- 1Department of Biology, University of Toronto Mississauga, Mississauga, ON, Canada
- 2Harkness Laboratory of Fisheries Research, Aquatic Research and Monitoring Section, Ontario Ministry of Natural Resources and Forestry, Peterborough, ON, Canada
Seasonality could play a crucial role in structuring species interactions. For example, many ectotherms alter their activity, habitat, and diet in response to seasonal temperature variation. Species also vary widely in physiological traits, like thermal preference, which may mediate their response to seasonal variation. How behavioral responses to seasonality differ between competing species and alter their overlap along multiple niche axes in space and time, remains understudied. Here, we used bulk carbon and nitrogen stable isotopes combined with stomach content analysis to determine the seasonal diet overlap between a native cold-water species [lake trout (Salvelinus namaycush)] and a range-expanding warm-water species [smallmouth bass (Micropterus dolomieu)] in two north-temperate lakes over 2 years. We coupled these analyses with fine-scale acoustic telemetry from one of the lakes to determine seasonal overlap in habitat use and activity levels. We found that dietary niche overlap was higher in the spring, when both species were active and using more littoral resources, compared to the summer, when the cold-water lake trout increased their reliance on pelagic resources. Telemetry data revealed that activity rates diverged in the winter, when lake trout remained active, but the warm-water smallmouth bass reduced their activity. Combining stable isotopes and stomach contents with acoustic telemetry was a powerful approach for demonstrating that species interactions are temporally and spatially dynamic. In our case, the study species diverged in their diet, habitat, and activity more strongly during certain times of the year than others, in ways that were consistent with their thermal preferences. Despite large differences in thermal preference, however, there were times of year when both species were active and sharing a common habitat and prey source (i.e., resource overlap was greater in spring than summer). Based on our findings, important ecological processes are occurring during all seasons, which would be missed by summer sampling alone. Our study stresses that quantifying multiple niche axes in both space and time is important for understanding the possible outcomes of altered seasonal conditions, including shorter winters, already arising under a changing climate.
Introduction
Species coexistence has captivated ecologist for decades. Foundational studies including theoretical modeling (Volterra, 1926; Lotka, 1932), laboratory (Gause, 1934; Park, 1954), and field experiments (Tansley, 1917; Connell, 1961) culminated in the competitive exclusion principle. This principle can be summarized as “complete competitors cannot co-exist” (Hardin, 1960). However, species may coexist if they occupy different habitats (spatial niche partitioning; Macarthur, 1958) or are favored by the physical environment at different times (temporal niche partitioning; Hutchinson, 1961). Species responses to spatial variation has received substantially more research attention than responses to temporal variation, despite the recognition that species can use different resources and be active at different times of the year (Chesson and Huntly, 1997; Shuter et al., 2012; McMeans et al., 2015).
Much of the work on temporal niche partitioning has focused on year-to-year differences in physical conditions (e.g., the amount of precipitation; Angert et al., 2009), but ecosystems also vary dramatically within a year from one season to the next. The role seasonality may play in coexistence has empirical (Shimadzu et al., 2013; McMeans et al., 2020) and theoretical support (Chesson, 2000; Mathias and Chesson, 2013). Central to these coexistence mechanisms are species responding differently to changing conditions. For example, coexistence may be promoted by seasonal patterns in light levels differentially impacting species’ foraging efficiency (Helland et al., 2011), or seasonal patterns in temperature and precipitation differentially impacting species’ metabolic rates (Szabó, 2016). Further, seasonally mediated coexistence has the potential to be widespread given that many ecosystems experience seasonal variation that impacts species interactions, including wet-dry seasonality in tropical river-floodplains (McMeans et al., 2019) and spring, summer, fall and winter seasonality in north-temperate lakes (Tonn and Magnuson, 1982).
North-temperate lakes (40–60°N) are ideal systems to explore species responses to seasonally changing conditions and consequences for niche overlap in time and space. These lakes undergo drastic seasonal changes in abiotic factors, including temperature and light minima during ice-covered winters ranging from 1 to 6 months (Shuter et al., 2012). For ectothermic organisms, like fish, temperature is a particularly important abiotic factor, affecting growth, locomotory activity, metabolism, and survival (Fry, 1947; Brett, 1971). Temperature influences biochemical processes and different species physiologies are optimized to perform best at specific temperatures (Brett, 1971; Beitinger and Fitzpatrick, 1979). Because of these optimized physiologies, fishes have distinct and well-established thermal preferences (the temperature species occupy when presented with a temperature range), which have been used to assign species to the warm, cool or cold-water thermal guild (Magnuson et al., 1979; Hasnain et al., 2018; Benoit et al., 2021).
Species in different thermal guilds may respond differently to changes in water temperatures as lakes’ thermal structure changes seasonally. During the summer, many north-temperate lakes thermally stratify, which can spatially segregate species belonging to different thermal guilds (Guzzo et al., 2016). Cold-water species mainly occupy deeper offshore water, with access to the warmer nearshore area in the summer restricted to short forays (Goyer et al., 2014; Pépino et al., 2015). In the spring, fall, and winter, the nearshore water is cooler, making the area more thermally accessible to cold-water fishes. While cooler nearshore water can increase foraging opportunities for cold-water species (Guzzo et al., 2017), niche overlap with warm-water species may also increase (Vander Zanden et al., 1999). However, during the winter, warm-water fishes are expected to perform poorly and can decrease their activity rates compared to cold-water fishes because of different responses to low temperatures (Shuter et al., 2012). Seasonal changes in water temperature that drives divergent habitat use, diet, or activity rate could therefore be a mechanism for promoting coexistence in north-temperate lakes (Helland et al., 2011; McMeans et al., 2020).
Rapid changes are occurring in north-temperate lakes that may disrupt existing coexistence mechanisms. Increasing air temperature is predicted to cause later ice-on in the fall and earlier ice-off in the spring (Keller, 2007). Predictions about the timing of these key seasonal events are supported by long-term studies of lakes in North America and globally (Benson et al., 2012; Sharma et al., 2016; Guzzo and Blanchfield, 2017; Woolway et al., 2020). Thus, the winter period in temperate lakes, as defined as a period of ice cover, is shrinking while the open-water period is expanding. To predict how species interactions will be altered under climate change scenarios, the role of current environmental periodicities in structuring competition and predation must be understood. However, current gaps in the literature limit our predictive ability. Many studies on temperate lakes have only considered the ice-free period due to the logistical and safety challenges of winter field work (Block et al., 2019). Studies that consider seasonal changes in both activity rates and resource use of potential competitors are especially rare. Combining existing methods, including stable isotopes and telemetry, is a promising approach for elucidating seasonal habitats, activity, and species interactions (McMeans et al., 2015; Marsden et al., 2021).
Here, we explored the niche overlap between fishes from the cold-water and warm-water thermal guild across all four seasons (spring, summer, fall, and winter) in two north-temperate lakes over a 2-year period. The native lake trout (Salvelinus namaycush) is our representative species of the cold-water guild and the range expanding smallmouth bass (Micropterus dolomieu) is our representative species of the warm-water guild. The thermal preference of the species differs substantially, with lake trout having a thermal preference of 11.8°C and smallmouth bass having a thermal preference of 25°C (Hasnain et al., 2018). Studies conducted during the open water period suggest that exploitative competition can occur between lake trout and smallmouth bass, where the latter reduce the diversity and abundance of littoral prey fish through predation (Vander Zanden et al., 1999; MacRae and Jackson, 2001). These reductions in prey fish may impact lake trout because they are mobile foragers that feed in both littoral and the pelagic zones (Tunney et al., 2012; Guzzo et al., 2017). In small lakes with smallmouth bass, lake trout consume less littoral prey fish and more pelagic zooplankton (Vander Zanden et al., 1999). Foraging on smaller prey, like zooplankton, has a higher energetic cost (Sherwood et al., 2002; Cruz-Font et al., 2019), so smallmouth bass invasion may negatively impact lake trout (Vander Zanden et al., 2004). Winter periods, when water temperatures decline and smallmouth bass reduce their activity, may therefore be crucial for increasing lake trout access to productive littoral zones (McMeans et al., 2020).
Our study builds on this previous knowledge and is the first to quantify overlap in activity rates, diet and habitat use of lake trout and smallmouth bass in all four seasons and across multiple lakes. Specifically, we ask how the overlap between lake trout and smallmouth bass in (1) habitat use, (2) activity, and (3) diet varies seasonally over two full annual cycles and in two lakes that support different food webs. We addressed our research questions using: (1) depth use and distance to shore from acoustic telemetry to determine habitat overlap, (2) movement rates from acoustic telemetry and the percent of non-empty stomachs as metrics of activity, and (3) stable isotopes of carbon (δ13C) and nitrogen (δ15N) supported by stomach contents to determine diet overlap. Because lake trout and smallmouth bass occupy different thermal guilds with distinct thermal preferences, we expected seasonal changes in niche overlap to be largely a product of different responses to seasonally changing water temperature and lake thermal structure. We expected that overlap in habitat, activity, and diet increases in the spring when both species are expected to be actively foraging in shallower, nearshore habitats. We also expected that winter, relative to summer, is a period of low activity for smallmouth bass and high littoral diet reliance for lake trout.
Materials and methods
Study system
Data were collected from two oligotrophic, dimictic north-temperate lakes that are ice covered for approximately 5 months of the year. The lakes are within Algonquin Provincial Park, Ontario (Figure 1), an area well known for lake trout angling opportunities. Lake of Two Rivers (45.5752, −78.4942) has a surface area of 306.4 ha, a maximum depth of 41.5 m and an average depth of 14.5 m (Ontario Ministry of Natural Resources and Forestry, 2022). Lake Opeongo (45.6743, −78.43756) has a surface area of 5154.2 ha, a maximum depth of 49.4 m, and an average depth of 13.7 m (Ontario Ministry of Natural Resources and Forestry, 2022). Hereafter, Lake Opeongo will be referred to as Lake 1 and Lake of Two Rivers will be referred to as Lake 2. Both lakes support self-sustaining populations of lake trout. Additionally, both lakes contain non-native smallmouth bass that have been moved outside of their natural distribution during historical stocking events. The fish communities also include brown bullhead (Ameiurus nebulosus), yellow perch (Perca flavescens), burbot (Lota lota), white sucker (Catostomus commersonii), and multiple cyprinid species. However, the fish communities differ in that Lake 1 has the pelagic prey fish, cisco (Coregonus artedii) and lake whitefish (Coregonus clupeaformis). These lakes were selected to determine if trends in seasonal resource partitioning between lake trout and smallmouth bass were consistent between lakes with different food webs. The presence of cisco combined with a larger surface area were expected to increase pelagic foraging and reduce littoral foraging of lake trout (Vander Zanden and Rasmussen, 1996; Schindler and Scheuerell, 2002) in Lake 1 compared to Lake 2.
Data collection
Water temperatures
Water temperatures were measured year-round throughout the water column. A string of data loggers (HOBO Temp Pro H20-001, Onset, Cape Cod, MA, USA) were deployed centrally in each lake. Data loggers were programmed to record temperature every 15 min and occupied depths of 1.5, 3, 4.5, 6, 7, 8, 9, 10, 11.5, 13, 20, and 27 m. Daily thermal profiles were created by calculating the mean daily temperature at each logger depth and predicting the temperature at 1 m intervals by fitting a spline to the data. Temperature data were also used to delineate seasons based on daily mean surface water temperatures (<6 m depth). Following existing telemetry studies on smallmouth bass and lake trout (Guzzo et al., 2017; McMeans et al., 2020), the period after ice-off but before mean surface temperatures exceeded 15°C was denoted as spring; summer was defined as the period during which surface temperatures were ≥15°C; fall began when lakes cooled to ≤15°C and lasted until winter, defined as ice-on to ice-off (Figure 2).
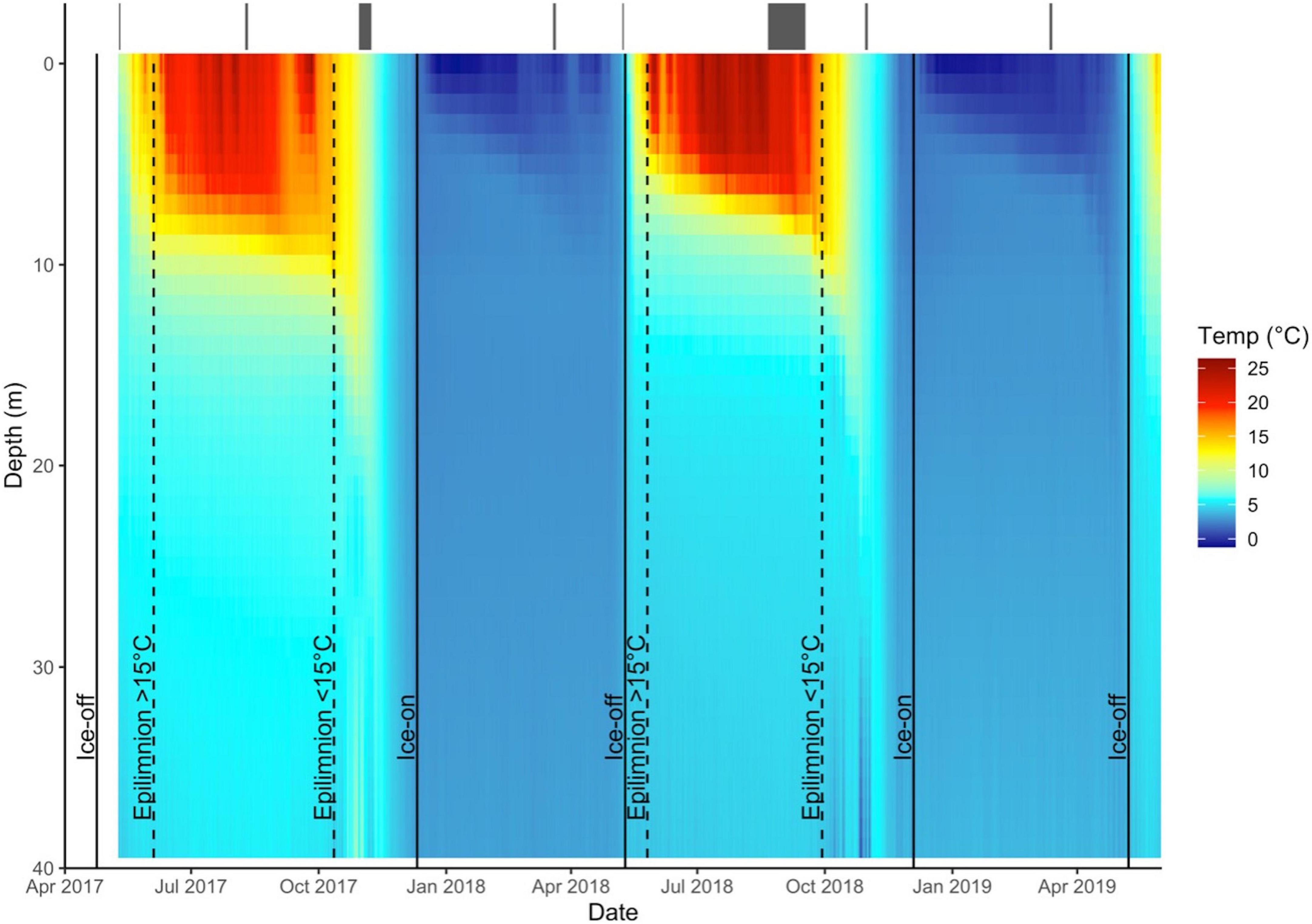
Figure 2. Thermal profile of Lake 2 (– cisco) from May 2017 to June 2019. Solid vertical lines indicate ice-on and ice-off events and dashed vertical lines indicate epilimnion water temperatures rising above or falling below 15°C. These lines delineate seasons, with spring between ice-off and water temperature greater than 15°C, summer when water temperatures are 15°C and above, fall between water temperature less than 15°C and ice-on, and winter between ice-on and ice-off. Gray bars at the top of the plot are the sampling periods in each season, spanning from the first to last day that fish were captured for stable isotope and stomach content analysis.
Acoustic telemetry
We used acoustic telemetry to examine seasonal variation in horizontal movement rates and spatial habitat overlap of lake trout and smallmouth bass. Acoustic telemetry was conducted only in Lake 2. A fine-scale positioning system was deployed in Lake 2 for the duration of the study period (spring 2017 to winter 2019). The positioning system included an array of 69 kHz VR2W acoustic receivers with co-located V16 time synchronization tags (InnovaSea Systems, Boston, MA, USA). Receivers and time synchronization tags (n = 54) were deployed at fixed locations designed to optimize acoustic coverage. A grid formation was used with a mean distance of 236 m between receivers. To assess the positioning system’s accuracy, six reference tags (V9P tags; InnovaSea Systems, Boston, MA, USA) were deployed at fixed locations within the array at depths of 5 and 18 m.
The positioning system also included acoustic transmitter tags that were surgically implanted in smallmouth bass (n = 17) and lake trout (n = 17). Transmitter tags were equipped with pressure (depth) sensors and were relatively small to minimize behavioral impacts on implanted fish (V9P tags; 9 × 31 mm, 2.8 g in water; InnovaSea Systems, Boston, MA, USA). The tags transmitted every 420 s on average, with a random acoustic transmission rate between 360 and 480 s to reduce signal collisions between tags. The estimated tag battery life was 912 days, exceeding the study duration.
Fish were captured for acoustic tagging with trap nets, gillnets, or angling gear in May 2017, September 2017, and May 2018. Following capture, fish were anaesthetized in a solution of tricaine methanesulfonate (MS-222) and placed on a surgery cradle with a maintenance dose of MS-222 flowing across their gills. Then, a surgical scalpel was used to make an incision posterior to the pectoral fin along the ventral midline, a transmitter tag was inserted into the body cavity, and the incision was stitched closed with 2–3 sutures (3-0 Monocryl synthetic absorbable sutures; Ethicon Inc., Somerville, NJ, USA). Fish total length was also recorded, scale samples were obtained, and an external T-bar anchor tag was applied. Performing the surgery and sampling was normally completed in under 3 min. Then, fish were placed in a cold-water recovery tank and their health was monitored (typically 10–15 min) before being returned to the lake.
Receiver data were processed to determine tagged fish position and depth. Receiver data were downloaded every 6 months and was sent to InnovaSea Systems (Boston, MA, USA) to determine the spatial positions (coordinates) of fish at the time of transmissions using hyperbolic positioning. Hyperbolic positioning involves converting differences in detection time between at least three receivers into differences in fish distance between receivers (Smith, 2013). The fine-scale positioning system produced 887,461 positions between May 23, 2017 and May 7, 2019, of which 63 and 37% were for lake trout and smallmouth bass, respectively. The higher percentage of positions for lake trout could be because lake trout occupy more central positions in the array (see Section “Results”), making tag transmissions more likely to be detected by ≥3 receivers (the requirement to calculate position). Each position had a corresponding depth value because pressure sensitive transmitting tags were used. The error on positions calculated from the fine-scale positioning system was assessed using the reference tags. The known position of fixed reference tags was compared to positions calculated from the fine-scale positioning system following Smith (2013). The positional error of reference tags was <6 m in over 95% of detections for reference tags at 5 m depth and over 85% of detections for reference tags at 18 m depth.
Fish and baseline sampling
Lake trout and smallmouth bass were sampled for stomach content and stable isotope analysis between the spring of 2017 and the winter of 2019, four times a year, for a total of eight sampling periods in both lakes. Fishes were captured through short sets of North American standard gill nets, trap nets, and angling. The objective was to sample 20 individuals of each species during each sampling period, however, this target was not always reached (Table 1). Lake trout were easily captured during all seasons. However, low numbers of smallmouth bass were captured during the fall and winter in each year, despite similar effort in each sampling period. Following fish capture, fish total length and sex were recorded, and livers were collected for stable isotope analysis. The stomach contents of lake trout and smallmouth bass were also identified during field sampling. Fish were identified to the species level and benthic macroinvertebrates to the order level when possible. It was also noted if stomachs were empty. Snails (Lake 1: n = 13, Lake 2: n = 15) and mussels (Lake 1: n = 13, Lake 2: n = 12) were collected to provide baseline stable isotope information for the benthic and pelagic habitats, respectively. These organisms were collected through snorkeling during the open water period. Attempts to use benthic grabs through the ice to capture snails and mussels failed. Putative prey were also collected using a variety of methods (Supplementary Note 1). Putative prey, baseline samples and liver tissue were frozen at −20°C and transferred to −80°C storage at the University of Toronto Mississauga within 2 weeks.
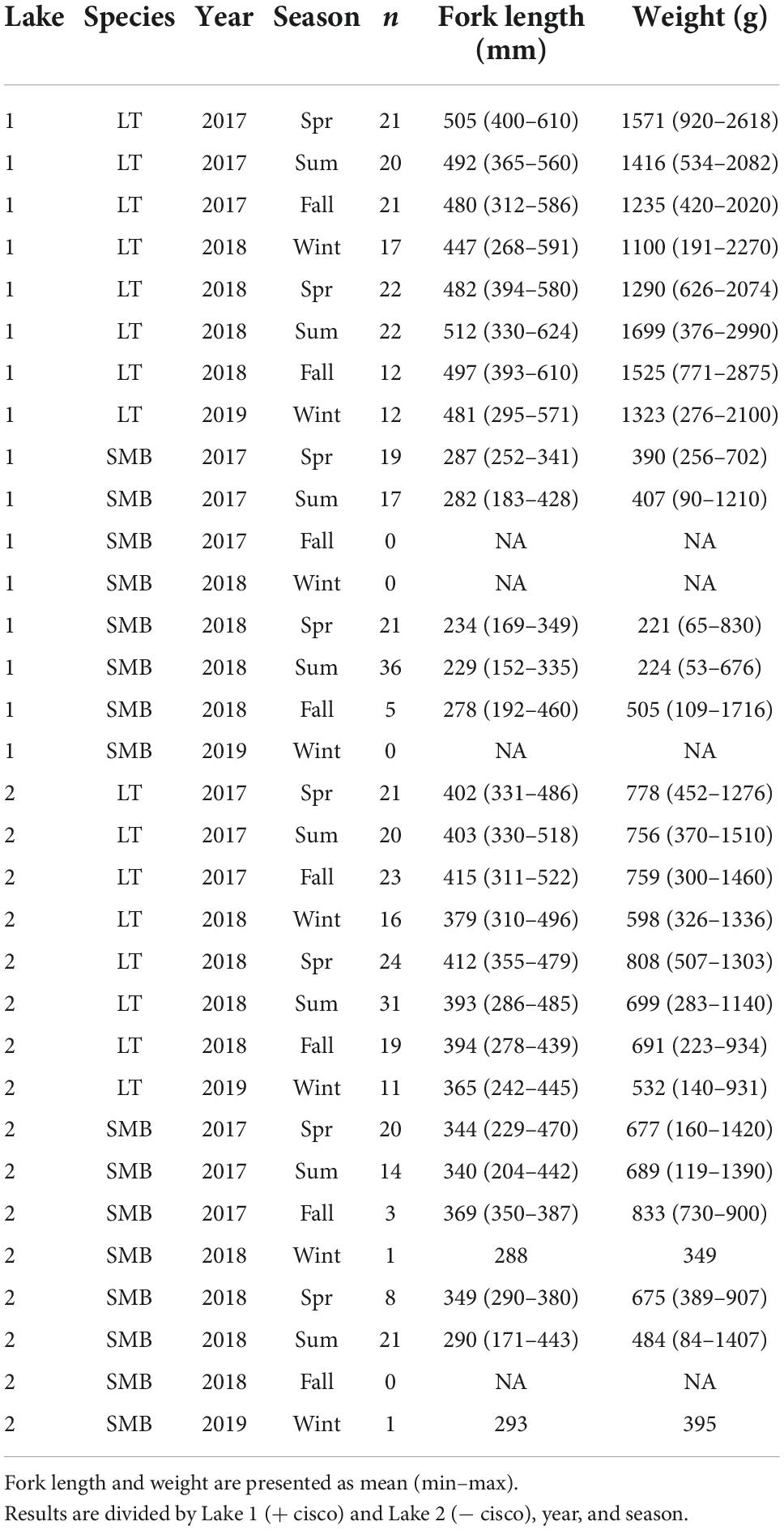
Table 1. Sample size (n) and biological data from lake trout (LT) and smallmouth bass (SMB) sampled for stomach contents and stable isotope analysis.
Stable isotopes
Stable isotopes are naturally occurring, non-decaying elements with different numbers of neutrons. The ratio of the heavy to light forms of stable isotopes in an organism’s tissue varies reliably with the isotopic signature of their energy sources (DeNiro and Epstein, 1978; Fry et al., 1978). Thus, stable isotope analysis can be used to characterize niches and determine assimilated energy sources (Boecklen et al., 2011). The ratio of 13C to 12C in a predator’s tissue closely resembles its main prey because of limited trophic enrichment (Peterson and Fry, 1987; Post, 2002). Thus, when primary producers have different carbon isotope ratios, energy can be traced from source to consumer. For example, benthic algae, the primary producer in the littoral zone, is enriched in 13C (higher ratio of 13C to 12C) compared to pelagic phytoplankton in the pelagic zone (France, 1995). Unlike carbon isotopes, the ratio of 15N to 14N increases substantially from prey to predator. Because of this trophic enrichment, nitrogen isotope ratios and can be used to estimate an organism’s trophic position. Stable isotope analysis is commonly used in ecology because the diet of a consumer is assimilated into its tissue on the timescale of months, allowing a larger window of foraging behavior to be captured than the traditional method of stomach content analysis (Wolf et al., 2009; Boecklen et al., 2011).
To prepare samples for stable isotope analysis, fish liver tissue samples and the soft tissue of snails and mussels were dehydrated for 48 h at 60°C and ground into a powder with a mortar and pestle. Subsamples of between 400 and 600 μg were enclosed in a 5 × 3.5 mm tin capsule for carbon and nitrogen stable isotope analysis. Samples were analyzed at the Fisk Lab Stable Isotope Facility, Great Lakes Institute for Environmental Research, University of Windsor. The facility uses a Costech 4010 Elemental Combustion System coupled with a Thermo Fisher Scientific Delta V Advantage Isotope Ratio Mass Spectrometer and a Thermo Fisher Scientific ConFlo IV Universal Continuous Flow Interface. Stable isotope ratios are expressed in delta (δ) notation, defined as the difference from a standard reference material in parts per thousand (‰):
The standard reference material for nitrogen is atmospheric N2 and the standard reference material for carbon is Vienna Pee Dee belemnite (V-PBD). Equipment precision was assessed through replicate analyses (n = 25) of four standard materials (NIST1577c, tilapia muscle internal standard, USGS 40 and Urea). The standard deviation of each standard material was ≤0.17‰ for δ15N and ≤0.19‰ for δ13C. Accuracy was determined by analyzing a certified standard (USGS 40) throughout sample runs. The difference between the mean of replicate analyses (n = 25) and the certified value of USGS 40 was 0.04‰ for δ15N and 0.10‰ for δ13C. The carbon to nitrogen ratio (C:N) is also determined through the stable isotope analysis process.
Stable isotope issues and considerations
There are several considerations when using stable isotopes to quantify seasonal shifts in assimilated energy sources. First, the stable isotope turnover in the tissue of focal consumers (i.e., lake trout and smallmouth bass) must be short enough to capture seasonal diet changes (Dalerum and Angerbjörn, 2005). The time it takes for a diet change to be reflected in the isotope signature of a predator’s tissue depends on the rate of tissue growth and catabolic replacement of existing tissue (Fry and Arnold, 1982). An animal’s tissue isotope turnover rate is often presented as isotope half-life, which is the number of days it takes for an animal’s tissue to be at 50% equilibrium with a new diet. The isotope half-life differs between tissues, with internal organs, like the liver, having a faster turnover time than muscle tissue (Boecklen et al., 2011). We used liver tissue instead of muscle tissue in this study because a faster turnover time is better suited for seasonal diet studies. Based on an ectotherm tissue-specific model with body mass as a variable, the isotopic half-life of liver tissue samples from a fish of average weight in our study is 52 days (Vander Zanden et al., 2015). Further, an in situ study of rainbow trout (Oncorhynchus mykiss) found that it takes ∼5–6 months for the liver tissue of a consumer to be at equilibrium with the stable isotope signature of a new diet (Skinner et al., 2017). Stable isotope turnover time may be slower in the winter than in the fall, spring, and summer because cooler water can decrease the turnover time in fish (Maitland et al., 2021). However, we did not estimate temperature or season specific stable isotope turnover because Vander Zanden et al.’s (2015) isotopic half-life model did not include temperature as a variable due to a weak effect and Skinner et al.’s (2017) study took place only during the open water period. These findings suggests that liver tissue may not fully equilibrate with the stable isotope signature of a seasonal (spring, summer, fall, and winter) diet, but should still begin to reflect seasonal diet shifts, if present. Tissue sample collection was aimed to be late enough in each season so that possible seasonal diet switches were reflected in the stable isotopes of the fish tissue. One exception is the spring, where sampling occurred close to or right at ice-out (the start of spring) because spring is a short season and provided only a limited window of opportunity for sampling. However, spring is also a period of growth and high foraging activity for lake trout (Morbey et al., 2010), potentially increasing isotopic turnover rate and the amount of spring diet integrated into the tissue of our spring sampled fish. Regardless, our spring tissue samples likely reflect the combined late winter and spring diet of the study fishes.
Second, the interpretation of energy source from δ13C can be confounded with lipid content, because lipids are depleted in 13C compared to other macromolecules (DeNiro and Epstein, 1977). Although there is debate on when it is appropriate to perform lipid correction (Arostegui et al., 2019), it is generally recommended when lipid content is high, varies between individuals, and differs between the tissue analyzed and the whole organism (Post et al., 2007; Arostegui et al., 2019). Therefore, we used the C:N ratio of each individual to perform mathematical lipid correction on the liver tissue of lake trout and smallmouth bass using the Post et al. (2007) percent lipid calculation with the Kiljunen et al. (2006) lipid normalization model. Of the commonly used mathematical correction models, these models were found to be the most accurate for correcting liver tissue when tested with taxonomically similar species (Skinner et al., 2016). All lake trout and smallmouth bass δ13C values presented and analyzed are lipid corrected.
Third, both δ13C and δ15N in consumers’ tissues can be influenced by stable isotope values of organisms at the base of the food web (Dalerum and Angerbjörn, 2005; Cobain et al., 2022). Phytoplankton are expected to become enriched in δ13C in the summer and δ15N can also be seasonally variable, due to changes in the source of C and N and isotope fractionation during uptake (O’Reilly et al., 2002; Woodland et al., 2012; Sugimoto et al., 2014). The isotope signature of lower trophic levels can also vary by lake (Cabana and Rasmussen, 1996). Baseline variation must therefore be taken into account both between lakes (Cabana and Rasmussen, 1996; Post, 2002) and between different seasons (Woodland et al., 2012; Visconti et al., 2014; Yeakel et al., 2016). Mussels (pelagic baseline) and snails (littoral baseline) were sampled from both lakes in the spring and the summer to explore isotopic variation at the base of the food web that might influence our dietary inferences. We selected unionid mussels instead of zooplankton for the pelagic baseline because mussels are recommended for correcting the stable isotope values of fish, due to their longer life and larger size, reducing short-term stable isotope variation (Cabana and Rasmussen, 1996; Post, 2002). Snails are recommended as littoral baselines for similar reasons (Post, 2002). Seasonal isotopic variation between summer and spring was minimal for both mussels and snails within each lake (Supplementary Figure 2). Therefore, we used the lake specific average δ15N value of mussels and snails as a baseline value in subsequent calculations.
Data processing and analysis
All data processing and analyses were performed in R version 4.0.2 (R Core Team, 2020).
Habitat divergence: Telemetry
Prior to analyzing the telemetry data, fish positions returned from the fine-scale positioning system were filtered to remove erroneous data. Data from seven fish (three lake trout and four smallmouth bass) were removed, as these fish were deemed dead or to have tag malfunctions. From the remaining 27 fish, ∼1.5% of the positions were removed by filtering out depths above the surface or below the bottom of the lake and positions that fell outside of the lake perimeter. After filtering, 815,148 positions (528,422 from lake trout, 286,726 from smallmouth bass) from 14 lake trout and 13 smallmouth bass remained. The mean fork lengths of the fish that were retained after filtering was 424 mm (range 326–521 mm) for lake trout and 382 mm (range 326–497 mm) for smallmouth bass.
Distance to shore and fish depth were obtained from the filtered telemetry data. A vector map of the lake was used to estimate the minimum distance to shore for each spatial position. Fish depths were already associated with each spatial position and required no additional processing. Distance to shore and fish depth are two meaningful niche axis that have been used to investigate habitat niches of fish, where depth was considered as the vertical axis and distance to shore was the horizontal axis (Guzzo et al., 2016; Versteeg et al., 2021). To calculate seasonal habitat niche overlap between lake trout and smallmouth bass, we used nicheROVER (R package; Swanson et al., 2015). Habitat niche regions for each species-season combination were created as a Bayesian ellipse. Two commonly used ellipse sizes were selected, the standard ellipses (40%) and an expanded ellipse (95%). Then, the probability of habitat overlap between the species was calculated using a Monte Carlo algorithm. Ten thousand independent and identically distributed draws were taken from the distributions of lake trout and overlap was calculated based on how many of those draws fell within the habitat niches of smallmouth bass. Habitat overlap was expressed as the probability that a random lake trout would be found in the habitat niche of smallmouth bass.
Activity divergence: Telemetry and empty stomachs
The average horizontal movement rate of lake trout and smallmouth bass, based on acoustic telemetry in Lake 2, was compared between sampling periods. The distance between successive position detections >20 min apart was divided by the time between detections to determine horizontal movement rate in m min–1. Speeds >40 m min–1 were filtered out, as this speed is faster than is likely possible (Cruz-Font et al., 2019). A total of 570,126 horizontal movement rates were obtained (383,965 from lake trout and 186,161 from smallmouth bass). Then, the mean horizontal movement rates for each species during each season-year combination of the study was calculated.
The percent of non-empty stomachs for lake trout and smallmouth bass in each sampling period was also reported to provide further insight into the species’ foraging activity. Empty stomach data is commonly used to determine patterns of food consumption (da Silveira et al., 2020), including seasonal changes in foraging activity (Block et al., 2020). Sampling periods with a higher percent of non-empty stomachs were assumed to reflect greater foraging activity than sampling periods with a lower percent of non-empty stomachs.
Diet divergence: Stable isotopes and stomach contents
Stable isotopes were first used to explore the diet overlap between lake trout and smallmouth bass. Isotopic overlap could only be compared in the spring and summer because insufficient numbers of smallmouth bass were captured in the fall and winter. Isotopic overlap was calculated using nicheROVER with δ13C (lipid corrected, see above) and δ15N data (R package; Swanson et al., 2015). We took that same approach as we did for calculating habitat niche overlap, with δ13C and δ15N instead of fish depth and distance to shore as niche axes. Isotopic overlap is expressed as the probability that a random lake trout will be found in the isotopic niche space of smallmouth bass.
To further explore seasonal trends in lake trout diet, for which we had data for all four seasons, we compared δ13C (indicating littoral vs. pelagic energy sources) and the trophic position of lake trout across the full 2-year time series (eight sampling periods). We calculated the trophic position of lake trout as:
where δ15NBaseline is the lake specific average δ15N of secondary consumers (snails and mussels). While the level of enrichment varies due to a number of factors, a trophic enrichment factor of 3.4‰ is widely accepted in freshwater systems (Post, 2002). The choice to use δ13C values instead of values converted to percent littoral reliance is explained in Supplementary Note 2.
We used linear models and pairwise comparisons of estimated marginal means to determine if there were differences in δ13C and the trophic position of lake trout between sampling periods (Searle et al., 1980; Lenth, 2020). The Tukey method (using the Studentized range distribution) was used to correct for multiple comparisons (Lenth, 2020). Trophic position and δ13C were analyzed separately, and separate linear models were used for each lake because of food web differences. Model selection was performed using backward stepwise selection, starting with the possible predictor variables of sampling period, total length, sex, and the interaction between sampling period and total length (James et al., 2013). We used p-value as the removal criterion and stopped at a p-value threshold of 0.05 (James et al., 2013).
To complement stable isotope data as an indicator of diet, we quantified the stomach contents of lake trout and smallmouth bass. While stable isotope analysis reveals time averaged assimilated energy, stomach content analysis provides a short-term taxonomically specific indicator of diet, on the scale of hours to days. We used percent frequency of occurrence, which is the percentage of stomachs with prey that contain a certain prey type. While many methods of determining the importance of each prey group exist (see reviews by Hynes, 1950; Hyslop, 1980; da Silveira et al., 2020), frequency of occurrence is a simple and robust method. Unlike bulk methods, frequency of occurrence does not suffer from bias introduced by categorizing loose digested material and is less impacted by order of prey ingestion and different rates of prey digestion (Baker et al., 2014). Prey were divided into the broad groups, “fish,” “macroinvertebrate,” “zooplankton,” and “other.” Fish were further divided to the species level and macroinvertebrates to the order level, receiving their own group if there were ≥5 occurrences or placed in the “other fish” or “other macroinvertebrate” group if there were <5 occurrences. When identification could be done to the broad grouping (fish and macroinvertebrate) but not to any higher taxonomic resolution due to digestion, the occurrence was added to the “unidentified fish” or “unidentified macroinvertebrate” category.
Results
Habitat divergence: Telemetry
Acoustic telemetry data (only available for Lake 2) reveals that the habitat of lake trout and smallmouth bass, as well as the probability of habitat overlap between the species, changes seasonally. A randomly selected lake trout has a low probability and associated credible interval of being in the habitat niche space of smallmouth bass in the summer (Table 2). The low probability of habitat overlap is driven by smallmouth bass occupying shallow positions in the water column in nearshore areas, while lake trout occupy deeper positions in the water column, generally further from shore (Figure 3). A randomly selected lake trout has a higher probability of being in the habitat niche of smallmouth bass in the winter than in the summer (Table 2). During the winter, the habitat niche of lake trout includes shallow water, in both nearshore and offshore areas, while the habitat niche of smallmouth bass is positioned slightly deeper in the water column and occupies a smaller range on the distance to shore niche axis (Figure 3). In the spring and fall, a randomly selected lake trout has the highest probability of being in the habitat niche space of smallmouth bass (Table 2). During these seasons, the niche of lake trout includes a wide range on the distances to shore axis and a position on the fish depth axis that is shallower than in the summer (Figure 3). During the spring and fall, overlap between the species occurs in the shallow fish depth and nearshore portion of lake trout’s habitat niche (Figure 3).
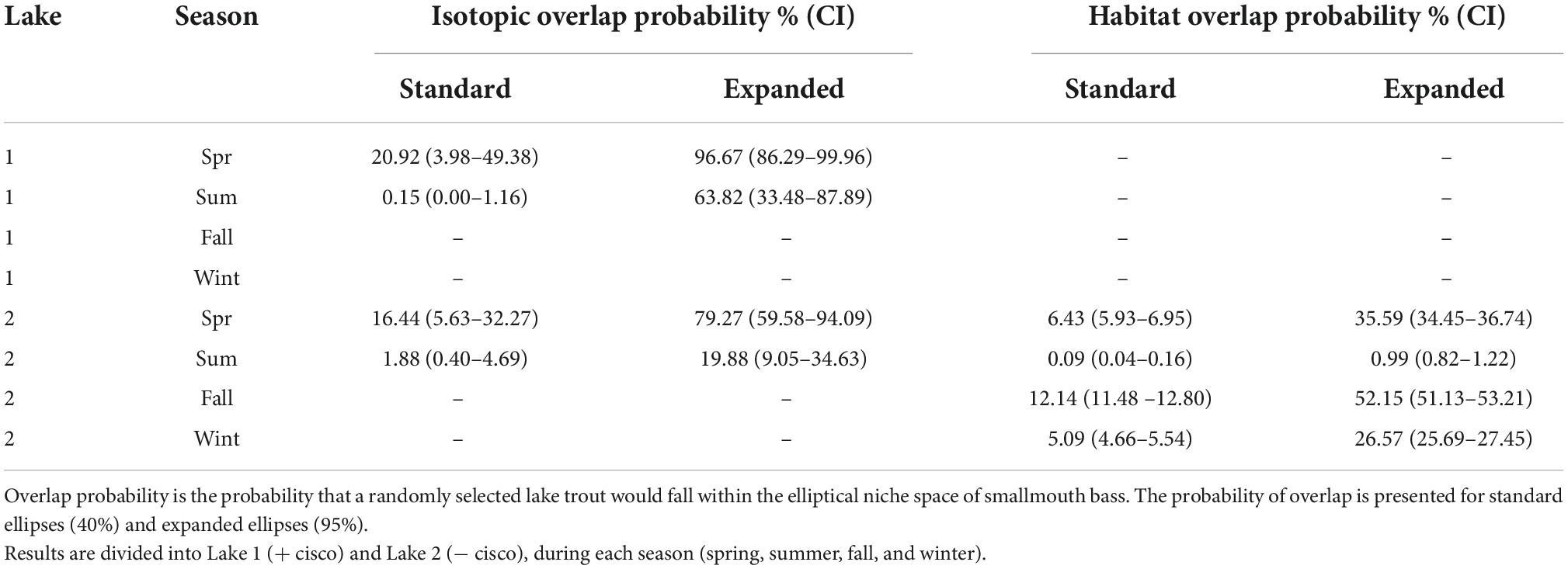
Table 2. Probability and credible interval (CI) of isotopic and habitat niche overlap between lake trout and smallmouth bass, calculated using nicheROVER (R package; Swanson et al., 2015).
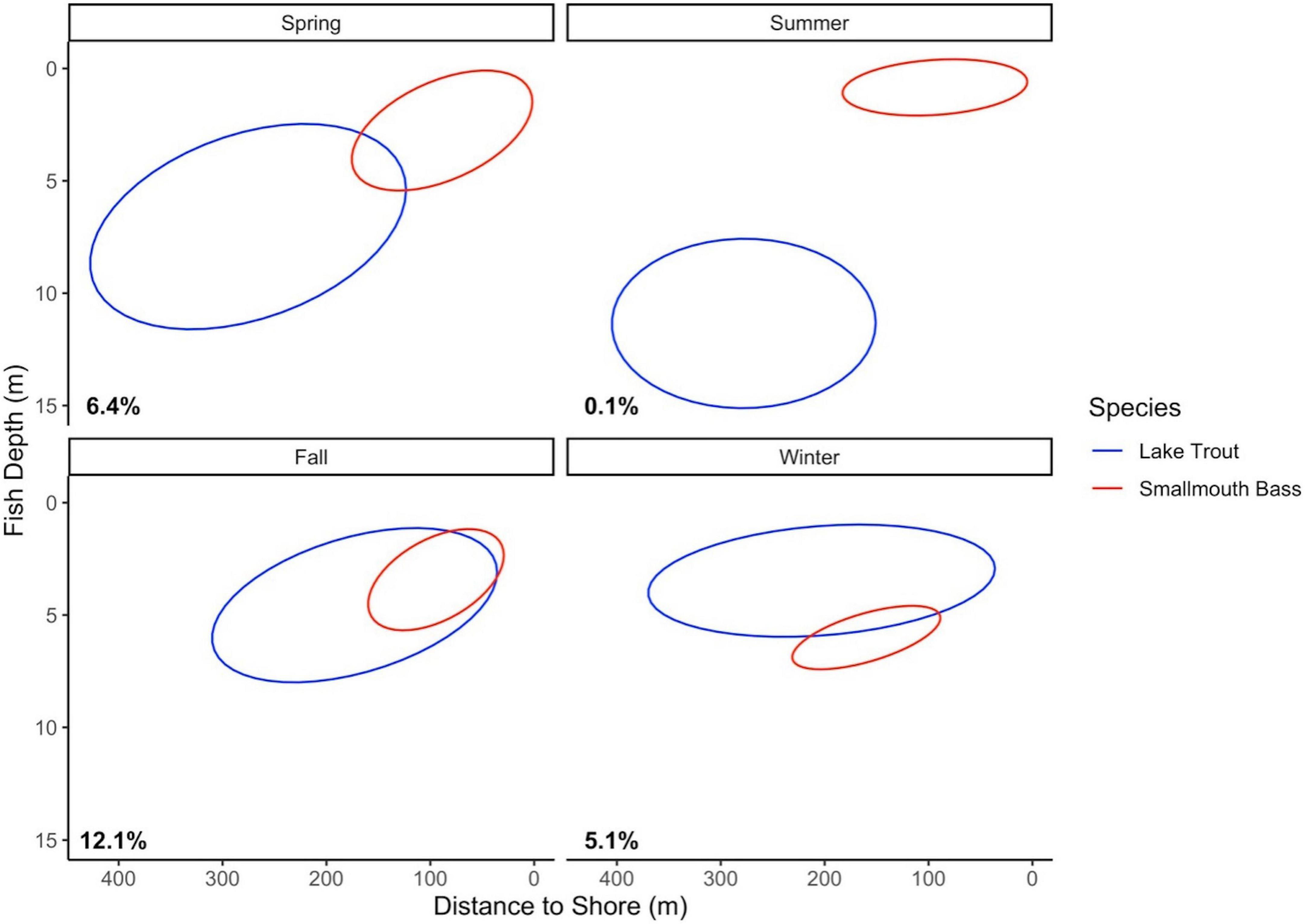
Figure 3. Habitat ellipses (40%) for lake trout (blue) and smallmouth bass (red), based on the niche axes fish depth (m) and distance to shore (m). The percent probability that a random lake trout will be in the 40% habitat niche space of smallmouth bass, calculated using the nicheROVER package, is in the lower left corner. Data were collected between spring 2017 and winter 2019 (two annual cycles) from an acoustic telemetry array in Lake 2 (– cisco). Results are separated into spring, summer, fall, and winter.
Activity divergence: Empty stomachs and telemetry
Lake trout were caught in all eight sampling periods and over 40% of stomachs sampled had contents (i.e., non-empty stomachs; Figure 4). The percent of non-empty lake trout stomachs was especially high in the spring, over 85% in each sampling period in both lakes (Figure 4). In contrast, low numbers of smallmouth bass were caught in the fall (n = 8 between both lakes and years) and winter (n = 2 between both lakes and years, Figure 4), despite similar effort across sampling periods. In addition, when smallmouth bass were caught in these seasons, there was a low percentage of stomachs with contents, except for the Fall 2017 sampling period in Lake 2 when all three smallmouth bass caught had stomach contents (Figure 4).
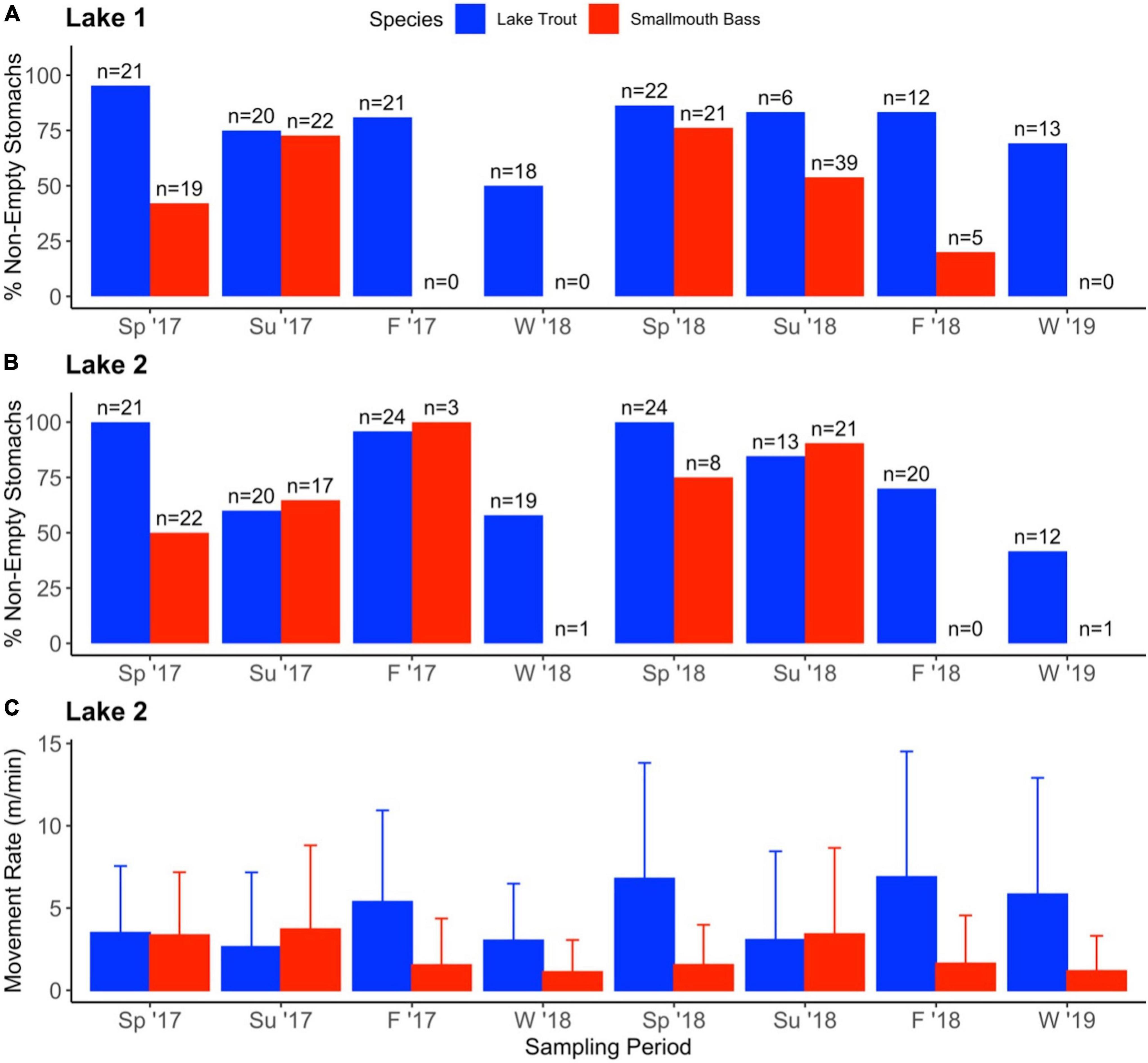
Figure 4. Activity of lake trout (blue) and smallmouth bass (red) over four seasons and 2 years. The percent of stomachs sampled that had contents in Lake 1 (+ cisco; A) and Lake 2 (– cisco; B), with the total number of fish that had stomachs sampled is denoted on top of each bar*. Mean (+ SD) seasonal movement rate of species (m/min) in Lake 2 based on acoustic telemetry data (C). *Note that more lake trout were caught in the summer 2018 sampling period than were sampled for stomach contents because the individuals were used in further study (not presented here).
Two years of movement rate data reveal distinct seasonal activity patterns for lake trout and smallmouth bass (Figure 4). The species had similar activity rates (mean ± SD) during the summer (Year 1: lake trout 2.6 m/min ± 4.5, smallmouth bass 3.7 m/min ± 5.1; Year 2: lake trout 3.1 m/min ± 5.4, smallmouth bass 3.4 m/min ± 5.2). In contrast, the mean activity rate of lake trout was higher than smallmouth bass in the fall (Year 1: lake trout 5.4 m/min ± 5.6, smallmouth bass 1.5 m/min ± 2.8; Year 2: lake trout 6.9 m/min ± 7.6, smallmouth bass 1.6 m/min ± 2.9) and the winter (Year 1: lake trout 3.0 m/min ± 3.5, smallmouth bass 1.1 m/min ± 1.9; Year 2: lake trout 5.8 m/min ± 7.1, smallmouth bass 1.2 m/min ± 2.1). In the spring of year 1, lake trout and smallmouth bass had a similar movement rate (lake trout 3.5 m/min ± 4.1, smallmouth bass 3.3 m/min ± 3.8). However, in the spring of year 2, lake trout had a higher movement rate than smallmouth bass (lake trout 6.8 m/min ± 7.0, smallmouth bass 1.5 m/min ± 2.4).
Diet divergence: Stable isotopes and stomach contents
Spring and summer
The diet overlap between lake trout and smallmouth bass was investigated in the spring and summer when both species were actively foraging and successfully captured in sufficient numbers (Table 1 and Supplementary Table 1). Based on analysis using nicheROVER with δ13C and δ15N data, a randomly selected lake trout has a higher probability of being in the isotopic niche space of smallmouth bass in the spring compared to the summer (Table 2, Figure 5). The higher probability of niche overlap was largely due to the isotope ellipse of lake trout being higher on the δ13C axis (higher littoral reliance) and the isotope ellipse of smallmouth bass being higher on the δ15N axis (higher trophic position) in the spring vs. summer (Figure 5).
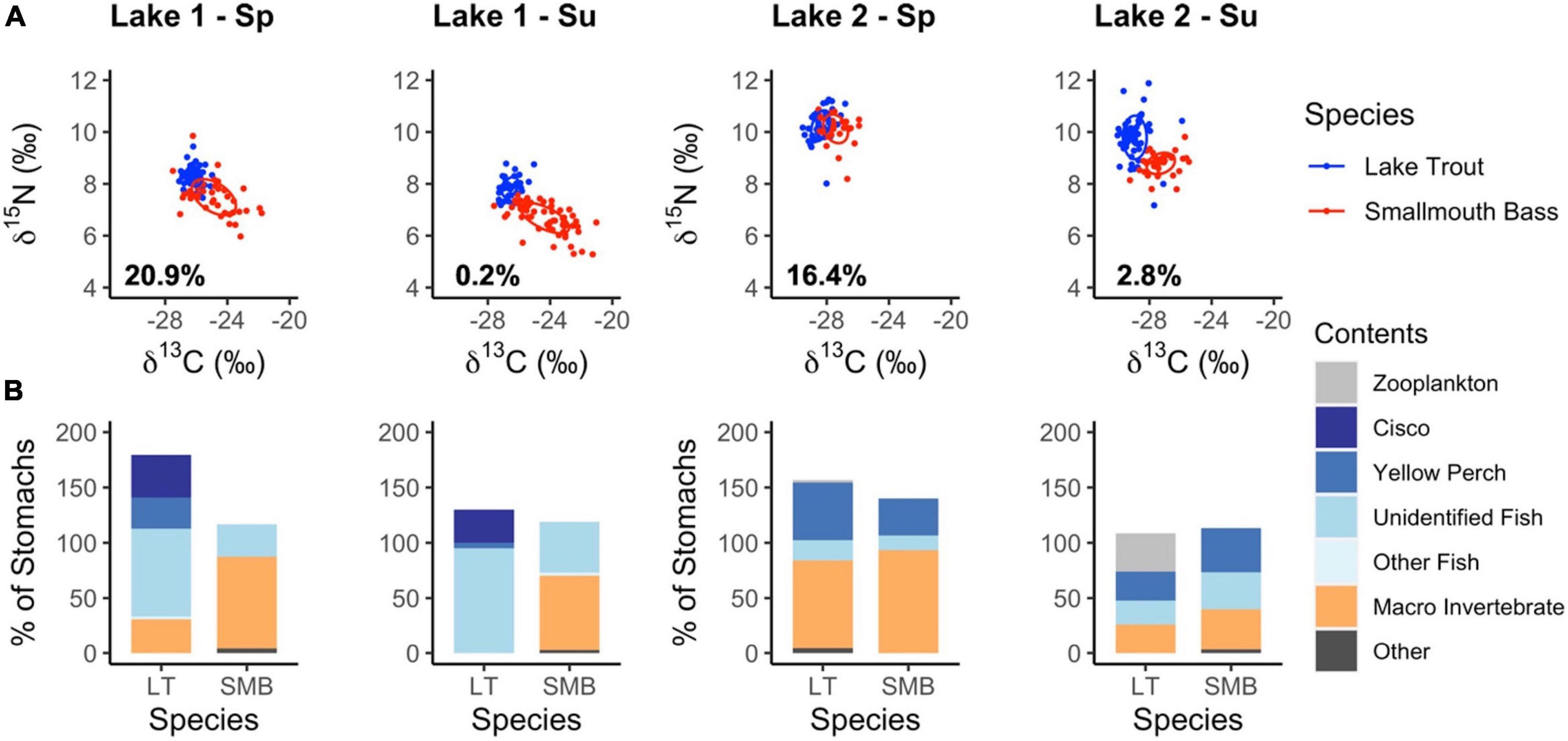
Figure 5. Isotope biplot with ellipses where individuals have a 40% probability of being found, with the percent probability that a random lake trout will be in the isotopic niche space of smallmouth bass in the lower left corner (A). The percent of non-empty lake trout and smallmouth bass stomachs that contained certain prey types (B). Results are separated into spring (Sp) and Summer (Su) and Lake 1 (+ cisco) and Lake 2 (– cisco).
The frequency of occurrence of prey items in the stomachs of lake trout and smallmouth bass support the stable isotope data, also suggesting larger dietary overlap in the spring and divergence in the summer (Figure 5). In Lake 1, both lake trout and smallmouth bass consume fish and benthic macroinvertebrates in the spring, with some observations of consuming the same taxa (Ephemeroptera, Odonata, and crayfish; Supplementary Figures 3, 4). Conversely, in the summer, lake trout were piscivorous, with most identified fish being pelagic cisco. A similar pelagic shift is not observed for smallmouth bass, which continue to feed on benthic macroinvertebrates, especially crayfish (Supplementary Figure 4). In Lake 2 during the spring, lake trout and smallmouth bass both consume yellow perch and benthic macroinvertebrates. In the summer, lake trout consume zooplankton while smallmouth bass do not. Overall, in both lakes there is more similarity in the diet of lake trout and smallmouth bass in the spring and less in the summer as lake trout increase reliance on pelagic prey (cisco in Lake 1 and zooplankton in Lake 2). Further, the higher occurrence of potentially littoral prey in lake trout stomachs during the spring is consistent with the isotope ellipses being higher on the δ13C axis. However, the isotope ellipses of smallmouth bass being higher on the δ15N axis in the spring is not consistent with the stomach content data, as macroinvertebrates that dominate the spring diet have a lower δ15N signature than fish (Supplementary Figures 5, 6).
Lake trout year-round diet
Factors included in the multiple linear regression models of lake trout trophic position based on backward selection were fish total length, sampling period, and sex. Sampling period had a significant effect on lake trout trophic position in Lake 1 (F7,139 = 22.95, p > 0.001; Supplementary Table 2) and Lake 2 (F7,157 = 11.04, p > 0.001; Supplementary Table 3). Pairwise comparisons of estimated marginal means (Supplementary Table 4) revealed that the trophic position of lake trout in Lake 1 was higher in the winter and spring than the summer (Figure 6; Supplementary Table 5). In Lake 2, pairwise comparisons of estimated marginal means (Supplementary Table 6) showed the same pattern in the first year as Lake 1, but in the second year, trophic position was not statistically significantly different between spring and summer (Figure 6; Supplementary Table 7).
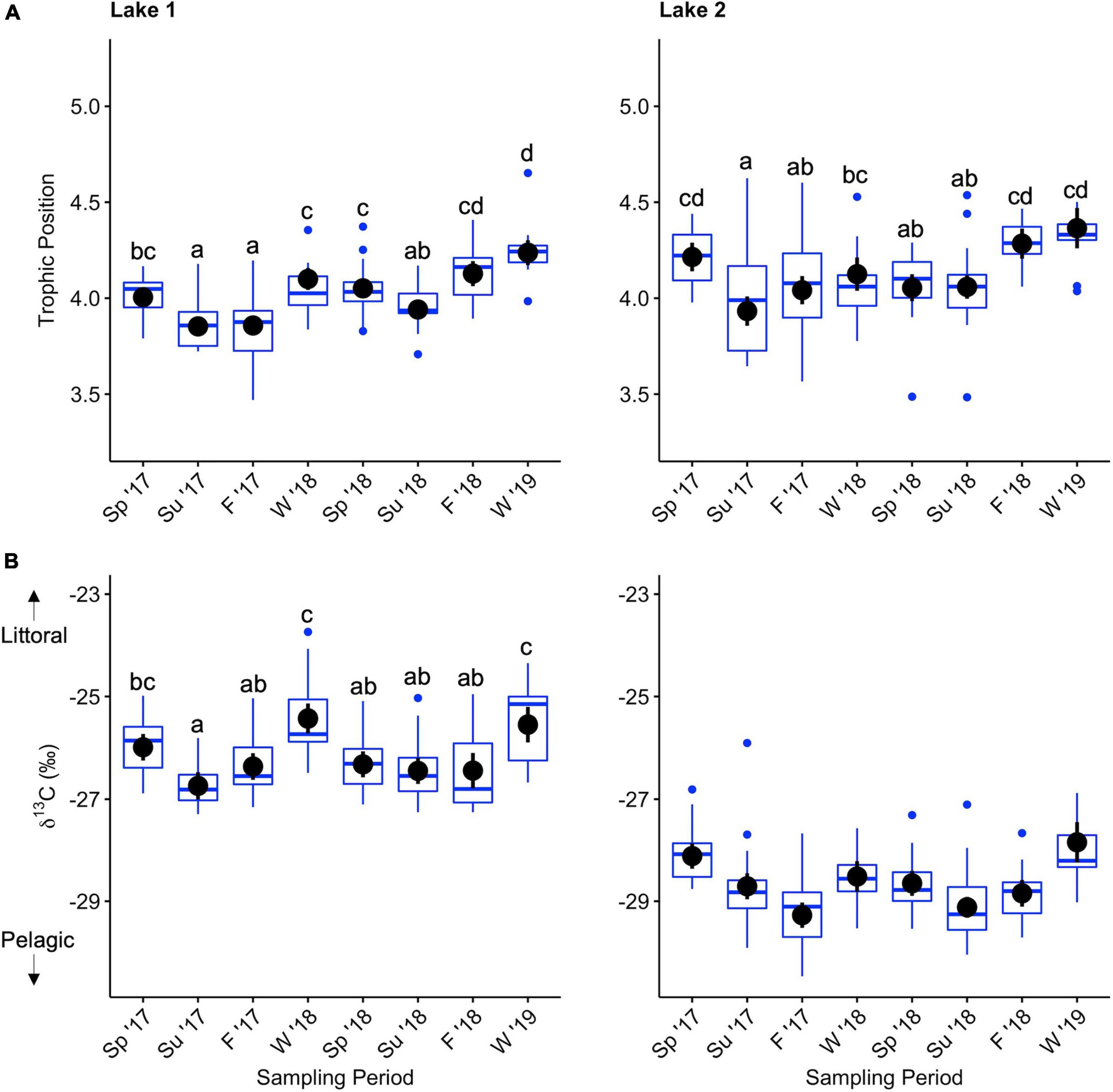
Figure 6. Boxplot of trophic position (A) and lipid corrected δ13C (B) of lake trout liver in Lake 1 (+ cisco; left) and Lake 2 (– cisco; right) over 2 years of sampling during each season. Black dots are the estimated marginal means and vertical lines through the black dots are the associated confidence intervals. Different letters indicate a significant difference in the mean values between sampling periods. Note that post-hoc tests were not performed on δ13C in Lake 2 due to an interaction between fish length and sampling period that would make results misleading.
The linear model of lake trout δ13C in Lake 1 included only sampling period, while the linear model of Lake 2 included sampling period, fish total length, and an interaction between sampling period and fish total length. Sampling period had a significant effect on the δ13C value of lake trout in Lake 1 (F7,139 = 9.77, p < 0.001; Supplementary Table 8) and Lake 2 (F7,157 = 11.54, p < 0.001; Supplementary Table 9). Pairwise comparisons of estimated marginal means (Supplementary Table 10) revealed that in Lake 1 the δ13C value of lake trout was higher (higher reliance on littoral energy) in the winter than the summer and fall in both years (Figure 6; Supplementary Table 11). In Lake 2, an interaction between fish total length and sampling period (F7,157 = 2.52, p = 0.018; Supplementary Table 9) complicates the interpretation of the main effect of sampling period. However, lake trout had a higher δ13C value in the winter than the summer, except for the largest fish sampled in the first summer (Supplementary Figure 7).
The frequency of occurrence of prey items in the stomachs of lake trout support the δ13C data and indicate a higher consumption of littoral prey in the winter than the summer. In the winter in Lake 1, benthic macroinvertebrates were found in some lake trout stomachs (Supplementary Figure 3), which can have a more littoral δ13C signature than cisco (Supplementary Figures 5, 6). In Lake 2, lake trout consumed pelagic zooplankton in the summer, which were not observed in the winter diet (Supplementary Figure 3).
Discussion
Exploring seasonal changes in the behavior of interacting species is important for understanding how climate change might alter species coexistence mechanisms. Despite their importance, seasonal studies on the behavior of interacting species, including in the field of aquatic ecology, are relatively scarce (Helland et al., 2008; McMeans et al., 2015; Sutton et al., 2021). Here, we investigated the seasonal niche overlap in habitat, activity rates, and diet between native lake trout and range-expanding smallmouth bass in north-temperate lakes. As predicted, we found that the niche overlap between lake trout and smallmouth bass changes seasonally. Isotopic and habitat overlap was higher in the spring than the summer and both species were actively foraging during these seasons. Lake trout and smallmouth bass partitioned their habitat and diet more in the summer, when lake trout remained more pelagic. Activity diverged most strongly during winter, when lake trout were actively accessing nearshore habitats and prey, while smallmouth bass were effectively inactive.
Habitat: Low habitat overlap in the summer
Our telemetry data reveal that smallmouth bass and lake trout both seasonally alter their habitats, leading to little habitat overlap in the summer and moderate habitat overlap in the spring, fall, and winter. Our use of “habitat” specifically refers to the depth and distance to shore that fish occupy. Previous studies of seasonal fish location have similarly found that fishes occupy different depths and habitat types seasonally in both lentic (Suski and Ridgway, 2009) and lotic systems (Gillette et al., 2006).
Smallmouth bass remained at shallow depths and relatively close to shore in the spring, summer, and fall, corresponding to the warmest waters available in the lake. The habitat smallmouth bass occupied was the deepest and furthest offshore in the winter, where the warmest water is available (4°C) due to reverse stratification (i.e., surface water immediately below the ice is the coldest water in the lake). Smallmouth bass have a warm thermal preference (Hasnain et al., 2018) and their habitat use follows the warmer water in the lake. This suggests that behavioral thermoregulation is a primary driver of observed seasonal movements, although factors like spring spawning could also contribute to seasonal habitat use. Other warm-water fishes, like largemouth bass, similarly change their position in the water column seasonally, with the timing of changes concurrent with changes in water temperature (Hanson et al., 2007).
Similarly, lake trout occupied distinct seasonal habitats, which may be due to their thermal preference and changing water temperatures. Water temperature is a primary determinant of suitable lake trout habitat (Plumb and Blanchfield, 2009). Lake trout’s summer habitat was offshore in deeper water because nearshore water was too warm to be occupied for extended periods of time. Summer water temperature at shallow depths (above the thermocline) exceeds 15°C, which has been defined as lake trout’s usable temperature threshold based on the habitat lake trout occupy in other Canadian Shield lakes (Plumb and Blanchfield, 2009; Guzzo and Blanchfield, 2017). In the spring, fall, and winter, when shallow water is no longer too warm to be usable for extended periods of time, lake trout extend their spatial habitat into shallower, nearshore areas in our study lakes. Lake trout’s use of nearshore habitat in the fall could also be due in part to spawning, which occurs in shallow water on cobbled shoals (Callaghan et al., 2016; Riley et al., 2019). In Algonquin Park, lake trout spawn in late October over about 10 days (Martin, 1957).
Due to these species-specific habitat changes, likely driven by changes in water temperature and distinct thermal preferences, the amount of habitat overlap between lake trout and smallmouth bass changes seasonally. Lake trout and smallmouth bass have the lowest habitat overlap in the summer when stratified water creates spatially distinct niches, with warm epilimnetic waters satisfying smallmouth bass’s thermal preference and cooler metalimnion and hypolimnion water satisfying lake trout’s thermal preference. Key ecological studies on these species’ interactions have been confined to this summer period of low habitat overlap (Vander Zanden et al., 1999, but see McMeans et al., 2020). However, we found habitat overlap is the greatest during the spring and fall, when water becomes isothermal and lake trout’s habitat is shallower and more nearshore than in the summer.
Activity: Smallmouth bass decrease activity in the fall and winter
While ectotherms like fish can change their habitat to thermoregulate, thermally optimal habitat is not always available. Changing activity rates is another strategy species use to cope with changing water temperature (Shuter et al., 2012). Reductions in movement rate offers fish a massive energy savings due to lower metabolic costs (Speers-Roesch et al., 2018).
We found that smallmouth bass were less active than lake trout in the fall and winter based on lower movement rates from telemetry data. While early studies reported that species in the warm-water thermal guild, like smallmouth bass, were dormant in the winter (Crawshaw, 1984), more recent work describes them as moving minimally and not actively feeding (Shuter et al., 2012). Our results support the more recent findings, as movement rates in the winter are lower than other seasons, but not zero. This is also consistent with previous acoustic telemetry studies on smallmouth bass, including a study in Lake 1, which found that individuals exhibited reduced vertical movement during the winter (Suski and Ridgway, 2009). Smallmouth bass also had a lower average movement rate than lake trout in the fall when water temperature was warmer than in the winter but remained below smallmouth bass’s thermal preference.
The finding of lower activity in the fall and winter based on movement rate is supported by low numbers of smallmouth bass caught and generally a greater portion of empty stomachs. Other studies support warm-water fish foraging minimally during these times. For example, warm-water pumpkinseed (Lepomis gibbosus) and bluegill (Lepomis macrochirus) had empty stomachs more frequently and lower daily energy consumption in the fall and winter compared to the spring and summer (Block et al., 2020). Overall, smallmouth bass are less active and potentially foraging less than lake trout in the fall and winter.
Diet overlap: Higher diet overlap in the spring than the summer
Our seasonally resolved study found that the isotopic niche overlap between lake trout and smallmouth bass is higher in the spring than the summer in both lakes. Consistent with the stable isotope data, the diet of lake trout and smallmouth bass is more similar in the spring than the summer, as both species consume macroinvertebrates more frequently. Increased consumption of macroinvertebrates by lake trout and smallmouth bass in the spring could be due to insect emergences. For example, chironomid pupae emerge and float to the surface in the spring. Ephemeroptera emergence also peaks in the spring (May and June), although some species have a second peak in the late summer (Rowe and Berrill, 1989). The isotopic niche of lake trout was higher on the δ13C axis in the spring, as is expected with the increased consumption of potentially littoral benthic macroinvertebrates. In contrast, the isotopic niche of smallmouth bass was higher on the δ15N axis in the spring than the summer. Despite no increase in the frequency of occurrence of higher trophic position prey (e.g., fish) in spring vs. summer smallmouth bass stomachs, the amount of assimilated energy from higher trophic position prey may have increased. However, this speculation requires more detailed stomach content and energetic analysis. A physiological explanation is also possible. Periods of low food intake can cause protein reserves in the body to be used, leading to 14N being preferentially excreted and a δ15N enrichment in body tissues (Doi et al., 2017). Thus, the residual effects of limited foraging by smallmouth bass in the winter could have caused the enriched δ15N we observed in the spring.
Previous studies on the impact of smallmouth bass on lake trout’s diet suggest that these species can compete for nearshore prey. In lakes without smallmouth bass, lake trout, on average, have a higher reliance on littoral energy (Vander Zanden et al., 1999). Additionally, after smallmouth bass were removed from a north-temperate lake, lake trout increased their consumption of littoral prey and had a corresponding increase in their reliance on littoral energy (Lepak et al., 2006). Our finding of higher isotopic niche overlap and a more similar diet between lake trout and smallmouth bass in the spring than the summer suggests that potential consumptive competition between the species could be more pronounced in the spring.
Lake effects on seasonal diet overlap
Temperate lakes vastly differ in size, shape, depth, and community composition, potentially impacting food web structure and species interactions. In smaller lakes, mobile top predators like lake trout increase their foraging in the littoral zone (Schindler and Scheuerell, 2002; Tunney et al., 2012). In lakes that lack pelagic prey fish, littoral foraging is also strengthened because lake trout must rely more on littoral food sources (Vander Zanden and Rasmussen, 1996). Variation in lake characteristics that affect the extent of littoral foraging by lake trout may impact diet overlap with nearshore smallmouth bass. We expected lake trout to rely more on littoral prey and have a higher diet overlap with smallmouth bass in the spring and summer in the smaller lake that lacks an offshore forage fish (Lake 2) vs. the larger lake with pelagic cisco (Lake 1).
Counter to our prediction, the probability of lake trout being in the isotopic niche space of smallmouth bass was higher in Lake 1 than Lake 2, in both the spring and summer. The smaller isotopic niche size of lake trout in Lake 1 (with cisco) could be a contributing factor to this surprising finding, because for a set area of niche overlap in isotope space, the probability of overlap will increase with decreasing niche size. The dominance of yellow perch in the diet of lake trout in Lake 2 (no cisco) is also a possible explanation. We found lake trout consumed both young of the year and adult yellow perch. In another Algonquin Park lake without cisco, 80% of yellow perch consumed by lake trout were young of the year (Martin, 1952). Yellow perch consume zooplankton as juveniles (Brown et al., 2009) and the carbon stable isotope signature of young of the year yellow perch in our study is consistent with other pelagic prey (Supplementary Figures 5, 6). Therefore, the consumption of young of the year yellow perch with a pelagic stable isotope signature, occupying an analogous position to cisco, could reduce the isotopic niche overlap with smallmouth bass in Lake 2.
Seasonal lake trout diet: Lake trout occupy a higher trophic position and obtain more littoral energy in the winter than the summer
Stable isotope results supported by stomach contents data were explored year-round for lake trout to quantify if and how lake trout diet changed during the winter when smallmouth bass were largely inactive. We found lake trout generally had a higher δ13C value and occupied a significantly higher trophic position in the winter compared to the summer in both lakes. A higher δ13C value indicates that more of lake trout’s energy ultimately comes from the nearshore environment, either from directly preying on nearshore prey or by consuming prey that have consumed nearshore prey themselves. These stable isotope results are consistent with stomach content data. Lake trout’s diet in Lake 1 is dominated by pelagic cisco, but benthic macroinvertebrates are present in the winter and yellow perch were found more frequently in the winter than the summer. Adult yellow perch could contribute to the higher trophic position, based on their ecology and stable isotope signature in Lake 1. Benthic macroinvertebrates, like larval insects, could contribute to the more nearshore signal in the winter in Lake 1 because they mainly occupy the littoral zone (Koroiva and Pepinelli, 2019). However, they can also be found in profundal benthic habitat (e.g., some Diptera larvae), and we did not extensively characterize the stable isotope ecology of larval insects in our study lakes. In the winter in Lake 2, zooplankton, a taxon with a strong pelagic signal and low trophic position, were not detected but higher trophic position and potentially more littoral yellow perch were.
The observed changes in the stable isotope signature and diet of lake trout may align with seasonal changes in prey availability, including pulses in prey abundance, combined with changes in prey thermal accessibility. For example, zooplankton generally have a seasonal pulse of availability in the summer (Sommer et al., 1986; Jensen, 2019), coinciding with when they are detected in lake trout’s diet. In addition, nearshore prey with a more littoral stable isotope signature, are more thermally accessible to lake trout in the winter than the summer. Although lake trout can access the littoral prey by making short forays in summer (Morbey et al., 2006; Plumb et al., 2014; Guzzo et al., 2017), these forays decrease the time lake trout spend at rest, so are not as energetically advantageous as consuming prey that are thermally accessible (Cruz-Font et al., 2019). In addition to the nearshore area being more thermally accessible during the winter, smallmouth bass are largely inactive and thus likely not consumptively drawing down nearshore resources like in the summer (Vander Zanden et al., 1999).
Overall, we find that during the winter, a period of low activity for smallmouth bass, lake trout have a higher trophic position and obtain more energy from the littoral pathway. Lake trout populations that feed on larger higher trophic position organisms (e.g., fish vs. zooplankton) use less energy for foraging, leading to higher growth efficiency and larger adult body sizes (Pazzia et al., 2002; Shuter et al., 2016; Cruz-Font et al., 2019). Other fishes similarly change their diet in the winter to prey that are energetically advantageous (Eloranta et al., 2013; Stockwell et al., 2014). In addition, lake trout’s C:N ratio in the liver was generally high in the winter, suggesting that lipids in the liver were not depleted by winter conditions. Thus, winter diet switches could be beneficial for lake trout, but this idea requires further study.
Caveats and future directions
Predator stable isotope signatures not only depend on their own habitat and diet, but on the habitat and diet of their prey. Therefore, considering the seasonal ecology of prey species is important for understanding the stable isotope signature of top predators like lake trout and smallmouth bass. For example, cisco biomass increases nearshore in the late fall, as fish congregate before spawning (Yule et al., 2009). Cisco can also be found in shallow waters in the winter and spring, and while they primarily consume zooplankton, their diet also includes larval insects, crustaceans, and algae (Holm et al., 2009). If cisco feed on more nearshore prey during the winter in our study lakes, this could contribute to the increased littoral stable isotope signal of Lake 1 lake trout in the winter, along with the increased consumption of invertebrates based on stomach contents. Yellow perch were also commonly consumed by both lake trout and smallmouth bass, and yellow perch diet can change seasonally (Block et al., 2020; Duxbury, 2020). For example, yellow perch in Lake Champlain switched their diet from fish and benthic macroinvertebrates in the winter to zooplankton in the summer (Block et al., 2020). We lack data about the seasonal ecology of prey in our study lakes and in general little is known about the seasonal ecology of many prey fish species. Further study of prey fish abundance, habitat, and diet and how these factors change through time would improve our understanding of top predator seasonal niches (Turschak et al., 2022).
There are some potential biases in the stomach content data. It is possible that zooplankton and other small organisms are underrepresented in the diet, as small prey (higher surface area to volume ratio) are digested more quickly than larger prey (Legler et al., 2010). Other organisms may be overrepresented because of features like exoskeletons that slow down digestion (Kionka and Windell, 1972). Stomachs could be found empty less often and contain more prey types in the winter because rates of digestion are slower at colder temperatures (Legler et al., 2010). In addition, the frequency of occurrence of prey may not be representative of assimilated energy because prey types vary in their biomass and energy content. Importantly, stomach contents are only a snapshot of diet, so they can miss temporally constrained but potentially energetically important items (Fernandes and McMeans, 2021). All tools have limitations, which stresses the importance of combining multiple ecological tools (here, stable isotopes, stomach contents and telemetry).
While we quantified seasonal niche overlap, future studies should investigate the impacts of smallmouth bass invasion on lake trout. Previous studies have shown that in the summer, smallmouth bass reduce lake trout’s consumption of nearshore prey in lakes without cisco (Vander Zanden et al., 1999). Based on our finding of high diet overlap in the spring in lakes with and without cisco, future studies should include both lake types and be seasonally resolved. A high C:N ratio (Supplementary Figure 1) and large size of adult lake trout in Lake 1 compared to lakes without pelagic prey fish (Pazzia et al., 2002), indicates that cisco could buffer the impacts of smallmouth bass invasion on lake trout, as previously suggested (Vander Zanden et al., 2004; Sharma et al., 2009). Studies on the impact of smallmouth bass could include comparing lake trout diet and other factors like abundance (Larocque et al., 2021), bioenergetics, and growth rates in lakes with and without smallmouth bass, throughout an invasion, or through a historical reconstruction (e.g., Morbey et al., 2007).
Lake trout had a higher pelagic reliance and trophic position in Lake 2 (no cisco) than expected. Both results might be explained through the consumption of adult yellow perch and planktivorous young of the year yellow perch based on their stable isotope signature. Yellow perch have a higher δ15N (trophic position) than other available prey (zooplankton and benthic macroinvertebrates) and young of the year yellow perch have a low (pelagic) δ13C value (Supplementary Figure 5). However, we can only speculate about which diet items caused the observed stable isotope signature based on stomach contents and visually inspecting the stable isotope signatures of predators and prey. Future work should use stable isotope mixing models to determine the specific contributions of each prey type to the overall isotope signature.
Finally, our finding that lake trout trophic positions are higher in the winter than the summer has implication for contaminant modeling. Organisms with a higher trophic position, including lake trout, generally have higher contaminant loads due to bioaccumulation (Vander Zanden and Rasmussen, 1996). Therefore, contaminant models that use trophic positions determined through summer sampling only may underestimate the concentration of contaminants, such as mercury, in lake trout and the human health risks of consumption. Further work is required to explore this idea and seasonal contaminant concentrations overall.
Conclusion and implications
(1) Lake trout and smallmouth bass had a higher isotope and habitat overlap in the spring than the summer. Smallmouth bass also had low activity rates in the fall and winter based on acoustic telemetry in Lake 2, suggesting potential competition is the highest in the spring. Spring is an important time for lake trout to forage as it is the season with the highest energy allocation to growth (Morbey et al., 2010). Increases in air temperature due to climate change in small Boreal Shield lakes may lengthen spring, defined as the period between ice-off and when surface water temperatures reach 15°C (Guzzo and Blanchfield, 2017). While longer access to the littoral area without thermal consequence may be beneficial for lake trout, the impact of a potentially longer period of heightened isotope and habitat overlap with smallmouth bass is unknown.
(2) The isotope overlap and diet similarity between lake trout and smallmouth bass was higher in the spring than the summer in both a lake with and a lake without a pelagic prey fish (i.e., cisco). Based on a previous summer study (Vander Zanden et al., 1999), lake trout in lakes with pelagic prey fish have been classified as not vulnerable to the impacts of smallmouth bass invasion (Sharma et al., 2009). We found high lipid levels in lake trout inhabiting lakes with cisco, potentially supporting their buffering effect against the negative impacts of smallmouth bass. However, the impact of smallmouth bass on lake trout diet and bioenergetics in the spring is unknown but potentially negative given our finding of niche overlap. We suggest that the classification of lake trout lakes with cisco as not vulnerable to the impacts of smallmouth bass invasion should be re-examined using a seasonal perspective.
(3) Our paper supports temporal-mediated coexistence occurring between fishes in different thermal guilds because our study species partitioned a shared resource by accessing it at different times (Chesson, 2000). Physiological traits, such as thermal preference, can drive the different responses to winter that underpin coexistence in freshwater fishes (McMeans et al., 2020). In this study, cold-water lake trout altered their diet and habitat in the winter, with more of their energy coming from the nearshore area in the winter than the summer. In contrast, warm-water smallmouth bass reduced their activity in the winter, decoupling themselves from the nearshore prey they depend on during the summer. Overall, lake trout and smallmouth bass’s different responses to cold winters leads to nearshore diet items being partitioned in time (only lake trout access nearshore prey in the winter). Therefore, shorter winters (defined as the period of ice cover) due by climate change (Woolway et al., 2020), may impact coexistence between lake trout and smallmouth bass and between other species in different thermal guilds. Although coexistence mechanisms involve resource partitioning, resource partitioning alone does not guarantee stable species coexistence (Chesson, 2000). To determine if coexistence is stable, future studies should investigate long-term population growth rates and the strength of intraspecific vs. interspecific competition.
(4) Our temporally and spatially resolved study provides an example of how the niches of coexisting species in different thermal guilds are partitioned along multiple niche axes. Niche partitioning was likely driven by different responses to seasonally changing water temperatures. Spatial axes, like diet (consuming littoral or pelagic prey) and habitat (depth and distance to shore) diverged most in the summer, when stratified water creates thermally distinct volumes within lakes. The temporal axis (activity), diverged most in the winter, possibly driven by cold temperatures. Our work suggests that conducting field work exclusively in the summer, as is common practice, can lead to an incomplete understanding of species interactions. Conclusions based on summer work alone, may underestimate the strength of interactions between species in different thermal guilds. Although our study focused on smallmouth bass and lake trout, and centered largely around temperature variation, the findings are broadly applicable to other potential competitors and seasonally variable conditions (e.g., light, dissolved oxygen, resource density). We recommend that a seasonal perspective is also adopted in future studies, to continue to bridge the knowledge gap between species ecology in the summer and other seasons. This improved understanding is increasingly important as the range of warm water species is shifting northward due to climate change, increasing the geographic overlap of warm and cold and cool water species (Sharma et al., 2007).
Data availability statement
The raw data supporting the conclusions of this article will be made available by the authors, without undue reservation.
Ethics statement
The animal study was reviewed and approved by University of Toronto Biological Sciences Local Animal Care Committee.
Author contributions
EB, BM, and MR conceived the study idea. EB and BM processed the stable isotope samples and drafted the original manuscript. MR conducted the telemetry field work. MG and TM managed and processed the telemetry and temperature data. EB curated stomach content data. EB and MG performed the statistical analyses. EB, MG, and TM drafted the figures and tables. MG, TM, and MR provided the feedback and edits. All authors contributed to the article and approved the submitted version.
Funding
EB was supported by a Canada Graduate Scholarship–Master’s from the Natural Sciences and Engineering Research Council of Canada and the University of Toronto School of Graduate Studies. BM was funded by a Natural Sciences and Engineering Research Council of Canada Discovery Grant (RGPIN-2017-06794). MG was funded by the Natural Sciences and Engineering Research Council of Canada and The W. Garfield Weston Foundation.
Acknowledgments
We are grateful to Brian Shuter, Cindy Chu, Shannon McCauley, and reviewers BM and DG-R for comments that have improved this manuscript. We thank Tim Fernandes, Nick Lacombe, Krystal Mitchell, Courtney Taylor, Sofia Pereira, and Shazreh Salam for assistance in the field and laboratory.
Conflict of interest
The authors declare that the research was conducted in the absence of any commercial or financial relationships that could be construed as a potential conflict of interest.
Publisher’s note
All claims expressed in this article are solely those of the authors and do not necessarily represent those of their affiliated organizations, or those of the publisher, the editors and the reviewers. Any product that may be evaluated in this article, or claim that may be made by its manufacturer, is not guaranteed or endorsed by the publisher.
Supplementary material
The Supplementary Material for this article can be found online at: https://www.frontiersin.org/articles/10.3389/fevo.2022.986459/full#supplementary-material
References
Angert, A. L., Huxman, T. E., Chesson, P., and Venable, L. D. (2009). Functional tradeoffs determine species coexistence via the storage effect. Proc. Natl. Acad. Sci. U.S.A. 106, 11641–11645.
Arostegui, M. C., Schindler, D. E., and Holtgrieve, G. W. (2019). Does lipid-correction introduce biases into isotopic mixing models? Implications for diet reconstruction studies. Oecologia 191, 745–755. doi: 10.1007/s00442-019-04525-7
Baker, R., Buckland, A., and Sheaves, M. (2014). Fish gut content analysis: Robust measures of diet composition. Fish Fish. 15, 170–177. doi: 10.1111/faf.12026
Beitinger, T. L., and Fitzpatrick, L. C. (1979). Physiological and ecological correlates of preferred temperature in fish. Am. Zool. 19, 319–329.
Benoit, D. M., Jackson, D. A., and Chu, C. (2021). Partitioning fish communities into guilds for ecological analyses: An overview of current approaches and future directions. Can. J. Fish. Aquat. Sci. 78, 984–993. doi: 10.1139/cjfas-2020-0455
Benson, B. J., Magnuson, J. J., Jensen, O. P., Card, V. M., Hodgkins, G., Korhonen, J., et al. (2012). Extreme events, trends, and variability in Northern Hemisphere lake-ice phenology (1855-2005). Clim. Change 112, 299–323. doi: 10.1007/s10584-011-0212-8
Block, B. D., Denfeld, B. A., Stockwell, J. D., Flaim, G., Grossart, H. P. F., Knoll, L. B., et al. (2019). The unique methodological challenges of winter limnology. Limnol. Oceanogr. Methods 17, 42–57. doi: 10.1002/lom3.10295
Block, B. D., Stockwell, J. D., and Marsden, J. E. (2020). Contributions of winter foraging to the annual growth of thermally dissimilar fish species. Hydrobiologia 847, 4325–4341. doi: 10.1007/s10750-020-04428-2
Boecklen, W. J., Yarnes, C. T., Cook, B. A., and James, A. C. (2011). On the use of stable isotopes in trophic ecology. Annu. Rev. Ecol. Evol. Syst. 42, 411–440. doi: 10.1146/annurev-ecolsys-102209-144726
Brett, J. R. (1971). Energetic responses of salmon to temperature. A study of some thermal relations in the physiology and freshwater ecology of sockeye salmon (Oncorhynchus nerka). Am. Zool. 11:99–113.
Brown, T. G., Runciman, B., Bradford, M. J., and Pollard, S. (2009). A biological synopsis of yellow perch (Perca flavescens). Can. Manuscr. Rep. Fish. Aquat. Sci. 2883, 1–28.
Cabana, G., and Rasmussen, J. B. (1996). Comparison of aquatic food chains using nitrogen isotopes. Proc. Natl. Acad. Sci. U.S.A. 93, 10844–10847.
Callaghan, D. T., Blanchfield, P. J., and Cott, P. A. (2016). Lake trout (Salvelinus namaycush) spawning habitat in a northern lake: The role of wind and physical characteristics on habitat quality. J. Great Lakes Res. 42, 299–307. doi: 10.1016/j.jglr.2015.07.001
Chesson, P. (2000). Mechanisms of maintenance of species diversity. Annu. Rev. Ecol. Syst. 31, 343–366. doi: 10.1146/annurev.ecolsys.31.1.343
Chesson, P., and Huntly, N. (1997). The roles of harsh and fluctuating conditions in the dynamics of ecological communities. Am. Nat. 150, 519–553. doi: 10.1086/286080
Cobain, M. R. D., McGill, R. A. R., and Trueman, C. N. (2022). Stable isotopes demonstrate seasonally stable benthic-pelagic coupling as newly fixed nutrients are rapidly transferred through food chains in an estuarine fish community. J. Fish Biol. 1–15. doi: 10.1111/jfb.15005
Connell, J. H. (1961). The influence of interspecific competition and other factors on the distribution of the barnacle Chthamalus stellatus. Ecology 42, 710–723. doi: 10.2307/1933500
Crawshaw, L. I. (1984). Low-temperature dormancy in fish. Am. J. Physiol. 246, 479–486. doi: 10.1152/ajpregu.1984.246.4.R479
Cruz-Font, L., Shuter, B. J., Blanchfield, P. J., Minns, C. K., and Rennie, M. D. (2019). Life at the top: Lake ecotype influences the foraging pattern, metabolic costs and life history of an apex fish predator. J. Anim. Ecol. 88, 702–716. doi: 10.1111/1365-2656.12956
da Silveira, E. L., Semmar, N., Cartes, J. E., Tuset, V. M., Lombarte, A., Ballester, E. L. C., et al. (2020). Methods for trophic ecology assessment in rishes: A critical review of stomach analyses. Rev. Fish. Sci. Aquac. 28, 71–106. doi: 10.1080/23308249.2019.1678013
Dalerum, F., and Angerbjörn, A. (2005). Resolving temporal variation in vertebrate diets using naturally occurring stable isotopes. Oecologia 144, 647–658. doi: 10.1007/s00442-005-0118-0
DeNiro, M. J., and Epstein, S. (1977). Mechanism of carbon isotope fractionation associated with lipid synthesis. Science 197, 261–263.
DeNiro, M. J., and Epstein, S. (1978). Influence of diet on the distriution of carbon isotopes in animals. Ecology 42, 495–506. doi: 10.1002/mop.25285
Doi, H., Akamatsu, F., and González, A. L. (2017). Starvation effects on nitrogen and carbon stable isotopes of animals: An insight from meta-analysis of fasting experiments. R. Soc. Open Sci. 4:170633. doi: 10.1098/rsos.170633
Duxbury, B. (2020). The effect of seasonality on yellow perch ecology and ecotoxicology within Lake Manganese In Biological Science. [master’s thesis]. Houghton MI: Michigan Technological University.
Eloranta, A. P., Mariash, H. L., Rautio, M., and Power, M. (2013). Lipid-rich zooplankton subsidise the winter diet of benthivorous Arctic charr (Salvelinus alpinus) in a subarctic lake. Freshw. Biol. 58, 2541–2554. doi: 10.1111/fwb.12231
Fernandes, T., and McMeans, B. C. (2021). Spotty at best: Brook trout exploit large, adult spotted salamanders in the early spring. Ecology 102, 1–4. doi: 10.1002/ecy.3202
France, R. L. (1995). Differentiation between littoral and pelagic food webs in lakes using stable carbon isotopes. Limnol. Oceanogr. 40, 1310–1313. doi: 10.4319/lo.1995.40.7.1310
Fry, B., and Arnold, C. (1982). Rapid 13C/12C turnover during growth of brown shrimp (Penaeus aztecus). Oecologia 54, 200–204. doi: 10.1007/BF00378393
Fry, B., Joern, A., and Parker, P. L. (1978). Grasshopper food web analysis: Use of carbon isotope ratios to examine feeding relationships among terrestrial herbivores. Ecology 59, 498–506.
Fry, F. E. J. (1947). Effects of the environment on animal activity. Univ. Toronto Stud. Biol. Ser. 55, 1–62.
Gause, G. F. (1934). Experimental analysis of Vito Volterra’s mathematical theory of the struggle for existence. Science 79:1617.
Gillette, D. P., Tiemann, J. S., Edds, D. R., and Wildhaber, M. L. (2006). Habitat use by a Midwestern U.S.A. riverine fish assemblage: Effects of season, water temperature and river discharge. J. Fish Biol. 68, 1494–1512. doi: 10.1111/j.0022-1112.2006.001037.x
Goyer, K., Bertolo, A., Pépino, M., and Magnan, P. (2014). Effects of lake warming on behavioural thermoregulatory tactics in a cold-water stenothermic fish. PLoS One 9:e92514. doi: 10.1371/journal.pone.0092514
Guzzo, M. M., and Blanchfield, P. J. (2017). Climate change alters the quantity and phenology of habitat for lake trout (Salvelinus namaycush) in small Boreal Shield lakes. Can. J. Fish. Aquat. Sci. 74, 871–884. doi: 10.1139/cjfas-2016-0190
Guzzo, M. M., Blanchfield, P. J., and Rennie, M. D. (2017). Behavioral responses to annual temperature variation alter the dominant energy pathway, growth, and condition of a cold-water predator. Proc. Natl. Acad. Sci. U.S.A. 114, 9912–9917. doi: 10.1073/pnas.1702584114
Guzzo, M. M., Blanchfield, P. J., Chapelsky, A. J., and Cott, P. A. (2016). Resource partitioning among top-level piscivores in a sub-Arctic lake during thermal stratification. J. Great Lakes Res. 42, 276–285. doi: 10.1016/j.jglr.2015.05.014
Hanson, K. C., Cooke, S. J., Suski, C. D., Niezgoda, G., Phelan, F. J. S., Tinline, R., et al. (2007). Assessment of largemouth bass (Micropterus salmoides) behaviour and activity at multiple spatial and temporal scales utilizing a whole-lake telemetry array. Hydrobiologia 582, 243–256. doi: 10.1007/s10750-006-0549-6
Hasnain, S. S., Escobar, M. D., and Shuter, B. J. (2018). Estimating thermal response metrics for North American freshwater fish using Bayesian phylogenetic regression. Can. J. Fish. Aquat. Sci. 75, 1878–1885. doi: 10.1139/cjfas-2017-0278
Helland, I. P., Finstad, A. G., Forseth, T., Hesthagen, T., and Ugedal, O. (2011). Ice-cover effects on competitive interactions between two fish species. J. Anim. Ecol. 80, 539–547. doi: 10.1111/j.1365-2656.2010.01793.x
Helland, I. P., Harrod, C., Freyhof, J., and Mehner, T. (2008). Co-existence of a pair of pelagic planktivorous coregonid fishes. Evol. Ecol. Res. 10, 373–390.
Holm, E., Mandrak, N. E., and Burridge, M. E. (2009). The ROM field guide to freshwater fishes of Ontario, 6th Edn. Toronto: Royal Ontario Museum.
Hynes, H. B. N. (1950). The food of fresh-water sticklebacks (Gasterosteus aculeatus and Pygosteus pungitius), with a review of methods used in studies of the food of fishes. J. Anim. Ecol. 19, 36–58. doi: 10.2307/1570
Hyslop, E. J. (1980). Stomach contents analysis – a review of methods and their application. J. Fish Biol. 17, 411–429. doi: 10.1111/j.1095-8649.1980.tb02775.x
James, G., Witten, D., Hastie, T., and Tibshirani, R. (2013). An introduction to statistical learning. New York, NY: Springer. doi: 10.1007/978-1-4614-7138-7
Jensen, T. C. (2019). Winter decrease of zooplankton abundance and biomass in subalpine oligotrophic Lake Atnsjøen (SE Norway). J. Limnol. 78, 348–363. doi: 10.4081/jlimnol.2019.1877
Keller, W. (2007). Implications of climate warming for Boreal Shield lakes: A review and synthesis. Environ. Rev. 15, 99–112. doi: 10.1139/A07-002
Kiljunen, M., Grey, J., Sinisalo, T., Harrod, C., Immonen, H., and Jones, R. I. (2006). A revised model for lipid-normalizing δ13C values from aquatic organisms, with implications for isotope mixing models. J. Appl. Ecol. 43, 1213–1222. doi: 10.1111/j.1365-2664.2006.01224.x
Kionka, B. C., and Windell, J. T. (1972). Differential movement of digestible and indigestible food fractions in rainbow trout, Salmo gairdneri. Trans. Am. Fish. Soc. 101, 112–115.
Koroiva, R., and Pepinelli, M. (2019). “Distribution and habitats of aquatic insects,” in Aquatic insects: Behavior and ecology, eds K. Del-Claro and R. Guillermo (Cham: Springer International Publishing), 11–33.
Larocque, S. M., Johnson, T. B., and Fisk, A. T. (2021). Trophic niche overlap and abundance reveal potential impact of interspecific interactions on a reintroduced fish. Can. J. Fish. Aquat. Sci. 78, 765–774. doi: 10.1139/cjfas-2020-0204
Legler, N. D., Johnson, T. B., Heath, D. D., and Ludsin, S. A. (2010). Water temperature and prey size effects on the rate of digestion of larval and early juvenile fish. Trans. Am. Fish. Soc. 139, 868–875. doi: 10.1577/t09-212.1
Lenth, R. (2020). emmeans: Estimated marginal means, aka least-squares means. R package Version 1.5.2-1. Available online at: https://CRAN.R-project.org/package=emmeans
Lepak, J. M., Kraft, C. E., and Weidel, B. C. (2006). Rapid food web recovery in response to removal of an introduced apex predator. Can. J. Fish. Aquat. Sci. 63, 569–575. doi: 10.1139/f05-248
Lotka, A. J. (1932). The growth of mixed populations: Two species competing for a common food supply. J. Washingt. Acad. Sci. 22, 461–469.
Macarthur, R. H. (1958). Population ecology of some warblers of northeastern coniferous forests. Ecology 39, 599–619.
MacRae, P. S. D., and Jackson, D. A. (2001). The influence of smallmouth bass (Micropterus dolomieu) predation and habitat complexity on the structure of littoral zone fish assemblages. Can. J. Fish. Aquat. Sci. 58, 342–351. doi: 10.1139/cjfas-58-2-342
Magnuson, J. J., Crowder, L. B., and Medvick, P. A. (1979). Temperature as an ecological resource. Am. Zooplogist 19, 331–343. doi: 10.1093/icb/19.1.331
Maitland, B. M., Del Rio, C. M., and Rahel, F. J. (2021). Effect of temperature on 13C and 15N incoropration rates and discrimation factors in two North American fishes. Can. J. Fish. Aquat. Sci. 78, 1833–1840. doi: 10.1139/cjfas-2021-0057
Marsden, J. E., Blanchfield, P. J., Brooks, J. L., Fernandes, T., Fisk, A. T., Futia, M. H., et al. (2021). Using untapped telemetry data to explore the winter biology of freshwater fish. Rev. Fish Biol. Fish. 31, 115–134. doi: 10.1007/s11160-021-09634-2
Martin, N. V. (1952). A study of the lake trout, Salvelinus Namaycush, in two Algonquin Park, Ontario, lakes. Trans. Am. Fish. Soc. 81, 111–137.
Martin, N. V. (1957). Reproduction of lake trout in Algonquin Park, Ontario. Trans. Am. Fish. Soc. 86, 231–244.
Mathias, A., and Chesson, P. (2013). Coexistence and evolutionary dynamics mediated by seasonal environmental variation in annual plant communities. Theor. Popul. Biol. 84, 56–71. doi: 10.1016/j.tpb.2012.11.009
McMeans, B. C., Kadoya, T., Pool, T. K., Holtgrieve, G. W., Lek, S., Kong, H., et al. (2019). Consumer trophic positions respond variably to seasonally fluctuating environments. Ecology 100, 1–10. doi: 10.1002/ecy.2570
McMeans, B. C., McCann, K. S., Guzzo, M. M., Bartley, T. J., Bieg, C., Blanchfield, P. J., et al. (2020). Winter in water: Differential responses and the maintenance of biodiversity. Ecol. Lett. 23, 922–938. doi: 10.1111/ele.13504
McMeans, B. C., McCann, K. S., Humphries, M., Rooney, N., and Fisk, A. T. (2015). Food web structure in temporally-forced ecosystems. Trends Ecol. Evol. 30, 662–672. doi: 10.1016/j.tree.2015.09.001
Morbey, Y. E., Addison, P., Shuter, B. J., and Vascotto, K. (2006). Within-population heterogeneity of habitat use by lake trout Salvelinus namaycush. J. Fish Biol. 69, 1675–1696. doi: 10.1111/j.1095-8649.2006.01236.x
Morbey, Y. E., Couture, P., Busby, P., and Shuter, B. J. (2010). Physiological correlates of seasonal growth patterns in lake trout Salvelinus namaycush. J. Fish Biol. 77, 2298–2314. doi: 10.1111/j.1095-8649.2010.02804.x
Morbey, Y. E., Vascotto, K., and Shuter, B. J. (2007). Dynamics of piscivory by lake trout following a smallmouth bass invasion: A historical reconstruction. Trans. Am. Fish. Soc. 136, 477–483. doi: 10.1577/t06-070.1
O’Reilly, C. M., Hecky, R. E., Cohen, A. S., and Plisnier, P. D. (2002). Interpreting stable isotopes in food webs: Recognizing the role of time averaging at different trophic levels. Limnol. Oceanogr. 47, 306–309. doi: 10.4319/lo.2002.47.1.0306
Ontario Ministry of Natural Resources and Forestry (2022). Fish ON-Line. Available online at: https://www.lioapplications.lrc.gov.on.ca/fishonline/Index.html?viewer=FishONLine.FishONLine (accessed January 10, 2021)
Park, T. (1954). Experimental studies of interspecies competition II. Temperature, humidity, and competition in two species of Tribolium. Physiol. Zool. 27, 177–238.
Pazzia, I., Trudel, M., Ridgway, M., and Rasmussen, J. B. (2002). Influence of food web structure on the growth and bioenergetics of lake trout (Salvelinus namaycush). Can. J. Fish. Aquat. Sci. 59, 1593–1605. doi: 10.1139/f02-128
Pépino, M., Goyer, K., and Magnan, P. (2015). Heat transfer in fish: Are short excursions between habitats a thermoregulatory behaviour to exploit resources in an unfavourable thermal environment? J. Exp. Biol. 218, 3461–3467. doi: 10.1242/jeb.126466
Peterson, B. J., and Fry, B. (1987). Stable isotopes in ecosystem studies. Annu. Rev. Ecol. Syst. 18, 293–320.
Plumb, J. M., and Blanchfield, P. J. (2009). Performance of temperature and dissolved oxygen criteria to predict habitat use by lake trout (Salvelinus namaycush). Can. J. Fish. Aquat. Sci. 66, 2011–2023. doi: 10.1139/F09-129
Plumb, J. M., Blanchfield, P. J., and Abrahams, M. V. (2014). A dynamic-bioenergetics model to assess depth selection and reproductive growth by lake trout (Salvelinus namaycush). Oecologia 175, 549–563. doi: 10.1007/s00442-014-2934-6
Post, D. M. (2002). Using stable isotopes to estimate trophic position: Models, methods, and assumptions. Ecology 83, 703–718. doi: 10.2307/3071875
Post, D. M., Layman, C. A., Arrington, D. A., Takimoto, G., Quattrochi, J., and Montaña, C. G. (2007). Getting to the fat of the matter: Models, methods and assumptions for dealing with lipids in stable isotope analyses. Oecologia 152, 179–189. doi: 10.1007/s00442-006-0630-x
R Core Team (2020). R: A language and environment for statistical computing. Vienna: R Foundation for Statistical Computing.
Riley, S. C., Marsden, J. E., Ridgway, M. S., Konrad, C. P., Farha, S. A., Binder, T. R., et al. (2019). A conceptual framework for the identification and characterization of lacustrine spawning habitats for native lake charr Salvelinus namaycush. Environ. Biol. Fishes 102, 1533–1557. doi: 10.1007/s10641-019-00928-w
Rowe, L., and Berrill, M. (1989). The life cycles of five closely related mayfly species (Ephemeroptera: Heptageniidae) coexisting in a small southern Ontario stream pool. Aquat. Insects 11, 73–80. doi: 10.1080/01650428909361351
Schindler, D. E., and Scheuerell, M. D. (2002). Habitat coupling in lake ecosystems. Oikos 98, 177–189. doi: 10.1034/j.1600-0706.2002.980201.x
Searle, S. R., Speed, F. M., and Milliken, G. A. (1980). Population marginal means in the linear model: An alternative to least squares means. Am. Stat. 34, 216–221. doi: 10.1080/00031305.1980.10483031
Sharma, S., Jackson, D. A., and Minns, C. K. (2009). Quantifying the potential effects of climate change and the invasion of smallmouth bass on native lake trout populations across Canadian lakes. Ecography 32, 517–525. doi: 10.1111/j.1600-0587.2008.05544.x
Sharma, S., Jackson, D. A., Minns, C. K., and Shuter, B. J. (2007). Will northern fish populations be in hot water because of climate change? Glob. Chang. Biol. 13, 2052–2064. doi: 10.1111/j.1365-2486.2007.01426.x
Sharma, S., Magnuson, J. J., Batt, R. D., Winslow, L. A., Korhonen, J., and Aono, Y. (2016). Direct observations of ice seasonality reveal changes in climate over the past 320-570 years. Sci. Rep. 6, 1–11. doi: 10.1038/srep25061
Sherwood, G. D., Pazzia, I., Moeser, A., Hontela, A., and Rasmussen, J. B. (2002). Shifting gears: Enzymatic evidence for the energetic advantage of switching diet in wild-living fish. Can. J. Fish. Aquat. Sci. 59, 229–241. doi: 10.1139/f02-001
Shimadzu, H., Dornelas, M., Henderson, P. A., and Magurran, A. E. (2013). Diversity is maintained by seasonal variation in species abundance. BMC Biol. 11:98. doi: 10.1186/1741-7007-11-98
Shuter, B. J., Finstad, A. G., Hellend, I. P., Zweimüller, I., and Hölker, F. (2012). The role of winter phenology in shaping the ecology of freshwater fish and their sensitivities to climate change. Aquat. Sci. 74, 637–657. doi: 10.1007/s00027-012-0274-3
Shuter, B. J., Giacomini, H. C., de Kerckhove, D., and Vascotto, K. (2016). Fish life history dynamics: Shifts in prey size structure evoke shifts in predator maturation traits. Can. J. Fish. Aquat. Sci. 73, 693–708. doi: 10.1139/cjfas-2015-0190
Skinner, M. M., Cross, B. K., and Moore, B. C. (2017). Estimating in situ isotopic turnover in rainbow trout (Oncorhynchus mykiss) muscle and liver tissue. J. Freshw. Ecol. 32, 209–217. doi: 10.1080/02705060.2016.1259127
Skinner, M. M., Martin, A. A., and Moore, B. C. (2016). Is lipid correction necessary in the stable isotope analysis of fish tissues? Rapid Commun. Mass Spectrom. 30, 881–889. doi: 10.1002/rcm.7480
Sommer, U., Gliwicz, Z., Lampert, W., and Duncan, A. (1986). The PEG-model of seasonal succession of planktonic events in fresh waters. Arch. Für Hydrobiol. 106, 433–471.
Speers-Roesch, B., Norin, T., and Driedzic, W. R. (2018). The benefit of being still: Energy savings during winter dormancy in fish come from inactivity and the cold, not from metabolic rate depression. Proc. R. Soc. B Biol. Sci. 285:20181593. doi: 10.1098/rspb.2018.1593
Stockwell, J. D., Yule, D. L., Hrabik, T. R., Sierszen, M. E., and Isaac, E. J. (2014). Habitat coupling in a large lake system: Delivery of an energy subsidy by an offshore planktivore to the nearshore zone of Lake Superior. Freshw. Biol. 59, 1197–1212. doi: 10.1111/fwb.12340
Sugimoto, R., Sato, T., Yoshida, T., and Tominaga, O. (2014). Using stable nitrogen isotopes to evaluate the relative importance of external and internal nitrogen loadings on phytoplankton production in a shallow eutrophic lake (Lake Mikata Japan). Limnol. Oceanogr. 59, 37–47. doi: 10.4319/lo.2014.59.1.0037
Suski, C. D., and Ridgway, M. S. (2009). Seasonal pattern of depth selection in smallmouth bass. J. Zool. 279, 119–128. doi: 10.1111/j.1469-7998.2009.00595.x
Sutton, A. O., Studd, E. K., Fernandes, T., Bates, A. E., Bramburger, A. J., Cooke, S. J., et al. (2021). Frozen out: Unanswered questions about winter biology. Environ. Rev. 29, 431–442. doi: 10.1139/er-2020-0127
Swanson, H. K., Lysy, M., Power, M., Stasko, A. D., Johnson, J. D., and Reist, J. D. (2015). A new probabilistic method for quantifying n-dimensional ecological niches and niche overlap. Ecology 96, 318–324.
Szabó, P. (2016). Ideal free distribution of metabolic activity: Implications of seasonal metabolic-activity patterns on competitive coexistence. Theor. Popul. Biol. 111, 1–8. doi: 10.1016/j.tpb.2016.05.001
Tansley, A. G. (1917). On competition between Galium saxatile L. (G. hercynicum Weig.) and Galium sylvestre Poll. (G. Asperum Schreb.) on different types of soil. J. Ecol. 5, 173–179. doi: 10.2307/2255655
Tonn, W. M., and Magnuson, J. J. (1982). Patterns in the species composition and richness of fish assemblages in northern Wisconsin lakes. Ecology 63, 1149–1166. doi: 10.2307/1937251
Tunney, T. D., McCann, K. S., Lester, N. P., and Shuter, B. J. (2012). Food web expansion and contraction in response to changing environmental conditions. Nat. Commun. 3, 1–9. doi: 10.1038/ncomms2098
Turschak, B., Bronte, C. R., Czesny, S., Gerig, B., Happel, A., Höök, T. O., et al. (2022). Temporal variation in the niche partitioning of Lake Michigan salmonines as it relates to alewife abundance and size structure. Can. J. Fish. Aquat. Sci. 79, 487–502. doi: 10.1139/cjfas-2021-0027
Vander Zanden, J. M., and Rasmussen, J. B. (1996). A trophic position model of pelagic food webs: Impact on contaminant bioaccumulation in lake trout. Ecol. Monogr. 66, 451–477.
Vander Zanden, M. J., Casselman, J. M., and Rasmussen, J. B. (1999). Stable isotope evidence for the food web consequences of species invasions in lakes. Nature 401, 464–467. doi: 10.1038/46762
Vander Zanden, M. J., Clayton, M. K., Moody, E. K., Solomon, C. T., and Weidel, B. C. (2015). Stable isotope turnover and half-life in animal tissues: A literature synthesis. PLoS One 10:e0116182. doi: 10.1371/journal.pone.0116182
Vander Zanden, M. J., Olden, J. D., Thorne, J. H., and Mandrak, N. E. (2004). Predicting occurrences and impacts of smallmouth bass introductions in north temperate lakes. Ecol. Appl. 14, 132–148.
Versteeg, E. J., Fernandes, T., Guzzo, M. M., Laberge, F., Middel, T., Ridgway, M., et al. (2021). Seasonal variation of behavior and brain size in a freshwater fish. Ecol. Evol. 11, 14950–14959. doi: 10.1002/ece3.8179
Visconti, A., Volta, P., Fadda, A., Di Guardo, A., and Manca, M. (2014). Seasonality, littoral versus pelagic carbon sources, and stepwise 15N-enrichment of pelagic food web in a deep subalpine lake: The role of planktivorous fish. Can. J. Fish. Aquat. Sci. 71, 436–446. doi: 10.1139/cjfas-2013-0178
Volterra, V. (1926). Fluctuations in the abundance of a species considered mathematically. Nature 118, 558–560. doi: 10.1038/118558a0
Wolf, N., Carleton, S. A., and del Rio, C. M. (2009). Ten years of experimental animal isotopic ecology. Funct. Ecol. 23, 17–26. doi: 10.1111/j.1365-2435.2008.01529.x
Woodland, R. J., Rodríguez, M. A., Magnan, P., Glémet, H., and Cabana, G. (2012). Incorporating temporally dynamic baselines in isotopic mixing models. Ecology 93, 131–144. doi: 10.1890/11-0505.1
Woolway, R. I., Kraemer, B. M., Lenters, J. D., Merchant, C. J., O’Reilly, C. M., and Sharma, S. (2020). Global lake responses to climate change. Nat. Rev. Earth Environ. 1, 388–403. doi: 10.1038/s43017-020-0067-5
Yeakel, J. D., Bhat, U., Smith, E. A. E., and Newsome, S. D. (2016). Exploring the isotopic niche: Isotopic variance, physiological incorporation, and the temporal dynamics of foraging. Front. Ecol. Evol. 4:1. doi: 10.3389/fevo.2016.00001
Yule, D. L., Stockwell, J. D., Schreiner, D. R., Evrard, L. M., Balge, M., and Hrabik, T. R. (2009). Can pelagic forage fish and spawning cisco (Coregonus artedi) biomass in the western arm of Lake Superior be assessed with a single summer survey? Fish. Res. 96, 39–50. doi: 10.1016/j.fishres.2008.09.012
Keywords: seasonality, stable isotope, acoustic telemetry, trophic niche, lake trout, smallmouth bass, thermal guild, coexistence
Citation: Bloomfield EJ, Guzzo MM, Middel TA, Ridgway MS and McMeans BC (2022) Seasonality can affect ecological interactions between fishes of different thermal guilds. Front. Ecol. Evol. 10:986459. doi: 10.3389/fevo.2022.986459
Received: 05 July 2022; Accepted: 15 September 2022;
Published: 06 October 2022.
Edited by:
John Whiteman, Old Dominion University, United StatesReviewed by:
Bryan Maitland, University of Wisconsin–Madison, United StatesDan Gibson-Reinemer, Adams State University, United States
Copyright © 2022 Bloomfield, Guzzo, Middel, Ridgway and McMeans. This is an open-access article distributed under the terms of the Creative Commons Attribution License (CC BY). The use, distribution or reproduction in other forums is permitted, provided the original author(s) and the copyright owner(s) are credited and that the original publication in this journal is cited, in accordance with accepted academic practice. No use, distribution or reproduction is permitted which does not comply with these terms.
*Correspondence: Emma J. Bloomfield, ZW1tYUBzZXBhbC5jYQ==