- 1Station of Alxa League Aviation Forest Guard, Alxa Left Banner, China
- 2Station of Alxa League Forestry and Grassland of Pest Control and Quarantine, Alxa Right Banner, China
- 3Station of Alxa League Forestry and Grassland Protection, Alxa Left Banner, China
- 4Institute of Gulang Forestry and Grassland, Minqin, China
- 5Institute of Alxa Forestry and Grassland, Alxa Left Banner, China
- 6State Key Laboratory of Aridland Crop Science, Gansu Agricultural University, Lanzhou, China
Apocynum venetum L. is an endangered perennial species mainly distributed in the semi-arid lands and plays an important role in protecting ecological environment; meanwhile, it is also widely used as a traditional Chinese medicine. While physiological changes of seed germination under drought stress have been conducted, the adaptive mechanism to semi-arid environment is still unknown. Here, the physiological and transcriptional changes during seed germination of A. venetum under different PEG-6000 treatments (5 to 20%) were examined. The germination characteristics (germination rate, radicle length and fresh weight) were promoted under moderate drought (5% PEG). The activities of antioxidant enzymes (SOD and POD) and contents of osmolytes (soluble sugar, MDA and Pro) were increased while the CAT and APX activities and the protein content decreased with the increase of PEG concentrations. A total of 2159 (1846 UR, 313 DR) and 1530 (1038 UR, 492 DR) DEGs were observed during seed germination at 5 and 25% PEG vs. CK, respectively; and 834 co-expressed DEGs were classified into 10 categories including stress response (67), primary metabolism (189), photosynthesis and energy (83), cell morphogenesis (62), secondary metabolism (21), transport (93), TF (24), transcription (42), translation (159) and bio-signaling (94). The RELs of representative genes directly associated with drought stress and seed germination were coherent with the changes of antioxidant enzymes activities and osmolytes contents. These findings will provide useful information for revealing adaptive mechanism of A. venetum to semi-arid environment.
Introduction
Apocynum venetum L. (family Apocynaceae) is a perennial semi-shrub species that widely distributed in the temperate zones of Eurasia, North America, northern China, etc., especially in saline–alkali land and sandy soils (Flora of China, 1977; Xie et al., 2012; Rong et al., 2015). The plants can provide significant environmental benefits by preventing land degradation caused by salinization and desertification and offer opportunities to develop desert farming in the arid zones (Ping et al., 2014; Rouzi et al., 2018; Jiang et al., 2021). Meanwhile, it commonly known as “luobuma” in China and has been widely used as a traditional Chinese medicine to treat cardiac disease, hypertension and nephritis, and used for anti-irritability, anti-cancer and anti-radiation in recent years (Xie et al., 2012).
In the semi-arid lands, A. venetum plants present flourishing growth because they are easy to propagate and have the ability to withstand the harsh desert environment (Ma et al., 2000; Ning et al., 2010; Jiang et al., 2021). As is known, seed germination is the key stage for the plant life cycle and affects the growth and distribution of plant populations, especially under adverse abiotic conditions (Ren et al., 2011; Campobenedetto et al., 2020). In order to find out the effects of abiotic stresses on seed germination of A. venetum, several studies have been conducted on the physiological and biochemical changes. Specifically, the seed germination rate of freshly matured seeds was promoted to some extent under low PEG-6000 (0 to 10%), not significantly affected under low salinity concentrations (0 to 200 mmol/L), while significantly decreased with the increasing of drought and salinity stresses (Zhang et al., 2007; Xu et al., 2015; Jiang et al., 2021); in addition, the seed germination rate was better performance at 10/25 and 15/30°C (12-h alternating) than any other temperatures, significantly decreased with prolongation of seed storage period, while not affected by the light (Liu et al., 2015; Jiang et al., 2021). For the physiological changes during seed germination in response to drought stress, the contents of electrolyte leakage rates and osmolytes [i.e., MDA, Pro and soluble sugar], and the activities of antioxidant enzymes [i.e., SOD, APX, GR and POD] were increased, while the CAT activity was descended in general trend with the increasing of PEG-6000 concentrations (0-30%) (Xu et al., 2015; Zhao and Dai, 2015).
Actually, there are different resistances to drought stress for seed germination of other psammophytes. For example, seed germination rates of Platycodon grandiflorum and Halimodendron halodendron were not affected under 4 and 5% PEG, respectively (Ding and Zhang, 2016; Qi et al., 2021); and seed germination rates of Artemisia Argyi and Pinus sylvestris were increased under 5 and 10% PEG, respectively (Zhu et al., 2006; Zhang et al., 2019). Extensive investigations have demonstrated that plants respond and adapt to drought stress through various physiological, biochemical and transcriptional processes, thereby acquiring stress tolerance (Zhu, 2002; Shinozaki and Yamaguchi-Shinozaki, 2007). There are also different changes of physiological indexes during seed germination in response to drought stress. For example, the contents of MDA, Pro and soluble protein as well as the activities of SOD and POD in foxtail millet (Setaria italica) were increased under PEG treatments (Pei et al., 2014); the contents of soluble sugar, Pro and glycine betaine as well as the activities of SOD and POD were increased, while the contents of soluble protein decreased in Ajowan (Trachyspermum ammi) under PEG treatments (Rohamare et al., 2014); and the contents of MDA and Pro as well as the activities of SOD and POD were increased under water potential −0.2 MPa PEG, while decreased with increasing of PEG concentrations (Shi et al., 2010). For the transcriptional changes in plants in response to drought stress, hundreds of genes for protein kinases and TFs (e.g., bZIP, NAC, and AP2/ERF TF families) that control key processes in response to drought stress have been identified including signal perception, signal transduction, and transcriptional regulation (Hu and Xiong, 2014). For example, overexpression of TaSNAC4-3A in Arabidopsis led to seed germination and root growth when exposed to drought stress (Mei et al., 2021); the expression of TaNCED gene was up-regulated by ABA treatment and drought stress, while its overexpression delayed the seed germination of wheat (Triticum aestivum) (Tong et al., 2017); and the expression of ARAG1 that is an ABA-responsive DREB gene was up-regulated by ABA treatment and drought stress, and it was over-expressed in germinating seeds of rice (Oryza sative) (Zhao et al., 2010). Above results indicate that seed germination under drought stress involves a wide range of responses including: physiological characteristics (i.e., germination characteristics), cellular metabolism (i.e., osmolytes accumulation and enzymes activity), and molecular regulation (i.e., differential expression of genes and proteins), leading to the adaptation to water deficit.
To date, seed germination characteristics and physiological changes under drought stress have been conducted, and germination rate promoted under moderate drought has been demonstrated for the psammophytes A. venetum (Zhang et al., 2007; Xu et al., 2015; Zhao and Dai, 2015; Jiang et al., 2021), while the response mechanism to drought stress is still unknown. In this study, the difference of the seed germination characteristics and the changes of antioxidant enzyme activity, osmolytes content and genes expression level in A. venetum were examined under different PEG-6000 treatments.
Materials and methods
Plant materials
Fully mature seeds of Apocynum venetum L. were collected from Alxa Left Banner, China (1050 m a.s.l.; E103°42′14.77″, N38o18′5.62″) in October 2019. The species was identified by Associated Professor Yubi Zhou (Northwest Institute of Plateau Biology, Chinese Academy of Sciences, Xining, China). Seeds were air dried at room temperature and stored at 4°C refrigerator in air-tight bags in the dark. In March 2020, seeds were cleaned with tap water and successively immersed in 50°C water for 15 min and 70% ethanol for 20 s. After rinsed with sterile water for 5 times, 20 seeds were sown in a Petri-dish (9 cm diameter, two layers of gauze on the bottom) with 5 mL PEG-6000 water solution including: 0% (CK), 5, 10, 15, 20, and 25%. Each treatment has ten independent biological replicates.
The seeds were germinated in a dark growth chamber set at a constant temperature of 22°C. After 3 d, uniform seedlings were individually transplanted in a new above mentioned Petri-dish with 5 mL solution of different PEG-6000 concentrations, and then placed in a growth chamber at 22°C with 16 h/8h photoperiod (400 μmol/m2/s flu) and 70% relative humidity. In order to provide adequate water for seeds germination and seedlings growth, sterile water was timely added to the Petri-dish according to the actual evaporation and seed absorption.
Measurement of germination characteristics
Seed germination rate was recorded after 3 d. Growth parameters (i.e., hypocotyl length, radicle length and fresh weight) of seedlings were measured after 6 d. Measurements were made on two seeds or seedlings for each of the ten biological replicates of each treatment.
Determination of antioxidant enzyme activities
Four indicators including SOD, POD, CAT, and APX were determined using a spectrometer (Thermo Evolution 201, Waltham, MA, United States). Briefly, SOD activity was determined based on the ability to inhibit the photochemical reduction of nitro blue tetrazolium chloride at 560 nm (Stewart and Bewley, 1980); POD activity was determined by the guaiacol colorimetric method at 470 nm (Hammerschmidt et al., 1982); CAT activity was determined by UV absorption method at 240 nm (Chen, 2002); and APX activity was determined based on the decrease in the absorbance of the oxidized ascorbate at 290 nm (Shen et al., 1996).
Determination of osmolytes content
Four indexes including soluble sugar, protein, MDA, and Pro contents of drought response were determined using a spectrometer (Thermo Evolution 201, Waltham, MA, United States). Briefly, soluble sugar content was determined by the phenolsulfuric acid method (Dubois et al., 1956); protein content was determined by the comassie brilliant blue colorimetric method (Bradford, 1976); MDA content was determined by the thiobarbituric acid reaction (Li, 2000); and Pro content was determined by the sulfosalicylic acid-acid ninhydrin method (Shi, 2016).
Transcriptomic analysis
RNA extraction, cDNA library construction, and illumina sequencing
Total RNA samples of CK, 5 and 25% PEG treatments after 6 d with three biological replicates were extracted using an RNA kit (R6827, Omega Bio-Tek, Norcross, GA, United States). The amount of RNA was quantified using a NanoDrop ND-1000 spectrophotometer (Nanodrop Technologies, Wilmington, NC, United States), and the quality was determined using an Agilent 2100 Bioanalyzer (Agilent Technologies, Palo Alto, CA, United States). One cDNA library was constructed from total RNA of CK, 5 and 25% PEG-6000 seedlings, and the processes of enrichment, fragmentation, reverse transcription, synthesis of the second-strand cDNA and purification of cDNA fragments were applied according to previous protocols (Li et al., 2018). Reads was generated using an Illumina HiSeqTM 4000 platform (Gene Denovo Biotechnology Co., Ltd., Guangzhou, China).
Reads filtration, assembly, unigene expression analysis, and basic annotation
Raw reads were filtered using a FASTQ system to obtain high-quality clean reads by removing reads containing adapters, removing reads containing more than 10% of unknown nucleotides (N), and removing low quality reads containing more than 50% of low quality (Q-value ≤ 20) bases (Chen et al., 2018). Clean reads was assembled using Trinity (Grabherr et al., 2011). The expression level of each transcript was nor-malized to the RPKM values. Differential expression analysis of transcripts was performed using DESeq2 software (Love et al., 2014) between different groups. The differential expression levels between 5 and 25% PEG vs. CK were determined with the criteria of the false discovery rate (FDR) < 0.05 and | log2(fold-change)| > 1. The function of DEGs was annotated using BLAST against the databases including Nr, KEGG, KOG, SwissProt, and GO with e-value ≤ 10–5 as a threshold (Conesa et al., 2005).
qRT-PCR validation
The primer sequence (Supplementary Table 1) was designed using a primer-blast tool in NCBI and synthesized by Sangon Biotech (Shanghai, China). First cDNA was synthesized using a RT Kit (KR116, Tiangen, China). PCR amplification was performed using a SuperReal PreMix (FP205, Tiangen, China). Melting curve was analyzed at 72°C for 34 s. Actin gene was used as a reference control. The RELs of genes were calculated using a 2–ΔΔCt method (Willems et al., 2008).
Statistical analysis
All the measurements were performed using three biological replicates. Statistical analysis was performed via ANOVA and Duncan multiple comparison tests, and SPSS 22.0 was the software package used with P < 0.05 as the basis for statistical differences.
Results
Germination characteristics under polyethylene glycol treatments
Seed germination characteristics were significantly affected by drought stress (P < 0.05; Figure 1). The seed germination rate and fresh weight were significantly increased under 5% PEG, and the radicle length was significantly increased at 5% and 10% PEG compared with CK, while significantly decreased with the increase of PEG concentrations (Figures 1A,C,D); the hypocotyl length was gradually decreased with the increase of PEG concentrations (Figure 1B).
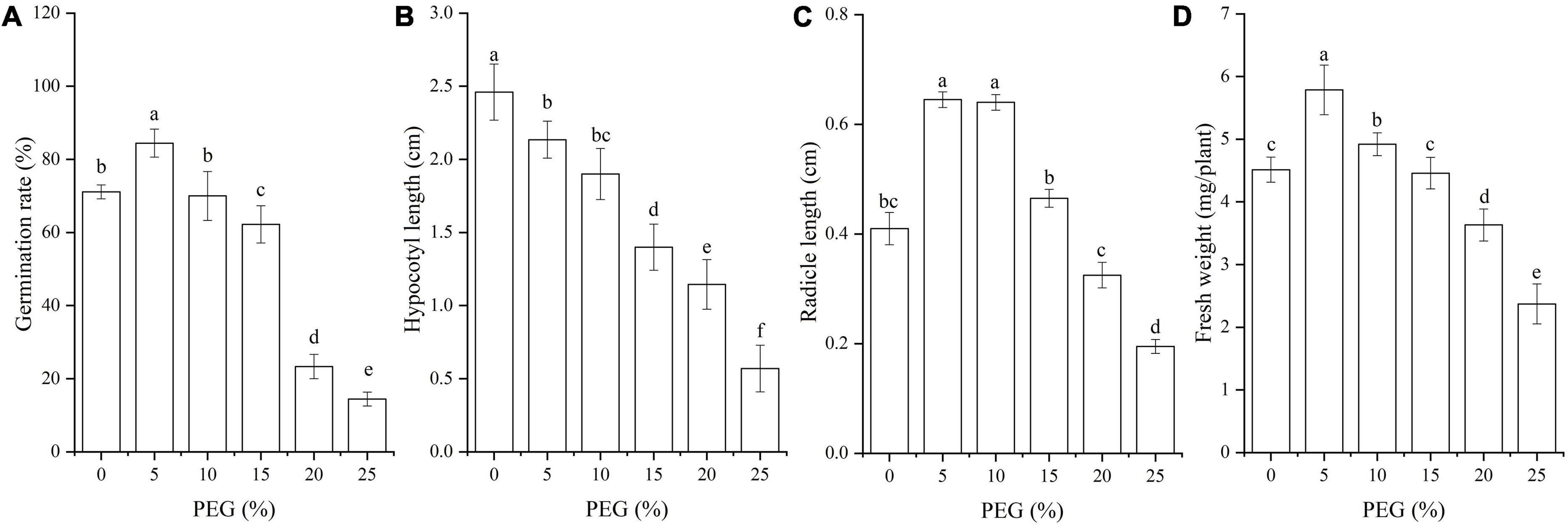
Figure 1. Changes of seed germination rate (A), hypocotyl length (B), radicle length (C) and fresh weight (D) of A. venetum seedlings under different PEG treatments. Different letters represents a significant difference (P < 0.05) among different PEG treatments.
Antioxidant enzyme activities under polyethylene glycol treatments
Significant differences in antioxidant enzyme activities were observed under drought stress (P < 0.05; Figure 2). Specifically, the SOD activity was significantly decreased at 5% PEG compared with CK, while gradually increased with the increase of PEG concentrations (Figure 2A); the POD activity was significantly increased with the increase of PEG concentrations (Figure 2B); the CAT and APX activities was decreased in general trend with the increase of PEG concentrations, although there was no significant difference among the 5, 10, and 15% PEG treatments (Figures 2C,D).
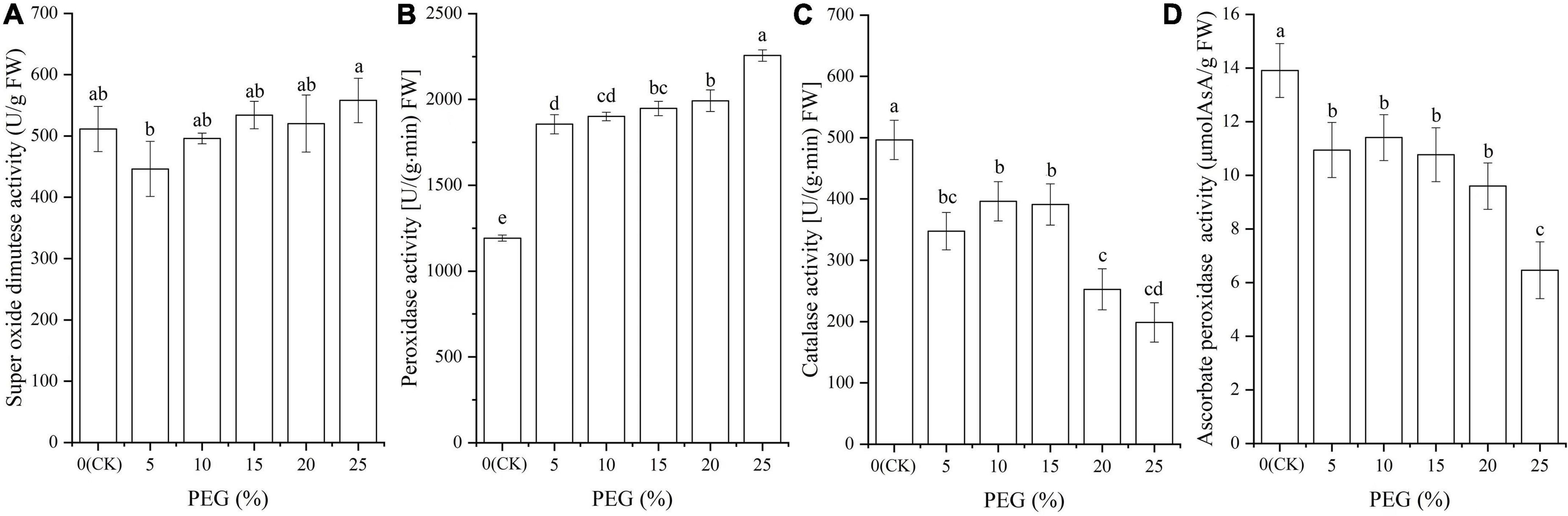
Figure 2. Changes of antioxidant enzyme activities of SOD (A), POD (B), CAT (C) and APX (D) in A. venetum seedlings under different PEG treatments. Different letters represents a significant difference (P < 0.05) among different PEG treatments.
Osmolytes changes under polyethylene glycol treatments
Significant differences in osmolytes contents were also observed under drought stress (P < 0.05; Figure 3). Specifically, the contents of soluble sugar and MDA were decreased at 5% PEG compared with CK, while significantly increased with the increase of PEG concentrations (Figures 3A,C); The protein content was significantly increased at 5%, 10% and 15% PEG treatments compared with CK, while significantly decreased with the increase of PEG concentrations (Figure 3B); and the Pro content was significantly increased with the increase of PEG concentrations (Figure 3D).
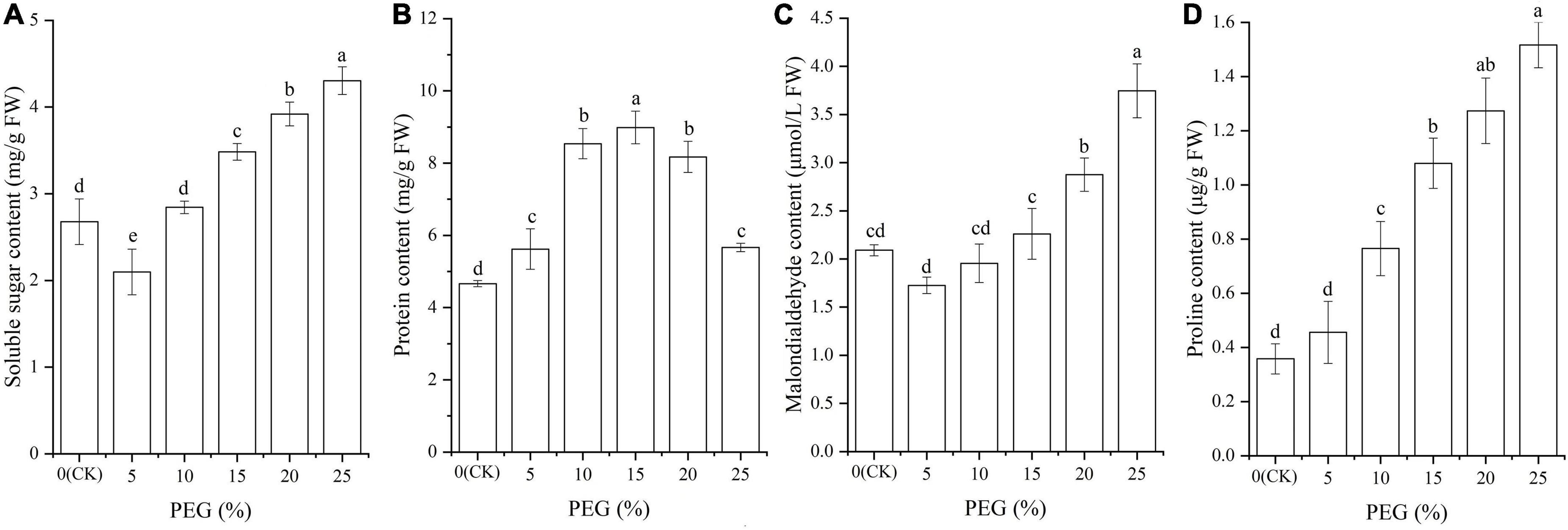
Figure 3. Changes of soluble sugar (A), protein (B), malondialdehyde (C), and proline (D) contents in A. venetum seedlings under different PEG treatments. Different letters represents a significant difference (P < 0.05) among different PEG treatments.
Transcriptomic analysis between different polyethylene glycol treatments
Global gene analysis
To reveal the regulation mechanism of moderate drought promoting seed germination, comparison of transcripts at CK, 5 and 25% PEG was analyzed. A total 51.43, 53.17, and 57.48 million high-quality reads were obtained after filtering from raw data, and 42.12, 43.48, and 47.00 million unique reads as well as 0.81, 0.80, and 0.81 million multiple reads were mapped at the CK, 5 and 25% PEG, respectively (Table 1). A total of 52,305 unigenes were annotated against the KEGG (34,198), KOG (22,630), Nr (36,077) and SwissProt (27,467) databases (Table 1 and Supplementary Figure 1). Using KEGG database, 65.38% unigenes were enriched into 140 biochemical pathways (Supplementary Figure 2). Using KOG database, 43.27% unigenes encoding the identified proteins were classified into 25 functional categories (Supplementary Figure 3). Using NR database, the top 10 species includes: Coffea arabica, Carica papaya, Coffea eugenioides, Pistacia vera, Actinidia chinensis, Vitis vinifera, Citrus clementine, Theobroma cacao, Olea europaea and Citrus sinensis (Supplementary Figure 4). Using SwissProt database, 52.51% unigenes were annotated. Using GO database, 53 terms were classified into biological process (Hu and Xiong, 2014), cellular component (Zhang et al., 2019), and molecular function (Xu et al., 2015) (Supplementary Figure 5).
Differentially expressed genes at 5 and 25% polyethylene glycol versus (vs.) CK
A total of 2159 (1846 UR, 313 DR) and 1530 (1038 UR, 492 DR) genes were differently expressed at 5 and 25% PEG vs. CK, respectively (Figure 4), based on the violin plot of expression (Supplementary Figure 6), principal component analysis (PCA) (Supplementary Figure 7), and heat map (Supplementary Figure 8).
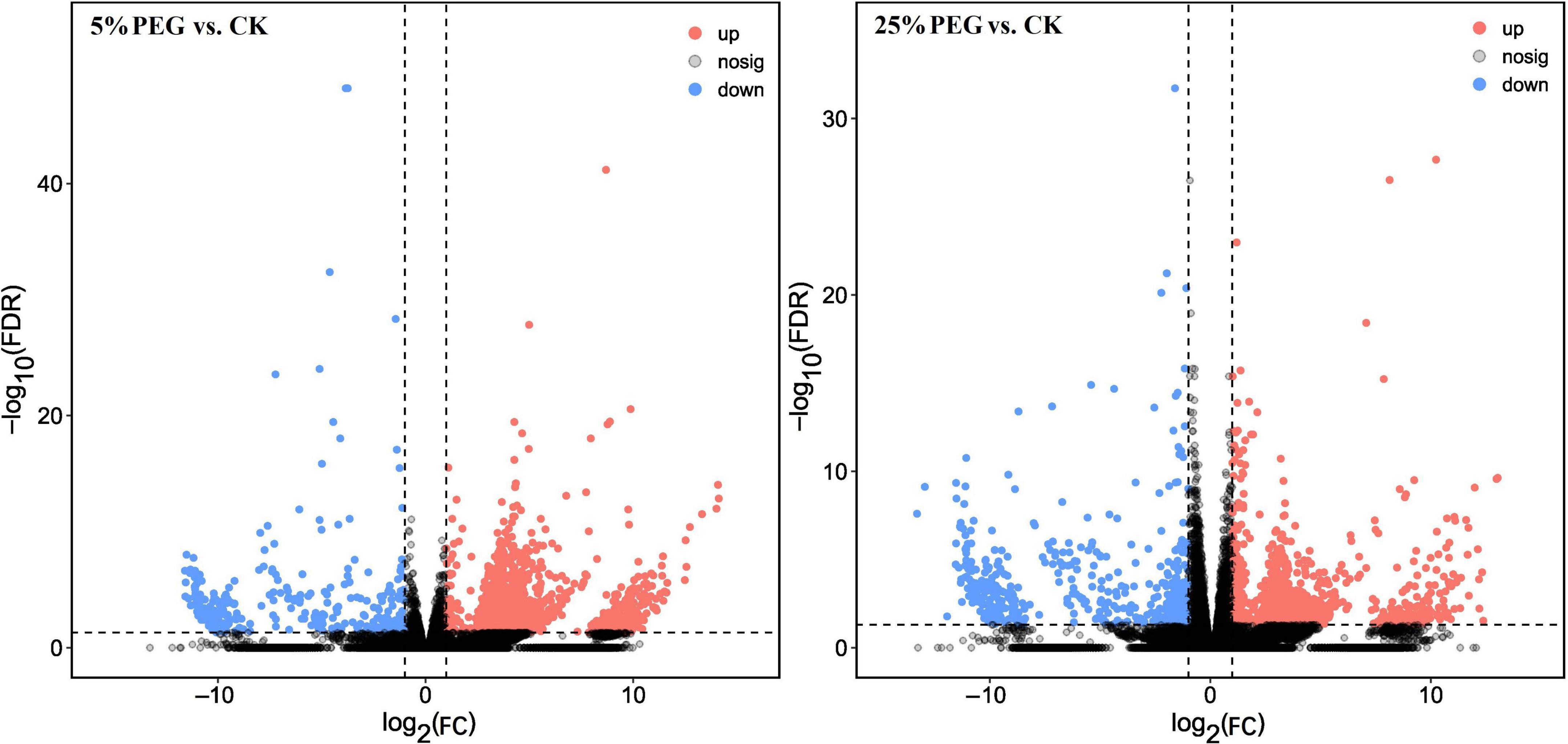
Figure 4. Volcano plot of differential comparison under 5 and 25% PEG vs. CK. UR, up regulation; DR, down regulation.
Classification of differentially expressed genes
Among the 2159 and 1530 DEGs, 1907 and 1315 genes were identified from the KEGG, KOG, or SwissProt databases at 5% and PEG 25% vs. CK, respectively (Figures 5A,B). Of the identified DEGs, 834 genes were co-expressed (Figure 5C). Based on the biological functions, the 834 genes were classified into 10 categories including: stress response (67 genes), primary metabolism (189 genes), cell morphogenesis (62 genes), TF (19 genes), bio-signaling (99 genes), photosynthesis and energy (83 genes), secondary metabolism (21 genes), transport (93 genes), transcription (42 genes), and translation (159 genes) (Figure 5D).
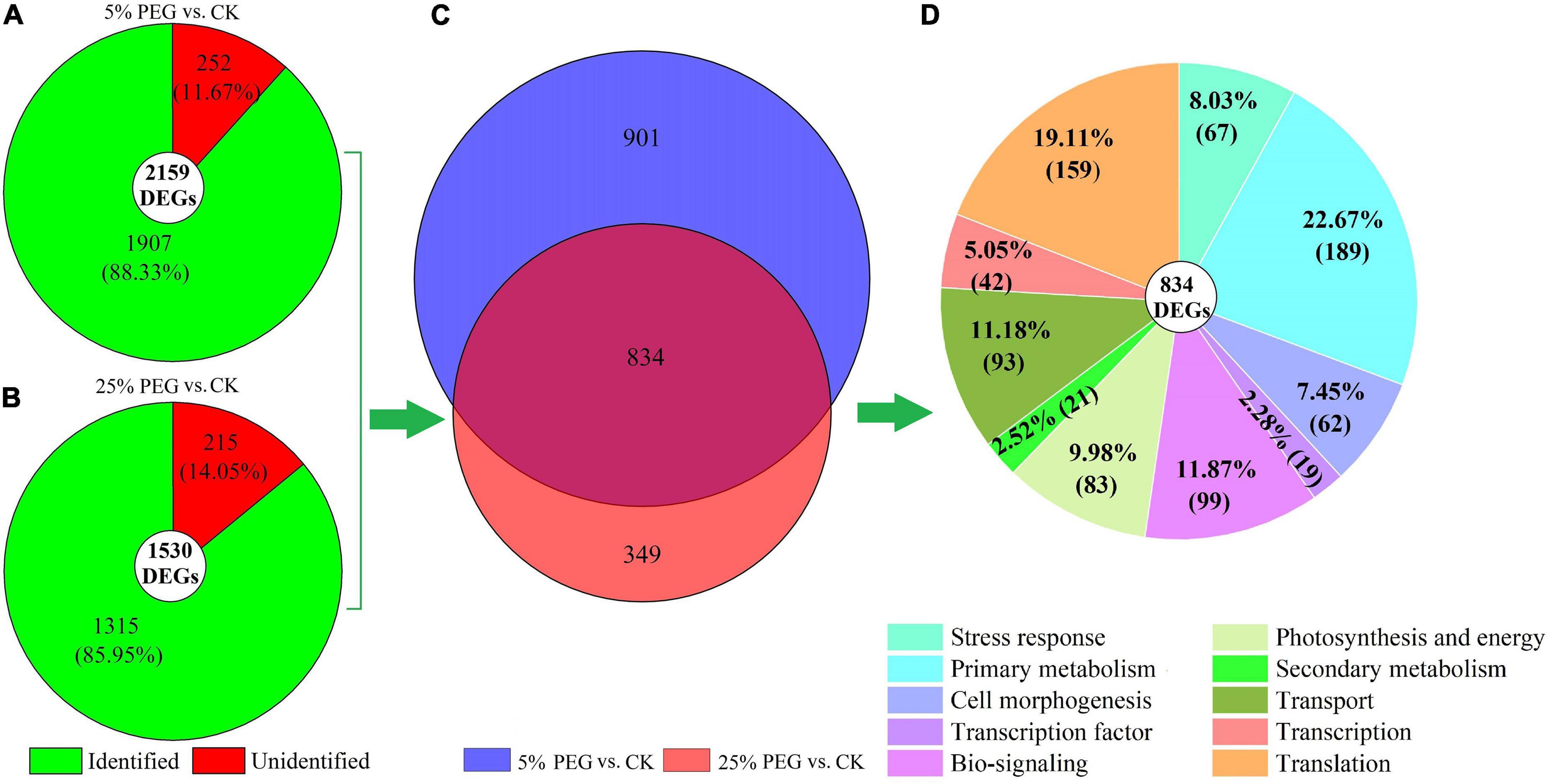
Figure 5. Distribution and classification of DEGs under 5 and 25% PEG vs. CK. Images (A,B) represents the DEGs under 5 and 25% PEG vs. CK, respectively; image (C) represents the co-expressed genes; image (D) represents the classification of the co-expressed genes.
Specifically classification of differentially expressed genes and expression level validated by qRT-PCR
Differentially expressed genes directly associated with drought stress and antioxidant enzyme
In order to highlight drought tolerance and reveal the relationship of antioxidant enzyme activities with DEGs, 18 genes directly associated with drought stress and antioxidant enzyme were screened from the 67 DEGs involved in stress response including: water deprivation (e.g., DHN1, RCD1, and LIPC), SOD (SODA), POD (e.g., Gpx3, PER42, and PER52), and CAT (e.g., Cat, CAT1, and CAT2) (Table 2); and other 49 genes were associated with temperature, salinity and pathogens stresses, etc (Supplementary Table 2). The expression levels of 18 representative genes directly associated with drought tolerance were validated with 14 genes up-regulated and 4 genes down-regulated at 5 and 25% PEG vs. CK, and their RELs were almost consistent with RPKM values (Figure 6 and Table 2).
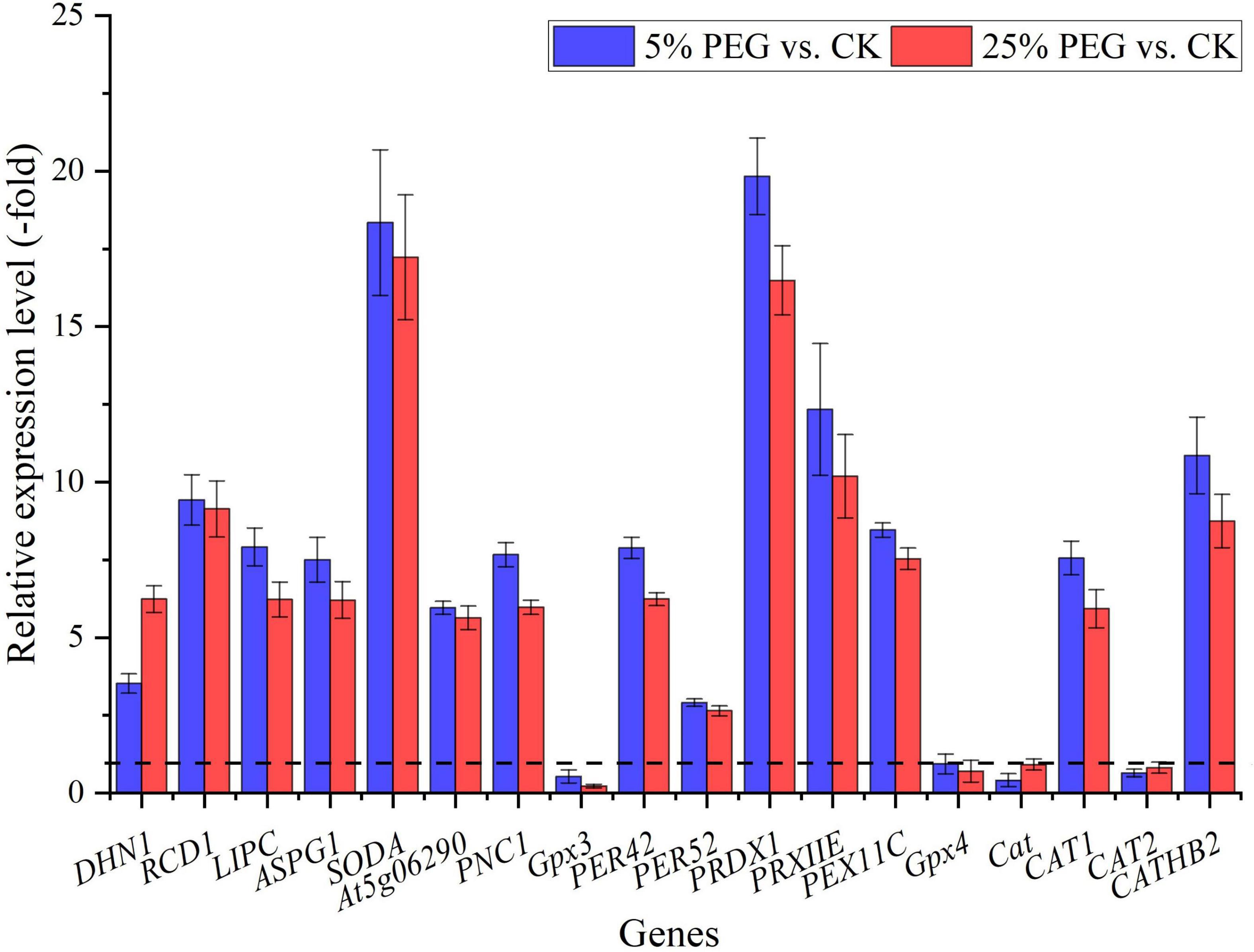
Figure 6. The relative expression level of genes directly associated with drought stress in A. venetum seedlings under 5 and 25% PEG vs. CK, as determined by qRT-PCR (mean ± SD, n = 3).
Differentially expressed genes directly associated with soluble sugar and protein metabolism
In order to reveal the relationship of osmolytes contents with DEGs, 37 genes directly associated with soluble sugar (22 genes) and protein metabolism (15 genes) were screened from the 189 DEGs involved in primary metabolism, with soluble sugar metabolism including: glucose (PGM1, SDH, and GPU), sucrose (SUS2 and SUS3), fructose (e.g., FKFBP, FBP, and FBA), galactose (e.g., BGAL, UGE1, and GOLS1) and starch (SBEI, AMY3, and GWD3); and protein metabolism including: Cpe, Ctsb, and CLPC, etc (Table 3); and other 152 genes were associated with fatty acid, lipid metabolism, amino acid, etc (Supplementary Table 3). The expression levels of 12 representative genes associated with soluble sugar (8 genes) and protein metabolism (4 genes) were validated with all up-regulated at 5 and 25% PEG vs. CK, and their RELs were consistent with RPKM values (Figure 7 and Table 3).
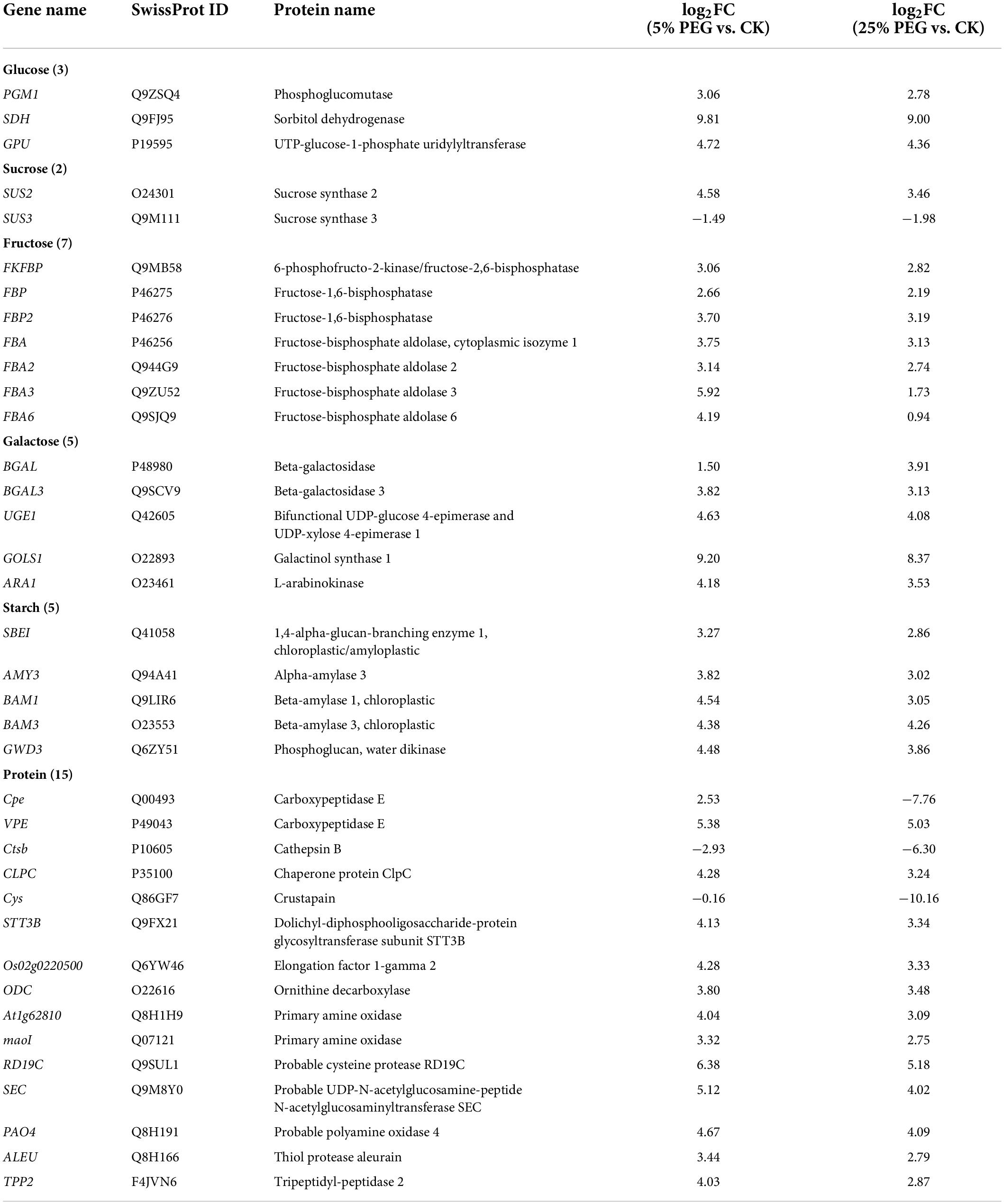
Table 3. Thirty seven DEGs directly associated with soluble sugar and protein metabolism under 5 and 25% PEG vs. CK.
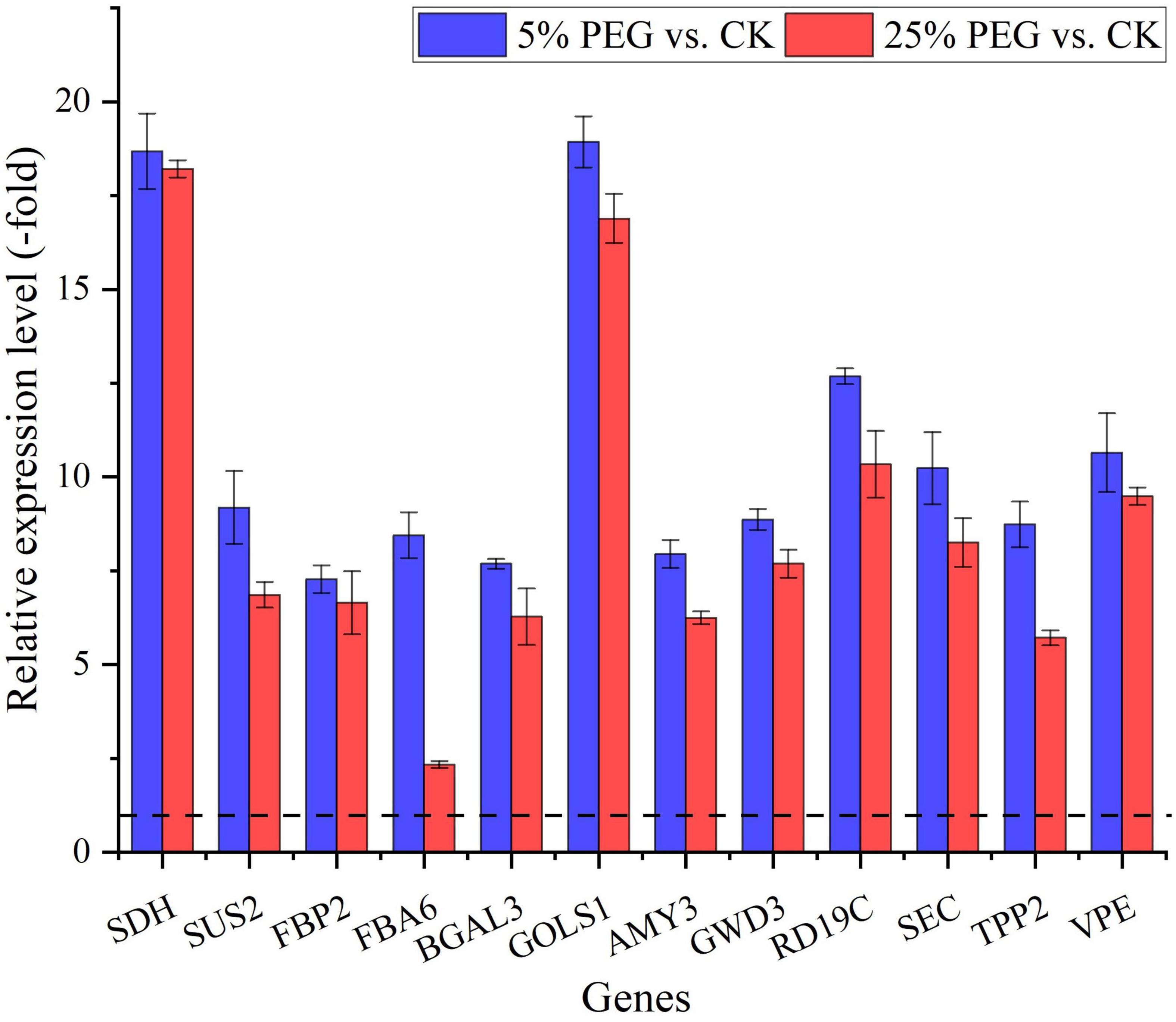
Figure 7. The relative expression level of genes directly associated with soluble sugar and protein metabolism in A. venetum seedlings under 5 and 25% PEG vs. CK, as determined by qRT-PCR (mean ± SD, n = 3).
Differentially expressed genes directly associated with cell morphogenesis for seed germination
In order to highlight seed germination, 8 genes directly associated with seed germination were screened from the 62 DEGs involved in cell morphogenesis including: ANN5, MEE14, REC2, TET8, CESA1, CESA2, CESA3, and LGALS1 (Table 4); and other 54 genes were associated with flower development, fruit development, programmed cell death, etc (Supplementary Table 4). The expression levels of the 8 genes associated with seed germination were validated with all up-regulated at 5 and 25% PEG vs. CK, and their RELs were consistent with RPKM values (Figure 8 and Table 4).
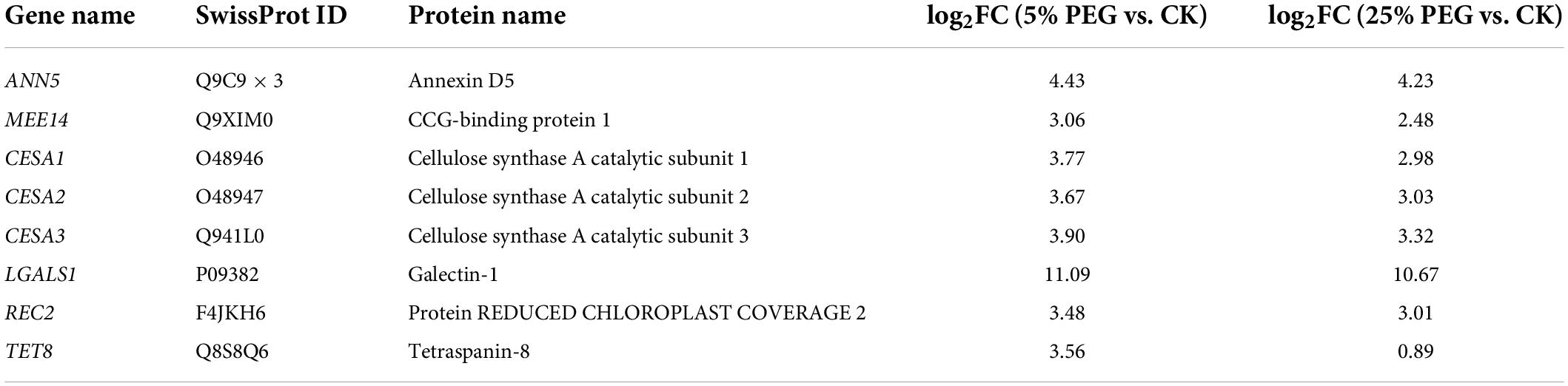
Table 4. Eight genes directly associated with cell morphogenesis for seed germination under 5 and 25% PEG vs. CK.
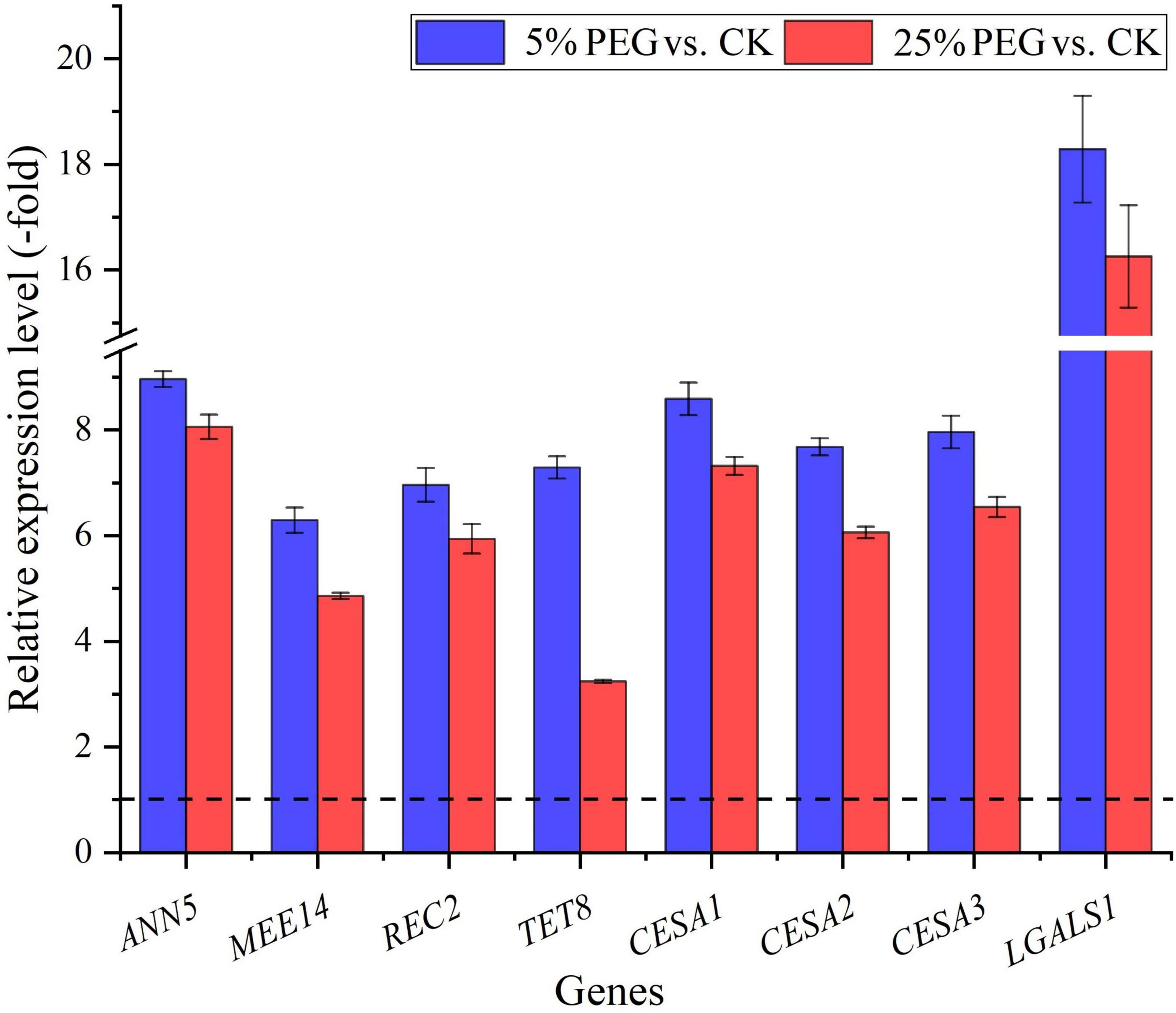
Figure 8. The relative expression level of genes directly associated with cell morphogenesis for seed germination in A. venetum seedlings under 5 and 25% PEG vs. CK, as determined by qRT-PCR (mean ± SD, n = 3).
Transcription factors directly associated with stress response and seed germination
In order to highlight the role of TFs, 5 TFs directly associated with stress response and seed germination were screened from the 19 differentially expressed TFs including: MYB73, WRKY4, NAC083, NAC091, and TCP14 (Table 5); and other 11 TFs were associated with were associated with biotic stress, regulation of transcription by RNA polymerase II, flower development, etc. (Supplementary Table 5). The expression levels of the 4 representative TFs were validated with all up-regulated at 5 and 25% PEG vs. CK, and RELs were consistent with RPKM values (Figure 9 and Table 5).
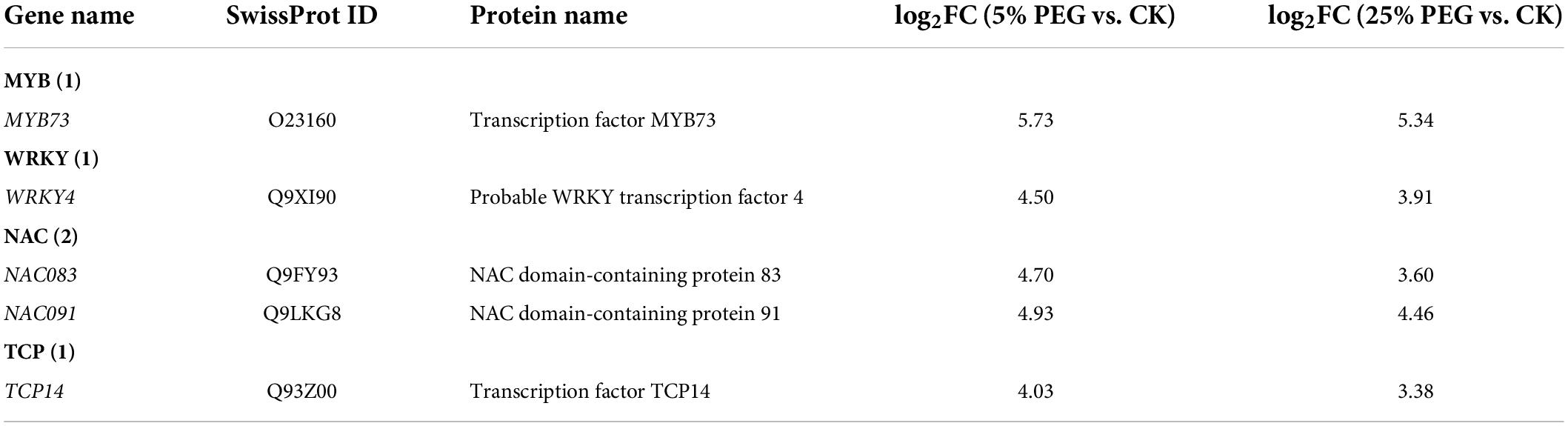
Table 5. Five TFs directly associated with stress response and seed germination under 5 and 25% PEG vs. CK.
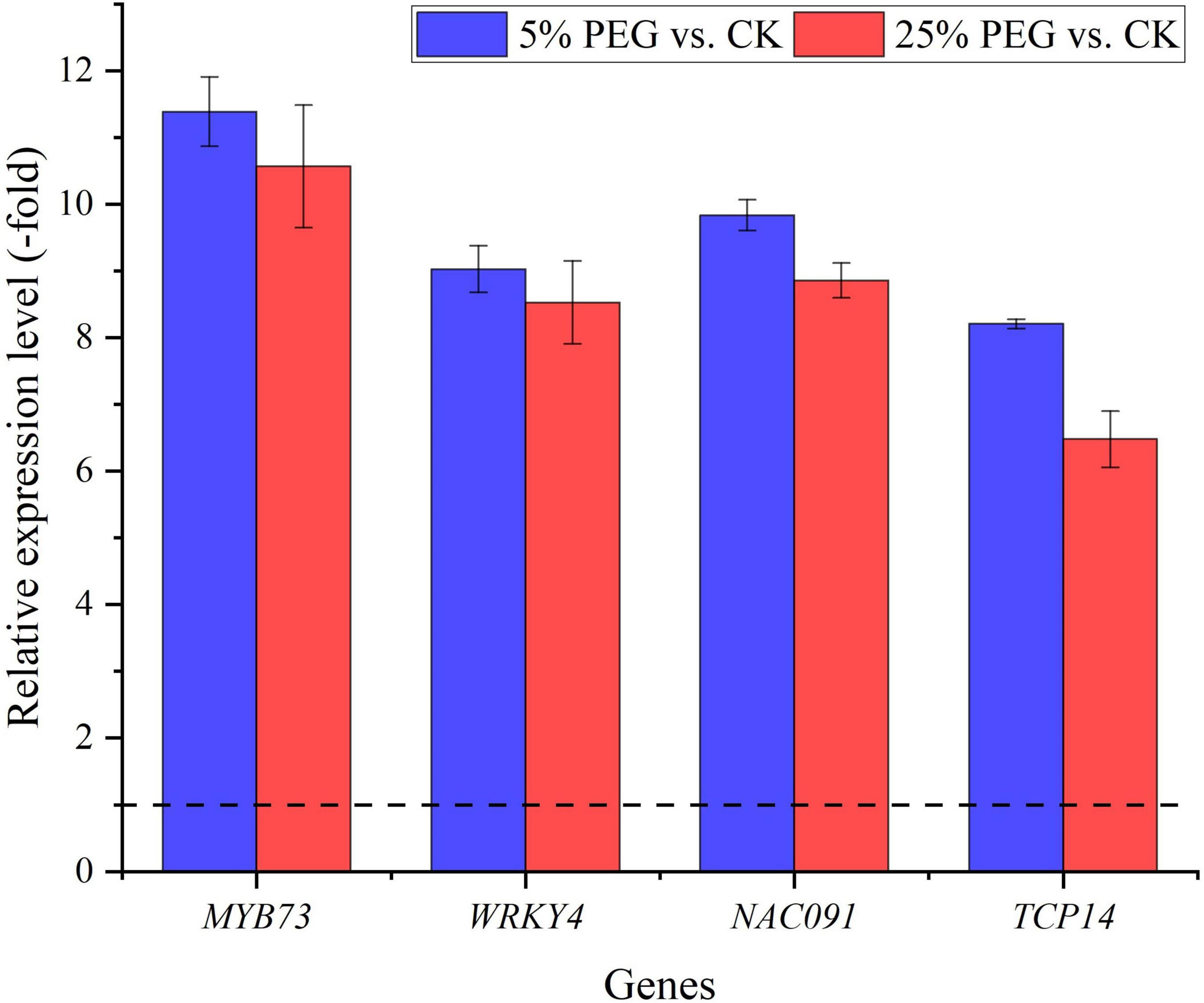
Figure 9. The relative expression level of TFs directly associated with stress response and seed germination in A. venetum seedlings at 5 and 25% PEG vs. CK, as determined by qRT-PCR (mean ± SD, n = 3).
Differentially expressed genes directly associated with hormone response
In order to highlight the role of endogenous hormones in drought stress and seed germination, 23 genes directly associated with hormone response were screened from the 99 DEGs involved in bio-signaling including: GA (HD16, GASA2, and GASA4), IAA (e.g., ABP19A, ARF2B, and IAA14), ABA (e.g., CPK6, RGLG2, and GRDP1), ETH (e.g., ERF013, EIN3, and CTL1) and CTK response (AHK3) (Table 6); and other 76 genes were associated with other bio-signaling such as protein kinases, protein phosphatase, calcium sensor, etc. (Supplementary Table 6). The expression levels of 11 representative genes associated with hormone response were validated, with all up-regulated at 5 and 25% PEG vs. CK, and RELs were consistent with RPKM values (Figure 10 and Table 6).
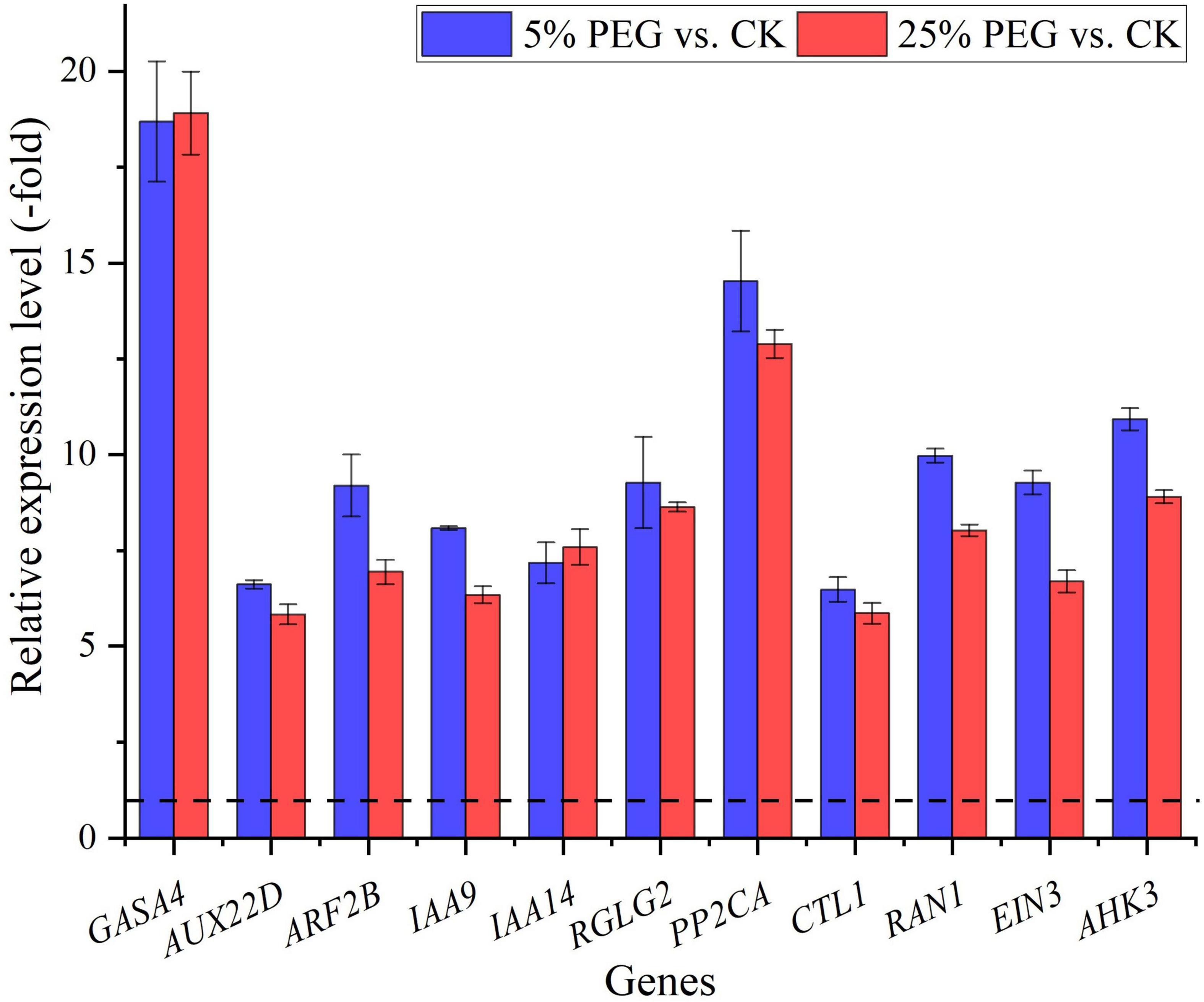
Figure 10. The relative expression level of genes associated with hormone response in A. venetum seedlings under 5 and 25% PEG vs. CK, as determined by qRT-PCR (mean ± SD, n = 3).
Discussion
Under both natural and agricultural conditions, plants are frequently exposed to unfavorable environments such as drought stress, high or low temperature, and salinity, which restrict plant growth and development; while plant adaptations that are genetically determined confer stress resistance (Taiz and Zeiger, 2010). Drought is one of the most environmental stresses that seriously inhibit plant growth by disrupting various physiological and biochemical processes such as nutrition uptake, photosynthesis, and cellular metabolism (Yordanov et al., 2000; Rampino et al., 2006; Rivero et al., 2007; Farooq et al., 2009; Jaleel et al., 2009; Carmo-Silva et al., 2012). Here, we found that the germination characteristics (i.e., germination rate, hypocotyl length, radicle length and fresh weight), antioxidant enzyme activities (i.e., SOD, POD, CAT, and APX), osmolytes contents (i.e., soluble sugar, protein, MDA and Pro), and gene expression were significantly affected during seed germination of A. venetum under different PEG treatments.
Extensive experiments have demonstrated that stress resistance in response to drought stress is involved in the changes of phenotypes (e.g., leaf expansion, leaf abscission and root extension), antioxidant enzyme activities (e.g., SOD, POD, and CAT), and osmolyte contents (e.g., soluble sugar, protein and Pro) (Zhang et al., 2022). In this study, the seed germination rate, hypocotyl length and fresh weight were promoted under 5% PEG (Figure 1); the activities of SOD and POD and contents of soluble sugar, MDA and Pro were increased, while the activities of CAT and APX were decreased with the increase of PEG concentrations (Figures 2, 3). These results indicate that moderate drought can promote seed germination of A. venetum by adjusting the antioxidant enzyme activities and osmolyte contents. Drought response is not only involved in the changes of phenotypes, antioxidant enzymes and osmolytes but also transcriptional alternations (Zhang et al., 2022). Here, 834 DEGs were observed to be co-expressed in A. venetum during seed germination under 5% and 25% PEG vs. CK, with 10 categories classified including: stress response, primary metabolism, cell morphogenesis, TFs, bio-signaling, photosynthesis and energy, secondary metabolism, transport, transcription, and translation (Figures 4, 5).
For the 18 genes directly associated with drought stress and antioxidant enzymes (Table 2), 4 genes (DHN1, RCD1, LIPC, and ASPG1) involved in water deprivation have been observed to be up-regulated in Arabidopsis and Solanum tuberosum under drought stress (Gillet et al., 1998; Ahlfors et al., 2004; Gaudet et al., 2011; Yao et al., 2012). In this study, the 4 genes were also observed to be up-regulated under 5 and 25% PEG (Figure 6). For the biological functions of other 14 genes associated with antioxidant enzymes (Table 2), SODA is involved in reduce the oxidative damage to cells caused by drought (Guo et al., 2013); At5g06290 plays a role in cell protection against oxidative stress by etoxifying peroxides (Horling et al., 2003); PNC1 is involved in response to environmental responses (Schuller et al., 1996); Gpx3 and Gpx4 catalyzes glutathione the reduction of hydrogen peroxide, lipid peroxides and organic hydroperoxide (Esworthy et al., 1991; Yant et al., 2003); PER42 and PER52 are involved in removing H2O2 (Chen and Schopfer, 1999); PRDX1 and PRXIIE catalyze hydrogen peroxide and play a role in cell protection against oxidative stress by detoxifying peroxides (Kang et al., 1998; Rouhier and Jacquot, 2005); PEX11C is involved in peroxisomal proliferation (Orth et al., 2007); CATs (Cat, CAT1, and CAT2) protect cells from the toxic effects of hydrogen peroxide (Gaudet et al., 2011); and CATHB2 is involved in the regulation of senescence, a developmental form of PCD in plants (McLellan et al., 2009). In this study, most of the genes involved in SOD and POD were up-regulated, which is in accordance with the increased SOD and POD activities; and the down-regulation of Cat and CAT2 might play important roles in the decreased CAT activity under drought stress (Figures 2, 6).
For the 37 genes directly associated with soluble sugar and protein metabolism (Table 3), 22 genes were observed to participate in soluble sugar metabolism, for example, SUS2 is involved in providing UDP-glucose and fructose for various metabolic pathways (Angeles-Nunez and Tiessen, 2010); GOLS1 is involved in the biosynthesis of raffinose family oligosaccharides (Panikulangara et al., 2004); and AMY3 is involved in stress-induced starch degradation (Smith et al., 2005). A total of 15 genes were observed to participate in protein metabolism, for example, for example, RD19C and VPE are involved in cellular protein catabolic process (Alonso and Granell, 1995; Gaudet et al., 2011); and TPP2 is involved in degrading oxidized proteins generated by environmental stress (Book et al., 2005). In this study, the up-regulation of these genes is in accordance with the soluble sugar and protein contents under drought stress (Figures 3, 7).
For the biological functions of the 8 genes directly associated with seed germination (Table 4), briefly, ANN5 is involved in germination (Zhu et al., 2014); MEE14 is involved in the development of endosperm (Pagnussat et al., 2005); REC2 can regulate meristematic tissue proliferation by integrating developmental signals (Skylar et al., 2011); TET8 is involved in the regulation of cell differentiation and defense response (Wang et al., 2015); CESAs (CESA1, CESA2 and CESA3) are critical for cell expansion during germination (Burn et al., 2002); and LGALS1 plays a role in regulating apoptosis, cell proliferation and cell differentiation (He and Baum, 2004). Thus, their differential expression could play important roles in seed germination under drought stress (Figure 8).
TFs have been demonstrated to regulate plant growth and metabolism in response to abiotic stresses (Dubos et al., 2010). In this study, 5 TFs directly associated with stress response and seed germination (Table 5). Specifically for the stress response, MYB73 has been found to be involved in salt stress response (Kim et al., 2013); WRKY4 has a positive role in resistance to necrotrophic pathogens while a negative effect on plant resistance to biotrophic pathogens (Lai et al., 2008); and NAC TFs play critical roles in plant abiotic stress responses (Mei et al., 2021). For the seed germination, TCP14 regulates the activation of embryonic growth potential during seed germination in Arabidopsis (Tatematsu et al., 2008). Here, their up-regulation may play critical roles in conferring drought tolerance for seed germination of A. venetum (Figure 9).
For the biological functions of the 23 genes directly associated with hormone response (Table 6), briefly, GASA4 plays a role in the promotion of GA responses such as regulation of seed germination (Roxrud et al., 2007); AUX22D, ARF2B, IAA9 and IAA14 are repressors of auxin response genes (Hashimoto and Yamamoto, 1997; Liscum and Reed, 2002; Hao et al., 2015); RGLG2 acts as a negative regulator of drought stress response (Cheng et al., 2012); PP2CA acts as a major negative regulator of ABA responses during seed germination (Rodrigues et al., 2013); CTL1 is essential for normal plant growth and development and contributes to drought stress (Zhong et al., 2002); RAN1 is essential for ethylene signaling (Alonso et al., 1999); EIN3 acts as a positive regulator in the ethylene response pathway (Zhang et al., 2017); and AHK3 acts as a negative regulator of drought stress responses and ABA signaling (Spichal et al., 2004). As is known, GAs control various aspects of seed germination by activating vegetative growth of the embryo, weakening a growth-constraining endosperm layer surrounding the embryo, and mobilizing stored food reserves of the endosperm; in addition, GAs also stimulate the production of numerous hydrolases, notably α-amylase (Taiz and Zeiger, 2010). Thus, their differential expression may also play critical roles in promoting seed germination of A. venetum under drought stress (Figure 10).
Of course, genes involved in photosynthesis and energy (Supplementary Table 7), secondary metabolism (Supplementary Table 8), transport (Supplementary Table 9), transcription (Supplementary Table 10), and translation (Supplementary Table 11) may also participate in seed germination of A. venetum under drought stress, which will be further analyzed in the following studies. Based on above studies on the physiological and transcriptional changes, a model of moderate drought-enhanced seed germination of A. venetum is proposed (Figure 11). Briefly, when seeds exposed to drought stress, the gene regulatory will be triggered and related genes will be differentially expressed; then the bio-signaling such as hormone response (e.g., GASA4, IAAs, and PP2Cs) will be generated, subsequently, the genes related to drought stress response (e.g., DHN1, SODA, and PERs), soluble sugar metabolism (e.g., PGM1, SUSs, and PBPs), protein metabolism (e.g., Cpe, Ctsb, and CLPC), and TFs (e.g., MYPs, WRKYs, and NACs) will be up-regulated, which will promote the antioxidant enzymes activities (e.g., SOD and POD) and osmolytes contents (e.g., soluble sugar, MDA, and Pro) that protect the cells from water deficit-induced membrane injury; finally, these changes will be in favor of building the cell morphogenesis, enhancing the seed germination, and conferring the ability to adapt to the semi-arid environment.
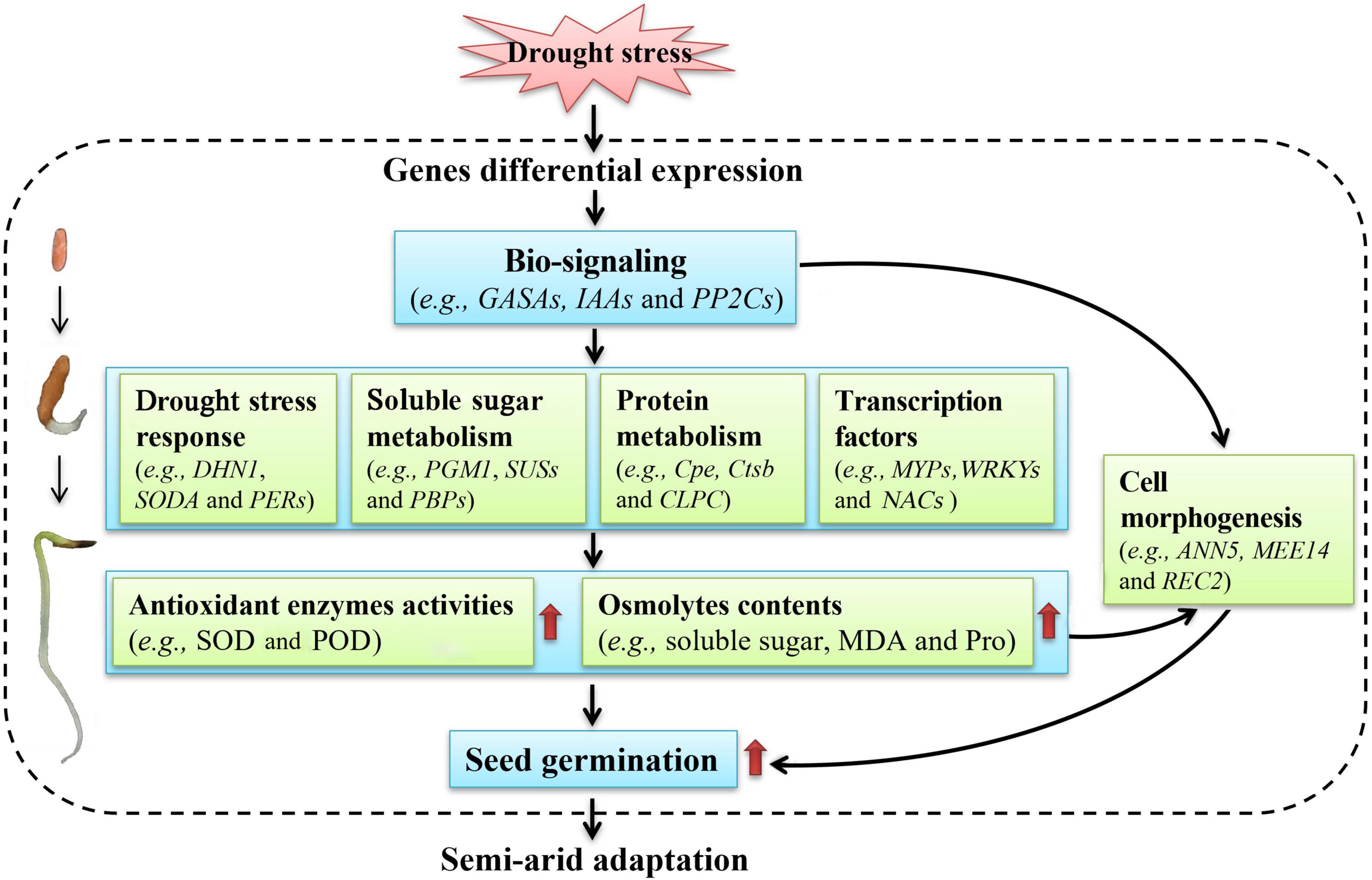
Figure 11. A proposed model of moderate drought-enhanced seed germination of A. venetum adapts to semi-arid environment.
Conclusion
From the above observations, the seed germination of A. venetum can be promoted under moderate drought, and significant changes of antioxidant enzymes activities, osmolytes contents, and genes expression levels in A. venetum were observed during seed germination under different drought stresses, which indicate that A. venetum can be adaptive to drought stress by integrating physiological and transcriptional responses. The specific roles of candidate genes in conferring the ability of drought resistance will require additional studies.
Data availability statement
The datasets presented in this study can be found in online repositories. The names of the repository/repositories and accession number(s) can be found below: https://www.ncbi.nlm.nih.gov/bioproject/PRJNA847749.
Author contributions
ZW: conceptualization, funding acquisition, and writing—original draft preparation. PC, JZ, DL, and WW: resources. XC: data curation, formal analysis, and validation. ML: project administration and writing—review and editing. All authors have read and agreed to the published version of the manuscript.
Funding
This research was funded by the Major Special Projects of Science and Technology in Inner Mongolia Autonomous Region (ZDZX2018057), Key Research and Transformation Projects of Qinghai Province (2019-SF-171), and State Key Laboratory of Aridland Crop Science Gansu Agricultural University (GSCS-2021-Z03).
Conflict of interest
The authors declare that the research was conducted in the absence of any commercial or financial relationships that could be construed as a potential conflict of interest.
Publisher’s note
All claims expressed in this article are solely those of the authors and do not necessarily represent those of their affiliated organizations, or those of the publisher, the editors and the reviewers. Any product that may be evaluated in this article, or claim that may be made by its manufacturer, is not guaranteed or endorsed by the publisher.
Supplementary material
The Supplementary Material for this article can be found online at: https://www.frontiersin.org/articles/10.3389/fevo.2022.975771/full#supplementary-material
Abbreviations
APX, ascorbate peroxidase; CAT, catalase; DEGs, differentially expressed genes; DR, down-regulated; GO, Gene Ontology; GR, glutathione reductase; KEGG, Kyoto Encyclopedia of Genes and Genomes; KOG, euKaryotic orthologous groups of proteins; MDA, malondialdehyde; NCBI, National Center for Biotechnology Information; NR, Non-redundant protein database; PCA, Principal component analysis; PEG, polyethylene glycol; Pro, proline; qRT-PCR, Real time quantitative PCR; RELs, relative expression levels; RPKM, Reads Per kb per Million; SOD, superoxide dismutase; SwissProt, Swiss-protein; TF, transcription factor; UR, up-regulated.
References
Ahlfors, R., Lang, S., Overmyer, K., Jaspers, P., Brosche, M., Tauriainen, A., et al. (2004). Arabidopsis RADICAL-INDUCED CELL DEATH1 belongs to the WWE protein-protein interaction domain protein family and modulates abscisic acid, ethylene, and methyl jasmonate responses. Plant Cell 16, 1925–1937. doi: 10.1105/tpc.021832
Alonso, J. M., and Granell, A. (1995). A putative vacuolar processing protease is regulated by ethylene and also during fruit ripening in Citrus fruit. Plant Physiol. 109, 541–547. doi: 10.1104/pp.109.2.541
Alonso, J. M., Hirayama, T., Roman, G., Nourizadeh, S., and Ecker, J. R. (1999). EIN2, a bifunctional transducer of ethylene and stress responses in Arabidopsis. Science 284, 2148–2152. doi: 10.1126/science.284.5423.2148
Angeles-Nunez, J. G., and Tiessen, A. (2010). Arabidopsis sucrose synthase 2 and 3 modulate metabolic homeostasis and direct carbon towards starch synthesis in developing seeds. Planta 232, 701–718. doi: 10.1007/s00425-010-1207-9
Book, A. J., Yang, P., Scalf, M., Smith, L. M., and Vierstra, R. D. (2005). Tripeptidyl peptidase II. An oligomeric protease complex from Arabidopsis. Plant Physiol. 138, 1046–1057. doi: 10.1104/pp.104.057406
Bradford, M. M. (1976). A rapid and sensitive method for the quantitation of microgram quantities of protein utilizing the principle of protein dye binding. Anal Biochem. 72, 248–254. doi: 10.1016/0003-2697(76)90527-3
Burn, J. E., Hocart, C. H., Birch, R. J., Cork, A. C., and Williamson, R. E. (2002). Functional analysis of the cellulose synthase genes CesA1, CesA2, and CesA3 in Arabidopsis. Plant Physiol. 129, 797–807. doi: 10.1104/pp.010931
Campobenedetto, C., Grange, E., Mannino, G., van Arkel, J., Beekwilder, J., Karlova, R., et al. (2020). A biostimulant seed treatment improved heat stress tolerance during cucumber seed germination by acting on the antioxidant system and glyoxylate cycle. Front Plant Sci. 11:836. doi: 10.3389/fpls.2020.00836
Carmo-Silva, A. E., Gore, M. A., Andrade-Sanchez, P., French, A. N., Hunsaker, D. J., and Salvucci, M. E. (2012). Decreased CO2 availability and inactivation of Rubisco limit photosynthesis in cotton plants under heat and drought stress in the field. Environ Exp Bot. 83, 1–11. doi: 10.1016/j.envexpbot.2012.04.001
Chen, S., Zhou, Y., Chen, Y., and Gu, J. (2018). fastp: an ultra-fast all-in-one FASTQ preprocessor. Bioinformatics. 34, i884–i890. doi: 10.1101/274100
Chen, S. X., and Schopfer, P. (1999). Hydroxyl-radical production in physiological reactions. A novel function of peroxidase. Eur J Biochem. 260, 726±735. doi: 10.1046/j.1432-1327.1999.00199.x
Chen, X. M. (2002). Comparison of three different methods of determining catalase activities in cut flowers. Chin J Trop Agr. 22, 13–16.
Cheng, M. C., Hsieh, E. J., Chen, J. H., Chen, H. Y., and Lin, T. P. (2012). Arabidopsis RGLG2, functioning as a RING E3 ligase, interacts with AtERF53 and negatively regulates the plant drought stress response. Plant Physiol. 158, 363–375. doi: 10.1104/pp.111.189738
Conesa, A., Gotz, S., Garcia-Gomez, J. M., Terol, J., Talon, M., and Robles, M. (2005). Blast2GO: a universal tool for annotation, visualization and analysis in functional genomics research. Bioinformatics 21, 3674–3676. doi: 10.1093/bioinformatics/bti610
Ding, Y. B., and Zhang, H. Y. (2016). Effects of PEG drought simulation on seed germination and seedling growth of platycodon grandiflorum (Jacq.) A. DC. Shandong Agr Sci. 48, 51–53,57.
Dubois, M., Gilles, K. A., Hamilton, J. K., Rebers, P. A., and Smith, F. (1956). Colorimetric method for determination of sugars and related substances. Anal Chem. 28, 350–356. doi: 10.1021/ac60111a017
Dubos, C., Stracke, R., Grotewold, E., Weisshaar, B., Martin, C., and Lepiniec, L. (2010). MYB transcription factors in Arabidopsis. Trends Plant Sci. 15, 573–581. doi: 10.1016/j.tplants.2010.06.005
Esworthy, R. S., Chu, F. F., Paxton, R. J., Akman, S., and Doroshow, J. H. (1991). Characterization and partial amino acid sequence of human plasma glutathione peroxidase. Arch Biochem Biophys. 286, 30–336. doi: 10.1016/0003-9861(91)90048-N
Farooq, M., Wahid, A., Kobayashi, N., Fujita, D., and Basra, S. (2009). Plant drought stress: effects, mechanisms and management. Agron Sustain Dev. 29, 185–212. doi: 10.1051/agro:2008021
Gaudet, P., Livstone, M. S., Lewis, S. E., and Thomas, P. D. (2011). Phylogenetic-based propagation of functional annotations within the Gene Ontology consortium. Brief Bioinform. 12, 449–462. doi: 10.1093/bib/bbr042
Gillet, B., Beyly, A., Peltier, G., and Rey, P. (1998). Molecular characterization of CDSP 34, a chloroplastic protein induced by water deficit in Solanum tuberosum L. plants, and regulation of CDSP 34 expression by ABA and high illumination. Plant J. 16, 257–262. doi: 10.1046/j.1365-313x.1998.00292.x
Grabherr, M. G., Haas, B. J., Yassour, M., Levin, J. Z., Thompson, D. A., et al. (2011). Full-length transcriptome assembly from RNA-Seq data without a reference genome. Nat Biotechnol. 29, 644–652. doi: 10.1038/nbt.1883
Guo, C. K., Ge, X. C., and Ma, H. (2013). The rice OsDIL gene plays a role in drought tolerance at vegetative and reproductive stages. Plant Mol Biol. 82, 239–253. doi: 10.1007/s11103-013-0057-9
Hammerschmidt, R., Nuckles, E. M., and Kuć, J. (1982). Association of enhanced peroxidase activity with induced systemic resistance of cucumber to Colletotrichum lagenarium. Physiol Plant Pathol. 20, 73–82. doi: 10.1016/0048-4059(82)90025-X
Hao, Y., Hu, G., Breitel, D., Liu, M., Mila, I., Frasse, P., et al. (2015). Auxin response factor SlARF2 is an essential component of the regulatory mechanism controlling fruit ripening in tomato. PLoS Genet. 11:E1005649–E1005649. doi: 10.1371/journal.pgen.1005649
Hashimoto, H., and Yamamoto, K. T. (1997). Three more members of the Aux/IAA gene family from mung bean (Vigna radiata) hypocotyl. Plant Gene Register GR97–GR137.
He, J., and Baum, L. G. (2004). Presentation of galectin-1 by extracellular matrix triggers T cell death. J Biol Chem. 279, 4705–4712. doi: 10.1074/jbc.M311183200
Horling, F., Lamkemeyer, P., Koenig, J., Finkemeier, I., Kandlbinder, A., Baier, M., et al. (2003). Divergent light-, ascorbate-, and oxidative stress-dependent regulation of expression of the peroxiredoxin gene family in Arabidopsis. Plant Physiol. 131, 317–325. doi: 10.1104/pp.010017
Hu, H., and Xiong, L. (2014). Genetic engineering and breeding of drought-resistant crops. Annu Rev Plant Biol. 65, 715–741. doi: 10.1146/annurev-arplant-050213-040000
Jaleel, C. A., Manivannan, P., Wahid, A., Farooq, M., Al-Juburi, H. J., Soasundaram, R., et al. (2009). Drought stress in plants: a review on morphological characteristics and pigments composition. Int J Agric Biol. 11, 100–105. doi: 10.3763/ijas.2009.0459
Jiang, L., She, C. W., Tian, C. Y., Tanveer, M., and Wang, L. (2021). Storage period and different abiotic factors regulate seed germination of two Apocynum species-cash crops in arid saline regions in the northwestern China. Front Plant Sci. 12:671157. doi: 10.3389/fpls.2021.671157
Kang, S. W., Chae, H. Z., Seo, M. S., Kim, K., Baines, I. C., and Rhee, S. G. (1998). Mammalian peroxiredoxin isoforms can reduce hydrogen peroxide generated in response to growth factors and tumor necrosis factor-alpha. J Biol Chem. 273, 6297–6302. doi: 10.1074/jbc.273.11.6297
Kim, J. H., Nguyen, N. H., Jeong, C. Y., Nguyen, N. T., Hong, S. W., and Lee, H. (2013). Loss of the R2R3 MYB, AtMyb73, causes hyper-induction of the SOS1 and SOS3 genes in response to high salinity in Arabidopsis. J Plant Physiol. 170, 1461–1465. doi: 10.1016/j.jplph.2013.05.011
Lai, Z., Vinod, K., Zheng, Z., Fan, B., and Chen, Z. (2008). Roles of Arabidopsis WRKY3 and WRKY4 transcription factors in plant responses to pathogens. BMC Plant Biol. 8:68–68. doi: 10.1186/1471-2229-8-68
Li, H. S. (2000). Principles and techniques of plant physiological biochemical experimental. Beijing: High Education Press, 260–261.
Li, M. F., Sun, P., Kang, T. L., Xing, H., Yang, D. L., Zhang, J. L., et al. (2018). Mapping podophyllotoxin biosynthesis and growth related transcripts with high elevation in Sinopodophyllum hexandrum. Ind Crop Prod. 124, 510–518. doi: 10.1016/j.indcrop.2018.08.007
Liscum, E., and Reed, J. W. (2002). Genetics of Aux/IAA and ARF action in plant growth and development. Plant Mol Biol. 49, 387–400. doi: 10.1023/A:1015255030047
Liu, Z. H., Wang, N., Chen, L. Y., and Geng, L. (2015). Measurement and analysis of physiological and biochemical indexes during natural aging on Apocynum seed. Seed 34, 43–46. doi: 10.15258/sst.2015.43.2.22
Love, M. I., Huber, W., and Anders, S. (2014). Moderated estimation of fold change and dispersion for RNAseq data with DESeq2. Genome Biol. 15, 550. doi: 10.1186/s13059-014-0550-8
Ma, J., Li, J. Z., and Liu, X. M. (2000). Micromorphological characters of the seeds of Apocynum venetum and Poacynum hendersonii. Acta Bot Boreali-Occidentalia 20, 476–479.
McLellan, H., Gilroy, E. M., Yun, B. W., Birch, P. R., and Loake, G. (2009). Functional redundancy in the Arabidopsis cathepsin B gene family contributes to basal defence, the hypersensitive response and senescence. New Phytol. 183, 408–418. doi: 10.1111/j.1469-8137.2009.02865.x
Mei, F., Chen, B., Li, F., Zhang, Y., Kang, Z., Wang, X., et al. (2021). Overexpression of the wheat NAC transcription factor TaSNAC4-3A gene confers drought tolerance in transgenic Arabidopsis. Plant Physiol Biochem. 160, 37–50. doi: 10.1016/j.plaphy.2021.01.004
Ning, J. F., Zheng, Q. S., Yang, S. H., Zou, X. Z., Sun, L. L., and Chen, Y. (2010). Impact of high salt stress on Apocynum venetum growth and ionic homeostasis. Chin J Appl Ecol. 21, 325–330. doi: 10.3724/SP.J.1142.2010.40521
Orth, T., Reumann, S., Zhang, X., Fan, J., Wenzel, D., Quan, S., et al. (2007). The PEROXIN11 protein family controls peroxisome proliferation in Arabidopsis. Plant Cell. 19, 333–350. doi: 10.1105/tpc.106.045831
Pagnussat, G. C., Yu, H. J., Ngo, Q. A., Rajani, S., Mayalagu, S., Johnson, C. S., et al. (2005). Genetic and molecular identification of genes required for female gametophyte development and function in Arabidopsis. Development. 132, 603–614. doi: 10.1242/dev.01595
Panikulangara, T. J., Eggers-Schumacher, G., Wunderlich, M., Stransky, H., and Schoeffl, F. (2004). Galactinol synthase1. A novel heat shock factor target gene responsible for heat-induced synthesis of raffinose family oligosaccharides in Arabidopsis. Plant Physiol. 136, 3148–3158. doi: 10.1104/pp.104.042606
Pei, S. S., Yin, M. Q., Wen, Y. Y., Huang, M. J., Zhang, B., Guo, P. Y., et al. (2014). Physiological response of Foxtail Millet to drought stress during seed germination and drought resistance evaluation. J Nucl Agr Sci. 28, 1897–1904.
Ping, X. Y., Lin, C. T., Bai, Y., Liu, Q. T., and Lu, X. S. (2014). The ecological effects of planting Apocynum venetum in the plain of the Altay Region, Xinjiang province. Acta Prat Sinica 23, 49–58.
Qi, R. L., Sharen, T. Y., Wang, M. Z., Haa, L., and Yang, W. B. (2021). Seed germination characteristics of Halimodendron halodendron (Pall.) Voss under salt and drought stress. Northern Hort. 15, 81–88.
Rampino, P., Pataleo, S., Gerardi, C., Mita, G., and Perrotta, C. (2006). Drought stress response in wheat: physiological and molecular analysis of resistant and sensitive genotypes. Plant Cell Environ. 29, 2143–2152. doi: 10.1111/j.1365-3040.2006.01588.x
Ren, J., Yu, F. K., and Tao, L. (2011). Research advances on the germination of desert plants under stress. Bull Bot Res. 31, 121–128. doi: 10.3724/SP.J.1011.2011.00110
Rivero, R. M., Kojima, M., Gepstein, A., Sakakibara, H., Mittler, R., Gepstein, S., et al. (2007). Delayed leaf senescence induces extreme drought tolerance in a flowering plant. Proc Natl Acad Sci USA. 104, 19631–19636. doi: 10.1073/pnas.0709453104
Rodrigues, A., Adamo, M., Crozet, P., Margalha, L., Confraria, A., Martinho, C., et al. (2013). ABI1 and PP2CA phosphatases are negative regulators of Snf1-related protein kinase1 signaling in Arabidopsis. Plant Cell. 25, 3871–3884. doi: 10.1105/tpc.113.114066
Rohamare, Y., Dhumal, K. N., and Nikam, T. D. (2014). Response of Ajowan to water stress induced by polyethylene glycol (PEG) 6000 during seed germination and seedling growth. J Environ Biol. 35, 789–793. doi: 10.1007/s11367-014-0772-8
Rong, Y. P., Li, H. X., and Johnson, D. A. (2015). Germination response of Apocynum venetum seeds to temperature and water potential. J Appl Bot Food Qual. 88, 202–208.
Rouhier, N., and Jacquot, J. P. (2005). The plant multigenic family of thiol peroxidases. Free Radic Biol Med. 38, 1413–1421. doi: 10.1016/j.freeradbiomed.2004.07.037
Rouzi, A., Halik, U., Thevs, N., Welp, M., and Aishan, T. (2018). Water efficient alternative crops for sustainable agriculture along the Tarim basin: a comparison of the economic potentials of Apocynum pictum, Chinese red date and cotton in Xinjiang, China. Sustainability 10, 35. doi: 10.3390/su10010035
Roxrud, I., Lid, S. E., Fletcher, J. C., Schmidt, E. D., and Opsahl-Sorteberg, H. G. (2007). GASA4, one of the 14-member Arabidopsis GASA family of small polypeptides, regulates flowering and seed development. Plant Cell Physiol. 48, 471–483. doi: 10.1093/pcp/pcm016
Schuller, D. J., Ban, N., van Huystee, R. B., McPherson, A., and Poulos, T. L. (1996). The crystal structure of peanut peroxidase. Structure. 4, 311–321. doi: 10.1016/S0969-2126(96)00035-4
Shen, W. B., Xu, L. L., Ye, M. B., and Zhang, R. X. (1996). Study on determination of ASP activity. Plant Physiol Comm. 32, 203–205.
Shi, W., Xu, H. L., Zhao, X. F., Ling, H. B., and Li, Y. (2010). Physiological and biochemical responses to drought stress during seed germination of Glycyrrhiza inflata. Acta Ecologica Sinica 30, 2112–2117. doi: 10.1080/00949651003724790
Shinozaki, K., and Yamaguchi-Shinozaki, K. (2007). Gene networks involved in drought stress response and tolerance. J Exp Bot. 58, 221–227. doi: 10.1093/jxb/erl164
Skylar, A., Sung, F., Hong, F., Chory, J., and Wu, X. (2011). Metabolic sugar signal promotes Arabidopsis meristematic proliferation via G2. Dev Biol. 351, 82–89. doi: 10.1016/j.ydbio.2010.12.019
Smith, A. M., Zeeman, S. C., and Smith, S. M. (2005). Starch degradation. Annu Rev Plant Biol. 56, 73–98. doi: 10.1146/annurev.arplant.56.032604.144257
Spichal, L., Rakova, N. Y., Riefler, M., Mizuno, T., Romanov, G. A., Strnad, M., et al. (2004). Two cytokinin receptors of Arabidopsis thaliana, CRE1/AHK4 and AHK3, differ in their ligand specificity in a bacterial assay. Plant Cell Physiol. 45, 1299–1305. doi: 10.1093/pcp/pch132
Stewart, R. R., and Bewley, J. D. (1980). Lipid peroxidation associated with accelerated aging of soybean axes. Plant Physiol. 65, 245–248. doi: 10.1104/pp.65.2.245
Taiz, L., and Zeiger, E. (2010). “Plant Physiology,” in Responses and Adaptations to Abiotic Stress, eds J. Radin, R. Bressan, M. C. Drew, P. M. Hasegawa, R. Locy, M. V. Mickelbart, et al. (Sunderland MA: Sinauer Associates Inc.), 620–621.
Tatematsu, K., Nakabayashi, K., Kamiya, Y., and Nambara, E. (2008). Transcription factor AtTCP14 regulates embryonic growth potential during seed germination in Arabidopsis thaliana. Plant J. 53, 42–52. doi: 10.1111/j.1365-313X.2007.03308.x
Tong, S. M., Xi, H. X., Ai, K. J., and Hou, H. S. (2017). Overexpression of wheat TaNCED gene in Arabidopsis enhances tolerance to drought stress and delays seed germination. Biol Plantarum 61, 64–72. doi: 10.1007/s10535-016-0692-5
Wang, F., Muto, A., Van de Velde, J., Neyt, P., Himanen, K., Vandepoele, K., et al. (2015). Functional analysis of the Arabidopsis TETRASPANIN Gene Family in plant growth and development. Plant Physiol. 169, 2200–2214. doi: 10.1104/pp.15.01310
Willems, E., Leyns, L., and Vandesompele, J. (2008). Standardization of real-time PCR gene expression data from independent biological replicates. Anal Biochem. 379, 127–129. doi: 10.1016/j.ab.2008.04.036
Xie, W. Y., Zhang, X. Y., Wang, T., and Hu, J. J. (2012). Botany, traditional uses, phytochemistry and pharmacology of Apocynum venetum L. (Luobuma): a review. J Ethnopharmacol. 141, 1–8. doi: 10.1016/j.jep.2012.02.003
Xu, Z. P., Wan, T., Cai, P., Zhang, Y. R., Yu, J., and Meng, C. (2015). Effects of PEG simulated drought stress on germination and physiological properties of Apocynum venetum seeds. Chin J Grassland 37, 75–80.
Yant, L. J., Ran, Q., Rao, L., Van Remmen, H., Shibatani, T., Belter, J. G., et al. (2003). The selenoprotein GPX4 is essential for mouse development and protects from radiation and oxidative damage insults. Free Radic Biol Med. 34, 496–502. doi: 10.1016/S0891-5849(02)01360-6
Yao, X., Xiong, W., Ye, T., and Wu, Y. (2012). Overexpression of the aspartic protease ASPG1 gene confers drought avoidance in Arabidopsis. J Exp Bot. 63, 2579–2593. doi: 10.1093/jxb/err433
Yordanov, I., Velikova, V., and Tsonev, T. (2000). Plant responses to drought, acclimation, and stress tolerance. Photosynthetica. 38, 171–186. doi: 10.1023/A:1007201411474
Zhang, F., Wang, L., Qi, B., Zhao, B., Ko, E. E., Riggan, N. D., et al. (2017). EIN2 mediates direct regulation of histone acetylation in the ethylene response. Proc Natl Acad Sci USA. 114, 10274–10279. doi: 10.1073/pnas.1707937114
Zhang, H. M., Zhu, J. H., Gong, Z. Z., and Zhu, J. K. (2022). Abiotic stress responses in plants. Nat Rev Genet. 23, 104–119. doi: 10.1038/s41576-021-00413-0
Zhang, X., Li, R., and Shi, F. (2007). Effects of salt stress on the seed germination of Apocynum venetum. Acta Scientiarum Universitatis Nankaiensis 40, 13–18. doi: 10.1016/S1262-3636(14)72198-6
Zhang, Y. K., Li, Y., Zhang, J. H., Song, D. W., and Wang, Y. Y. (2019). Effects of salt damage and simulated drought conditions on seed germination of Artemisia Argyi. Seed 38, 7–11.
Zhao, H., and Dai, H. P. (2015). Physiological response of Apocynum venetum L. seedlings osmotic stress. Bangl J Bot. 44, 551–556. doi: 10.3329/bjb.v44i4.38569
Zhao, L., Hu, Y., Chong, K., and Wang, T. (2010). ARAG1, an ABA-responsive DREB gene, plays a role in seed germination and drought tolerance of rice. Ann Bot. 105, 401–409. doi: 10.1093/aob/mcp303
Zhong, R., Kays, S. J., Schroeder, B. P., and Ye, Z. H. (2002). Mutation of a chitinase-like gene causes ectopic deposition of lignin, aberrant cell shapes, and overproduction of ethylene. Plant Cell. 14, 165–179. doi: 10.1105/tpc.010278
Zhu, J., Wu, X., Yuan, S., Qian, D., Nan, Q., An, L., et al. (2014). Annexin5 plays a vital role in Arabidopsis pollen development via Ca2+-dependent membrane trafficking. PLoS One. 9:e102407–e102407. doi: 10.1371/journal.pone.0102407
Zhu, J. J., Kang, H. Z., Tan, H., and Xu, M. L. (2006). Effects of drought stresses induced by polyethylene glycol on germination of Pinus sylvestris var. mongolica seeds from natural and plantation forests on sandy land. J For Res. 11, 319–328. doi: 10.1007/s10310-006-0214-y
Keywords: Apocynum venetum, moderate drought, seed germination, physiological change, transcriptional change, semi-arid environment
Citation: Wu Z, Chang P, Zhao J, Li D, Wang W, Cui X and Li M (2022) Physiological and transcriptional responses of seed germination to moderate drought in Apocynum venetum. Front. Ecol. Evol. 10:975771. doi: 10.3389/fevo.2022.975771
Received: 22 June 2022; Accepted: 04 August 2022;
Published: 07 September 2022.
Edited by:
Dechang Cao, Kunming Institute of Botany (CAS), ChinaReviewed by:
Jin-Lin Zhang, Lanzhou University, ChinaHao Ma, Nanjing Agricultural University, China
Copyright © 2022 Wu, Chang, Zhao, Li, Wang, Cui and Li. This is an open-access article distributed under the terms of the Creative Commons Attribution License (CC BY). The use, distribution or reproduction in other forums is permitted, provided the original author(s) and the copyright owner(s) are credited and that the original publication in this journal is cited, in accordance with accepted academic practice. No use, distribution or reproduction is permitted which does not comply with these terms.
*Correspondence: Mengfei Li, bG1mQGdzYXUuZWR1LmNu