were cleaned in deionised water, and the coarsest grains (∼63–125 μm) were hand-picked, mounted in an epoxy round, cut, and polished. The polished samples were sputter-coated in carbon. An initial suite of three samples was analysed using a Jeol JXA8230 Superprobe at the University of Iowa, with repeat analyses and an additional eight samples using a JXA8530F Hyperprobe at the University of Auckland. For both instruments, points were analysed using an accelerating voltage of 15 kV and a 2-nA electron beam current. A defocussed 10-μm beam was used in order to minimise Na loss, with a 5-μm diameter required for a few smaller grains. Analysis was completed with 10-s peak and 10-s total background dwell times for all elements. To test for Na migration, some analyses were collected in two 1-s accumulated peak measurements with 1-s dwell time on background for the second measurement. The accumulated Na measurements showed that post-measurement correction of Na migration was not required. Analyses were calibrated and quality cross-checked against secondary glass standards ATHO-G (MPI-DING), BCR-2G, (USGS), and VG-568 (NMNH 72854).
The age-depth model is based on the radiocarbon ages of seven plant macrofossils and 34 210Pb analyses; see Maloney et al. (2022). All 14C dates were re-calibrated using the Southern Hemisphere calibration curve (Hogg et al., 2020) to years before present (cal. years BP). To build the age-depth model we used Bacon v2.2 (Blaauw et al., 2021).
We analysed 188 sediment samples (every 2 cm) for carbon-to-nitrogen (C/N) ratio, which is used as an indicator of the source of organic material in a lake (Rice and Hanson, 1984). See Supplementary Information for full C/N method. The C/N ratio can vary, since algae are more enriched in nitrogen and depleted in carbon compared with terrestrial vascular plants (Tyson, 1995). A C/N ratio of >20 usually indicates a system in which terrestrial organic material is dominant (Jones et al., 2013), whereas a ratio of 10–20 likely signifies a mixture of terrestrial and aquatic organic inputs. A ratio of <10 is more likely to suggest more aquatic plants were present (García-Alix et al., 2012). C/N samples were measured at the British Geological Survey, in Nottingham, UK.
To determine plant community changes, we analysed 32 samples of 1-cm3 volume for pollen and spores. The sampling resolution is about 1 sample per ∼70 years (14 cm) for the majority of the sequence and one sample every ∼5 years (1 cm) above and below the Kuwae tephra layer (Supplementary Information). We followed a standard procedure to extract and count pollen and spores (Erdtman and Wodehouse, 1944).
Pollen grains were counted and identified at × 400 magnification with detailed identification and photography of grains at × 1000 magnification (Supplementary Information). Unidentified types were described, photographed, and assigned a code; obscured or damaged grains were grouped as unknown. Poaceae pollen grains were grouped as either <40 μm, >40 μm, >60 μm or as multiporate grains. We used the Australian National University (2007) Australasian Pollen and Spore Atlas to identify the pollen and spores.
Micro-particles of charcoal were quantified from 32 samples by counting in the size range 10–125 μm alongside exotic Lycopodium spores and pollen on pollen slides (Wang et al., 1999). Particles were identified in the pollen slides as opaque, black, and angular fragments. Macro-charcoal samples were prepared from the >125-μm sieved fraction created in pollen preparation and were bleached with 6% H2O2 until the reaction stopped. All macro-charcoal fragments in each 1 cm3 sample were counted using a stereo microscope (Whitlock and Larsen, 2001).
The head capsules of chironomid larvae were extracted by disaggregating 2 cm3 samples of sediment with 10% KOH (following Brooks et al., 2007). The disaggregated samples were then sieved to <90 μm. The head capsules were then picked and mounted with Hydro-Matrix onto microscope slides. The counting and identification was done at × 400 magnification and taxonomically identified using Brooks et al. (2007) and Andersen et al. (2013), and more specifically with a focus on the Southern hemisphere, following Dieffenbacher-Krall et al. (2007) and the New Zealand subfossil chironomid taxonomy which can be found at https://climatechange.umaine.edu/perl/nzguide.html. Ten samples were analysed, with an average temporal resolution of c. 200 years.
To identify the occurrence and timing of transitions, we created the pollen and spores and chironomid diagrams using Tilia 2.9.1 software and numerically zoned them using depth-constrained cluster analysis (CONISS) (Grimm, 1987; Grimm, 1990). All pollen taxa with an abundance of >1%, apart from unknown types, were included in the CONISS calculations. The same analysis was carried out for the chironomid data. In addition, the arboreal (trees and shrubs) to non-arboreal (herbs) pollen ratio was calculated for all depths.
Using pollen and spore percentages and chironomid percentages we performed detrended correspondence analysis (DCA) in R (RStudio Team, 2015; R Core Team, 2017) with the vegan package (Oksanen et al., 2007) with the assumption that the DCA axis 1 scores represent turnover (Bush et al., 2004; Blarquez et al., 2014; Castilla-Beltrán et al., 2021a,Nogué et al., 2021). Unknown pollen types, which were assigned a code (see section “Supplementary Information”), were included in the DCA. DCA axis 1 scores were used since they are considered optimal for analysing percentage data which contains many zeros (Hill and Gauch, 1980).
Canonical correspondence analysis (CCA) was conducted on the pollen and spore percentage data also using the vegan R package. Micro-charcoal, macro-charcoal (regional and local fire, respectively), reconstructed precipitation rate (mm day–1), Ti/incoherent scatter (erosion), and magnetic susceptibility (tephra and erosional events) were incorporated into the CCA as environmental variables. To fill data gaps in the precipitation rate dataset (to facilitate comparisons with the other proxies) we interpolated between data points. See Maloney et al. (2022) for how precipitation rate values were calculated.
The opportunity to carry out a detailed tephra analysis allowed us to assign the source of the main tephra horizon in the record with some confidence. Samples between 166 and 160 cm contain dominantly clear, vesicular, angular, and un-weathered volcanic glass, with subordinate brown fresh volcanic glass, feldspar, and pyroxene grains (with adhering glass). The coarsest particles of 2–2.5 mm diameter, and highest concentration of volcanic particles were in the 162–160 cm core segment. While we recognise that a firm identification requires further data, the glass texture, associated minerals, and glass major-element composition shows a good match to glass analysed on the same instrument in this study from near-source sites around the Kuwae Caldera (Figure 2 and Supplementary Information). The age-depth model (Supplementary Information) suggests that the 6 cm layer of Kuwae tephra was deposited 650–510 cal. years BP (95% confidence) which is close to estimates of the Kuwae eruption occurring in the 1450s CE (Gao et al., 2006; Plummer et al., 2012).
Figure 2. Major element composition of the volcanic glass identified between depth compared to known sources where possible.
The sample from 169 to 167 cm contained mainly weathered and highly altered crystalline rock fragments (andesitic to mafic based on mineralogy) as well as rare fragments of clear and highly vesicular glass. The composition of these rare glass fragments is not similar to the Kuwae tephra, but instead matches the Efate Pumice Formation tephra (∼1.7 Ma; Stewart et al., 2010), which is a common contributor to colluvial and alluvial sediments on Efate (Figure 2). These early Pleistocene glass and older weathered lithic grains from basement rocks, cf. Ash et al. (1978), were likely reworked into the lake following earthquakes or during major rain events or cyclones. The 173–171 cm samples contained no fresh glass, but inorganic particles were dominated by weathered and altered crystalline rock fragments (Figure 2). Most compositional analysis was unsuccessful on this material, but two analyses showed an andesitic composition, consistent with basement rock on Efate (Ash et al., 1978).
Over 100 pollen types were identified, around 5% of which were assigned a code since they could not be identified. The pollen diagram is divided into four pollen assemblage zones based on the CONISS dendrogram (Figure 3). The following percentage values are calculated as means for each zone.
Figure 3. Pollen percentage diagram for Lake Emaotul showing pollen and spores that have been taxonomically identified and have >1% abundance in any one sample. Taxa are grouped into trees and shrubs (green), dryland herbs (red), wetland herbs (yellow), other herbs (orange), aquatic plants (blue), and ferns and fern allies (brown). Taxa that occur in low abundances have been shown with × 5 exaggeration (paler shaded areas). The purple-shaded area represents the area where Kuwae volcanic tephra was identified, and the pale blue shaded area is the period of climate shift from wetter to drier conditions.
Zone one (341–258 cm, >1620–1120 cal. years BP) comprises of nine samples and is dominated by secondary forest taxa Acalypha (30%), Pandanus (4%), Macaranga (20%) Trema (13%), and Homalanthus (1%) with occurrences of Poaceae (7%), Cyperaceae (2%), trilete psilate fern spores (7%), and monolete psilate fern spores (1%).
Zone two (258–155 cm, 1120–560 cal. years BP) consists of 13 samples and has relatively high proportions of Acalypha (21%), Macaranga (19%), Pandanus (6%), Trema (9%), and Homalanthus (3%). Cyperaceae (4%), Poaceae (8%), psilate trilete spores (12%), psilate monolete spores (2%) also exhibit relatively higher proportions.
Zone three (155–24.5 cm, 560 cal. years BP to 1930 CE) consists of seven samples and shows a decline in secondary forest taxa Acalypha (to 16%) Trema (to 6%), Macaranga (to 16%) and an increase in Persicaria-type (to 2%), Poaceae (to 17%), Nymphoides (to 1%), Pandanus (to 9%), and trilete psilate spores (to 13%). Cyperaceae remains at 4% and monolete psilate fern spores declines to 1%.
Zone four (24.5–1 cm, 1930–2015 CE) consists of three pollen samples. In this zone, Acalypha Trema, Macaranga, and Pandanus decline to 12, 0.5, 14, and 4%, respectively. Herbaceous taxa Asteraceae (5% abundance), Persicaria-type (2% abundance), and Poaceae (17% abundance) increase. The aquatic plant Nymphoides increases to 2%, as do monolete (to 2%) and trilete fern spores (to 26%) whereas Cyperaceae remains at 4%.
Ten chironomid taxa were identified. The chironomid diagram (Figure 4) is divided into three zones using CONISS (Grimm, 1987).
Figure 4. Chironomid percentage diagram, the period of climate transition from wetter to drier is shown in pale blue and the Kuwae tephra is shown as a purple shaded area.
Zone one (357–252 cm, >1600–1090 cal. years BP) consists of four samples and is dominated by Procladius (46%) and Tanytarsus funebris- type (41%).
Sub-zone two (252–221.5 cm, 1090–910 cal. years BP) consists of one chironomid sample and has high proportions of Chironomus anthracinus-type (52%) and Orthocladiinae type 1 (similar to Pseudorthocladius) (8%). Procladius and Tanytarsus funebris- type decline to 4 and 24%, respectively.
Zone three (221.5–61 cm, 910–160 cal. years BP) consists of five samples and is similar to zone one where Procladius increases to 36% and Tanytarsus funebris- type increases to 55%. Chironomus anthracinus-type decreases again to 2%.
The core stratigraphy consisted of gyttja throughout. We structured this section following the pollen zones from Figure 3 (red dashed line). Micro-charcoal concentrations, as shown in Figure 5, are variable in pollen zone one and the mean value is 1,300,000 particles per cm3, macro-charcoal concentrations are lower (7 particles per cm3). In pollen zone two, micro-charcoal concentrations are relatively lower (1,190,000 particles per cm3) and macro-charcoal particles are still 7 particles per cm3. In pollen zone three, micro-charcoal particle concentrations decrease again to 790,000 particles per cm3 but there is a relative increase in macro-charcoal particle concentrations to 14 particles per cm3. In pollen zone four, micro-charcoal particle concentrations remain relatively low at 205,000 particles per cm3 but macro-charcoal concentrations increase to 57 particles per cm3. The ratio of arboreal to non-arboreal pollen varies throughout the record (between 0.4 and 1.96). The most notable shifts in the ratio occur after the Kuwae eruption and in the upper part of the record (pollen zone four). Following the Kuwae eruption, AP/NAP increases and then declines after ∼20 years. The lowest AP/NAP is within pollen zone four. The C/N ratio fluctuates thorough the record but does not display any change during the shift to drier conditions (Figure 5). However, the ratio largely varies around between 12 and 16 with one notable decline to 7.8 at 185 cm (∼700 cal. years BP), prior to the Kuwae eruption suggesting a greater proportion of algal material.
Figure 5. Summary diagram of methods used in the study. From left to right: magnetic susceptibility (tephra/erosion), Ti/incoherent scatter (erosion), reconstructed precipitation rate mm day– 1 and 2Hdinosterol all redrawn from Maloney et al. (2022). What follows is new data from this study; micro- and macro charcoal particle concentrations per cm3, indicating regional and local fires. Arboreal pollen/non-arboreal pollen ratio (AP/NAP) indicating forest openness, C/N indicating organic matter source, and chironomid and vegetation turnover (DCA axis 1 scores). The purple shaded area represents samples where Kuwae volcanic tephra is present. The blue shaded area represents the transition from wetter to drier conditions during the onset of the 1100 cal. years BP shift to drier climate conditions. The red dashed lines are consistent with zonal divisions within the pollen diagram. The mean temporal resolution, where 1 cm of sediment represents 5 years (1–8 years range) for all the palaeoecological proxies is shown in the Supplementary Information. The mean error for each 1 cm slice of the core was 140 years (95% confidence ranges, see Supplementary Information).
We used a turnover measure (DCA axis 1) to assess the degree of change in the composition of both chironomids and pollen due to the shift to drier conditions (Maloney et al., 2022) and the Kuwae eruption (Figure 5). Both chironomid and vegetation turnover increased during the shift toward drier conditions ∼1100 cal. years BP. While chironomid turnover showed no change following the Kuwae eruption, the vegetation values indicate greater taxonomic turnover following the eruption. There was also a sharp increase and then decline in the arboreal to non-arboreal pollen ratio (AP/NAP) following the eruption.
Canonical correspondence analysis (CCA) was used to determine which environmental drivers have dominant associations with each pollen zone. CCA axis 1 explained 39% of the variance (eigenvalue 0.05) and CCA axis 2 explained 22% (eigenvalue 0.04) (Figure 6). Environmental variables on the positive side of CCA axis 1 include regional fires, precipitation rate (mm day –1), erosion (Ti/incoherent scatter), and tephra/erosional events indicated by magnetic susceptibility (correlation scores 0.47, 0.61, 0.9, 0.01, respectively, see section “Supplementary Information”). One environmental variable, macro-charcoal particles (local fires), was located on the negative side of CCA axis 1 (correlation score −0.5). Pollen taxa from zone one (in red) are mostly positioned in the positive half of the CCA axis 1 and associated with precipitation and erosion. Pollen zone two (in orange) transitions from the positive to the negative half of CCA axis 1 with tephra/erosion (magnetic susceptibility) as the most influential environmental variable of this period and finally pollen zones three (in green) and four (in blue), which occur after 560 cal. years BP, are found in the negative side of CCA axis 1 and explained by local fires.
Figure 6. Canonical correspondence analysis (CCA) of pollen percentages. Environmental variables include regional fires (micro-charcoal), local fires (macro-charcoal), precipitation rate (mm day– 1), erosion (Ti/incoherent scatter), and volcanic eruptions/erosion (magnetic susceptibility). Pollen assemblages are coloured according to their zones.
Drought in the SW Pacific islands is currently considered a threat to both island ecosystems and their human societies (e.g., Sear et al., 2020). After ∼1100 cal. years BP, there was a shift toward drier climate conditions (Figure 5; Maloney et al., 2022). Precipitation values changed from around 6.8 mm day–1 to 4.4 mm day–1 over a period of ∼70 years (Maloney et al., 2022), a significant shift in moisture availability. Both vegetation and chironomid turnover temporarily increased across the transition from wetter to drier climate conditions (Figure 5). The vegetation change was characterised by an increase in Cyperaceae, Poaceae, and ferns, which then declined to previous values within ∼80 years (Figure 3). According to the CCA analysis, precipitation, and erosion plot with samples from pollen zone one (>1620–1120 cal. years BP) prior to the change to drier conditions, linking both environmental drivers of change as the most significant. Indeed, erosion (Ti/inc) is well known to be linked with sedimentary in-wash (e.g., Sear et al., 2020), which here correlates with higher precipitation. While there is a higher turnover at the transition to drier conditions, the individual taxon changes are subtle and somewhat transient (Figure 5).
The changes in the chironomid community show a strong response to the moisture shift, then recovery, but with different abundances of the dominant taxa than prior to the shift. While all main taxa declined during the transition, Chironomus anthracinus type increased; this caused high turnover in the short term (Figures 4, 5). Chironomus anthracinus is known to be able to withstand low levels of oxygen and can tolerate unfavourable conditions (Hamburger et al., 1994, 1995). The increase in Chironomus anthracinus and relative decrease in other taxa may suggest a decrease in oxygen or even anoxia following the climate shift. Turnover decreased within ∼135 years, despite climate conditions remaining relatively dry. The C/N ratio shows no clear response to the climate transition, indicating that there was no shift in the balance of terrestrial and algal input to the lake with the shift to drier conditions. This possibly suggests that the overall biomass in the catchment did not change much, and also that the organic input to the lake was not strongly driven by precipitation-driven in-wash.
The Kuwae eruption is considered one of the largest eruptions of the last millennium (Neukom et al., 2014), and it had far more impact on the forest of Efate than did the moisture change. The CCA picks out the Kuwae eruption as the most significant association with the fossil pollen assemblage in pollen zone two (1120–560 cal. years BP) (Figure 6). It is notable that vegetation turnover not only increased sharply after the Kuwae eruption, but it remained high throughout the subsequent record (Figure 5). It is generally accepted that after a volcanic eruption there may be changes in vegetation composition, such as an increase in grass abundance (see examples in “Supplementary Information”). In Efate, after the Kuwae eruption sequence, an increase in grass pollen (Poaceae) is linked with a decline in AP/NAP, suggesting increased occurrence of open patches (Figure 5).
The tree and shrub post-eruption dynamics show Acalypha (native shrub or small tree) and Trema (native tree) decreasing within ∼20 years of the deposition of the Kuwae tephra, while Persicaria-type, Solanaceae, and Nymphoides increased together with grasses (Figure 3). Initially, damage to small trees and shrubs such as Acalypha and Trema could have created space for grasses and sedges to expand, and the increase in grasses may also be explained by its relatively short generation time compared with woody vegetation, which gives the group the ability to profit relatively quickly from nutrient enrichment following volcanic eruptions (Urrutia et al., 2007). It is important to note, however, that our results show that the forests surrounding Lake Emaotul were already disturbed at the start of this record, as indicated by the presence of Acalypha, Macaranga, and Trema, which are considered secondary, disturbance, and early successional taxa (Whittaker et al., 1989; Fall, 2010; Åkesson et al., 2021) and the continuous presence of fire, which is often associated with human occupation on Pacific islands (e.g., Fall, 2005; Stevenson et al., 2017). Although our results show that the Kuwae eruption impacted on the forest surrounding Lake Emaotul, local human impacts during the period of the Kuwae eruption cannot be ruled out as a driver for change. For example, there is archaeological evidence that human populations moved southwards from Sheppard and Epi islands to Efate following the eruption (Garanger, 1972), and an increase in population size could have led to some level of deforestation. However, there is no clear link between the declining taxa (Acalypha and Trema) and known human uses of them.
Despite numerous examples of change in vegetation composition (Supplementary Information), recovery rates of vegetation following volcanic eruptions are poorly understood. Palaeoecological evidence of tephra deposition events have typically shown short-lived impacts (decades to centuries, e.g., Lotter and Birks, 1993; Montoya et al., 2021). For example, the main patterns that emerge from analysing large datasets of pollen time-series from tropical forests suggest that forest recovery rates are faster for large, infrequent events, such as volcanic eruptions, than for human-induced burning (Cole et al., 2014). The analysis by Cole et al. (2014) also suggests that arboreal taxa typically recover to ∼95% abundance after >200 years. On Efate, the arboreal taxa Acalypha and Trema had largely recovered their pre-eruption abundance in ∼260 years. Prior to the eruption, Acalypha and Trema values were 23 and 9% of the total pollen sum, respectively. After 260 years, they reached 19 and 11%, respectively. These two taxa responded differently to other arboreal taxa such as Macaranga, Pandanus, Sapindaceae, and Sapotaceae, which showed no obvious decline after the eruption. Despite the partial recovery of Acalypha and Trema, turnover remained higher for all taxa ∼625 years following the eruption (Figure 5).
While the forests of Efate showed changes linked to the volcanic eruption, Lake Emaotul chironomid communities remained unperturbed (Figure 4). Addition of volcanic tephra of basaltic/andesitic composition to the lake could be expected to deliver nutrients, including substantial S, Fe, and other cations (Cronin et al., 1998), and this may have led to algal blooms (e.g., Harper et al., 1986; Roberts et al., 1997; Eastwood et al., 2002; Telford et al., 2004), which might be expected to show as a change in C/N. The Emaotul C/N record shows short-term variability, but no clear directional change is evident following the eruption, with ratio values before and after being ∼13 (Figure 5), a value indicative of a mixed source of organic matter (García-Alix et al., 2012); if there was a post-eruption increase in algal biomass, it is not detectable. As suggested for forested sites in Ecuador (see Montoya et al., 2021), the dense canopy of the forest trees surrounding Lake Emaotul may have partially shielded aquatic ecosystems from major impact by blocking some airborne tephra fall on to the lakes surface. The inconsistent responses of algal productivity to volcanic tephra-fallout recorded to date suggest site- or catchment-specific factors may be important in determining responses. At Lake Emaotul, chironomids also showed no significant change following the eruption. Either they recovered so quickly that changes were not identified in this record or they are not sensitive to volcanic tephra inputs at this site.
Archaeological evidence indicates that people had been actively modifying the landscape of Efate ∼1400 years prior to the start of this record (1600 cal. years BP), since the first human settled Vanuatu ∼3000 years ago (Petchey et al., 2014; Bedford et al., 2018). Notably, the CCA analysis (Figure 6) shows an association between samples from the period 560 cal. years BP to 1950 CE and local fires, indicating that the local use of fires, suggested by the increase in macro-charcoal particles (Figure 5) could have been an important driver of change (Clark, 1988; Whitlock and Millspaugh, 1996). During pollen zone four (1930–2015 CE), an increase in Asteraceae, Poaceae, Solanaceae, and Persicaria-type pollen, and fern spores, a decrease in woody taxa (e.g., Acalypha and Trema), together with higher concentrations of macro-charcoal concentrations spanning the last ∼90 years is strongly indicative of opening of the forest and transition toward the wetland areas and secondary forests we see today surrounding Lake Emaotul. These findings agree with other studies from Vanuatu; for example, human impacts are seen in pollen diagrams from ∼3000 cal. years BP onward from Aneityum island, Southern Vanuatu (∼320 km south of Efate). At Aneityum island, tree and shrub taxa (Eugenia, Celtis, and Trema) were replaced by grasses (Poaceae), sedges (Cyperaceae), ferns, accompanied by an increase in charcoal particles (Hope and Spriggs, 1982). Another example is the palaeoecological record from the island of Erromango (Figure 1, ∼150 km south of Efate), which shows evidence of burning throughout a 2000 year period during which humans were present (Hope et al., 1999). Human impacts may have been present prior to the last 1600 years, but despite their apparent impacts, the transition to drier climate ∼1100 cal. years BP and the Kuwae eruption were both strong enough disturbances to affect the vegetation surrounding Lake Emaotul.
It can be difficult to isolate and disentangle multiple drivers of change and their effects on an ecosystem when they occur close together in time. Efate has been affected by a major volcanic tephra fall and a marked climate transition. The Kuwae eruption (650–510 cal. years BP) and the transition from wetter to drier conditions occurring ∼1100–1000 cal. years BP are, however, well separated in time and both post-date settlement, which provides the opportunity to examine each in turn (while assuming no major change in human impact). The Kuwae eruption appears to have had a more lasting impact on vegetation than the period of climate change. In contrast, the aquatic ecosystem, as represented by chironomid assemblages, appears more sensitive to the ∼1100 cal. years BP shift to drier conditions than to the Kuwae eruption. While precipitation changes and the Kuwae eruption explained more variance in the pollen and spore data prior to ∼560 cal. years BP, local scale burning seems to have had a more important role within the last few centuries, over which there has been a major change in vegetation composition. Understanding legacies of volcanic and climatic impacts is relevant for the sustainable management of the islands and archipelagos natural resources.
The original contributions presented in this study are included in the article/Supplementary material, further inquiries can be directed to the corresponding author.
NS and SN contributed to conception and design of the study. NS wrote first draft of the manuscript and created the figures. SC, CL, AM, SB, TB, IC, ML, and JS contributed with sediment analyses. WG and MP helped with the content and structure of the manuscript. All authors contributed to manuscript revision, read, and approved the submitted version.
This work was supported by the Natural Environment Research Council (Grant Number NE/L002531/1). Cronin acknowledges support of the Australian Research Council (Grant Number DP200102320).
We thank Kenny Horkley (U. Iowa) and David Adams (U. Auckland) for their assistance with conducting electron microprobe analysis. Non-pollen datasets were generated with support of NERC Grant NE/N006674/1. 137Cs and 210Pb radioisotope dating was undertaken by GAU-Radioanalytical. Radiocarbon dates were provided by Direct-AMS, two funded by an Integrative Graduate Education and Research Traineeship Programme on Ocean Change Mini Grant (to AM). Thanks to BOSCORF for the Itrax data. We would like to thank Esline Garaebiti, Philip Malsale, and Alan Rarai of the Vanuatu Meteorology and Geo-Hazards Department of the Government of Vanuatu for their permission and support for this research, to the Ni-Vanuatu people who gave their permission to work on the lake, in particular Pastor Peter Kalmas. The staff at Aquana Beach Resort for their help with last minute logistics. Many thanks to Dr. Ignacio Jara Parra for assistance visualising the GPCP satellite-gauge data (Supplementary Information) and Dr. Alvaro Castilla-Beltrán for assistance with pollen grain identifications and statistical analyses. Huge thanks to Charlotte Hipkiss for help with the manuscript and useful discussions. Thanks to Alistair Monteath for helpful discussions about the tephra.
The authors declare that the research was conducted in the absence of any commercial or financial relationships that could be construed as a potential conflict of interest.
All claims expressed in this article are solely those of the authors and do not necessarily represent those of their affiliated organizations, or those of the publisher, the editors and the reviewers. Any product that may be evaluated in this article, or claim that may be made by its manufacturer, is not guaranteed or endorsed by the publisher.
The Supplementary Material for this article can be found online at: https://www.frontiersin.org/articles/10.3389/fevo.2023.1087577/full#supplementary-material
Åkesson, C. M., Mcmichael, C. N. H., Raczka, M. F., Huisman, S. N., Palmeira, M., Vogel, J., et al. (2021). Long-term ecological legacies in western Amazonia. J. Ecol. 109, 432–446. doi: 10.1111/1365-2745.13501
Allen, J. R. M., and Huntley, B. (2018). Effects of tephra falls on vegetation: A late-quaternary record from southern Italy. J. Ecol. 106, 2456–2472. doi: 10.1111/1365-2745.12998
Andersen, T., Ekrem, T., and Cranston, P. (2013). “The larvae of the holarctic chironomidae – introduction,” in The larvae of Chironomidae (Diptera) of the Holarctic region – Keys and diagnoses, Vol. 66, eds T. Andersen, P. S. Cranston, and J. H. Epler (Lund: Insect Systematics & Evolution, Supplement), 7–12.
Arnalds, O. (2013). The influence of volcanic tephra (ash) on ecosystems. Adv. Agron. 121, 331–380. doi: 10.1016/B978-0-12-407685-3.00006-2
Ash, R. P., Carney, J. N., and Macfarlane, A. M. (1978). Geology of Efate and offshore islands. New Hebrides Geol. Surv. Reg. Rep. 49.
Australian National University (2007). The Australasian pollen and spore atlas V1.0. Canberra: Australian National University.
Bedford, S., and Spriggs, M. (2014). “The archaeology of vanuatu: 3000 years of history across Islands of Ash and Coral,” in The oxford handbook of prehistoric Oceania, eds E. Cochrane and T. Hunt (Oxford: Oxford University Press).
Bedford, S., Siméoni, P., and Lebot, V. (2018). The anthropogenic transformation of an island landscape: Evidence for agricultural development revealed by LiDAR on the island of Efate, Central Vanuatu, South-West Pacific. Archaeol. Ocean. 53, 1–14. doi: 10.1002/arco.5137
Blaauw, M., Christen, J. A., Lopez, M. A. A., Vazquez, J. E., Belding, T., Theiler, J., et al. (2021). Package “rbacon”.
Blarquez, O., Carcaillet, C., Frejaville, T., and Bergeron, Y. (2014). Disentangling the trajectories of alpha, beta and gamma plant diversity of North American boreal ecoregions since 15,500 years. Front. Ecol. Evol. 2:6. doi: 10.3389/fevo.2014.00006
Brooks, S. J., Langdon, P. G., and Heiri, O. (2007). The identification and use of palaearctic chironomidae larvae in palaeoecology. QRA technical guide. London: Quaternary Research Association.
Bush, M. B., Silman, M. R., and Urrego, D. H. (2004). 48,000 years of climate and forest change in a biodiversity hot spot. Science 303, 827–829. doi: 10.1126/science.1090795
Castilla-Beltrán, A., de Nascimento, L., Fernández-Palacios, J. M., Whittaker, R. J., Romeiras, M. M., Cundy, A. B., et al. (2021b). Effects of Holocene climate change, volcanism and mass migration on the ecosystem of a small, dry island (Brava, Cabo Verde). J. Biogeogr. 48, 1392–1405. doi: 10.1111/jbi.14084
Castilla-Beltrán, A., de Nascimento, L., Fernández-Palacios, J.-M., Whittaker, R. J., Willis, K. J., Edwards, M., et al. (2021a). Anthropogenic transitions from forested to human-dominated landscapes in southern Macaronesia. Proc. Natl. Acad. Sci. U.S.A. 118:e2022215118. doi: 10.1073/pnas.2022215118
Cillaurren, E., David, G., Grandperrin, R., and Doumenge, F. (2001). Atlas des pêcheries côtières de Vanuatu: un bilan décennal pour le développement= Coastal fisheries atlas of Vanuatu: a 10-year development assessment.
Clark, J. (1988). Particle motion and the theory of charcoal analysis: Source area, transport, deposition, and sampling. Quat. Res. 30, 67–80. doi: 10.1016/0033-5894(88)90088-9
Cole, L. E., Bhagwat, S. A., and Willis, K. J. (2014). Recovery and resilience of tropical forests after disturbance. Nat. Commun. 5, 1–7. doi: 10.1038/ncomms4906
Cole-Dai, J., Mosley-Thompson, E., Wight, S. P., and Thompson, L. G. (2000). A 4100-year record of explosive volcanism from an East Antarctica ice core. J. Geophys. Res. 105, 24431–24441. doi: 10.1029/2000JD900254
Combettes, C., Semah, A. M., and Wirrmann, D. (2015). High-resolution pollen record from Efate Island, central Vanuatu: Highlighting climatic and human influences on Late Holocene vegetation dynamics. C. R. Palevol 14, 251–261. doi: 10.1016/j.crpv.2015.02.003
Cronin, S. J., Hedley, M. J., Neall, V. E., and Smith, R. G. (1998). Agronomic impact of tephra fallout from the 1995 and 1996 Ruapehu Volcano eruptions, New Zealand. Environ. Geol. 34, 21–30. doi: 10.1007/s002540050253
Cronin, S. J., Neall, V. E., Lecointre, J. A., and Palmer, A. S. (1997). Changes in Whangaehu river lahar characteristics during the 1995 eruption sequence, ruapehu volcano, New Zealand. J. Volcanol Geothermal Res. 76, 47–61. doi: 10.1016/S0377-0273(96)00064-9
Dale, V. H., Delgado-Acevedo, J., and Macmahon, J. (2005). “Effects of modern volcanic eruptions on vegetation,” in Volcanoes and the environment, eds G. G. J. Ernst and J. Marti (Cambridge: Cambridge University Press). doi: 10.1017/CBO9780511614767.009
Dieffenbacher-Krall, A. C., Vandergoes, M. J., and Denton, G. H. (2007). An inference model for mean summer air temperatures in the Southern Alps, New Zealand, using subfossil chironomids. Quat. Sci. Rev. 26, 2487–2504. doi: 10.1016/j.quascirev.2007.06.016
Eastwood, W. J., Tibby, J., Roberts, N., Birks, H. J. B., and Lamb, H. F. (2002). The environmental impact of the Minoan eruption of Santorini (Thera): Statistical analysis of palaeoecological data from Gölhisar, southwest Turkey. Holocene 12, 431–444. doi: 10.1191/0959683602hl557rp
Erdtman, G., and Wodehouse, R. P. (1944). An introduction to pollen analysis. Philadelphia: LWW. doi: 10.1097/00010694-194403000-00006
Fall, P. L. (2005). Vegetation change in the coastal-lowland rainforest at Avai’o”vuna Swamp, Vava’u, Kingdom of Tonga. Quat. Res. 64, 451–459. doi: 10.1016/j.yqres.2005.08.003
Fall, P. L. (2010). Pollen evidence for plant introductions in a polynesian tropical island ecosystem, Kingdom of Tonga. Altered Ecol. 32, 253–271. doi: 10.22459/TA32.11.2010.14
Gao, C., Robock, A., Self, S., Witter, J. B., Steffenson, J. P., Clausen, H. B., et al. (2006). The 1452 or 1453 A.D. Kuwae eruption signal derived from multiple ice core records: Greatest volcanic sulfate event of the past 700 years. J. Geophys. Res. 111:D12107. doi: 10.1029/2005JD006710
Garanger, J. (1972). Archéologie des Nouvelles-Hébrides: Contribution à la connaissance des îles du Centre. Paris: Société des Océanistes. doi: 10.4000/books.sdo.859
García-Alix, A., Jiménez-Moreno, G., Anderson, R. S., Jiménez Espejo, F. J., and Delgado Huertas, A. (2012). Holocene environmental change in southern Spain deduced from the isotopic record of a high-elevation wetland in Sierra Nevada. J. Palaeolimnol. 48, 471–484. doi: 10.1007/s10933-012-9625-2
Gosling, W. D., de Kruif, J., Norder, S. J., de Boer, E. J., Hooghiemstra, H., Rijsdijk, K. F., et al. (2017). Mauritius on fire: Tracking historical human impacts on biodiversity loss. Biotropica 49, 778–783. doi: 10.1111/btp.12490
Grimm, E. (1990). TILIA and TILIA* GRAPH: PC spreadsheet and graphics software for pollen data. INQUA working group on data-handling methods, Newsletter, Vol. 4. 5e7.
Grimm, E. C. (1987). Coniss – a Fortran-77 program for stratigraphically constrained cluster-analysis by the method of incremental sum of squares. Comput. Geosci. 13, 13–35. doi: 10.1016/0098-3004(87)90022-7
Hamburger, K., Dall, P. C., and Lindegaard, C. (1994). Energy metabolism of Chironomus anthracinus (Diptera: Chironomidae) from the profundal zone of Lake Esrom, Denmark, as a function of body size, temperature and oxygen concentration. Hydrobiologia 294, 43–50. doi: 10.1007/BF00017624
Hamburger, K., Dall, P. C., and Lindegaard, C. (1995). Effects of oxygen deficiency on survival and glycogen content of Chironomus anthracinus (Diptera, Chironomidae) under laboratory and field conditions. Hydrobiologia 297, 187–200. doi: 10.1007/BF00019284
Harper, M. A., Howorth, R., and Mcleod, M. (1986). Late holocene diatoms in lake poukawa: Effects of airfall tephra and changes in depth. N. Z. J. Mar. Freshw. Res. 20, 107–118. doi: 10.1080/00288330.1986.9516135
Hartman, L. H., Kurbatov, A. V., Winski, D. A., Cruz-Uribe, A. M., Davies, S. M., Dunbar, N. W., et al. (2019). Volcanic glass properties from 1459 C.E. volcanic event in South Pole ice core dismiss Kuwae caldera as a potential source. Sci. Rep. 9:14437. doi: 10.1038/s41598-019-50939-x
Hassall, J. D. (2017). Static or dynamic : reconstructing past movement of the South Pacific Convergence Zone. Thesis (Ph D) – University of Southampton, Faculty of Social, Human and Mathematical Sciences, 2017, Original typescript. Ph.D. thesis.
Hickman, M., and Reasoner, M. A. (1994). Diatom responses to late Quaternary vegetation and climate change, and to deposition of two tephras in an alpine and a sub-alpine lake in Yoho National Park, British Columbia. J. Paleolimnol. 11, 173–188. doi: 10.1007/BF00686864
Hill, M. O., and Gauch, H. G. (1980). “Detrended correspondence analysis: an improved ordination technique,” in Classification and ordination. Advances in vegetation science, ed. E. van der Maarel (Dordrecht: Springer). doi: 10.1007/978-94-009-9197-2_7
Hoffmann, A. (2007). Looking to Epi: Further consequences of the Kuwae eruption, central Vanuatu, AD 1452. Bull Indo Pac. Prehist. Assoc. 26, 62–71. doi: 10.7152/bippa.v26i0.11994
Hogg, A. G., Heaton, T. J., Hua, Q., Palmer, J. G., Turney, C. S. M., Southon, J., et al. (2020). SHCal20 Southern hemisphere calibration, 0–55,000 Years cal BP. Radiocarbon 62, 759–778. doi: 10.1017/RDC.2020.59
Hope, G., and Spriggs, M. (1982). A preliminary pollen sequence from Aneityum Island, Southern Vanuatu. Bull. Indo Pac. Prehist. Assoc. 3, 88–94. doi: 10.7152/bippa.v3i0.11194
Hope, G., O’DEA, D., and Southern, W. (1999). “Holocene vegetation histories in the Western Pacific : alternative records of human impact,” in Le Pacifique de 5000 à 2000 avant le présent : suppléments à l’histoire d’une colonisation The Pacific from 5000 to 2000 BP : colonisation and transformations Conférence Lapita : Le Pacifique de 5000 à 2000 avant le Présent : Suppléments à l’Histoire d’une Colonisation, eds G. Jean-Christophe and I. Lilley (Port Vila, VAN) (Paris: Institut de Recherche Pour le Developpement).
Jones, T. D., Lawson, I. T., Reed, J. M., Wilson, G. P., Leng, M. J., Gierga, M., et al. (2013). Diatominferred late Pleistocene and Holocene palaeolimnological changes in the Ioannina basin, northwest Greece. J. Palaeolimnol. 49, 185–204. doi: 10.1007/s10933-012-9654-x
Kalfatak, D., and Jaensch, R. (2014). Directory of Wetlands of Vanuatu – 2014. Report to the secretariat of the pacific regional environment program.
Kier, G., Kreft, H., Lee, T. M., Jetz, W., Ibisch, P. L., Nowicki, C., et al. (2009). A global assessment of endemism and species richness across island and mainland regions. Proc. Natl. Acad. Sci. 106:9322. doi: 10.1073/pnas.0810306106
Lipson, M., Spriggs, M., Valentin, F., Bedford, S., Shing, R., Zinger, W., et al. (2020). Three phases of ancient migration shaped the ancestry of human populations in vanuatu. Curr. Biol. 30, 4846–4856.e6. doi: 10.1016/j.cub.2020.09.035
Losos, J. B., and Ricklefs, R. E. (2009). Adaptation and diversification on islands. Nature 457, 830–836. doi: 10.1038/nature07893
Lotter, A. F., and Birks, H. J. B. (1993). The impact of the Laacher See Tephra on terrestrial and aquatic ecosystems in the Black Forest, southern germany. J Quat. Sci. 8, 263–276. doi: 10.1002/jqs.3390080307
Mallick, D. (1975). Development of the New Hebrides archipelago. Philos. Trans. R. Soc. B 272, 277–285. doi: 10.1098/rstb.1975.0087
Maloney, A. E., Richey, J. N., Nelson, D. B., Hing, S. N., Sear, D. A., Hassall, J. D., et al. (2022). Contrasting common era climate and hydrology sensitivities from paired lake sediment dinosterol hydrogen isotope records in the south pacific convergence zone. Quat. Sci. Rev. 281:107421. doi: 10.1016/j.quascirev.2022.107421
Matthews-Bird, F., Brooks, S. J., Gosling, W. D., Gulliver, P., Mothes, P., and Montoya, E. (2017). Aquatic community response to volcanic eruptions on the Ecuadorian Andean flank: Evidence from the palaeoecological record. J. Paleolimnol. 58, 437–453. doi: 10.1007/s10933-017-0001-0
Mcnamara, K. E., and Prasad, S. S. (2014). Coping with extreme weather: Communities in Fiji and Vanuatu share their experiences and knowledge. Clim. Change 123, 121–132. doi: 10.1007/s10584-013-1047-2
Modenutti, B. E., Balseiro, E. G., Elser, J. J., Navarro, M. B., Cuassolo, F., Laspoumaderes, C., et al. (2013). Effect of volcanic eruption on nutrients, light, and phytoplankton in oligotrophic lakes. Limnol. Oceanogr. 58, 1165–1175. doi: 10.4319/lo.2013.58.4.1165
Montoya, E., Matthews-Bird, F., Brooks, S. J., and Gosling, W. D. (2021). Forests protect aquatic communities from detrimental impact by volcanic deposits in the tropical Andes (Ecuador). Reg. Environ. Change 21, 1–14. doi: 10.1007/s10113-021-01783-1
Mueller-Dombois, D. A., and Fosberg, F. R. (1998). Vegetation of the tropical Pacific islands. New York: Springer-Verlag. doi: 10.1007/978-1-4419-8686-3
Németh, K., Cronin, S. J., and White, J. D. (2007). Kuwae caldera and climate confusion. Open Geol. J. 1, 7–11. doi: 10.2174/1874262900701010007
Neukom, R., Gergis, J., Karoly, D. J., Wanner, H., Curran, M., Elbert, J., et al. (2014). Inter-hemispheric temperature variability over the past millennium. Nat. Clim. Change 4, 362–367. doi: 10.1038/nclimate2174
Nogué, S., Santos, A. M. C., Birks, H. J. B., Björck, S., Castilla-Beltrán, A., Connor, S., et al. (2021). The human dimension of biodiversity changes on islands. Science 372:488. doi: 10.1126/science.abd6706
Oksanen, J., Kindt, R., Legendre, P., O’HARA, B., Stevens, M. H. H., Oksanen, M. J., et al. (2007). The vegan package. Community Ecol. Package 10:719.
Pang, K. D. (1993). Climatic impact of the mid-fifteenth century Kuwae caldera formation, as reconstructed from historical and proxy data. Eos Trans. AGU 74:106.
Petchey, F., Spriggs, M., Bedford, S., Valentin, F., and Buckley, H. (2014). Radiocarbon dating of burials from the teouma lapita cemetery, Efate, Vanuatu. J. Archaeol. Sci. 50, 227–242. doi: 10.1016/j.jas.2014.07.002
Plummer, C. T., Curran, M. A. J., van Ommen, T. D., Rasmussen, S. O., Moy, A. D., Vance, T. R., et al. (2012). An independently dated 2000-yr volcanic record from Law Dome, East Antarctica, including a new perspective on the dating of the c. 1450s eruption of Kuwae, Vanuatu. Clim. Past Discuss. 8, 1567–1590. doi: 10.5194/cp-8-1929-2012
R Core Team (2017). R: A language and environment for statistical computing. Vienna: R Foundation for Statistical Computing.
Raos, A., and Mcphie, J. (2003). “The Submarine record of a large-scale explosive eruption in the vanuatu Arc: ~1 Ma Efate pumice formation,” in Explosive subaqueous volcanism, eds J. D. L. White, J. L. Smellie, and D. A. Clague (Washington DC: American Geophysical Union Geophysical Monograph Series), 273–283. doi: 10.1029/140GM18
Rice, D. L., and Hanson, R. B. (1984). A kinetic model for detritus nitrogen:role of the associated bacteria in nitrogen accumulation. Bull. Mar. Sci. 35, 326–340.
Roberts, N., Eastwood, W. J., Lamb, H. F., and Tibby, J. C. (1997). “The age and causes of mid-late Holocene environment change in southwest Turkey,” in Third millennium BC climate change and old world collapse. NATO ASI series, eds H. N. Dalfes, G. Kukla, and H. Weiss (Berlin: Springer). doi: 10.1007/978-3-642-60616-8_16
Robin, C., Monzier, M., and Eissen, J.-P. (1994). Formation of the mid-fifteenth century Kuwae caldera (Vanuatu) by an initial hydroclastic and subsequent ignimbritic eruption. Bull. Volcanol. 56, 170–183. doi: 10.1007/BF00279602
Sear, D. A., Allen, M. S., Hassall, J. D., Maloney, A. E., Langdon, P. G., Morrison, A. E., et al. (2020). Human settlement of East Polynesia earlier, incremental, and coincident with prolonged South pacific drought. Proc. Natl. Acad. Sci. U.S.A. 117, 8813–8819. doi: 10.1073/pnas.1920975117
Singh, K., Bule, L., Khan, M. G. M., and Ahmed, M. R. (2019). Wind energy resource assessment for Vanuatu with accurate estimation of Weibull parameters. Energy Explor. Exploitation 37, 1804–1832. doi: 10.1177/0144598719866897
Steadman, D. W., Pregill, G. K., and Burley, D. V. (2002). Rapid prehistoric extinction of iguanas and birds in Polynesia. Proc. Natl. Acad. Sci. U.S.A. 99, 3673–3677. doi: 10.1073/pnas.072079299
Stevenson, J., Benson, A., Athens, J. S., Kahn, J., and Kirch, P. V. (2017). Polynesian colonization and landscape changes on Mo’orea, French Polynesia: The lake temae pollen record. Holocene 27, 1963–1975. doi: 10.1177/0959683617715690
Stewart, R. B., Németh, K., and Cronin, S. J. (2010). Is Efate (Vanuatu, SW Pacific) a result of subaerial or submarine eruption? An alternative model for the 1 Ma efate pumice formation. Cent. Eur. J. Geosci. 2, 306–320. doi: 10.2478/v10085-010-0020-9
Taylor, F. W., Frohlich, C., Lecolle, J., and Strecker, M. (1987). Analysis of partially emerged corals and reef terraces in the central Vanuatu Arc: Comparison of contemporary coseismic and nonseismic with quaternary vertical movements. J. Geophys. Res. 92, 4905–4933. doi: 10.1029/JB092iB06p04905
Telford, R. J., Barker, P., Metcalfe, S., and Newton, A. (2004). Lacustrine responses to tephra deposition: Examples from Mexico. Quat. Sci. Rev. 23, 2337–2353. doi: 10.1016/j.quascirev.2004.03.014
Tyson, R. V. (1995). Sedimentary organic matter: organic facies and palynofacies. London: Chapman and Hall. doi: 10.1007/978-94-011-0739-6
Urrutia, R., Araneda, A., Cruces, F., Torres, L., Chirinos, L., Treutler, H. C., et al. (2007). Changes in diatom, pollen, and chironomid assemblages in response to a recent volcanic event in Lake Galletué (Chilean Andes). Limnologica 37, 49–62. doi: 10.1016/j.limno.2006.09.003
Vincent, D. G. (1994). The South-pacific convergence zone (Spcz) – a review. Mon. Weather Rev. 122, 1949–1970. doi: 10.1175/1520-0493(1994)122<1949:TSPCZA>2.0.CO;2
Wang, X., van der Kaars, S., Kershaw, P., Bird, M., and Jansen, F. (1999). A record of fire, vegetation and climate through the last three glacial cycles from Lombok Ridge core G6-4, eastern Indian Ocean, Indonesia. Palaeogeogr. Palaeoclimatol. Palaeoecol. 147, 241–256. doi: 10.1016/S0031-0182(98)00169-2
Whitlock, C., and Larsen, C. (2001). “Charcoal as a Fire Proxy,” in Tracking Environmental Change Using Lake Sediments: Terrestrial, Algal, and Siliceous Indicators, eds J. P. Smol, H. J. B. Birks, W. M. Last, R. S. Bradley, and K. Alverson (Dordrecht: Springer Netherlands). doi: 10.1007/0-306-47668-1_5
Whitlock, C., and Millspaugh, S. H. (1996). Testing the assumptions of fire-history studies: An examination of modern charcoal accumulation in Yellowstone National Park, USA. Holocene 6, 7–15. doi: 10.1177/095968369600600102
Whittaker, R. J., Bush, M. B., and Richards, K. (1989). Plant recolonization and vegetation succession on the Krakatau Islands, Indonesia. Ecol. Monogr. 59, 59–123. doi: 10.2307/2937282
Wilmshurst, J. M., and Mcglone, M. S. (1996). Forest disturbance in the central North Island, New Zealand, following the 1850 BP Taupo eruption. Holocene 6, 399–411. doi: 10.1177/095968369600600402
Wirrmann, D., Eagar, S. H., Harper, M. A., Leroy, E., and Semah, A. M. (2011). First insights into mid-Holocene environmental change in central Vanuatu inferred from a terrestrial record from Emaotfer Swamp, Efate Island. Quat. Sci. Rev. 30, 3908–3924.
Witter, J. B., and Self, S. (2007). The Kuwae (Vanuatu) eruption of AD 1452: Potential magnitude and volatile release. Bull. Volcanol. 69, 301–318.
Keywords: chironomids, fossil pollen, Kuwae eruption, South Pacific Convergence Zone (SPCZ), tephra, Vanuatu
Citation: Strandberg NA, Sear DA, Langdon PG, Cronin SJ, Langdon CT, Maloney AE, Bateman SL, Bishop T, Croudace IW, Leng MJ, Sachs JP, Prebble M, Gosling WD, Edwards M and Nogué S (2023) Island ecosystem responses to the Kuwae eruption and precipitation change over the last 1600 years, Efate, Vanuatu. Front. Ecol. Evol. 11:1087577. doi: 10.3389/fevo.2023.1087577
Received: 02 November 2022; Accepted: 30 January 2023;
Published: 23 February 2023.
Edited by:
Anna Maria Mercuri, University of Modena and Reggio Emilia, ItalyReviewed by:
Gill Plunkett, Queen’s University Belfast, United KingdomCopyright © 2023 Strandberg, Sear, Langdon, Cronin, Langdon, Maloney, Bateman, Bishop, Croudace, Leng, Sachs, Prebble, Gosling, Edwards and Nogué. This is an open-access article distributed under the terms of the Creative Commons Attribution License (CC BY). The use, distribution or reproduction in other forums is permitted, provided the original author(s) and the copyright owner(s) are credited and that the original publication in this journal is cited, in accordance with accepted academic practice. No use, distribution or reproduction is permitted which does not comply with these terms.
*Correspondence: Nichola A. Strandberg, bi5hLnN0cmFuZGJlcmdAc290b24uYWMudWs=
Disclaimer: All claims expressed in this article are solely those of the authors and do not necessarily represent those of their affiliated organizations, or those of the publisher, the editors and the reviewers. Any product that may be evaluated in this article or claim that may be made by its manufacturer is not guaranteed or endorsed by the publisher.
Research integrity at Frontiers
Learn more about the work of our research integrity team to safeguard the quality of each article we publish.