- 1National Research Council, Washington, DC, United States
- 2The Ocean Foundation, San Diego, CA, United States
- 3NOAA National Marine Fisheries Service, Southwest Fisheries Science Center, La Jolla, CA, United States
- 4Asociación ProCosta, Eastern Pacific Hawksbill Initiative (ICAPO), San Salvador, El Salvador
- 5NOAA National Marine Fisheries Service, Southeast Fisheries Science Center, Beaufort, CA, United States
In the eastern Pacific Ocean, hawksbill sea turtles (Eretmochelys imbricata) are adapted to use coastal habitats and ecosystems uncharacteristic of most other sea turtles. Once considered extirpated from this region, hawksbills had sought refuge in estuaries, nesting on muddy banks among the tangles of mangrove roots. This population is at high risk of bycatch during fishing efforts in the estuaries (blast fishing) and adjacent coastal rocky reefs (gillnets), and is further impacted by habitat degradation from coastal development and climate change. The conservation and population recovery of hawksbills in this region is highly dependent on management actions (e.g., nest relocation, habitat protection, bycatch mitigation), and a better understanding of how hawksbills use and move between distinct habitats will help prioritize conservation efforts. To identify multi-year habitat use and movement patterns, we used stable carbon (δ13C) and nitrogen (δ15N) isotope analysis of skin and bone growth layers to recreate movements between two isotopically distinct habitats, a nearshore rocky reef and a mangrove estuary, the latter distinguishable by low δ13C and δ15N values characteristic of a mangrove-based foodweb. We applied skeletochronology with sequential δ13C and δ15N analysis of annual growth layers, “skeleto+iso,” to a dataset of 70 hawksbill humeri collected from coastal El Salvador. The results revealed at least two unique habitat-use patterns. All turtles, regardless of stranding location, spent time outside of the mangrove estuaries during their early juvenile years (< 35 cm curved carapace length, CCL, age 0–5), showing that an oceanic juvenile stage is likely for this population. Juveniles ca. > 35 cm then began to recruit to nearshore areas, but showed divergent habitat-use as some of turtles occupied the coastal rocky reefs, while others settled into the mangrove estuaries. For turtles recruiting to the estuaries, settlement age and size ranged from 3 to 13 years and 35–65 cm CCL. For the adult turtles, age-at-sexual-maturity ranged from 16 to 26 years, and the maximum reproductive longevity observed was 33 years. The skeleto+iso also showed that adult hawksbills have long-term habitat fidelity, and the results demonstrate the importance of both mangrove estuary and nearshore rocky reefs to the conservation of hawksbills in the eastern Pacific.
Introduction
Hawksbill sea turtles (Eretmochelys imbricata) are a globally distributed species, considered critically endangered throughout their range (IUCN, 2018). Their unique keratinized carapace shells have made them the target of harvesting for the now-illegal tortoiseshell or bekko trade, and several populations are still recovering from this past historical harvesting (Limpus and Miller, 1990; LaCasella et al., 2021). In the eastern Pacific Ocean, hawksbills were once considered extirpated from the region, but were scientifically “rediscovered” in 2007 (Vásquez and Liles, 2008; Gaos and Yañez, 2012). This unique population of hawksbills in the eastern Pacific (EP) had sought refuge in dense mangrove estuaries, nesting in the sand banks dispersed among mangrove roots, an atypical behavior for sea turtles, in addition to more typical scattered nesting at contiguous, open-coast sandy beach sites (Bolten, 2003; Gaos et al., 2010, 2012a). This population remains at high risk of bycatch during fishing efforts in the estuaries (blast fishing; Liles et al., 2011; Wedemeyer-Strombel et al., 2021) and adjacent coastal rocky reefs (bottom-set gillnets; Liles et al., 2017), and is further impacted by habitat degradation from coastal development and climate change. The conservation and population recovery of hawksbills in the EP is highly dependent on management actions (i.e., nest relocation, habitat protection, bycatch mitigation), and a better understanding of how hawksbills use and move between distinct habitats that will help prioritize conservation efforts (Liles et al., 2015a,b, 2019).
Satellite tags and mark-recapture efforts using flipper or internal (PIT) tags are often the best methods for tracking sea turtle movements within and between habitats, as well as attempting to estimate residency duration in specific habitats (Plotkin, 2003; Godley et al., 2008; Hays and Hawkes, 2018). Yet these methods cannot easily provide sequential multi-year data on sea turtle location and movement because satellite tags rarely remain attached for more than ∼3 years (e.g., Hawkes et al., 2012), and are difficult to place on small and or rapidly-growing juveniles (Mansfield et al., 2021). Moreover, flipper and PIT tags can only provide information after the turtle has been captured once and tagged, after which data generation is limited to moments in time when the turtle is actively re-captured during on-site monitoring or recovered dead. As a result, these traditional methods leave gaps in our knowledge about the movements and life history patterns of these long-lived animals. Alternative methods, however, are emerging to help generate and recreate sequential multi-year habitat use data, including photo-identification (Dunbar et al., 2021), often with the help of community scientists (e.g., Hanna et al., 2021), and the combination of skeletochronology (the study of growth layers) with stable isotope analysis “skeleto+iso” (Snover et al., 2010; Avens et al., 2013, 2021; Ramirez et al., 2015; Turner Tomaszewicz et al., 2016, 2017a).
Skeletochronology analysis produces time-series data on individual turtles that includes estimated age, body size, annual growth, and calendar year for each growth layer identified within a bone (Avens et al., 2015; Turner Tomaszewicz et al., 2015b, 2017a; Goshe et al., 2020). The onset of maturity can also be estimated during the analysis, producing key demographic parameters such as age- and size-at-sexual-maturation (ASM, SSM) and reproductive longevity (Avens et al., 2020; Turner Tomaszewicz et al., 2022). When these data are used to guide precision sampling of individual bone annual growth layers for stable isotope analysis (Turner Tomaszewicz et al., 2016), biogeochemical data are added to the dataset and can inform habitat use and diet for each year as well. The result is a multi-year, sequential record for individual turtles on age- and size-specific habitat location, diet, and growth for specific calendar years. For long-lived and migratory species like sea turtles, this skeleto+iso method can recreate movements of hard-to-study life stages, such as post-hatchlings and young juveniles, or long-term foraging patterns of adults, which are important pieces of information to guide habitat-specific conservation efforts.
The application of stable isotopes to track animal movement is especially useful when there are at least two isotopically distinct habitats being considered (Seminoff et al., 2012; Ramirez et al., 2015; Vander Zanden et al., 2015; Turner Tomaszewicz et al., 2017a). A variety of physical and biological factors affect the stable isotope values in animals. Following the “you are what you eat” analogy, diet is reflective in consumer tissues (Fry, 2006), and differences across landscapes (and oceanscapes) also affect isotope patterns in animals (West et al., 2006; Hobson and Wassenaar, 2018). The isotopic values at the base of the foodweb in a particular location are largely dictated by the dominant nutrient cycling processes in each area (Montoya, 2008; McMahon et al., 2013). For example, in open ocean habitats where primary productivity is relatively low, stable carbon isotope values (δ13C) are typically lower in comparison to other high productivity regions such as coastal neritic zones (DeNiro and Epstein, 1978; Oczkowski et al., 2016; Espinasse et al., 2019). An exception to this low-to-high pattern moving from offshore-to-near shore occurs in some coastal areas where the δ13C values can be lower due to terrestrial inputs or C3 plant species, such as mangroves (France, 1995; Bouillon et al., 2008; Seminoff et al., 2021). Similarly, in nutrient-limited open ocean habitats, the stable nitrogen isotope (δ15N) values are typically lower than coastal areas due to nitrogen fixation by cyanobacteria being the dominant cycling regime in oligotrophic areas (Montoya et al., 2004; Deutsch et al., 2011). By contrast, in most neritic areas, denitrification is more present in foodwebs from the input of nutrient-cycling benthic prey (both flora and fauna), as well as the upwelling of cycled nitrogen into the pelagic water column foodweb, producing higher δ15N values (Deutsch et al., 2011; Seminoff et al., 2012; Fleming et al., 2016; Oczkowski et al., 2016). This pattern has been demonstrated for several sea turtle species such as North Pacific loggerheads (Caretta caretta) in the pelagic central North Pacific vs. in the neritic eastern North Pacific (Allen et al., 2013; Turner Tomaszewicz et al., 2017a), pelagic vs. benthic foraging loggerheads (McClellan et al., 2010; Ramirez et al., 2015) and green sea turtles (Chelonia mydas; Turner Tomaszewicz et al., 2018; Seminoff et al., 2021), and turtle moving among ocean basins (Wallace et al., 2006; Pajuelo et al., 2010; Seminoff et al., 2012).
In El Salvador, the two high-use habitats for hawksbills, mangrove estuaries and nearshore rocky reefs, are known to be isotopically distinct because of the uniquely low δ13C values that characterize the mangrove-based foodweb (Seminoff et al., 2021; Wedemeyer-Strombel et al., 2021). Like in other mangrove-based foodwebs, the low δ13C values are a result of the carbon cycle used by these marine angiosperms, which differ from other marine flora such as macroalgae and seagrasses that have higher δ13C values (Marshall et al., 2007; Bouillon et al., 2008). As a result, these mangrove habitats are isotopically distinct from open ocean and coastal reef areas (Fry and Ewel, 2003; McMahon et al., 2011; Wedemeyer-Strombel et al., 2021). These habitats in El Salvador—both estuaries and rocky reefs—are under increasing pressure from local fishing efforts, which have resulted in high levels of fishery-related bycatch and, subsequently, offer the opportunity to collect humeri samples from turtles stranded in each of these locations. Here, we applied skeleto+iso to the bones of these dead-stranded hawksbill turtles to characterize key demographic parameters such as age, growth rates, ASM, and SSM, and also establish multi-year habitat use, movement patterns and residency duration of each isotopically distinct habitat. These results will help inform habitat-specific risk assessments of hawksbills, where each type of spatially explicit threat requires a different approach to minimize bycatch and increase hawksbill survival rates.
Materials and methods
Study area
Our study was conducted at two primary nesting and foraging areas for hawksbills in the EP (Gaos et al., 2012a,2017; Liles et al., 2017): Bahía de Jiquilisco (13°13′N, 88°32′W) and Punta Amapala (13°08′N, 87°55′W) in El Salvador (Figure 1). Small-scale, nearshore fisheries provide important livelihood support for coastal residents at both sites. At the same time, these fisheries also constitute the greatest threat to hawksbills through bycatch mortality (Liles et al., 2011, 2017). Bahía de Jiquilisco and Punta Amapala are situated within the migration corridor of post-nesting hawksbills (Gaos et al., 2012b), further increasing the potential for fisheries interactions.
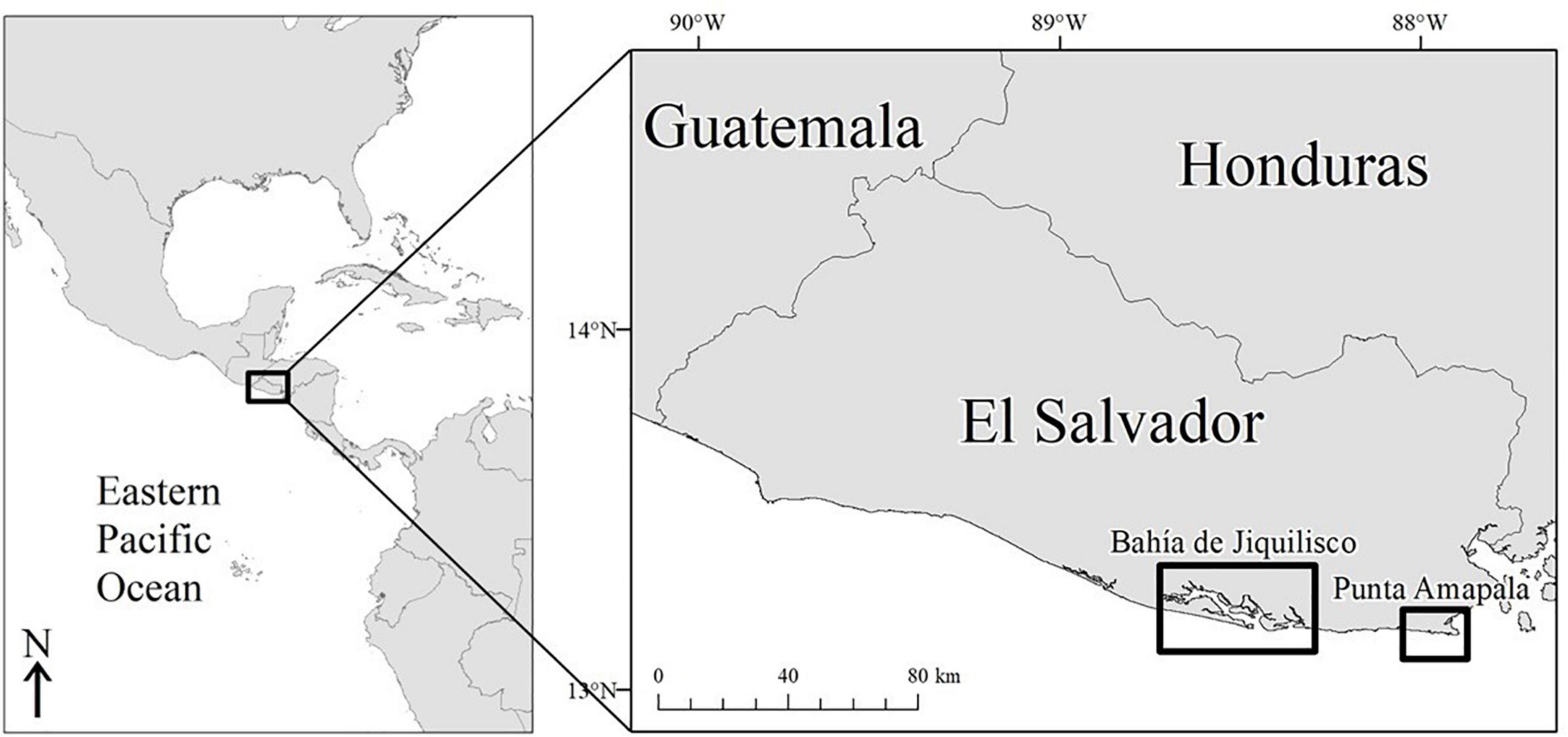
Figure 1. Study site in El Salvador where the 70 hawksbill turtles were recovered. A total of 35 turtles were recovered from the mangrove estuary, Bahía de Jiquilisco, and 35 were recovered from the rocky reef area at Punta Amapala.
Bahía de Jiquilisco (635 km2) is a National Conservation Area, RAMSAR wetland, and UNESCO Biosphere Reserve (MARN, 2014). It is the largest mangrove forest in El Salvador and includes numerous estuaries, channels, and islands. Bahía de Jiquilisco hosts ∼40% of known hawksbill nesting in the EP region, which primarily occurs along a series of inshore sand beaches. Blast fishing and bottom-set longlines are serious threats to hawksbills in the estuary, particularly during the nesting season (May–October) when reproductively active adults congregate near nesting beaches. Conversion of mangrove forests to shrimp farms and uncontrolled development of nesting habitat further threaten the species in Bahía de Jiquilisco.
Punta Amapala (26 km2), approximately 60 km east of Bahía de Jiquilisco, flanks the western border of the Gulf of Fonseca and is comprised primarily of submerged volcanic reef formations at depths ranging from 0 to 30 m. It hosts diverse marine communities, including corals, sponges, and fishes (Domínguez-Miranda, 2010), and constitutes one of the most important open-coast nesting beaches for hawksbills in the eastern Pacific (Liles et al., 2011; Gaos et al., 2017). Lobster gillnet fishing along the rocky reef is a major threat to hawksbills at Punta Amapala and represents the greatest single source of human-induced, in-water mortality of hawksbills in the eastern Pacific (Liles et al., 2017).
Sample collection and processing
During 2015–2019, tissue samples were collected from stranded and bycaught hawksbills from the two main study areas—Bahía de Jiquilisco and Punta Amapala (Figure 1). Upon carcass recovery, turtles were searched for flipper and passive integrated PIT tags, body size was measured as curved carapace length (CCL) and sex was assigned if (1) the turtle was tagged from a previous nesting event (F) or (2) a necropsy was conducted and gonads identified. Skin samples were collected using a scalpel and stored in salt until processed. Bone samples were removed, cleaned, and air dried until processed. All samples were sent to San Diego, CA under CITES permits for processing at the NOAA NMFS Southwest Fisheries Science Center. In the lab, skin samples were rinsed, cut, lyophilized, and then weighed to 1.5 mg and packed in tin capsules for SIA processing.
Bone samples were first processed for skeletochronological analysis and then stable isotope analysis as described in Turner Tomaszewicz et al. (2016) and Goshe et al. (2020). Briefly, we first cross-sectioned two 3-mm pieces from each humerus using an Isomet slow speed saw and diamond wafer blade. The proximal section was use for isotope analysis (see below) and the distal section was used for the skeletochronology aging analysis. The aging section was fixed in 10% buffered formalin and decalcified in a commercial “RDO” solution by Apex, before being sliced into 25-μm-thin sections using a freezing-stage sliding microtome by Leica. Thin sections were then stained in a modified Ehrlich’s stain, visually inspected for completeness, mounted to a slide, and digitally imaged to produce a high-resolution record of the bone revealing lines of arrested growth (LAGs) that denote the outer edges of individual skeletal growth marks. Imaging was conducted on an Olympus CX43 microscope using cellSens software. In hard-shelled sea turtles, annual LAG formation has been validated in multiple species, including hawksbills, and was therefore also assumed true for this population (hawksbill, Snover et al., 2012; other species: loggerhead, Kemp’s ridley, Snover and Hohn, 2004; green, Snover et al., 2011). The digitized images were then visually inspected by two independent readers (CTT, LA), following standard skeletochronology protocol, whereby each person marked the location and total count of LAGs observed in each bone (Avens and Snover, 2013; Turner Tomaszewicz et al., 2015a; Goshe et al., 2016), and the marked reads were compiled, compared, and a final consensus was reached.
Age, size, and growth estimation
For each consensus image, we determined the final LAG location and number for all bones and recorded the LAG diameters as measured along the antero-posterior axis (Zug et al., 1986). We used these data for age, size and growth estimation analyses as described below (Snover et al., 2007). First, any bone containing a distinctive diffuse mark, characteristic of the first-year annulus, marked the first year of a turtle’s life (Snover and Hohn, 2004; Goshe et al., 2016; Avens et al., 2021) and was directly aged (n = 43). Bones without the first-year annulus mark (n = 27) had resorbed some LAGs during bone growth, and required the application of a correction factor to estimate the number of LAGs lost as described in Avens et al. (2012; 2015; 2021). We established and applied correction factors for these bones, all of which had at least one LAG diameter smaller than the largest LAG from the directly aged bones (23.4 mm). The estimated age-at-stranding of each turtle was then calculated by summing together the total number of observed LAGs with the calculated number of LAGs lost. Once the final age was estimated for each bone, we assigned an estimated age to each subsequent LAG by subtracting one for each LAG moving inward from the outermost LAG.
Once age was estimated, we back-calculated body size (CCL) at each measured LAG using the relationship between body size (CCL) and humerus (and LAG) diameter to produce estimated size and incremental growth, following standard skeletochronology analytical methods (Snover et al., 2007; Avens et al., 2015; Goshe et al., 2016). Here, we applied the body proportional hypothesis (BPH)-corrected (Francis, 1990) allometric equation modified for application to sea turtles to yield a back-calculated CCL for each turtle at each measured LAG as recommended in Snover et al. (2007) and commonly applied in other sea turtle studies (Avens et al., 2012; Avens and Snover, 2013; Turner Tomaszewicz et al., 2018, 2022). First, we characterized the relationship between CCL and humerus section diameter using the following allometric equation (Snover et al., 2007; Goshe et al., 2010):
Here, L is the estimated length (CCL), Lop is the minimum hatchling carapace length, D is the humerus section diameter, Dop is the minimum hatchling humerus diameter, b is the slope of the relationship, and c is the proportional coefficient. To obtain values for east Pacific hawksbill hatchling CCL and humerus diameter we collected and measured these values for 20 hatchlings retrieved during nest exhumations from hatchery-relocated nests at Bahía de Jiquilisco sites, and recorded the minimum humerus diameter (1.7 mm) and minimum carapace length (3.5 cm). Hatchling carapace lengths were measured as straight carapace length (SCL), and here we assumed the SCL and CCL differences were less than 1–4 mm (based on paired SCL:CCL measurements, and comparable to standard measuring error, ProCosta, unpub.) and were therefore equivalent measurements for the purposes of this analysis. Parameters b and c were optimized using the non-linear least squares function “nls” in the “stats” package in R version 4.0.1 (R Core Team, 2021). Then, to apply this relationship to LAG diameters, we modified Equation 1 such that LAG diameter was used in place of humerus section diameter, to yield a back-calculated BPH-corrected body size (CCL) at time of LAG formation (Snover et al., 2007):
We used these back-calculated CCL estimates to then calculate the incremental annual growth for sequential growth layers. For year–1 growth, we used the difference between the size (CCL) at age 1 and the mean hatchling size (3.8 cm) used in this study.
For any individual turtle where we observed compression of LAG spacing at the lateral edge of the bone (i.e., rapprochement), which is associated with sexual maturity, we determined estimated age and size at maturation (ASM, SSM) (Francillon-Vieillot et al., 1990; Avens et al., 2012, 2015).
To model the size-at-age relationship of these hawksbills, we applied a generalized additive mixed model (GAMM) and a von Bertalanffy growth curve using the paired size, age, and growth data, from each individual turtle bone (Avens et al., 2015, 2017; Turner Tomaszewicz et al., 2018, 2022). The GAMM accounted for individual variation and repeated observations by including individual identity as a random effect (Chaloupka and Musick, 1997; Avens et al., 2013, 2015; Turner Tomaszewicz et al., 2018, 2022). We conducted the analysis using the “mgcv” package and the “gamm” function in R version 4.0.1 (Wood, 2017; R Core Team, 2021). After converting all measurable LAG diameters to back-calculated CCL estimates, we used the size-at-age data to fit a smoothing spline model to characterize size-at-age, and we generated the predicted fit for the same data, with 95% confidence intervals, using the “smooth.spline” function in the “stats” package in R (Hastie and Tibshirani, 1990; R Core Team, 2021). Next, we generated von Bertalanffy growth models using a bootstrapped method, as described in Avens et al. (2015, 2017) and Turner Tomaszewicz et al. (2018, 2022). To do this, we repeatedly sampled the back-calculated somatic growth rate data in the model to extract a single growth-at-length data point for each individual turtle in the sample and then used these non-parametric bootstrap samples to estimate the growth parameter, k, and estimate upper size limit, Linf, for Fabens modification of the von Bertalanffy growth curve. Here we conducted randomized re-sampling of the growth rate data 1,000 times to describe uncertainty in the von Bertalanffy parameters (Avens et al., 2015, 2017). For all analysis, we evaluated significance as α = 0.05, and present results as mean ± standard error unless otherwise noted.
Stable isotope analysis
To extract samples from individual growth layers for stable isotope analysis (SIA), the paired digital aging image from skeletochronology processing was used as a guide to target sampling paths on the adjacent proximal bone section (Turner Tomaszewicz et al., 2016, 2017a). Using one of two computer-guided micromill units (CM-2 Carpenter micromill and an Elemental Scientific Lasers MicroMill2), we extracted 1.5 mg of bone powder from individual growth layers and weighed samples into 5 × 9 mm2 tin capsules for SIA. No additional treatment was necessary for lipid extraction or removal of inorganic carbonate (Turner Tomaszewicz et al., 2015b, 2017b).
All samples were sent to and processed at the University of Florida, Gainesville, FL Stable Isotope Laboratory. Samples already loaded into tin capsules were placed in a 50-position automated Zero Blank sample carousel on a N.C. Technologies ECS 8020 elemental analyzer. After combustion in a quartz column at 1,000°C in an oxygen-rich atmosphere, the sample gas was transported in a He carrier stream and passed through a hot reduction column (650°C) consisting of elemental copper to remove oxygen. The effluent stream then passed through a chemical (magnesium perchlorate) trap to remove water followed by a 1.5-m gas chromatography (GC) column at 55°C to separate N2 from CO2. The sample gas next passed into a ConFlo IV interface and into the inlet of a Thermo Electron Delta V Advantage isotope ratio mass spectrometer running in continuous flow mode where the sample gas was measured relative to laboratory reference N2 and CO2 gases. All carbon isotopic results are expressed in standard delta notation relative to Vienna Pee Dee Belemnite (VPDB). All nitrogen isotopic results are expressed in standard delta notation relative to AIR. To account for the trace amount of carbonate in the cortical bone samples, the δ13C values of all bone samples were then mathematically corrected as recommended in Turner Tomaszewicz et al. (2015b) using the following equation specific for sea turtles in the Pacific:
To isotopically distinguish two separate habitats, we referenced hawksbill skin stable isotope values previously published and from the current study to help characterize the mangrove estuary (Bahía de Jiquilisco, Wedemeyer-Strombel et al., 2021) and the rocky reef / ocean (Punta Amapala, current study) as two isotopically distinct locations (inside or outside estuary). In Wedemeyer-Strombel et al. (2021), skin from juvenile (<65 cm CCL) hawksbills captured in Bahía de Jiquilisco had stable carbon isotope values that ranged from ca. –22 to –16‰ and were correlated with turtle body size; the smaller turtles (ca. 40 cm CCL) captured in the estuary had higher δ13C values (ca. –16‰) than the larger turtles (ca. 50 cm CCL, ca. –22‰) also captured in the estuaries. The same study also sampled prey from mangrove estuaries, with a mean δ13C value of –24‰ (–20‰ without the 4‰ trophic offset), further supporting lower δ13C values to characterize a mangrove-based foodweb. We also analyzed skin samples in the current study (see “Results” below) from turtles recovered from the two distinct locations to further support the designation of a δ13C values to characterize each habitat. Specifically, the skin samples collected from turtles in Punta Amapala had δ13C values between –14.9 and –17.6‰ (Table 2). Based on these new and previously published data, we assigned –18‰ as the threshold δ13C value for hawksbill skin indicating mangrove estuary habitat use and foraging. To ensure this threshold value from a skin tissue was directly comparable to stable isotope values from bone tissue (the samples used in the rest of the current study), we converted –18‰ skin to a bone-equivalent value using the equation from Turner Tomaszewicz et al. (2017b) and applied in other studies, including Turner Tomaszewicz et al. (2018). The application of this conversion equation yielded a bone threshold δ13C value of –18.03‰ to indicate use of the mangrove estuary habitat of Bahía de Jiquilisco; this minimal difference between the skin and bone δ13C value was expected given the findings of Turner Tomaszewicz et al. (2017b) which found a minimal difference between paired bone and skin tissues.
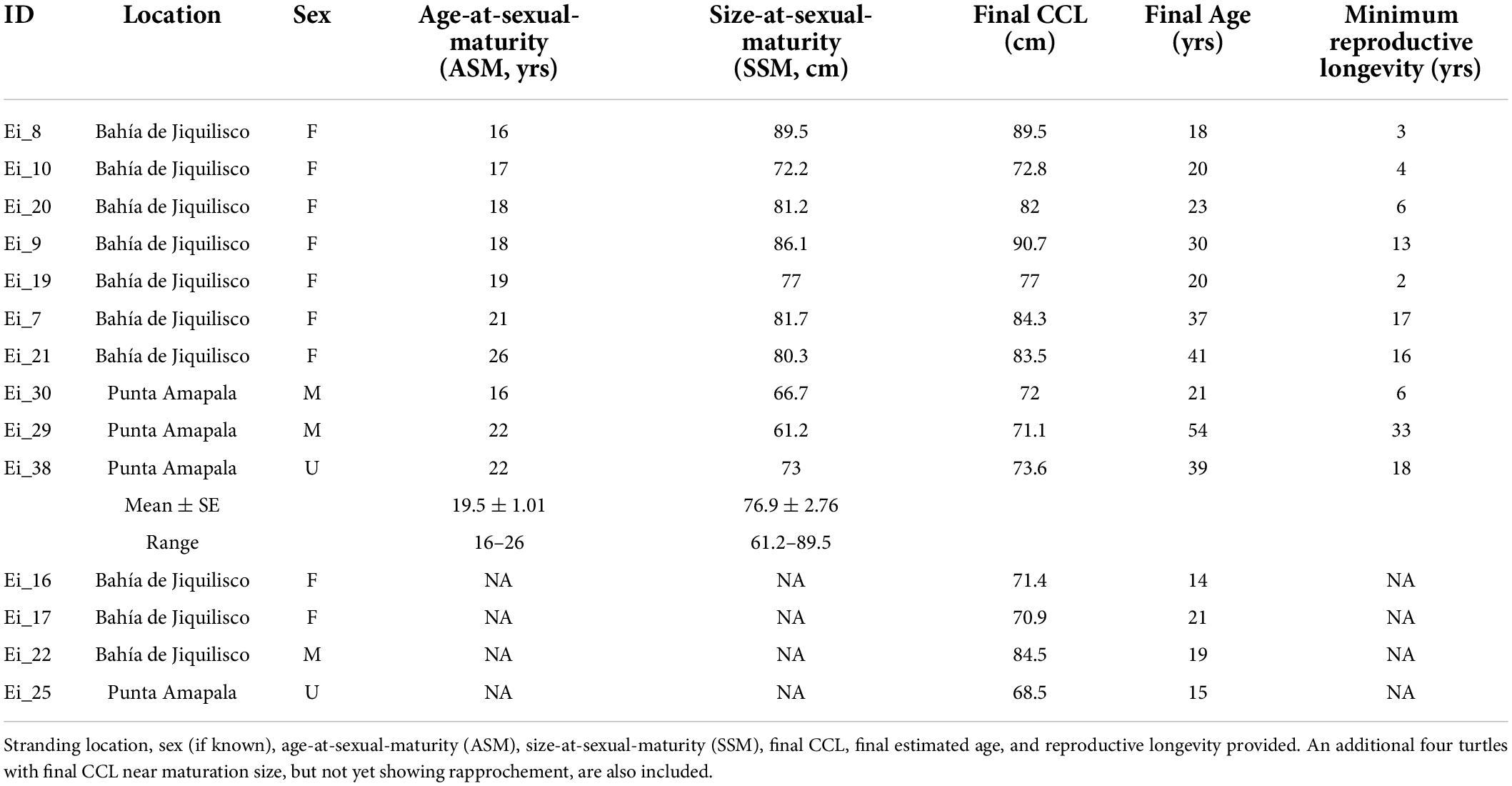
Table 1. Detailed information for the ten hawksbill turtles determined to reach maturity based on rapprochement observed during skeletochronology.

Table 2. The range, mean, and standard error (SE) of stable carbon (δ13C) and nitrogen (δ15N) isotope values of the two tissues sampled: bone individual growth layers, and skin.
Finally, we combined all the skeleto+iso data and to each growth layer we assigned a corresponding estimated age, body size, annual growth, calendar year, and habitat. The habitat assignment was based on stable isotope values (δ13C < –18‰ = mangrove estuary and δ13C > –18‰ = ocean/rocky reef), the final (outermost) growth layer was assigned to the habitat where the turtle stranded, regardless of SIA values. If the δ13C value did not match with the habitat in which the turtle was found, it was assumed to be newly recruited to that habitat. We then used these habitat-assignments to identify timing of habitat shifts (age and size), and to subsequently examine if there were any growth advantages associated with a particular habitat for a given size class (Wilcoxon Rank Sum, aka Mann–Whitney comparison, significance level at p < 0.05).
Results
Skeletochronology size and age estimates
A total of 70 bones were processed for age and size analysis, 12 of which were from females, four from males, and the remaining 54 of unknown sex. We identified 599 individual LAGs within the 70 bones processed for skeletochronology, and body sizes at stranding ranged from 28.5 to 90.7 cm. The sizes of the turtle recovered in Bahía de Jiquilisco (n = 35) ranged from 35.4 to 90.7 cm CCL [58.3 ± 2.9 cm (mean ± SE)], and the turtles recovered from Punta Amapala (n = 35) ranged in size from 28.5 to 73.6 cm CCL (41.4 ± 2.1 cm). The bones from the 70 turtles analyzed retained between 2 and 48 LAGs (8.3 ± 0.95), for a total of 580 identified and measured LAGs. At the time of carcass recovery, the CCL was recorded for 55 of the 70 turtles, and the following linear relationship between total humerus section diameter (THD) and turtle size (CCL) was used to estimate CCL for the remaining 15 turtles:
A total of 43 bones retained the year-1 annulus and could therefore be directly aged, where 1 LAG equals 1 year. The final age-at-stranding for these 43 turtles ranged from 2 to 10 (mean ± SE 5.0 ± 0.3). Using the measurable internal LAG diameters (n = 210) from these 43 directly-aged bones, we determined the best-fit correction factor equation (CF1) which was a linear equation:
where y is the LAG diameter and x is the LAG number. Using this CF1 equation, we estimated the number of LAGs lost due to resorption, where LAG diameter y was each bone’s innermost LAG or resorption core diameter, for the remaining 27 bones (those without an annulus, and with at least one LAG diameter less than 23.4 mm, the max THD of the Group 1 bones). For the 27 bones with CF1 applied, we then summed this resulting LAG-lost number with the number of LAGs retained in each bone to obtain final estimated age at stranding. The number of estimated LAGs lost (resorbed) from these larger bones ranged from one to 10 (mean ± SE 4.7 ± 0.5) LAGs. Final estimated age (LAGs retained + LAGs resorbed, with all ages rounded to the nearest whole number) for all 70 turtles ranged from 2 to 54 years old (mean ± SE 10.1 ± 1.2 years; Figure 2). The age at stranding for the turtles recovered in Bahía de Jiquilisco (n = 35) ranged from 3 to 41 years (mean ± SE 12.0 ± 1.6) and the turtles recovered from Punta Amapala (n = 35) ranged from 2 to 54 years (mean ± SE 8.2 ± 1.8; Figure 2).
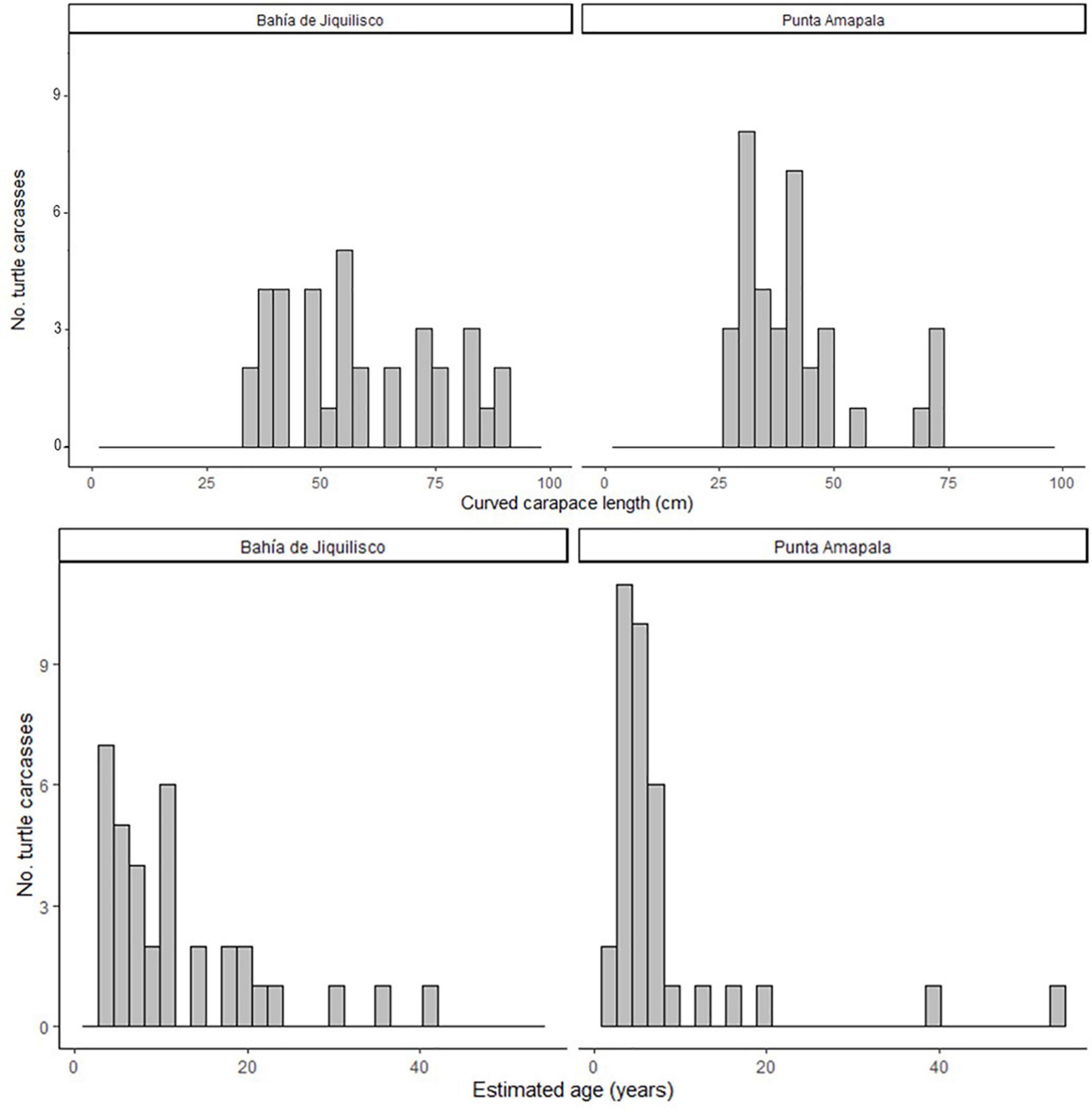
Figure 2. The body size (curved carapace length, CCL, cm) and estimated age (years) for the 70 hawksbill turtles analyzed for skeletochronology (35 from Bahía de Jiquilisco, 35 from Punta Amapala).
Back-calculated body size estimates corresponding to the measured LAG diameters ranged from 14.8 to 90.7 cm CCL (mean ± SE 60.8 ± 0.33). For all 70 bones, we applied east Pacific hawksbill-specific hatchling parameters (min CL: 3.5 cm, min hatchling THD: 1.7 mm, slope b = 2.526, proportionality coefficient c = 1.038) to the BPH back-calculated CCL equation (Equation 2) at each measurable LAG. This yielded 573 CCL-at-age estimates (Figures 3, 4) and 541 annual somatic growth estimates (Supplementary Figure 3).
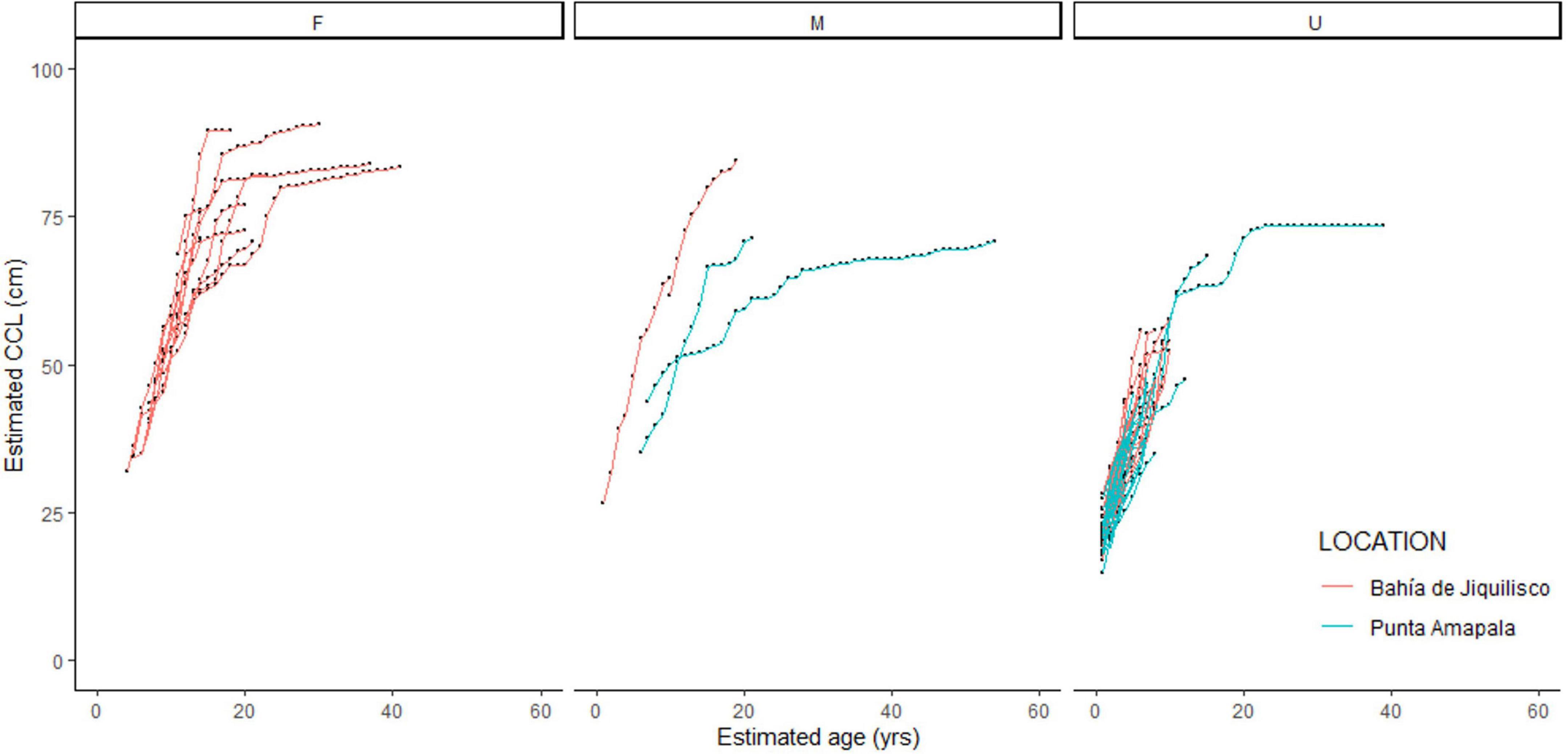
Figure 3. Estimated body size (curved carapace length, CCL, cm) and corresponding estimated age (years) for the 70 hawksbill turtles aged using skeletochronology. Individuals with known sex are separated as female (F), male (M) and unknown (U), and colors indicate stranding location, red = mangrove estuary of Bahía de Jiquilisco, blue = rocky reef area of Punta Amapala.
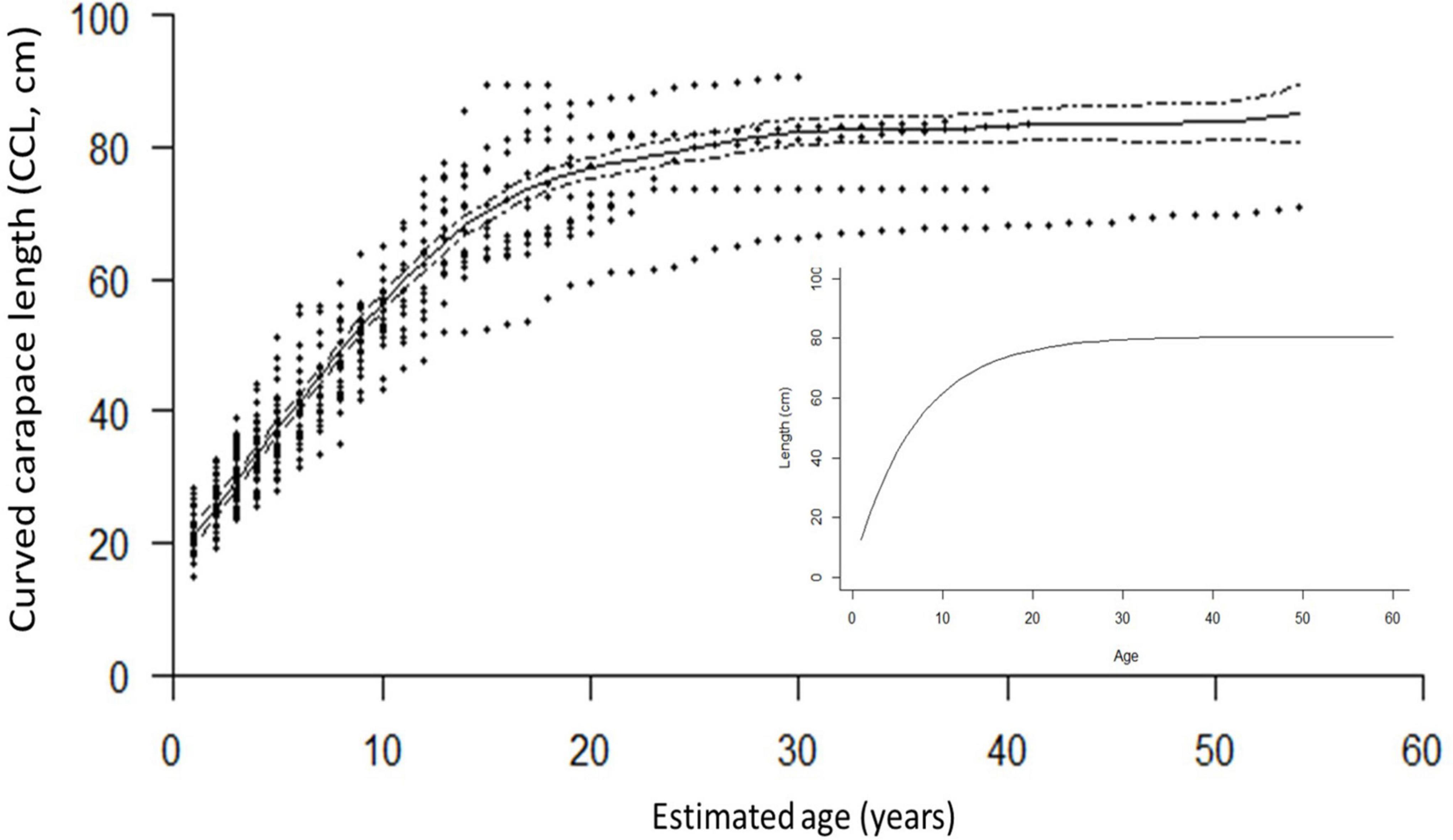
Figure 4. The generalized additive mixed model (GAMM) smoothing spline, fit to the length-at-age dataset of the 70 turtles, was significant (p < 0.0001, Edf = 7.457, adj. r2 = 0.887), and showed close correspondence with the bootstrapped von Bertalanffy model (inset), based on growth-at-length data. The mean SSM at rapprochement, 76.9 cm CCL, was used to estimate the growth parameters Linf: 80.6 ± 0.19 cm (95% CI: 80.2–81.0 cm) k: 0.142 ± 0.0008 (95% CI: 0.141–0.144).
Growth and maturation timing
Of the 541 incremental growth estimates, annual growth ranged from 0 to 24.4 cm CCL (mean ± SE 3.4 ± 0.07). The annual growth was greatest for age zero to one, as expected (mean 17.4 cm; range = 11.0 to 24.4 cm), and for all other ages (ages 2+), annual growth ranged from 0 to 12.6 cm CCL (mean 2.9 ± 0.04). Growth rates decreased with age and size, and are discussed in more detail below in context of habitat use (Figure 4, Supplementary Figure 3, and Supplementary Table 1). The growth modeled by both the GAMM smoothing spline and the bootstrapped von Bertalanffy models showed close congruence, with turtles increasing in size steadily until age ∼15, when growth then began to slow and approach near-zero annual growth at ∼80 cm CCL between age 20–30, the presumed onset of maturity (Figure 4).
A total of ten turtles showed LAG compaction indicating maturity, and the corresponding age and size at those growth layers represent the timing of maturity (Table 1). For these ten turtles, the SSM ranged from 61.2 to 89.5 cm CCL (mean ± SE 76.9 ± 2.76, coefficient of variation, CV = 0.11) and ASM ranged from 16 to 26 years (mean ± SE 19.5 ± 1.01, CV = 0.16), with the reproductive longevity (estimated as the number of LAGs or years beyond the onset of maturity) observed at a maximum 33 years (Table 1). Mean annual growth post-maturation was 0.24 cm/year (n = 108 growth layers). Seven of the 10 mature turtles were female, and were all recovered from Bahía de Jiquilisco. Two turtles were male and one turtle was unknown sex, and all three of these were recovered from Punta Amapala (Figure 3). The turtle with the largest SSM (89.5 cm CCL) was a female from Bahía de Jiquilisco with an estimated ASM of 16 years, and the turtle with the smallest SSM (61.2 cm CCL) was a male from Punta Amapala with ASM of 22 years (Table 1).
The GAMM smoothing spline, fit to the length-at-age dataset of the 70 turtles, was significant (p < 0.0001, Edf = 7.457, adj. r2 = 0.887; Figure 4). The mean estimated SSM obtained at rapprochement for the 10 mature bones analyzed in the current study (76.9 cm CCL) corresponded to a spline-predicted ASM of 20 years (95% CI: 18 to 23 years). The minimum SSM from rapprochement, 61.2 cm CCL, yielded a spline-predicted ASM of ∼11.5 years (Figure 4 and Table 1), and the mean nesting size for hawksbills in this region (81.6 cm CCL, Liles et al., 2011) yielded a spline-estimated ASM of ∼35 years (the ASM estimated by skeletochronology for a turtle with SSM near this size was 21 years for a 81.7 cm CCL female from Bahía de Jiquilisco; Table 1). The bootstrapped von Bertalanffy model, based on growth-at-length, estimated the ASM for the mean SSM (76.9 cm CCL) from rapprochement in this study at 22.5 ± 0.21 years (95% CI: 22.1 to 22.9 years), the estimated upper size limit, Linf, was 80.6 ± 0.19 cm (95% CI: 80.2 to 81.0 cm) and the intrinsic growth rate parameter, k, was 0.142 ± 0.0008 (95% CI: 0.141 to 0.144; Figure 4).
Stable isotope analysis and habitat use
We processed a total of 58 skin samples for the current study, 15 from Bahía de Jiquilisco (six of these were hatchlings: CL mean 3.8 cm; and the rest juveniles and adults: n = 9, CCL 39.7 to 84.5, mean 55.5 cm), and 43 from Punta Amapala (all juveniles CCL 32.9 to 53.6, mean 40.0 cm). The stable carbon isotope (δ13C) values of the samples from turtles captured in the mangrove estuary (Bahía de Jiquilisco) were lower in comparison to the δ13C values of the skin from turtles captured outside the estuary (Punta Amapala), mean ± SE –20.5 ± 5.29‰ vs. –16.2 ± 2.47‰, respectively, while the δ15N values were similar (Table 2). All skin samples collected from turtles in Punta Amapala had δ13C values greater than the designated threshold value of –18‰, further supporting the use of this value as an indicator of movement into a mangrove estuary.
Of the 70 turtles analyzed for age and size using skeletochronology, a subset of 40 bones were analyzed for sequential stable isotope analysis (20 recovered from Bahía de Jiquilisco and 20 from Punta Amapala). The size of the 20 turtles sampled from Bahía de Jiquilisco ranged from 35.4 to 90.7 cm CCL, with very few (6 out of 20) large turtles > 70 cm CCL (median 59.2 cm CCL), while the turtles from Punta Amapala ranged from 28.5 to 73.6 cm CCL (median 47.0 cm CCL, 3 out of 20 were > 70 cm CCL); from each group, a total of 95 and 89 individual growth layers were subsampled for SIA, respectively. The stable carbon isotope (δ13C) values for all samples (n = 184) ranged from –27.1 to –11.9‰ (mean ± SE –16.9 ± 1.25‰) and the stable nitrogen isotope (δ15N) values ranged from 9.7 to 18.0‰ (mean ± SE 13.6 ± 1.00‰; Table 2). When separated by final stranding location, the δ13C values (n = 95) ranged from –27.1 to –14.4 ‰ (mean ± SE –18.7 ± 1.92‰) and the δ15N values ranged from 9.7 to 15.6‰ (mean ± SE 13.0 ± 1.33‰) for the 20 turtles recovered in Bahía de Jiquilisco, while the samples from the 20 turtles recovered from Punta Amapala (n = 89) had δ13C values that ranged from –22.1 to –11.9‰ (mean ± SE –15.0 ± 1.60‰) and δ15N values that ranged from 11.1 to 18.0‰ (mean ± SE 14.3 ± 1.52‰; Table 2).
Using the SIA-assigned habitats and corresponding age and size estimates for each growth layer, multiyear habitat-use patterns were observed. All turtles, regardless of final stranding location, had δ13C values above the –18‰ threshold during years where body size was less than ca. 35 cm CCL (age range ca. 0–5 years), representative of spent time outside of the mangrove estuaries during these early juvenile years (mean ± SE δ13C: –15.0 ± 1.8‰ and δ15N: 14.1 ± 1.7‰, n = 30 turtles, 71 growth layers; Figure 5). The SIA values of growth layers with associated body size ca. > 35 cm showed divergent patterns, with some turtles’ δ13C values higher than the –18‰ threshold, associated with ocean and coastal rocky reef habitats (mean ± SE –15.3 ± 2.0‰ n = 22 turtles, 61 growth layers), while other turtles had δ13C values lower than –18‰, associated with the mangrove estuaries (mean ± SE –22.0 ± 3.2 ‰, n = 17 turtles, 48 growth layers; Figure 5). These same growth layer groups also had the same pattern for δ15N values, mean ± SE 14.4 ± 1.9‰ (n = 22 turtles, 61 growth layers) for those with body size ca. > 35 cm, and mean ± SE 11.8 ± 1.7‰ for the larger body sizes (n = 17 turtles, 48 growth layers; Figure 5).
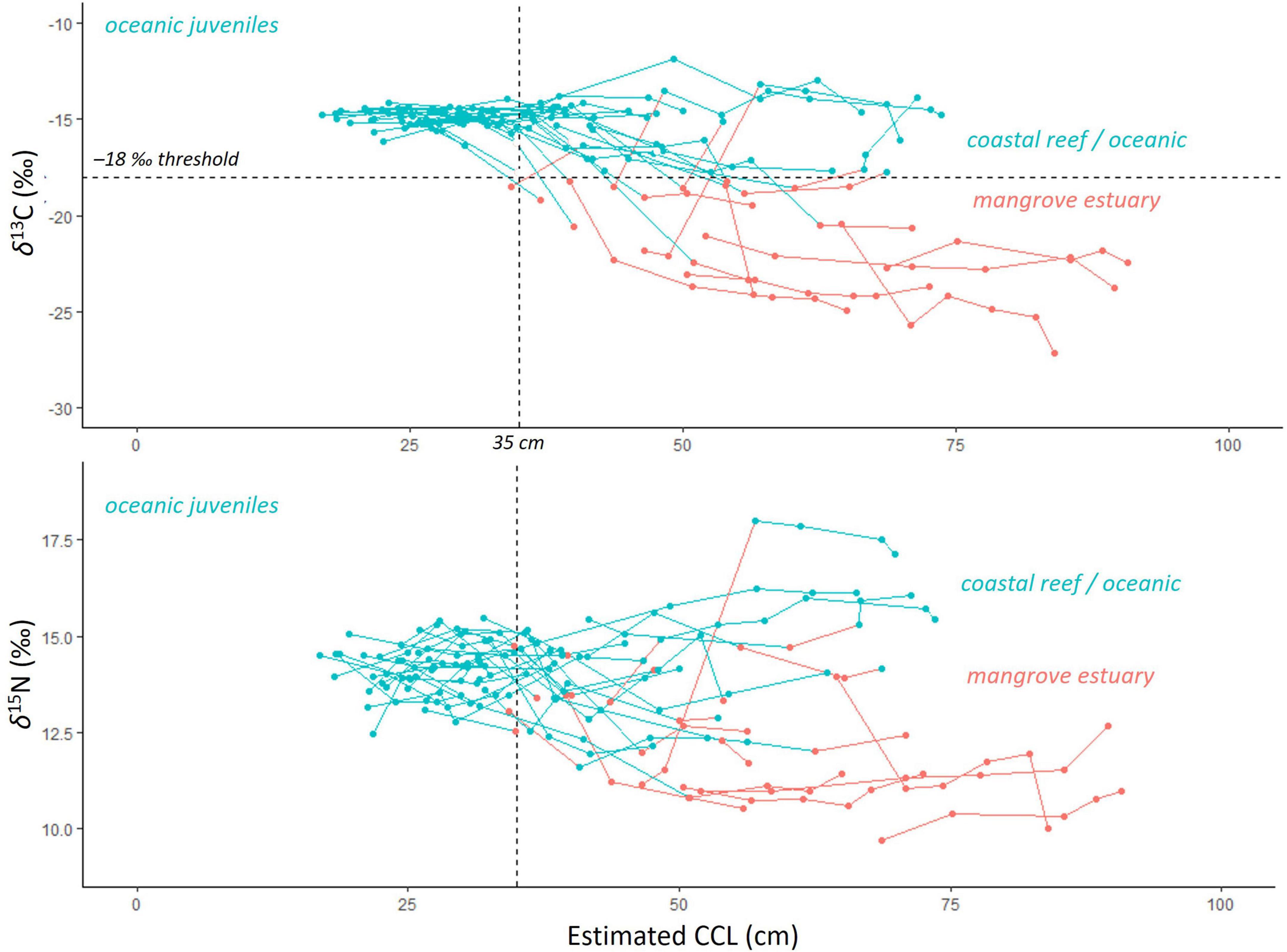
Figure 5. All stable isotope data, aligned with body size (curved carapace length, CCL, cm) for the 40 turtles processed by skeletochronology and then sampled for stable carbon (δ13C, top) and nitrogen (δ15N, bottom) isotope analysis. Lines show data for individual turtles. The threshold value of –18‰ δ13C is shown as the dashed horizontal line on the top plot, with values above representing the ocean / coastal reef habitat (blue), while the values below indicate mangrove estuary habitat (red). The δ15N values from the corresponding growth layers are color coded to indicate the δ13C-assigned habitat of “ocean” (> –18‰ δ13C) or “estuary” (< –18‰ δ13C). The 35 cm CCL body size is shown as the vertical dashed line and marks the size at which juveniles begin to recruit to near shore habitats.
By identifying the earliest (innermost) growth layer with δ13C values lower than the –18‰ threshold value, the timing of this habitat shift into the mangrove estuary was identified for 16 turtles recovered in Bahía de Jiquilisco (Figure 5 and Supplementary Figures 3A,B), which ranged from age 3–13 years old (mean ± SE 7.0 ± 0.69 years) and CCL from 34.8 to 64.8 cm (mean ± SE 45.9 ± 2.51 cm). The other four turtles sampled for SIA and recovered from Bahía de Jiquilisco had only stable isotope values associated with mangrove estuary habitat use, indicative of consistent, long-term habitat use (final ages: 20, 37, 18, 30 years; final CCLs: 72.8, 84.3, 89.5, 90.7 cm). The residency duration in mangrove estuarine habitats (likely in Bahía de Jiquilisco) for these four turtles ranged from 8 to 21 years (Figure 5 and Supplementary Figures 3A,B).
Of the 20 turtles recovered at Punta Amapala and sampled for SIA, 84 out of the 89 growth layer samples had δ13C values higher than the mangrove threshold value of –18‰, indicating near-exclusive use of habitats outside of mangrove estuaries for these turtles recovered at Punta Amapala’s rocky reef (Figure 5 and Supplementary Figures 3C,D). The five growth layer samples below the –18‰ threshold (–22.1 to –18.5‰) were collected from four different turtles, suggesting at least 1 year of previous mangrove estuary habitat use by these turtles during mid-juvenile years (ages: 7, 7, 8, 9, 14 years old; CCLs: 43.6, 50.0, 46.5, 48.6, 60.2 cm). Yet all four turtles showed several years of consistent habitat use and residency/fidelity outside of estuaries during their most recent years prior to stranding (Figure 5 and Supplementary Figures 3C,D).
Finally, when we compared the incremental growth rate data associated with the same size class groups but assigned to different habitats (mangrove estuary vs. ocean/rocky reef), we found slight growth advantages for turtles in the mangrove estuary of Bahía de Jiquilisco for two size class groups, 60–70 cm and 70–80 cm CCL (p ≤ 0.0001, Wilcoxon Rank Sum; Figure 6).
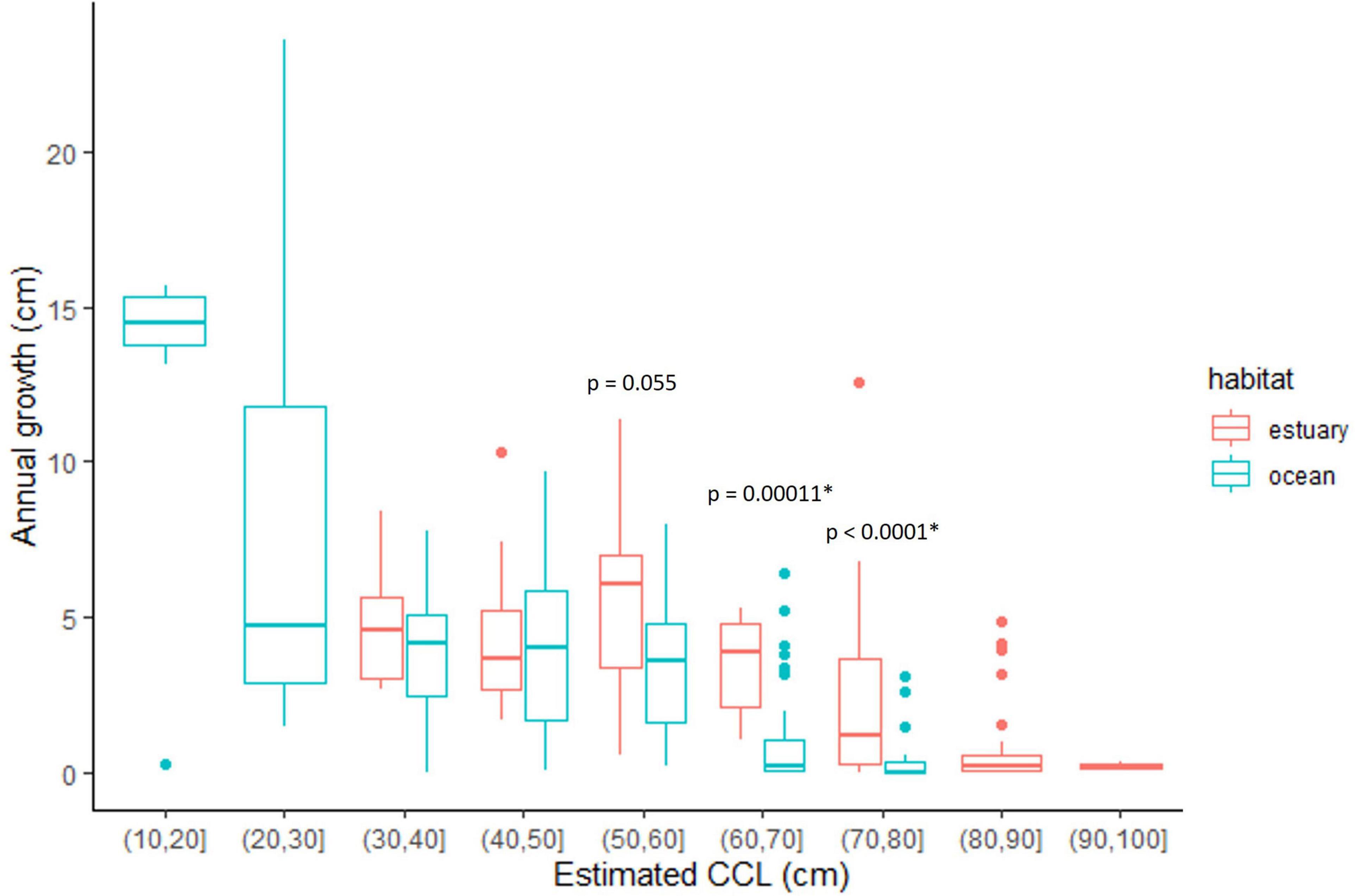
Figure 6. Annual growth (cm/yr) for growth layers assigned to a habitat–either mangrove estuary (in red), or rocky reef/ocean (in blue)– based on stable carbon isotope values (δ13C). The p-values show the results from the Mann–Whitney comparison tests for the relevant size-class groups. *Indicates p-values are significant at p < 0.05.
Discussion
Here we present useful demographic and population ecology parameters for hawksbills in the EP using skeletochronology, in addition to multi-year movement and habitat residency patterns using complementary stable isotope analysis (skeleto+iso). Our empirical estimates on the maturation timing for this population show maturity occurring between 16 and 26 years old, with potential differences between nesting and foraging location (mangrove estuary vs. open coast), dietary preference and between sexes (males vs. females) that are worth future investigations. The non-estuary stable isotope signal that was found consistently in the age 0–3 growth layers strongly suggests that this hawksbill population does not immediately settle into the mangrove estuary, but instead has an offshore, if not oceanic, juvenile stage.
Maturation
The maturation age range of 16–26 years, based on rapprochement, came from only 10 adult individuals, yet was similar to what has been estimated for other hawksbill populations in previous studies. Snover et al. (2012) and Avens et al. (2021) both used skeletochronology and reported ASM between 15 and 25 years and 17–22 for hawksbills in the western North Atlantic and Hawaii, respectively. Various mark-recapture studies have estimated hawksbill ASM ranging from 13 to 20 years (e.g., Yucatan region, Garduño-Andrade et al., 1999; Brazil, Bellini et al., 2019), and 14–24 years based on genetic inference for hawksbills nesting in Antigua [Levasseur et al., 2021; and see Avens et al. (2020) for further insight on ASM estimates]. These estimates are also substantially lower than the estimated average ASM reported for hawksbills in the Indo-Pacific region based on somatic growth rates (31–36 years; Limpus and Miller, 2000). The empirical results from the current study provide a baseline for this east Pacific population, and ideally the ASM estimate will be further refined in the future with increased adult samples for skeletochronology and validated using genetic fingerprinting (i.e., when a genetically sampled hatchling returns to nest as a genetically identified neophyte nester). Continued monitoring of both mangrove and open beach nesting sites will also help refine the demographic parameters estimated here, including reproductive longevity.
Based on the number of LAGs observed beyond rapprochement, estimated reproductive longevity ranged from 2 to 33 years (Table 1), and this estimated maximum longevity beyond maturation is in the mid-range of what has been found for several other sea turtle species (Avens and Snover, 2013; Kemp’s ridleys Lepidochelys kempii, 10 years, Avens et al., 2017; flatbacks Natator depressus, 31 years, Turner Tomaszewicz et al. in press; leatherbacks Dermochelys coriacea, 18–22 years, max 31 years, Avens et al., 2020; green turtles, > 40 years, Limpus and Chaloupka, 1997, 27 years, Turner Tomaszewicz et al., 2022; and loggerheads, 46 years, Avens et al., 2015). To date, nesting site monitoring at Bahía de Jiquilisco, which began in 2008, has recorded at least one turtle nesting for 14 years (n = 177 turtles tagged), and of the 27 turtles tagged while nesting at Punta Amapala since 2015, seven is the maximum number of years a turtle has been observed returning to nest (M. Liles, ProCosta, unpub.).
Given the range of ASM and SSM observed in the current study, future work should also focus on examining potential difference in growth rates and maturation timing between sexes and foraging location (see section below). The seven mature females in the current study were all recovered from the mangrove estuary (Bahía de Jiquilisco) and had estimated ASM ranging from 16 to 26 years (mean ± SE 19.3 ± 1.27 years), and SSM ranging from 72.2 to 89.5 cm CCL (mean ± SE 81.1 ± 2.14 cm). Unfortunately, there were no mature male turtles recovered from Bahía de Jiquilisco, only two males that were 65.1 and 84.5 cm CCL and 10 and 19 years old, respectively. Yet the larger, older male turtle was likely nearing maturity given that its body size and age was within the range observed for mature females from Bahía de Jiquilisco (Figure 3). This suggests similar sizes for adult males and females within the estuary (Figure 3).
At Punta Amapala, the two mature males recovered (71.1 and 72 cm CCL, and age 21 and 54 years old, respectively) were smaller than most of the mature turtles recovered at Bahía de Jiquilisco, and unfortunately there were no known females recovered at this location. The 54-year-old male was the oldest turtle aged in the current study, and suggests that at least some adult male turtles at Punta Amapala are smaller than those found in the mangrove habitat. This potential pattern is also observed for nesting females at the different sites. On-site monitoring of turtle body sizes at these nesting sites do show some potential size differences, with nesters at Bahía de Jiquilisco typically being larger (range: 68.2–98 cm, mean 83.5 cm CCL, n = 177) than those nesting at Punta Amapala (62.2–91 cm, mean 76.7 cm CCL, n = 27) and another open coast (non-estuary) site slightly north (Los Cóbanos: 63–97 cm, mean 80.5 cm CCL, n = 67; M. Liles, ProCosta, unpubl.). We acknowledge that the skeletochronology sample sizes are small, but together with the nesting size data, there is reason to encourage further research to explore the possibility of size difference by foraging and/or nesting site and to more fully characterize these important sex- and location-specific demographics.
Habitat use and movement patterns
The combination of sequential stable isotope analysis with skeletochronology facilitated the recreation of multi-year movement and habitat use patterns for 40 hawksbills. From this skeleto+iso analysis, three key habitat use patterns were revealed: (1) All post-hatchlings have a non-estuary juvenile stage where they likely occupy oceanic habitats for a minimum 1–3 years; (2) Juvenile turtles (∼35 cm CCL) then recruit to neritic habitats, but in at least two divergent ways—some settling into the nearshore rocky reefs and some settling into the mangrove estuaries; and (3) Turtles appear to have high fidelity to their selected habitat, with very little movement between the two habitats observed.
First, the non-estuary stable isotope signal, characterized by δ13C values greater than –18‰, was found consistently in all the age 0–3 growth layers, regardless of where the turtle eventually stranded. Previous speculation on post-hatchling movement has questioned if some hatchlings from mangrove nests might have short-dispersal oceanic developmental periods (Gaos et al., 2017) or perhaps even remain within the estuary, growing rapidly and never undertaking an oceanic juvenile stage characteristic of most hard-shelled sea turtle species (Bolten, 2003). However, findings here strongly suggest that this is not the case—that hawksbills in this population do not immediately settle into the mangrove estuary, but instead spend at least a few years in an offshore, if not oceanic, habitat during their post-hatchling juvenile stage. These results are also supported by the SIA of hawksbill scute layers by Wedemeyer-Strombel et al. (2021) which also found higher δ13C values in the earliest scute layers. This offshore movement pattern is further supported when the growth rates estimated in the current study are related to the length-frequency distribution of turtles found in both the mangrove estuary and the rocky reef habitats. In both Bahía de Jiquilisco and the rocky reefs at Punta Amapala, other than newly emerged hatchlings, no turtles < 14 cm CCL have ever been observed since more regular community-based monitoring in the area around 2007 (Gaos et al., 2010), and very few turtles < 30 cm CCL have been encountered (Liles et al., 2017).
In Bahía de Jiquilisco, the smallest turtle encountered was 35.4 cm CCL; in Punta Amapala, the average size retrieved from gillnets during Liles et al. (2017) was 31.6 cm CCL ± 14.7 S.D. (n = 20), and the smallest turtle used in the current study was 28.5 cm CCL and recovered at Punta Amapala. Using skeletochronology, the back calculated body sizes at age 1 for turtles found across both sites (n = 38) ranged in size from 14.8 to 28.2 cm CCL (mean 21.2 ± 3.4 cm), and at age 2 (n = 44) CCL ranged from 19.0 to 32.7 cm (mean 26.3 ± 4.0 cm). These sizes are similar to the size achieved at 1-year for three captive raised hawksbills collected from relocated nests in Bahía de Jiquilisco (364 days, straight carapace length, SCLs: 18.9, 23.7, 26.0 cm CCL; M. Liles, ProCosta, unpubl.). Together, these findings suggest that turtles are at least a year old when they recruit to neritic habitats after having spent time in oceanic regions.
Upon recruitment to a nearshore habitat, the turtles then appear to diverge in their habitat use patterns, with turtles of similar size, ca. 35 cm CCL, selecting different habitats in which to settle—in this case, either a mangrove estuary or rocky reef habitat. The mechanisms driving this divergent behavior are not clear and should be explored further. The selection of settlement habitat (estuary or reef) may be influenced by a variety of factors including physical environmental conditions (e.g., currents, water temperature, food availability), biological predisposition (e.g., genetics) or perhaps habitat-type is selected by chance (e.g., Bolten, 2003; Chevis et al., 2017; Griffin et al., 2020). Once turtles do settle into one of these neritic habitats, it is likely that they remain as foragers in that habitat-type through the rest of their lives, given that the adult turtles showed consistent habitat use with long term (10–30 years) residency durations. The four adult turtles from Bahía de Jiquilisco all showed constant low δ13C and δ15N values indicating mangrove estuary residency, and the two adult turtles from Punta Amapala that had constant high δ13C and δ15N values indicating residency outside of mangrove estuaries, likely at the rocky reefs along the open coast (Figure 5 and Supplementary Figure 4). In addition to the large mangrove estuary at Bahía de Jiquilisco, there are other similar estuaries in the region, also known to have foraging and nesting hawksbills, including Barra de Santiago to the north (Massey and McCord, 2017) and Estero Padre Ramos in Nicaragua to the south (Liles et al., 2011; Gaos et al., 2012b), and it is possible there is movement of foraging turtles between these different estuaries. Turtles inhabiting these nearby mangrove habitats are known to have stable carbon isotope values similar to those in Bahía de Jiquilisco (Wedemeyer-Strombel et al., 2021), so it is possible that some mangrove-foraging turtles do move between different mangrove estuaries. However, currently, there is more evidence that hawksbills have fidelity to just one mangrove estuary, as demonstrated by genetic studies, long-term recapture, and nesting histories showing high levels of natal foraging philopatry (Gaos et al., 2017, 2018).
Yet inspection of the SIA values of individual turtles also reveals more intricate patterns, including the potential for some individuals to undertake movements between the estuary and reef habitats. For instance, one of the Punta Amapala adult turtles that showed long term rocky reef (non-estuary) habitat use from the age of ca. 17 to death at 54 years old, had apparently spent time in the mangrove estuaries between the age of ca. 10 to ca. 17 (ca. 45–55 cm CCL; Figure 5 and Supplementary Figure 4). It is therefore likely that some flexibility exists within this population with regard to habitat use. Continuing research employing tagging (e.g., flipper, PIT, satellite) will help to further elucidate these complex movement patterns for east Pacific hawksbills in this region and to begin to identify the drivers and implications of these variable habitat use strategies.
Growth rates among different habitats
Overall, the annual growth rates were highest for the youngest (<5 years) and smallest turtles (<30 cm CCL), then gradually slowed as turtles grew and eventually approached maturity, a growth pattern typical for sea turtles (Figures 4, 6). Sea turtle growth is known to be affected by environmental conditions such as temperature and food availability and quality (Chaloupka and Musick, 1997; Balazs and Chaloupka, 2004; Eguchi et al., 2012), and has also been shown to be density dependent (Bjorndal et al., 2000). Stable isotopes are a useful tool to help better examine the potential effects some of these factors—particularly food and habitat—may have on growth. In the current study, the incremental growth data of the mid-size classes (50–80 cm CCL) suggests that habitat selection may affect growth, with a potential growth-advantage for those turtles settling into mangrove estuaries (Figure 6). It is unknown how or if this difference in growth rates may impact the ultimate fecundity of turtles once they become adults, but the pattern should be examined further to determine if there are somatic growth consequences of habitat choice.
Based on other trends documented for sea turtles, increased growth, for example, could result in (1) earlier maturation (lower ASM) and/or (2) larger size of maturation (larger SSM), both of which could translate to higher reproductive output if reproductive longevity is longer (given the lower ASM), or if clutch size is larger (given the larger SSM). These potential age- and size-related life history characteristics should be the focus of future work, as these differences have implications for population growth and ecology of this recovering stock.
Conservation implications
These long-term habitat use patterns and estimated demographic parameters are important in helping to understand the life history of this population and in guiding conservation efforts to prioritize critical habitat. Offshore and coastal rocky reef habitats are likely used by all hawksbills in this population during an early juvenile stage, and fishing and other anthropogenic activities that take place in these waters should be monitored to minimize impact to these turtles. Similarly, the age- and size-specific habitat use patterns showed that mangrove estuaries are very important, not only for providing long term habitats, but also by potentially facilitating rapid growth, which may positively affect the population’s recovery rate. Continued community engagement that is focused on monitoring hawksbills in these nearshore habitats while also working to minimize mortality (fishing-related bycatch, entanglement, blast fishing; and egg harvesting) has already made incredible progress in the past 15 years and is essential to the continuing recovery of this population.
Data availability statement
The original contributions presented in the study are included in the article/Supplementary material, further inquiries can be directed to the corresponding author/s.
Ethics statement
Ethical review and approval was not required for the animal study because all tissue samples used in this study were collected from already dead (stranded) sea turtles, with all appropriate collection and export/import permits in place.
Author contributions
CTT, JS, and ML conceived of the study. ML coordinated the sample collection in the field. CTT conducted the lab work, data analysis, and wrote the first draft of the manuscript. LA contributed to the skeletochronology data analysis. All authors revised and helped to edit the final manuscript.
Funding
This work was supported by a NOAA—NMFS Sea Turtle Stock Assessment Award 18–19, which supported CTT during a National Research Council Research Associateship.
Acknowledgments
We acknowledge the dedicated members of ProCosta in El Salvador for collecting, cleaning, and drying the hundreds of samples necessary for this study, especially Sofia Chavarria, Ani Henriquez, Carlos Pacheco, Marivn Pineda, Neftaly Sanchez, and Melissa Valle. At the Southwest Fisheries Science Center, we thank Allison Johnson and Toni Lohroff for assistance in processing the bone and skin samples, and Erin LaCasella for guiding the CITES sample import process.
Conflict of interest
The authors declare that the research was conducted in the absence of any commercial or financial relationships that could be construed as a potential conflict of interest.
Publisher’s note
All claims expressed in this article are solely those of the authors and do not necessarily represent those of their affiliated organizations, or those of the publisher, the editors and the reviewers. Any product that may be evaluated in this article, or claim that may be made by its manufacturer, is not guaranteed or endorsed by the publisher.
Supplementary material
The Supplementary Material for this article can be found online at: https://www.frontiersin.org/articles/10.3389/fevo.2022.983260/full#supplementary-material
References
Allen, C. D., Lemons, G. E., Eguchi, T., LeRoux, R. A., Fahy, C. C., Dutton, P. H., et al. (2013). Stable isotope analysis reveals migratory origin of loggerhead turtles in the Southern California Bight. Mar. Ecol. Prog. Ser. 472, 275–285. doi: 10.3354/meps10023
Avens, L., Goshe, L. R., Coggins, L., Shaver, D. J., Higgins, B., Landry, A. M., et al. (2017). Variability in age and size at maturation, reproductive longevity, and long-term growth dynamics for Kemp’s ridley sea turtles in the Gulf of Mexico. PLoS One 12:173999. doi: 10.1371/journal.pone.0173999
Avens, L., Goshe, L. R., Harms, C. A., Anderson, E. T., Hall, A. G., Cluse, W. M., et al. (2012). Population characteristics, age structure, and growth dynamics of neritic juvenile green turtles in the northeastern Gulf of Mexico. Mar. Ecol. Prog. Ser. 458, 213–229. doi: 10.3354/meps09720
Avens, L., Goshe, L. R., Pajuelo, M., Bjorndal, K. A., MacDonald, B. D., Lemons, G. E., et al. (2013). Complementary skeletochronology and stable isotope analyses offer new insight into juvenile loggerhead sea turtle oceanic stage duration and growth dynamics. Mar. Ecol. Prog. Ser. 491, 235–251. doi: 10.3354/meps10454
Avens, L., Goshe, L. R., Zug, G. R., Balazs, G. H., Benson, S. R., and Harris, H. (2020). Regional comparison of leatherback sea turtle maturation attributes and reproductive longevity. Mar. Biol. 167, 1–12. doi: 10.1007/s00227-019-3617-y
Avens, L., Ramirez, M. D., Goshe, L. R., Clark, J. M., Meylan, A. B., Teas, M., et al. (2021). Hawksbill sea turtle life-stage durations, somatic growth patterns, and age at maturation. Endang Species Res. 45, 127–145. doi: 10.3354/esr01123
Avens, L., and Snover, M. (2013). “Age and age estimation in sea turtles,” in Biology of Sea Turtles Volume III, eds J. Wyneken, K. J. Lohmann, and J. A. Musick (Boca Raton, FL: CRC Press), 97–133.
Avens, L. A., Goshe, L. R., Coggins, L., Snover, M. L., Pajuelo, M., Bjorndal, K. A., et al. (2015). Age and size at maturation- and adult-stage duration for loggerhead sea turtles in the western North Atlantic. Mar. Biol. 162, 1749–1767. doi: 10.1007/s00227-015-2705-x
Balazs, G. H., and Chaloupka, M. (2004). Spatial and temporal variability in somatic growth of green sea turtles (Chelonia mydas) resident in the Hawaiian Archipelago. Mar. Biol. 145, 1043–1059. doi: 10.1007/s00227-004-1387-6
Bellini, C., Santos, A. J. B., Patrício, A. R., Bortolon, L. F. W., Godley, B. J., Marcovaldi, M. A., et al. (2019). Distribution and growth rates of immature hawksbill turtles Eretmochelys imbricata in Fernando de Noronha, Brazil. Endang. Species Res. 40, 41–52. doi: 10.3354/esr00979
Bjorndal, K. A., Bolten, A. B., and Chaloupka, M. Y. (2000). Green turtle somatic growth model: evidence for density-dependence. Ecol. Appl. 10, 269–282. doi: 10.1890/1051-0761(2000)010[0269:GTSGME]2.0.CO;2
Bolten, A. B. (2003). “Variation in sea turtle life history patterns: neritic vs. oceanic developmental stages,” in The Biology of Sea Turtles, Vol. II, eds P. L. Lutz, J. Musick, and J. Wyneken (Boca Raton, FL: CRC Press), 243–257. doi: 10.1201/9781420040807.ch9
Bouillon, S., Connolly, R. M., and Lee, S. Y. (2008). Organic matter exchange and cycling in mangrove ecosystems: recent insights from stable isotope studies. J. Sea Res. 59, 44–58. doi: 10.1016/j.seares.2007.05.001
Chaloupka, M. Y., and Musick, J. A. (1997). “Age, growth and population dynamics,” in The Biology of Sea Turtles, Chap. 9, eds P. L. Lutz and J. A. Musick (Boca Raton, FL: CRC Press), 233–276.
Chevis, M. G., Godley, B. J., Lewis, J. P., Lewis, J. J., Scales, K. L., and Graham, R. T. (2017). Movement patterns of juvenile hawksbill turtles Eretmochelys imbricata at a Caribbean coral atoll: long-term tracking using passive acoustic telemetry. Endang. Species Res. 32, 309–319. doi: 10.3354/esr00812
DeNiro, M. J., and Epstein, S. (1978). Influence of diet on the distribution of carbon isotopes in animals. Geochim. Cosmochim. Acta 42, 495–506. doi: 10.1016/0016-7037(78)90199-0
Deutsch, C. A., Gruber, N. P., Key, R. M., Sarmiento, J. L., and Ganachaud, A. (2011). Denitrification and N2 fixation in the Pacific Ocean. Global Biogeochem. Cycles 15, 483–506. doi: 10.1029/2000GB001291
Domínguez-Miranda, J. P. (2010). Caracterización Biofísca del área marina frente a Playa Las Tunas, Playas Negras, Playas Blancas, Playa Maculís, y las Mueludas, Municipio de Conchagua, Departamento de La Unión, El Salvador. San Salvador: USAID, 83.
Dunbar, S. G., Anger, E. C., Parham, J. R., Kingen, C., Wright, M. K., Hayes, C. T., et al. (2021). HotSpotter: using a computer-driven photo-id application to identify sea turtles. J. Exp. Mar. Biol. Ecol. 535, 151490. doi: 10.1016/j.jembe.2020.151490
Eguchi, T., Seminoff, J. A., Leroux, R. A., Dutton, D. L., and Dutton, P. H. (2012). Morphology and growth rates of the green sea turtle (Chelonia mydas) in a northern-most temperate foraging ground. Herpetologica 68, 76–87. doi: 10.1655/HERPETOLOGICA-D-11-00050.1
Espinasse, B., Hunt, B. P. V., Batten, S. D., and Pakhomov, E. A. (2019). Defining isoscapes in the Northeast Pacific as an index of ocean productivity. Global Ecol. Biogeogr. 29, 246–261. doi: 10.1111/geb.13022
Fleming, A. H., Clark, C. T., Calambokidis, J., and Barlow, J. (2016). Humpback whale diets respond to variance in ocean climate and ecosystem conditions in the California Current. Glob. Change Biol. 22, 1214–1224. doi: 10.1111/gcb.13171
France, R. L. (1995). Carbon-13 enrichment in benthic compared to planktonic algae: foodweb implications. Mar. Ecol. Prog. Ser. 124, 307–312. doi: 10.3354/meps124307
Francillon-Vieillot, H., Arntzen, J. W., and Géraudie, J. (1990). Age, growth and longevity of sympatric Triturus cristatus T. marmoratus and their hybrids (Amphibia, Urodela): A Skeletochronological Comparison. J. Herpetol. 24, 13–22. doi: 10.2307/1564284
Francis, R. I. C. C. (1990). Back-calculation of fish length: A critical review. J. Fish Biol. 36, 883–902. doi: 10.1111/j.1095-8649.1990.tb05636.x
Fry, B. (2006). Stable Isotope Ecology. New York, NY: Springer New York, 308. doi: 10.1007/0-387-33745-8
Fry, B., and Ewel, K. C. (2003). Using stable isotopes in mangrove fisheries research - a review and outlook. Isotopes Environ. Health Stud. 39, 191–196. doi: 10.1080/10256010310001601067
Gaos, A. R., Abreu-Grobois, F. A., Alfaro-Shigueto, J., Amorocho, D., Arauz, R., Baquero, A., et al. (2010). Signs of hope in the eastern Pacific: international collaboration reveals encouraging status for a severely depleted population of hawksbill turtles Eretmochelys imbricata. Oryx 44, 595–601. doi: 10.1017/S0030605310000773
Gaos, A. R., Lewison, R. L., Jensen, M. P., Liles, M. J., Henriquez, A., Chavarria, S., et al. (2017). Natal foraging philopatry in eastern Pacific hawksbill turtles. R. Soc. Open Sci. 4:170153. doi: 10.1098/rsos.170153
Gaos, A. R., Lewison, R. L., Jensen, M. P., Liles, M. J., Henriquez, A., Chavarria, S., et al. (2018). Rookery contributions, movements and conservation needs of hawksbill turtles at foraging grounds in the eastern Pacific Ocean. Mar. Ecol. Prog. Ser. 586, 203–2016. doi: 10.3354/meps12391
Gaos, A. R., Lewison, R. L., Yañez, I. L., Wallace, B. P., Liles, M. J., Nichols, W. J., et al. (2012a). Shifting the life-history paradigm: discovery of novel habitat use by hawksbill turtles. Biol. Lett. 8, 54–56. doi: 10.1098/rsbl.2011.0603
Gaos, A. R., Lewison, R. L., Wallace, B. P., Yañez, I. L., Liles, M. J., Nichols, W. J., et al. (2012b). Spatial ecology of critically endangered hawksbill turtles Eretmochelys imbricata: implications for management and conservation. Mar. Ecol. Prog. Ser. 450, 181–194. doi: 10.3354/meps09591
Gaos, A. R., and Yañez, I. L. (2012). “Saving the eastern Pacific hawksbill from extinction: Last chance or chance lost?,” in Sea Turtles of the Eastern Pacific: Advances in Research and Conservation, eds J. A. Seminoff and B. P. Wallace (Tucson: University of Arizona Press), 244–262. doi: 10.2307/j.ctv21hrddc.14
Garduño-Andrade, M., Guzman, V., Miranda, E., Briseño-Dueñas, R., and Abreu-Grobois, F. A. (1999). Increases in hawksbill turtle (Eretmochelys imbricata) nestings in the Yucatan Peninsula, Mexico, 1977-1996: data in support of successful conservation? Chelonian Conserv. Biol. 3, 286–295.
Godley, B. J., Blumenthal, J. M., Broderick, A. C., Coyne, M. S., Godfrey, M. H., Hawkes, L. A., et al. (2008). Satellite tracking of sea turtles: where have we been and where do we go next? Endanger. Species Res. 4, 3–22. doi: 10.3354/esr00060
Goshe, L. R., Avens, L., Scharf, F. S., and Southwood, A. L. (2010). Estimation of age at maturation and growth of Atlantic green turtles (Chelonia mydas) using skeletochronology. Mar. Biol. 157, 1725–1740. doi: 10.1007/s00227-010-1446-0
Goshe, L. R., Avens, L., Snover, M. L., and Hohn, A. A. (2020). “Protocol for processing sea turtle bones for age estimation,” in Proceedings of the NOAA Technical Memorandum NMFS-SEFSC, 43. (Washington, DC: NOAA),
Goshe, L. R., Snover, M. L., Hohn, A. A., and Balazs, G. H. (2016). Validation of back-calculated body lengths and timing of growth mark deposition in Hawaiian green sea turtles. Ecol. Evol. 6, 3208–3215. doi: 10.1002/ece3.2108
Griffin, L. P., Smith, B. J., Cherkiss, M. S., Crowder, A. G., Pollock, C. G., Hillis-Starr, Z., et al. (2020). Space use and relative habitat selection for immature green turtles within a Caribbean marine protected area. Anim. Biotelemetry 8:22. doi: 10.1186/s40317-020-00209-9
Hanna, M. E., Chandler, E. M., Semmens, B. X., Eguchi, T., Lemons, G. E., and Seminoff, J. A. (2021). Citizen-sourced sightings and underwater photography reveal novel insights about green sea turtle distribution and ecology in Southern California. Front. Mar. Sci. 8:671061. doi: 10.3389/fmars.2021.671061
Hastie, T. J., and Tibshirani, R. J. (1990). “Generalized additive models,” in Monographs on Statistics and Applied Probability, Ed. G. Wetherill (London: Chapman and Hall), 43.
Hawkes, L. A., Tomas, J., Revuelta, O., Leon, Y. M., Blumenthal, J. M., Broderick, A. C., et al. (2012). Migratory patterns in hawksbill turtles described by satellite tracking. Mar. Ecol. Prog. Ser. 461, 223–232. doi: 10.3354/meps09778
Hays, G. C., and Hawkes, L. A. (2018). Satellite tracking sea turtles: opportunities and challenges to address key questions. Front. Mar. Sci. 5:432. doi: 10.3389/fmars.2018.00432
Hobson, K. A., and Wassenaar, L. I. (eds) (2018). Tracking Animal Migration with Stable Isotopes. Cambridge, MA: Academic Press. doi: 10.1016/B978-0-12-814723-8.00001-5
LaCasella, E. L., Jensen, M. P., Madden, H., Bell, I. P., Frey, A., and Dutton, P. H. (2021). Mitochondrial DNA profiling to combat the illegal trade in tortoiseshell products. Front. Mar. Sci. 7:595853. doi: 10.3389/fmars.2020.595853
Levasseur, K. E., Stapleton, S. P., and Quattro, J. M. (2021). Precise natal homing and an estimate of age at sexual maturity in hawksbill turtles. Anim. Conserv. 24, 523–535. doi: 10.1111/acv.12657
Liles, M. J., Gaos, A. R., Bolanos, A. D., Lopez, W. A., Arauz, R., Gadea, V., et al. (2017). Survival on the rocks: high bycatch in lobster gillnet fisheries threatens hawksbill turtles on rocky reefs along the Eastern Pacific coast of Central America. Latin Am. J. Aquat. Res. 45, 521–539. doi: 10.3856/vol45-issue3-fulltext-3
Liles, M. J., Jandres, M. V., Lopez, W. A., Mariona, G. I., Hasbun, C. R., and Seminoff, J. A. (2011). Hawksbill turtles Eretmochelys imbricata in El Salvador: nesting distribution and mortality at the largest remaining nesting aggregation in the eastern Pacific Ocean. Endanger. Species Res. 14, 23–30. doi: 10.3354/esr00338
Liles, M. J., Peterson, M. J., Lincoln, Y. S., Seminoff, J. A., Gaos, A. R., and Peterson, T. R. (2015a). Connecting international priorities with human wellbeing in low-income regions: lessons from hawksbill turtle conservation in El Salvador. Local Environ. 20, 1383–1404. doi: 10.1080/13549839.2014.905516
Liles, M. J., Peterson, M. J., Seminoff, J. A., Altamirano, E., Henríquez, A. V., Gaos, A. R., et al. (2015b). One size does not fit all: importance of adjusting conservation practices for endangered hawksbill turtles to address local nesting habitat needs in the eastern Pacific Ocean. Biol. Conservat. 184, 405–413. doi: 10.1016/j.biocon.2015.02.017
Liles, M. J., Peterson, T. R., Seminoff, J. A., Gaos, A. R., Altamirano, E., Henríquez, A. V., et al. (2019). Potential limitations of behavioral plasticity and the role of egg relocation in climate change mitigation for a thermally sensitive endangered species. Ecol. Evol. 9, 1603–1622. doi: 10.1002/ece3.4774
Limpus, C. J., and Chaloupka, M. (1997). Nonparametric regression modeling of green sea turtle growth rates (southern Great Barrier Reef). Mar. Ecol. Prog. Ser. 149, 23–34. doi: 10.3354/meps149023
Limpus, C. J., and Miller, J. D. (1990). The use of measured scutes of hawksbill turtles. Eretmochelys imbricata, in the management of the tortoiseshell (bekko) trade. Aust. Wildl. Res. 17, 633–639. doi: 10.1071/WR9900633
Limpus, C. J., and Miller, J. D. (2000). Final Report for Australian Hawksbill Turtle Population Dynamics Project. Unpublished Report to the Japan Bekko Association. Brisbane: Queensland Parks and Wildlife Services.
Mansfield, K. L., Wyneken, J., and Luo, J. (2021). First Atlantic satellite tracks of ‘lost years’ green turtles support the importance of the Sargasso Sea as a sea turtle nursery. Proc. R. Soc. B Biolog. Sci. 288:20210057. doi: 10.1098/rspb.2021.0057
MARN (2014). MARN y líderes locales del Bajo Lempa unen esfuerzos para construer Plan Ambiental. Riyadh: MARN.
Marshall, J. D., Brookes, J. R., and Lajtha, K. (2007). “Sources of variation in the stable isotopic composition of plants,” in Stable Isotopes in Ecology and Environmental Sciences, eds R. Michener and K. Lajtha (Oxford: Blackwell Publishing), 22–50. doi: 10.1002/9780470691854.ch2
Massey, L., and McCord, P. (2017). AMBAS in Action: How and all-women’s group is leading sea turtle conservation efforts in El Salvador. Capstone Thesis. San Diego, CA: University of California, San Diego.
McClellan, C. M., Braun-McNeill, J., Avens, L., Wallace, B. P., and Read, A. J. (2010). Stable isotopes confirm a foraging dichotomy in juvenile loggerhead sea turtles. J. Exp. Mar. Biol. Ecol. 387, 44–51. doi: 10.1016/j.jembe.2010.02.020
McMahon, K. W., Berumen, M. L., Mateo, I., Elsdon, T. S., and Thorrold, S. R. (2011). Carbon isotopes in otolith amino acids identify residency of juvenile snapper (Family: Lutjanidae) in coastal nurseries. Coral Reefs 30, 1135–1145. doi: 10.1007/s00338-011-0816-5
McMahon, K. W., Hamady, L., and Thorrold, S. R. (2013). A review of ecogeochemistry approaches to estimating movements of marine animals. Limnol. Oceanog. 58:697. doi: 10.4319/lo.2013.58.2.0697
Montoya, J. (2008). “Nitrogen stable isotopes in marine environments,” in Nitrogen in the Marine Environment, Chap. 29, eds D. G. Capone, D. A. Bronk, M. R. Mullholland, and E. J. Carpenter (Burlington, MA: Academic Press, Elsevier), 1277–1302. doi: 10.1016/B978-0-12-372522-6.00029-3
Montoya, J., Holl, C., Zehr, J., Hansen, A., Villareal, T., and Capone, D. G. (2004). High rates of N2 fixation by unicellular diazotrophs in the oligotrophic Pacific Ocean. Nature 430, 1027–1032. doi: 10.1038/nature02824
Oczkowski, A., Kreakie, B., McKinney, R. A., and Prezioso, J. (2016). Patterns in stable isotope values of nitrogen and carbon in particulate matter from the Northwest Atlantic continental shelf, from the Gulf of Maine to Cape Hatteras. Front. Mar. Sci. 3:252. doi: 10.3389/fmars.2016.00252
Pajuelo, M., Bjorndal, K. A., Alfaro-Shigueto, J., Seminoff, J. A., Mangel, J., and Bolten, A. B. (2010). Stable isotope dichotomy in loggerhead turtles reveals Pacific-Atlantic oceanographic differences. Mar. Ecol. Prog. Ser. 417, 277–285. doi: 10.3354/meps08804
Plotkin, P. T. (2003). “Adult migrations and habitat use,” in The Biology of Sea Turtles: II, eds P. Lutz, J. Musick, and J. Wyneken (Boca Raton, FL: CRC Press), 225–241. doi: 10.1201/9781420040807.ch8
R Core Team (2021). A Language and Environment for Statistical Computing. Vienna: R Foundation for Statistical Computing.
Ramirez, M. D., Avens, L., Seminoff, J. A., Goshe, L. R., and Heppell, S. S. (2015). Patterns of loggerhead turtle ontogenetic shifts revealed through isotopic analysis of annual skeletal growth increments. Ecosphere 6:244. doi: 10.1890/ES15-00255.1
Seminoff, J. A., Benson, S. R., Arthur, K. E., Dutton, P. H., Tapilatu, R., and Popp, B. N. (2012). Stable isotope tracking of endangered sea turtles: validation with satellite telemetry and δ15N analysis of amino acids. PLoS One 7:e37403. doi: 10.1371/journal.pone.0037403
Seminoff, J. A., Komoroske, L. M., Amorocho, D., Arauz, R., Chacón-Chaverrí, D., de Paz, N., et al. (2021). Large-scale patterns of green turtle trophic ecology in the eastern Pacific Ocean. Ecosphere 12:e03479. doi: 10.1002/ecs2.3479
Snover, M., Avens, L., and Hohn, A. (2007). Back-calculating length from skeletal growth marks in loggerhead sea turtles Caretta caretta. Endanger. Species Res. 3, 95–104. doi: 10.3354/esr003095
Snover, M. L., Balazs, G. H., Murakawa, S. K. K., Hargrove, S. K., Rice, M. R., and Seitz, W. A. (2012). Age and growth rates of Hawaiian hawksbill turtles (Eretmochelys imbricata) using skeletochronology. Mar. Biol. 160, 37–46. doi: 10.1007/s00227-012-2058-7
Snover, M. L., and Hohn, A. A. (2004). Validation and interpretation of annual skeletal marks in loggerhead (Caretta caretta) and Kemp’s ridley (Lepidochelys kempii) sea turtles. Fish. Bull. 102, 682–692.
Snover, M. L., Hohn, A. A., Crowder, L. B., and Macko, S. A. (2010). Combining stable isotopes and skeletal growth marks to detect habitat shifts in juvenile loggerhead sea turtles Caretta caretta. Endanger. Species Res. 13, 25–31. doi: 10.3354/esr00311
Snover, M. L., Hohn, A. A., Goshe, L. R., and Balazs, G. H. (2011). Validation of annual skeletal marks in green sea turtles Chelonia mydas using tetracycline labeling. Aquat. Biol. 12, 197–204. doi: 10.3354/ab00337
Turner Tomaszewicz, C. N., Avens, L., LaCasella, E. L., Eguchi, T., Dutton, P. H., LeRoux, R. A., et al. (2022). Mixed-stock aging analysis reveals variable sea turtle maturity rates in a recovering population. J. Wildl. Manag. 2022:e22217. doi: 10.1002/jwmg.22217
Turner Tomaszewicz, C. N., Seminoff, J. A., Avens, L., and Kurle, C. M. (2016). Methods for sampling sequential annual bone growth layers for stable isotope analysis. Methods Ecol. Evol. 7, 556–564. doi: 10.1111/2041-210X.12522
Turner Tomaszewicz, C. N., Seminoff, J. A., Avens, L. A., Goshe, L. R., Rodriguez-Baron, J. M., Peckham, S. H., et al. (2018). Expanding the coastal forager paradigm: Long-term pelagic habitat use by green turtles (Chelonia mydas) in the eastern Pacific Ocean. Mar. Ecol. Prog. Ser. 587, 217–234. doi: 10.3354/meps12372
Turner Tomaszewicz, C. N., Seminoff, J. A., Ramirez, M. D., and Kurle, C. M. (2015b). Effects of demineralization on the stable isotope analysis of bone samples. Rapid Commu. Mass Spectrom. 29, 1879–1888. doi: 10.1002/rcm.7295
Turner Tomaszewicz, C. N., Seminoff, J. A., Peckham, S. H., Avens, L., Goshe, L., Bickerman, K., et al. (2015a). Age and residency duration of loggerhead turtles at a North Pacific bycatch hotspot using skeletochronology. Biol. Conserv. 186, 134–142. doi: 10.1016/j.biocon.2015.03.015
Turner Tomaszewicz, C. N., Seminoff, J. A., Peckham, S. H., Avens, L., and Kurle, C. M. (2017a). Intrapopulation variability in the timing of ontogenetic habitat shifts in sea turtles revealed using δ15N values from bone growth rings. J. Anim. Ecol. 86, 694–704. doi: 10.1111/1365-2656.12618
Turner Tomaszewicz, C. N., Seminoff, J. A., Price, M., and Kurle, C. M. (2017b). Stable isotope discrimination factors and between-tissue isotope comparisons for bone and skin from captive and wild green sea turtles (Chelonia mydas). Rapid Commun. Mass Spectrom. 231, 1903–1914. doi: 10.1002/rcm.7974
Vander Zanden, H. B., Tucker, A. D., Hart, K. M., Lamont, M. M., Fujisaki, I., Addison, D. S., et al. (2015). Determining foraging area origin in a migratory marine vertebrate by integrating stable isotope analysis and satellite tracking: a novel approach. Ecol. Appl. 25, 320–335. doi: 10.1890/14-0581.1
Vásquez, M., and Liles, M. J. (2008). “Estado actual de las tortugas marinas en El Salvador, con énfasis en la tortuga carey,” in Proceedings of the XII Congreso de la Sociedad Mesoamericana para la Biología y la Conservación, San Salvador.
Wallace, B. P., Seminoff, J. A., Kilham, S. S., Spotila, J. R., and Dutton, P. H. (2006). Leatherback turtles as oceanographic indicators: Stable isotope analyses reveal a trophic dichotomy between ocean basins. Mar. Biol. 149, 953–960. doi: 10.1007/s00227-006-0247-y
Wedemeyer-Strombel, K. R., Seminoff, J. A., Liles, M. J., Sánchez, R. N., Chavarría, S., Valle, M., et al. (2021). Fishers’ ecological knowledge and stable isotope analysis reveal mangrove estuaries as key developmental habitats for critically endangered sea turtles. Front. Conserv. Sci. 2:796868. doi: 10.3389/fcosc.2021.796868
West, J. B., Bowen, G. J., Cerling, T. E., and Ehleringer, J. R. (2006). Stable isotopes as one of nature’s ecological recorders. Trends Ecol. Evol. 21, 408–414. doi: 10.1016/j.tree.2006.04.002
Wood, S. N. (2017). Generalized Additive Models: An Introduction With R, Generalized Additive Models: An Introduction with R, 2nd Edn. (Boca Raton, FL: CRC Press), doi: 10.1201/9781315370279
Keywords: sea turtle, stable isotopes, post-hatchlings, habitat use, conservation
Citation: Turner Tomaszewicz CN, Liles MJ, Avens L and Seminoff JA (2022) Tracking movements and growth of post-hatchling to adult hawksbill sea turtles using skeleto+iso. Front. Ecol. Evol. 10:983260. doi: 10.3389/fevo.2022.983260
Received: 30 June 2022; Accepted: 01 August 2022;
Published: 24 August 2022.
Edited by:
Jason Newton, University of Glasgow, United KingdomReviewed by:
Yik Hei Sung, Lingnan University, ChinaAndré Sucena Afonso, Center for Marine and Environmental Sciences (MARE), Portugal
Copyright © 2022 Turner Tomaszewicz, Liles, Avens and Seminoff. This is an open-access article distributed under the terms of the Creative Commons Attribution License (CC BY). The use, distribution or reproduction in other forums is permitted, provided the original author(s) and the copyright owner(s) are credited and that the original publication in this journal is cited, in accordance with accepted academic practice. No use, distribution or reproduction is permitted which does not comply with these terms.
*Correspondence: Calandra N. Turner Tomaszewicz, Y2FsaS50dXJuZXJAbm9hYS5nb3Y=