- 1Department of Biology, University of Ottawa, Ottawa, ON, Canada
- 2Department of Earth and Environmental Sciences, University of Ottawa, Ottawa, ON, Canada
- 3Great Lakes Forestry Centre, Canadian Forest Service, Natural Resources Canada, Sault Ste. Marie, ON, Canada
- 4Department of Integrative Biology, University of Guelph, Guelph, ON, Canada
- 5Institut Botànic de Barcelona (IBB), CSIC-Ajuntament de Barcelona, Barcelona, Catalonia, Spain
Anthropogenic activities are exposing insects to elevated levels of toxic metals and are altering the bioavailability of essential metals. Metals and metal isotopes have also become promising tools for the geolocation of migratory insects. Understanding the pathways of metal incorporation in insect tissues is thus important for assessing the role of metals in insect physiology and ecology and for the development of metals and metal isotopes as geolocation tools. We conducted a diet-switching experiment on monarch butterflies [Danaus plexippus (L.)] with controlled larval and adult diets to evaluate the sources of 23 metals and metalloids, strontium isotopes, and lead isotopes to insect wing tissues over a period of 8 weeks. Concentrations of Ca, Co, Mo, and Sb differed between the sexes or with body mass. Ni and Zn bioaccumulated in the insect wing tissues over time, likely from the adult diet, while increases in Al, Cr, Cd, Cu, Fe, and Pb were, at least partially, from external sources (i.e., dust aerosols). Bioaccumulation of Pb in the monarch wings was confirmed by Pb isotopes to mainly be sourced from external anthropogenic sources, revealing the potential of Pb isotopes to become an indicator and tracer of metal pollution exposure along migratory paths. Concentrations of Ba, Cs, Mg, Na, Rb, Sr, Ti, Tl, and U appeared to be unaffected by intrinsic factors or additions of metals from adult dietary or external sources, and their potential for geolocation should be further explored. Strontium isotope ratios remained indicative of the larval diet, at least in males, supporting its potential as a geolocation tool. However, the difference in strontium isotope ratios between sexes, as well as the possibility of external contamination by wetting, requires further investigation. Our results demonstrate the complexity of metal incorporation processes in insects and the value of studying metals to develop new tools to quantify pollution exposure, metal toxicity, micronutrient uptake, and insect mobility.
1. Introduction
Some metals are important micronutrients that sustain life while others have profound implications as toxic metals that reduce survival and fitness. Essential metals are required for metabolic functions such as that the organism cannot survive when the metal is removed from the diet. Essential metals participate in enzyme catalysis, protein structure, cellular signaling, and the creation of electrochemical potentials at membranes (Maret, 2016; Dow, 2017) and it is estimated that up to 30% of proteins are metalloproteins (Aptekmann et al., 2022). Within organisms, these essential metals follow a dose–response curve, in which low doses cause morbidity due to metal deficiency and high doses cause toxicological effects. Conversely, many non-essential metals are toxic even at low doses. However, despite the fundamental importance of metals, little is known about the pathways of metal incorporation into insects in comparison to non-metal macronutrients like C, H, N, and S (Dow, 2017; Kaspari, 2021).
Insects incorporate essential and non-essential metals through their larval and adult diets. When some of these essential metals are limited in the environment, populations of herbivorous insect species can be strongly affected (Kaspari, 2020; Reihart et al., 2021), whereas abundant essential metals in the environment can boost population sizes (Prather et al., 2018). For example, the community composition and abundance of grasshoppers is influenced by plant concentrations of N, P, Mg, Na and K in grasslands (Joern et al., 2012). Some toxic metals are also bioaccumulated through dietary sources with detrimental effects on insect populations. For example, experiments have demonstrated that Spodoptera litura Fabricius moths bioaccumulate Ni from their adult diet with negative impacts on growth rate and fecundity (Sun et al., 2013). However, diet might not be the only source of metal incorporation into insect tissues. Unlike vertebrates, insects respire through a system of tubes called tracheae that form a complex network of gas-filled vessels throughout the body. Many metals, such as Pb and Cd, are concentrated in anthropogenic aerosols and can potentially circulate within the tracheal system (Negri et al., 2015). In addition, chitin, a structural polysaccharide, forms insect exoskeletons and is a powerful metal absorbent (Anastopoulos et al., 2017) that could facilitate metal incorporation in insect tissues through adhesion to the surface of the exoskeleton (Negri et al., 2015). Some elements, such as Sr, Ca, Mg, and Ba, are highly soluble in water and it is possible that they could exchange and diffuse deeper into insect tissues when wetted. Recent controlled studies on metabolically inert keratinous tissues of vertebrates (e.g., Feathers: Crowley et al., 2021; hair: Fauberteau et al., 2021) demonstrated that many metals in keratin are rapidly exchanged and concentrated from external sources (e.g., wet absorption, pollution, dust aerosols; Hu et al., 2018, 2019, 2020; Rodiouchkina et al., 2022). In addition to their incorporation through diet, exogenous bioaccumulation could influence the concentration of metals in chitinous insect tissues.
Understanding the sources and mechanisms of metal incorporation into insect tissues is especially urgent in the context of anthropogenic habitat alteration because human activities have changed the concentrations of toxic and essential metals in the environment. Human activities such as smelting, mining, industrialization, and agriculture are causing insects to be increasingly exposed to toxic metals at rates above the natural baselines they evolved to tolerate (Monchanin et al., 2021). Even when regulated, these activities can have adverse effects on insects because invertebrates are more susceptible than vertebrates to toxic metals like Cd and Pb and, therefore, often experience adverse effects well below anthropocentric permissible concentration limits (Hopkin, 1990; Mogren and Trumble, 2010; Monchanin et al., 2021). Similarly, activities such as CO2 enrichment or the application of nitrogen and phosphate fertilizers can alter the availability of essential metals to insects though the nutrient dilution of plants (Welti et al., 2020; Nessel et al., 2021). Limited availability of essential metals can inhibit growth and development and reduce fitness; thus, conservation efforts need to consider both changes to the bioavailability of essential metals to organisms (Filipiak and Filipiak, 2022) and exposure to toxic metals.
Besides these toxicological and micronutrient availability concerns, understanding the mechanisms of metal incorporation into insect tissues is essential to develop new geolocation tools for insect population ecology. The analysis of metal and metal isotopes in insect tissues has become a promising avenue for developing tools aimed at understanding insect migration. Metals (e.g., Tigar and Hursthouse, 2016), metal ratios (e.g., Holder et al., 2014), combined metal composition (i.e., chemoprints, mineral component analysis, chemometrics; e.g., Lin et al., 2019) and metal isotopes (Holder et al., 2014; Reich et al., 2021) have been used to assess long-term patterns of insect dispersal. Insect wings are the primary tissue used to develop these geolocation tools. It has been demonstrated that, for some metal and metal isotopes, the insect wings reflect the chemical signature of the larval diet (e.g., Flockhart et al., 2015). By comparing the chemical signature of the metal or metal isotopes of the insect tissue to metal-specific baseline maps (called “metalscapes” or isoscapes), one can then estimate the location where the tissue formation occurred (i.e., the natal origin). However, making proper geolocation inferences with these metals requires a clear understanding of metal and metal isotope incorporation into insect tissue over time because alteration of the natal signature via, for example, the uptake of metals through adult feeding, would void any geolocation inferences (Dempster et al., 1986). Insect wings are primarily composed of chitin and proteins, and are not completely inert like vertebrate keratin. They host androconial organs (i.e., pheromone glands), sensory receptors, trachea, nerves, and have hemolymph circulating through their veins (Tsai et al., 2020). Consequently, the circulation of hemolymph could lead to some metal exchange with the rest of the insect body leading to potential modification of metal concentrations during the adult life, as has been observed for hydrogen isotope values (Lindroos et al., 2023). Additionally, exogenous bioaccumulation could contribute to the incorporation of many metals during adult life through aerosol deposition and wetting as has been observed with keratinous tissues (Hu et al., 2018, 2019). However, the potential addition of metals to insect wings after tissue formation has not been explicitly investigated.
In this study, we aimed to better characterize the incorporation mechanisms of a suite of metal and metal isotopes in insect wings over time and advance the development of metal-based tools for insect ecology. We conducted a laboratory-based diet-switching experiment to explore the environmental and dietary sources of 23 metals and metalloids, strontium isotopes (measured as 87Sr/86Sr ratios), and lead isotopes (measured as 208Pb/206Pb and 207Pb/206Pb ratios) in insect wings over an 8-week timespan. For each metal, we evaluated the effects of intrinsic factors on metal concentrations and assessed the potential sources of any bioaccumulation. We also assessed the potential for 87Sr/86Sr in insect wings to be altered by wetting. Finally, we evaluated the potential of the metal and metal isotopes for providing geolocation or pollution exposure information. By understanding the incorporation of metals and metal isotopes in insect wings over time, we will be better able to test the assumptions of geolocation using metal biomarkers and assess the metal exposure risks of insects.
2. Materials and methods
We chose for this study a representative winged insect, the monarch butterfly [Danaus plexippus (L.)]. The monarch butterfly is an iconic migratory insect famous for its annual, multi-generational, round-trip migration from the Oyamel fir forests of Central Mexico, through the United States, to Canada, and back. Monarchs are a well-suited species to investigate the incorporation of metal and metal isotope signals. First, they have a large geographic range with known elemental and isotopic variations meaningful for geolocation (Wieringa et al., 2020; Reich et al., 2021). Second, monarch larvae are specialist herbivores dependent on milkweed (Asclepias spp.). Plants are known to uptake and regulate metals in a species-specific manner; thus, this relatively high host-specificity will likely minimize potential discrepancies in metal signatures associated with differences in larval host plant species (McLean et al., 1983; Tibbett et al., 2021). Third, metal exposure is of particular concern for monarchs because milkweed is often found in disturbed habitats, such as roadsides, which often correlates with higher pollution exposure (Phillips et al., 2021). As for most insects, the incorporation and toxicology of metals in monarch butterflies are mostly unknown, although Zn has been shown to decrease larval survival under some conditions (e.g., when individuals have low access to macronutrients; Shephard et al., 2020, 2021, 2022).
To explore the incorporation of metal and metal isotopes, we first conducted a diet-switching experiment on monarch butterflies over an 8-week period. We raised larvae from eggs with controlled milkweed dietary inputs and then maintained the adults with a controlled adult diet. We measured the changes in metal concentrations and isotopes in monarch wings at weekly time intervals and compared the metal abundances found in our laboratory experiment to the range of natural metal abundances in the wings of 100 wild-caught monarch butterflies. Next, we explored the incorporation mechanisms of different metals by calculating enrichment factors to the adult diet and a proxy of mineral dust for each metal, analyzing lead isotopes in the monarch wings to assess the source of Pb bioaccumulation, and performing a wetting experiment for Sr. Finally, we measured the concentrations of 12 metals in ashed milkweed samples from across the eastern United States and assessed our ability to use soil and milkweed samples in the construction of metalscapes for monarch butterflies (Supplementary Material).
2.1. Diet-switching experiment
We conducted a diet-switching experiment in the summer of 2019 to assess the sources and preservation of metals and metalloids (hereinafter referred to collectively as metals) and metal isotope signals in monarch wings throughout the life of an adult butterfly. Eggs were collected from a single wild-caught female, allowing us to assume that all the specimens in this experiment are at least half-siblings and control for maternal effects. After collection, eggs were transferred to individual 500 ml glass rearing containers with a mesh lid and placed in a controlled environmental chamber (Biochambers Inc., model LTCB-19) held at a light to dark schedule of 12 h:12 h, light temperature of 28°C and dark temperature of 26°C, and humidity of 60%. These conditions reflect optimal conditions for monarch development (Dargent et al., 2021).
Caterpillars were fed wild common milkweed (Asclepias syriaca L.) leaves that were collected daily from Aug. 9–22, 2019, from a single 30 m2 site in Ottawa, Ontario, Canada (45.41°, −75.67°). The collection site is in an urban park next to the Rideau River and is near a light rail station and bike paths. The leaves were cleaned of surface contaminates (e.g., dust) using distilled deionized (DDI) water before being fed ad libitum to the caterpillars. Sub-samples of the cleaned milkweed leaves were collected for analysis to check the assumption that milkweed leaves taken from the same locality will have similar elemental and isotopic signatures over the 2-week period of collection. Frass was removed from the containers daily.
After the monarchs eclosed from the chrysalises (Aug. 29–31, 2019), they were transferred to mesh butterfly cages in an indoor laboratory. Butterflies were kept two per cage, but a cloth divider was installed to minimize wing damage from collisions between individuals. The cages were misted twice a day with DDI water to maintain humidity and were given a light-to-dark schedule of about 12 h:12 h. DDI water was used to avoid additions of metals by water absorption. The temperature in the laboratory was maintained by the building’s normal operations and maintenance schedule at approximately 20°C. Once per day, the adult butterflies were given a fresh serving of 50% maple syrup solution in a tube with a foam faux-flower perch and allowed to feed ad libitum. The adult food was renewed every day to avoid accumulation of metals from external sources (e.g., dust) in the maple syrup solution to ensure that metals sourced from the diet and exogenous aerosols could be distinguished. Because the butterflies do not always recognize the unnatural food source, individuals were also hand-fed the maple syrup for at least 1 minute every other day. The first six monarchs were sampled immediately post-eclosion (Day 0; no feeding), and two monarchs were subsequently sampled each week until up to 8 weeks post-eclosion (n = 22). Immediately after sampling, monarchs were placed into a freezing chamber at −18°C. Later, wings were dissected from the body and placed in glassine envelopes until elemental analysis. The time between collection and elemental or isotopic analysis ranged from a few months to 18 months.
2.1.1. Trace element analysis
Elemental analysis of monarch wings, larval diet (i.e., milkweed leaves) and adult diet (i.e., 50% maple syrup solution) was performed in two analytical runs via inductively coupled plasma mass spectrometry (ICP-MS; Agilent 8800 ICP-QQQ, Agilent Technologies Inc., Santa Clara, CA, United States) at the Department of Earth and Environmental Sciences, University of Ottawa. The first analytical run in February 2020 measured 23 metals in monarch wings (n = 18) and maple syrup (n = 4): aluminum (Al), arsenic (As), barium (Ba), calcium (Ca), cadmium (Cd), cobalt (Co), chromium (Cr), copper (Cu), cesium (Cs), iron (Fe), magnesium (Mg), manganese (Mn), molybdenum (Mo), sodium (Na), nickel (Ni), lead (Pb), rubidium (Rb), antimony (Sb), strontium (Sr), titanium (Ti), thallium (Tl), uranium (U), and zinc (Zn). To remove any surface contaminants (e.g., dust), a single forewing from each monarch was cleaned using pressurized nitrogen gas for about 2 min. at 10 psi (Reich et al., 2021). The monarch wings and maple syrup samples were then digested in perfluoroalkoxy alkanes (PFA) vials (Savillex, LLC., Eden Prairie, MN, United States) using 1 ml 16 M HNO3 (double distilled TraceMetal™ Grade; Fisher Chemical, Mississauga, ON, Canada) and 0.1 ml ultraclean hydrogen peroxide (for trace analysis Grade; Sigma-Aldrich, Italy) for 12 h at 120°C. After digestion, the samples were dried on a hot plate at 90°C and then re-dissolved in 1 ml of 2% v/v HNO3 (double distilled). A 100 μl aliquot of each solution was extracted, diluted to 2 ml 2% v/v HNO3, and centrifuged. Calibration standards were prepared using single element certified standards (SCP Science Inc., Montreal, QC, Canada). Detection limits were conservatively estimated as three times the standard deviation of the blanks within each run. For monarch wings, 4 measurements of As, 2 of Tl, and 8 of U were below the detection limit. Concentrations of Cd, Cs, Tl, and U were lower than the calibration range of 1.56 to 100 ppb (~200, ~800, ~900, and ~ 4,600 times lower, respectively). Metal concentrations in maple syrup were comparatively low, with all 4 As and U measures and 1 Fe measure below the detection limit. Additionally, Cd, Tl, and Pb were all well below the calibration range (~600, ~200 and ~ 200 times lower, respectively). Intra-run relative standard deviation (RSD) of the repeated standard analysis was below 3% except for Cs (RSD = 21%), Sb (RSD = 19%), Rb (RSD = 67%), and Fe (RSD = 38%).
For the second analytical run in May 2021, milkweed samples (n = 3) and analytical replicates of monarch wings (n = 19) were analyzed for 15 metals: Al, Ba, Ca, Cd, Co, Cr, Cu, Fe, Mg, Mn, Ni, Pb, Sr, Ti, and Zn. Milkweed samples were cleaned with DDI water, an ultrasonic bath (10 min.), and a MilliQ water rinse. Wing samples were dry-cleaned with pressurized nitrogen gas for 2 min. at about 10 psi for a limited, repeated elemental analysis of the monarch wings to assess if there was sample contamination of Pb during storage. For monarch tissues, a single hindwing was used for most samples. However, some samples had too low Pb concentrations and both hindwings (i.e., L2) or both hindwings and the remaining forewings were used (i.e., L11, L16, L17, L21, and L5). Samples were then digested in concentrated nitric acid (16 M; distilled TraceMetal™ Grade; Fisher Chemical, Canada) using microwave digestion (Anton Paar Multiwave 7,000, Austria): the samples were heated from ambient temperature to 250°C at a steady rate in 20 min. and then left at 250°C for 15 min. in a pressurized chamber. An aliquot of 200 μl from each sample was separated and diluted to 2 ml of 2% v/v HNO3 (double distilled) and run on the ICP-MS. For monarch wings, 16 measurements of Co and 17 of Cd were below the detection limit; only Cd was lower than the calibration range (~155 times lower). Metal concentrations in milkweed were comparatively high, with Ca and Mg well above the calibration range (~900 and ~ 200 times higher, respectively). Intra-run RSD of the repeated standard analysis was below 3% except for Mg (RSD = 70%), Ca (RSD = 157%), and Fe (RSD = 143%).
2.1.2. Strontium isotope ratios analysis
The 87Sr/86Sr analysis was performed in two runs at the Isotope Geochemistry and Geochronology Research Center, Carleton University using a ThermoScientific™ Neptune™ high-resolution multi-collector inductively coupled plasma mass spectrometer (MC-ICP-MS; Thermo Fisher Scientific, Bremen, Germany). To prepare for the first run in February 2020, milkweed samples (n = 3) were cleaned using DDI water and an ultrasonic bath (10 min.), then oven-dried at 80°C before being ashed at 600°C for 4 h. to facilitate digestion. They were then digested in perfluoroalkoxy alkanes (PFA) vials (Savillex, LLC., Eden Prairie, MN, United States) using 1 ml 16 M HNO3 (double distilled TraceMetal™ Grade; Fisher Chemical, Mississauga, ON, Canada) and 0.1 ml ultraclean hydrogen peroxide (for trace analysis Grade; Sigma-Aldrich, Italy) for 12 h. at 120°C. After digestion, the samples were dried on a hot plate at 90°C. Dried samples were re-dissolved in 1 ml of 2% v/v HNO3 (double distilled). A 100 μl aliquot of each solution was extracted, diluted to 2 ml 2% v/v HNO3, and the concentration of Sr was measured using the ICP-MS in October 2019. The remaining ∼900 μl aliquot (90%) of sample digest was dried down and re-dissolved in 0.05 ml 6 M HNO3. The separation of Sr was processed in 100 μl microcolumn loaded with Sr-spec Resin™ (100–150 μm; Eichrom Technologies, LLC). The matrix was rinsed out using 6 M HNO3, and Sr was collected with 0.05 M HNO3. After separation, eluates were dried and re-dissolved in 2 ml 2% v/v HNO3 for 87Sr/86Sr analysis.
For the second analytical run in September 2020, the remaining aliquots (~90%) of the monarch wing (n = 18) and maple syrup (n = 4) samples run on the ICP-MS in February 2020 were used. Samples were dried down and re-dissolved in 0.5 ml 6 M HNO3. The separation of Sr was processed in 100 μl microcolumn loaded with Sr-spec Resin™ (100–150 μm; Eichrom Technologies, LLC). The matrix was rinsed out using 6 M HNO3, and Sr was collected with 0.05 M HNO3. The microcolumn process was repeated to ensure complete separation. After separation, the eluates were dried, and monarch wing samples were re-dissolved in 200 μl 2% v/v HNO3 and maple syrup in 2 ml 2% v/v HNO3 for 87Sr/86Sr analysis.
For both analytical runs, sample solutions were introduced to the MC-ICP-MS using a microFAST MC single-loop system (Elemental Scientific Inc., Omaha, NE, United States). The sample introduction flow rate was 30 μl/min, and the loading volume was 200 μl for the milkweed and maple syrup and 100 μl for the monarch wing samples. The solution was introduced using a PFA nebulizer, double-pass quartz spray chamber, quartz torch, and nickel sample and skimmer cones. Isotopes 82Kr, 83Kr, 84Sr, 85Rb, 86Sr, 87Sr, and 88Sr were simultaneously measured in L4, L3, L2, L1, C, H1, and H2 Faraday cups, respectively. Measurements of samples were made using a static multi-collector routine that consisted of one block of either 70 (milkweed and maple syrup) or 30 cycles (monarch wings) with an integration time of 4.194 s/cycle. 84Sr and 86Sr have isobaric interferences from 84Kr and 86Kr, respectively. 87Sr has an isobaric interference from 87Rb. The interferences of 84Sr and 86Sr were corrected by subtracting the amount of 84Kr and 86Kr corresponding to the 83Kr signal. Interference of 87Sr was corrected by subtracting the amount of 87Rb corresponding to the 85Rb signal. Instrumental mass fractionation was corrected by normalizing 86Sr/88Sr to 0.1194 using the exponential law (Moore et al., 1982). Strontium isotope compositions are reported as 87Sr/86Sr ratios. Procedural blanks were always negligible, with good reproducibility of 87Sr/86Sr for NIST SRM987 (0.710251 ± 0.000003 (1 SD), n = 2). Two 100 ng/g pure Sr standards were measured along with the samples as in-house standards (SrSCP: 0.70816 ± 0.00013, n = 11; GSC: 0.70756 ± 0.00002, n = 4). The long-term reproducibility of the SrSCP in-house standard is (0.70822 ± 0.00004, n = 106).
2.1.3. Lead isotope ratios analysis
We analyzed the lead isotope ratios (208Pb/206Pb, 207Pb/206Pb) of monarch wing and milkweed samples to refine the sources of Pb in the monarch wings. The remaining aliquots (~90%) of the microwave-digested milkweed (n = 3) and monarch (n = 19) samples run on the ICP-MS in May 2021 were used. The separation of Pb was processed in polyethylene columns (Bio-Rad, Hercules, California, United States) loaded with 0.6 ml analytical grade anion exchange resin (AG 1-X8, 100–200 mesh, chloride form; Bio-Rad, Hercules, California, United States). Initial rinsing steps used 1 M HBr (OPTIMA™, Fisher Chemical, Canada) followed by the collection of the Pb fraction with ultra-clean 6 M HCl. A second pass through the column was performed to further purify Pb. After separation, the Pb eluates were dried and re-dissolved in 260 μl and 460 μl 2% v/v HNO3, for monarch and milkweed samples, respectively (TraceMetal™ Grade; Fisher Chemical, Canada). A thallium spike (in a Tl:Pb mass ratio of 1:4) was added to each sample for mass bias correction against 203Tl/205Tl = 0.418922.
The Pb isotopes analysis was performed at the Isotope Geochemistry and Geochronology Research Center, Carleton University using a Thermo Scientific™ Neptune™ high-resolution multi-collector inductively coupled plasma mass spectrometer (MC-ICP-MS; Thermo Fisher Scientific, Bremen, Germany). Sample solutions in 2% v/v HNO3 were aspirated using a PFA nebulizer, double-pass quartz spray chamber, quartz torch, and nickel sample and skimmer cones. Isotopes 202Hg, 203Tl, 204Pb, 205Tl, 206Pb, 207Pb, and 208Pb were simultaneously measured in L3, L2, L1, C, H1, H2 and H3 Faraday cups, respectively. Measurements of samples were made using a static multi-collector routine that consisted of 1 block of 70 cycles for milkweed and 35–40 cycles for monarchs with an integration time of 8.389 s/cycle. Procedural blanks were negligible (<50 pg). The reported Pb isotope ratios are corrected for offsets between the analyzed and reported NBS981 (Todt et al., 1996). For a period of 12 months, average ratios and uncertainties (± SD, n = 47) of NBS981 bracketing samples were 206Pb/204Pb = 16.9310 ± 0.0095, 207Pb/204Pb = 15.4851 ± 0.0012, 208Pb/204Pb = 36.6783 ± 0.0035, 208Pb/206Pb = 2.16634 ± 0.00011, and 207Pb/206Pb = 0.91460 ± 0.00003.
2.1.4. Statistical analysis
To examine patterns in metal concentrations and isotopes in the monarch wings over time, and to group elements and isotopes with similar characteristics, we used multivariate multiple regression and principal component analysis (PCA). General linear models were constructed with sex, time (days), and body mass (mg) as fixed effects; multiple models were run independently because there were differences in sample size due to exclusions from the quality control process. All models were visually checked for normality, independence, and homoscedasticity of the model residuals. Body mass and sex are strongly associated (point-biserial correlation = −0.70; Supplementary Figure S3), so linear models were re-run without sex as an explanatory variable to test the sensitivity of the results to the confounding variables (Supplementary Table S3). Twenty metals were included in the PCA because of unequal sample size due to As, Tl, and U having measurements below the detection limit. All statistical analyses were performed using R (version 4.1.0; R Core Team, 2013), and a commented R markdown document has been provided (see Data Availability Statement).
2.1.5. Mobility index and enrichment factors
To explore the transfer of metals between trophic levels, we calculated the elemental mobility index for each metal between the larval diet (i.e., milkweed) and the monarchs sampled on the day of eclosion (i.e., Day 0). The mobility index was calculated as the ratio between the metal concentration in the monarch wings and the larval diet (Tigar and Hursthouse, 2016). A value greater than one indicates that the monarch wing has a higher metal concentration than its larval food and that the metal is biomagnified; whereas a value of less than one indicates that the monarch wing has lower metal concentrations than its larval food and the metal has been biodiluted.
Enrichment factors (EF) were also calculated between the adult diet and monarch wings (EFms) and between the upper continental crust (Rudnick and Gao, 2014) and monarch wings (EFcc) to assess the source of metals in butterfly wings. The upper continental crust is used as a proxy for average exogenous contamination from mineral dust, which allows the EF to represent how much of a trace element is sourced endogenously (i.e., from within the organism itself) versus obtained through exogenous contamination (i.e., from the continental crust; Hu et al., 2019). We calculated the EF using calcium concentration as a normalization factor (Kabata-Pendias and Mukherjee, 2007; Equation 1). Ca is one of the major elements in the continental crust and an abundant metal in monarch wings (Supplementary Table S2); normalization to Ca makes the EF calculation less sensitive to variation between samples (Hu et al., 2019). An EF of greater than one implies that the metal is enriched in the monarch relative to the source (e.g., diet (EFms), crust (EFcc)), and, therefore, the source has low potential to contaminate the monarch signal. Conversely, an EF of less than one indicates that this metal in monarch wings is susceptible to contamination from that source.
2.2. Wetting experiment
Because 87Sr/86Sr are currently the main metal isotopic tool used in insects, we conducted an additional experiment to refine the sources of Sr in wings and assess the potential contamination of Sr due to wetting. Submerging keratin (e.g., hair) in water can result in exogenous contamination of Sr (Hu et al., 2020), but this contamination route is untested for insect chitin. In our diet-switching experiment, the adult monarchs are not wetted, and, therefore, the Sr content and 87Sr/86Sr ratios are uncontaminated by wetting. However, in the wild, monarchs might be wetted by rain or other environmental waters, which could possibly lead to exogeneous Sr contamination via exchange, removal, or addition. Since butterfly wings are superhydrophobic (Pass, 2018), we hypothesized that the Sr in the wings would not be contaminated by submergence in water. We designed a simple wetting experiment to test whether exogeneous Sr could be integrated into the monarch wings when submerged in water. Two beakers of 80 ml 300 ppb Sr certified standard purchased from SCP Science Inc. (Montreal, QC, Canada) were prepared and neutralized with 60 μl 0.5 N NaOH. Two monarch butterfly wings were cleaned of surface dust using pressured nitrogen gas (10 psi for 4 min.). The wings were submerged in the beakers of solution and agitated. At designated time steps, from before the wings were submerged to 28 days after submergence, a 100 μl aliquot of the solution was removed from each beaker, diluted to 3 ml 2% v/v HNO3 (TraceMetal™ Grade; Fisher Chemical, Canada), and centrifuged. These aliquots were then measured using the ICP-MS to monitor the change in Sr concentration of the beakers over time.
2.3. Natural metal concentrations in monarchs
To estimate the natural metal composition of wild monarch butterflies, the wings of 100 monarch butterflies from eastern North America were analyzed for a suite of 12 elements (i.e., Al, Ba, Cd, Co, Cr, Cu, Mg, Mn, Ni, Pb, Sr, Zn). The butterflies were captured in the spring of 2011 from sites in Texas, Oklahoma, and Missouri as part of a previous study (Flockhart et al., 2013). Methodological details can be found in the Supplementary Material.
3. Results
3.1. Metals classified into four categories
The concentrations of Al, As, Ba, Ca, Cd, Co, Cr, Cs, Cu, Fe, Mg, Mn, Mo, Na, Ni, Pb, Rb, Sb, Sr, Ti, Tl, U, and Zn in the wings of the monarch butterflies, the larval diet (i.e., milkweed), and the adult diet (i.e., maple syrup) from the diet-switching experiment are reported in Supplementary Table S2. In the monarch wings, Ca was the most abundant metal (mean ± SD; 640 ± 160 ng/mg, n = 18) followed by Mg (290 ± 180 ng/mg, n = 18) and Fe (87 ± 34 ng/mg, n = 18). Uranium was the least abundant (0.0010 ± 0.0003 ng/mg, n = 10), with many samples falling below the detection limit. In the larval diet, alkaline earth metals Ca, Mg, and Sr were the most abundant (Ca: 18 ± 2 μg/mg, n = 3; Mg: 4.2 ± 0.3 μg/mg, n = 3; Sr: 190 ± 20 ng/mg, n = 3) and Cd was the least abundant (9.5 ± 2.2 ng/g, n = 3). Similarly, in the adult diet Ca (190 ± 37 ng/mg, n = 4) and Mg (73 ± 5, ng/mg, n = 4) were the most abundant and Cd the least abundant (0.4 ± 0.1 ng/g, n = 4).
Principal component analysis (PCA) was used to help categorize the metals in the monarch wings from the diet-switching experiment. The first principal component (PC1) explained 43.8% of the variation and was driven mainly by Al, Fe, Pb, Cr, and Cs (Figures 1A; Supplementary Figure S1A). A linear regression of PC1 with time category (i.e., early, late) and sex (R2 = 0.80) detected a difference in PC1 between Week 0 and Weeks 1 to 8 (β = 5.5 ± 0.9, 6.3, p < 0.001; Figure 1B), but not between the sexes (p = 0.1). PC2, driven by Cu, Na, Mn, Ca, and Cd (Supplementary Figure S1B), accounted for 16.1% of the variation. A linear regression with day and sex (R2 = 0.52) found that PC2 trended temporally (β = 0.05 ± 0.02, 3.5, p = 0.003) and differed between the sexes (β = 2.3 ± 0.6, 3.7, p = 0.002), suggesting that the metals driving PC2 may be influenced by biological variables (Figure 1B).
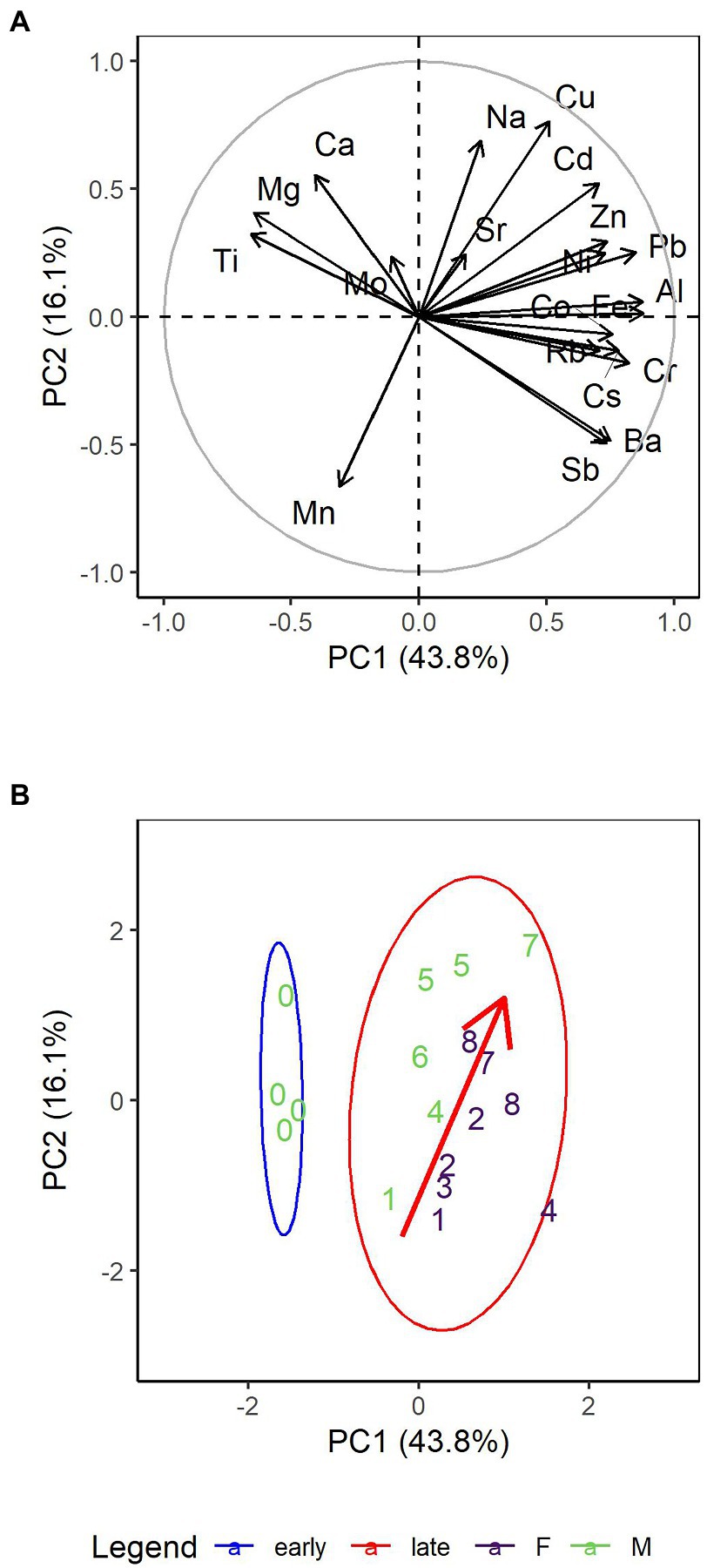
Figure 1. Principal components analysis biplots of 20 metals (excluding As, Tl, and U due to measurements below the detection limit) in monarch butterfly wings (A) Loading plot of the first two principal components for monarch wings from all weeks explaining 59.9% of the variation. (B) PCA score plot of monarch wings from all weeks; numbers depict the week at which the individual was sampled. There is a no overlap between the 95% data ellipses of early dates (i.e., Week 0; blue ellipse) and later dates (i.e., Weeks 1 to 8; red ellipse). There is a trend, mainly in PC2, from Week 1 to 8; a red arrow has been superimposed on the biplot to illustrate this trend. There is also grouping between female (purple) and male (green) butterflies.
Multivariate multiple regression found that metals in monarch wings showed distinctive associations with time (days), sex, and body mass (mg; Table 1). If metals are accumulated from endogenous or exogenous sources, we would expect a positive correlation between time and metal concentration; such associations were found for Al, Cd, Cu, Fe, Pb, Ni, and Zn. Significant negative correlations with time were found for As and Mn, suggesting that these metals are removed from the butterfly wings over time. Calcium concentrations in male monarch wings were, on average (± SE), 190 ng/mg (± 60) higher than in females (Figure 2A). Conversely, on average males had 0.04 ng/mg (± 0.01) less Co and 1.0 ng/mg (± 0.5) less Sb. Body mass was found to have a significant association with Mo (β = −0.0006 ± 0.0002, −2.6, p < 0.05).
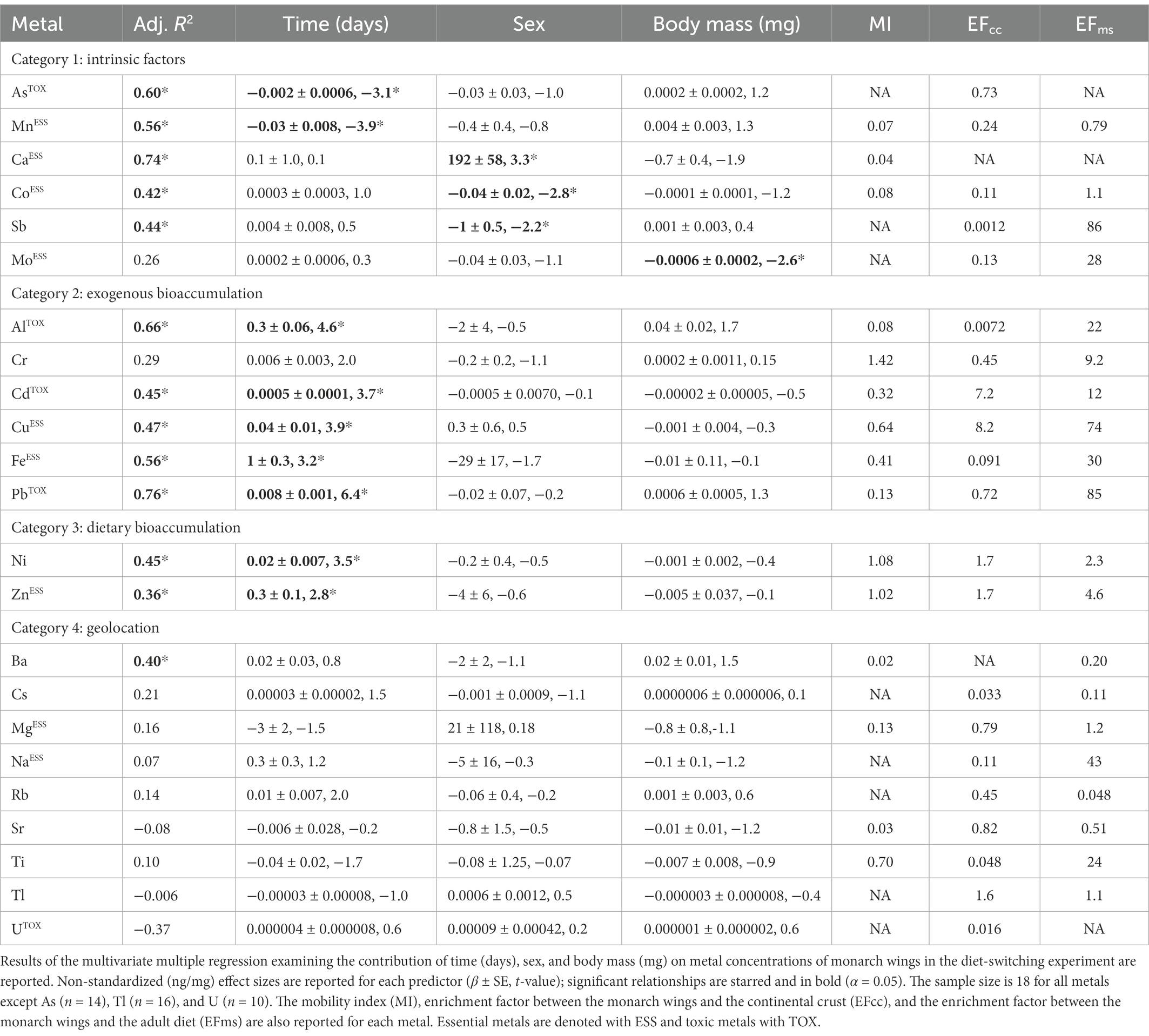
Table 1. Metals are clustered into four categories based on their characteristics in the diet-switching experiment.
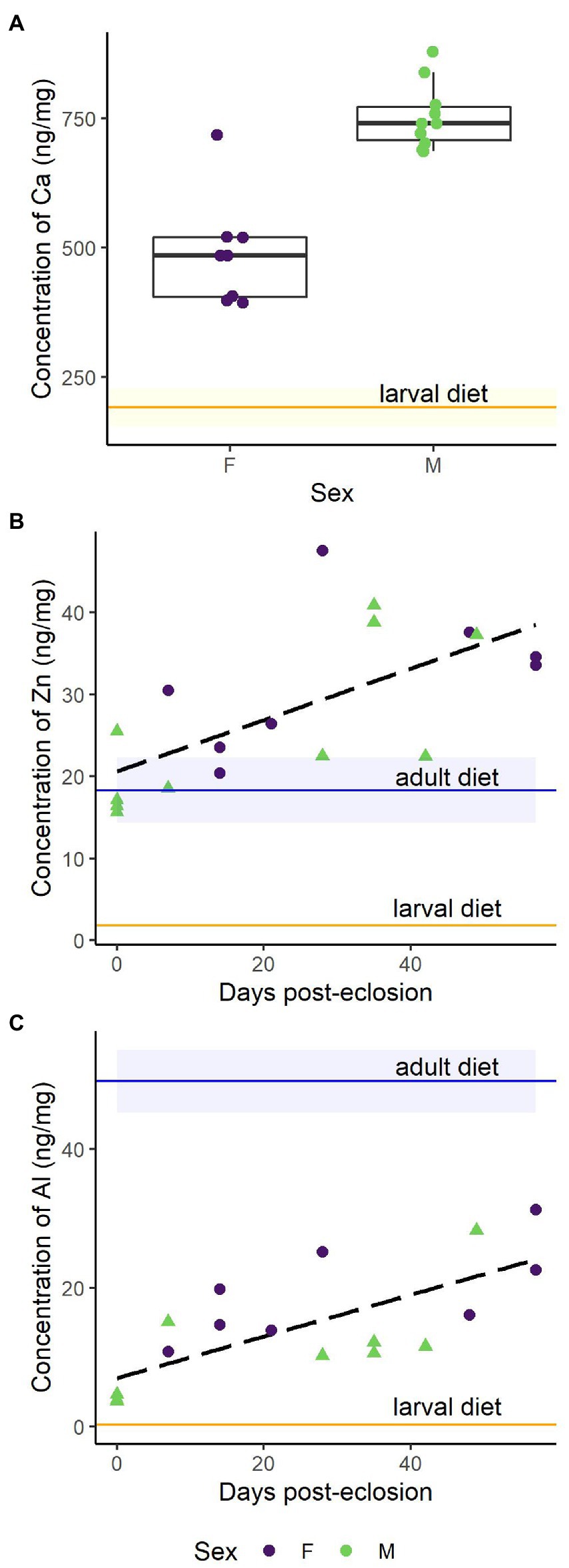
Figure 2. Metal concentration in monarch wings (1 ng/mg = 1 ppm) from the diet-switching experiment for representative metals classified in different functional categories. Horizontal blue lines depict the mean concentration (± SD) in the larval diet, milkweed (n = 3), and horizontal orange lines represent the mean concentration (± SD) in the adult diet, maple syrup (n = 4). (A) Category 1: Boxplot of Ca concentration (ng/mg) in monarch wings by sex (RSD = 4%). Sex-based differences were detected with males (green) having more Ca than females (purple). The milkweed concentration (18,500 ± 1800 ng/mg) is not shown as it far exceeds that of the monarch wings. (B) Category 2: The concentration of Zn (ng/mg) in monarch wings increased over the 8 weeks (RSD = 1%). (C) Category 3: The concentration of Al (ng/mg) in monarch wings increased over the 8 weeks (RSD = 2%).
Enrichment factors and mobility indices calculated for each of the 23 metals showed distinctive patterns in the concentrations between the monarch butterfly wings and the larval diet (i.e., milkweed), the adult diet (i.e., maple syrup), and a proxy for mineral dust (i.e., continental crust). Chromium was the only metal with a mobility index greater than one indicating that it is biomagnified in monarch wings compared to milkweed (Table 1). Nickel and Zn had mobility indices close to one. The remaining elements showed low concentrations in monarch wings compared to milkweed indicating biodilution of these metals. The lowest mobility indices were found among the alkaline earth metals (i.e., Mg (0.13), Ca (0.04), Sr (0.03), and Ba (0.02)). Enrichment factors less than one suggest that the metal was susceptible to contamination from either the adult diet (EFms) or mineral dust (EFcc). Of the metals that showed an increase in concentration over time, Al and Fe showed particularly low EFcc, indicating that the increase in concentration could be due to additions of Al and Fe from mineral dust. Comparatively low EFms for Ni and Zn suggest that the bioaccumulation seen in these metals may be due to additions from the adult diet.
Based on the results of the PCAs, multiple multivariate regression, mobility indices, and enrichment factors, metals were separated into four categories: (1) metals affected by intrinsic factors (e.g., sex, body mass), (2) metals showing bioaccumulation likely from external sources, (3) metals showing bioaccumulation likely from dietary sources, and (4) metals without significant effects of intrinsic factors that maintain the initial metal concentration over time (Table 1; Figure 3). Metals were categorized as Category 1 if sex or mass were associated with metal concentration or there was a decrease in concentration over time. A few of these metals (i.e., Ca, Mn) were the main drivers of PC2 (Figures 1A; Supplementary Figure S1B). Metals assigned to Category 1 were As, Ca, Co, Mn, Mo, and Sb. For example, Ca was significantly more abundant in male wings than in female wings (Figure 2A), but no effect of time was detected. Category 2 metals had a positive association with time and relatively low EFcc, indicating that their increases in concentration over time were more likely to be sourced from mineral dust rather than from the adult diet. For instance, despite low Al levels in the maple syrup (EFms = 22), the concentration of Al in the monarch wings increased over time (Figure 2C). As a terrigenous metal, it is more likely that the accumulated Al is sourced from exogenous mineral dust (ECcc = 0.0072). Some of the Category 2 elements are terrigenous metals, including Al and Fe, but others are associated with anthropogenic pollution (i.e., Cd, Cu, Cr, Pb). Several of the Category 2 metals are essential to living organisms (i.e., Cr, Cu, Fe), but others are toxic to animals even at low concentrations (i.e., Al, Cd, Pb). Category 3 metals (i.e., Ni, Zn) also had a positive association with time, but had high EFcc and relatively low EFms and are therefore potentially contaminated endogenously through the adult diet. As an illustration, the monarch wings showed a two-fold accumulation of Zn over time from a concentration similar to that of the larval diet (Figure 2B). The level of this essential metal was lower in the adult diet than in the larval diet, but the EFms was relatively low (4.6), suggesting that the increase in Zn may be sourced, at least partially, from the adult diet. Finally, metals were assigned to Category 4 if they showed no significant associations with time, sex, or body mass. These metals were Ba, Cs, Mg, Na, Rb, Sr, Ti, Tl, and U.
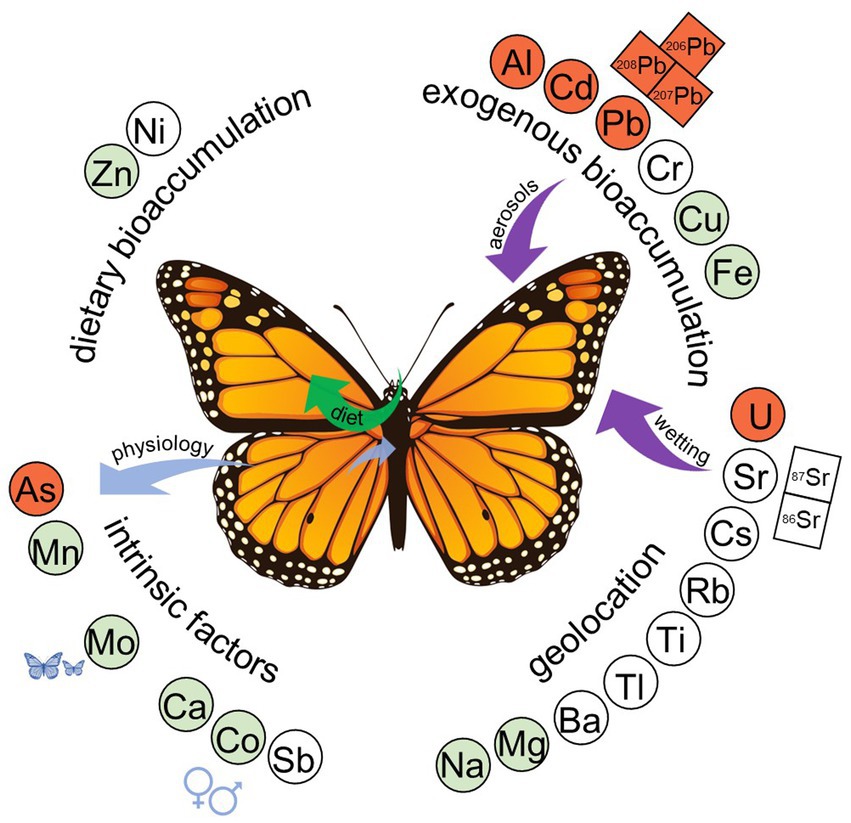
Figure 3. Twenty-three metals were classified into four categories based on their characteristics in the diet-switching experiment. Essential metals (green) and highly toxic metals (red) are indicated; metal isotopes are indicated by boxes. Category 1, intrinsic factors (bottom-left), includes two sub-categories: (1) metals (i.e., As, Mn) that decrease in the wing over time through unknown mechanisms (blue arrows), and (2) metals that are influenced by body mass or sex (i.e., Ca, Co, Sb, Mo). Category 2, exogenous bioaccumulation (top-right), includes metals that are, at least partly, accumulated from external sources (purple arrows), such as via aerosol dust (e.g., Pb and Pb isotopes; Figure 5) or under wetting conditions (e.g., Sr; Figure 4D). Category 3, dietary bioaccumulation (top-left), included metals that are likely accumulated from the adult diet (green arrow). Finally, Category 4, geolocation (bottom-right), includes metals that maintained similar concentrations over the course of the experiment and are good candidates for geolocation studies.
3.2. Strontium and strontium isotope ratios
The Sr and 87Sr/86Sr of the diet-switching experiment monarch wings, larval diet, and adult diet are reported in Supplementary Table S2. Based on its characteristics, Sr was considered as Category 4 (Table 1), a metal that does not bioaccumulate and is not significantly affected by sex or body mass. The mobility index (0.03) indicated that Sr is biodiluted in the monarch wings. These low concentrations did not have significant associations with time, sex, or body mass (Table 1), although a non-significant sex-based pattern could be observed (Figure 4A).
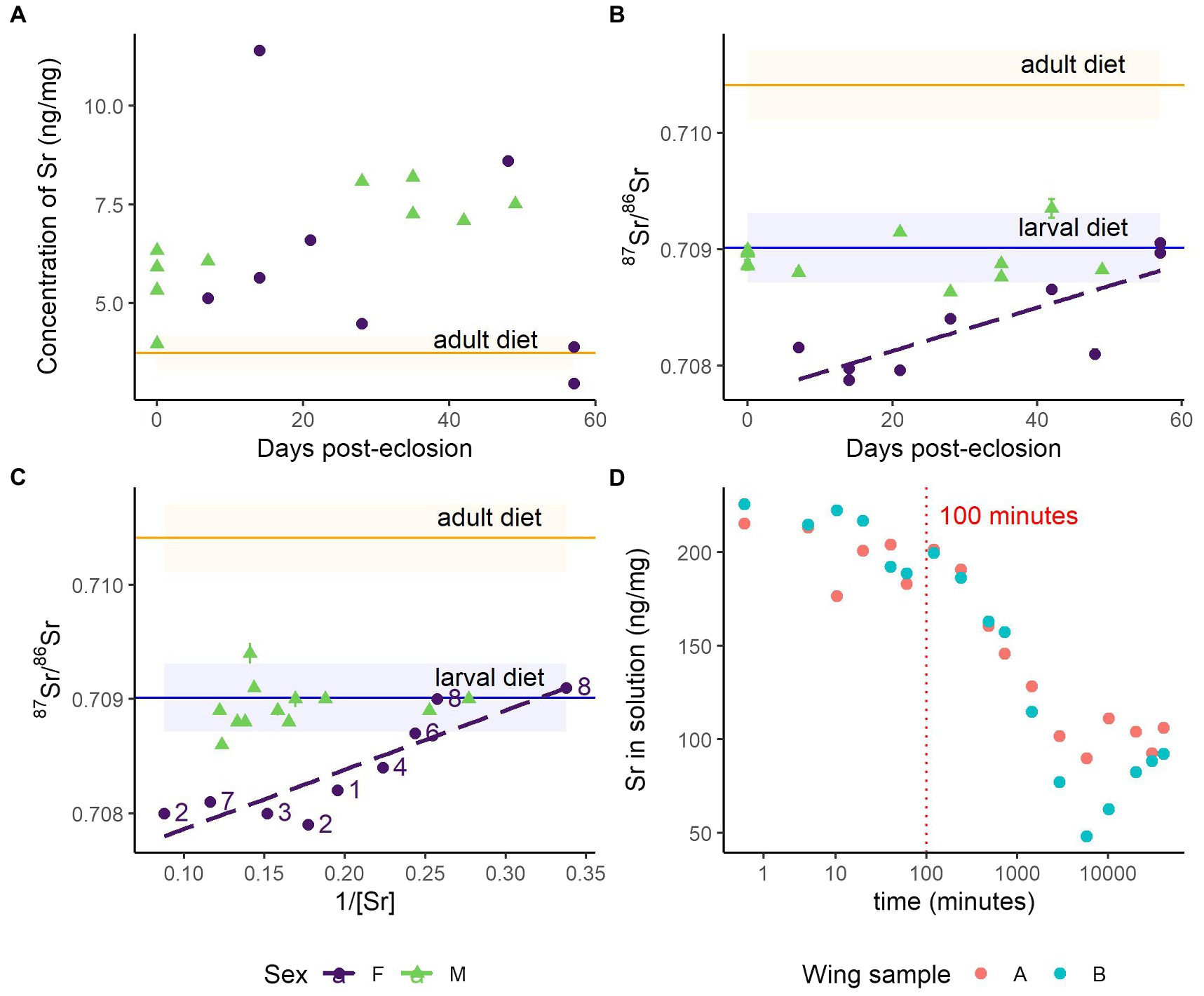
Figure 4. Strontium and strontium isotope ratios in monarch wings during the diet-switching and wetting experiments. Horizontal blue lines depict the mean concentration (± SD) in the larval diet, milkweed (n = 3), and horizontal orange lines represent the mean concentration (± SD) in the adult diet, maple syrup (n = 4). (A) Concentration of Sr (ng/mg) in monarch wings over 8 weeks (RSD = 1%). The concentration of Sr in the larval diet is not shown as it far exceeds that of the monarchs (189 ± 21 ng/mg). (B) Strontium isotope ratios (± SE) in monarch wings over 8 weeks; the 87Sr/86Sr ratios of the female monarch wings show a linear relationship with time. (C) Strontium isotope ratios (± SE) against the inverse of strontium concentration. The significant linear relationship for females is displayed; numbers depict the weeks post-eclosion that the female monarch was sampled. (D) Concentration of Sr (ng/mg) in solution through time during the wing wetting experiment (n = 2).
Multiple regression of monarch wing 87Sr/86Sr was performed with predictors time (days), sex, the inverse of Sr (mg/ng), and interactions between time and sex and the inverse of Sr and sex (adj. R2 = 0.79). As expected, the range of male 87Sr/86Sr matched well with the 87Sr/86Sr of the larval diet and did not change over time (p = 0.2; Figure 4B) or with the inverse of Sr (p = 0.2; Figure 4C). However, on average, the wings of the female monarchs were 0.0014 less radiogenic than the males (β = 0.0014 ± 0.0004, 3.6, p < 0.01) and the 87Sr/86Sr of the female wings increased slowly over time (β = 0.000010 ± 0.000004, 2.3, p = 0.03), although the lack of female monarchs sampled at Week 0 may have been biasing this result (Figure 4B). If the adult diet is responsible for the increasing 87Sr/86Sr of the female monarch wings over time, it seems unlikely that it is through accumulation, as no positive association between Sr and time was detected (p = 0.8; Table 1). The 87Sr/86Sr of the female monarch wings was associated with the inverse of Sr (β = 0.0037 ± 0.0011, 3.4, p < 0.01), such that females with higher concentrations of Sr had 87Sr/86Sr more disparate from those of the males and larval diet (Figure 4C). Given that radiogenic strontium isotopes do not fractionate but instead indicate sources of Sr, the principles of mixing models can be used to find missing endmembers (i.e., the 87Sr/86Sr of pure sources of Sr to the monarch wings). The intercept of regression indicated a missing endmember for the female monarchs with a less radiogenic 87Sr/86Sr of about 0.7073. Females with higher abundances of Sr in their wings tended to be those sampled earlier in the experiment; lower concentrations of Sr in the Week 8 female monarch wings aligned them with the expected 87Sr/86Sr of the larval diet (Figure 4C). Thus, the female monarchs were likely exposed to the less radiogenic Sr source either as larvae or as young adults (i.e., less than a week old).
The wetting experiment shows that exogenous contamination of Sr in butterfly wings by wetting is possible. After we submerged the monarch wing in the aqueous solution, we detected substantial changes in the Sr concentration of the solution after 100 min. (Figure 4D). A new equilibrium was reached after about a week. Since the amount of Sr in the solution decreased over time, it is likely that the Sr accumulated in the wing.
3.3. Lead and lead isotope ratios
Lead was categorized into Category 2 because Pb concentrations in the monarch wings increased over time, likely sourced from mineral dust (Table 1; Figure 5A). Of all the toxic metals, only Pb levels in the milkweed (0.22 ± 0.06 ng/mg, n = 3) and monarch wings (0.30 ± 0.20 ng/mg, n = 18) were found to exceed the permissible limits of human food (i.e., 0.01–1 ng/mg; FAO, WHO, 2019), but Pb levels in the maple syrup (1.1 ± 0.6 ng/g, n = 4) were well below health guidelines. Lead isotope ratios showed that the larval diet matched well with regional environmental 208Pb/206Pb and 207Pb/206Pb signatures measured in snowpack (Figure 5B; Simonetti et al., 2000a,b). The early monarchs (i.e., Week 0) also matched with the larval and environmental Pb isotopes, but within a week, the Pb isotopes of the monarch wings deviated to a distinct signature with higher 208Pb/206Pb and 207Pb/206Pb. Although the Pb isotope ratios were measured a year after the Pb levels, repeated measures showed that storage did not significantly change the initial Pb abundances (paired t-test: t = −1.3, df = 16, p = 0.20).
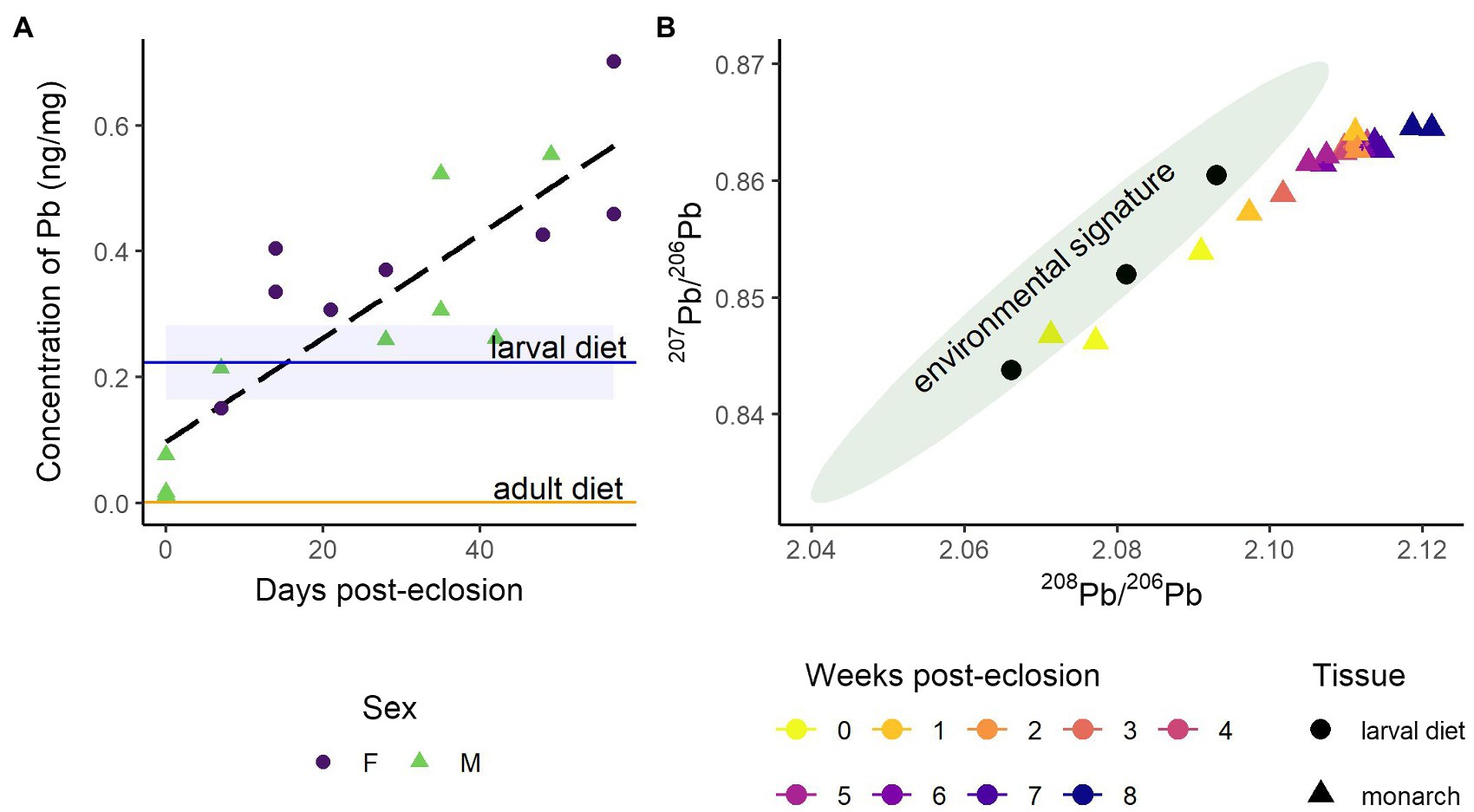
Figure 5. Change in lead and lead isotope ratios in monarch wings during the diet-switching experiment (A) Concentration of Pb (ng/mg) in monarch wings over 8 weeks (RSD = 1%); the linear relationship (dashed black line) with time is for all samples. The horizontal blue line depicts the mean concentration of Pb (± SD) in the larval diet, milkweed (n = 3), and the horizontal orange line represents the mean concentration of Pb (± SD) in the adult diet, maple syrup (n = 4). (B) Biplot of 207Pb/206Pb and 208Pb/206Pb ratios. Monarch wings are depicted as triangles with colors representing the age of the butterfly in weeks; the standard error is smaller than the symbols. Measurements of the larval diet are depicted as black dots (n = 3). The green 95% data ellipse shows the range of regional lead isotope ratios measured in snowpack (Simonetti et al., 2000a,b).
3.4. Natural metal concentrations in lab-raised and wild monarchs
In this lab-based study, we controlled the environmental conditions of the monarch caterpillars and adults, minimized conspecific interactions, reduced flight capacity, and fed the adults a non-natural and choice-restricted diet. Thus, the variation in metal concentrations in the laboratory monarch wings is expected to be different from that of wild monarch wings. These differences can clarify important processes. Comparisons with wild monarch wings show that wing concentrations were similar for Cr, Mn, and Ni (Supplementary Figure S5). Conversely, Mg, Al, Co, Cd, Ba, Pb had higher concentrations in the wild monarch wings and Cu, Zn, and Sr had lower concentrations in the wild monarchs (Supplementary Figure S5).
4. Discussion
4.1. Regulation of metals in insect wings
The 23 metals analyzed in the diet-switching experiment were classified into four categories based on whether they were influenced by intrinsic factors like sex and body mass (Category 1), bioaccumulated metals from external sources (Category 2), bioaccumulated metals from dietary sources (Category 3), or maintained the natal signature (Category 4; Figure 3). Metals classified in Category 1, including As, Mn, Mo, and Ca, showed a decreasing concentration in the wings through time or a correlation with the intrinsic characteristics of individuals. These observations suggest that the levels of these metals are regulated by the insect. Category 1 metals were grouped into two sub-categories based on their distinct trends during the experiment (Table 1). The first sub-category included As and Mn, which showed decreasing concentrations in wings throughout the adult life (Table 1), suggesting that monarchs may reallocate these metals from the wings to other parts of the body or excrete them (Tibbett et al., 2021). Manganese is an essential co-factor in many enzymes but can impact insect behavior at low doses and, like all metals, is toxic in excess (Ben-Shahar, 2018). Caterpillars of Lymantria dispar and Cabera pusaria have been shown to excrete excess Mn through frass and molting (Kula et al., 2014; Martinek et al., 2020), but adult mechanisms of excretion have not been shown and Mn homeostasis has not been studied in monarchs. Alternatively, As and Mn could be more concentrated in wing scales and decrease through time in the entire wing due to the progressive abrasion of scales as the individual ages.
The second sub-category of Category 1 included several metals (i.e., Ca, Co, Mo, Sb) that showed an influence of sex and/or body mass. However, sex and body mass were strongly correlated, with females being consistently heavier than males, making it challenging to disentangle the direct influence of mass from that of sex (Supplementary Figure S3). Molybdenum showed a negative correlation to body mass (Table 1). Molybdenum is an essential metal that acts as a co-factor for many oxidase enzymes (Dow, 2017), some of which relate to wing pigmentation (Feindt et al., 2018) and immune processes (Selvey, 2020). The decrease in Mo concentration in wings with increasing body size might be related to differential regulation of the metal relative to body size, energy reserves, or sex (Orłowski et al., 2020). Alternatively, the decrease in Mo with increasing body size could be driven by differences in surface area-to-mass ratios. Female monarchs have been noted to have thicker wings than males (Davis and Holden, 2015), leading to lower surface area-to-mass ratios in the wings. If Mo is predominantly found at the surface of the wing (e.g., pigmentation), this lower surface area-to-mass ratio could explain the pattern seen in this experiment (Kowalski et al., 1989). Similarly, we found that male monarch wings had significantly more Ca than females (Figure 2A). Notwithstanding the confounding effect of body mass, sex-based differences in Ca have also been detected in whole-body samples of butterflies (Dempster et al., 1986) and damselfly wings (Stuhr et al., 2018), suggesting that sex is the main driver of the observed differences in Ca in wings. Calcium is a known component of insect chorion (Studier, 1996; Nickles et al., 2002) and reproductive organs (Clark, 1958), and Ca has been found to be preferentially allocated to reproductive organs (Mesjasz-Przybyłowicz et al., 2014). This could explain the lower concentrations of Ca found in the wings of female monarchs, as it is possible that Ca is preferentially allocated or reallocated from the wings to reproductive organs for oogenesis. Sex-based differences in metal composition may reflect sexual dimorphism in nutritional demands, which can result in sex-specific nutritional limitations and have ecological consequences (Judd et al., 2010; Espeset et al., 2019; Filipiak et al., 2021). Future studies could investigate the potential for sexual dimorphism in the nutritional demands of monarch butterflies in natural environments and the resultant impacts on population demography, survival, and migratory success (e.g., Snell-Rood et al., 2014).
Little is known about the pathways of metal allocation and regulation in insects. Metallomics, the study of the metal-containing biomolecules that an organism uses, aims to identify and compare metal utilization, function, evolutionary trends, and interactions (Ogra and Hirata, 2017). Of the six metals in Category 1 (Table 1), only the regulatory pathways of Ca, Co, and Mo have been well-characterized (Dow, 2017; Zhang et al., 2019), illustrating the large knowledge gap that the newly-developing field of metallomics is seeking to fill. Metal isotopes that fractionate with physiological processes can be particularly useful to advance understanding but have been under-utilized in insects thus far (but see Nitzsche et al., 2020, 2022). For example, in vertebrates, calcium isotopes have been effectively used to investigate sex-specific modifications of Ca homeostasis due to pregnancy, lactation, and egg-laying (Tacail et al., 2020). Analyzing calcium isotope ratios of different insect tissues could shed light on Ca homeostasis in insects, such as the role of calcium-storing spherites (Dow, 2017), and the sources, mechanisms, and ecological consequences of sex-based differences in Ca.
4.2. Bioaccumulation of metals in insect wings
We identified a suite of metals, including highly toxic (e.g., Al, Cd, Pb) and essential metals (e.g., Zn, Fe), that accumulated in the wings of the monarch butterflies over time (Categories 2 and 3; Table 1). Metals classified in Category 2 (i.e., Al, Cr, Cd, Cu, Fe, and Pb) increased in concentration over time in the monarch wings and had low enrichment factors between the monarch wings and continental crust (Table 1). These observations indicate that these metals were sourced, at least partially, from external sources such as mineral dust. For example, Fe and Al are highly concentrated in terrigenous dust, and previous studies on animal hair have shown that these elements were primarily sourced from exogenous sources in keratin tissues (Kempson et al., 2006; Hu et al., 2018). Our experiment minimized any accumulation of aerosol dusts in the food by replacing the food daily, thus any additions of metal from aerosols are likely incorporated exogenously. Although the initial deposition of aerosols on insects is likely superficial, the metals could become embedded in an organic matrix (i.e., epicuticular waxes; Negri et al., 2015), or, as has been shown previously for keratin, the metals could slowly penetrate deeper into the tissue when mobilized by high relative humidity or wetting (Hu et al., 2018, 2019). This pattern of diffusion is evidenced by the gradient of concentrations of these metals in keratinous tissue, from very high concentrations on the surface to low concentrations deeper in the tissue (Hu et al., 2018). In insects, high concentrations of Al in the cuticle of honeybees have also been observed, suggesting similar exogenous contamination (Shaw et al., 2018). However, given that butterfly wings are living tissue and that Fe is an essential element with known regulatory pathways (Slobodian et al., 2021), there are alternate explanations for the increase of Fe in wings through time. First, our experiment examined a single body part and therefore cannot comment on systemic metal homeostasis (Orłowski et al., 2020); Fe could be reallocated from different parts of the insect body for sequestration in response to perturbations to systemic homeostasis. Additionally, most of the accumulation of Fe in wings was observed between Week 0 and Week 1 (Figure 1B) and could be related to sclerotization and maturation processes as the wings finish forming within a few days post-eclosion (Honegger et al., 2008). Finally, Fe in wings could increase due to changes in Fe requirements over time, as has been observed in other insects (Shaw et al., 2018; Andreani et al., 2021). Finally, metamorphosis is an energetically-costly process, and the teneral butterfly could be Fe-limited, causing a short-term rapid uptake of Fe to reach a regulatory threshold within the first week in an accumulator-regulator dynamic (Tibbett et al., 2021).
Further studies could use natural metal isotopes or isotope tracing to help constrain metal sources in insect tissues, as we exemplify in this study with the use of Pb isotopes (see discussion below). Similar to Al and Fe, Pb accumulated rapidly in the monarch wings with a twentyfold increase over the 8 weeks. In parallel, we observed a temporal shift in Pb isotope ratios of the wings from a composition matching the local environment of the larval diet to a novel isotopic composition (Figure 5B). This indicated that the accumulating Pb is likely from external sources of atmospheric deposition and dust present in the laboratory air, such as from lead paint, pipes, or non-local mineral dust from a rock preparation laboratory located in the same building. Our work supports previous studies showing the contamination of insect tissues by exogenous Pb sources (e.g., Negri et al., 2015; Zhou et al., 2018). The amount of Pb in the wings of the laboratory-reared monarchs exceeded permissible limits for human food, which is between 0.01 and 1 ng/mg (FAO, WHO, 2019). Similarly, Pb in the wings of wild monarchs was also found to be above permissible limits (Supplementary Table S4) to a greater extent than the laboratory monarchs (F(1, 116) = 24, p < 0.001); our experimental results suggest that the high Pb levels accumulated in wild monarch wings are likely sourced from anthropogenic pollution encountered during their migration. Lead is toxic to insects and can cause mortality and sub-lethal effects such as reduced growth rate, body size, fecundity, and locomotor activity (Hirsch et al., 2003; Di et al., 2016; Zhou et al., 2021).
Migratory species could be at an even higher risk of adverse effects from metal exposure than non-migratory species (Seewagen, 2020). Migration is a complex behavior, and migratory insects can travel hundreds to thousands of kilometers and are potentially exposed to many sources of metal pollution along their migratory routes. However, the potential effects of toxic metals on migratory insect survival and migratory behavior are virtually unknown. Anthropogenic sources of Pb have unique Pb isotope ratios; thus, Pb and Pb isotope ratios of biological tissues could be used as indicators of environmental contamination sources (Smith et al., 2021). This principle has been applied to assess the local pollution exposure of non-migrating insects, such as honeybees (e.g., Zhou et al., 2018; Smith and Weis, 2020). We argue that Pb and Pb isotope ratios could also be used as bioindicators of pollution exposure for migratory insects. As an individual insect moves along a migratory path, it will accumulate Pb from the different atmospheric pollution sources it encounters along the way. Measuring Pb isotope ratios in migratory butterflies could provide a tool to reconstruct migratory paths and assess the exposure to pollution of individuals going through this route. This tool would be key to relating migratory success and pollution exposure and could also validate migration routes reconstructed through pollen metabarcoding (Suchan et al., 2018) and wind trajectory modeling (Otuka et al., 2005). To quantify how Pb with unique isotope ratios and concentrations are transferred to the wings from pollution sources, new laboratory studies exposing or feeding insects to isotopically-distinct Pb pollution sources having concentrations similar to those in natural systems could be conducted. Ultimately, Pb isotopes in migratory insects could help identify key sources of pollution that are detrimental to specific species.
Metals classified in Category 3 (i.e., Ni and Zn) grouped metals which also bioaccumulated, but unlike Category 2, these metals were characterized by a relatively low enrichment factor between the monarch wings and adult diet (Table 1). Nickel is not known to be an essential element to insects. Lepidopterans show variable effects to Ni exposure, from decreased body size and fecundity to no effect (Sun et al., 2013; Kobiela and Snell-rood, 2018), suggesting that regulation mechanisms are species-specific (Tibbett et al., 2021). Nickel concentrations in the wings of monarchs in the diet-switching experiment did not significantly differ from those of wild monarchs (Supplementary Figure S5; p = 0.4), suggesting that concentrations may be below regulatory thresholds and thus increasing due to non-specific uptake of metal from the adult diet. Conversely, Zn was found to be lower in wild monarch wings than in the diet-switching experiment (Supplementary Figure S5; F(1, 116) = 40, p < 0.001), suggesting that the monarchs in the diet-switching experiment may have excess Zn. The high Zn in the laboratory monarch wings is unlikely to be purely a result of high Zn in the larval diet because the Zn levels in the milkweed provided in the diet-switching experiment (18 ± 4 ng/mg, n = 3; Supplementary Table S2) are low compared to Zn concentrations measured in wild milkweed (10–66 ng/mg, Mitchell et al., 2020). Zinc is essential for insect reproduction and immunity (Cardoso-Jaime et al., 2022), and Zn homeostasis is known to be strictly controlled in insects (Xiao and Zhou, 2016; Slobodian et al., 2021). However, excessive Zn can lead to decreased survival, growth rate, body size, and longevity (Jin et al., 2020). Although butterfly species are known to have different Zn tolerances, monarchs are sensitive to Zn under certain conditions (Shephard et al., 2022). The accumulation of Zn seen in the diet-switching experiment could reflect an upregulation of Zn metalloproteins due to environmental or physiological conditions, such as increased metabolic processes (Butt et al., 2018). Zinc isotopes have been suggested as a tool to explore the dietary sources of Zn to insects, but applications are currently hampered by the complicated pathways of Zn homeostasis and Zn fractionation in the body (Evans et al., 2017; Wanty et al., 2017; Nitzsche et al., 2020), which are poorly understood in insects compared to humans (e.g., Tanaka and Hirata, 2018).
4.3. Geolocation using metals and metal isotopes
Although metals and metal isotopes that bioaccumulate have the potential to impart geographical information, as has been proposed for Pb isotopes, they are unsuitable for provenance studies that aim to estimate the natal origins of insects. Good candidate metals for the geolocation of migratory insects are those that (1) are unaltered in the tissues of dispersing insects, (2) have predictable spatial patterns, and (3) demonstrate a strong relationship between environmental and biological signatures. Nine metals belonging to Category 4 fit the first requirement and were unaltered over time in the monarch wings: Ba, Cs, Mg, Na, Rb, Sr, Ti, Tl, and U (Table 1). For example, the alkaline earth metals Sr and Mg showed consistent concentrations in the monarch wings over time, suggesting that the natal signature is conserved. Continuous-surface geolocation using metal concentrations would require spatial models of metal variation across the landscape (i.e., metalscapes). Mapping Sr and Mg of soil samples across the eastern United States demonstrated that these metals have distinctive spatial patterns, at least in the soil (Supplementary Figure S6). However, correlations between metal concentrations in the soil and the biosphere (i.e., milkweed) were weak and non-significant (Supplementary Table S5). Previous studies have also found similarly weak correlations in both natural (Wieringa et al., 2020) and controlled laboratory conditions (Lin et al., 2021). Exploration of possible predictors of Sr and Mg concentrations in milkweed highlighted land use, milkweed species, month, and year, but not soil concentration (Supplementary Table S5). It is possible that some metal concentrations may vary at too fine a scale to be suitable for geolocation using continuous surfaces (Norris et al., 2007). Before metals can be used as geolocation biomarkers, further studies will need to improve the predictive power of spatial reference models.
Despite the apparent stability of the concentration of these metals from Category 4 through time, caution should be taken in applying these metals for insect geolocation. Of these metals, Mg and Na are essential metals and are thus expected to be regulated. Our results cannot differentiate between metals whose concentrations were unaltered in the monarch wings due to a lack of bioaccumulation and those that were held at a stable concentration through regulation for metal homeostasis. In the case of homeostasis, the metal concentration may be altered under different stressors not experienced in this experiment, such as starvation, disease, different environmental conditions, or increases in metal exposure. These stressors may alter the ability of the organism to maintain homeostasis (Hopkin, 1990) and thus disrupt the apparent larval signature. Similarly, not all sources of potential exogenous contamination were explored in this study. For example, we showed that Sr in monarch wings is susceptible to contamination or exchange after about 100 min. when submerged in Sr-concentrated aqueous solutions (Figure 4D). This supports the finding that Sr is soluble and that exogenous contamination of Sr in keratinous hair readily occurs when hair is submerged in water (Hu et al., 2018, 2020). Monarchs are behaviorally averse to submergence in water and are unlikely to be exposed to these artificial conditions in nature (i.e., full submergence in highly Sr concentrated waters). However, they are likely to be exposed to rain which usually has a low Sr concentration, except near coastal areas, but can have isotopically variable signatures (Nakano et al., 2001). Further studies will need to investigate the effect on Sr concentration and 87Sr/86Sr of wetting from rainwater with varied concentrations and isotopic signatures. As evidenced by our wetting experiment, it would take over an hour of full submersion in Sr-concentrated water to exchange Sr in the wing because the superhydrophobic property on the surface of the wings limit the exchange of metals. However, the regular or sudden wetting by rain during storms could potentially alter, accumulate, or exchange some Sr in the wings, particularly in coastal regions where Sr is more concentrated in rainwater. Consequently, future studies should investigate the susceptibility of Sr and other metals to contamination under real-world conditions, including rain, aerosols, and pollution. Raising butterflies in the wild at specific sites with known metal and metal isotope composition could help reinforce the applicability of these tools for geolocation. We have defined a suite of metals suitable for the geolocation of monarch butterflies, but it may be inappropriate to extrapolate these findings to other organisms, including other Lepidoptera. The specialized structures that aid in metal transport can differ between insect taxa, leading to phylogenetic-based differences in metal uptake, transfer, speciation, and segregation (Tibbett et al., 2021). This may be especially important for non-nectarivorous species because nectar contains low concentrations of metals compared to the diets of herbivores or carnivores (e.g., dragonflies). Caution should also be taken when using whole-body samples rather than a specific insect tissue with relatively low metabolic activity (Orłowski et al., 2020); it can be assumed that whole-body specimens will show greater changes over time than the wings due to greater inputs from the adult diet (Dempster et al., 1986). Even in cases where the adult insect does not feed and, thus, no dietary input is expected (e.g., eastern spruce budworm), metals which may be altered exogenously should be carefully evaluated. Ultimately, many sources of variability in metal composition need to be further explored, including the effects of host plant (Bowden et al., 1984), microbial communities (Consuegra et al., 2020), parasitism (Sures, 2004), type of metamorphosis and life-stage, reproductive status, homeostatic mechanisms, and their interactions (Tibbett et al., 2021).
Strontium isotope ratios have already been applied to the geographic assignment of monarch butterflies and other lepidopteran species and showed great promise for increasing the precision of isotope-based geographic assignment (Reich et al., 2021; Dargent et al., 2022). Here, we confirmed the assumption that the 87Sr/86Sr of monarch wings remain the same over time and continue to represent the larval signature despite adult feeding, at least in males (Figure 4B). While we showed that the Sr in wings was susceptible to exchange in extreme wetting conditions (Figure 4D), we argue that these conditions are unlikely in nature and do not question the applicability of this geolocation tracer. More surprisingly, we also observed sex-based differences in 87Sr/86Sr, with females having a lower 87Sr/86Sr than both the males and their larval diet (Figures 4B,C). As 87Sr/86Sr trace the mixing of isotopically distinct sources (and not isotopic fractionation), this observation suggests that there is an unidentified source of Sr to the female monarchs. Strontium is chemically similar to Ca and is inadvertently substituted for calcium ions in the body (Capo et al., 1998). Based on our results that Ca and, to a lesser degree, Sr in wings vary by sex (Figures 2A, 4A), we argue that the difference in 87Sr/86Sr is potentially related to the preferential allocation of Ca (and thus Sr) to female reproductive organs for oogenesis, although no eggs were laid during the experiment. In this case, the 87Sr/86Sr of the wings would be determined by the milkweed 87Sr/86Sr from a discrete timestep rather than an integration of the entire larval stage. However, previous studies investigating 87Sr/86Sr in monarch wings did not detect any differences between males and females (Flockhart et al., 2015), and it is possible that our result reflects the low number of samples. Alternatively, the differences in 87Sr/86Sr between the sexes could be due to the high sensitivity of butterfly wings immediately after eclosion. The female monarchs emerged from their chrysalises 1 day earlier than the males (Supplementary Figure S4). The day that the females eclosed coincided with an observation that paint odors could be smelled in the laboratory where the adult monarchs were kept, although the laboratory itself was not painted. Many indoor paints contain high concentrations of Sr (Van Gorkum and Bouwman, 2005), as do gypsum-based mixtures (Huang, 2020), which are often applied to fill holes and sanded smooth prior to painting. High concentrations of dust from either of these substances present on the day the female monarchs eclosed from their chrysalises could have deposited on the surface of the wings, and since butterfly wings are soft and wet on the day of eclosion, it is possible that external Sr could have mobilized and penetrated the wing tissues. We suggest that future studies explore the link between sex and 87Sr/86Sr in monarch wings, and the sensitivity of metals in butterfly wings to environmental conditions on the day of eclosion.
4.4. Natural metal concentrations
The metal concentrations measured in the wings of the laboratory monarchs are unlikely to be representative of monarchs in natural environments. All the monarchs in the laboratory experiment were siblings, limiting their genetic diversity and the independence of replicates (Merritt and Bewick, 2017). Comparing the concentrations of metals found in the wings of monarchs in our laboratory study to concentrations of metals in the wings of wild monarchs, we can see that wild monarch wings had significantly higher concentrations of Mg, Al, Co, Cd, Ba, and Pb, but lower concentrations of Cu, Zn, and Sr (Supplementary Figure S5). Differences in the concentration of Category 4 metals (i.e., Mg, Ba, Sr) are likely due to different concentrations of these elements in the larval diets of the wild monarchs. Differences in the bioaccumulating metals from Categories 2 and 3 (i.e., Al, Cd, Pb, Cu, Zn) are likely due to differences in the metal concentrations of the larval diet, metal concentrations in the adult diet, environmental exposure to metals, and age of the butterflies. Of particular interest is Al, which showed much higher concentrations in the wild monarch wings, likely derived from the higher exposure to mineral dust in natural systems than in laboratory conditions. Comparisons of Zn and Na in the monarch wings with concentrations found in monarch abdomens (Shephard et al., 2021) shows that concentrations in the abdomen are approximately three times higher than in the wings. This large difference is likely due to higher allocations of essential Zn and Na to the abdomen for metabolic processes, but could also be influenced by differences in the metal concentrations of the larval diet, unabsorbed metals contained within the digestive tract of the abdomen, and differences in nutrient requirements between environments. Similar differences have been found in beetles, where abdominal samples had higher concentrations of metals than elytra and only some metals were correlated between the tissues (Orłowski et al., 2020).
5. Conclusion
This controlled diet-switching experiment demonstrated that the incorporation of metal and metal isotopes within the wing of monarch butterflies has distinct sources. Some elements (e.g., Mn, Mo, Ca) are likely incorporated from the diet but their concentrations are further modulated throughout the adult life by physiological or metabolic processes. Using those metals and their isotopes (e.g., Ca isotopes) could bring new perspectives on the distinct physiological needs and biogeochemistry of adult butterflies. Other elements are bioaccumulated from the larval and adult diet (e.g., Ni, Zn) and their concentrations keep increasing throughout the adult stage. Many metals also have increasing concentrations through time and come, at least in part, from exogenous sources including aerosols (e.g., Al, Fe, Pb). Using those metals or their isotopes (e.g., Pb) could help track the pollution exposure of individuals either locally or through their migratory travels. Finally, a few metals have concentrations that remain stable in the wing throughout the adult life (e.g., Sr, Mg). Those metals are good candidates for developing geolocation tools in population ecology studies. However, even those metals are not exempt from potential contamination as demonstrated with the rapid exchange of Sr when wings are submerged in water. Despite these caveats, this study supported the use of 87Sr/86Sr ratios as a geolocation tool of natal origin and identified other possible geolocation tools. Finally, this study also underlined the complexity of metal incorporation processes and sources in a single insect species’ tissue. The accumulation of metals absorbed or exchanged by wetting from atmospheric sources is a major incorporation mechanism for several metals in insect wings. Due to the high body surface area to volume ratio of insects and the absorbent properties of their chitinous exoskeleton, this mechanism might be a prominent contributor of metal incorporation in insect tissues with possible toxicological consequences and roles in controlling insect population dynamics.
Data availability statement
The datasets presented in this study can be found in online repositories. The names of the repository/repositories and accession number(s) can be found below: Open Science Foundation (https://doi.org/10.17605/OSF.IO/N4PVM).
Author contributions
MR conceived the idea for the study and led the writing of the manuscript. MR, FD, and HK designed the insect rearing component. MR, CB, MK, FD, and LH designed the metal and metal isotope analysis component. TF and RN collected the wild monarch and milkweed samples. MR, MK, LH, and CB analyzed the data. All authors contributed to the drafts and gave final approval for publication.
Funding
This study was funded by the New Frontiers in Research Fund (CB and GT) and Syngenta Canada, Inc. (RN). GT acknowledges funding from the grant PID2020-117739GA-I00 from MCIN/AEI/10.13039/501100011033 and the grant LINKA20399 from the CSIC iLink program. MR was supported by the Queen Elizabeth II Graduate Scholarship in Science and Technology (QEII-GSST) and an Ontario Graduate Scholarship.
Acknowledgments
We would like to thank Shuanquan Zhang for his expert guidance in operating the MC-ICP-MS at Carleton University, Joseph Spencer for running the Pb columns, Smita Mohanty for help with the ICP-MS, and Emma Brown, Daniela Quintero, and Aldo Camilo Martinez-Becerril for assisting with animal husbandry.
Conflict of interest
This study received funding from Syngenta Canada, Inc. The funder was not involved in the study design, collection, analysis, interpretation of data, the writing of this article or the decision to submit it for publication. All authors declare no other competing interests.
Publisher’s note
All claims expressed in this article are solely those of the authors and do not necessarily represent those of their affiliated organizations, or those of the publisher, the editors and the reviewers. Any product that may be evaluated in this article, or claim that may be made by its manufacturer, is not guaranteed or endorsed by the publisher.
Supplementary material
The Supplementary material for this article can be found online at: https://www.frontiersin.org/articles/10.3389/fevo.2023.1085903/full#supplementary-material
References
Anastopoulos, I., Bhatnagar, A., Bikiaris, D., and Kyzas, G. (2017). Chitin adsorbents for toxic metals: a review. Int. J. Mol. Sci. 18:114. doi: 10.3390/ijms18010114
Andreani, G., Ferlizza, E., Cabbri, R., Fabbri, M., Bellei, E., and Isani, G. (2021). Essential (Mg, Fe, Zn and Cu) and non-essential (Cd and Pb) elements in predatory insects (Vespa crabro and Vespa velutina): a molecular perspective. Int. J. Mol. Sci. 22:228. doi: 10.3390/ijms22010228
Aptekmann, A. A., Buongiorno, J., Giovannelli, D., Glamoclija, M., Ferreiro, D. U., and Bromberg, Y. (2022). Mebipred: identifying metal-binding potential in protein sequence. Bioinformatics 38, 3532–3540. doi: 10.1093/bioinformatics/btac358
Ben-Shahar, Y. (2018). The impact of environmental Mn exposure on insect biology. Front. Genet. 9, 1–7. doi: 10.3389/fgene.2018.00070
Bowden, J., Digby, P. G. N., and Sherlock, P. L. (1984). Studies of elemental composition as a biological marker in insects. I. the influence of soil type and host-plant on elemental composition of Noctua pronuba (Lepidoptera: Noctuidae). Bull. Entomol. Res. 75, 675–687. doi: 10.1017/S0007485300015947
Butt, A., Qurat-ul-Ain Rehman, K., Khan, M. X., and Hesselberg, T. (2018). Bioaccumulation of cadmium, lead, and zinc in agriculture-based insect food chains. Environ. Monit. Assess. 190:698. doi: 10.1007/s10661-018-7051-2
Capo, R. C., Stewart, B. W., and Chadwick, O. A. (1998). Strontium isotopes as tracers of ecosystem processes: theory and methods. Geoderma 82, 197–225. doi: 10.1016/S0016-7061(97)00102-X
Cardoso-Jaime, V., Broderick, N. A., and Maya-Maldonado, K. (2022). Metal ions in insect reproduction: a crosstalk between reproductive physiology and immunity. Curr. Opin. Insect Sci. 52:100924. doi: 10.1016/j.cois.2022.100924
Clark, E. W. (1958). A review of literature on calcium and magnesium in insects. Ann. Entomol. Soc. Am. 51, 142–154. doi: 10.1093/aesa/51.2.142
Consuegra, J., Grenier, T., Baa-Puyoulet, P., Rahioui, I., Akherraz, H., Gervais, H., et al. (2020). Drosophila-associated bacteria differentially shape the nutritional requirements of their host during juvenile growth. PLoS Biol. 18:e3000681. doi: 10.1371/journal.pbio.3000681
Crowley, B. E., Bataille, C. P., Haak, B. A., and Sommer, K. M. (2021). Identifying nesting grounds for juvenile migratory birds with dual isotope: an initial test using North American raptors. Ecosphere 12:e03765. doi: 10.1002/ecs2.3765
Dargent, F., Candau, J. N., Studens, K., Perrault, K. H., Reich, M. S., and Bataille, C. P. (2022). Characterizing eastern spruce budworm’s large-scale dispersal events through flight behavior and stable isotope analyses. BioRxiv Preprint. doi: 10.1101/2022.10.20.513023
Dargent, F., Gilmour, S. M., Brown, E. A., Kassen, R., and Kharouba, H. M. (2021). Low prevalence of the parasite Ophryocystis elektroscirrha at the range edge of the Eastern North American monarch (Danaus plexippus) butterfly population. Can. J. Zool. 99, 409–413. doi: 10.1139/cjz-2020-0175
Davis, A. K., and Holden, M. T. (2015). Measuring intraspecific variation in flight-related morphology of monarch butterflies (Danaus plexippus): which sex has the best flying gear? J. Insects 2015, 1–6. doi: 10.1155/2015/591705
Dempster, J. P., Lakhani, K. H., and Coward, P. A. (1986). The use of chemical composition as a population marker in insects: a study of the brimstone butterfly. Ecol. Entomol. 11, 51–65. doi: 10.1111/j.1365-2311.1986.tb00279.x
Di, N., Hladun, K. R., Zhang, K., Liu, T. X., and Trumble, J. T. (2016). Laboratory bioassays on the impact of cadmium, copper and lead on the development and survival of honeybee (Apis mellifera L.) larvae and foragers. Chemosphere 152, 530–538. doi: 10.1016/j.chemosphere.2016.03.033
Dow, J. A. (2017). The essential roles of metal ions in insect homeostasis and physiology. Curr. Opin. Insect Sci. 23, 43–50. doi: 10.1016/j.cois.2017.07.001
Espeset, A., Kobiela, M. E., Sikkink, K. L., Pan, T., Roy, C., and Snell-Rood, E. C. (2019). Anthropogenic increases in nutrients alter sexual selection dynamics: a case study in butterflies. Behav. Ecol. 30, 598–608. doi: 10.1093/beheco/arz004
Evans, R. D., Wang, W., Evans, H. E., and Georg, R. B. (2017). Variation in Zn, C, and N isotope ratios in three stream insects. Facets 1, 205–216. doi: 10.1139/facets-2016-0023
FAO, WHO. (2019). Codex Alimentarius: International Food Standards. Geneva: World Health Organization.
Fauberteau, A. E., Chartrand, M. M. G., Hu, L., St-Jean, G., and Bataille, C. P. (2021). Investigating a cold case using high-resolution multi-isotope profiles in human hair. Forensic Chem. 22:100300. doi: 10.1016/j.forc.2020.100300
Feindt, W., Oppenheim, S. J., DeSalle, R., Goldstein, P. Z., and Hadrys, H. (2018). Transcriptome profiling with focus on potential key genes for wing development and evolution in Megaloprepus caerulatus, the damselfly species with the world’s largest wings. PLoS One 13, 1–17. doi: 10.1371/journal.pone.0189898
Filipiak, M., and Filipiak, Z. M. (2022). Application of ionomics and ecological stoichiometry in conservation biology: nutrient demand and supply in a changing environment. Biol. Conserv. 272:109622. doi: 10.1016/j.biocon.2022.109622
Filipiak, M., Woyciechowski, M., and Czarnoleski, M. (2021). Stoichiometric niche, nutrient partitioning and resource allocation in a solitary bee are sex-specific and phosphorous is allocated mainly to the cocoon. Sci. Rep. 11:652. doi: 10.1038/s41598-020-79647-7
Flockhart, D. T. T., Kyser, T. K., Chipley, D., Miller, N. G., and Norris, D. R. (2015). Experimental evidence shows no fractionation of strontium isotopes (87Sr/86Sr) among soil, plants, and herbivores: implications for tracking wildlife and forensic science. Isot. Environ. Health Stud. 51, 372–381. doi: 10.1080/10256016.2015.1021345
Flockhart, D. T. T., Wassenaar, L. I., Martin, T. G., Hobson, K. A., Wunder, M. B., and Norris, D. R. (2013). Tracking multi-generational colonization of the breeding grounds by monarch butterflies in Eastern North America. Proc. R. Soc. B 280:20131087. doi: 10.1098/rspb.2013.1087
Hirsch, H. V. B., Mercer, J., Sambaziotis, H., Huber, M., Stark, D. T., Torno-Morley, T., et al. (2003). Behavioral effects of chronic exposure to low levels of lead in Drosophila melanogaster. Neurotoxicology 24, 435–442. doi: 10.1016/S0161-813X(03)00021-4
Holder, P. W., Armstrong, K., Van Hale, R., Millet, M.-A., Frew, R., Clough, T. J., et al. (2014). Isotopes and trace elements as natal origin markers of Helicoverpa armigera-an experimental model for biosecurity pests. PLoS One 9:e92384. doi: 10.1371/journal.pone.0092384
Honegger, H. W., Dewey, E. M., and Ewer, J. (2008). Bursicon, the tanning hormone of insects: recent advances following the discovery of its molecular identity. J. Comp. Physiol. A Neuroethol. Sens. Neural Behav. Physiol. 194, 989–1005. doi: 10.1007/s00359-008-0386-3
Hopkin, S. P. (1990). Critical concentrations, pathways of detoxification and cellular ecotoxicology of metals in terrestrial arthropods. Funct. Ecol. 4:321. doi: 10.2307/2389593
Hu, L., Fernandez, D. P., and Cerling, T. E. (2018). Longitudinal and transverse variation of trace element concentrations in elephant and giraffe hair: implication for endogenous and exogenous contributions. Environ. Monit. Assess. 190:644. doi: 10.1007/s10661-018-7038-z
Hu, L., Fernandez, D. P., and Cerling, T. E. (2019). Trace element concentrations in horn: endogenous levels in keratin and susceptibility to exogenous contamination. Chemosphere 237:124443. doi: 10.1016/j.chemosphere.2019.124443
Hu, L., Fernandez, D. P., Cerling, T. E., and Tipple, B. J. (2020). Fast exchange of strontium between hair and ambient water: implication for isotopic analysis in provenance and forensic studies. PLoS One 15, e0233712–e0233714. doi: 10.1371/journal.pone.0233712
Huang, X., (2020). Characterization of Drywall Products for Assessing Impacts Associated with End-of-life Management. Washington, DC: U.S. Environmental Protection Agency.
Jin, P., Chen, J., Zhan, H., Huang, S., Wang, J., and Shu, Y. (2020). Accumulation and excretion of zinc and their effects on growth and food utilization of Spodoptera litura (Lepidoptera: Noctuidae). Ecotoxicol. Environ. Saf. 202:110883. doi: 10.1016/j.ecoenv.2020.110883
Joern, A., Provin, T., and Behmer, S. T. (2012). Not just the usual suspects: insect herbivore populations and communities are associated with multiple plant nutrients. Ecology 93, 1002–1015. doi: 10.1890/11-1142.1
Judd, T. M., Magnus, R. M., and Fasnacht, M. P. (2010). A nutritional profile of the social wasp Polistes metricus: differences in nutrient levels between castes and changes within castes during the annual life cycle. J. Insect Physiol. 56, 42–56. doi: 10.1016/j.jinsphys.2009.09.002
Kabata-Pendias, A., and Mukherjee, A. B., (2007). Trace Elements from Soil to Human. Springer, Berlin, Heidelberg.
Kaspari, M. (2020). The seventh macronutrient: how sodium shortfall ramifies through populations, food webs and ecosystems. Ecol. Lett. 23, 1153–1168. doi: 10.1111/ele.13517
Kaspari, M. (2021). The invisible hand of the periodic Table: how micronutrients shape ecology. Annu. Rev. Ecol. Evol. Syst. 52, 199–219. doi: 10.1146/annurev-ecolsys-012021-090118
Kempson, I. M., Skinner, W. M., and Kirkbride, K. P. (2006). Advanced analysis of metal distributions in human hair. Environ. Sci. Technol. 40, 3423–3428. doi: 10.1021/es052158v
Kobiela, M. E., and Snell-rood, E. C. (2018). Integrative and comparative biology nickel exposure has complex transgenerational effects in a butterfly. Integr. Comp. Biol. 58, 1008–1017. doi: 10.1093/icb/icy096
Kowalski, R., Davies, S. E., and Hawkes, C. (1989). Metal composition as a natural marker in anthomyiid fly Delia radicum (L.). J. Chem. Ecol. 15, 1231–1239. doi: 10.1007/BF01014825
Kula, E., Martinek, P., Chromcová, L., and Hedbávný, J. (2014). Development of Lymantria dispar affected by manganese in food. Environ. Sci. Pollut. Res. 21, 11987–11997. doi: 10.1007/s11356-014-3075-5
Lin, T., Chen, P., Chen, X., Shen, J., Zhong, S., Sun, Q., et al. (2021). Geographical classification of Helicoverpa armigera (Lepidoptera: Noctuidae) through mineral component analysis. Anal. Lett. 54, 669–683. doi: 10.1080/00032719.2020.1777560
Lin, T., Chen, X., Li, B., Chen, P., Guo, M., Zhou, X., et al. (2019). Geographical origin identification of Spodoptera litura (Lepidoptera: Noctuidae) based on trace element profiles using tobacco as intermedium planted on soils from five different regions. Microchem. J. 146, 49–55. doi: 10.1016/j.microc.2018.12.051
Lindroos, E. E., Bataille, C. P., Holder, P. W., Talavera, G., and Reich, M. S. ((2023). Temporal stability of δ2h in insect tissues: implications for isotope-based geographic assignments. Front. Ecol. Evol. 11:1060836. doi: 10.3389/fevo.2023.1060836
Maret, W., (2016). Metallomics: A Primer of Integrated Biometal Sciences. London: Imperial College Press.
Martinek, P., Hedbávný, J., Kudláček, T., Šťasta, M., and Kula, E. (2020). Adverse responses of Cabera pusaria caterpillars to high dietary manganese concentration. Entomol. Exp. Appl. 168, 635–643. doi: 10.1111/eea.12919
McLean, J. A., Laks, P., and Shore, T. L. (1983). “Comparisons of elemental profiles of the western spruce budworm reared on three host foliages and artificial medium” in Proceedings, Forest defoliator Host Interactions: A Comparison between Gypsy Moth and Spruce Budworms. eds. R. L. Talerico and M. Montgomery (Broomall, PA: U.S. Department of Agriculture), 33–40.
Merritt, T. J. S., and Bewick, A. J. (2017). Genetic diversity in insect metal tolerance. Front. Genet. 8, 1–6. doi: 10.3389/fgene.2017.00172
Mesjasz-Przybyłowicz, J., Orłowska, E., Augustyniak, M., Nakonieczny, M., Tarnawska, M., Przybyłowicz, W., et al. (2014). Elemental distribution in reproductive and neural organs of the Epilachna nylanderi (Coleoptera: Coccinellidae), a phytophage of nickel hyperaccumulator Berkheya coddii (Asterales: Asteraceae) by micro-PIXE. J. Insect Sci. 14:152. doi: 10.1093/jisesa/ieu014
Mitchell, T. S., Agnew, L., Meyer, R., Sikkink, K. L., Oberhauser, K. S., Borer, E. T., et al. (2020). Traffic influences nutritional quality of roadside plants for monarch caterpillars. Sci. Total Environ. 724:138045. doi: 10.1016/j.scitotenv.2020.138045
Mogren, C. L., and Trumble, J. T. (2010). The impacts of metals and metalloids on insect behavior. Entomol. Exp. Appl. 135, 1–17. doi: 10.1111/j.1570-7458.2010.00967.x
Monchanin, C., Devaud, J. M., Barron, A. B., and Lihoreau, M. (2021). Current permissible levels of metal pollutants harm terrestrial invertebrates. Sci. Total Environ. 779:146398. doi: 10.1016/j.scitotenv.2021.146398
Moore, L. J., Murphy, T. J., Barnes, I. L., and Paulsen, P. J. (1982). Absolute isotopic abundance ratios and atomic weight of a reference sample of strontium. J. Res. Natl. Bur. Stand. 87, 1–8. doi: 10.6028/jres.087.001
Nakano, T., Jeon, S.-R., Shindo, J., Fumoto, T., Okada, N., and Shimada, J. (2001). Sr isotopic signature in plant-derived Ca in rain. Water Air Soil Pollut. 130, 769–774. doi: 10.1023/A:1013849905563
Negri, I., Mavris, C., Di Prisco, G., Caprio, E., and Pellecchia, M. (2015). Honey bees (Apis mellifera, L.) as active samplers of airborne particulate matter. PLoS One 10:e0132491. doi: 10.1371/journal.pone.0132491
Nessel, M. P., Konnovitch, T., Romero, G. Q., and González, A. L. (2021). Nitrogen and phosphorus enrichment cause declines in invertebrate populations: a global meta-analysis. Biol. Rev. 96, 2617–2637. doi: 10.1111/brv.12771
Nickles, E. P., Ghiradella, H., Bakhru, H., and Haberl, A. (2002). Egg of the Karner blue butterfly (Lycaeides melissa samuelis): morphology and elemental analysis. J. Morphol. 251, 140–148. doi: 10.1002/jmor.1079
Nitzsche, K. N., Shin, K. C., Kato, Y., Kamauchi, H., Takano, S., and Tayasu, I. (2020). Magnesium and zinc stable isotopes as a new tool to understand Mg and Zn sources in stream food webs. Ecosphere 11, 1–20. doi: 10.1002/ecs2.3197
Nitzsche, K. N., Wakaki, S., Yamashita, K., Shin, K. C., Kato, Y., Kamauchi, H., et al. (2022). Calcium and strontium stable isotopes reveal similar behaviors of essential Ca and nonessential Sr in stream food webs. Ecosphere 13, 1–19. doi: 10.1002/ecs2.3921
Norris, D. R., Lank, D. B., Pither, J., Chipley, D., Ydenberg, R. C., and Kyser, T. K. (2007). Trace element profiles as unique identifiers of western sandpiper (Calidris mauri) populations. Can. J. Zool. 85, 579–583. doi: 10.1139/Z07-024
Ogra, Y., and Hirata, T. (Eds.), (2017). Metallomics: Recent Analytical Techniques and Applications. Springer, Japan, Tokyo.
Orłowski, G., Mróz, L., Kadej, M., Smolis, A., Tarnawski, D., Karg, J., et al. (2020). Breaking down insect stoichiometry into chitin-based and internal elemental traits: patterns and correlates of continent-wide intraspecific variation in the largest European saproxylic beetle. Environ. Pollut. 262:114064. doi: 10.1016/j.envpol.2020.114064
Otuka, A., Dudhia, J., Watanabe, T., and Furuno, A. (2005). A new trajectory analysis method for migratory planthoppers, Sogatella furcifera (Horváth) (Homoptera: Delphacidae) and Nilaparvata lugens (Stål), using an advanced weather forecast model. Agric. For. Entomol. 7, 1–9. doi: 10.1111/j.1461-9555.2005.00236.x
Pass, G. (2018). Beyond aerodynamics: the critical roles of the circulatory and tracheal systems in maintaining insect wing functionality. Arthropod Struct. Dev. 47, 391–407. doi: 10.1016/j.asd.2018.05.004
Phillips, B. B., Bullock, J. M., Gaston, K. J., Hudson-Edwards, K. A., Bamford, M., Cruse, D., et al. (2021). Impacts of multiple pollutants on pollinator activity in road verges. J. Appl. Ecol. 58, 1017–1029. doi: 10.1111/1365-2664.13844
Prather, C. M., Laws, A. N., Cuellar, J. F., Reihart, R. W., Gawkins, K. M., and Pennings, S. C. (2018). Seeking salt: herbivorous prairie insects can be co-limited by macronutrients and sodium. Ecol. Lett. 21, 1467–1476. doi: 10.1111/ele.13127
R Core Team. (2013). R: A Language and Environment for Statistical Computing. R Foundation for Statistical Computing, Vienna, Austria.
Reich, M. S., Flockhart, D. T. T., Norris, D. R., Hu, L., and Bataille, C. P. (2021). Continuous-surface geographic assignment of migratory animals using strontium isotopes: a case study with monarch butterflies. Methods Ecol. Evol. 12, 2445–2457. doi: 10.1111/2041-210X.13707
Reihart, R. W., Angelos, K. P., Gawkins, K. M., Hurst, S. E., Montelongo, D. C., Laws, A. N., et al. (2021). Crazy ants craving calcium: macronutrients and micronutrients can limit and stress an invaded grassland brown food web. Ecology 102:e03263. doi: 10.1002/ecy.3263
Rodiouchkina, K., Rodushkin, I., Goderis, S., and Vanhaecke, F. (2022). Longitudinal isotope ratio variations in human hair and nails. Sci. Total Environ. 808:152059. doi: 10.1016/j.scitotenv.2021.152059
Rudnick, R. L., and Gao, S. (2014). “Composition of the continental crust” in Treatise on Geochemistry. eds. H. D. Holland and K. K. Turekian (Amsterdam, Netherlands: Elsevier Ltd.), 1–51.
Seewagen, C. L. (2020). The threat of global mercury pollution to bird migration: potential mechanisms and current evidence. Ecotoxicology 29, 1254–1267. doi: 10.1007/s10646-018-1971-z
Selvey, A. D.. (2020). Transcriptomic Exploration of the Vanessa cardui Immune System. University of Nevada, Reno.
Shaw, J. A., Boyd, A., House, M., Cowin, G., and Baer, B. (2018). Multi-modal imaging and analysis in the search for iron-based magnetoreceptors in the honeybee Apis mellifera. R. Soc. Open Sci. 5, 1–13. doi: 10.1098/rsos.181163
Shephard, A. M., Brown, N. S., and Snell-Rood, E. C. (2022). Anthropogenic zinc exposure increases mortality and antioxidant gene expression in monarch butterflies with low access to dietary macronutrients. Environ. Toxicol. Chem. 41, 1286–1296. doi: 10.1002/etc.5305
Shephard, A. M., Mitchell, T. S., Henry, S. B., Oberhauser, K. S., Kobiela, M. E., and Snell-Rood, E. C. (2020). Assessing zinc tolerance in two butterfly species: consequences for conservation in polluted environments. Insect Conserv. Divers. 13, 201–210. doi: 10.1111/icad.12404
Shephard, A. M., Mitchell, T. S., and Snell-rood, E. C. (2021). Monarch caterpillars are robust to combined exposure to the roadside micronutrients sodium and zinc. Conserv. Physiol. 9, 1–15. doi: 10.1093/conphys/coab061
Simonetti, A., Gariépy, C., and Carignan, J. (2000a). Pb and Sr isotopic evidence for sources of atmospheric heavy metals and their deposition budgets in Northeastern North America. Geochim. Cosmochim. Acta 64, 3439–3452. doi: 10.1016/S0016-7037(00)00446-4
Simonetti, A., Gariépy, C., and Carignan, J. (2000b). Pb and Sr isotopic compositions of snowpack from Québec, Canada: inferences on the sources and deposition budgets of atmospheric heavy metals. Geochim. Cosmochim. Acta 64, 5–20. doi: 10.1016/S0016-7037(99)00207-0
Slobodian, M. R., Petahtegoose, J. D., Wallis, A. L., Levesque, D. C., and Merritt, T. J. S. (2021). The effects of essential and non-essential metal toxicity in the Drosophila melanogaster insect model: a review. Toxics 9:269. doi: 10.3390/toxics9100269
Smith, K. E., and Weis, D. (2020). Evaluating spatiotemporal resolution of trace element concentrations and Pb isotopic compositions of honeybees and hive products as biomonitors for urban metal distribution. GeoHealth 4:e2020GH000264. doi: 10.1029/2020GH000264
Smith, K. E., Weis, D., Scott, S. R., Berg, C. J., Segal, Y., and Claeys, P. (2021). Regional and global perspectives of honey as a record of lead in the environment. Environ. Res. 195:110800. doi: 10.1016/j.envres.2021.110800
Snell-Rood, E. C., Espeset, A., Boser, C. J., White, W. A., and Smykalski, R. (2014). Anthropogenic changes in sodium affect neural and muscle development in butterflies. PNAS 111, 10221–10226. doi: 10.1073/pnas.1323607111
Studier, E. H. (1996). Composition of bodies of cave crickets (Hadenoecus subterraneus), their eggs, and their egg predator, Neaphaenops tellkampfi. Am. Midl. Nat. 136, 101–109. doi: 10.2307/2426635
Stuhr, S., Truong, V. K., Vongsvivut, J., Senkbeil, T., Yang, Y., Al Kobaisi, M., et al. (2018). Structure and chemical organization in Damselfly Calopteryx haemorrhoidalis wings: a spatially resolved FTIR and XRF analysis with synchrotron radiation. Sci. Rep. 8, 8413–8419. doi: 10.1038/s41598-018-26563-6
Suchan, T., Talavera, G., Sáez, L., Ronikier, M., and Vila, R. (2018). Pollen metabarcoding as a tool for tracking long-distance insect migrations. Mol. Ecol. Resour. 19, 149–162. doi: 10.1111/1755-0998.12948
Sun, H. X., Tang, W. C., Chen, H., Chen, W., Zhang, M., Liu, X., et al. (2013). Food utilization and growth of cutworm Spodoptera litura Fabricius larvae exposed to nickel, and its effect on reproductive potential. Chemosphere 93, 2319–2326. doi: 10.1016/j.chemosphere.2013.08.025
Sures, B. (2004). Environmental parasitology: relevancy of parasites in monitoring environmental pollution. Trends Parasitol. 20, 170–177. doi: 10.1016/j.pt.2004.01.014
Tacail, T., Le Houedec, S., and Skulan, J. L. (2020). New frontiers in calcium stable isotope geochemistry: perspectives in present and past vertebrate biology. Chem. Geol. 537:119471. doi: 10.1016/j.chemgeo.2020.119471
Tanaka, Y. K., and Hirata, T. (2018). Stable isotope composition of metal elements in biological samples as tracers for element metabolism. Anal. Sci. 34, 645–655. doi: 10.2116/analsci.18SBR02
Tibbett, M., Green, I., Rate, A., De Oliveira, V. H., and Whitaker, J. (2021). The transfer of trace metals in the soil-plant-arthropod system. Sci. Total Environ. 779:146260. doi: 10.1016/j.scitotenv.2021.146260
Tigar, B. J., and Hursthouse, A. (2016). Applying biogeochemistry to identify the geographic origins of insects-a model using Prostephanus truncatus. J. Environ. Stud. 2:9. doi: 10.13188/2471-4879.1000004
Todt, W., Cliff, R. A., Hanser, A., and Hofmann, A. W.. (1996). Evaluation of a 202Pb–205Pb Double Spike for High - Precision Lead Isotope Analysis. American Geophysical Union, Washington, DC, 429–437.
Tsai, C.-C., Childers, R. A., Nan Shi, N., Ren, C., Pelaez, J. N., Bernard, G. D., et al. (2020). Physical and behavioral adaptations to prevent overheating of the living wings of butterflies. Nat. Commun. 11, 551–514. doi: 10.1038/s41467-020-14408-8
Van Gorkum, R., and Bouwman, E. (2005). The oxidative drying of alkyd paint catalysed by metal complexes. Coord. Chem. Rev. 249, 1709–1728. doi: 10.1016/j.ccr.2005.02.002
Wanty, R. B., Balistrieri, L. S., Wesner, J. S., Walters, D. M., Schmidt, T. S., Stricker, C. A., et al. (2017). In vivo isotopic fractionation of zinc and biodynamic modeling yield insights into detoxification mechanisms in the mayfly Neocloeon triangulifer. Sci. Total Environ. 609, 1219–1229. doi: 10.1016/j.scitotenv.2017.07.269
Welti, E. A. R., Roeder, K. A., de Beurs, K. M., Joern, A., and Kaspari, M. (2020). Nutrient dilution and climate cycles underlie declines in a dominant insect herbivore. Proc. Natl. Acad. Sci. U. S. A. 117, 7271–7275. doi: 10.1073/pnas.1920012117
Wieringa, J. G., Nagel, J., Nelson, D. M., Carstens, B. C., and Gibbs, H. L. (2020). Using trace elements to identify the geographic origin of migratory bats. PeerJ 8, e10082–e10023. doi: 10.7717/peerj.10082
Xiao, G., and Zhou, B. (2016). What can flies tell us about zinc homeostasis? Arch. Biochem. Biophys. 611, 134–141. doi: 10.1016/j.abb.2016.04.016
Zhang, Y., Ying, H., and Xu, Y. (2019). Comparative genomics and metagenomics of the metallomes. Metallomics 11, 1026–1043. doi: 10.1039/c9mt00023b
Zhou, J., Chen, J., and Shu, Y. (2021). Lead stress affects the reproduction of Spodoptera litura but not by regulating the vitellogenin gene promoter. Ecotoxicol. Environ. Saf. 208:111581. doi: 10.1016/j.ecoenv.2020.111581
Keywords: metal isotopes, strontium isotopes (87Sr/86Sr), lead isotopes, isotope-based geographic assignment, chemoprint, monarch butterfly (Danaus plexippus), metal pollution
Citation: Reich MS, Kindra M, Dargent F, Hu L, Flockhart DTT, Norris DR, Kharouba H, Talavera G and Bataille CP (2023) Metals and metal isotopes incorporation in insect wings: Implications for geolocation and pollution exposure. Front. Ecol. Evol. 11:1085903. doi: 10.3389/fevo.2023.1085903
Edited by:
Keith Alan Hobson, Western University, CanadaReviewed by:
Stefan Siebert, North-West University, South AfricaMichał Filipiak, Jagiellonian University, Poland
Copyright © 2023 Reich, Kindra, Dargent, Hu, Flockhart, Norris, Kharouba, Talavera and Bataille. This is an open-access article distributed under the terms of the Creative Commons Attribution License (CC BY). The use, distribution or reproduction in other forums is permitted, provided the original author(s) and the copyright owner(s) are credited and that the original publication in this journal is cited, in accordance with accepted academic practice. No use, distribution or reproduction is permitted which does not comply with these terms.
*Correspondence: Megan S. Reich, ✉ bWVnYW4ucmVpY2hAdW90dGF3YS5jYQ==; Clément P. Bataille, ✉ Y2JhdGFpbGxAdW90dGF3YS5jYQ==