- 1Warnell School of Forestry and Natural Resources, University of Georgia, Athens, GA, United States
- 2College of Arts and Sciences, Queens University of Charlotte, Charlotte, NC, United States
Among mobile terrestrial animals, movement among microsites can allow individuals to behaviorally moderate their body temperatures and rates of water loss, which can have important consequences for activity times, growth, fecundity, and survival. Ground-layer vegetation can change the availability and variability of microclimates; however, gaps in our understanding of how individuals interact with the microclimates created by vegetation limit our ability to inform management actions for wildlife. Amphibians can simultaneously balance operant body temperatures and water loss and the availability of heterogeneous microclimates should moderate how effectively they are able to do so. However, relatively few studies have attempted to mechanistically demonstrate how ground vegetation-driven effects on microclimatic variation may affect amphibian performance and survival. Agent-based modeling (ABM) can incorporate behavior and other mechanisms to understand how animals interact with their environments to result in larger scale patterns. They are effective for exploring alternative scenarios and representing the uncertainty in systems. Here, we use ABMs to integrate field and laboratory measurements of movement behavior, physiology, and plant effects on near-ground microclimate to explore how ground vegetation and the availability of terrestrial refugia may affect the survival and terrestrial distributions of juvenile gopher frogs (Rana capito) under two weather regimes. We also examine how assumptions regarding micro-scale movement (< 1 m2) affect the influence of ground vegetation on survival and settlement within refugia. While all variables affected settlement and survival, our models predict that inter-annual variation in weather and the density and spatial distribution of permanent refugia likely have the greatest influence on juvenile survival. The benefit of increased ground vegetation was dependent on the reasonable assumption that gopher frogs exhibit microclimate habitat selection throughout the day and night to limit water loss. Our models suggest that vegetation would be most beneficial to amphibians under warmer weather regimes provided there is sufficient rainfall.
1. Introduction
Many mobile animals move among microclimates as a means to balance physiological demands and constraints (Domínguez–Guerrero et al., 2019; Huey, 1991; Pike and Mitchell, 2013; Scheffers et al., 2014). For terrestrial animals, movement among microsites can allow individuals to behaviorally moderate their body temperatures and rates of water loss, which can have important consequences for activity times, growth, fecundity, and survival (Tracy, 1976; Huey, 1991; Kinlaw, 1999; Cortés et al., 2000; Moseley et al., 2004; Patten et al., 2005; Bartelt et al., 2010; Homyack et al., 2011; Peterman and Semlitsch, 2013; Scheffers et al., 2014; McEntire and Maerz, 2019). Ground-layer vegetation can have important effects on the availability and variability of microclimates (Gates, 1965; Rosenberg et al., 1983). During the day, vegetation can lower temperatures and increase humidity via shading (Gates, 1965; Rosenberg et al., 1983). At night, vegetation can radiate heat and insulate ground radiation, resulting in warmer night temperatures, while areas of open ground can cool rapidly to temperatures lower than that observed under vegetation (Rosenberg et al., 1983; Hossack et al., 2009; Köhler et al., 2011). Thus, vegetation can create more stable thermal environments with higher humidity, and a mixture of ground vegetation and open areas can create variable microclimates that allow animals to move locally to regulate body temperatures and associated water loss.
The availability of microclimates can have important fitness and population consequences for animals. For instance, the lesser prairie-chicken (Tympanuchus pallidicinctus) inhabits hot, dry prairies of the south-central United States, and has been shown to use sites with greater density and cover of dryland shrubs because of access to lower temperatures, reduced wind exposure, and higher humidity (Patten et al., 2005). As the availability of these favorable microclimatic refuges increased, lesser prairie-chicken survival also increased (Grinnell, 1917; Patten et al., 2005). While similar outcomes have been documented for amphibians (Rittenhouse et al., 2008; Semlitsch et al., 2009; Earl and Semlitsch, 2015), reptiles (Costa et al., 2020), and birds (Visco et al., 2015), gaps in our understanding of how individuals interact with microclimates created by vegetation limit our ability to inform management actions for wildlife.
Amphibians and reptiles are particularly sensitive to the effects of climate on their abilities to be active and are, therefore, potentially highly dependent on the availability of diverse microclimates. Among amphibians, which have skin prone to evaporative water loss, the availability of heterogeneous microclimates should moderate whether and how amphibians can simultaneously balance operant body temperatures and water loss (Brattstrom, 1979; Jorgensen, 1997; Guscio et al., 2008; Köhler et al., 2011). Indeed, microhabitat diversity and availability has been linked to amphibian survival (Rittenhouse et al., 2008), occupancy (Constible et al., 2001; Haggerty et al., 2019), performance (Earl and Semlitsch, 2015), and movement (Putnam and Bennett, 1981; Smith et al., 1987; Bartelt et al., 2010; Earl and Semlitsch, 2015); however, relatively few studies have attempted to mechanistically demonstrate how ground vegetation-driven effects on microclimatic variation may affect amphibian performance and survival (but see Bartelt et al., 2010). This has direct relevance to amphibian conservation because many amphibians occupy sites where vegetation communities have been altered by human activities. Altered habitat heterogeneity—particularly cases where ground cover has been reduced—are often presumed to limit an amphibian’s ability to behaviorally moderate their operating temperature or water loss. For instance, the effects of forestry practices on amphibian terrestrial performance can depend on how those practices affect microhabitat structure and species-specific behavioral strategies for using suitable microclimates (Rittenhouse et al., 2008; Semlitsch et al., 2009). Laboratory and field-based choice experiments can and have shown how microclimates affect the operant capacities of species (Brattstrom, 1979; Guscio et al., 2008; Köhler et al., 2011; Pittman et al., 2014), but logistical challenges have limited the ability to study how fine-scale interactions with microclimates may affect amphibian movement and performance at larger spatial scales and across variable climates (but see Lertzman-Lepofsky et al., 2020).
Agent-based modeling (ABM), also known as individual-based modeling, is a framework for answering ecological questions through the behaviors and interactions of individuals with each other and their environment (Grimm and Railsback, 2005; Railsback and Grimm, 2012). One important concept in an ABM framework is that modeling individual behavior and interactions leads to the emergence of system-level properties (Grimm and Railsback, 2005; Railsback and Grimm, 2012). This allows modeling of fine-scale individual processes to observe potential population or system level patterns at large spatial or temporal scales. Importantly, ABMs uniquely can incorporate behavioral mechanisms with other mechanisms to understand how animals interact with their environments to result in these larger scale patterns. ABM models are also effective for exploring alternative scenarios and representing the uncertainty in systems, making ABMs an effective tool for comparing outcomes and exploring causal relationships between fine scale processes and large-scale patterns in a timely and cost-effective manner (Railsback and Grimm, 2012). This is particularly useful for difficult to study species or processes because short-term and small-scale processes that can be measured in field studies can be modeled in a manner to estimate larger scale emergent patterns and reveal mechanistic knowledge gaps that need future research.
Here, we use an ABM to integrate field and laboratory measurements of movement behavior, physiology, and plant effects on near-ground microclimate to explore how ground vegetation and the availability of terrestrial refugia may affect the survival and terrestrial distributions of juvenile gopher frogs (Rana capito). We examined how the assumption that frogs move locally (<1 m) among microclimates alters the effect of ground vegetation on survival and settlement within refugia. The gopher frog is ranked as a Species of Greatest Conservation Need (SGCN) in all states within its range (Alabama, Florida, Georgia, North Carolina, South Carolina, and Tennessee) and is currently under review for listing under the Endangered Species Act. Population declines for this species have been linked to habitat loss and the conversion and degradation of remnant habitat (Hammerson and Jensen, 2004; Jensen, 2005, 2008). The post metamorphic period is particularly risky for pond breeding frogs such as the gopher frog. Following metamorphosis, juveniles disperse from natal ponds through unfamiliar terrestrial upland habitat during the summer to locate permanent refuge. Gopher frogs primarily utilize underground locations (e.g., burrows of gopher tortoises or stump holes) as permanent refuge in the upland environment and have a short window of opportunity, about 2 weeks, after completing metamorphosis to disperse from natal wetlands to these terrestrial refuges (Maerz et al., unpublished data; Roznik and Johnson, 2009a; Hunt, 2019). Underground refuges are more climatically stable and individuals can maintain optimum body temperatures and water balance; and survival is high once inside (Roznik et al., 2009; Roznik and Johnson, 2009a; Pike and Mitchell, 2013). Juvenile frogs (hereafter metamorphs) which do not locate burrows are highly unlikely to survive (Maerz et al., unpublished data; Roznik and Johnson, 2009a; Hunt, 2019). Radio-tracking has revealed broad-scale patterns of mostly linear movement across the landscape when frog locations are recorded at the hour-scale, but fine-scale movement patterns at the meter or minute scale are mostly unknown (Maerz et al., unpublished data; Roznik and Johnson, 2009a; Hunt, 2019). During the day, dispersing metamorphs will shelter under groundcover and in small mammal burrows (Maerz et al., unpublished data; Hunt, 2019). At night, frogs have been tracked to open areas, beneath vegetation, and have been observed climbing low vegetation (Maerz et al., unpublished data; Hunt, 2019). It is unknown whether and how these animals may be selecting among available microhabitats to limit evaporative water loss or for other purposes. Water conserving behavior during nightly dispersal movement may alter the influence of groundcover vegetation on survival of juvenile gopher frogs (Rana capito) in variable landscapes.
2. Methods
2.1. Model overview
The purpose of this model is to explore how behavior influences the effect of habitat and environmental variables on survival of juvenile gopher frogs in variable landscapes. Our model focused on predicting fine-scale dispersal movement through model landscapes representing upland pine savanna habitats which vary in their availability of groundcover vegetation, temporary refuges (e.g., small mammal burrows), and permanent refuges (e.g., gopher tortoise burrows or stump holes). We chose to model behavior in relation to vegetation because of its influence on fine-scale temperature and humidity which directly impact dehydration rates. Among amphibians, behavior (e.g., restricting activity, seeking refuge, and water conserving posture) is a key strategy for moderating evaporative water loss (Hillman et al., 2009). For permanent refuges in our model, we focused on gopher tortoise burrows because they are a key long-term refuge throughout much of the gopher frog range and tortoise habitat and population management is common at many gopher frog sites. The model estimates the probability of survival, the probability of survivors locating a permanent refuge, and the distance survivors travel within 12 days of initiating dispersal from hypothetical cohorts of 2,500 individuals without exposure to predation or other causes of mortality. Mortality in the model is driven solely by biophysical models of dehydration and rehydration. We based our model on prior models of amphibian dehydration rates (Tracy, 1976; Campbell, 1977; Bartelt et al., 2010; Riddell and Sears, 2015) and current knowledge on gopher frog metamorph dispersal behavior including behavior within the same landscape where we conducted field measurements (Roznik and Johnson, 2009a,b; Hunt, 2019).
We created a base model without microclimate selection behavior while moving at night; frogs in this model randomly encounter vegetation patches and associated microclimates. The alternative model included microclimate selection behavior; model frogs moved among microhabitats within a patch (1 m2) to reduce evaporative water loss. In both model versions, if frogs dehydrated below a 10% water loss threshold (defined below), they ceased dispersal and shifted behavior to conserve water loss by occupying areas of coolest temperature and highest relative humidity within the patch. Following rehydration, frogs resumed dispersal. Frogs died if they lost 20% of their starting biomass to water loss. Based on daytime field observations, model frogs that were not within burrows during daylight hours sought cover beneath vegetation where temperature was lower and humidity was higher. Contrasting the outcome of the two models allowed us to explore how assumptions about the behavior of frogs affects the predicted effect of vegetation on frog dispersal and survival. We also ran each model under two alternative weather regimes: cooler, drier and warmer, wetter. These regimes were created from actual weather data during the two most common months of metamorph dispersal, May and June.
2.2. Model landscape development
Detailed methods and descriptions of model structure are presented in the standard Overview, Design concepts, and Details (ODD) protocol (Grimm et al., 2006, 2010) in Appendix 1. We used NetLogo (Version 6.1.1, U. Wilensky, 1999) to simulate gopher frog dispersal on a 2,000 × 2,000 patch landscape, with each patch representing one square meter of upland habitat excepting a single, centered wetland patch. The number of permanent refuges was varied based on published values of gopher tortoise and large mammal burrows per hectare in fire-maintained and non-fire-maintained habitat (2.1 ± 2/ha fire-maintained, 0.5 ± 0.7/ha non-fire-maintained, Ashton et al., 2008). To avoid generating unrealistic values, we used a pre-determined set of values (0–5 per ha) in simulations. Burrows were then spatially distributed based on a gamma distribution of distances from the wetland generated from field data of gopher tortoise observations (alpha = 1.108, lambda = 487.9211; Marshall et al., 2022). Temporary refuges were randomly located in the landscape at a density of either “None” (no temporary refuges), “Low” (5 per ha), “Medium” (15 per ha), or “High” (30 per ha) based on literature values of oldfield mouse (Peromyscus polionotus) abundance and burrow construction (Sumner and Karol, 1929; Davenport, 1964; O'Farrell et al., 1977; Klein and Layne, 1978; Mabry et al., 2003; Morris et al., 2011) and consultation with an expert (SB Castleberry, personal communication). Vegetation within pine savannas can vary from complete cover (100%) to bare (0%) depending on soils as well as historical and current land-use and management (Kirkman et al., 2004; Van Lear et al., 2005). To explore how this variation influences metamorph survival, we set mean vegetation cover each model run and used a random normal distribution to assign individual patch values. Individual behavior within patches was governed by the water-balance sub-model.
Daily weather patterns were generated from a 5-year historical weather dataset to create stochastic but realistic weather patterns of temperature, humidity, and rain (Appendix 2). Each simulated day (24 model steps) a file containing a 24-h weather pattern record was randomly selected and used to set hourly temperature, humidity, and rainfall. Soil moisture tension was modeled based on days since last rainfall for use in rehydration equations. While raining and within 24-h of rain, soil moisture tension was set to 4,400 pascals. For days without rain, we set soil moisture tension to 47,800 pascals if rain had not occurred for 2–3 days previously and 100,000 pascals if rain had not occurred for 4 days or more (Appendix 1). We used algorithms to model ground temperature and humidity relative to air temperature and humidity in exposed areas and under groundcover vegetation (see Appendix 2). Frog water balance (dehydration and rehydration) was calculated on a minute-scale for each hour (see below, Appendix 1). We ran simulations for emergence occurring during cooler, drier, and warmer, wetter weather regimes modeled after historical weather data in May and June to test the effect of weather regime on model outcomes. Simulations ran 100 times for each set of parameters and each simulation ran for 12 days with day and night-time hourly time steps. Hours of daylight each month were set to the monthly average (May–June) for the latitude of our focal field site in Butler, Georgia (32.5571° N, 84.2382° W). The code is available in Appendix 3.
2.3. Agent simulation
We simulated 2,500 individual mobile agents (hereafter gopher frogs) and randomly generated their body mass (m, grams) based on a random gamma distribution of metamorphic mass from 9 years of tadpole rearing data (alpha = 12.27, lambda = 2.85 g; Maerz unpublished data). We chose to use captive rearing data because no data is available on the distribution of masses of metamorphic gopher frogs from natural populations. Therefore, captive-reared tadpoles were the best, most objective, and potentially least biased data available. We calculated individuals’ surface area using the formula:
where SA is equal to the surface area in cm2 and m is the mass of the frog in grams (McClanahan and Baldwin, 1969).
While amphibians are often modeled as free water surfaces with minimal or no resistance to water loss, skin resistance has been documented in many amphibians (Hillman et al., 2009 and references therein; Spotila and Berman, 1976; Riddell and Sears, 2015). We therefore estimated gopher frog skin resistance from our own laboratory study and equations from Tracy (1976), (see Appendix 2 for methods and calculation). We then simulated each frog’s skin resistance from our derived gamma distribution (alpha = 15.07, lambda = 9.92 s cm−1). Using data collected as part of another experiment, we set all individuals’ water loss threshold for ceasing movement to 10% of their original mass and death to 20% (Burrow et al., 2021).
We estimated frog body temperature using algorithms derived from field experiments with live gopher frogs to relate ground temperature to frog temperature separately during the day and night (Appendix 2).
where is the frog’s temperature and is the ground temperature, both in Celsius.
2.4. Model process
We modeled metamorph movement using hourly time-steps. Each hour current rain status, air temperature, and relative humidity were read into the model from a randomly selected file within a set of 24-h weather pattern files (cooler, drier or warmer, wetter; Appendices 1, 2). Once per 24-h soil moisture tension was set based on the number of days since it last rained; at initialization this parameter was set to zero since frogs were in or near the wetland. Frogs set their initial body temperature and experienced humidity based on the hourly values for air temperature and relative humidity.
All frogs began dispersing at night when the humidity was 80% or higher. Once out of the wetland, frogs calculated their water-balance (dehydrate and rehydrate) and then moved each hour. Based on field observations (Hunt, 2019), we had frogs move linearly based on an individual, randomly-selected heading. For this model, we did not include random deviations in heading, so all frogs moved in a straight-line. At night, frogs set their movement rate for the hour using a random normal distribution of movement rates measured during field tracking (6.7 ± 0.94 m/h; Roznik and Johnson, 2009a). Frog movement within patches was modeled as pseudo-movement; the model is not spatially explicit at the sub-patch level but governed by the behavior version and water-balance procedure. As frogs moved at night, if an individual’s water deficit reached or exceeded 10% of its mass, it entered water stress behavior and “moved” to the coolest area within their current patch, which would have been an open area. This behavior continued until the water deficit was below 10% of the frog’s mass, it died, or sunrise. Frogs with less than 10% water loss continued moving to a new patch. If a frog’s proportion of mass lost to dehydration was greater than or equal to 20% it died. During the day, frogs did not leave the wetland or move off their terrestrial current patch (1 m2; Appendix 1). Adjustments to the environmental temperature and humidity frogs experienced were made during the water-balance procedure depending on if the frog was exposed or under ground cover vegetation (Appendices 1, 2). During the day, if the frog was not in a burrow and any level of vegetation was present on their current patch, frogs sheltered beneath vegetation. Frogs which encountered permanent refuge (tortoise burrow) on their current patch moved into the refuge and remained there until the simulation ended. Multiple frogs were able to occupy each available permanent refuge. Frog pseudo-movement within patches and exposure was governed by the behavior version.
We modeled two scenarios of frog behavior. Frogs either had a random probability of being under vegetation within a patch or would “move” into open areas which had cooler temperatures and higher humidity overnight at our focal sites (Appendix 2). This psuedo-movement resulted in changes to temperature and humidity equations within the water-balance procedure not actual movement. In both scenarios, if a frog lost 10% or more of its water and there was an unoccupied temporary refuge (i.e., small mammal burrow) on their current patch, they “moved” into the refuge until rehydrated and then continued dispersing. Frogs of any hydration status which encountered a permanent refuge (i.e., tortoise burrow) remained in the permanent refuge for the duration of the simulation. If no refuge was available on the frog’s current patch and the model included microclimate selection behavior, at night the frog “moved” to an open area on the patch where temperature was lower until it rehydrated, died, or reached sunrise.
We calculated frog dehydration rate using the equation:
where EWL is evaporative water loss (g cm−2 s−1); R is the resistance value of the frog to dehydration (s cm−1); is the vapor pressure density at the frog surface and is assumed to be equal to the saturation vapor pressure density (Tracy, 1976); is the vapor pressure density of the air given the current relative humidity. We estimated these values using standard equations (Appendix 1). To estimate the grams of water lost per minute for each frog, we multiplied the evaporative water loss rate by the individual’s surface area exposed to the air (cm2). We ignored any possible evaporative water loss due to active respiration as this loss would be minimal compared to the skin’s surface area.
We calculated frog rehydration rate using the equation.
where rehydration ( is measured in grams per cm2 per minute, is the water potential of the frog in pascals, is the soil moisture tension in pascals, SA is the surface area of the frog (cm2), and K is the hydraulic conductance, set at 0.00000013 g cm−2 min−1 pa−1 as calculated for Leopard frogs (Rana pipiens; Tracy, 1976). We estimated these values using standard equations (Appendix 1). To estimate the grams of water gained per minute for each frog, we multiplied the rehydration rate by the individual’s ventral surface area exposed to the soil (cm2). Frogs located underground in temporary or permanent refuge were assumed to fully rehydrate and did not dehydrate. Mortality due to water loss was incorporated into the water-balance sub-model. If a frog’s proportion of mass lost to dehydration was greater than or equal to the level of water loss tolerance before death (20%), it died.
2.5. Simulation and data analysis
Our final parameter space included 10 microhabitat values (1–10), six permanent refuge (burrows) values (0–5), and four other-refuge values (none, low, medium, and high). For each of the 240 possible combinations of parameter values, we ran 100 replicate simulations under each weather regime. Each datapoint, therefore, represents the response for a single model run using a particular set of parameter values. We modeled survival and the proportion of surviving frogs located in burrows as proportions using a two-vector response variable where one vector contained the number of successes and one contained the number of failures. For linear distance traveled, we recorded the mean distance surviving frogs traveled for each model run. We completed all analyses in R Statistical Software (v4.0.3; R Core Team, 2021) using the lme4 (Bates et al., 2015), ggplot2 (Wickham, 2016), and ggeffects (Lüdecke, 2018) packages. Step-wise regression suggested all variables were significant predictors, therefore we analyzed the proportion of surviving metamorphs and the proportion of surviving metamorphs in permanent refuge by fitting a set of generalized linear models with binomial distribution using covariate combinations of permanent refuge (burrows), temporary refuge (other ref), vegetation cover (microhabitat), and weather regime (weather) as well as interactions between these covariates (Table 1; Supplementary Tables 1–3). We fit these same covariate combinations for a set of linear models to examine the mean linear distance surviving frogs traveled. We assessed collinearity among covariates (performance package; Lüdecke et al., 2021); all variance inflation factors were 1 suggesting no collinearity. We compared models in a set using AIC and the top model for each outcome of interest was chosen for prediction (Akaike, 1973; Supplementary Tables 1–3).
2.6. Sensitivity analysis
We tested the sensitivity of model output to the value of skin resistance by varying this parameter up and down 50% from our experimental value (standard value: 1.5193 s cm−1; sensitivity range tested: 1.1395–1.8991 s cm−1). Gopher frog metamorph tolerance to desiccation, while based on data collected as part of another experiment, has not been rigorously tested. Therefore, we also tested the model’s sensitivity to water loss tolerance before death (standard value: 0.20; sensitivity range tested: 0.10–0.30) and the proportion of this value that triggered water stress behavior (standard value: 0.50; sensitivity range tested: 0.20–0.80). We used simple linear regression and compared the resulting slopes to evaluate model sensitivity.
3. Results
For both microclimate selection behavior versions of the ABM, the four-way interaction model between the number of burrows, microhabitat, weather regime, and other refuge was the top model for predicting survival and surviving frogs in burrows (Supplementary Tables 1, 2). This model was also the top model for the linear distance traveled of surviving frogs under the no microclimate selection behavior version (Supplementary Table 3). Under the microclimate selection behavior version, linear distance was best described by a model with a three-way interaction between burrows, microhabitat, and weather regime with an additive effect of other refuge (Supplementary Table 3). While significant, the influence of other refuge was minimal and we therefore held this variable at the “Medium” value for prediction and plotting (“Medium”other refuge = 15/ha).
3.1. Survival
Metamorph survival was higher under the warmer, wetter regime and increased with burrow density in both weather regimes (Figures 1A,B). Increasing vegetation had a positive effect on survival under the warmer, wetter regime and was strongest when frogs did not exhibit microclimate selection behavior and burrow availability was limited (Figures 1A,B). Vegetation had a weak to neutral effect under the cooler, drier regime regardless of whether frogs were modeled with microclimate selection behavior or not (Figures 1A,B).
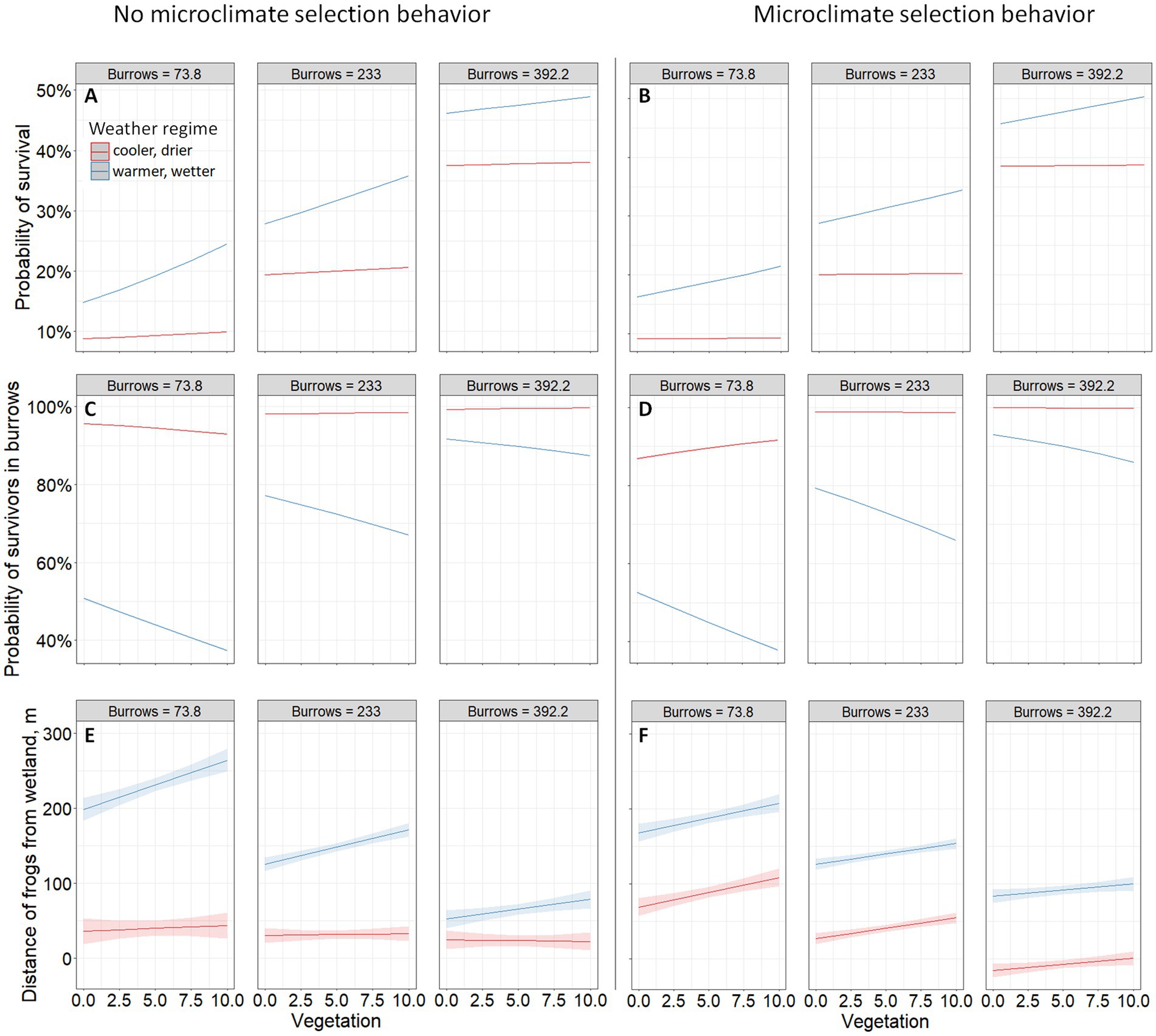
Figure 1. Predicted probability of survival 12 days after dispersal initiation (A,B), probability of surviving frogs in burrows (C,D), and distance of surviving frogs from the wetland (E,F) by vegetation cover and burrow availability in two weather regimes (warmer, wetter or cooler, and drier) with 95% confidence intervals (A–D very tight) for simulated gopher frog (Rana capito) metamorphs under two alternative models of behavior (left—no microclimate selection behavior while moving at night; right—microclimate selection behavior while moving at night). In all models, other refuge was held at the “Medium” level of 15/ha. The top model for each response of interest was chosen for prediction.
3.2. Survivors in burrows
Under the cooler, drier regime, surviving frogs had a 93–100% probability of being in a burrow after 12 days and, apart from when burrow density was low, the effect of vegetation and microclimate selection behavior on the percentage of surviving frogs in burrows was negligible (Figures 1C,D). Under the warmer, wetter regime, surviving frogs had a lower probability of being in a burrow (37–92%), meaning the likelihood that a frog was alive without having found a tortoise burrow increased (Figures 1C,D). The proportion of survivors inside burrows decreased as vegetation cover increased (Figures 1C,D). In other words, the proportion of frogs still alive and on the surface because they had not located a tortoise burrow after 12 days was positively correlated with ground vegetation cover. This effect was similar whether frogs were modeled with microclimate selection behavior or not (Figures 1C,D).
3.3. Linear distance traveled by survivors
Surviving frogs traveled farther under the warmer, wetter regime than under the cooler, drier regime, and this effect was stronger when frogs were not modeled with microclimate selection behavior (Figures 1E,F). Under the cooler, drier regime, frogs traveled farther when they were modeled with microclimate selection behavior (Figures 1E,F). Frogs also traveled farther as vegetation cover increased and burrow density decreased except under the cooler, drier regime without microclimate selection behavior (Figures 1E,F).
3.4. Sensitivity
Under the cooler, drier regime the mean percent of surviving frogs was minimally sensitive to skin resistance (βno behavior = 0.96, βbehavior = −1.07; Supplementary Table 4), water loss tolerance (βno behavior = 1.40, βbehavior = 1.37; Supplementary Table 4), and water stress parameter values (βno behavior = 1.34, βbehavior = −0.30; Supplementary Table 4; Supplementary Figures 1A–C). Under the warmer, wetter regime the mean percent of surviving frogs was more sensitive to skin resistance (βno behavior = 5.44, βbehavior = 5.94; Supplementary Table 4) and water loss tolerance parameter values (βno behavior = 9.44, βbehavior = 8.62; Supplementary Table 4) but mostly insensitive to water stress parameter values (βno behavior = 1.89, βbehavior = −2.31; Supplementary Table 4; Supplementary Figures 1A–C). While the mean percent of surviving frogs varied 0.3–1.4% across our sensitivity tests under the cooler, drier regime (Supplementary Figures 1A–C), survival varied 1.9–9.4% across parameter values under the warmer, wetter regime (Supplementary Figures 1A,B). For most sensitivity scenarios variation in survival was not related to model parameterization (Supplementary Figures 1A–C).
During the cooler, drier regime the mean percent of surviving frogs in burrows was insensitive to changes in parameter values (Supplementary Figures 1D–F; Supplementary Table 4). Under the warmer, wetter weather regime, the mean percent of surviving frogs in burrows decreased with higher values of skin resistance and water loss tolerance (skin resistance: βno behavior = −9.44, βbehavior = −11.06) and increased with water loss tolerance (βno behavior = 9.44, βbehavior = 8.62; Supplementary Table 4) but was minimally sensitive to water stress (βno behavior = −2.59, βbehavior = −3.37; Supplementary Table 4; Supplementary Figures 1D–F). During the warmer, wetter regime, as skin resistance and water loss tolerance parameter values increased, the mean percentage of surviving frogs in burrows declined approximately 9.5–11.6 and 14.5–17.4%, respectively, (Supplementary Figures 1D,E). Under the cooler, drier regime across all parameters and behavior models, approximately 98% or more of the surviving frogs were in burrows (Supplementary Figures 1D–F).
4. Discussion
The availability of tortoise burrows and a wetter weather regime had the largest positive effects on simulated frog survival. The effect of burrow availability is consistent with several studies of juvenile gopher frog survival and density (Maerz et al., unpublished data; Roznik and Johnson, 2009a; Hunt, 2019; Smith et al., 2021). We do note that our model assumed that—based solely on water balance—a juvenile gopher frog that found a tortoise burrow would survive the entire 12-day simulation. This is a simplification, as other factors such as predation are likely to result in mortality of frogs in these habitats (Roznik and Johnson, 2009a; Hunt, 2019). Nonetheless, several studies show that short-term juvenile gopher frog survival is nearly zero if animals do not quickly find suitable animal burrows or stump hole refugia—usually within a few days—and corresponding high afterward (Roznik and Johnson, 2009a; Hunt, 2019). Thus, our model results support field studies that indicate management focused on increasing and sustaining high gopher tortoise densities should increase survival and recruitment of gopher frogs at managed co-occupied sites. Gopher tortoise abundance and associated burrow density is higher in areas of suitable soil that are managed with high frequency prescribed fire that reduces canopy cover and increases ground vegetation (Ashton et al., 2008). Thus, it is likely that—in practice—management actions to increase tortoise abundance will also lead to increased ground cover.
Our two weather regimes were based on real patterns experienced by gopher frogs at our focal field study sites depending on whether juveniles emerge early (May) or later (June). Even though temperatures are typically cooler in May, there is—on average—6% lower relative humidity and 8% less rain. That simulated frog survival was lower under this weather regime predicts that despite cooler temperatures, which lower evaporative water loss, even relatively modest reductions in rainfall and humidity may have large effects on dispersing juvenile frog survival. Furthermore, weather patterns during juvenile dispersal from wetlands may be an important driver of interannual variation in juvenile survival. Amphibians are well known for exhibiting high recruitment stochasticity (Semlitsch et al., 1996; Alford and Richards, 1999) and others have noted the influence of weather on recruitment (Dodd, 1994; Greenberg et al., 2017). Greenberg et al. (2017) found precipitation was related to juvenile recruitment in 21 of 22 studied species, including a positive correlation for juvenile gopher frogs, while temperature had minor effects, and no correlation for gopher frogs. However, as is common in amphibian research, this study only tracked recruitment up to, not through, metamorph emergence and dispersal. Outside of a handful of studies, most notably on the great crested newt (Triturus cristatus) for which weather was a weak predictor of juvenile recruitment, few studies of recruitment include the juvenile dispersal period and weather (Weinbach et al., 2018; Cayuela et al., 2020; but see also Cayuela et al., 2016). Filling this gap in our understanding of the influence of weather and climate patterns on metamorphic survival would require long-term resource-intensive monitoring but would also increase our understanding of amphibian ecology and population dynamics.
Our agent-based biophysical model predicts that the microclimatic influence of vegetation on frog survival, rates of settling in tortoise burrows, and distance dispersed was dependent on the weather regime they experience. Because the major effect of plants is reducing experienced temperatures during the day, the daytime shading effect of vegetation was less important during the cooler, drier regime. Under the cooler, drier weather regimes, vegetation had little predicted effect on survival and settling rates while during warmer, wetter weather regimes, increasing ground vegetation improved frog survival and allowed more survivors to persist outside of burrows. This increased survival outside of burrows highlights the benefits of vegetation on water balance. In other words, our model predicts that increasing vegetation cover allows for longer juvenile persistence during periods of dispersal until suitable terrestrial refugia can be found. Our model also predicts that the effect of increasing vegetation will result in greater dispersal distances except during the cooler, drier regime when frogs were modeled without microclimate selection behavior. During the cooler, drier regime when simulated frogs were modeled with microclimate selection behavior (i.e., use vegetation during the day and open patches at night to remain more hydrated), increased vegetation allowed them to travel farther at night and resulted in greater dispersal distances from the wetland.
Studies demonstrate that frogs move among microhabitats to optimize the benefits of warm temperatures for metabolic efficiency with the costs of elevated water loss (Brattstrom, 1979). However, frog movement decisions at the microscale, including whether frogs are actively selecting among microclimates or whether frogs select for or against vegetation based on associations with microclimate, are still mostly unknown (Bovo et al., 2018). Persistent knowledge gaps limit our ability to understand how amphibians balance and optimize the competing pressures to disperse, optimize body temperature, limit excessive water loss, feed, and avoid predation, as well as the proximate mechanisms including environmental cues and internal regulators of these behaviorally mediated processes (Bovo et al., 2018). Early work suggests that these interactions may not be intuitive. For example, Rittenhouse et al. (2009) found that adults that remained sheltered near aquatic breeding habitat (high frequency of small movement, low net displacement) were at higher desiccation risk than those that risked upland migration (low frequency of movement, high net displacement). It is unlikely that individuals can reduce risks of temperature and water loss simultaneously in a single environment, much less with additional risks, which further complicates physiologically-based movement models because detailed knowledge of animal behavior at this scale is currently lacking (Lertzman-Lepofsky et al., 2020). Though relatively complex, our models, like others to date, remain simplistic and illuminate a substantial gap in mechanistic knowledge of frog behavior that has important consequences for inferences we draw (Bovo et al., 2018). Our model only includes two possible versions of frog behavior, and these are based on assumptions that, while reasonable, have not been empirically tested. We do know from field studies that juvenile gopher frogs that are not in burrows preferentially occupy vegetation during the day (Hunt, 2019; Burrow et al., 2021), and we have observed dispersing juvenile gopher frogs moving and resting in open patches and climbing vegetation during the night. Our weather regimes were modeled during periods of dispersal when temperatures are generally optimally warm, and we did not integrate any metabolic performance functions into our model. In addition, we did not consider other factors that may determine how amphibians interact with structurally mediated microclimates. In reality, vegetation also provides cover and reduces mortality from some predators such as snakes and birds (Hunt, 2019) or some invertebrates (Burrow et al., 2021), but can also concentrate some invertebrate predators, resulting in higher mortality for juvenile amphibians (DeVore and Maerz, 2014). We echo the sentiments of others (e.g., Pittman et al., 2014) who have highlighted our limited understanding of fine-scale movement behavior of juvenile, and many adult, amphibians and repeat their call for expanded research in this area.
There are some important qualifications when interpreting our model results. We caution against interpreting our survival rates as representative of actual survival of animals in the field. Our survival rates across simulations were ~9–50% with and without microclimate selection behavior over 12 days, which was similar to survival rates of radio-tracked juvenile gopher frogs over a similar time period (8–50%, Hunt, 2019; 12.5%, Roznik and Johnson, 2009b). However, in these field studies, mortality was predominately attributed to predation while mortality attributed to desiccation in the field was low (highest 3.1%, Roznik and Johnson, 2009b; Hunt, 2019). It is likely our biophysical model based solely on water loss rates may overestimate mortality, likely because it fails to account for some known and unknown rates or processes (e.g., use of other temporary refugia such as downed woody debris; Bovo et al., 2018). Rates and sources of mortality from telemetry studies are also likely biased because telemetry requires non-random focus on the largest juveniles capable of carrying a radio. Juvenile movement and survival are often positively correlated with size (Altwegg and Reyer, 2003; Berven, 1990; Hunt, 2019; Maerz et al., unpublished data), and field studies of juvenile gopher frogs used significantly larger individuals (mean mass 7.67 g, Hunt, 2019; 7.4 ± 0.2 g, Roznik and Johnson, 2009b). Our model used a distribution derived from entire cohorts of juvenile gopher frogs (mean mass 4.31 ± 1.30 g). It is very likely that smaller juvenile frogs would have desiccation mortality risk not captured by telemetry studies. Predation and dehydration may be partially compensatory sources of mortality. Behavioral demands can result in elevated mortality (e.g., animals in greater need of food or water will engage in riskier behaviors; Bjornson and Anderson, 2018; Houston et al., 1993; McNamara and Houston, 1992). Water deficits, weather constraints, and other needs may constrain antipredator behaviors or increase exposure to predation such as the need to move from suboptimal microclimates when conditions are drier (Rittenhouse et al., 2009). Thus, though the direct cause of observed mortality may be predation, it is possible that predation risk in the field was linked to other physiological or behavioral demands. Further studies would be needed to disentangle these possibly interrelated factors.
Individual frogs in our model moved reasonably comparable distances (up to 300.5 [286.2, 314.8] m without and 216.8 [205.1, 228.5] m with microclimate selection behavior) to field studies. Roznik et al. (2009) recorded movement distances for juveniles and adults of our study species up to 691 m (mean maximum 106.6 ± 16.5 m; mean 60.4 ± 8.5 m). Hunt (2019) recorded a smaller maximum distance of 441 m but greater mean maximum distance (151 ± 97.8 m). A simplifying aspect of our model was the use of linear dispersal paths and a random normal movement rate rather than paths and/or rates that varied by time, temperature, or individual body size. However, juvenile gopher frogs have been shown to exhibit mostly linear movement with few directional changes (Roznik et al., 2009; Hunt, 2019). As noted above, juvenile movement is often positively correlated with size (Altwegg and Reyer, 2003; Berven, 1990; Hunt, 2019; Maerz et al., unpublished data), and field studies by necessity track significantly larger individuals (mean mass 7.67 g, Hunt, 2019; 7.4 ± 0.2 g, Roznik and Johnson, 2009b). Some authors have reported greater movement or movement rates in larger individuals (Hunt, 2019; Maerz et al., unpublished data) but other have not (Roznik et al., 2009; Köhler et al., 2011).
We note the opportunity for further refinement of parameters used as inputs in the biophysical models of water balance that might improve survival estimates. First, our model does not account for boundary layer resistance which can reduce water loss (Hillman et al., 2009). Boundary layer resistance depends on boundary layer thickness, which is determined by wind velocity, wind turbulence, frog size, and frog shape (Hillman et al., 2009). Including boundary layer resistance may improve and increase survival estimates because this resistance can be as great or greater than skin resistance (Hillman et al., 2009). However, given the small size of juvenile gopher frogs, the boundary layer of the ground is likely larger than the frog and therefore may minimally impact water loss estimates (Hillman et al., 2009, p17). Second, our model, like most models, struggles to fully capture soil moisture tension variation in the field (McEntire and Maerz, 2019). Soil moisture tension is a critical determinate of frogs’ ability to draw water from the soil (Tracy, 1976; Hillman et al., 2009). Refining this parameter would require intensive monitoring of soil moisture and moisture tension in the field over time and in variable conditions to generate realistic models of soil moisture.
Despite these limitations our models demonstrate that inter-annual variation in weather regimes, as well as the density and spatial distribution of permanent refugia likely influence juvenile gopher frog survival. Additionally, assumptions about behavior and how researchers model behavior decisions can alter the effect size and direction for environmental variables of interest under some conditions but not others (Bovo et al., 2018). This inconsistency suggests that researchers must be cautious when building, analyzing, and interpreting models with behavioral components. Our models suggest that for gopher frogs, management which focuses on improving habitat for gopher tortoises or retaining stump holes is likely to benefit gopher frogs. Management for ground vegetation will benefit gopher frogs particularly on sites with limited burrow availability. Our model suggests that vegetation would most likely be beneficial under warmer weather regimes (climate) provided there is sufficient rainfall, suggesting vegetation management may be a relevant tool for addressing climate change impacts on pond-breeding amphibian populations. With increasing knowledge of gopher frog behavior and ecophysiology, our model can be adapted to be increasingly realistic and allow for models of how fine scale interactions of individuals with their local environment result in emergent patterns of survival and spatial distributions that remain otherwise empirically intractable. Our field data supported model can be adapted for other amphibian species, and as demonstrated in this paper, we believe such models will be particularly useful for informing management actions for priority amphibian species like the gopher frog.
Data availability statement
The original contributions presented in the study are included in the article/Supplementary material, further inquiries can be directed to the corresponding author.
Ethics statement
All methods were approved by the University of Georgia Institutional Animal Care and Use Committee (AUP# A2018 02-019-A4). The collection of animals for use in this research was permitted by the Georgia Department of Natural Resources (GA DNR Permit 1000602439).
Author contributions
AB conceived the research, conducted field and laboratory work, developed the model code, analyzed the data, and wrote the manuscript. KM developed the model code and provided comments on data analysis and the manuscript. JM conceived the research and provided comments on data analysis and co-drafted and edited the manuscript. All authors contributed to the article and approved the submitted version.
Funding
This work was supported by a National Science Foundation Graduate Research Fellowship under grant no. 049347-06, a P. E. O. Scholar Award awarded to AB, and a GA DNR State Wildlife Grant awarded to JM. Any opinions, findings, and conclusions or recommendations expressed in this material are those of the authors and do not necessarily reflect the views of the National Science Foundation.
Acknowledgments
We thank James Hunt and Maisie MacKnight for field work assistance, Coles Ehlers for assistance with development and testing gopher frog physical models, and Sherry Savrda for assistance with biophysical models. We also thank two reviewers whose comments helped us in improving the manuscript.
Conflict of interest
The authors declare that the research was conducted in the absence of any commercial or financial relationships that could be construed as a potential conflict of interest.
Publisher’s note
All claims expressed in this article are solely those of the authors and do not necessarily represent those of their affiliated organizations, or those of the publisher, the editors and the reviewers. Any product that may be evaluated in this article, or claim that may be made by its manufacturer, is not guaranteed or endorsed by the publisher.
Supplementary material
The Supplementary material for this article can be found online at: https://www.frontiersin.org/articles/10.3389/fevo.2023.1026541/full#supplementary-material
References
Akaike, H. (1973). “Information theory and an extension of the maximum likelihood principle” in 2nd International Symposium on Information Theory, Tsahkadsor, Armenia, USSR. eds. B. N. Petrov and F. Csáki; Budapest: Akadémiai Kiadó, 267–281, September 2–8, 1971.
Alford, R. A., and Richards, S. J. (1999). Global amphibian declines: A problem in applied ecology. Annu. Rev. Ecol. Syst. 30, 133–165. doi: 10.1146/annurev.ecolsys.30.1.133
Altwegg, R., and Reyer, H. (2003). Patterns of natural selection on size at metamorphosis in Water frogs. Evolution 57, 872–882.
Ashton, K. C., Engelhardt, B. M., and Branciforte, B. S. (2008). Gopher tortoise (Gopherus polyphemus) abundance and distribution after prescribed fire reintroduction to Florida scrub and sandhill at Archbold Biological Station. J. Herpetol. 42, 523–529. doi: 10.1670/06-246.1
Bartelt, P. E., Klaver, R. W., and Porter, W. P. (2010). Modeling amphibian energetics, habitat suitability, and movements of western toads, Anaxyrus (Bufo) boreas, across present and future landscapes. Ecol. Model. 221, 2675–2686. doi: 10.1016/j.ecolmodel.2010.07.009
Bates, D., Maechler, M., Bolker, B., and Walker, S. (2015). Fitting linear mixed-effects models using lme4. J. Stat. Softw. 67, 1–48. doi: 10.18637/jss.v067.i01
Berven, K. A. (1990). Factors affecting population fluctuations in larval and adult stages of the wood frog (Rana sylvatica). Ecology 71, 1599–1608.
Bjornson, F., and Anderson, G. W. (2018). Body condition, rather than size, predicts risk-taking and resource holding potential in hatchery reared juvenile lake sturgeon Acipenser fulvescens. J. Fish. Biol. 93, 1188–1196.
Bovo, R. P., Navas, C. A., Tejedo, M., Valença, S. E. S., and Gouveia, S. F. (2018). Ecophysiology of amphibians: information for best mechanistic models. Diversity 10, 1–14. doi: 10.3390/d10040118
Brattstrom, B. H. (1979). Amphibian temperature regulation studies in the field and laboratory. Am. Zool. 19, 345–356. doi: 10.1093/icb/19.1.345
Burrow, A. B., Crawford, B. A., and Maerz, J. C. (2021). Groundcover and native ant predation influence survival of metamorphic amphibians in a southeastern pine savanna undergoing restoration. Restor. Ecol. 29:e13410. doi: 10.1111/rec.13410
Cayuela, H., Arsovski, D., Thirion, J. M., Bonnaire, E., Pichenot, J., Boitaud, S., et al. (2016). Demographic responses to weather fluctuations are context dependent in a long-lived amphibian. Glob. Chang. Biol. 22, 2676–2687. doi: 10.1111/gcb.13290
Cayuela, H., Griffiths, R. A., Zakaria, N., Arntzen, J. W., Priol, P., Lena, J. P., et al. (2020). Drivers of amphibian population dynamics and asynchrony at local and regional scales. J. Anim. Ecol. 89, 1350–1364. doi: 10.1111/1365-2656.13208
Constible, J. M., Gregory, P. T., and Anholt, B. R. (2001). Patterns of distribution, relative abundance, and microhabitat use of anurans in a boreal landscape influenced by fire and timber harvest. Ecoscience 8, 462–470. doi: 10.1080/11956860.2001.11682676
Cortés, A., Miranda, E., Rosenmann, M., and Rau, J. R. (2000). Thermal biology of the fossorial rodent Ctenomys fulvus from the Atacama desert, northern Chile. J. Therm. Biol. 25, 425–430. doi: 10.1016/S0306-4565(00)00005-X
Costa, B. M., Pantoja, D. L., Sousa, H. C., de Queiroz, T. A., and Colli, G. R. (2020). Long-term, fire-induced changes in habitat structure and microclimate affect Cerrado lizard communities. Biodivers. Conserv. 29, 1659–1681. doi: 10.1007/s10531-019-01892-8
Davenport, L. B. Jr. (1964). Structure of two Peromyscus polionotus populations in old-field ecosystems at the AEC Savannah river plant. J. Mammal. 45, 95–113. doi: 10.2307/1377298
DeVore, J., and Maerz, J. C. (2014). Grass invasion increases top-down pressure on an amphibian via structurally mediated effects on an intraguild predator. Ecology 95, 1724–1730. doi: 10.1890/13-1715.1
Dodd, C. K. (1994). The effects of drought on population structure, activity, and orientation of toads (Bufo quercicus and B. terrestris) at a temporary pond. Ethol. Ecol. Evol. 6, 331–319. doi: 10.1080/08927014.1994.9522985
Domínguez–Guerrero, S. F., Muñoz, M. M., Pasten–Téllez, D. J., Arenas–Moreno, D. M., Rodríguez–Miranda, L. A., Manríquez–Morán, N. L., et al. (2019). Interactions between thermoregulatory behavior and physiological acclimatization in a wild lizard population. J. Therm. Biol. 79, 135–143. doi: 10.1016/j.jtherbio.2018.12.001
Earl, J. E., and Semlitsch, R. D. (2015). Importance of forestry practices relative to microhabitat and microclimate changes for juvenile pond-breeding amphibians. For. Ecol. Manag. 357, 151–160. doi: 10.1016/j.foreco.2015.08.023
Gates, D. M. (1965). Heat transfer in plants. Sci. Am. 213, 76–84. doi: 10.1038/scientificamerican1265-76
Greenberg, C. H., Zarnoch, S. J., and Austin, J. D. (2017). Weather, hydroregime, and breeding effort influence juvenile recruitment of anurans: implications for climate change. Ecosphere 8:e01789. doi: 10.1002/ecs2.1789
Grimm, V., Berger, U., Bastiansen, F., Eliassen, S., Ginot, V., Giske, J., et al. (2006). A standard protocol for describing individual-based and agent-based models. Ecol. Model. 198, 115–126. doi: 10.1016/j.ecolmodel.2006.04.023
Grimm, V., Berger, U., DeAngelis, D. L., Polhill, J. G., Giske, J., and Railsback, S. F. (2010). The ODD protocol: A review and first update. Ecol. Model. 221, 2760–2768. doi: 10.1016/j.ecolmodel.2010.08.019
Grimm, V., and Railsback, S. F.. (2005). Individual-Based Modeling and Ecology. New Jersey, Princeton University Press
Grinnell, J. (1917). The niche-relationships of the California thrasher. Auk 34, 427–433. doi: 10.2307/4072271
Guscio, C. G., Hossack, B. R., Eby, L. A., and Corn, P. S. (2008). Post-breeding habitat use by adult boreal toads (Bufo boreas) after wildfire in glacier National Park, USA. Herpetol. Conserv. Biol. 3, 55–62.
Haggerty, C. J. E., Crisman, T. L., and Rohr, J. R. (2019). Direct and indirect effects of pine silviculture on the larval occupancy and breeding of declining amphibian species. J. Appl. Ecol. 56, 2652–2662. doi: 10.1111/1365-2664.13493
Hammerson, G., and Jensen, J.. (2004). Lithobates capito. The IUCN Red List of Threatened Species. Available at: http://dx.doi.org/10.2305/IUCN.UK.2004.RLTS.T58564A11786752.en (Accessed February 8, 2020).
Hillman, S. S., Withers, P. C., Drewes, R. C., and Hillyard, S. D.. (2009). Ecological and Environmental Physiology of Amphibians. Oxford, Oxford University Press
Homyack, J. A., Haas, C. A., and Hopkins, W. A. (2011). Energetics of surface-active terrestrial salamanders in experimentally harvested forest. J. Wildl. Manag. 75, 1267–1278. doi: 10.1002/jwmg.175
Hossack, B. R., Eby, L. A., Guscio, C. G., and Corn, P. S. (2009). Thermal characteristics of amphibian microhabitats in a fire-disturbed landscape. For. Ecol. Manag. 258, 1414–1421. doi: 10.1016/j.foreco.2009.06.043
Houston, A. I., McNamara, J. M., and Hutchinson, J. M. C. (1993). General results concerning the trade-off between gaining energy and avoiding predation. Philos. Trans. Roy. Soc. London Ser. B. Biol. Sci. 341, 375–397.
Huey, R. B. (1991). Physiological consequences of habitat selection. Am. Nat. 137 (Jun., 1991)), S91–S115.
Hunt, J. (2019). Improving monitoring and habitat assessment for gopher frog (Rana [Lithobates] capito) management in Georgia. Master’s Thesis. [Athens (GA)]: University of Georgia
Jensen, J. (2005). “Gopher frogs, Rana capito” in Amphibian Declines: The Conservation Status of United States Species. ed. M. J. Lannoo (Berkley, CA: University of California Press), 536–538.
Jorgensen, C. B. (1997). 200 years of amphibian water economy: from Robert Townsend to the present. Biol. Rev. Camb. Philos. Soc. 72, 153–237. doi: 10.1017/s0006323196004963
Kinlaw, A. (1999). A review of burrowing by semi-fossorial vertebrates in arid environments. J. Arid Environ. 41, 127–145. doi: 10.1006/jare.1998.0476
Kirkman, L. K., Coffey, K. L., Mitchell, R. J., and Moser, E. B. (2004). Ground cover recovery patterns and life-history traits: implications for restoration obstacles and opportunities in a species-rich savanna. J. Ecol. 92, 409–421. doi: 10.1111/j.0022-0477.2004.00883.x
Klein, H. G., and Layne, J. N. (1978). Nesting behavior in four species of mice. J. Mammal. 59, 103–108. doi: 10.2307/1379879
Köhler, A., Sadowska, J., Olszewska, J., Trzeciak, P., Berger-Tal, O., and Tracy, C. R. (2011). Staying warm or moist? Operative temperature and thermal preferences of common frogs (Rana temporaria), and effects on locomotion. Herpetol. J. 21, 17–26.
Lertzman-Lepofsky, G. F., Kissel, A. M., Sinervo, B., and Palen, W. J. (2020). Water loss and temperature interact to compound amphibian vulnerability to climate change. Glob. Chang. Biol. 26, 4868–4879. doi: 10.1111/gcb.15231
Lüdecke, D. (2018). Ggeffects: tidy data frames of marginal effects from regression models. J. Open Sour. Softw. 3:772. doi: 10.21105/joss.00772
Lüdecke, D., Ben-Shachar, M. S., Patil, I., Waggoner, P., and Makowski, D. (2021). Performance: an R package for assessment, comparison and testing of statistical models. J. Open Sour. Softw. 6:3139. doi: 10.21105/joss.03139
Mabry, K. E., Dreelin, E. A., and Barrett, G. W. (2003). Influence of landscape elements on population densities and habitat use of three small-mammal species. J. Mammal. 84, 20–25. doi: 10.1644/1545-1542(2003)084<0020:IOLEOP>2.0.CO;2
Marshall, C. D., Maerz, J. C., Smith, L. L., Elliott, M., McGuire, S., and Crawford, B. A.. (2022). Using ancillary data to model the terrestrial distribution of an elusive frog. Wildlife Biology: in review WLB-2022-01044.
McClanahan, L. Jr., and Baldwin, R. (1969). Rate of water uptake through the integument of the desert toad, Bufo punctatus. Comp. Biochem. Physiol. 28, 381–389. doi: 10.1016/0010-406X(69)91351-6
McEntire, K. D., and Maerz, J. C. (2019). Integrating ecophysiological and agent-based models to simulate how behavior moderates salamander sensitivity to climate. Front. Ecol. Evol. 7:22. doi: 10.3389/fevo.2019.00022
McNamara, J. M., and Houston, A. I. (1992). Risk-sensitive foraging-A review of the theory. Bull. Math. Biol. 54, 355–378.
Morris, G., Hostetler, J. A., Oli, M. K., and Conner, L. M. (2011). Effects of predation, fire, and supplemental feeding on populations of two species of Peromyscus mice. J. Mammal. 92, 934–944. doi: 10.1644/10-MAMM-A-419.1
Moseley, K. R., Castleberry, S. B., and Ford, W. M. (2004). Coarse woody debris and pine litter manipulation effects on movement and microhabitat use of Ambystoma talpoideum in a Pinus taeda stand. For. Ecol. Manag. 191, 387–396. doi: 10.1016/j.foreco.2004.01.015
O'Farrell, M. J., Kaufman, D. W., and Lundahl, D. W. (1977). Use of live-trapping with the assessment line method for density estimation. J. Mammal. 58, 575–582. doi: 10.2307/1380005
Patten, M. A., Wolfe, D. H., Shochat, E., and Sherrod, S. K. (2005). Effects of microhabitat and microclimate selection on adult survivorship of the lesser prairie-chicken. J. Wildl. Manag. 69, 1270–1278. doi: 10.2193/0022-541X(2005)069[1270:EOMAMS]2.0.CO;2
Peterman, W. E., and Semlitsch, R. D. (2013). Fine-scale habitat associations of a terrestrial salamander: the role of environmental gradients and implications for population dynamics. PLoS One 8:e62184. doi: 10.1371/journal.pone.0062184
Pike, D. A., and Mitchell, J. C. (2013). Burrow-dwelling ecosystem engineers provide thermal refugia throughout the landscape. Anim. Conserv. 16, 694–703. doi: 10.1111/acv.12049
Pittman, S. E., Osbourn, M. S., and Semlitsch, R. D. (2014). Movement ecology of amphibians: A missing component for understanding population declines. Biol. Conserv. 169, 44–53. doi: 10.1016/j.biocon.2013.10.020
Putnam, R. W., and Bennett, A. F. (1981). Thermal dependence of behavioral performance of anuran amphibians. Anim. Behav. 29, 502–509. doi: 10.1016/S0003-3472(81)80111-X
R Core Team (2021). R: A language and environment for statistical computing. R Foundation for Statistical Computing, Vienna, Austria. Available at: https://www.R-project.org/
Railsback, S. F., and Grimm, V.. (2012). Agent-Based and Individual-Based Modeling: A Practical Introduction. Princeton: Princeton University Press
Riddell, E. A., and Sears, M. W. (2015). Geographic variation of resistance to water loss within two species of lungless salamanders: implications for activity. Ecosphere 6:art86. doi: 10.1890/ES14-00360.1
Rittenhouse, T. A. G., Harper, E. B., Rehard, L. R., and Semlitsch, R. D. (2008). The role of microhabitats in the desiccation and survival of anurans in recently harvested oak-Hickory forest. Copeia 2008:807. doi: 10.1643/CH-07-176
Rittenhouse, T. A. G., Semlitsch, R. D., and Thompson, F. R. III (2009). Survival costs associated with wood frog breeding migrations: effects of timber harvest and drought. Ecology 90, 1620–1630. doi: 10.1890/08-0326.1
Rosenberg, N. J., Blad, B. L., and Verma, S. B.. (1983). Microclimate: The Biological Environment 2nd Edn. New York: John Wiley & Sons, Inc
Roznik, E. A., and Johnson, S. A. (2009a). Burrow use and survival of newly metamorphosed gopher frogs (Rana capito). J. Herpetol. 43, 431–437. doi: 10.1670/08-159R.1
Roznik, E. A., Johnson, S. A., Greenberg, C. H., and Tanner, G. W. (2009). Terrestrial movements and habitat use of gopher frogs in longleaf pine forests: A comparative study of juveniles and adults. For. Ecol. Manag. 259, 187–194. doi: 10.1016/j.foreco.2009.10.007
Roznik, E. A., and Johnson, S. A. (2009b). Rana capito (gopher frog). Burrow cohabitation Herpetol. Rev. 40:209.
Scheffers, B. R., Edwards, D. P., Diesmos, A., Williams, S. E., and Evans, T. A. (2014). Microhabitats reduce animal's exposure to climate extremes. Glob. Chang. Biol. 20, 495–503. doi: 10.1111/gcb.12439
Semlitsch, R. D., Scott, D. E., Pechmann, J. H. K., and Gibbons, J. W. (1996). “Structure and dynamics of an amphibian community: evidence from a 16-year study of a natural pond” in Long-Term Studies of Vertebrate Communities. eds. M. L. Cody and J. A. Smallwood (San Diego, CA: Academic Press), 217–248.
Semlitsch, R. D., Todd, B. D., Blomquist, S. M., Calhoun, A. J. K., Gibbons, J. W., Gibbs, J. P., et al. (2009). Effects of timber harvest on amphibian populations: understanding mechanisms from forest experiments. Bioscience 59, 853–862. doi: 10.1525/bio.2009.59.10.7
Smith, L. S., Howze, J. M., Staiger, J. S., Sievers, E. R., Burr, D., and Enge, K. M. (2021). Added value: gopher tortoise surveys provide estimates of gopher frog abundance in tortoise burrows. J. Fish Wildl. Manag. 12, 3–11. doi: 10.3996/JFWM-20-030
Smith, E. M., Pough, F. H., Collazo, A., and Rhodes, D. H. (1987). The abundance of salamanders in forest stands with different histories of disturbance. For. Ecol. Manag. 20, 1–9.
Spotila, J. R., and Berman, E. N. (1976). Determination of skin resistance and the role of the skin in controlling water loss in amphibians and reptiles. Comp. Biochem. Physiol. 55, 407–411. doi: 10.1016/0300-9629(76)90069-4
Sumner, F. B., and Karol, J. J. (1929). Notes on the burrowing habits of Peromyscus polionotus. J. Mammal. 10, 213–215. doi: 10.2307/1373928
Tracy, C. R. (1976). A model of the dynamic exchanges of water and energy between a terrestrial amphibian and its environment. Ecol. Monogr. 46, 293–326. doi: 10.2307/1942256
Van Lear, D. H., Carroll, W. D., Kapeluck, P. R., and Johnson, R. (2005). History and restoration of the longleaf pine-grassland ecosystem: implications for species at risk. For. Ecol. Manag. 211, 150–165. doi: 10.1016/j.foreco.2005.02.014
Visco, D. M., Michel, N. L., Boyle, W. A., Sigel, B. J., Woltmann, S., and Sherry, T. W. (2015). Patterns and causes of understory bird declines in human-disturbed tropical forest landscapes: A case study from Central America. Biol. Conserv. 191, 117–129. doi: 10.1016/j.biocon.2015.05.018
Weinbach, A., Cayuela, H., Grolee, O., Besnard, A., and Joly, P. (2018). Resilience to climate variation in a spatially structured amphibian population. Sci. Rep. 8:14607. doi: 10.1038/s41598-018-33111-9
Wilensky, U. (1999). NetLogo. Available at: http://ccl.northwestern.edu/netlogo/ (Accessed March 12, 2017).
Keywords: ground cover, behavior, gopher frog, Rana capito, dispersal, movement, weather, refuge
Citation: Burrow AK, McEntire KD and Maerz JC (2023) Estimating the potential drivers of dispersal outcomes for juvenile gopher frogs (Rana capito) using agent-based models. Front. Ecol. Evol. 11:1026541. doi: 10.3389/fevo.2023.1026541
Edited by:
Leonardo Montagnani, Free University of Bozen-Bolzano, ItalyReviewed by:
Ross Hinderer, University of Montana, United StatesLifeng Zhu, Nanjing University of Chinese Medicine, China
Copyright © 2023 Burrow, McEntire and Maerz. This is an open-access article distributed under the terms of the Creative Commons Attribution License (CC BY). The use, distribution or reproduction in other forums is permitted, provided the original author(s) and the copyright owner(s) are credited and that the original publication in this journal is cited, in accordance with accepted academic practice. No use, distribution or reproduction is permitted which does not comply with these terms.
*Correspondence: Angela K. Burrow, ✉ YW5nZWxhLmJ1cnJvd0Bjb3JuZWxsLmVkdQ==