- 1Sección Etología, Facultad de Ciencias, Universidad de la República, Montevideo, Uruguay
- 2Departamento de Ecología, Center of Applied Ecology and Sustainability (CAPES), Pontificia Universidad Católica de Chile, Santiago, Chile
- 3Departamento de Ciencias Ecológicas, Facultad de Ciencias, Universidad de Chile, Santiago, Chile
Both the mean and the variation in environmental temperature are increasing globally. Indeed, the predicted increases in temperature range from 2 to 4°C in the next 50 years. Ectotherms control body temperature by means of behavior selecting microsites with different temperatures, which makes them more susceptible to changes in climate. Nevertheless, lizards living in high mountain environments have developed several mechanisms to inhabit and colonize variable environments with extreme temperatures. These mechanisms include a high metabolism to be active at lower temperatures and viviparity to improve embryonic development. Despite behavioral thermoregulation acting as a buffer to changes in environmental temperature, other traits such as life-history traits may be less flexible. Consequently, in an attempt to understand how lizards cope with harsh habitats, we evaluated some physiological traits and responses of females of Liolaemus bellii from two contrasting slope sites with differences in environmental temperature and humidity, but at the same altitude in the southern Andes range. We collected pregnant females from opposite slopes and maintained them until parturition in a common-garden experiment. Females from the south-facing slope (S-slope) had higher preferred body temperature (Tpref) values before and after parturition and exhibited higher daily energy expenditure before parturition. Nevertheless, no difference in Tpref was shown by their offspring, suggesting a developmental plastic response or adaptation to lower environmental temperature. For instance, the higher metabolism during pregnancy could be associated with a shorter activity period on the snowy S-slope. Additionally, females from the S-slope had larger kidneys and gave birth later than N-slope females, likely due to developmental plasticity or genetic differentiation. How fixed these traits are, in individuals from the contrasting slopes, will determine the response capacity of the L. bellii population to climate change.
Introduction
Global air temperature has increased by 0.12°C over the past decades, with a projected increase ranging from 1.0 to 4.0°C (IPCC Climate Change, 2013). How organisms may respond to increasing temperature is a complex but critical challenge (Lavergne et al., 2010). Ectotherms are particularly susceptible to climate change because their vital rates are directly influenced by environmental temperature (Paaijmans et al., 2013). Consequently, most research has focused on understanding how these organisms, particularly their life-history traits, will be affected by increasing temperature (Angilletta, 2009). As temperatures increase, organisms respond through genetic adaptation (Hoffmann and Sgrò, 2011), phenotypic plasticity (Chevin et al., 2010), shifting their geographical range (Parmesan et al., 1999; Hill et al., 2002; Moreno-Rueda et al., 2012), or by a combination of these processes.
Behavioral traits are commonly more labile and flexible than morphological, or even physiological ones (Mayr, 1959; Blomberg et al., 2003), thus the ability to control body temperature behaviorally could be beneficial to respond to temperature changes. Nonetheless, some authors argue that regulatory behavior could inhibit evolutionary change in thermal physiology traits (Huey et al., 2003). The evidence for lability in thermoregulatory behavior is mixed. For example, preferred body temperature (Tpref) responds to environmental temperature in spiders, with a phylogenetic correlation between the temperature of the activity period and Tpref in several Tarantula species (Montes de Oca et al., 2020). On the other hand, among several lizard phylogenetic clades, Tpref is historically constrained (Azócar et al., 2013), which makes it difficult for species to respond to climate change. Species with a limited capacity to adapt to climate change may be forced to migrate or move to colder places, especially in species with dispersal capacity such as butterflies or dragonflies (Parmesan et al., 1999; Hill et al., 2002). Indeed, several such species have shown poleward shifts in their geographical distribution (Thomas, 2010), some having moved their distribution upwards in elevational gradients (Enriquez-Urzelai et al., 2019; Zu et al., 2021). It is thus worth pondering what could happen with species at their upper limit in an elevation gradient and/or with low dispersal capacity. This is a major problem for most mountain species facing climate change, whose particular environments present formidable barriers for dispersal. Additionally, several mountain species have evolved to adapt to colder temperatures, which could be an evolutionary dead end Pincheira-Donoso et al., 2013, making it imperative to understand their flexibility and response capacity to increasing environmental temperature.
Recent work in lizards has focused on phenotypic plasticity of thermal traits in response to environmental temperature (Refsnider et al., 2018) and to humidity (Rozen-Rechels et al., 2021) with pregnant lizards shown to be sensitive to both environmental variables (Dupoué et al., 2020). Since body temperature (Tb) has a non-linear effect on most ectotherm performance variables (Angilletta, 2009; Bozinovic et al., 2011), behavioral thermoregulation needs to be studied. Indeed, most mountain lizards have evolved in response to lower temperatures by increasing their metabolism (Plasman et al., 2020) and by modifying their life-history traits, showing egg retention and viviparity (Mathies and Andrews, 1995). The most accepted hypothesis for the evolution of viviparity in squamate reptiles is the cold climate model (Tinkle and Gibbons, 1977; Shine, 1983; Pyron and Burbrink, 2014), which proposes that parental care through egg retention is similar to nest guarding or offspring providing (Shine and Brown 2008). Hence, egg retention may be beneficial to embryonic development by providing maternal thermoregulation.
The environmental heterogeneity in mountains could drive adaptative processes over short distances (Nevo, 1995). Genotypic and phenotypic differences across taxa and over short distances have been reported in the northern hemisphere, with taxa from bacteria to insects responding to this “evolution canyon” (Nevo, 1995). South-facing slopes (S-slope) in canyons north of the equator receive higher solar radiation than north-facing slopes (N-slope), which generates significant microsite climatic differences (Sikorski and Nevo, 2005; Nevo, 2009, 2012). In the southern hemisphere, where south-facing slopes receive less solar radiation than north-facing slopes, studies have focused mostly on plant communities (Badano et al., 2005), showing for example, that flowering in N-slopes starts earlier than in S-slopes (Rozzi et al., 1989). In this study, we measured several physiological and life-history traits (e.g., Tpref, metabolism, internal organs, and parturition day) in reproductive females of an Andean lizard, Liolaemus bellii, in response to contrasting environmental temperatures and humidity between S-slopes and N-slopes. Specifically, we performed a common-garden experiment with pregnant females of L. bellii from two contrasting facing slopes at similar elevations maintained under the same laboratory conditions.
Materials and methods
Species model
Liolaemus bellii is a viviparous lizard, giving birth to five offspring on average in early summer; it is endemic to Chile and distributed in the Andean areas of Valparaiso and Metropolitana Regions, inhabiting rocky environments from 2,050 to 3,053 meters above sea level (m.a.s.l.) (Donoso-Barros, 1966; Labra et al., 2001; Núñez et al., 2010; Pincheira-Donoso et al., 2013). We studied females from two accessible sites with similar altitudes: The south-facing slope at La Parva (33° 19’ 59” S, 70° 17’ 06” W, 2852 m a.s.l.) and the north-facing slope at El Colorado (33° 20’ 32”S, 70° 17’ 19”W, 2778 m a.s.l.). Aerial photographs from both sites are shown in Supplementary Figure 1. To characterize the temperatures and humidity of microsites, we placed three temperature and humidity data loggers (iButton hygrochrons) for 1 week in each site in December 2014. One data logger was placed on a rock fully exposed to the sun, another under a rock inside a crevice, and the last was partially covered under a shrub.
Animal collection and husbandry
A total of 39 pregnant females (16 from La Parva and 23 from El Colorado) were collected from November to January in three consecutive austral summers (2012–2015). They were captured with snare traps and transported to the laboratory (570 m.a.s.l.) on the same day. They were weighed and measured (total and snout to vent length, TL and SVL, respectively) the same day and each was placed individually in a terrarium (35 × 25 × 45 cm) with fine sediment and rocks from the capture locality. Water was given ad libitum, and a paper cylinder was provided as shelter. They were fed with larvae of Tenebrio molitor 3 days per week during the entire experimental period. They were maintained at room temperature (24°C) with a natural photoperiod, under natural light, and with water in a Petri dish. Each terrarium had an infrared lamp (250 W) to provide a thermal gradient during daytime; lights were switched on for 1 h and off for another hour, from 1,000 to 1800. The date of parturition and litter size was recorded for each female.
Measurements of Tpref
Measurements were taken every week before parturition and once after parturition. Females were placed in a terrarium of ca. 150 × 40 × 30 cm that had in one extreme a 250 W infrared lamp and ice packs under sand in the opposite extreme, to generate a thermal gradient of 15 to 45°C. After 2 hours of habituation, we registered surface body temperature (Ts) with a laser thermometer (Minitemp, Raytek) placed at 15 cm from each individual. To be sure that Ts was a good proxy of lizard core body temperature (Tb), we measured Ts as described above, and immediately after we registered Tb in the cloaca through Cu-Constantan thermocouples. We repeated both measurements in 31 individuals along a range of body temperatures (Supplementary Figure 2). Because we found a positive and significant relation between both temperatures, hereafter, we label Ts as Tpref. As females gave birth in the laboratory, we also measured Tpref once in the offspring during the first 33 days after birth (13 ± 11 days, mean and standard deviation). A total of 77 offspring from 16 females were measured.
Respirometry
Metabolic records were taken of each female weekly before parturition and once after parturition. The daily energy expenditure (DEE) was estimated through indirect calorimetry in a flow-through respirometry system (Sable systems, Inc.) which recorded an individual’s CO2 production over 24 h. Each female was placed in a 1 L glass metabolic chamber inside of a temperature-controlled cabinet (30 ± 0.5°C) where the chamber received room air previously passed through columns of water and CO2 filters (Drierite® and Baralyme®, respectively) at a rate of 200 ml/min−1. The excurrent air was passed again through a column of Drierite before passing through a CO2 analyzer (FoxBox, Sable System, Henderson, Nevada, USA). Each respirometry trial lasted for at least 25.5 h. For DEE estimation, we used the area under the CO2 production curve of the last 24 h from the record. Despite individuals not being fed inside the climatic cabinet, they were seen to carry on all normal activities such as locomotion (Lighton, 2018). From the record, we estimated MR during the day by averaging CO2 production of the lowest 10 min of inactivity between 1,100 and 1,500; we named this measure daily metabolic rate (MRday). The same procedure was used to estimate a nightly metabolic rate (MRnight) by averaging the lowest 10 min of inactivity between 1,100 and 0300. As females were reproductive and non-fasted for CO2 records, we cannot call it standard metabolic rate (SMR). Outputs from the CO2 analyzer (%) and the flow meter were digitalized using Universal Interface II (Sable Systems, Henderson, Nevada, USA) and recorded on a personal computer using EXPEDATA data acquisition software (Sable Systems, Henderson, Nevada, USA).
Internal organs
After parturition, females were sacrificed according to ethics protocols (see ethics statement below) and dissected to remove internal organs (liver, kidneys, heart, lungs, reproductive tract, digestive tract, and abdominal fat bodies). All organs were washed with 0.9% NaCl solution, dried at 60°C together with its carcass and tail, and then weighed (precision 0.0001 g, PRECISA, HF 225SM-DR, series H390) periodically until all water content was removed and weight remained constant.
Statistical analysis
To determine physiological differences between sites, we fitted general linear mixed models (GLMM) to each response variable (DEE, MRnigth, MRday, and Tpref). Female was added as a random intercept to account for inter-female variation in the models (Zuur et al., 2009). In the corresponding table, we show the sample size (i.e., females) for each variable because we have different sample sizes for each. This is due to some females giving birth before metabolism was measured. Because we were not able to predict when females would give birth, we measured Tpref and metabolism weekly. This way, we ensured a standardized period of time before parturition to record this trait. For metabolism variables (DEE, MRnight, and MRday), we fitted GLMM to each response before and after parturition with body mass (mb), site, and their interaction term as predictor variables. To test Tpref differences between females, we fitted GLMMs with the site, Time of Tpref measurement (before or after parturition), and their interaction term as predictor variables. To estimate differences between dates of parturition, we first converted the date to the number of days from the first day of the year (January 01). We were not able to use Julian calendar days because when the dates were converted, they showed large differences between, for example, December 31 (2014365 Julian calendar days) and January 01 (2015001 Julian calendar days) when there is a 1-day difference. We fitted a GLMM with body mass (mb), site, and the interaction between body mass and site as predictors. To test for differences in female internal organs between sites, we performed GLMMs with the mass of each organ as response variable and site, mb, and their interaction as predictors. We also checked if Tpref varied with the date of measurement for the offspring. After that, we fitted nested models to test the Tpref differences of the offspring between sites, with mb and site as predictors, and female as a random effect. Model selection was performed using Akaike’s criterion (Akaike, 1974, 1998) and a log ratio test (Zuur et al., 2009). For all fitted models, assumptions were checked visually (Zuur et al., 2009). All statistical analyses were performed using R software (R Core Team, 2018) and the packages nlme (3.1–137) (Pinheiro et al., 2017), MuMIn (1.42.1) (Barton, 2009), multcomp (1.4–8) (Hothorn et al., 2016), and ggplot2 (3.3.0) (Wickham et al., 2016).
Results
Environment description
We observed differences in temperature between both slope sites for the three microsites during all recorded days (Supplementary Figures 3, 4). As expected, the N-slope site showed higher temperatures than the S-slope. We also observed higher relative humidity on the S-slope. The aerial images showed that differences between sites involved snow cover during spring, with the south-facing slope remaining with snow cover until October, while the north-facing slope was completely snow-free by then (Supplementary Figure 1). Additionally, during May, the S-slope exhibited snow cover, while the N-slope did not (Supplementary Figure 1).
Preferred body temperatures
We found a significant difference in the Tpref of animals from the different sites. N-slope females had higher Tpref values than those from the S-slope (Figure 1; Supplementary Table 1). We also found differences in Tpref at the time of measurement; with pregnant females having lower body temperatures than those after parturition regardless of the site (Figure 1; Table 1). The best model for Tpref included site and time (Table 1), with females from the south-facing site showing lower body temperatures than those from the north-facing site before and after parturition (Figure 1). Together, predictor variables explained 14% of the variance and the random effect (females plus fixed part) explained 38% of the variance. This means that there is important variation explained at the individual level. We did not find a significant effect of time of measurement (t value = 1.795, df = 75, p = 0.08) or site on the Tpref of offspring (t value = 0.311, df = 13, p = 0.976) (Figure 2).
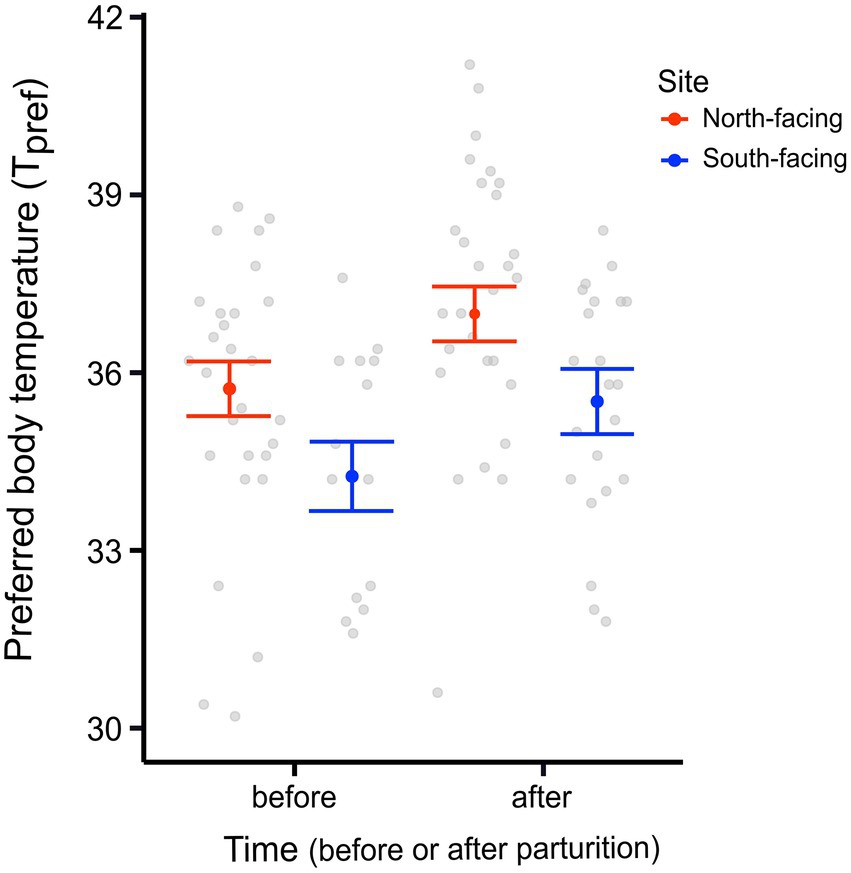
Figure 1. Preferred body temperature (Tpref) of adult females from two L. bellii sites at North-facing and South-facing slopes before and after parturition. Filled dots represent the mean, whiskers indicate SE estimated from the best model, and open dots are raw data.
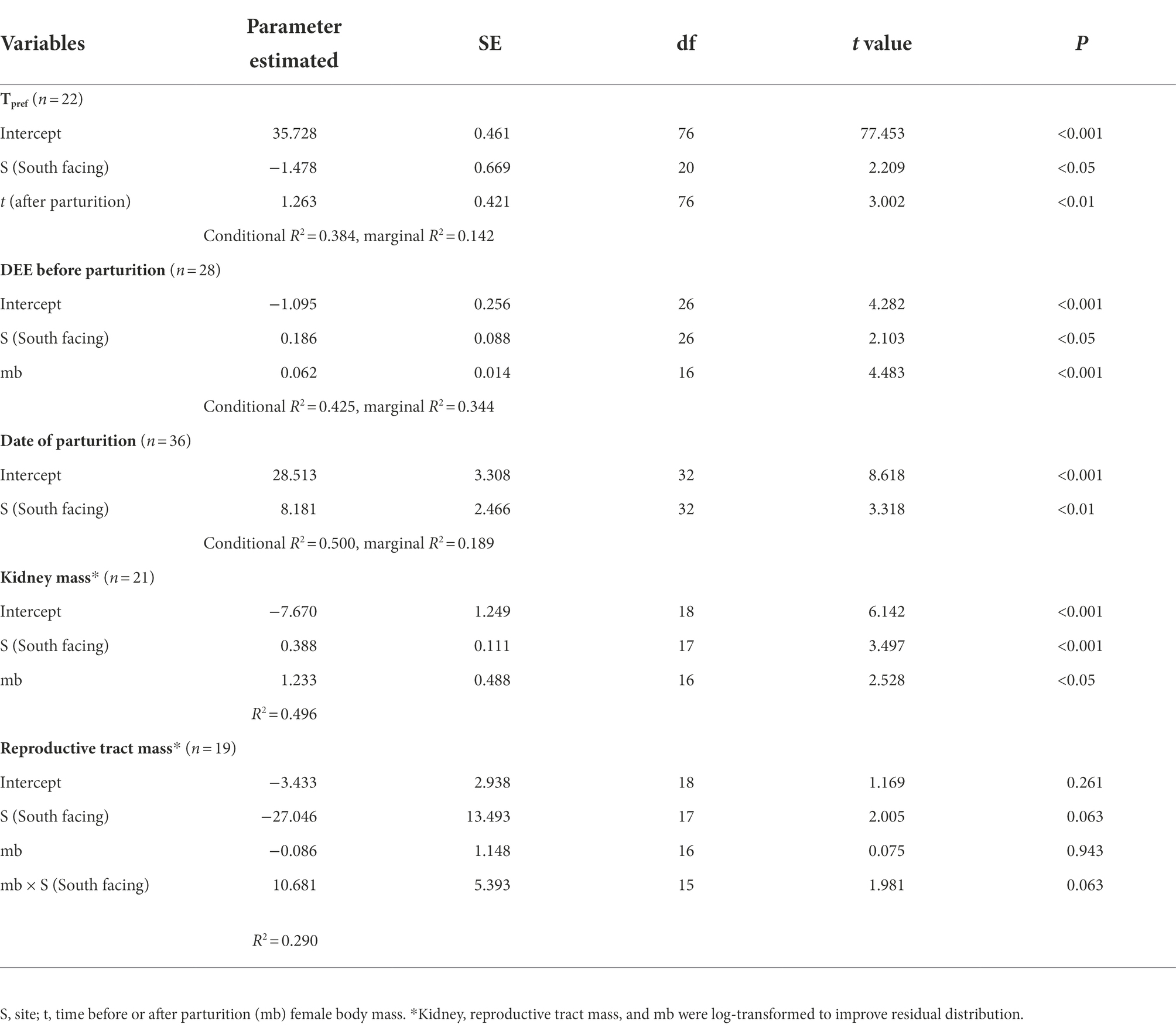
Table 1. Summary of the best-fitted models for physiological and life-history traits in an Andean viviparous lizard.
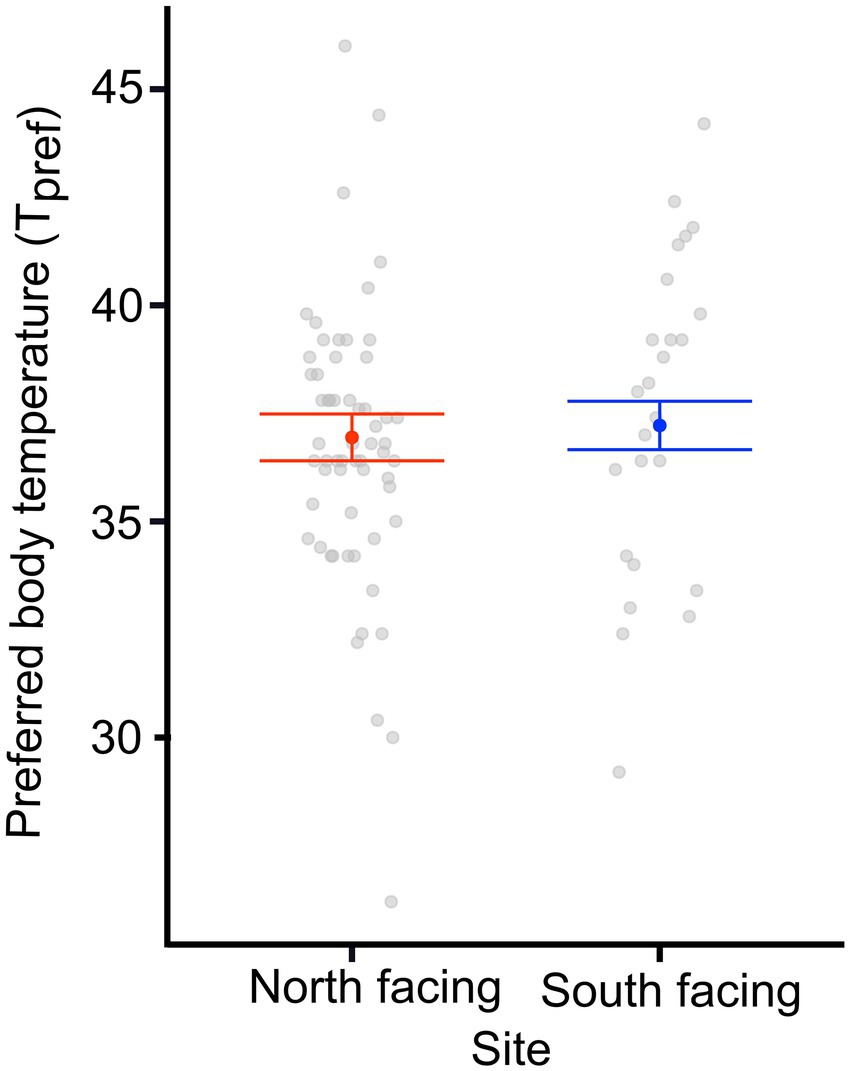
Figure 2. Preferred body temperature (Tpref) of offspring from two L. bellii populations at North-facing and South-facing slopes. Filled dots represent the mean, whiskers indicate SE estimated from the best model, and open dots are raw data. Notice that the best model only included body size; thus we show Tpref for an average-sized individual from either locality.
Metabolic rates
The DEE of the pregnant females was significantly different between sites (Table 1; Figure 3), with those from the colder locality having larger DEE values. Nevertheless, DEE differences were not observed in females after parturition (Supplementary Tables 1, 2). In fact, the best model for DEE before parturition included site (S) and body mass (mb) (Table 1), while the model for DEE after parturition only included mb (Supplementary Table 3). It is clear that pregnant females from the S-slope showed higher DEE than those from the N-slope (Figure 3; Table 1) after controlling by mb. We also found that the best model for MRday and MRnight of females before parturition only included mb (Supplementary Table 1). The same result was found for MRday after parturition (Supplementary Tables 2, 4–6). Nevertheless, for MRnight after parturition, we found that the best model also included population alongside mb as predictor variables but this model explained only 7% of the variance (Supplementary Tables 2, 7).
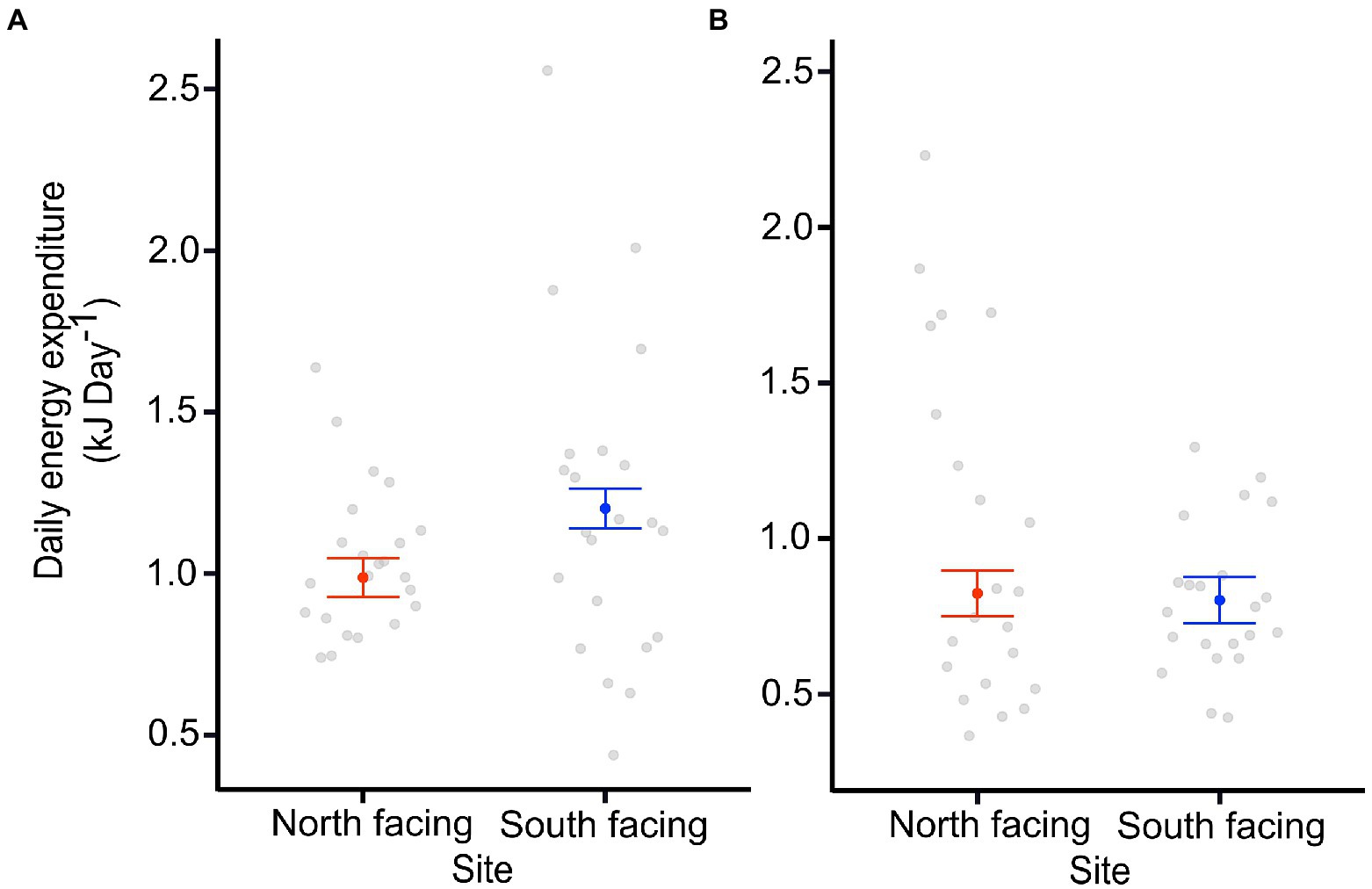
Figure 3. Daily energy expenditure (DEE) of adult females from two sites at North-facing and South-facing slopes before (A) and after parturition (B). Filled dots represent the mean for an average-sized female, whiskers indicate SE estimated from the best model. Open dots are raw data.
Life-history traits
We found that the date of parturition was significantly different between females from either site (Table 1; Figure 4), with females from the south-facing slope giving birth later than those from the north-facing slope. The best model for date of birth only included site as a predictor (Table 1; Supplementary Table 8), mb was not included. Females from the N-slope gave birth at 359 ± 5 days; that is, approximately 25 December of each year, while those from S-slope gave birth at 366 ± 11 days, that is, approximately 01 January (Figure 4). The latter showed more variation in the birth dates, with some females giving birth as late as February (390 days). In comparison, the latest births of N-slope females were approximately January 05 (370 days). Additionally, females did not differ between sites in body size (t value = 0.583, df = 31, p = 0.56, Figure 5), litter size (t value = 0.964, df = 34, p = 0.34, Figure 5), or offspring size (t value = 0.869, df = 13, p = 0.401, Figure 5). Litter size was determined by female body size (t value = 4.512, df = 34, p < 0.001).
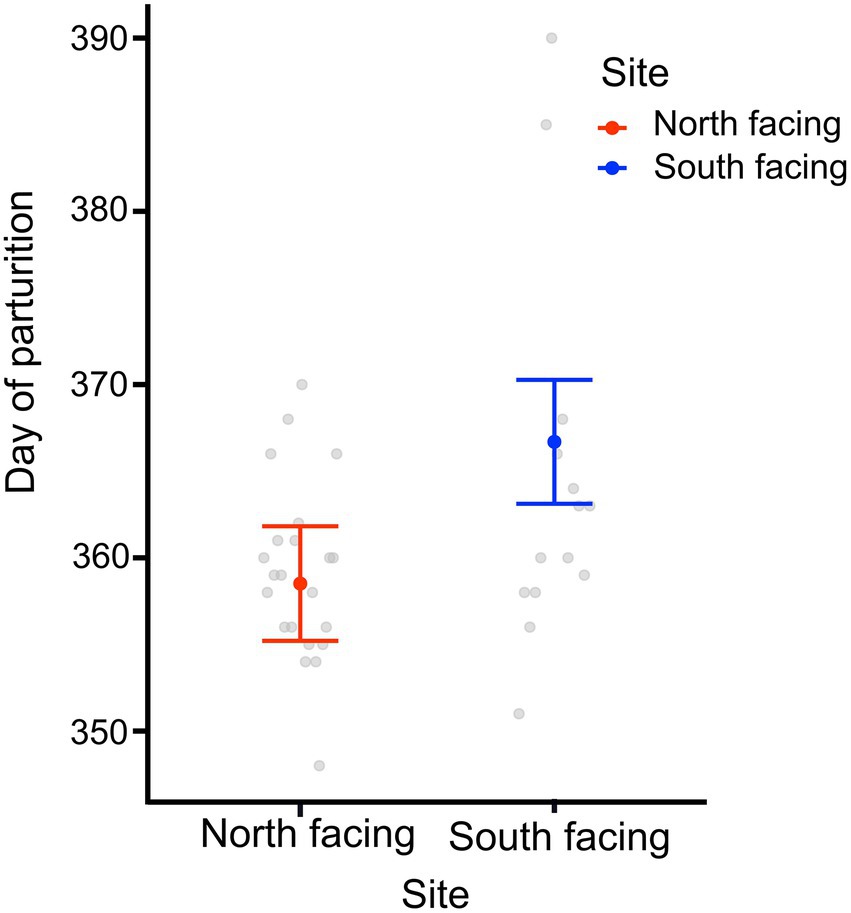
Figure 4. Day of parturition of adult females and from two L. bellii sites at North-facing and South-facing slopes. Filled dots represent the mean, whiskers indicate SE estimated from the best model, and open dots are raw data. Days are rooted on January 01 of each year and are represented by days often greater than 365 because austral summers start in December and end in March of the following year.
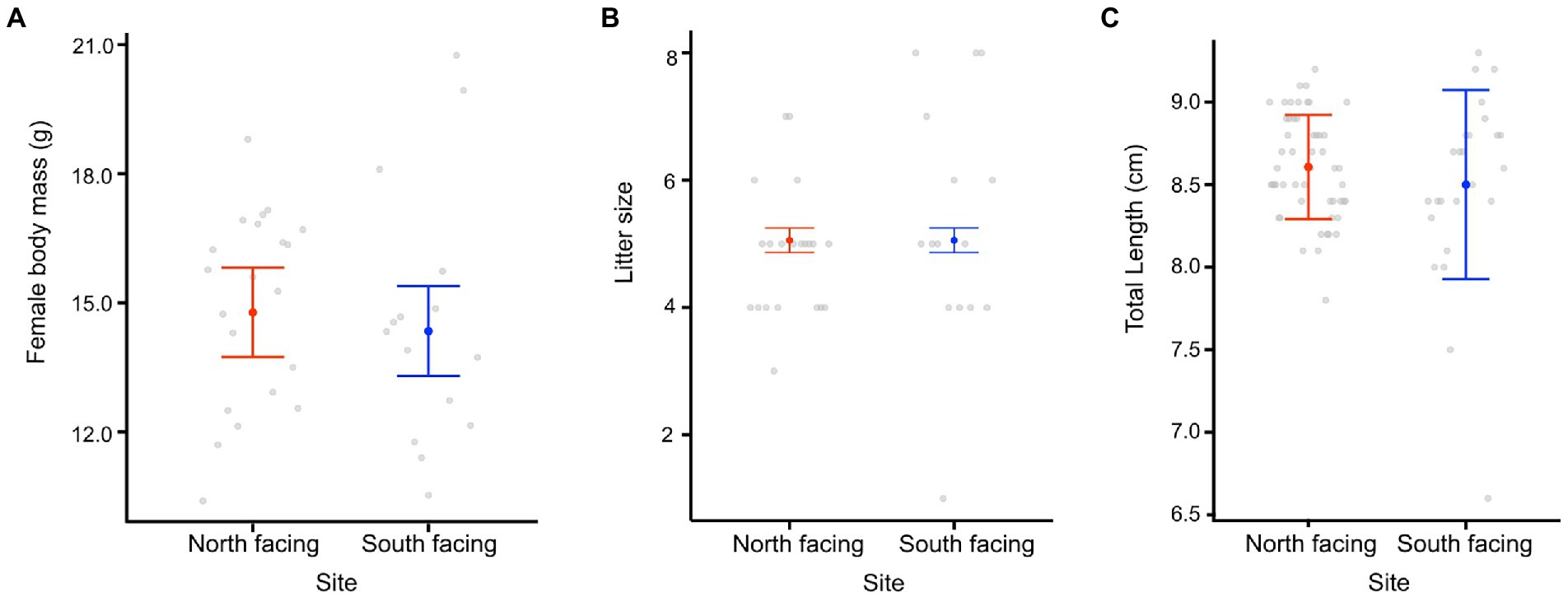
Figure 5. Female body mass (A), litter size (B), and offspring size (C) from two L. bellii sites at North-facing and South-facing slopes. Filled dots represent the mean, whiskers indicate SE estimated from the best model, and open dots are raw data.
Internal organs
For all internal organs, only kidney dry mass was different between females from the two sites (Table 1; for model selection see Supplementary Table 9). The best model for kidney mass included mb and site (Table 1), showing that females from the S-slope have bigger kidneys than those from the north-facing site (Figure 6). Further, the model for reproductive tract mass included site, mb, and their interaction term (Supplementary Table 8), but significant differences were not observed between sites (Table 1).
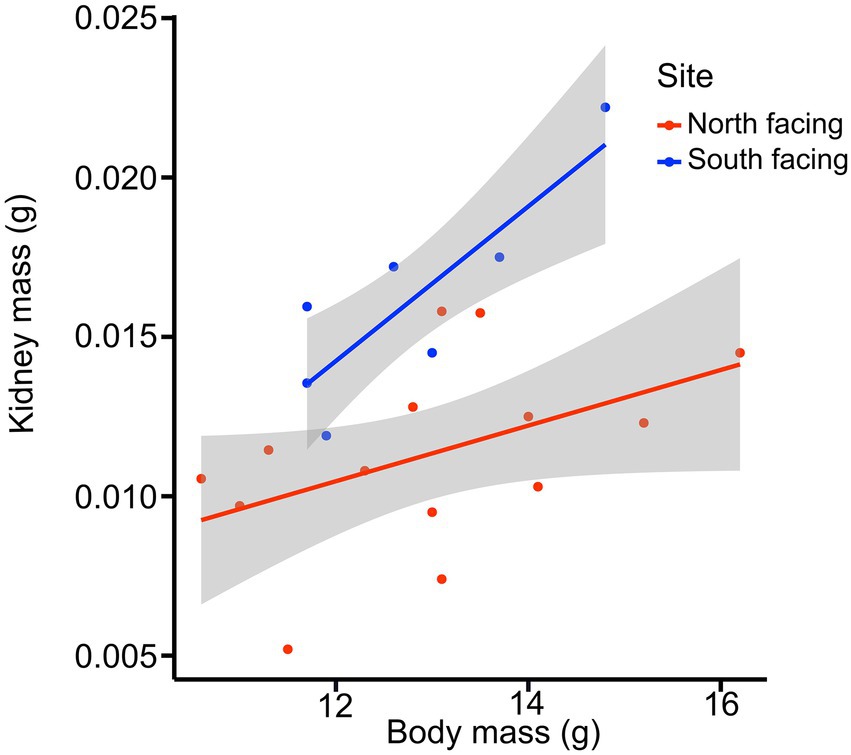
Figure 6. Kidney mass of females from two L. bellii populations at North-facing and South-facing slopes in relation to body mass (mb). Shaded areas show 95% confidence intervals of the regressions.
Discussion
We discovered that pregnant females from two contrastingly facing slopes in the Andean climatic microhabitat showed differences in several physiological and life-history traits, such as the day of parturition (Figures 1, 3, 4). Nevertheless, the litter size, offspring size, and female body mass did not significantly differ between sites (Figure 5). We found that females from the S-slope had lower Tpref values compared to females from the warmer N-slope (Figure 1). This difference was also observed after parturition, with females from the colder site always having lower body temperatures. It could be argued that such differences in physiological traits were not a short-term acclimatory response because females were kept in the laboratory under common-garden conditions. In addition, we did not find significant differences in the Tpref of the offspring (Figure 2), thus indicating that adult Tpref, is a developmental acclimatory or adaptive response. This response is more likely a reaction to differences in environmental temperature (Supplementary Figures 3, 4), supporting the local optima hypothesis (Levinton, 1983), which posits that individuals respond to colder environmental temperatures by matching Tpref with the environmental temperature. Thus, individuals living at lower environmental temperatures may still reach their optimum functioning temperature (Levinton, 1983). Our results may also provide evidence for the labile hypothesis (Hertz et al., 1983), which posits that thermal traits respond to directional selection due to environmental heterogeneity, contrary to the evolutionary constraint point of view raised by the static hypothesis (Hertz et al., 1983).
Similarly, the metabolic adaptation hypothesis posits that individuals dwelling at lower environmental temperatures, as is the case with high altitudes (Plasman et al., 2020) or latitudes (Ibargüengoytía et al., 2010), show higher metabolic rates in order for them to be active at those lower environmental temperatures, thus suggesting an optimal time-energy use (Gaston and Chown, 1999; Addo-Bediako et al., 2002). We found DEE differences between females from the two sites during pregnancy, but they were not maintained after parturition (Figure 3). Therefore, it is likely that the increased metabolism could be a time-use compensation for shorter activity periods as a consequence of snow cover permanence during autumn and spring in the S-slope (Supplementary Figure 1). However, we cannot discard the idea that the DEE differences between pregnant females at the two sites were a consequence of their different activity levels.
We also found that females from the more humid S-slope had larger kidneys than those from the drier N-slope (Figure 6). We speculate that at sites with more moisture, larger kidneys are necessary to process excess water, which is concordant with the observation that vertebrates facing chronic antidiuresis tend to have smaller kidneys than those that live in wetter environments (McNab, 2002; Sabat et al., 2006). We did not find between-site differences in the digestive tract. The digestive tract, kidney, and liver are internal organs that strongly influence metabolic variables such as BMR and SMR (Konarzewski and Diamond, 1995; Chappell et al., 1999; Russell and Chappell, 2007). Nevertheless, it is important to note that females were sacrificed and their internal organs dissected after parturition; therefore, any potential differences during pregnancy could not be detected. Moreover, it has been demonstrated that L. bellii decreases gut size in autumn and winter during their inactivity period, to save energy and avoid maintaining expensive and unnecessary tissue (Naya et al., 2008). We do not know what happens to gut size after parturition, but it is likely that it decreases. Additionally, while obtaining a more balanced dataset with a larger number of observations is desirable, it is logistically impossible to record all variables before females gave birth.
Regarding life-history traits, the date of birth was slightly different between females from the two sites, with those from the south-facing slope giving birth later (Figure 4). The difference was marginal and mainly determined by three females captured in January that gave birth in February. As mentioned, offspring and litter size were similar between females from either site (Figure 5). Because the fertilization date of the females is unknown, we cannot determine if there were differences in neonate retention time. The lizard Sceloporus scalaris, a high-elevation species from North America, exhibits population variation in life-history traits between populations at different altitudes (Mathies and Andrews, 1995). Females from high-elevation start reproduction earlier in spring to compensate for low temperatures and they also increase egg retention to facilitate embryonic development. This is concordant with the earlier onset of activity shown by high-elevation populations of the lizard Psammodromus algirus in Spain (Zamora-Camacho et al., 2013). Thus, we can assume that a similar phenomenon could be occurring among Liolaemus bellii, responding to different temperatures between temperature-contrasting slopes at the same elevation.
Finally, genetic and phenotypic divergence due to sharp microsite differences between contrasting facing slopes over short distances has been widely demonstrated among microorganisms and invertebrates (Nevo, 2009, 2012). Here, we found physiological differences in female lizards responding to microsite differences. They were observed in several physiological and life-history traits, possibly owing to developmental plasticity in Tpref, but some of the traits could also be due to local thermal adaptations, or a combination of the two. This will have to be addressed in future studies, taking into account multi-factorial abiotic effects. Regardless of the differentiation process, the phenotypic diversification observed in high-mountain species could lead to an evolutionary dead end in the context of climate change.
Data availability statement
The raw data supporting the conclusions of this article will be made available by the authors, without undue reservation.
Ethics statement
All the experiments using these lizards were conducted in agreement with institutional ethical standards and the national animal protection law. All experimental protocols, including collection and transport of pregnant females, were reviewed and approved by the Scientific Ethical Committee for Animal and Environment Care and the Scientific Ethical Committee for Research Safety of the Universidad de Chile, and the collection of individuals was approved for the Servicio Agrícola y Ganadero (SAG) under File number #3345. These protocols are in accordance with the basic principles set forth in Chilean Law N°20380 on Animal Protection (2009), the European Union Directive 2010/63/EU, and the Guide for the Care and Use of Experimental Animals (NRC, 8th Edition, 2010), to all of which this institution ascribes. We declare that no animal died during field collection, transportation, housing, or during the experimental period.
Author contributions
SC-B, PS, and FB conceived and designed the experiments. MO and SC-B performed the experiments. SC-B analyzed the data and wrote the original draft. PS and FB reviewed and edited the manuscript. All authors contributed to the article and approved the submitted version.
Funding
This research was funded by FONDECYT 3130514 and 1190007, ANID PIA/Basal FB0002, and PEDECIBA.
Acknowledgments
We thank N. Medina, S. Martel, and A. Rodríguez for helping during the experimentation; C. Garin for his help during female collection and identification; and F. Jaksic for syntax.
Conflict of interest
The authors declare that the research was conducted in the absence of any commercial or financial relationships that could be construed as a potential conflict of interest.
Publisher’s note
All claims expressed in this article are solely those of the authors and do not necessarily represent those of their affiliated organizations, or those of the publisher, the editors and the reviewers. Any product that may be evaluated in this article, or claim that may be made by its manufacturer, is not guaranteed or endorsed by the publisher.
Supplementary material
The Supplementary material for this article can be found online at: https://www.frontiersin.org/articles/10.3389/fevo.2022.974968/full#supplementary-material
References
Addo-Bediako, A., Chown, S., and Gaston, K. (2002). Metabolic cold adaptation in insects: a large-scale perspective. Funct. Ecol. 16, 332–338. doi: 10.1046/j.1365-2435.2002.00634.x
Akaike, H. (1974). A new look at the statistical model identification. IEEE Trans. Autom. Control 19, 716–723. doi: 10.1109/TAC.1974.1100705
Akaike, H. (1998). Autoregressive Model Fitting for Control: Selected Papers of Hirotugu Akaike. Springer, 153–170, doi: 10.1007/978-1-4612-1694-0_12
Angilletta, M. J. (2009). Thermal Adaptation: A Theoretical and Empirical Synthesis. Oxford University Press, New York, doi: 10.1093/acprof:oso/9780198570875.001.1
Azócar, D. L. M., Vanhooydonck, B., Bonino, M. F., Perotti, M. G., Abdala, C. S., Schulte, J. A., et al. (2013). Chasing the Patagonian sun: comparative thermal biology of Liolaemus lizards. Oecologia 171, 773–788. doi: 10.1007/s00442-012-2447-0
Badano, E. I., Cavieres, L. A., Molina-Montenegro, M. A., and Quiroz, C. (2005). Slope aspect influences plant association patterns in the Mediterranean matorral of Central Chile. J. Arid Environ. 62, 93–108. doi: 10.1016/j.jaridenv.2004.10.012
Barton, K. (2009). MuMIn: Multi-Model Inference: R Package Version http://r-forge. r-project. org/projects/mumin/. 1–79.
Blomberg, S. P., Garland, T. Jr., and Ives, A. R. (2003). Testing for phylogenetic signal in comparative data: behavioral traits are more labile. Evolution 57, 717–745. doi: 10.1111/j.0014-3820.2003.tb00285.x
Bozinovic, F., Bastías, D. A., Boher, F., Clavijo-Baquet, S., Estay, S. A., and Angilletta, M. J. Jr. (2011). The mean and variance of environmental temperature interact to determine physiological tolerance and fitness. Physiol. Biochem. Zool. 84, 543–552. doi: 10.1086/662551
Chappell, M. A., Bech, C., and Buttemer, W. A. (1999). The relationship of central and peripheral organ masses to aerobic performance variation in house sparrows. J. Exp. Biol. 202, 2269–2279. doi: 10.1242/jeb.202.17.2269
Chevin, L.-M., Lande, R., and Mace, G. M. (2010). Adaptation, plasticity, and extinction in a changing environment: towards a predictive theory. PLoS Biol. 8:e1000357. doi: 10.1371/journal.pbio.1000357
Donoso-Barros, R. (1966). Reptiles of Chile. Ediciones de la Universidad de Chile: Santiago de Chile.
Dupoué, A., Blaimont, P., Rozen-Rechels, D., Richard, M., Meylan, S., Clobert, J., et al. (2020). Water availability and temperature induce changes in oxidative status during pregnancy in a viviparous lizard. Funct. Ecol. 34, 475–485. doi: 10.1111/1365-2435.13481
Enriquez-Urzelai, U., Bernardo, N., Moreno-Rueda, G., Montori, A., and Llorente, G. (2019). Are amphibians tracking their climatic niches in response to climate warming? A test with Iberian amphibians. Clim. Chang. 154, 289–301. doi: 10.1007/s10584-019-02422-9
Gaston, K. J., and Chown, S. L. (1999). Elevation and climatic tolerance: a test using dung beetles. Oikos 86, 584–590.
Hertz, P. E., Huey, R. B., and Nevo, E. (1983). Homage to Santa Anita: thermal sensitivity of sprint speed in agamid lizards. Evolution 35, 1075–1084.
Hill, J. K., Thomas, C. D., Fox, R., Telfer, M. G., Willis, S. G., Asher, J., et al. (2002). Responses of butterflies to twentieth century climate warming: implications for future ranges. P Roy Soc. B. Biol. Sci. 269, 2163–2171. doi: 10.1098/rspb.2002.2134
Hoffmann, A. A., and Sgrò, C. M. (2011). Climate change and evolutionary adaptation’. Nature 470, 479–485.
Hothorn, T., Bretz, F., Westfall, P., Heiberger, R. M., Schuetzenmeister, A., Scheibe, S., et al. (2016). Package “Multcomp” Simultaneous Inference in General Parametric Models. Project for Statistical Computing: Vienna, Austria.
Huey, R. B., Hertz, P. E., and Sinervo, B. (2003). Behavioral drive versus behavioral inertia in evolution: a null model approach. Am. Nat. 161, 357–366. doi: 10.1086/346135
Ibargüengoytía, N. R., Medina, S. M., Fernández, J. B., Gutiérrez, J. A., Tappari, F., and Scolaro, A. (2010). Thermal biology of the southernmost lizards in the world: Liolaemus sarmientoi and Liolaemus magellanicus from Patagonia, Argentina. J. Therm. Biol. 35, 21–27. doi: 10.1016/j.jtherbio.2009.10.003
IPCC Climate Change (2013). The Physical Science Basis: Working Group I Contribution to the Fifth Assessment Report of the Interational Panel on Climate Change. Cambridge University Press: Cambridge.
Konarzewski, M., and Diamond, J. (1995). Evolution of basal metabolic rate and organ masses in laboratory mice. Evolution 49, 1239–1248. doi: 10.1111/j.1558-5646.1995.tb04450.x
Labra, A., Beltrán, S., and Niemeyer, H. M. (2001). Chemical exploratory behavior in the lizard Liolaemus bellii. J. Herpetol. 35, 51–55. doi: 10.2307/1566022
Lavergne, S., Mouquet, N., Thuiller, W., and Ronce, O. (2010). Biodiversity and climate change: integrating evolutionary and ecological responses of species and communities. Annu. Rev. Ecol. Syst. 41, 321–350. doi: 10.1146/annurev-ecolsys-102209-144628
Levinton, J. S. (1983). The latitudinal compensation hypothesis: growth data and a model of latitudinal growth differentiation based upon energy budgets: I interspecific comparison of Ophryotrocha (Polychaeta: Dorvilleidae). Biol. Bull. 165, 686–698. doi: 10.2307/1541471
Lighton, J. R. (2018). Measuring Metabolic Rates: A Manual for Scientists. Oxford University Press: New York.
Mathies, T., and Andrews, R. M. (1995). Thermal and reproductive biology of high and low elevation populations of the lizard Sceloporus scalaris: implications for the evolution of viviparity. Oecologia 104, 101–111. doi: 10.1007/BF00365568
Mayr, E. (1959). “The emergence of evolutionary novelties,” in The Evolution of Life. ed. S. Tax (Chicago, Chicago: University of Chicago Press), 640.
McNab, B. K. (2002). The Physiological Ecology of Vertebrates: A View From Energetics. London: Cornell University Press.
Montes de Oca, L., Pérez-Miles, F., and Clavijo-Baquet, S. (2020). The reproductive period of tarantulas is constrained by their thermal preferences (Araneae, Theraphosidae). J. Therm. Biol. 92:102665. doi: 10.1016/j.jtherbio.2020.102665
Moreno-Rueda, G., Pleguezuelos, J. M., Pizarro, M., and Montori, A. (2012). Northward shifts of the distributions of Spanish reptiles in association with climate change. Conserv. Biol. 26, 278–283. doi: 10.1111/j.1523-1739.2011.01793.x
Naya, D. E., Veloso, C., and Bozinovic, F. (2008). Physiological flexibility in the Andean lizard Liolaemus bellii: seasonal changes in energy acquisition, storage and expenditure. J. Comp. Physiol. B 178, 1007–1015. doi: 10.1007/s00360-008-0292-6
Nevo, E. (1995). Asian, African and European biota meet at ‘evolution Canyon’Israel: local tests of global biodiversity and genetic diversity patterns. Proc. Royal Soc. B. Biol. Sci. 262, 149–155. doi: 10.1098/rspb.1995.0189
Nevo, E. (2009). Evolution in action across life at “evolution canyons” Israel. Trends Ecol. Evol. 1:e3. doi: 10.4081/eb.2009.e3
Nevo, E. (2012). “Evolution canyon,” a potential microscale monitor of global warming across life. Proc. Natl. Acad. Sci. U. S. A. 109, 2960–2965. doi: 10.1073/pnas.1120633109
Núñez, H., Veloso, A., Espejo, P., Veloso, C., Cortés, A., and Araya, S. (2010). Nuevas especies de Phymaturus (grupo palluma) para la zona Cordillerana Central de Chile (Reptilia, Sauria, Liolaemidae). Mus. Nac. Hist. Nat. Chil. 59, 41–74.
Paaijmans, K. P., Heinig, R. L., Seliga, R. A., Blanford, J. I., Blanford, S., Murdock, C. C., et al. (2013). Temperature variation makes ectotherms more sensitive to climate change. Glob. Chang. Biol. 19, 2373–2380. doi: 10.1111/gcb.12240
Parmesan, C., Ryrholm, N., Stefanescu, C., Hill, J. K., Thomas, C. D., Descimon, H., et al. (1999). Poleward shifts in geographical ranges of butterfly species associated with regional warming. Nature 399, 579–583. doi: 10.1038/21181
Pincheira-Donoso, D., Tregenza, T., Witt, M. J., and Hodgson, D. J. (2013). The evolution of viviparity opens opportunities for lizard radiation but drives it into a climatic cul-de-sac. Glob. Ecol. Biogeogr. 22, 857–867. doi: 10.1111/geb.12052
Pinheiro, J., Bates, D., DebRoy, S., Sarkar, D., Heisterkamp, S., Van Willigen, B., et al. (2017). Package “nlme” linear and nonlinear mixed effects models, version. 3, 1–89.
Pla‑sman, M., Bautista, A., McCue, M. D., and Díaz de la Vega-Pérez, A. H. (2020). Resting metabolic rates increase with elevation in a mountain-dwelling lizard. Integr. Zool. 15, 363–374. doi: 10.1111/1749-4877.12434
Pyron, R. A., and Burbrink, F. T. (2014). Early origin of viviparity and multiple reversions to oviparity in squamate reptiles. Ecol. Lett. 17, 13–21. doi: 10.1111/ele.12168
R Core Team (2018). R: A Language and Environment for Statistical Computing. R Foundation for Statistical Computing: Vienna, Austria. https://www.R-project.org/.
Refsnider, J. M., Qian, S. S., Streby, H. M., Carter, S. E., Clifton, I. T., Siefker, A. D., et al. (2018). Reciprocally transplanted lizards along an elevational gradient match light environment use of local lizards via phenotypic plasticity. Funct. Ecol. 32, 1227–1236. doi: 10.1111/1365-2435.13071
Rozen-Rechels, D., Rutschmann, A., DupouÉ, A. É., Blaimont, P., Chauveau, V., Miles, D. B., et al. (2021). Interaction of hydric and thermal conditions drive geographic variation in thermoregulation in a widespread lizard. Ecol. Monogr. 91:e01440. doi: 10.1002/ecm.1440
Rozzi, R., Molina, J. D., and Miranda, P. (1989). Microclima y períodos de floración en laderas de exposición ecuatorial y polar en los Andes. Rev. Chil. Hist. Nat. 62, 75–84.
Russell, G., and Chappell, M. (2007). Is BMR repeatable in deer mice? Organ mass correlates and the effects of cold acclimation and natal altitude. J. Comp. Physiol. B 177, 75–87. doi: 10.1007/s00360-006-0110-y
Sabat, P., Maldonado, K., Farina, J. M., and Del Rio, C. M. (2006). Osmoregulatory capacity and the ability to use marine food sources in two coastal songbirds (Cinclodes: Furnariidae) along a latitudinal gradient. Oecologia 148, 250–257. doi: 10.1007/s00442-006-0377-4
Shine, R. (1983). Reptilian viviparity in cold climates: testing the assumptions of an evolutionary hypothesis. Oecologia 57, 397–405. doi: 10.1007/BF00377186
Shine, R., and Brown, G. P. (2008). Adapting to the unpredictable: reproductive biology of vertebrates in the Australian wet–dry tropics. Philos. Trans. R. Soc. Lond. B. Biol. 363, 363–373. doi: 10.1098rstb.2007.2144.
Sikorski, J., and Nevo, E. (2005). Adaptation and incipient sympatric speciation of Bacillus simplex under microclimatic contrast at “evolution canyons” I and II, Israel. Proc. Natl. Acad. Sci. U. S. A. 102, 15924–15929. doi: 10.1073/pnas.0507944102
Thomas, C. D. (2010). Climate, climate change and range boundaries. Divers. Distrib. 16, 488–495. doi: 10.1111/j.1472-4642.2010.00642.x
Tinkle, D. W., and Gibbons, J. W. (1977). The distribution and evolution of viviparity in reptiles. Misc. Publ. Mus. Zool. Univ. Mich. 154, 1–47.
Wickham, H., Chang, W., and Wickham, M. H. (2016). Package ‘ggplot2’. Create elegant data visualizations using the grammar of graphics. Version 2, 1–189.
Zamora-Camacho, F., Reguera, S., Moreno-Rueda, G., and Pleguezuelos, J. (2013). Patterns of seasonal activity in a Mediterranean lizard along a 2200 m altitudinal gradient. J. Therm. Biol. 38, 64–69. doi: 10.1016/j.jtherbio.2012.11.002
Zu, K., Wang, Z., Zhu, X., Lenoir, J., Shrestha, N., Lyu, T., et al. (2021). Upward shift and elevational range contractions of subtropical mountain plants in response to climate change. Sci. Total Environ. 783:146896. doi: 10.1016/j.scitotenv.2021.146896
Keywords: Andes range, body temperature, Chile, Liolaemus bellii, metabolism
Citation: Clavijo-Baquet S, Orellana MJ, Sabat P and Bozinovic F (2022) How do ectotherms perform in cold environments? Physiological and life-history traits in an Andean viviparous lizard. Front. Ecol. Evol. 10:974968. doi: 10.3389/fevo.2022.974968
Edited by:
Edward Ivimey-Cook, University of Glasgow, United KingdomReviewed by:
Ramakrishnan Vasudeva, University of East Anglia, United KingdomGregorio Moreno-Rueda, University of Granada, Spain
Copyright © 2022 Clavijo-Baquet, Orellana, Sabat and Bozinovic. This is an open-access article distributed under the terms of the Creative Commons Attribution License (CC BY). The use, distribution or reproduction in other forums is permitted, provided the original author(s) and the copyright owner(s) are credited and that the original publication in this journal is cited, in accordance with accepted academic practice. No use, distribution or reproduction is permitted which does not comply with these terms.
*Correspondence: Sabrina Clavijo-Baquet, c2NsYXZpam9AYmlvLnB1Yy5jbA==; c2FicmluYWNsYXZpam9AZmNpZW4uZWR1LnV5