- 1Bend Field Office, Oregon Fish and Wildlife Office, U.S. Fish and Wildlife Service, Bend, OR, United States
- 2Ohio Center for Ecology and Evolutionary Studies, Department of Biological Sciences, Ohio University, Athens, OH, United States
Maintaining viable populations of large reptiles is often challenging in road fragmented landscapes. While mitigation structures can reduce impacts, few studies have investigated how mitigation success can be affected by roadside habitats. In southeast Ohio, USA, we evaluated mitigation effectiveness for state-endangered timber rattlesnakes (Crotalus horridus) at a new highway in a forested landscape. Road construction at the study site created a wide corridor of open canopy habitats (the right-of-way; ROW) containing roadcuts and stone piles. However, exclusion fencing was constructed along the forest-ROW boundary, leaving the open canopy habitats on the road-side of the fence. Over three years, we monitored 6 rattlesnakes using radiotelemetry and found that rattlesnakes repeatedly crossed the fence to access forest-edge and ROW habitats. Rattlesnakes ostensibly crossed through damaged sections of the fence. The ROW was used most intensively by gravid females (n = 2), with their core home ranges overlapping the ROW by more than 50 percent. Despite the fence crossings, all home ranges were bounded by the highway and no rattlesnake road mortality was observed. Operative temperature models revealed that the ROW provided warmer thermal regimes that were rare or unavailable in the forest. On average, field preferred gestation temperatures (Tb = 29.7°C, SD = 1.8) could be attained or exceeded for more than 5 times as many hours per day in the ROW (7.8 hours) than in the forest (1.4 hours). Habitat selection models indicated gravid females selected warmer thermal habitats that were spatially concentrated in the ROW and edge habitats, while non-gravid snakes avoided the ROW beyond the forest edge. Habitat use within the ROW was mostly limited to rocky microhabitat structures, especially riprap stone piles and subsurface rock crevices on roadcuts, which provided buffered thermal regimes with refugia from extreme temperatures during the day and warmer Te through the night. In forested landscapes, we encourage road planners to consider whether new road corridors are likely to introduce basking sites, and if so, maintain those features on the habitat-side of exclusion fencing, and consider restoring basking sites in the surrounding forest to reduce the potential for ecological trap formation.
1 Introduction
Road fragmented landscapes are becoming increasingly ubiquitous, with at least 25 million kilometers of new roads anticipated by 2050, representing a 60% increase to the global road network (Laurance et al., 2014). The continued expansion of the global road network stresses many wildlife populations through direct effects on survivorship and indirect effects resulting from landscape change (Forman and Alexander, 1998; Watson et al., 2018). Roads commonly impact wildlife populations through wildlife-vehicle collisions, population fragmentation, and resource loss and isolation (Forman and Alexander, 1998; Forman et al., 2003; Marsh and Jaeger, 2015). While modified and fragmented landscapes fail to meet traditional definitions of wilderness, they often retain some native biodiversity and are proving to be more valuable in landscape conservation strategies than once thought (Neilly et al., 2016; Wintle et al., 2018; Phillips et al., 2020; New et al., 2021).
The conservation value of road fragmented landscapes is likely enhanced when paired with effective mitigation (Clevenger et al., 2001; Dodd et al., 2004; Jackson et al., 2015). To sustain wildlife populations in road fragmented landscapes, best management practices often recommend exclusion fencing and crossing structures as means of reducing wildlife-vehicle collisions and maintaining ecological connectivity (Rajvanshi et al., 2001; Huijser et al., 2008; Clevenger and Huijser, 2011; Gunson et al., 2016; Boyle et al., 2021). These mitigation designs often consider the landscape context from the perspective of eliminating mortality hotspots and protecting animal movement corridors (e.g., Gunson et al., 2012).
An often-overlooked consideration of mitigation design is how roadside habitats, often created and maintained as part of the right-of-way (ROW), function within landscapes and affect mitigation success. We consider ROWs generically as human-made linear corridors maintained to service transportation infrastructure or utilities, including roads, gas pipelines, and electrical transmission lines. These corridors are often maintained through vegetation control, especially the removal of overstory trees. Road construction typically results in the loss, fragmentation, and modification of habitats, but in certain landscapes the road ROW may generate novel heterogeneity that serves as functional habitat or even refuge in an inhospitable matrix (Clevenger and Huijser, 2011; Kasten et al., 2016).
By altering the availability and configuration of habitats, roads can affect animal behaviors, such as resource selection and movement (Sartorius et al., 1999; Klingenböck et al., 2000; Berger, 2007; Andrews et al., 2008; Abrahms et al., 2016). One of the most common resources altered by roads is the thermal environment. In forested landscapes, vegetation is often controlled along roadsides, maintaining open canopy corridors of early successional vegetation and edge habitats exposed to increased insolation (Trombulak and Frissell, 2000; Forman et al., 2003; Harper et al., 2005; Kearney et al., 2009). In mountainous regions, the geophysical environment is often altered through earth-moving to create passes at reduced grades, leaving exposed rock escarpments adjacent to the road at modified slopes and aspects (roadcuts), and thus altering heat load (Sears et al., 2011). Stone piles (riprap) are sometimes dumped along the roadside to direct drainage and control erosion, but also function as buffered microhabitats that can serve as heat sinks (Huey et al., 1989). These thermal resources maintained in road corridors and other ROWs are sometimes used or preferred by heliothermic ectotherms, including forest-dwelling reptiles that require basking sites and or thermally exposed nesting habitat (Sartorius et al., 1999; Klingenböck et al., 2000; Blouin-Demers and Weatherhead, 2001; Shine et al., 2002). Because thermal resources can directly affect physiology and behavior, especially in ectotherms (Huey, 1982; Huey, 1991; Angilleta, 2009), such roadside habitats have the potential to benefit wildlife populations when these resources are otherwise scarce on the landscape.
However, when roadside habitats attract animals to the proximity of roads, use of those resources can come at the cost of increased wildlife-vehicle collisions or mortality from other causes (Langen et al., 2015). From this follows three important considerations: 1) if roadside areas provide habitat resources selected by wildlife, they could function as either compensatory habitats or ecological traps depending upon the cost of use (Jackson et al., 2015); 2) efficacy of road mitigation structures, particularly exclusion fencing, may be affected by roadside habitat composition and configuration relative to the landscape; and 3) effective placement of mitigation structures may allow would-be traps to function as viable habitat. In summary, if road corridors can provide habitat resources and the primary cost of using those habitats is road mortality, then it may be important for mitigation structures to allow access to roadside habitats while preventing access to the roadway itself.
One species that exemplifies roadside habitat tradeoffs is the timber rattlesnake (=Crotalus horridus). Timber rattlesnakes are large forest-dwelling snakes native to eastern North America and reported to be declining by the IUCN Red List. While numerous studies report negative demographic and genetic responses to roads (Rudolph et al., 1999; Clark et al., 2010; Bushar et al., 2015), other researchers have reported timber rattlesnakes to use roadcuts and other open canopy habitats found along roadsides, particularly by gravid (pregnant) individuals (Brown, 1993; Reinert and Zappalorti, 1998; Anderson, 2010). The species shows stark intraspecific variation in habitat preferences, ostensibly due to their foraging and reproductive strategies that are seemingly made exclusive by thermal constraints (Gardner-Santana and Beaupre, 2009). Timber rattlesnakes are ambush predators, and often remain sedentary for multiple days while waiting for prey (Reinert et al., 1984). This foraging strategy may drive preference for forested habitats with high canopy closure where thermoconforming will not result in exceeding thermal tolerances, forcing individuals to abandon an ambush site or expend excess energy metabolically. In contrast, gravid females maintain precise and elevated body temperatures that facilitate embryonic development (Gardner-Santana and Beaupre, 2009). Throughout pregnancy, gravid females often forgo foraging and show preference for canopy gaps that feature rock or woody microhabitat cover, providing basking sites with refuge where individuals can actively thermoregulate (Reinert, 1984; Herr et al., 2020). Thus, it would be expected that creation of an open canopy ROW could affect conspecifics differently by generating gestation habitat at the cost of foraging habitat. Landscape heterogeneity introduced by ROWs could thus be important to populations where basking sites are sparsely distributed across the landscape. However, because the species’ life history is also characterized by delayed maturity, high adult survival, and infrequent reproduction (Ernst and Ernst, 2003), use of these roadside habitats could easily compromise population viability if it results in additive mortality.
Few studies have investigated how road mitigation design and roadside habitats interact to affect animal behavior and mitigation performance in snakes (Macpherson et al., 2021). We evaluated the effects of a mitigated highway on timber rattlesnakes in southeast Ohio, where the species is listed as Endangered under State law (Ohio Revised Code 1531.25). We report how a mitigated highway affected movement and space use by rattlesnakes, and how thermal resources impacted mitigation success as a function of exclusion fence design and placement. We predicted that thermal conditions preferred by gravid females would be available for more hours of the day in the ROW and edge habitats compared with the surrounding forest, and that preferred gestation habitats would be concentrated within the ROW. We also predicted that surface habitats within the ROW would exceed voluntary thermal tolerances, and that non-gravid snakes would avoid those habitats.
2 Materials and methods
2.1 Study site
We studied timber rattlesnakes in the Wayne National Forest (WNF) in Ohio, USA at the Nelsonville Bypass (NVBP). The WNF comprises 271 km2 of non-contiguous forestland distributed across southeastern Ohio. The NVBP is a high speed (112 km/h), high volume (17,000 vehicles/day), four-lane divided highway (13.6 km length) that was constructed in WNF between 2007 and 2013, and was opened to traffic in October, 2013 (for a map of the study site, see Supplementary Figure 1). The woodland along the NVBP is an Oak-Hickory (Quercus-Carya) deciduous forest with rolling hills that feature sandstone outcroppings that are mostly shaded by overstory trees. The NVBP fragmented the forest landscape and created a large ROW (approximately 250 ha) of predominantly open canopy habitats. We define the NVBP ROW as the modified habitat area created during road construction, and not the paved road itself. Along the NVBP, the width of the ROW between the road and the forest ranged from 5–150 m on either side of the highway. The ROW habitats included road cuts of exposed sandstone, early successional stands of black locust (Robinia pseudoacacia) and sumac (Rhus sp.), fields dominated by mixed grasses and weedy vegetation (Poaceae, Asteraceae), barren slopes with exposed soils, and large riprap stone piles installed for erosion and drainage control (for photos of these habitats, see Supplementary Figures 2, 3).
Timber rattlesnakes were known to occur in this part of WNF based on incidental encounters reported to the Ohio Department of Natural Resources, but little was known about the population prior to construction of the NVBP. Pre-construction surveys (200 person hours) performed by local wildlife consultants failed to detect timber rattlesnakes in the project area, but two individuals were discovered within the ROW during construction. The Ohio Department of Transportation (ODOT) installed rattlesnake mitigation structures along 1.6 km of the NVBP in the area where rattlesnakes were encountered (described below). While parts of our research spanned the entire 13.6 km length of the NVBP, we focused most of our rattlesnake field work around the 1.6 km section of highway that was mitigated for rattlesnakes, and the adjacent ROW and forest habitats.
2.2 Mitigation design
To prevent deer and other large wildlife from entering the roadway, ODOT constructed a 2.4 m tall exclusion fence (“wildlife fence”) along the entire 13.6 km length of NVBP. The wildlife fence was installed along the ROW-forest boundary (i.e., at the forest edge), and thus ranged from 5–150 m away from the road. However, the mesh of the wildlife fence was approximately 15 cm by 15 cm (height by width), making it permeable to smaller wildlife including rattlesnakes. To prevent rattlesnakes from entering the ROW, ODOT installed a rattlesnake exclusion fence (“snake fence”; 6.35 mm mesh galvanized hardware cloth, 0.9–1.2 m tall, buried < 0.2 m into the ground) along both sides of the highway for 1.6 km spanning the area where rattlesnakes were observed during construction (for maps and photos, see Supplementary Figures 1, 4). The eastern half of the snake fence was attached to the base of the wildlife fence and traversed steep terrain across the ROW-forest boundary, while the western half of the snake fence was built as a free standing fence (detached from the wildlife fence) and traveled closer to the road within the ROW (7–100 m from the road). This western section of the snake fence was rerouted away from the primary wildlife fence to protect a suspected rattlesnake overwintering site in the area.
Five wildlife crossing structures (“ecopassages”) were installed along the NVBP. Two of these structures were corrugated steel culverts (52 m long, 1.2 m diameter) designated as small wildlife crossings (SWC) to accommodate smaller wildlife including rattlesnakes. The SWCs featured a grated ceiling at the road median that provided some natural lighting, but conditions within the ecopassage remained poorly lit (for maps and photos, see Supplementary Figures 1, 5). The other three crossings included a gas-line underpass, a large wildlife culvert (e.g. deer), and an all-terrain vehicle underpass. Of the ecopassages along the NVBP, only one of the SWCs interfaced with the snake fence and overlapped the known area of rattlesnake activity. Therefore, 4 of the 5 crossing structures lacked fencing to direct small wildlife such as snakes to the crossing structures.
2.3 Rattlesnake capture
We deployed 32 tin coverboard piles and 12 two-way box traps (Grant et al., 1992; Burgdorf et al., 2005) along the snake fence to live trap and capture rattlesnakes from 2015 through 2017. Traps and coverboards distributed on both the forest and ROW sides of the snake fence. Traps and coverboard piles were spaced 100–150 m apart along the snake fence. Trap placement was partially constrained by fence integrity and terrain. Traps were placed along priority sections of fencing where the fence remained structurally intact, as the fencing was needed to act as a drift fence, diverting snakes to the traps. We avoided placing traps on steep slopes to ensure that traps could be checked safely and would remain flush against the ground. In 2015, traps were distributed along the snake fence on both the westbound and eastbound sides of the highway (6 box traps and 16 coverboard piles on each side of the NVBP). In 2016, all 12 box traps were relocated to the westbound side of the highway due to a lack of trapping success on the eastbound side and higher quality rattlesnake habitat (warmer aspects and den sites) occurring near the snake fencing on the westbound side. Traps were monitored throughout the rattlesnake activity seasons, which began around late April when rattlesnakes emerged (egress) from their winter hibernacula (den) and concluded around early October when rattlesnakes returned (ingress) to their dens. Exact dates of egress and ingress varied from year to year depending on the individual and weather.
2.4 Radio telemetry
We used radio telemetry to detect rattlesnake fence crossings, ecopassages use, mortality and habitat use. Adult and large sub-adult rattlesnakes were implanted with temperature-sensitive Advanced Telemetry Systems® R1680 transmitters (transmitters measured 3.6 g, < 1% body mass for adult rattlesnakes and < 1.5% body mass for subadult rattlesnakes used in our study; Reinert and Cundall, 1982). We used snout-to-vent length (SVL) to determine maturity based on Brown (1991) and Aldridge and Brown (1995) (adult males ≥ 78 cm SVL; adult females ≥ 84 cm SVL). After surgery, snakes were released at the point of capture following a two to seven day recovery period. Snakes were relocated via VHF telemetry (with visual confirmation whenever possible) approximately three times per week (every 48 to 72 hours) throughout the activity season, and GPS locations were recorded using a Garmin® GPS Map 64 (3 m accuracy).
2.5 Additional measures to assess mitigation performance
While radio telemetry was our primary method for monitoring the fate and movement of known individuals, we further evaluated mitigation performance using road mortality surveys and camera monitoring of the ecopassages. We surveyed for road mortality by driving the 13.6 km length of the NVBP in both directions 5 to 7 days/week throughout the rattlesnake activity season (mid-April through mid-October in 2015 and 2016), driving in the right lane at reduced speeds (48–80 kmph depending on traffic conditions) while scouting for rattlesnakes and other reptiles crossing or dead on the road (DOR). After finding a carcass, we documented the species, GPS location, whether it was in the snake fenced area, and when possible, sex and age class. From 2015 through 2016, we used IR Buckeye Game Cameras® placed at both entrances and at the middle of each SWC (three cameras per SWC) to monitor wildlife movement. Cameras were bolted to the ceiling of the culvert, angled downward, and set to detect motion (see the Supplementary Materials for additional information on our analysis of road mortality and wildlife crossing data).
2.6 Evaluating space use
A combination of home range estimation techniques were used to quantify space use relative to the mitigation structures. We generated 100% minimum convex polygons (MCP) for all rattlesnakes each activity season to evaluate whether maximum length of home ranges exceeded the length of snake fencing or occurred in areas that do not overlap snake fencing, which would indicate that the fencing was not sufficient in extent. We also generated 50% kernel density estimators (KDE; Worton, 1989) to calculate the overlap of core activity areas with the ROW to evaluate if important activity centers occurred within the exclusion zone. We generated KDE using the PLUGIN bandwidth operator (Millspaugh et al., 2012). We did not produce KDE home ranges for individuals captured late in the activity season (after early July), as incomplete activity season data would have the potential to misidentify important activity centers. All home ranges were generated in R (version 4.1.2; R Core Development Team, 2022) using the AdehabitatHR package for MCPs (version 0.4.19; Calenge, 2006) and ks package for KDE (version 1.13.5; Duong, 2007).
2.7 Evaluating habitat selection
We contrasted macrohabitat selection and avoidance between gravid and non-gravid timber rattlesnakes using Manly selection ratios under a 3rd order Type II availability design (available habitat pooled across the population; Johnson, 1980; Manly et al., 2002). We considered forest, edge, and right-of-way (ROW) as the major macrohabitat types at the study site (Table 1) and evaluated habitat selection during the period through which females were gravid (June through August). Our use-sample included telemetry relocations from each individual assigned to the respective habitat types. A pooled availability sample was used because home ranges overlapped and lacked natural geographic barriers. The available habitat was defined as follows: 1) 100% MCPs were generated for each snake, 2) a buffer distance equal to the radius of the home range assuming a circular geometry was applied to each MCP (to include available resources immediately outside the observed home range), 3) the buffered MCPs were merged, and 4) clipped at the road because rattlesnakes showed evidence of road avoidance at the NVBP (a correlated random walk analysis on our telemetered snakes is provided in the Road Avoidance section of the Supplementary Materials; timber rattlesnakes have also demonstrated road avoidance in other studies, e.g., Andrews and Gibbons, 2005; Nordberg et al., 2021). Forest, edge, and ROW habitat availability was digitized in ArcMap version 10.4 (ESRI, 2016) using aerial imagery (ESRI imagery basemap) and LiDAR canopy data (2.5 m LiDAR collected and made available by the Ohio Geographically Referenced Information Program), where edge habitat was defined as areas within 15 meters of the forest-open canopy ecotone, and ROW habitat included all areas between the road and edge habitat, characterized by an open overstory and other human-made habitat features. Selection ratios were calculated for gravid and non-gravid snakes separately in R using the ‘widesII’ function in the adehabitatHS package (version 0.3.16; Calenge, 2006), with α = 0.008 to correct for multiple comparisons between reproductive classes and among the three habitat types. Selection ratios were interpreted as follows: wi > 1 indicated selection for habitat i, wi < 1 indicated avoidance of habitat i, and wi overlapping 1 suggested habitat i was used randomly with respect to its availability.
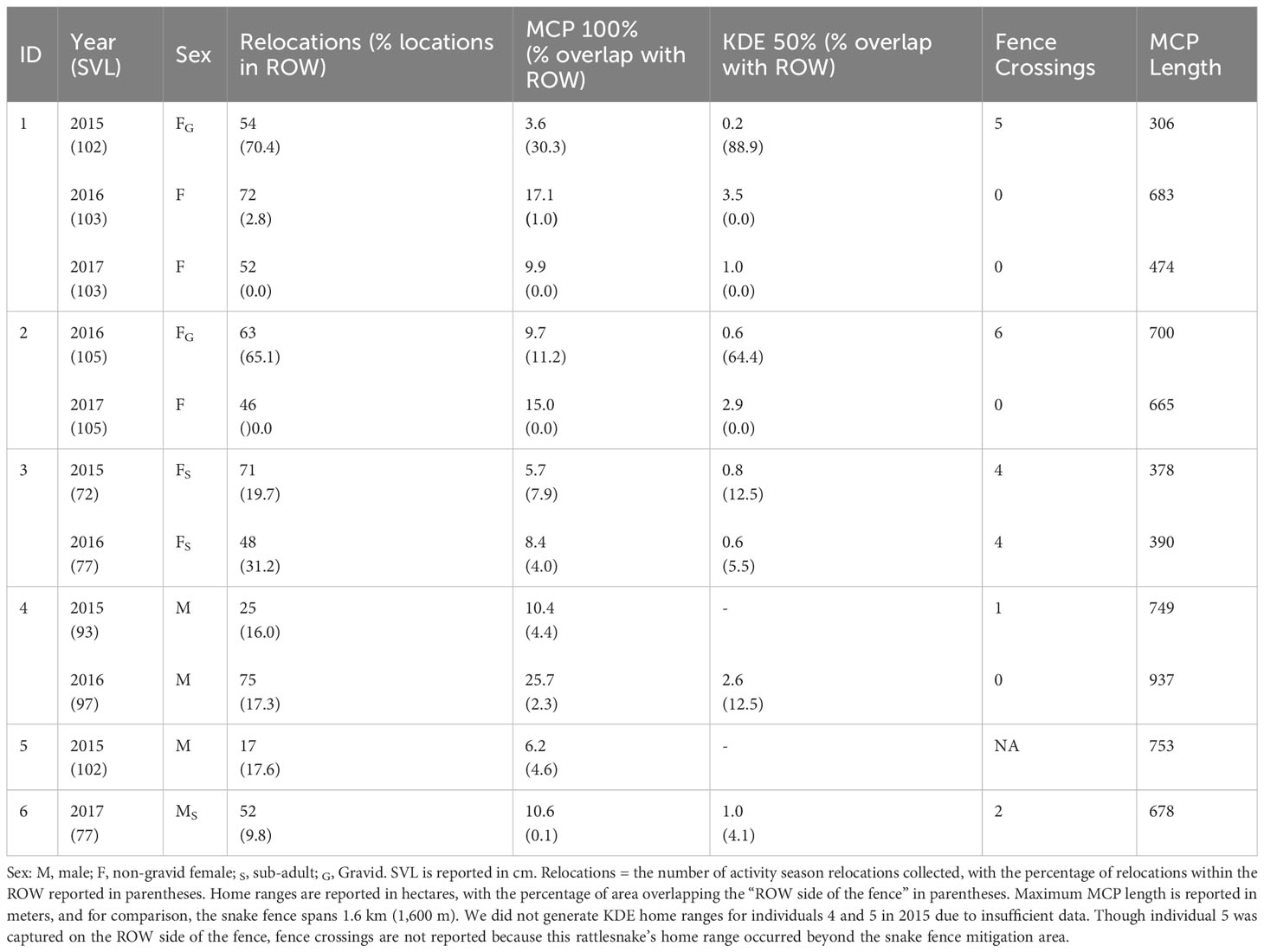
Table 1 Home range and fence crossing data observed from radio telemetry of timber rattlesnakes at the Nelsonville Bypass.
2.8 Thermal habitats and body temperature
We evaluated if thermal conditions influenced habitat selection at the NVBP by comparing habitat types in their availability of gestation and thermally restrictive temperatures. We used operative temperature models (OTMs) to quantify the spatiotemporal availability of ecologically relevant thermal resources across the landscape (Dzialowski, 2005). OTMs mimic an animal’s passive heat exchange and can therefore estimate the operative environmental temperature (Te), often defined as the equilibrium body temperature that an animal would achieve under prevailing environmental conditions in the absence of metabolic heating and evaporative cooling (Dzialowski, 2005). OTMs were constructed from 15.2 cm lengths of copper tubing (3.8 cm diameter, 0.15 cm thickness) and painted to approximate the reflectivity of timber rattlesnakes (e.g., Wills and Beaupre, 2000; Nordberg and Cobb, 2016; Nordberg and Cobb, 2017). Each OTM contained a Thermochron® iButton data loggers (DS1921G ± 0.5°C resolution) that recorded temperature every 20 minutes. OTM design specifications and validation procedures are provided in the Supplementary Materials. In 2016 field season, we distributed OTMs across forest, edge, and ROW macrohabitats (35-39 OTMs per habitat; 110 total) on the ground to quantify operative temperature availability. OTMs were distributed non-randomly at snake activity areas and paired with random walk sites. Activity areas were informed by rattlesnake locations observed through radio telemetry data during the 2015 and 2016 field seasons. Random walk locations were chosen by sampling random distances and bearings from each activity area, and were generated in R using uniform distributions, with distances being sampled from the interquartile range of movement step-lengths observed in telemetry data (10–70 m), and bearings being sampled from 1 to 360°. This model placement was intended to sample ecologically random variation used by, and available to, rattlesnakes at the study site.
Our radio transmitters were temperature sensitive and used to measure snake field active body temperatures (hereafter Tb; see Supplementary Materials). To estimate field preferred gestation Tb (Tg), we used a Lotek SRX 800® (Ottowa, Canada) remote telemetry receiver that monitored Tb in a free-ranging gravid female during the 2016 field season. Tb was recorded every 20 minutes and averaged for mean hourly Tb. We evaluated mean hourly Tb to determine whether average hourly Tb stabilized during photophase (daylight hours), suggesting a field preferred Tb. Due to the rugged terrain and range of our equipment, we were unable to monitor Tb of multiple snakes simultaneously using the remote receiver. To estimate voluntary thermal tolerances (VMAX; Camacho et al., 2018), we considered multiple thresholds: 1) the maximum Tb observed in any of the free ranging telemetered snakes, 2) the maximum Tb observed in a non-gravid snake while not performing an explicitly thermoregulatory behavior (e.g. post-surgery healing, shedding), and 3) values of VMAX reported in the literature (Brattstrom, 1965; Brown et al., 1982). We estimated Tb of all snakes each time they were tracked in the field by measuring the inter-pulse period (Supplementary Materials). In addition, we implanted a male rattlesnake with a Thermochron® iButton data logger (DS1921G ± 0.5°C resolution) that recorded Tb hourly throughout August and September 2016.
After identifying ecologically relevant temperatures (Tg and VMAX), we compared differences among habitats both statistically and graphically. Treating OTMs as the unit of replication, we quantified metrics of daily average thermal conditions for each habitat type including: average Te, standard deviation (within and among OTMs), maximum Te, minimum Te, hours within gestation Tb, hours above average gestation Tb, and hours above VMAX. We summarized these daily indices across habitat types using both the mean and median, and estimated within habitat variation (SD among OTMs). We tested for differences among habitats in the number of hours provided within gestation Tb and exceeding VMAX thresholds using generalized linear mixed effects models, specifying the Poisson distribution (GLMM; Pinheiro and Bates, 2000). Habitat type was modeled as a fixed effect, and OTM identity and ordinal date were modeled as crossed random effects to account variation among models and heteroscedasticity introduced by variation among days. We assessed whether thermal metrics were significantly different among habitats using Tukey post hoc tests with Bonferroni corrections, correcting α for the number of among habitat comparisons and thermal metrics tested. Statistical tests were performed in R version 4.1.2 (R Core Team, 2022) using the lme4 package for GLMMs (version 1.1-30; Bates et al., 2015) and multcomp package for multiple comparisons (version 1.4-20; Hothorn et al., 2008). We graphically evaluated mean hourly Te of each habitat type relative to the mean hourly gestation Tb (monitored gravid female) by plotting means with 95% confidence intervals, and across multiple spatiotemporal scales through heatmaps, area plots, and bar plots, assigning Te measurements to temperature classes relative to average gestation Tb bounded by standard deviations (SD) and VMAX (Supplementary Material Table 4).
2.9 Gestation habitat resource selection
We compared the spatial availability of gestation habitat within and outside the ROW by modeling a resource selection function (RSF) for gravid females under a use-available design (Manly et al., 2002). Models were fit using generalized linear models (GLM; McCullagh and Nelder, 1989) specifying the binomial distribution and the logit-link function. Use sites were selected as gravid female relocations throughout the summer months (June–August), but because rattlesnakes may remain at the same gestation site for days at a time, relocations were rarefied to include only unique locations. Sites where snakes spent extensive time are likely more important than locations where use was transient, and thus use-locations were weighted relative to the duration of time spent at each location, with the total weight summing to the number of unique locations. The extent of available habitat was defined using the same procedure as for the Manly selection ratios but included only home ranges of gravid snakes (3rd order Type II availability; Johnson, 1980; Manly et al., 2002). Within that extent, we randomly sampled 10,000 availability-locations in R using the SP package (version 1.5-0; Bivand et al., 2013); this sample size was chosen to saturate the available habitat and thus generate representative availability distributions (Northrup et al., 2013). The 10,000 availability points were weighted so that their collective value was equal to the number of use locations (n = 47) to avoid biasing the standard errors of parameter estimates (Barbet-Massin et al., 2012).
Our RSF covariates included: 1) two spatially explicit thermal landscapes; 2) canopy cover (derived from 2.5 m resolution LiDAR collected in 2014 by the Ohio Geographically Referenced Information Program); 3) Continuous Heat Insolation Load Index (CHILI, Theobald et al., 2015; calculated at 2.5 m resolution from a LiDAR derived DEM in Google Earth Engine, Gorelick et al., 2017); and 4) habitat edgeness (i.e., canopy heterogeneity, calculated by taking the standard deviation of a canopy cover raster using focal statistics). We chose these variables based on habitat preferences reported for timber rattlesnakes (Reinert, 1984) and other studies of reptiles in fragmented landscapes where snakes selected forest edges, ostensibly for their warmer temperatures (e.g., Blouin-Demers and Weatherhead, 2002; Waldron et al., 2006; Nordberg et al., 2021; but see also Wittenberg, 2012). Thermal resources are thought to be a fundamental driver of habitat selection in reptiles, and thus Te surfaces have the potential to model the thermal resource environment directly, whereas heat load, canopy cover, and habitat edgeness are all structural proxies (Huey, 1991; Reinert, 1993; Sears et al., 2011). We modeled thermal landscapes by predicting field collected Te data using spatial covariates (heat load indices and canopy cover) and weather station data using linear mixed effects models (Fridley, 2009; see Supplementary Materials).
Before multivariable models were developed, we evaluated each covariate in univariable models to identify the optimal scale and functional form (linear versus quadratic) using AICc, retaining the scale and functional form that resulted in the lowest AICc value for each covariate. To avoid multicollinearity, we evaluated correlation structure prior to building multivariable models, and evaluated variance inflation (VIF) for all of our multivariable models. We assessed VIF using the car package (version 3.1-0; Fox and Weisberg, 2019). We modelled thermal landscapes using canopy cover and heat load indices, and thus we avoided building RSF models that included both Te surfaces in combination with either canopy cover or heat load covariates, as these were highly collinear. The most complex multivariable models in the candidate set were Te + edgeness and canopy cover + heat load + edgeness. These models were compared with all subsets including univariable forms and a null model. The top model from the candidate set was identified using an information theoretic approach and evaluated based on AICc (Burnham and Anderson, 2002).
After developing the RSF, we evaluated the distribution of gestation resources at the NVBP to determine whether selected habitats were concentrated within the ROW. Across the range of predicted RSF values, we calculated the proportion of modelled habitat of equal or greater value that was contained within the ROW (relative to its availability). To perform these evaluations, the RSF output was rasterized as follows. If the top model was multivariable, we projected it by taking the exponential form of the logistic model and multiplying the model coefficients to their respective covariate raster layers (e.g., Johnson et al., 2006; McDonald, 2013). If the top model was univariable, we would use the raw raster (of the covariate) based on the range of values observed in the use-distribution.
3 Results
3.1 Captures and radio telemetry
Across three field seasons, we monitored box traps for 4,956 traps nights, made 2784 coverboard observations, and spent approximately 3,450 person hours in the field. This effort yielded 501 reptile captures (9 species of snakes, 1 species of turtle, and 1 species of lizard). We captured 18 timber rattlesnakes: four adults (2♂, 2♀), two juveniles (1♂, 1♀), and 12 neonates (5♂, 7♀). We telemetered the four adults and two juveniles and generated 575 relocations over three field seasons (167 relocations of four individuals in 2015, 258 relocations of four individuals in 2016, 150 relocations of three individuals in 2017).
3.2 Mitigation performance
Three of the adult rattlesnakes (2♂, 1♀) were initially captured within the ROW habitats including one male that was captured beyond the linear extent of the snake fencing (i.e., an area of the NVBP that lacked snake fencing). All six telemetered snakes traveled to ROW habitats at least once, and all five of the tracked snakes within the rattlesnake mitigation area crossed the snake fence (22 crossings; Table 1). Snakes apparently crossed through gaps in the snake fence where it was damaged, or traveled over the fence where overgrowth was present. The snake fence was damaged in multiple locations by erosion, corrosion, accumulating debris, tree falls, and overgrowth. Despite the snake fence being a permeable barrier, no telemetered rattlesnakes were killed or injured on the road. We did not observe telemetered rattlesnakes cross or attempt to cross the road directly or by way of the ecopassages. Snake mortality (n = 2; 1♀ subadult, 1 ♂ adult) was caused by predation within the forest based on their carcass locations and wounds. Other species of reptiles were observed dead on the road, including in areas with snake mitigation fencing (Supplementary Materials). While no reptiles were observed crossing the small wildlife crossings, mammals used these structures extensively (see Evaluating the Effectiveness of the Small Wildlife Ecopassages in the Supplementary Materials).
3.3 Space use
We generated 100% MCP home ranges and 50% KDE core activity areas for each individual per year (Table 1), resulting in 11 MCP home ranges from 6 individuals and 9 KDE core areas from 5 individuals. We excluded 2 individuals from KDE analysis in 2015 because they were captured late in the activity season, which would have biased identification of core activity areas. MCP home ranges for rattlesnakes ranged from 3.6–25.7 hectares and maximum home range lengths ranged from 306–937 meters (Table 1; Supplementary Figure 8). All rattlesnakes included both forest and ROW habitats in at least one year, yet all MCP home ranges were apparently bounded by the highway (Supplementary Figure 8). None of the maximum MCP lengths exceeded the length of the snake fence (1.6 km), but one adult female dispersed linearly beyond the extent of snake fencing, and one adult male was captured in the NVBP ROW beyond the snake fence mitigation area (Supplementary Figure 8). Due to incomplete tracking data, we generated KDE home ranges for two individuals in 2015, four individuals in 2016, and three individuals in 2017 (9 home ranges from 5 individuals; Table 1). Six of nine 50% KDE home ranges overlapped the ROW and both gravid rattlesnakes’ core home range overlapped the ROW by more than 50 percent (Table 1).
3.4 Habitat selection
Male and non-gravid female rattlesnakes spent most of their time within the forest habitats (68.8–100 percent of relocations on the forest side of the snake fence) while gravid females spent nearly all their time in edge and ROW habitats until giving birth (87.2 to 95.0 percent of relocations). These patterns were reflected in Manly selection ratios, which indicated that gravid females (87 relocations of 2 ♀) selected edge habitat, avoided forest habitat, and use of open canopy ROW habitat was not different from expected use based on availability (overall selection: χ2 = 274.8, df = 4, p < 0.001; Figure 1). Conversely, non-gravid rattlesnakes (273 relocations of 5 individuals; 2 ♂, 3 ♀) avoided ROW habitats, and use of both forest and edge habitats was not significantly different from expected use based on availability (overall selection: χ2 = 91.9, df = 10, p < 0.001; Figure 1).
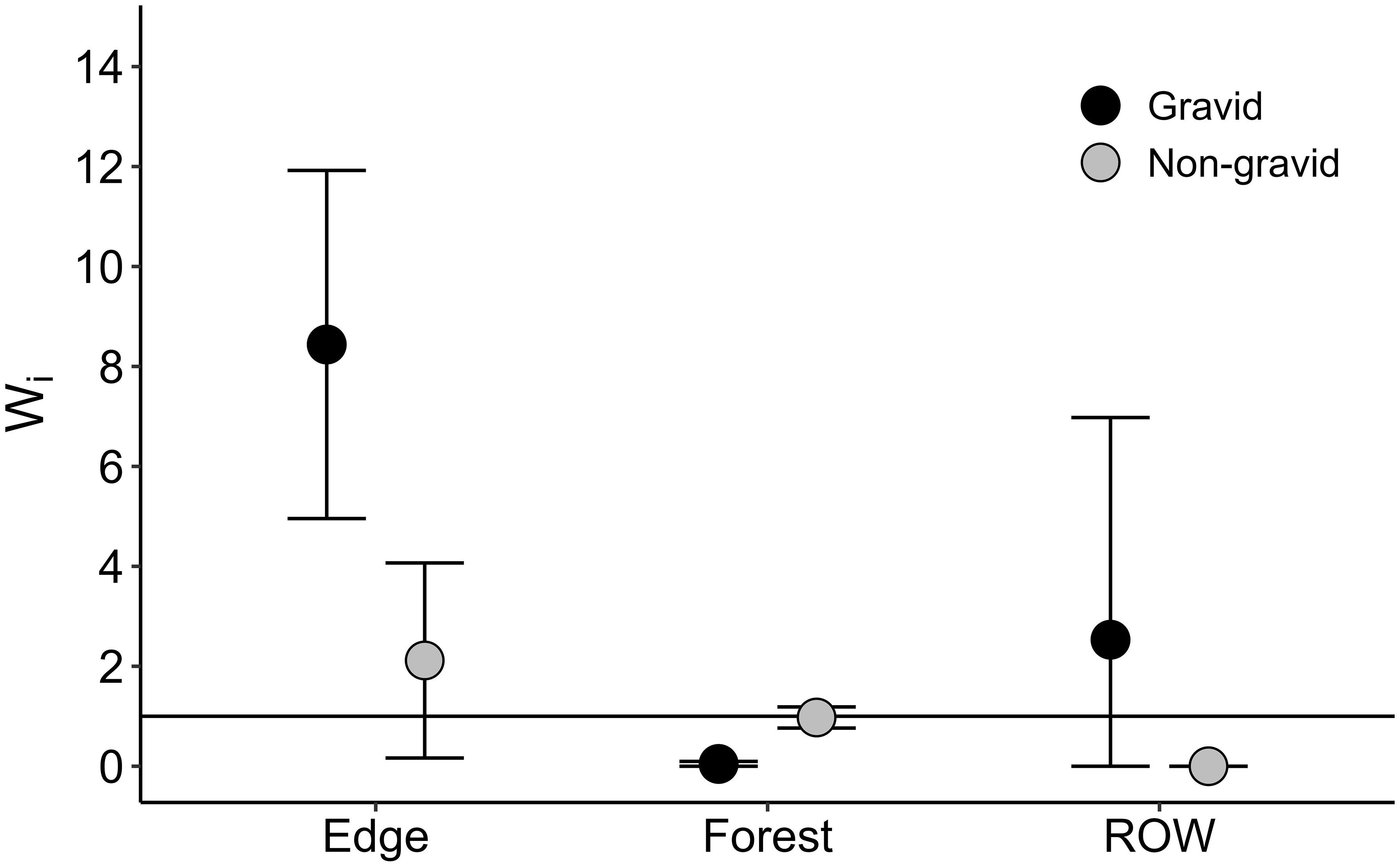
Figure 1 Macrohabitat selection for gravid and non-gravid timber rattlesnakes at the Nelsonville Bypass study site. Manly selection ratios were evaluated under a Type II availability design. α and 95% confidence intervals were adjusted for multiple comparisons. Wi are selection ratio coefficients, where Wi > 1 suggests positive selection for habitat i and Wi < 1 suggests avoidance of a habitat i. The horizontal line through 1 represents the expected usage of habitat i based on its availability (i.e., expected use), and thus confidence intervals overlapping 1 suggest that habitat usage was not statistically different from expected based on its availability.
3.5 Body temperature and thresholds
We recorded 2,754 field active body temperature measurements (hereafter Tb) from 5 rattlesnakes throughout the 2015, 2016, and 2017 activity seasons. We collected 881 hourly Tb measurements from a gravid female using the remote receiver during the warmest period of the summer (26 July to 17 August, 2016) and 1320 hourly Tb measurements from a male rattlesnake with an implanted iButton (2 August to 25 September, 2016). We collected an additional 553 Tb measurements during daylight hours from five radio-tagged rattlesnakes during the three activity seasons, with most observations occurring between May–September, 2015–2017. To compare hourly Tb profiles, we constrained the data to a common range of dates, 2–17 August, 2016. The monitored gravid female maintained a mean hourly Tb range between 26.7–30.4°C (overall mean Tb = 28.7°C, SD = 1.9, n = 626 with 13–35 measurements per hour), which varied considerably less than the range of mean hourly Te of each habitat which exhibited higher maximum temperatures in the afternoons and lower minimum temperatures in the early mornings (Figure 2A). In contrast, the male rattlesnake implanted with an iButton had a mean hourly Tb range between 22.2–28.6°C (overall mean Tb = 24.9°C, SD = 3.1, n = 384 with 16 measurements per hour), which generally conformed to Te observed in the forest with a time lag (Figure 2A).
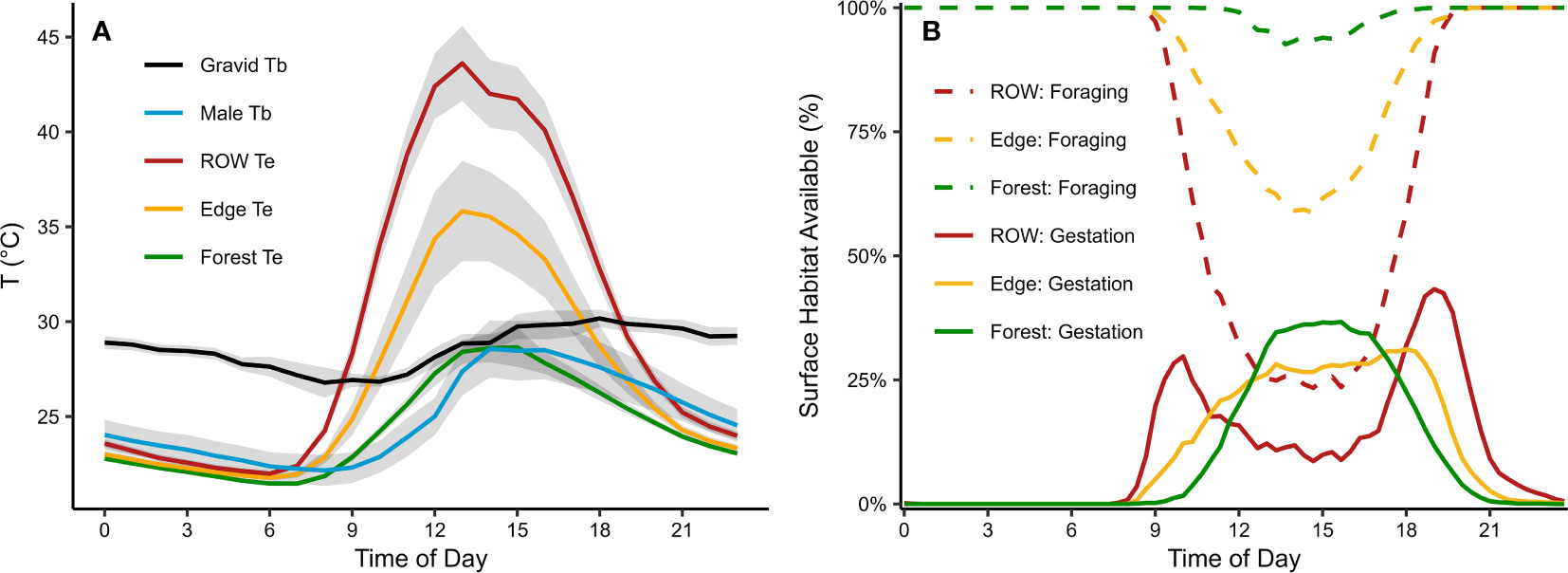
Figure 2 Mean operative temperature conditions and surface availability of suitable thermal habitats at the Nelsonville Bypass. (A) Mean operative temperatures (Te) within each macrohabitat along with the mean hourly body temperatures (Tb) from a gravid female and an adult male timber rattlesnake; gray ribbons are 95% confidence intervals. To allow for comparison between curves, Te and Tb data were summarized across a common range of dates during the warmest period of the summer (August 2, 2016 to August 17, 2016). (B) Surface availability of habitat areas at the Nelsonville Bypass based on suitable temperatures for gestation or foraging. Surface availability for gestation was defined as the percent of operative temperature models where Te was between 27.9 and 31.5°C. Surface availability of foraging habitat was defined as the percent of operative temperature models where Te ≤ 34.0°C.
For the gravid rattlesnake, Tb plateaued in the afternoon at about 30°C and mean hourly Tb ranged within 0.5°C from 1500–2100 hours and within 1°C from 1500–2300 hours (Figure 2A). For the period of 1500–2300 hours, mean Tb = 29.7 and SD = 1.8°C, and we thus considered 29.7 ± 1.8°C as the range of field preferred gestation temperatures (hereafter Tg). The maximum recorded Tb, 39.8°C, was a gravid female in 2015 and approaches the critical thermal maximum reported for many reptiles (Brattstrom, 1965). Among non-gravid snakes, the maximum observed Tb was 34.0°C, which approaches the VMAX reported for other crotalids (Brattstrom, 1965). Other timber rattlesnake studies have reported VMAX ranging from 31.5–37.4°C (Brattstrom, 1965; Brown et al., 1982; Wills and Beaupre, 2000). With little consensus among studies, we considered both VMAX values observed in our study for analyses (VMAX1 = 34.0°C, VMAX2 = 39.8°C), as behavioral thermal tolerances may differ with respect to sex or physiological conditions such as reproductive status.
3.6 Thermal resources
We recorded more than 460,000 Te readings across 70 summer days in 2016 (n = 110 OTMs, June 21–August 29). ROW and edge habitats provided warmer Te for longer durations of the day compared with forested habitats (Figures 2, 3). The number of hours Te occurred within Tg ±1 SD each day was not found to be statistically different among the habitats (Table 2). However, the number of hours that Te reached or exceeded Tg was significantly different among the habitats. Te reached or exceeded Tg for a mean of 7.8 hours per day in the ROW (median = 8.3), 4.2 hours per day in the edge (median = 4.3), and 1.4 hours per day in the forest (median = 0.3; P < 0.004 for all comparisons; Table 2). Fewer than 40 percent of the OTMs within the forest were able to reach Tg for one hour per day on average (median across days by OTM), though approximately 10 percent of forest OTMs were able to meet or exceed Tg for three or more hours per day (maximum = 4.7 hours). In contrast, all ROW OTMs reached or exceeded Tg for a minimum of 4.2 hours per day on average. At any point in the day, median Te (calculated across all OTMs within a habitat every 20 minutes, Figure 3A) reached or exceeded Tg ±1 SD on 41 of 70 days (58 percent) in the forest, 63 days (90 percent) in the edge, and 69 days (98 percent) in the ROW (Figure 3A). Afternoon Te in the ROW commonly exceeded voluntary thermal tolerances at the surface throughout the summer, while Te rarely reached those limits in the forest (Figures 2, 3). Te exceeded VMAX1 for a mean of 5.6 hours per day in the ROW (median = 6.3), 2.5 hours per day in the edge (median = 1.0), and 0.3 hours per day in the forest (median = 0.0; P < 0.004 for all comparisons of VMAX1 and VMAX2; Table 2). At the hottest hours of the day, only 23 and 39 percent of the ROW OTMs remained below the VMAX1 and VMAX2 thresholds, respectively, compared to 59 and 72 percent of OTMs in the edge habitat, and 93 and 97 percent of OTMs in the forest (Figures 2, 3). However, gravid rattlesnakes frequently used microhabitat structures within the ROW, especially riprap stone piles and subsurface rock crevices on roadcuts. These microhabitats provided buffered thermal regimes with refugia from extreme temperatures during the day and warmer Te through the night (Supplementary Figure 10).
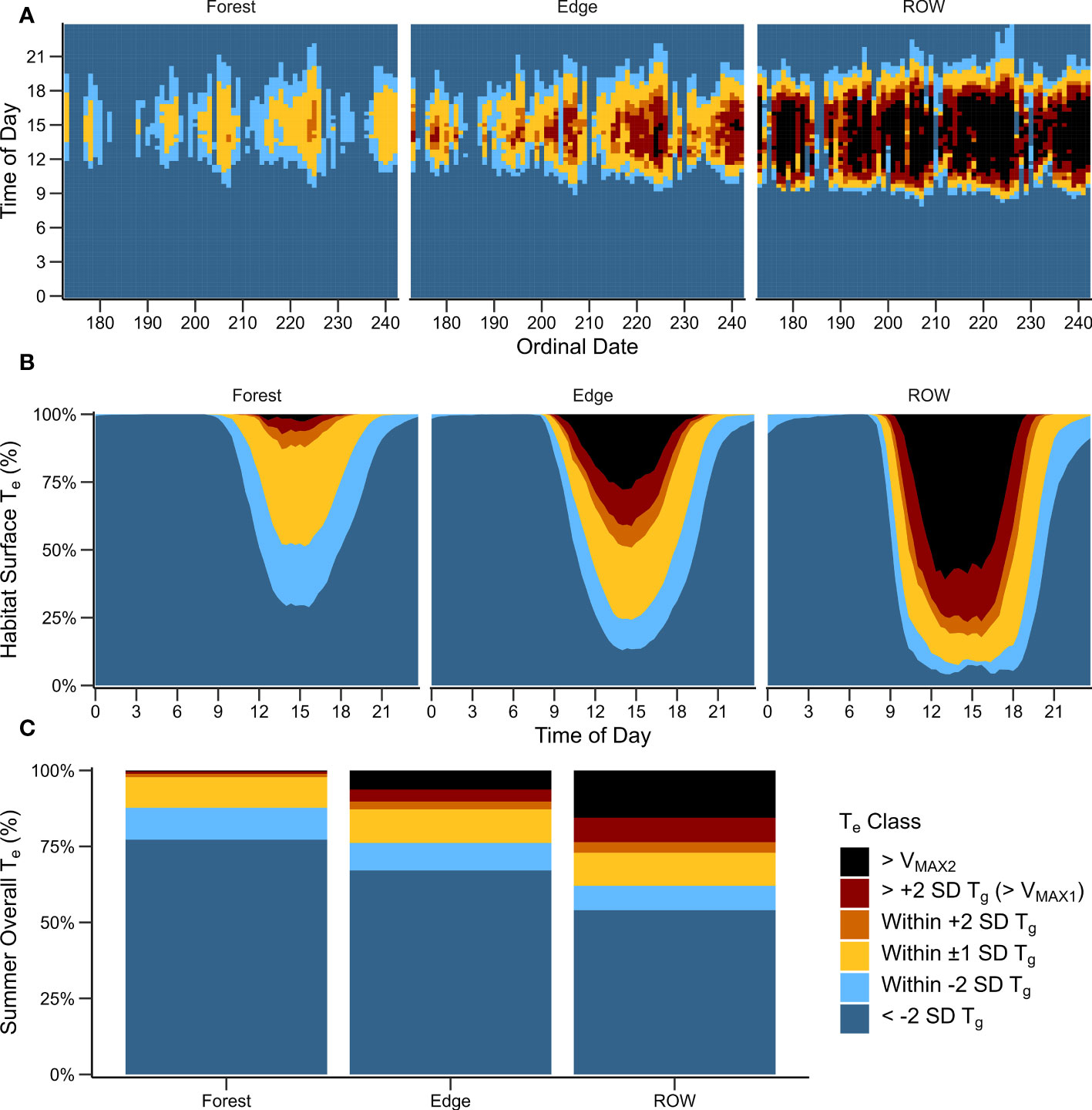
Figure 3 Spatiotemporal availability of thermal resources across habitats at the Nelsonville Bypass. Te classes are in relation to field preferred gestation Tb (29.7°C, SD = ± 1.8°C), and the observed voluntary maximum Tb (VMAX2 = 39.8°C). (A) Median Te class available within each habitat for 20-minute intervals from June 21–August 29, 2016. (B) Proportion of habitat (OTMs) available within each Te class across 20-minute intervals for each habitat across the summer. (C) Overall proportion of time each habitat experienced a given Te class across the summer. Te Classes are described in Supplementary Table 5.
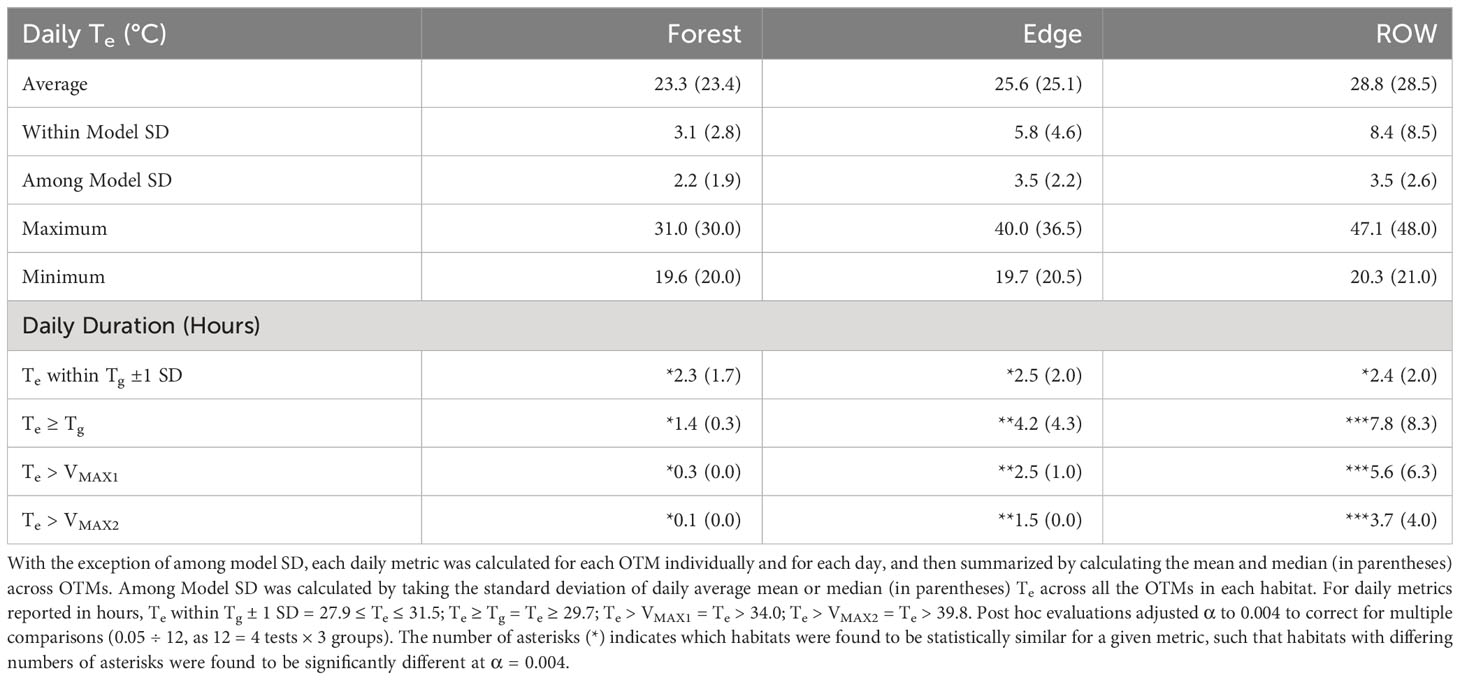
Table 2 Summary and comparative statistics for operative temperature data in three macrohabitats at the Nelsonville Bypass.
3.7 Gestation habitat resource selection
We collected 87 relocations from 2 gravid individuals during the 2015 and 2016 field seasons, which were rarefied to 47 unique use-locations. We generated eight candidate models (Table 3) for the RSF after optimizing scale and functional form of covariates and removing collinear variables (none of the models contained variables with VIF > 1.1). The top model identified by AICC was a univariable model that contained TeAVG, and identified that gravid snakes preferred locations on the landscape with higher TeAVG (odds ratio = 3.56, ß = 1.27, SE = 0.23, P < 0.001, McFadden’s Pseudo R2 = 0.57). Models were not averaged due to issues that would have arisen with multicollinearity (Cade, 2015), but also because alternative models were not competitive with the top model (ΔAICC ≥ 3.57). Gravid female use-availability plots suggested positive selection for locations where TeAVG > 25°C (Figure 4). Approximately 91 percent of the gravid female use locations occurred at locations where TeAVG ≥ 25°C despite these areas representing only 23 percent of the available habitat area (Figure 4). Within the available habitat extent (63.6 hectares), 94 percent of the ROW area (9.0 of 9.5 hectares) exhibited TeAVG ≥ 25°C, compared to only 9 percent of the forest (5.1 of 54.1 hectares), suggesting that thermal habitats preferred by gravid rattlesnakes were concentrated within the ROW.
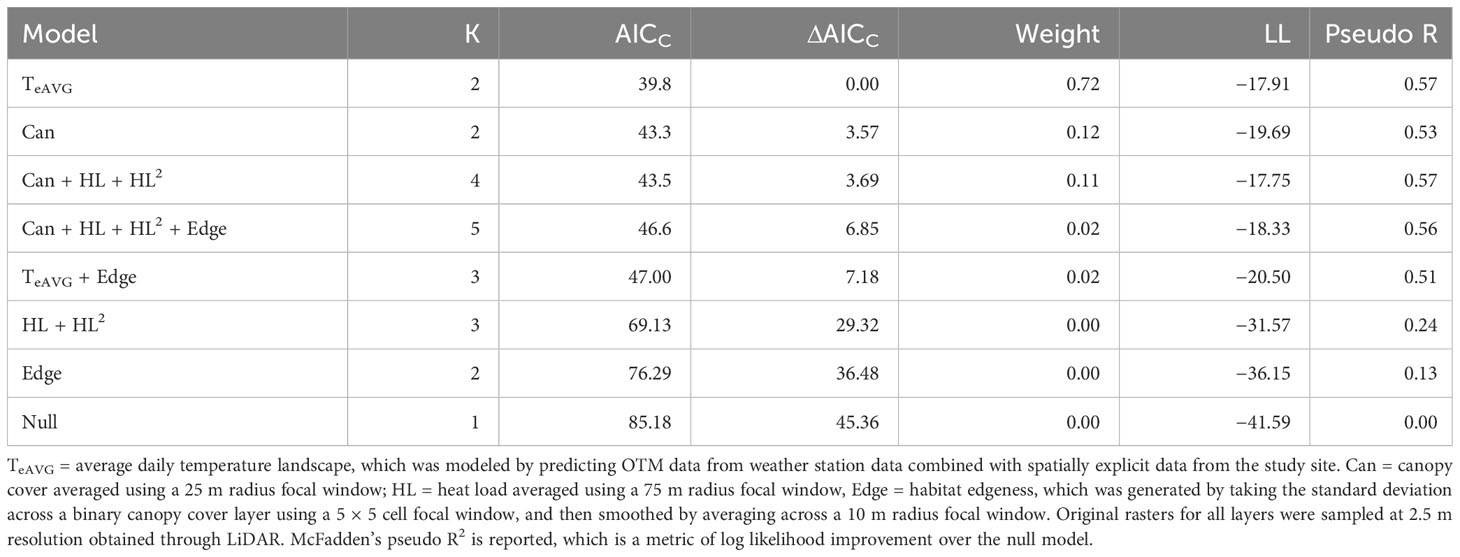
Table 3 Resource selection function (RSF) models evaluated for gravid female timber rattlesnakes at the Nelsonville Bypass.
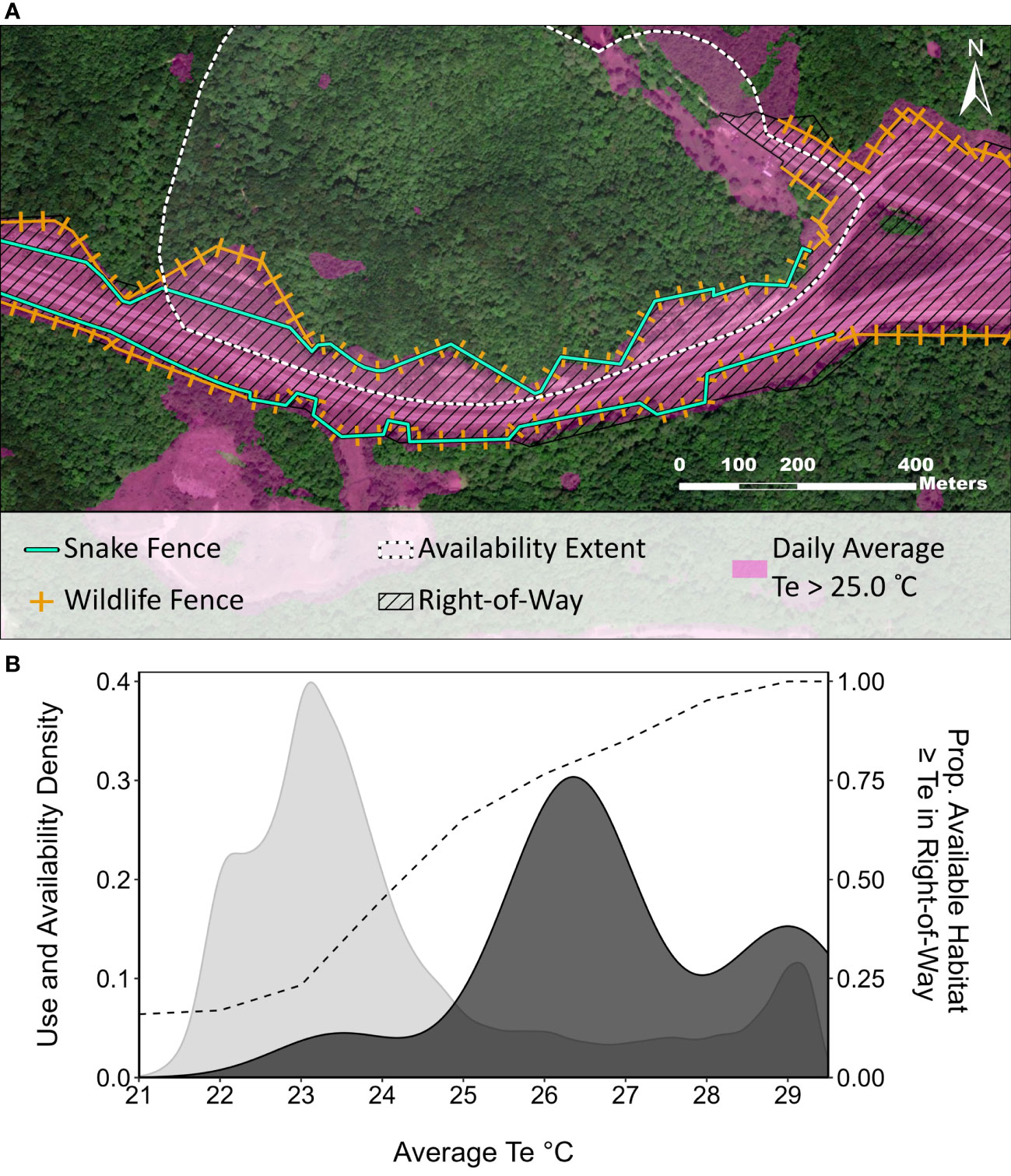
Figure 4 Thermal resources availability relative to the road corridor. (A) Map of the Nelsonville Bypass in the area available to gravid rattlesnakes, showing the location of exclusion fencing relative to modelled thermal habitats selected by gravid females (Average Te > 25°C). (B) Density plots of the use sample (dark gray) and availability distributions (light gray) for values on the Average Te thermal landscape, which was identified as the most important covariate in gravid rattlesnake resource selection functions. The dashed line shows the proportion of the available habitat area that meets or exceeds a given Te value and occurs within the Nelsonville Bypass ROW.
4 Discussion
We found that exclusion fencing at the NVBP failed to exclude rattlesnakes from the ROW, with rattlesnakes repeatedly crossing in and out of the ROW, likely through damaged sections of the exclusion fence. Similar to other road mitigation studies, these failures resulted from design specifications including improper materials, fence placement and extent, and lack of maintenance (Baxter-Gilbert et al., 2015; Huijser et al., 2016). For most of its length, the fencing at the NVBP was built at the edge of the ROW (as far as 100 meters away from the road in some locations), ostensibly to identify the ODOT-National Forest property boundary, where it sustained significant damage. Gaps were created beneath the fence by washouts on steep slopes, blowouts where soils and plant matter accumulated on eroding road cuts and forested hillsides, and corrosion in areas with acidic soils (Supplementary Figure 6). Rattlesnakes could have also passed over the fence by climbing overgrowth and tree falls that crushed the fence (Supplementary Figure 6). Nonetheless, nine rattlesnakes were captured in boxtraps or under coverboards along the snake fence indicating that the fence did divert movement in locations where it remained structurally intact. Unfortunately, annual maintenance did not prevent the fence from falling into disrepair, and damages along the fence went ignored in numerous places.
We did not observe evidence of rattlesnakes using the crossing structures to maintain population connectivity across the highway. Ultimately, we could not determine whether rattlesnakes or other reptiles did not use the crossing structures due to flawed mitigation design, unfavorable conditions within the crossings, the small population size (low probability of rattlesnakes encountering the crossing), or inadequate camera sensors. The damaged fencing and its poor interfacing with the crossing structures inhibited our ability to meaningfully evaluate whether the crossing structures could maintain rattlesnake population connectivity. Only one of five crossing structures at the NVBP, a SWC, was interfaced with the snake fence. In addition, the SWC that was interfaced with fence was properly interfaced with the fencing on only the northside of the NVBP, as the fencing ran above and behind the culvert entrance on the southside of the NVBP (Supplementary Figure 5). The snake fencing on the northside of the bypass, where rattlesnakes were tracked, was intact for approximately 100 meters on either side of culvert entrance. At least three of the rattlesnakes we tracked came within 50 meters of the crossing structure, and one individual was initially captured along the fence within five meters of the crossing structure. However, none of the rattlesnakes we tracked showed repeated usage of habitats within 100 meters of the crossing. Considering the gaps in the exclusion fence, the small population size, and the limited rattlesnake activity near the crossing structures, the NVBP rattlesnakes likely had few, if any, encounters with the crossing structures.
Other studies have shown difficulty detecting reptiles and amphibians using Passive IR cameras, and it is possible that we too failed to detect successful crossings that could have occurred (Hobbs and Brehme, 2017; Pomezanski and Bennett, 2018). However, we find it unlikely that we missed timber rattlesnake crossings given that we detected multiple species of reptile much smaller in size at the entrances of these crossings. We find it more likely that rattlesnakes did not use the structures due to the fencing and interfacing design flaws identified above. In addition, other rattlesnake studies have observed reluctance to cross through structures similar to those built at the NVBP (Colley et al., 2017; Laidig and Golden, 2004). The conditions throughout the SWCs were poorly lit and score low by metrics of openness (i.e., low width:length ratio; Yanes et al., 1995). While the NVBP crossing structures were used extensively by mammals, rattlesnakes and other reptiles were not observed crossing the structures. Rattlesnakes and other reptiles may have been deterred from crossing by chemosensory signs of predatory mammals that frequented the structures. Raccoons (Procyon lotor) and Virginian opossums (Didelphis virginiana) were frequently observed in the crossings and are known snake predators (Supplementary Materials).
The use of ROW and edge habitats, overlap of core home ranges with the ROW (Table 1), and neutral to positive selection for those human-made habitats by gravid females (Figure 1) all suggested that the NVBP ROW provided important habitat for the rattlesnakes. Using operative temperature modelling, we observed warmer thermal regimes available in the edge and ROW habitats (Figures 2, 3) and found that landscape temperatures selected by gravid females were concentrated within the habitats created by road construction (Figure 4). Daytime Te in the ROW often exceeded Tg, but the warmer Te profiles of the ROW extended the temporal availability of preferred thermal habitat for gravid females relative to the forest. While mean hourly forest Te approached Tg ±1 SD during midday hours, edge and ROW habitats would often reach and exceed Tg for four to eight hours of the day (Figure 2; Table 2) with the ROW showing peaks in Tg availability in the morning and evening hours (Figure 2B). Within the ROW, gestation sites used by gravid females were exposed to continuous full sun during the day, but were located at deep rock crevices (holes and fissures emerging from the roadcuts) or stone piles (drainage control riprap). These microhabitats provided thermal refugia that allowed snakes to escape extreme temperatures during the afternoon and maintain elevated Tb through the night as Te dropped above-ground (Supplementary Figure 10). These microhabitat features within the ROW provided a broad thermal gradient that facilitated precise thermoregulation throughout gestation (Figure 2; Supplementary Figure 10). The combination of open canopy, south facing slopes, diverse microhabitats, and rocky retreats within the ROW apparently created preferred gestation sites where rattlesnakes could maintain elevated body temperatures throughout the day and night.
With afternoon temperatures routinely exceeding VMAX in the ROW (Figures 2, 3), much of this area would have been unsuitable for sit-and-wait ambush foraging which was reflected in the avoidance of ROW habitats by non-gravid snakes (Figure 1). Female rattlesnakes that made use of the ROW through gestation did not return to the ROW after giving birth in the same activity season, and did not return to ROW in their post-partum activity season except during ecdysis. Non-gravid snakes crossed the exclusion fence infrequently, but when they did, they spent most of their time in edge habitats at the fringe of the ROW while exhibiting behaviors linked to thermoregulation such as shedding or healing from injuries (e.g., transmitter replacement). The two adult males we tracked both traveled to the ROW or edge habitat during ecdysis until shedding. Thus, both gravid and non-gravid rattlesnakes were apparently crossing the snake fence to access warmer habitats available at the forest edge and within the ROW. The landscape matrix surrounding the NVBP is primarily forested, and we speculate that basking and gestation sites are more limited than foraging habitat. For this reason, the benefits of the novel thermal habitats created by the ROW may outweigh the costs of lost foraging habitat. However, because the mitigation structures do not exclude rattlesnakes from the roadway nor maintain connectivity, eventual road mortality and genetic drift may detrimentally affect the rattlesnake population over time (Rudolph et al., 1999; Clark et al., 2010; Bushar et al., 2015).
Despite detecting a significant number of Eastern Box Turtle (Terrapene carolina) road mortalities at the NVBP (Supplementary Materials), we did not observe rattlesnake road mortality via either radio telemetry or road mortality surveys. Our vehicle speeds were not ideal for rigorous monitoring of road mortality (minimum speeds were required by ODOT to avoid creating hazardous traffic conditions), which may have limited our ability to detect small reptile carcasses. However, carcasses of turtles and large snakes were easily visible on the road because the surface of the highway was still relatively new, providing visual contrast (Supplementary Figure 7). Correlated random walk simulations indicated that rattlesnake movements were consistent with road avoidance (Supplementary Materials), which echoes numerous studies where timber rattlesnakes have demonstrated an aversion to crossing roads based on experimental trials (Andrews and Gibbons, 2005) and telemetry data collected in road fragmented landscapes (Nordberg et al., 2021; Tipton et al., 2023). Road avoidance in other species has been shown to scale with the road size and traffic intensity (Brehme et al., 2013), and the NVBP is a four-lane divided highway, with high traffic volumes (exceeding 17,000 vehicles per day) traveling at high speeds (112 km/hr). If rattlesnakes did attempt to cross the NVBP, the probability of road mortality would be high because timber rattlesnakes cross roads slowly and often pause for oncoming traffic (Andrews and Gibbons, 2005). The small rattlesnake population at the NVBP and the long generation times of timber rattlesnakes suggest that additive road mortality could cause rapid population decline or extirpation.
Our study site was selected based on the location of the mitigated highway, which was co-located with a small and endangered population of rattlesnakes. Working with this small population severely limited our sample size of rattlesnakes. While small sample sizes require caution, our study does not claim any novel discoveries regarding timber rattlesnake spatial ecology, habitat selection, or thermal biology. Our observations are consistent with the ecology of the species established by decades of past field research (Reinert, 1984; Reinert et al., 1984; Reinert and Zappalorti, 1998; Waldron et al., 2006; Gardner-Santana and Beaupre, 2009; Nordberg et al., 2021; Tipton et al., 2023), including our observations of field active body temperatures and roadside habitat use (Reinert and Zappalorti, 1998; Gardner-Santana and Beaupre, 2009). Yet, our study is valuable to the road ecology conversation because it is among few that have examined the intersection of spatial, thermal, and road ecology (e.g., Peaden et al., 2017). Specifically, our spatially explicit characterization of thermal habitat resources allowed us to examine how the distribution of those resources likely influenced movement and habitat selection with respect to roadside habitats and mitigation fencing. The structural design failings of the snake exclusion fence, use of ROW habitats by rattlesnakes, the quantification and comparison of thermal habitat resources, and the physical properties that likely attracted rattlesnakes to the ROW are all observations that may be applicable to timber rattlesnake population management and road mitigation planning in general.
4.1 Roads and thermal habitat resources
At the NVBP, road construction introduced resource heterogeneity to the landscape that ostensibly motivated fence crossings by attracting rattlesnakes to the forest edge and ROW. Our results are echoed in many other studies that have observed use of, or preference for, roadsides, edge habitats, and other ROWs (Langen et al., 2015). Use of, or preference for, human-made ROWs has been reported for many species of reptiles across broad geographies including snakes and lizards in North America (Elaphe obsoleta, Blouin-Demers and Weatherhead, 2002), Europe (Zamenis longissimus, Kovar et al., 2014; Chameleo chameleon, Hódar et al., 2000), South America (Ameiva ameiva, Vitt et al., 1998; Sartorius et al., 1999), and Australia (Egernia major, Klingenböck et al., 2000; Bassiana duperreyi, Shine et al., 2002). Another commonality among these studies was that reptiles were apparently drawn to these modified habitats to access thermal resources, often at the expense of increased mortality risk, and sometimes with population level consequences. For example, it has been reported widely that open roadside habitats often attract female turtles for nesting (Haxton, 2000), which can result in ecological trap formation and female biased mortality (Aresco, 2005; Steen et al., 2006).
At the NVBP, the ROW provided a large corridor of open canopy and rocky microhabitats within a closed canopy forest matrix that was used by rattlesnakes and other reptiles. Structurally, these landscape changes were analogous to habitat improvement techniques used for reptiles in thermally limited environments. For example, at local scales, forest canopy thinning was an effective restoration technique to improve habitat quality and reptile species diversity at sites in Australia and North America (Webb et al., 2005; Pike et al., 2011; Hromada et al., 2018). Similarly, many thermophilic reptiles use microhabitats characterized by rock outcroppings for their physical and thermal properties (Reinert, 1984; Huey et al., 1989; Croak et al., 2008). These species can be affected by the removal or shading of rocky habitats (Shine et al., 1998; Pike et al., 2011), and benefit from their restoration and sunning (Croak et al., 2010; Pike et al., 2011). Multiple studies have reported negative effects of forest succession and canopy closure on temperate reptile populations and communities, which resulted in the declines and extirpations of some snake and lizard populations (Hall, 1994; Ballinger and Watts, 1995; Jäggi and Baur, 1999; Fitch, 2006).
Simulations and experiments predict that ectotherms can thermoregulate more effectively and efficiently in landscapes with small and dispersed patches of thermal resources as opposed to landscapes with fewer but larger patches of thermal resource (Sears et al., 2016). Yet, our study and others have shown that large disturbances can create thermal habitats that are otherwise uncommon or absent in small canopy gaps (Vitt et al., 1998; Sartorius et al., 1999). Specifically, large canopy openings and broad east-west clearings allow for extended hours of direct solar radiation for basking. Analogous human-made ROWs come in a variety of forms aside from road corridors, such as electric, gas, and other utility corridors. These linear features can have similar ecological properties given that they are also maintained in an open canopy or early successional state. As observed in our study and others, the thermal habitats created by these ROWs are sometimes sought by reptiles (e.g., Vitt et al., 1998; Sartorius et al., 1999), which may be of consequence to population vital rates given the range of behaviors and physiological processes governed by thermal biology (Angilleta, 2009).
We acknowledge that most roadsides exist as bare or mowed grass features and lack the diverse microhabitat features we observed at the NVBP. Roadsides often exist as degraded habitats that favor weedy species, introduce edge-effects harmful to forest interior species, and can create ecological traps (Chalfoun et al., 2002; McKinney, 2006; Langen et al., 2015). However, there are instances where ROWs contain suitable microhabitats such as road cuts, stone piles, and downed logs, which may have value to reptile populations if there is a deficit of basking habitat resources in the surrounding landscape. We hypothesize that ROWs are more likely to attract reptiles where they create a stark resource gradient with the surrounding landscape, for example, in wide ROWs created in forested landscapes, where reptiles (or other ectotherms) have limited basking sites. Conversely, narrow ROWs and landscapes where the creation of a ROW does not create a stark resource gradient, as in arid, treeless landscapes and urban environments, would be less likely to attract reptiles. These novel resource gradients may be most consequential in landscapes where past timber practices or fire suppression have resulted in more homogenous forest conditions with closed canopy structure. Again, we consider these cases to be exceptions to the rule, and that the value of such ROWs to wildlife is contingent on the presence of suitable habitats contained within the corridor and the absence of ecological traps.
4.2 Management recommendations and conclusions
Our observations of flawed mitigation design and rattlesnake behavior in roadside habitats resulted in two site-specific recommendations at the NVBP. First, building an exclusion fence 5 to 10 meters from the road, along the length of the bypass, and interfaced with crossing structures (e.g., Langton and Clevenger, 2021) would better protect the NVBP timber rattlesnake population, eliminate the most common sources of structural damage, and facilitate routine maintenance of the fence. We reiterate that the continued effectiveness of any mitigation fencing requires regularly scheduled maintenance and upkeep that should be planned for in design and budgeting. Second, because the thermally exposed habitat features selected by gravid rattlesnakes will continue to undergo succession, the population would also benefit from the restoration and maintenance of basking sites (canopy gaps with stone piles, rock crevices, or large hollowed logs as refugia) within the surrounding forest on the habitat-side of the fence.
We do not advocate the construction of roads through landscapes as a form of habitat enhancement because wildlife populations are often adversely affected by roads (see Introduction). However, when roads and other ROWs are to be built through wildlife habitat, it is worth considering the landscape context and affected resource environments (e.g., thermal, forage, or shelter resources), how wildlife may respond to novel resources, and whether mortality or other adverse effects are likely to increase during use of, or movement to and from, those resources (i.e., ecological trap formation). These same considerations are also worth visiting when designing wildlife fencing (Jakes et al., 2018). Habitat heterogeneity, particularly where stark resource gradients are formed between the ROW and the surrounding landscape, has the potential to introduce strong resource selection pressures. In cases where such resource gradients are present, fence placement may be consequential to species attracted to those resources, and particularly when ROW resources are associated with the reproductive success of a given species. When roads introduce open canopy habitats and rocky features to forested landscapes, we recommend evaluating the potential value of these habitat features to local reptile populations, whether those features can be maintained on the habitat-side of exclusion fencing, and whether basking habitats could be restored away from the road to lessen the selection pressure for roadsides habitats and reduce the probability of ecological trap formation. Understanding whether these resource selection pressures are likely to form may help design more effective road mitigation for reptile populations.
Data availability statement
The raw data supporting the conclusions of this article will be made available by the authors, without undue reservation.
Ethics statement
The study was conducted in accordance with the local legislation and institutional requirements. The animal study was approved by Ohio University Institutional Animal Care and Use Committee (approval issued to WR under protocols 14-L-018 and 13-L-023). The study was also conducted under the Scientific Collection Permit #18-84 issued by the Ohio Department of Natural Resources.
Author contributions
WR and GS conceived the study and wrote the grants. GS and WR developed the study design. GS conducted the field work and analyzed the data. GS wrote the manuscript with input from WR. The findings and conclusions in this article are those of the authors and do not necessarily represent the views of the U.S. Fish and Wildlife Service. All authors contributed to the article and approved the submitted version.
Funding
The study was funded by the Ohio Department of Transportation under ODOT Agreement 26656 CFDA 20.205 and ODOT Agreement 27695.
Acknowledgments
We would like to thank Matt Perlik, Mike Austin and the Ohio Department of Transportation, Lynda Andrews and the Wayne National Forest, and Ohio Division of Wildlife for their support of this project. We thank Viorel Popescu for substantial analytical feedback, as well as Shawn Kuchta and Joseph Johnson for their comments on earlier versions of this manuscript. We thank Seth Cones, Isabel Fisk Baruque, Christine Hanson, Kelly Johnson, Adam Kabrick, Tyler Stewart, and Aspen Wilson for their dedicated assistance in the field. Finally, we would like to thank the reviewers and editor for their feedback which greatly improved the manuscript.
Conflict of interest
The authors declare that the research was conducted in the absence of any commercial or financial relationships that could be construed as a potential conflict of interest.
Publisher’s note
All claims expressed in this article are solely those of the authors and do not necessarily represent those of their affiliated organizations, or those of the publisher, the editors and the reviewers. Any product that may be evaluated in this article, or claim that may be made by its manufacturer, is not guaranteed or endorsed by the publisher.
Supplementary material
The Supplementary Material for this article can be found online at: https://www.frontiersin.org/articles/10.3389/fevo.2023.1059461/full#supplementary-material
References
Abrahms B., Jordan N. R., Golabek K. A., McNutt J. W., Wilson A. M., Brashares J. S. (2016). Lessons from integrating behaviour and resource selection: activity-specific responses of African wild dogs to roads: Integrating behaviour and resource selection. Anim. Conserv. 19, 247–255. doi: 10.1111/acv.12235
Aldridge R. D., Brown W. S. (1995). Male Reproductive Cycle, Age at Maturity, and Cost of Reproduction in the Timber Rattlesnake (Crotalus horridus). J. Herpetol. 29, 399. doi: 10.2307/1564990
Anderson C. D. (2010). Effects of Movement and Mating Patterns on Gene Flow among Overwintering Hibernacula of the Timber Rattlesnake (Crotalus horridus). Copeia 2010, 54–61. doi: 10.1643/CH-08-121
Andrews K. M., Gibbons J. W. (2005). How do highways influence snake movement? Behavioral responses to roads and vehicles. Copeia 2005, 772–782. doi: 10.1643/0045-8511(2005)005[0772:HDHISM]2.0.CO;2
Andrews K. M., Gibbons J. W., Jochimsen D. M. (2008). “Ecological effects of roads on amphibians and reptiles: A literature review,” in Urban Herpetology. Eds. Mitchell J. C., Brown R.E.J., Bartholomew B. (Salt Lake City, Utah: Society for the Study of Amphibians & Reptiles), 121–143.
Angilleta J. M.J. (2009). Thermal Adaptation: A Theoretical and Empirical Synthesis (Oxford, New York: Oxford University Press).
Aresco M. J. (2005). The effect of sex-specific terrestrial movements and roads on the sex ratio of freshwater turtles. Biol. Conserv. 123, 37–44. doi: 10.1016/j.biocon.2004.10.006
Ballinger R. E., Watts K. S. (1995). Path to extinction: impact of vegetational change on lizard populations on arapaho prairie in the Nebraska Sandhills. Am. Midland Nat. 134, 413–417. doi: 10.2307/2426313
Barbet-Massin M., Jiguet F., Albert C. H., Thuiller W. (2012). Selecting pseudo-absences for species distribution models: how, where and how many? Methods Ecol. Evol. 3, 327–338. doi: 10.1111/j.2041-210X.2011.00172.x
Bates D., Maechler M., Bolker B., Walker S. (2015). Fitting linear mixed-effects models using lme4. J. Stat. Software 67, 1–48. doi: 10.18637/jss.v067.i01
Baxter-Gilbert J. H., Riley J. L., Lesbarreres D., Litzgus J. D. (2015). Mitigating reptile road mortality: Fence failures compromise ecopassage effectiveness. PloS One 10, 1–15. doi: 10.1371/journal.pone.0120537
Berger J. (2007). Fear, human shields and the redistribution of prey and predators in protected areas. Biol. Lett. 3, 620–623. doi: 10.1098/rsbl.2007.0415
Bivand R. S., Pebesma E. J., Gómez-Rubio V. (2013). Applied spatial data analysis with R. 2nd ed (New York, NY: Springer).
Blouin-Demers G., Weatherhead P. J. (2001). Thermal ecology of black rat snakes (Elaphe obsoleta) in a thermally challenging environment. Ecology 82, 3025–3043. doi: 10.1890/0012-9658(2001)082[3025:TEOBRS]2.0.CO;2
Blouin-Demers G., Weatherhead P. J. (2002). Habitat-specific behavioural thermoregulation by black rat snakes (Elaphe obsoleta obsoleta). Oikos 97, 59–68. doi: 10.1034/j.1600-0706.2002.970106.x
Boyle S. P., Keevil M. G., Litzgus J. D., Tyerman D., Lesbarrères D. (2021). Road-effect mitigation promotes connectivity and reduces mortality at the population-level. Biol. Conserv. 261, 109230. doi: 10.1016/j.biocon.2021.109230
Brattstrom B. H. (1965). Body temperatures of reptiles. Am. Midland Nat. 73, 376–422. doi: 10.2307/2423461
Brehme C. S., Tracey J. A., McClenaghan L. R., Fisher R. N. (2013). Permeability of roads to movement of scrubland lizards and small mammals. Conserv. Biol. 27, 710–720. doi: 10.1111/cobi.12081
Brown W. S. (1991). Female reproductive ecology in a northern population of the timber rattlesnake, Crotalus horridus. Herpetologica 47, 101–115. Available at: http://www.jstor.org/stable/3892821.
Brown W. S. (1993). Biology, Status, and Management of the Timber Rattlesnake (Crotalus horridus): a Guide for Conservation (St. Louis MO: SSAR Herpetological Circular No. 22), 78pp.
Brown W., Pyle D., Greene K., Friedlaender J. (1982). Movements and temperature relationships of timber rattlesnakes (Crotalus horridus) in Northeastern New York. J. Herpetol. 16, 151–161. doi: 10.2307/1563808
Burgdorf S., Rudolph D., Conner R., Saenz D., Schaefer R. (2005). A successful trap design for capturing large terrestrial snakes. Herpetol. Rev. 36, 421–424.
Burnham K. P., Anderson D. R. (2002). Model Selection and Multimodel Inference: A Practical Information-Theoretic Approach (New York: Springer-Verlag).
Bushar L. M., Bhatt N., Dunlop M. C., Schocklin C., Malloy M. A., Reinert H. K. (2015). Population isolation and genetic subdivision of timber rattlesnakes (Crotalus horridus) in the New Jersey pine barrens. Herpetologica 71, 203–211. doi: 10.1655/HERPETOLOGICA-D-14-00030
Cade B. S. (2015). Model averaging and muddled multimodel inferences. Ecology 96, 2370–2382. doi: 10.1890/14-1639.1
Calenge C. (2006). The package “adehabitat” for the R software: A tool for the analysis of space and habitat use by animals. Ecol. Model. 197, 516–519. doi: 10.1016/j.ecolmodel.2006.03.017
Camacho A., Rusch T., Ray G., Telemeco R. S., Rodrigues M. T., Angilletta M. J. (2018). Measuring behavioral thermal tolerance to address hot topics in ecology, evolution, and conservation. J. Thermal Biol. 73, 71–79. doi: 10.1016/j.jtherbio.2018.01.009
Chalfoun A. D., Thompson F. R. III, Ratnaswamy M. J. (2002). Nest predators and fragmentation: a review and meta analysis. Conserv. Biol. 16, 306–318. doi: 10.1046/j.1523-1739.2002.00308.x
Clark R. W., Brown W. S., Stechert R., Zamudio K. R. (2010). Roads, interrupted dispersal, and genetic diversity in timber rattlesnakes. Conserv. biol.: J. Soc. Conserv. Biol. 24, 1059–1069. doi: 10.1111/j.1523-1739.2009.01439.x
Clevenger A. P., Chruszcz B., Gunson K. E. (2001). Highway mitigation fencing reduces wildlife-vehicle collisions. Wildlife Soc. Bull. 29, 646–653. Available at: https://www.jstor.org/stable/3784191
Clevenger A. P., Huijser M. (2011). Wildlife Crossing Structure Handbook Design and Evaluation in North America (Washington, D.C: Federal Highway Administration).
Colley M., Lougheed S. C., Otterbein K., Litzgus J. D. (2017). Mitigation reduces road mortality of a threatened rattlesnake. Wildlife Res. 44, 48–59. doi: 10.1071/WR16130
Croak B. M., Pike D. A., Webb J. K., Shine R. (2008). Three-dimensional crevice structure affects retreat site selection by reptiles. Anim. Behav. 76, 1875–1884. doi: 10.1016/j.anbehav.2008.08.011
Croak B. M., Pike D. A., Webb J. K., Shine R. (2010). Using artificial rocks to restore nonrenewable shelter sites in human-degraded systems: Colonization by fauna. Restor. Ecol. 18, 428–438. doi: 10.1111/j.1526-100X.2008.00476.x
Dodd C. K., Barichivich W. J., Smith L. L. (2004). Effectiveness of a barrier wall and culverts in reducing wildlife mortality on a heavily traveled highway in Florida. Biol. Conserv. 118, 619–631. doi: 10.1016/j.biocon.2003.10.011
Duong T. (2007). ks: Kernel density estimation and kernel discriminant analysis for multivariate data in R. J. Of Stat. Software 21, 1–16. doi: 10.1139/F2011-179
Dzialowski E. M. (2005). Use of operative temperature and standard operative temperature models in thermal biology. J. Thermal Biol. 30, 317–334. doi: 10.1016/j.jtherbio.2005.01.005
Ernst C. H., Ernst E. M. (2003). Snakes of the United States and Canada (Washington, D.C: Smithsonian Press).
ESRI (2016). ArcGIS Desktop. Version 10.4 (Redlands, CA: Redlands, California, USA, Environmental Systems Research Institute).
Fitch H. S. (2006). Ecological succession on a natural area in Northeastern Kansas from 1948 to 2006. Herpetol. Conserv. Biol. 1, 1–5. Available at: https://www.herpconbio.org/volume_1/issue_1/Fitch_2006.pdf
Forman R. T. T., Alexander L. E. (1998). Roads and their major ecological effects. Annu. Rev. Ecol. Systematics 29, 207–231. doi: 10.1146/annurev.ecolsys.29.1.207
Forman R. T. T., Sperling D., Bissonette J. a, Clevenger a P., Cutshall C. D., Dale V. H., et al. (2003). Road ecology: science and solutions (Washington D.C: Island Press).
Fox J., Weisberg S. (2019). An R companion to applied regression (Thousand Oaks CA: Sage publications).
Fridley J. D. (2009). Downscaling Climate over Complex Terrain: High Finescale (<1000 m) Spatial Variation of Near-Ground Temperatures in a Montane Forested Landscape (Great Smoky Mountains). J. Appl. Meteorol. Climatol. 48, 1033–1049. doi: 10.1175/2008JAMC2084.1
Gardner-Santana L. C., Beaupre S. J. (2009). Timber Rattlesnakes (Crotalus horridus) Exhibit Elevated and Less Variable Body Temperatures during Pregnancy. Copeia 2009, 363–368. doi: 10.1643/CP-07-271
Gorelick N., Hancher M., Dixon M., Ilyushchenko S., Thau D., Moore R. (2017). Google Earth Engine: Planetary-scale geospatial analysis for everyone. Remote Sens. Environ. 202, 18–27. doi: 10.1016/j.rse.2017.06.031
Grant B. W., Tucker A. D., Lovich J. E., Mills A. M., Dixon P. M., Gibbons J. W. (1992). “The use of coverboards in estimating patterns of reptile and amphibian biodiversity,” in Wildlife 2001: populations. Eds. McCullough D. R., Barrett R. H. (London: Elsevier Applied Science), 379–403.
Gunson K. E., Ireland D., Schueler F. (2012). A tool to prioritize high-risk road mortality locations for wetland-forest herpetofauna in Southern Ontario, Canada. North-Western Journal of Zoology 8, 409–413. Available at: https://biozoojournals.ro/nwjz/content/v8n2/nwjz.121401.Gunson.pdf
Gunson K., Seburn D., Kintsch J., Crowley J. (2016). Best management practices for mitigating the effects of roads on amphibian and reptile species at risk in Ontario. Ontario Ministry of Natural Resources and Forestry Queen's Printer for Ontario, Ontario 7-8. 112 pp. Available at: https://files.ontario.ca/bmp_herp_2016_final_final_resized.pdf
Hall R. J. (1994). Herpetofaunal diversity of the Four Holes Swamp, South Carolina (Washington D.C.: U.S. Department of the Interior).
Harper K. A., Macdonald E., Burton P. J., Chen J., Brosofske K. D., Saunders S. C., et al. (2005). Edge influence on forest structure and composition in fragmented landscapes. Conserv. Biol. 78, 356–782. doi: 10.1111/j.1523-1739.2005.00045.x
Haxton T. (2000). Road mortality of snapping turtles, Chelydra serpentina, in cental Ontario during their nesting period. Can. Field-Naturalist 2000, 106–110.
Herr M. W., Avery J. D., Langkilde T., Howey C. A. F. (2020). Trade-off between thermal quality and predation risk at timber rattlesnake gestation sites. J. Herpetol. 54, 196. doi: 10.1670/18-073
Hobbs M. T., Brehme C. S. (2017). An improved camera trap for amphibians, reptiles, small mammals, and large invertebrates. PloS One 12, e0185026. doi: 10.1371/journal.pone.0185026
Hódar J. A., Pleguezuelos J. M., Poveda J. C. (2000). Habitat selection of the common chameleon (Chamaeleo chamaeleon) (L.) in an area under development in southern Spain: implications for conservation. Biol. Conserv. 94, 63–68. doi: 10.1016/S0006-3207(99)00163-9
Hothorn T., Bretz F., Westfall P. (2008). Simultaneous inference in general parametric models. Biometrical J. 50, 346–363. doi: 10.1002/bimj.200810425
Hromada S. J., Howey C. A. F., Dickinson M. B., Perry R. W., Roosenburg W. M., Gienger C. M. (2018). Response of reptile and amphibian communities to the reintroduction of fire in an oak/hickory forest. For. Ecol. Manage. 428, 1–13. doi: 10.1016/j.foreco.2018.06.018
Huey R. B. (1982). “Temperature, physiology, and the ecology of reptiles,” in Biology of the Reptilia (New York: Academic Press).
Huey R. B. (1991). Physiological consequences of habitat selection. Am. Nat. 137, S91–S115. doi: 10.1086/285141
Huey R. B., Peterson C. R., Arnold S. J., Porter W. P. (1989). Hot rocks and not-so-hot rocks: retreat-site selection by garter snakes and its thermal consequences. Ecology 70, 931–944. doi: 10.2307/1941360
Huijser M. P., Fairbank E. R., Camel-Means W., Graham J., Watson V., Basting P., et al. (2016). Effectiveness of short sections of wildlife fencing and crossing structures along highways in reducing wildlife-vehicle collisions and providing safe crossing opportunities for large mammals. Biol. Conserv. 197, 61–68. doi: 10.1016/j.biocon.2016.02.002
Huijser M. P., McGowen P., Fuller J., Hardy A., Kociolek A., Clevenger A. P., et al. (2008). Wildlife-Vehicle Collision Reduction Study: Report to Congress (Washington D.C.: Federal Highway Administration).
Jackson S. D., Smith D. J., Gunson K. E. (2015). “Mitigating road effects on small animals,” in Roads & Ecological Infrastructure: Concepts and Applications for Small Animals. Eds. Andrews K. M., Nanjappa P., Riley S. P. D. (Baltimore, MD: Johns Hopkins University Press), 177–207.
Jäggi C., Baur B. (1999). Overgrowing forest as a possible cause for the local extinction of Vipera aspis in the northern Swiss Jura mountains. Amphibia-Reptilia 20, 25–34. doi: 10.1163/156853899X00033
Jakes A. F., Jones P. F., Paige L. C., Seidler R. G., Huijser M. P. (2018). A fence runs through it: A call for greater attention to the influence of fences on wildlife and ecosystems. Biol. Conserv. 227, 310–318. doi: 10.1016/j.biocon.2018.09.026
Johnson D. H. (1980). The comparison of usage and availability measurements for evaluating resource preference. Ecology 61, 65–71. doi: 10.2307/1937156
Johnson C. J., Nielsen S. E., Merrill E. H., Mcdonald T. L., Boyce M. S. (2006). Resource selection functions based on use–availability data: theoretical motivation and evaluation methods. J. Wildlife Manage. 70, 347–357. doi: 10.2193/0022-541X(2006)70[347:RSFBOU]2.0.CO;2
Kasten K., Stenoien C., Caldwell W., Oberhauser K. S. (2016). Can roadside habitat lead monarchs on a route to recovery. J. Insect Conserv. 20, 1047–1057. doi: 10.1007/s10841-016-9938-y
Kearney M., Shine R., Porter W. P. (2009). The potential for behavioral thermoregulation to buffer “cold-blooded” animals against climate warming. Proc. Natl. Acad. Sci. United States America 106, 3835–3840. doi: 10.1073/pnas.0808913106
Klingenböck A., Osterwalder K., Shine R. (2000). Habitat use and thermal biology of the “Land Mullet” Egernia major, a large scincid lizard from remnant rain forest in southeastern Australia. Copeia 2000, 931–939. doi: 10.1643/0045-8511(2000)000[0931:HUATBO]2.0.CO;2
Kovar R., Brabec M., Vita R., Bocek R. (2014). Mortality rate and activity patterns of an aesculapian snake (Zamenis longissimus) population divided by a busy road. J. Herpetol. 48, 24–33. doi: 10.1670/12-090
Laidig K. J., Golden D. M. (2004). Assessing Timber Rattlesnake Movements near a Residential Development and Locating New Hibernacula in the New Jersey Pinelands (New Lisbon, NJ: Pinelands Commission and New Jersey Department of Environmental Protection).
Langen T. A., Andrews K. M., Brady S. P., Karraker N. E., Smith D. J. (2015). “Road effects on habitat quality for small animals,” in Roads & Ecological Infrastructure: Concepts and Applications for Small Animals. Eds. Andrews K. M., Nanjappa P., Riley S. P. D. (Baltimore, MD: Johns Hopkins University Press), 57–93.
Langton T. E. S., Clevenger A. P. (2021). Measures to reduce road impacts on amphibians and reptiles in California. Best management practices and technical guidance. Prepared by Western Transportation Institute for California Department of Transportation, Division of Research and Innovation and System Information. Available online at: https://dot.ca.gov/-/media/dot-media/programs/research-innovation-system-information/documents/final-reports/ca20-2700-finalreport-a11y.pdf.
Laurance W. F., Clements G. R., Sloan S., O’Connell C. S., Mueller N. D., Goosem M., et al. (2014). A global strategy for road building. Nature 513, 229–232. doi: 10.1038/nature13717
Macpherson M. R., Litzgus J. D., Weatherhead P. J., Lougheed S. C. (2021). Barriers for big snakes: incorporating animal behaviour and morphology into road mortality mitigation design. Glob. Ecol. Conserv 26, e01471. doi: 10.1016/j.gecco.2021.e01471
Manly B. F. J., McDonald L. L., Thomas D. L., Mcdonald T. L., Erickson W. P. (2002). Resource selection by animals: statistical design and analysis for field studies (Dordrecht, Netherlands: Kluwer Academic Publishers). doi: 10.2307/5247
Marsh D. M., Jaeger J. A. G. (2015). “Direct effects of roads on small animal populations,” in Roads & ecological infrastructure: concepts and applications for small animals. Eds. Andrews K. M., Nanjappa P., Riley S. P. D. (Baltimore, MD: Johns Hopkins University Press), 42–56.
McCullagh P., Nelder J. A. (1989). Generalized linear models. Monographs on statistics and applied probability, Chapman and Hall. (New York).
McDonald T. L. (2013). The point process use-availability or presence-only likelihood and comments on analysis. J. Anim. Ecol. 82, 1174–1182. doi: 10.1111/1365-2656.12132
McKinney M. L. (2006). Urbanization as a major cause of biotic homogenization. Biol. Conserv. 127, 247–260. doi: 10.1016/j.biocon.2005.09.005
Millspaugh J. J., Kesler D. C., Kays R. W., Gitzen R. A., Schulz J. H., Rota C. T., et al. (2012). “Analysis of radiotelemetry data,” in The wildlife techniques manual. Ed. Silvy N. J. (Baltimore: Johns Hopkins University Press), 258–283.
Neilly H., Vanderwal J., Schwarzkopf L. (2016). Balancing biodiversity and food production: a better understanding of wildlife response to grazing will inform off-reserve conservation on rangelands. Rangeland Ecol. Manage. 69, 430–436. doi: 10.1016/j.rama.2016.07.007
New T. R., Sands D. P. A., Taylor G. S. (2021). Roles of roadside vegetation in insect conservation in Australia. Austral Entomol. 60, 128–137. doi: 10.1111/aen.12511
Nordberg E., Ashley J., Hoekstra A. A., Kirkpatrick S., Cobb V. A. (2021). Small nature preserves do not adequately support large-ranging snakes: Movement ecology and site fidelity in a fragmented rural landscape. Global Ecol. Conserv. 28, e01715. doi: 10.1016/j.gecco.2021.e01715
Nordberg E. J., Cobb V. A. (2016). Midwinter emergence in hibernating timber rattlesnakes (Crotalus horridus). J. Herpetol. 50, 203–208. doi: 10.1670/14-113
Nordberg E. J., Cobb V. A. (2017). Body temperatures and winter activity in overwintering timber rattlesnakes (Crotalus horridus) in tennessee, USA. Herpetol. Conserv. Biol. 12, 606–615. Available at: https://www.herpconbio.org/Volume_12/Issue_3/Nordberg_Cobb_2017.pd
Northrup J. M., Hooten M. B., Anderson C. R. Jr., Wittemyer G. (2013). Practical guidance on characterizing availabilty in resource selection functions under a use – availability design. Ecology 94, 1456–1463. doi: 10.1890/12-1688.1
Peaden M. J., Justin Nowakowski A., Tuberville T. D., Buhlmann K. A., Todd B. D. (2017). Effects of roads and roadside fencing on movements, space use, and carapace temperatures of a threatened tortoise. Biol. Conserv. 214, 13–22. doi: 10.1016/j.biocon.2017.07.022
Phillips B. B., Wallace C., Roberts B. R., Whitehouse A. T., Gaston K. J., Bullock J. M., et al. (2020). Enhancing road verges to aid pollinator conservation: A review. Biol. Conserv. 250, 108687. doi: 10.1016/j.biocon.2020.108687
Pike D. A., Webb J. K., Shine R. (2011). Removing forest canopy cover restores a reptile assemblage. Ecol. Appl. 21, 274–280. doi: 10.1890/09-2394.1
Pinheiro J. C., Bates D. M. (2000). Mixed effects models in S and S-Plus (New York: Springer Verlag). Available at: http://www.amazon.com/Mixed-Effects-Models-S-S-Plus/dp/0387989579.
Pomezanski D., Bennett L. (2018). Developing recommendations for monitoring wildlife underpass usage using trail cameras. Environ. Monit Assess. 190, 413. doi: 10.1007/s10661-018-6794-0
Rajvanshi A., Mathur V. B., Teleki G. C., Mukherjee S. K. (2001). Roads, Sensitive Habitats and Wildlife: Environmental Guideline for India and South Asia (Toronto: Wildlife Institute of India, Dehradun and Canadian Environmental Collaborative Ltd).
R Core Team (2022) R: A language and environment for statistical computing. Available at: https://www.r-project.org/.
Reinert H. K. (1984). Habitat separation between sympatric snake populations. Ecology 65, 478–486. doi: 10.2307/1941410
Reinert H. K. (1993). “Habitat selection in snakes,” in Snakes: Ecology and Behavior. Eds. Seigel R. A., Collins J. T. (Caldwell, New Jersey: The Blackburn Press), 201–240.
Reinert H. K., Cundall D. (1982). An improved surgical implantation method for radio-tracking snakes. Copeia 3, 702–705. doi: 10.2307/1444674
Reinert H. K., Cundall D., Bushar L. M. (1984). Foraging behavior of the timber rattlesnake, crotalus horridus. Copeia 1984, 976–981. doi: 10.2307/1445342
Reinert H. K., Zappalorti R. T. (1998). Timber rattlesnakes (Crotalus horridus) of the pine barrens: their movement patterns and habitat preference. Copeia 1988, 964–978. doi: 10.2307/1445720
Rudolph D. C., Burgdorf S. J., Conner R. N., Schaefer R. R. (1999). “Preliminary evaluation of the impact of roads and associated vehicular traffic on snake populations in eastern Texas,” in Proceedings of the International Conference on Wildlife Ecology and Transportation Department of Transportation, Florida Department of Transportation, Tallahassee, Florida. Fl-ER-73-99. 129–136.
Sartorius S. S., Vitt L. J., Colli G. R. (1999). Use of naturally and anthropogenically disturbed habitats in Amazonian rainforest by the teiid lizard Ameiva ameiva. Biol. Conserv. 90, 91–101. doi: 10.1016/S0006-3207(99)00019-1
Sears M. W., Raskin E., Angilletta M. J. (2011). The world is not flat: defining relevant thermal landscapes in the context of climate change. Integr. Comp. Biol. 51, 666–675. doi: 10.1093/icb/icr111
Sears M. W., Angilletta M. J., Schuler M. S., Borchert J., Dilliplane K. F., Stegman M. (2016). Configuration of the thermal landscape determines thermoregulatory performance of ectotherms. Proc Natl Acad Sci U S A 113, 10595–10600. doi: 10.1073/pnas.1604824113
Shine R., Barrott E. G., Elphick M. J. (2002). Some like it hot: effects of forest clearing on nest temperatures of montane reptiles. Ecology 83, 2808–2815. doi: 10.1890/0012-9658(2002)083[2808:SLIHEO]2.0.CO;2
Shine R., Webb J. K., Fitzgerald M., Sumner J. (1998). The impact of bush-rock removal on an endangered snake species, Hoplocephalus bungaroides (Serpentes: Elapidae). Wildlife Res. 25, 285. doi: 10.1071/WR97022
Steen D. A., Aresco M. J., Beilke S. G., Compton B. W., Condon E. P., Dodd C. K., et al. (2006). Relative vulnerability of female turtles to road mortality. Anim. Conserv. 9, 269–273. doi: 10.1111/j.1469-1795.2006.00032.x
Theobald D. M., Harrison-Atlas D., Monahan W. B., Albano C. M. (2015). Ecologically-relevant maps of landforms and physiographic diversity for climate adaptation planning. PloS One 10, 1–17. doi: 10.1371/journal.pone.0143619
Tipton A. F., Vázquez-Diosdado J. A., DeSantis D. L. (2023). Scale-dependent effects of roadways on the movement behavior of a large-bodied pit viper (Crotalus horridus). Front. Ecol. Evol. 11. doi: 10.3389/fevo.2023.1007743
Trombulak S. C., Frissell C. A. (2000). Review of ecological effects of roads on terrestrial and aquatic communities. Conserv. Biol. 14, 18–30. doi: 10.1046/j.1523-1739.2000.99084.x
Vitt L. J., Avila-pires T. C. S., Caldwell J. P., Veronica R. L. (1998). The impact of individual tree harvesting on thermal environments of lizards in amazonian rain forest. Conserv. Biol. 12, 654–664. doi: 10.1046/j.1523-1739.1998.96407.x
Waldron J. L., Lanham J. D., Bennett S. H. (2006). Using behaviorally-based seasons to investigate canebrake rattlesnake (crotalus horridus) movement patterns and habitat selection. Herpetologica 62, 389–398. doi: 10.1655/0018-0831(2006)62[389:UBSTIC]2.0.CO;2
Watson J. E. M., Venter O., Lee J., Jones K. R., Robinson J. G., Possingham H. P., et al. (2018). Protect the last of the wild. Nature 563, 27–30. doi: 10.1038/d41586-018-07183-6
Webb J. K., Shine R., Pringle R. M. (2005). Canopy removal restores habitat quality for an endangered snake in a fire suppressed landscape. Copeia 2005, 894–900. doi: 10.1643/0045-8511(2005)005
Wills C. A., Beaupre S. J. (2000). An application of randomization for detecting evidence of thermoregulation in timber rattlesnakes (Crotalus horridus) from Northwest Arkansas. Physiol. Biochem. Zool. 73, 325–334. doi: 10.1086/316750
Wintle B. A., Kujala H., Whitehead A., Cameron A., Veloz S., Kukkala A., et al. (2018). Global synthesis of conservation studies reveals the importance of small habitat patches for biodiversity. Proc. Natl. Acad. Sci. U.S.A. 116, 909–914. doi: 10.1073/pnas.1813051115
Wittenberg R. D. (2012). Foraging ecology of the timber rattlesnake (Crotalus horridus) in a fragmented agricultural landscape. Herpetol. Conserv. Biol. 7, 449–461. Available at: https://herpconbio.org/Volume_7/Issue_3/Wittenberg_2012.pdf
Worton B. (1989). Kernel methods for estimating the utilization distribution in home-range. Ecology 70, 164–168. doi: 10.2307/1938423
Keywords: road ecology, landscape, heterogeneity, reptile, timber rattlesnake, thermal biology, resource selection
Citation: Sisson GP and Roosenburg WM (2023) The rocks are hotter on the other side of the fence: roadside habitats should inform mitigation design. Front. Ecol. Evol. 11:1059461. doi: 10.3389/fevo.2023.1059461
Received: 01 October 2022; Accepted: 17 August 2023;
Published: 25 September 2023.
Edited by:
Cheryl S. Brehme, Western Ecological Research Center, United StatesReviewed by:
Max Jones, Virginia Tech, United StatesSergio López, University of Science and Arts of Chiapas, Mexico
Copyright © 2023 Sisson and Roosenburg. This is an open-access article distributed under the terms of the Creative Commons Attribution License (CC BY). The use, distribution or reproduction in other forums is permitted, provided the original author(s) and the copyright owner(s) are credited and that the original publication in this journal is cited, in accordance with accepted academic practice. No use, distribution or reproduction is permitted which does not comply with these terms.
*Correspondence: Garrett P. Sisson, Z2FycmV0dF9zaXNzb25AZndzLmdvdg==