- 1Biochemistry and Toxicology Division, Department of Zoology, University of Calicut, Malappuram, Kerala, India
- 2Western Ghat Regional Centre, Zoological Survey of India, Kozhikode, Kerala, India
The main objective of this study was to investigate the taxonomic significance of wing phenotypic variations (size and shape) for classifying potter wasps. This is the first study investigating the wing size and shape variations, as well as wing asymmetry, sexual dimorphism, wing integration, and phylogenetic signal analysis of all known Antodynerus species from the Indian subcontinent: A. flavescens, A. limbatus, and A. punctatipennis. We used forewings and hindwings for geometric morphometric analysis, and we proved that each species’ wing had unique size and shape variations, as well as significant right–left wing asymmetry and sexual dimorphism across the Antodynerus species, as verified by discriminant function analysis. Wings of Vespidae are longitudinally folded; based on that, we tested two alternative wing modular hypotheses for evaluating the wing integration, using two subsets organization, such as anterior–posterior (AP) and proximal-distal (PD) wing modular organization. We proved that Antodynerus species wings are highly integrated units (RV > 0.5), and we rejected our hypothesis at p < 0.05. The morphospace distribution analysis revealed that each species has its unique morphospace boundary, although they share some level of homoplasy, which suggests to us that we can use wing morphometric traits for Antodynerus species delimitation. In addition, we revealed the phylogenetic signal of Antodynerus species. Surprisingly, we found a shape-related phylogenetic signal in the forewing, and there is no significant (p > 0.05) phylogenetic signal in forewing size, hindwing shape, and size. We observed that the Antodynerus species’ forewing shape is evolutionarily more highly constrained than the hindwing. We found that A. limbatus and A. flavescens with distinct geographical distribution share a similar evolutionary history, while A. punctatipennis evolved independently.
Introduction
In many biological research studies, the identification and classification of species is the first and essential step that enables researchers to provide recognized entities (Mayr, 1969; Gaston and O’Neill, 2004). However, taxonomic diversity and the complexity of interspecific variations usually make species identification challenging (Meier et al., 2006; Bickford et al., 2007). The venation patterns of the wings are frequently used to identify insects at the order or family level (Comstock and Needham, 1899). More recently, geometric morphometrics (GM) has been widely utilized to analyze subtle variation among insect genera, species, and even populations, sexes, and castes within the same species (Baylac and Daufresne, 1996; Baylac et al., 2003; Villemant et al., 2007; Bai et al., 2011; De Meulemeester et al., 2012; Perrard et al., 2012; Outomuro et al., 2013; Mahima et al., 2021). Variations in wing venation patterns could be considered as a generalist marker for species identification at different classification levels because genetic drift between taxa should significantly influence wing venation morphology more than other factors of variation (Perrard et al., 2014). The evolutionary history of a taxon predominantly controls variations in wing venations. These variations could be used for generic- and species-level identification. These shape variables (venation disparities) are suitable for landmark-based analysis (Perrard et al., 2014). GM has several advantages compared to traditional taxonomic approaches. First of all, GM is purely based on the statistical theory of shape (Kendall et al., 1999), which allows quantitative multivariate analysis of shapes (Rohlf and Marcus, 1993; Adams et al., 2004). GM, applied to the vein junctions of the wings, has previously been used to distinguish honeybee subspecies (Tofilski, 2008), Sphex species (Tuzun, 2009), some species of Syrphidae (Francuski et al., 2009) and in genus and species level, classification of Stenogastrinae wasps (Baracchi et al., 2011).
Geometric morphometrics analysis is very limited in Vespidae, especially in solitary wasps, compared to other hymenopteran groups (Aytekin et al., 2007; Wappler et al., 2012; Schwarzfeld and Sperling, 2014; Shih et al., 2020; Jouault and Nel, 2021). The Vespidae family is divided into six subfamilies, of which three are comprised of solitary wasps, such as Euparagiinae, Masarinae, and Eumeninae (Perrard et al., 2017). The subfamily Eumeninae, the potter wasps are the biologically most diverse subfamily of Vespidae, with more than 3,500 known species and 210 genera (Pickett and Carpenter, 2010; Hermes et al., 2013). This group is interesting, not only for its predatory habits but also for various nest construction procedures that indicate a wide range of behavioral complexity (Grandinete et al., 2015). Even though they congregate around a suitable nesting site, most Eumeninae wasps are solitary, while a few genera are known to be communal nesters (Yamane, 1990; Hermes et al., 2013). In India, the Eumeninae are less well-known than the other social subfamilies (Vespinae, Polistinae, and Stenogastrinae). Resolving the taxonomic position of these wasps represents a significant challenge in India because no extensive research has ever been conducted on them, and there have been no revisions in this subfamily (Pannure et al., 2016). Due to the group’s richness and morphological complexity, the generic- and species-level classification of Eumeninae is exceptionally challenging (Hermes et al., 2013).
This study focused on the genus Antodynerus de Saussure, 1855 (Vespidae: Eumeninae) from the Indian subcontinent. Genus Antodynerus is widely distributed in the Ethiopian, Oriental, and Palaearctic regions. The genus has 54 species and numerous subspecies, most of which are found in the Ethiopian region (Carpenter et al., 2009; Gusenleitner, 2010). There are seven species in the Palaearctic region and three in the Oriental region. All three Oriental species have been identified from the Indian subcontinent: A. flavescens (Fabricius, 1775), A. limbatus (de Saussure, 1852), and A. punctatipennis (de Saussure, 1853) (Girish Kumar and Carpenter, 2013). The dichotomous identification key of Antodynerus species is primarily based on the few morphological features present in the body (Girish Kumar and Carpenter, 2013) rather than on the wing morphology. In this study, we used the wing morphology of Antodynerus for species-level identification purposes. The wings help to distinguish morphological discrepancies because of their two-dimensional nature, while venation patterns represent morphologically well-defined landmarks (i.e., shape variables) (Pan et al., 2008). GM has been successfully used to study insect wing venation patterns, and it has also been useful in insect identification at the individual level (Baylac et al., 2003), in differentiation between sibling species (De La Rua et al., 2001; Klingenberg, 2008), and delimitation of genera and congeneric species boundaries. Based on this perspective, we hypothesized that GM’s use allowed us to explain morphological similarities and disparities among Antodynerus species. Our central hypothesis is that the wing could be an essential tool for species identification in solitary wasps. It is also helpful to analyze wing asymmetry, sexual dimorphism, wing covariation, and phylogenetic signal. In this study, we examined all known Antodynerus species from the Indian subcontinent. The aims of the present study were as follows: (1) to analyze the size, shape, right–left asymmetry, and sexual asymmetry in forewings and hindwings among the Antodynerus species; (2) to investigate the morphological wing covariation using two alternative hypotheses: anterior–posterior (AP) and proximal-distal (PD) wing modular hypothesis; and (3) to test the shape and size phylogenetic signal in the Antodynerus species collected from the Indian subcontinent.
Key to species and subspecies of the genus Antodynerus de Saussure from the Indian subcontinent (Girish Kumar and Carpenter, 2013):
1. The median area of the propodeum with a deep, broad fovea from which the median carina runs to the orifice, with short transverse striae beside the carina; postero-lateral margin of propodeum with a strong transversely carinate projection, below that with distinct short transverse striae. 10–12 mm ------------------------ A. limbatus (de Saussure, 1852) (Figure 1D).
– The median area of the propodeum with a shallow narrow fovea from which the median carina runs to the orifice, without distinct transverse striae; the postero-lateral margin of propodeum rounded, without a strong transversely carinate projection, below that with evanescent transverse striae ---------------- 2
2. Frons, ocellar area, and vertex with closely arranged strong punctures, the diameter of punctures greater than the distance between punctures. 7–8 mm --------------------- A. punctatipennis (de Saussure, 1853) (Figure 1G).
– Frons sparsely punctured, diameters of punctures less than the distance between punctures; ocellar area almost smooth without punctures; vertex with moderately strong punctures except at middle and area toward occipital carina smooth [A. flavescens (Fabricius, 1775)] -------------------- 3
3. Body completely yellow with yellow to ferruginous antennae; an ocellar area with a short curved brown band ------------------------ A. f. karachiensis Giordani Soika, 1970.
– Body predominantly reddish brown, usually with distinct yellow and black markings; ocellar area usually with a short black band. 9–11 ;mm --------------------- A. f. flavescens (Fabricius, 1775) (Figure 1A).
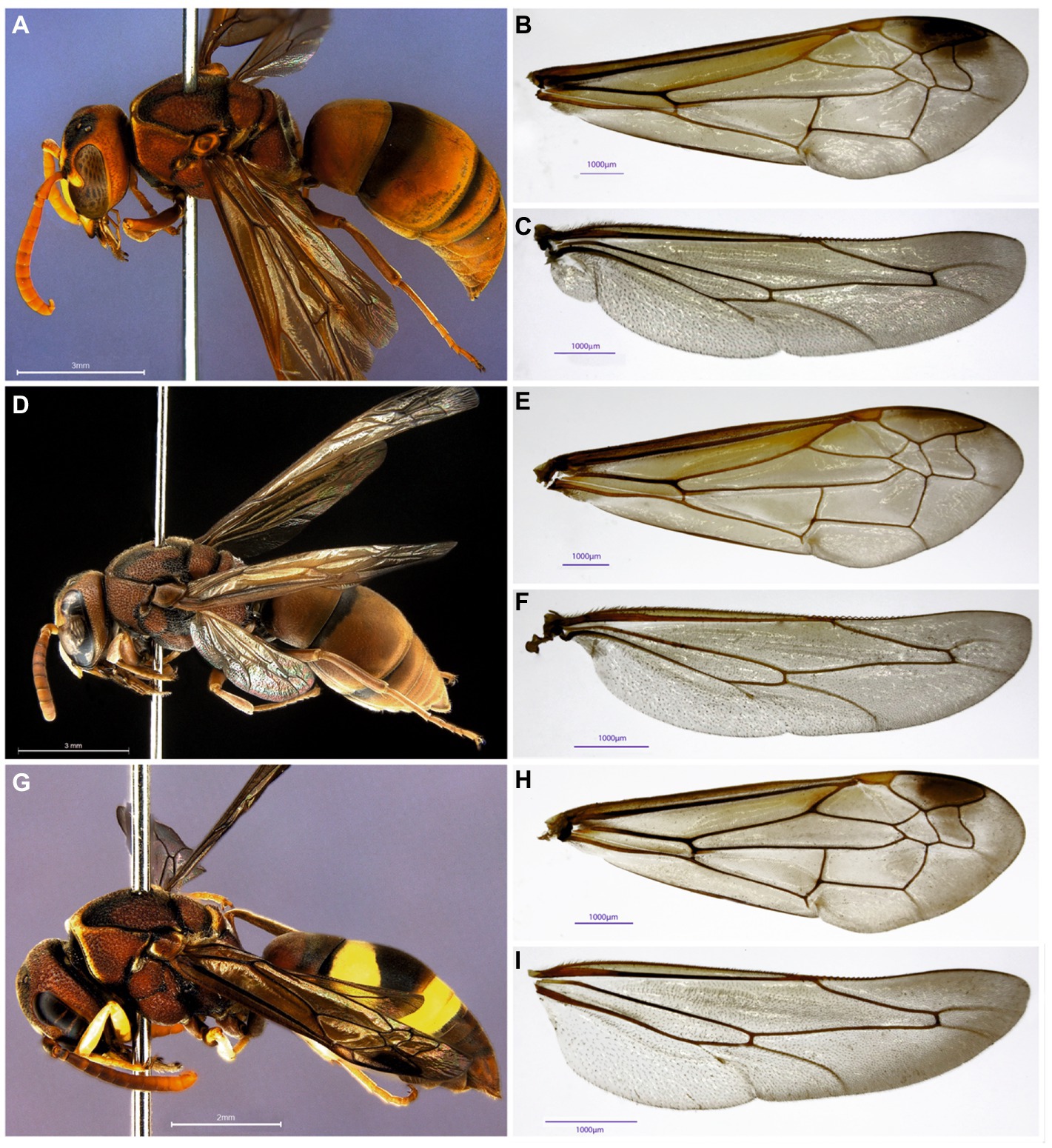
Figure 1. Antodynerus de Saussure, 1855 (A) A. flavescens habitus, forewing (B) and hindwing (C); (D) A. limbatus habitus, forewing (E) hindwing (F); (G) A. punctatipennis habitus, forewing (H) and hindwing (I).
Materials and methods
Dataset
We sampled all known species of the genus Antodynerus (de Saussure, 1855) from the Indian subcontinent for this investigation. All specimens used in the GM study were from our private and scientific collections, Western Ghat Regional Centre, Zoological Survey of India (ZSI), Kozhikode, Kerala, India. GM analysis datasets were as follows: 21 specimens in A. flavescens (Fabricius, 1775): 10 males and 11 females (Figures 1A–C), 22 specimens in A. limbatus (de Saussure, 1852): 10 males and 12 females (Figures 1D–F), and 21 specimens in A. punctatipennis (de Saussure, 1853): 10 males and 11 females (Figures 1G–I). To avoid pseudoreplication, we included in our investigation representatives from different years (from 2015 to 2018) and distinct localities. We were unable to increase sample numbers due to the problems in associated fieldwork, i.e., due to the research permits, low species abundance, species that occurred in remote and isolated places, and so on. Nonetheless, we maintained a homogeneous population of Antodynerus species datasets, i.e., an approximately equal number of males and females and the equal number of specimens from each species were used for GM analysis to avoid false-positive results. Specimen number selection is critical in GM analysis because homogeneous datasets will provide more concise results than heterogeneous datasets.
Geometric morphometrics analysis
Each species’ wings (forewings and hindwings) were dissected to make microscopic slides for Geometric morphometrics (GM) analysis. Wing images were photographed by Zeiss SteREO Discovery V20 with Zeiss 505 camera. Based on the wing venation pattern, 26 homologous landmarks were identified in the forewings (Figure 2A) and nine landmarks in the hindwings (Figure 2B). For landmarking, the images were initially converted into x and y coordinates using the tpsUtil v 1.68 software, and then landmarking was performed using the tpsDig2 v 2.26 software (Rohlf, 2015). To avoid an error landmarking procedure, the wings were digitalized twice. A Procrustes ANOVA was conducted to determine whether the means square (MS) values for the individuals were lower than the error (Fruciano, 2016; Klingenberg, 2016; Mahima et al., 2021). Prior to conducting various statistical analyses, the tpsSmall V 1.34 tool was used to determine the correlation between the Procrustes and tangent distances (Rohlf, 2003). The goal of this analysis is to determine whether the size of the variance in the dataset is small enough for additional statistical analysis (Rohlf, 2015; Mahima et al., 2021).
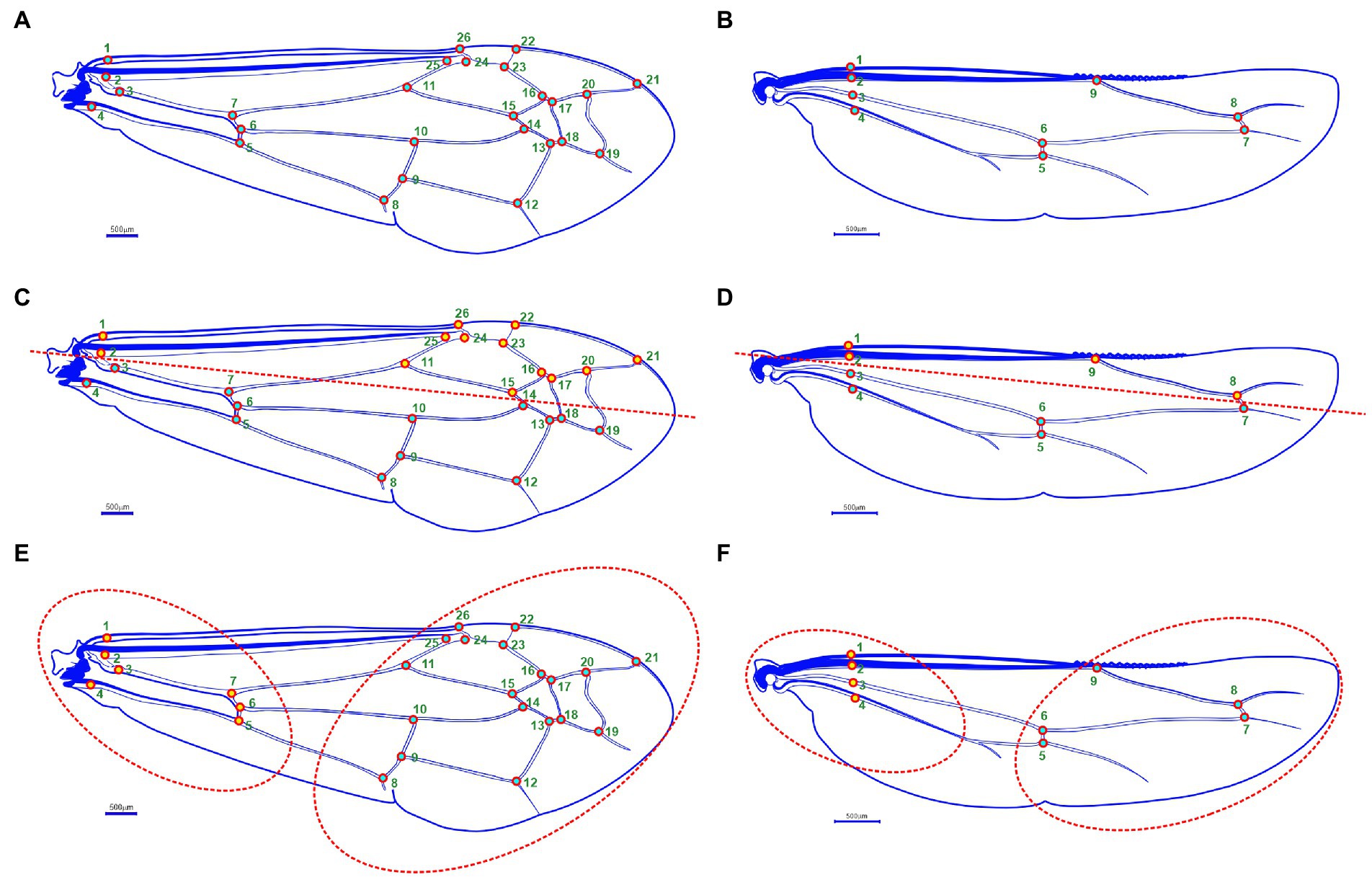
Figure 2. Wing morphology of Antodynerus species; (A) landmarks (N = 26) on the forewing, (B) landmarks (N = 9) on the hindwing. Covariation analysis: wing modular organization hypothesis – (C) forewing antero-posterior (AP) wing modular hypothesis, (D) hindwing antero-posterior (AP) wing modular hypothesis, (E) forewing proximal-distal (PD) wing modular hypothesis, and (F) hindwing proximal-distal (PD) wing modular hypothesis.
All morphometric analyses were performed using the MorphoJ tool (Klingenberg, 2011). To estimate wing size, we computed centroid size (CS), a geometric measure of size calculated as the square root of the sum of the squared distance from a set of landmarks (Bookstein, 1991). The statistical significance between male and female wings was verified using the non-parametric Mann–Whitney U-test. The statistical analysis was conducted in SPSS 20.0, and statistical significance was set at p < 0.05 for all comparisons.
Next, we applied generalized Procrustes analysis (Rohlf and Slice, 1990), which eliminates variation in scale, position, and orientation of wings, to obtain a matrix of shape coordinates, i.e., Procrustes coordinates. Following the generalized Procrustes analysis (GPA), Procrustes shape and size ANOVA (Rohlf and Slice, 1990) and principal component analysis (PCA; Jolliffe, 2002) were performed to investigate the shape, size, asymmetry, and sexual dimorphism of Antodynerus species. For the right–left wing asymmetry analysis, we combined the right and left wings of each species and performed one-way ANOVA independently. In the case of sexual asymmetry analysis, we combined the wing of male and female Antodynerus species wings and performed one-way ANOVA; for example, we combined the male right forewing and female right forewing of A. limbatus and performed the size and shape ANOVA. Canonical variate analysis (CVA) and discriminant function analysis (DFA; Gumiel et al., 2003; Villemant et al., 2007) are conducted to determine the trait-based morphospace distribution of Antodynerus species. A permutation test with 10,000 iterations was used to evaluate the statistical significance level. After CVA, Mahalanobis distance (Cooke and Terhune, 2014) across species data were used for hierarchical cluster dendrogram analysis. 2-block partial least squares (2B-PLS) analysis was used to determine the size and shape covariation among the Antodynerus species. Block 1 indicated the size and Block 2 indicated the shape relationship of the Antodynerus species.
Wing integration analysis using the PLS covariation method
We hypothesized that Antodynerus species wings are not a single integrated unit, i.e., it is an association of two subsets/blocks. For a comparative purpose, we selected two alternative types of wing axis organizations hypothesis: (i) anterior–posterior (AP) organization and (ii) proximal-distal (PD) organization (Anand et al., 2022; Karthika et al., 2022). Partial least square (PLS) within the configuration statistical method was used to analyze the degree of covariation between blocks. This analysis helps to understand the differences in shape that most closely covariate between blocks and indicates their relative contribution to the overall covariation between blocks (Klingenberg et al., 2003; Klingenberg, 2009). For hypothesis validation, forewing 26 landmarks were divided into two blocks: hypothesis 1—anterior–posterior (AP) wing axis with landmarks in block 1: 3, 4, 5, 6, 7, 8, 9, 10, 12, 13, 14, 18, and 19 and with landmarks in block 2: 1, 2, 11, 15, 16, 17, 20, 21, 22, 23, 24, 25, and 26 (Figure 2C); hypothesis 2—proximal-distal (PD) wing axis (i.e., wing base and wing blade region) with landmarks in block 1: 8, 9, 10, 11, 12, 13, 14, 15, 16, 17, 18, 19, 20, 21, 22, 23, 24, 25, and 26 and with landmarks in block 2: 1, 2, 3, 4, 5, 6, and 7 (Figure 2E).
In hindwing covariation analysis, nine landmarks were divided into two blocks: hypothesis 1—anterior–posterior (AP) wing axis with landmarks in blocks 1: 3, 4, 5, 6, and 7 and with landmarks in blocks 2: 1, 2, 8, and 9 (Figure 2D); hypothesis 2—proximal-distal (PD) wing axis with landmarks in block 1: 5, 6, 7, 8, and 9 and with landmarks in block 2: 1, 2, 3, and 4 (Figure 2F). In the PLS1 analysis, the integration magnitude among the subsets of points of interest was quantified as the RV coefficient. The RV coefficient higher than 0.5 indicated that the strength of the covariation between the block is high (i.e., the wing is considered as the single integrated unit) and the RV coefficient less than 0.5, and vice versa (Escoufier, 1973; Klingenberg, 2009). A 10,000 permutational analysis was used to evaluate the statistical significance level.
Phylogenetic signal analysis
Before analyzing the evolutionary shape and size phylogeny, the ancestral state reconstruction was done using Mesquite V3 (Maddison and Maddison, 2016). We mapped the PC score onto wing shape phylogeny and the centroid size onto wing size-based phylogeny. To track the phylogenetic signal of the symmetrical wing shape and size, a 10,000 permutational analysis was used to simulate the null hypothesis of the absence of a phylogenetic structure (Maddison, 1991; Rohlf, 2001; Laurin, 2004; Klingenberg and Gidaszewski, 2010; Pelabon et al., 2014; Žikić et al., 2017). We applied the multivariate regression analysis, i.e., size as an independent variable, and shape as the dependent variable to test the association of species-specific wing size and the venation pattern (wing allometry). A 10,000 permutational test was performed for regression analysis to assess statistical significance (Monteiro, 1999; Klingenberg, 2016; Žikić et al., 2017).
Results
For all morphological traits, the variation of each specimen in shape space was perfectly correlated with tangent space. In all species, the forewing and hindwing regression slopes are very close to 1.0 and the presence of a high correlation coefficient. It indicated that shape changes in each species’ wings (i.e., forewings and hindwings) are accurately captured for subsequent statistical analysis. It was clear from the one-way ANOVA analysis that there were no landmarking errors in our datasets because the mean square (MS) values for the individuals were lower than the error (Supplementary Tables S1–S3).
Wing asymmetry of Antodynerus species
The existence of wing size variations among Antodynerus species is clearly demonstrated by centroid size (CS) analysis. The centroid size of A. flavescens female forewings was 14638.35 ± 130.43; the female hindwings was 7495.189 ± 557.57; the male forewings was 12514.85 ± 166.27; and male hindwings was 5537.72 ± 220.13. A. limbatus female forewings centroid size is 14567.28 ± 538.32; the female hindwings was 6358.17 ± 64.61; the male forewings was 13047.32 ± 473.63; and the male hindwings was 5713.25 ± 57.03. A. punctatipennis female forewings centroid size was 12175.01 ± 123.15; the female hindwings was 5066.93 ± 53.47; the male forewings was 11014.72 ± 94.98; and the male hindwings was 4670.56 ± 61.58. By comparing the centroid size among the Antodynerus species, we found that A. flavescens had the largest wing size, followed by A. limbatus and A. punctatipennis (Figure 3).
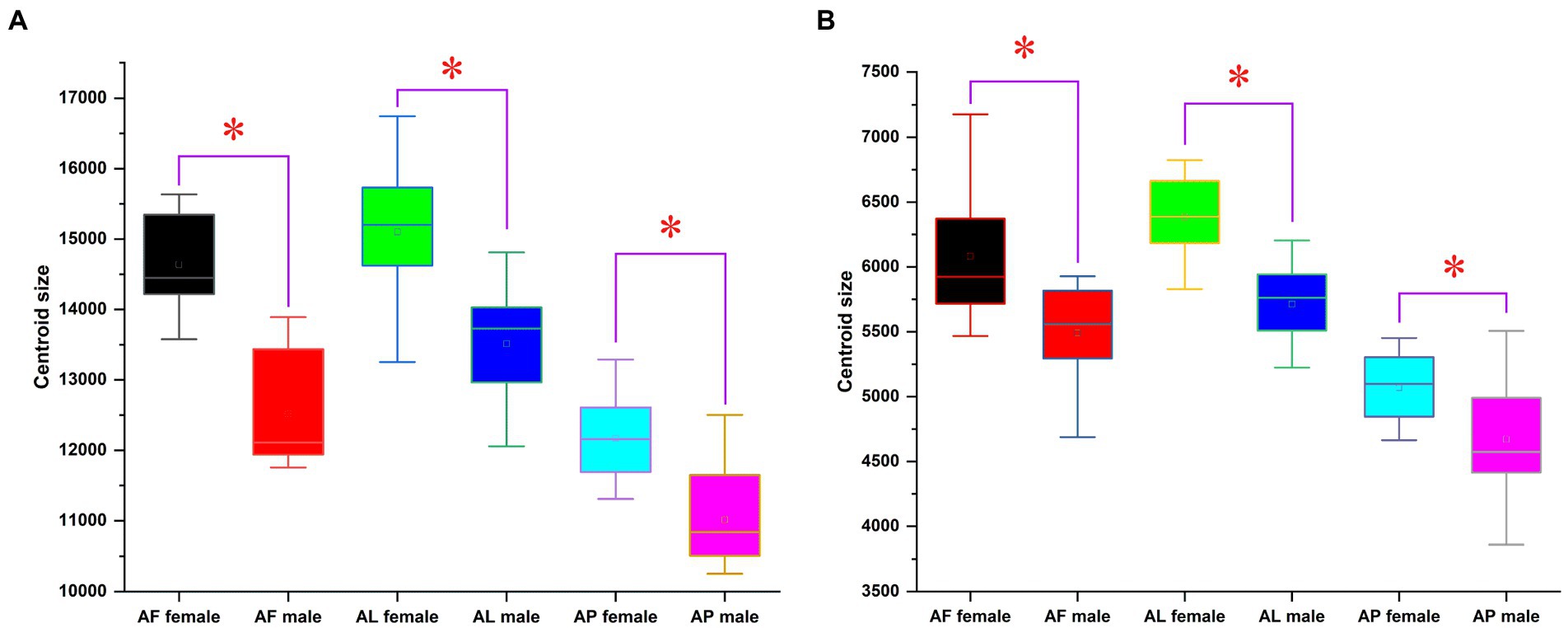
Figure 3. Comparison between centroid size of the (A) forewing (FW) and (B) hindwing (HW) of Antodynerus species. Significant discrimination (Mann–Whitney U-test) is marked with a star. AF, A. flavescens; AP, A. punctatipennis; AL, A. limbatus.
We combined the right and left forewings and hindwings, separately for the wing asymmetry after which we performed the Procrustes shape and size ANOVA. In A. limbatus, size-related directional asymmetry (DA) and fluctuation asymmetry (FA) were absent in male forewings and hindwings. Nevertheless, significant (p < 0.05) shape-related DA was present in all groups, and FA was observed only in female forewings and hindwings. Compare to size difference (right–left wing asymmetry), significant shape-related wing asymmetries were observed in forewings and hindwings of both sexes, and significant size differences were observed in male hindwings (Supplementary Table S1). Highly heterogenous wing asymmetry was observed in A. flavescens, because significant (p < 0.0001) size- and shape-related FA and DA were observed in female forewings and hindwings of A. flavescens, the right and left wings of females were not identical. However, in males, size-related significant DA was present only in the hindwing, and size-related FA was absent in male forewings and hindwings. Significant shape-related DA was present in male forewings and hindwings, while significant shape differences were observed in male right and left wings (Supplementary Table S2). A. punctatipennis, female population was very stable, there was no significant size- and shape-related FA and DA (except in change with hindwings shape, presence of DA), and there was no significant shape and size differences between right and left forewings and hindwings of female, i.e., left and right wings were more symmetrical. Significant size- and shape-related DA were present in male hindwings, while significant shape-related DA was found only in the male forewing, i.e., left and right male forewings, as well as hindwings, are more identical, with less asymmetry (Supplementary Table S3). Asymmetry of left and right forewings (Figure 4) and hindwing (Figure 5) were further confirmed by using DFA.
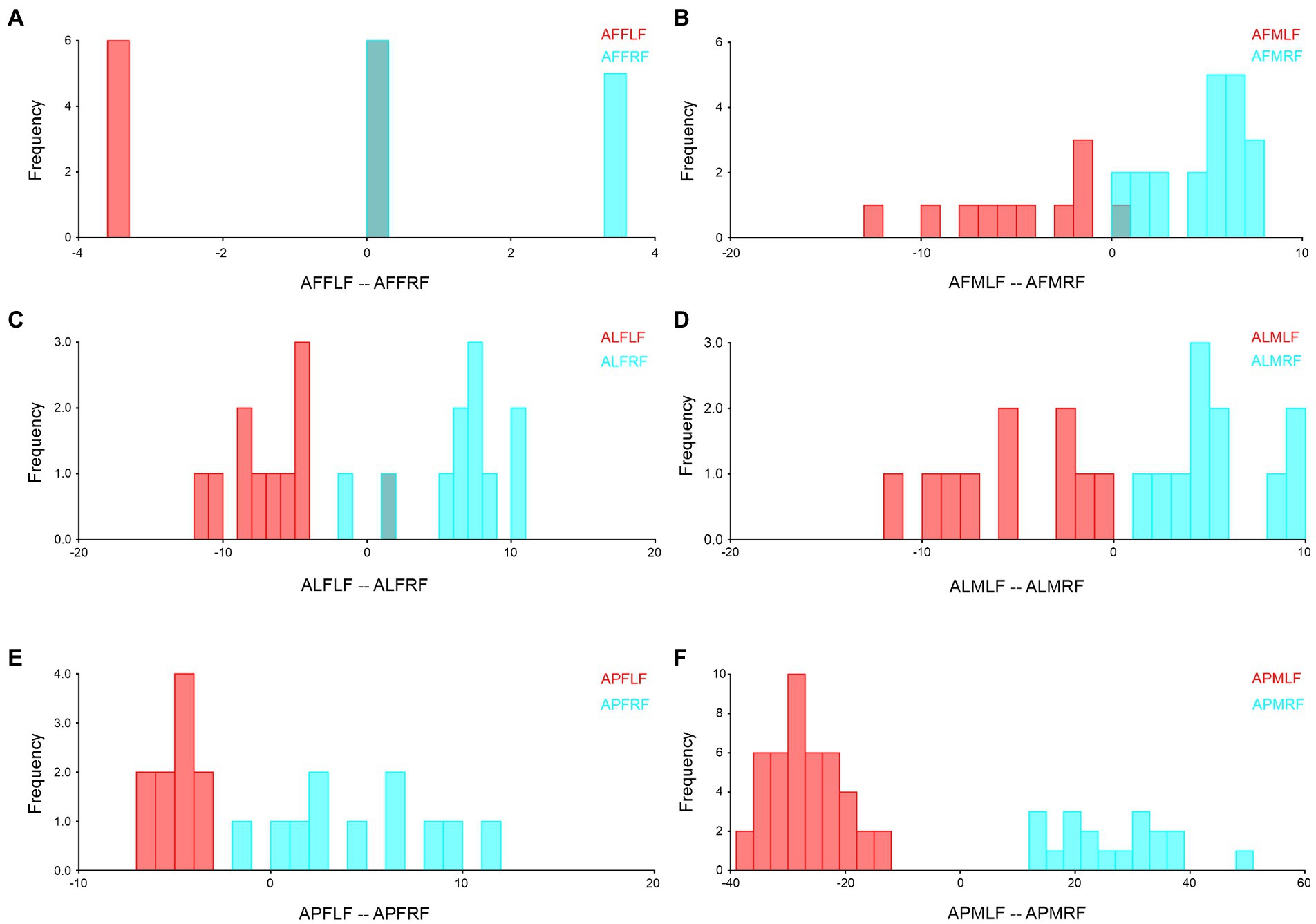
Figure 4. Forewing right–left asymmetry of Antodynerus species were verified by DFA. (A) A. flavescens female left and right forewing, (B) A. flavescens male left and right forewing, (C) A. limbatus female left and right forewing, (D) A. limbatus male left and right forewing, (E) A. punctatipennis female left and right forewing, and (F) A. punctatipennis male left and right forewing. Alphabet code used in figures, first alphabet indicated as the genus name, second letter as species name, third letter as sex (male or female), fourth letter as wing side (left or right), and fifth letter as wing position (fore or hindwing). AFFLF, A. flavescens female left forewing.
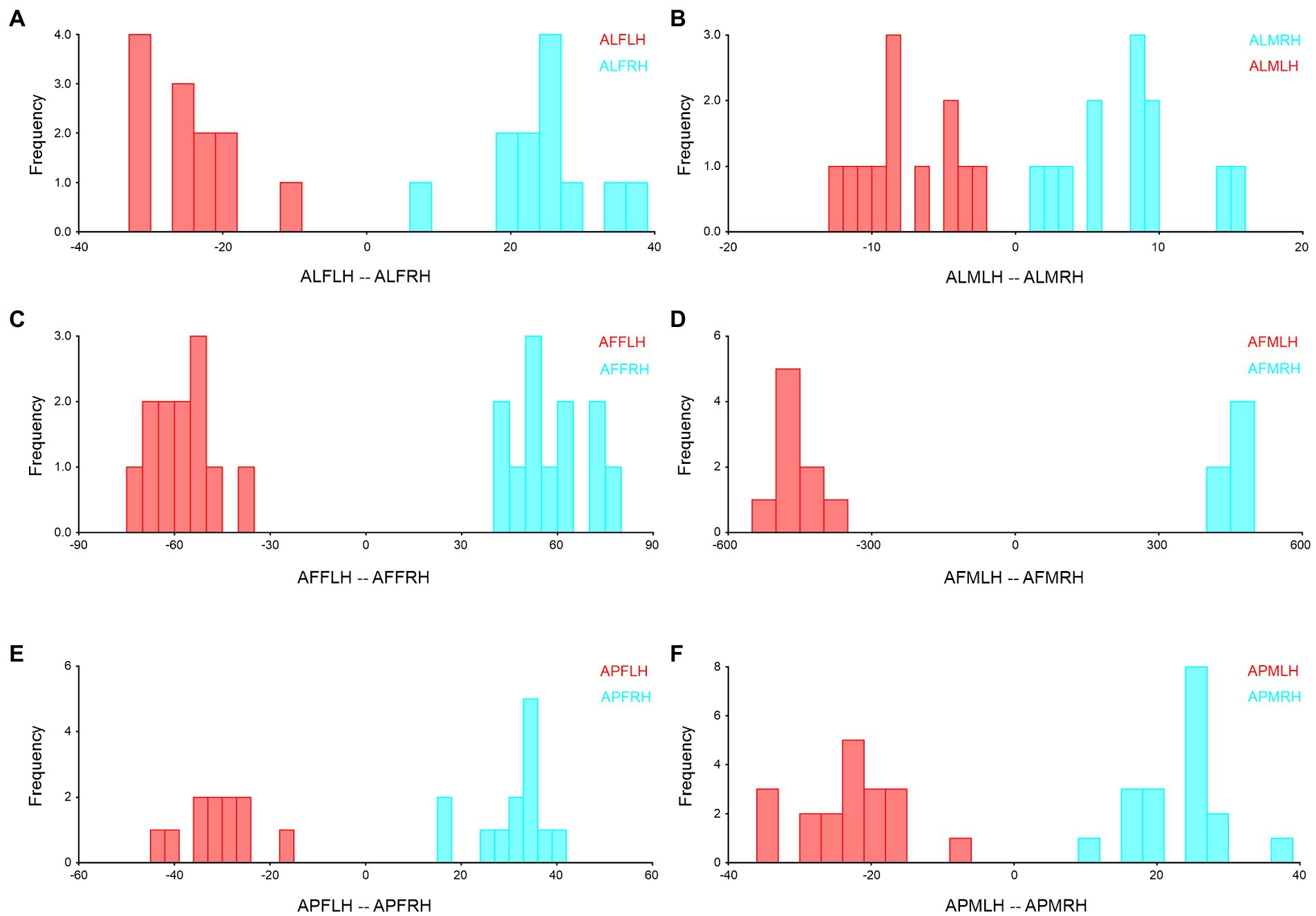
Figure 5. Hindwing right–left asymmetry of Antodynerus species were verified by DFA. (A) A. limbatus female left and right hindwing, (B) A. limbatus male left and right hindwing, (C) A. flavescens female left and right hindwing, (D) A. flavescens male left and right hindwing, (E) A. punctatipennis female left and right hindwing, and (F) A. punctatipennis male left and right hindwing. Alphabet code used in figures, first alphabet indicated as the genus name, second letter as species name, third letter as sex (male or female), fourth letter as wing side (left or right), and fifth letter as wing position (fore or hindwing). ALFLH, A. limbatus female left hindwing.
Sexual asymmetry of Antodynerus species
The sexual wing asymmetry analysis of Antodynerus species proved that significant size and shape differences existed in Antodynerus species, while in addition to size variations, shape variations can be used as a prominent tool for distinguishing the sexes with Antodynerus species. Compared to other species, A. flavescens has significant size- and shape-related sex-trait-based wing asymmetry. DA was found in all asymmetry analyses, while FA was only present in A. flavescens. Based on shape and size Procrustes ANOVA analysis, we found a high level of wing sex-trait asymmetry in A. flavescens and followed by A. limbatus and A. punctatipennis (high-to-low wing asymmetry). The sexual asymmetries of Antodynerus species in their forewing (Figure 6) and hindwing (Figure 7) were further verified by using DFA.
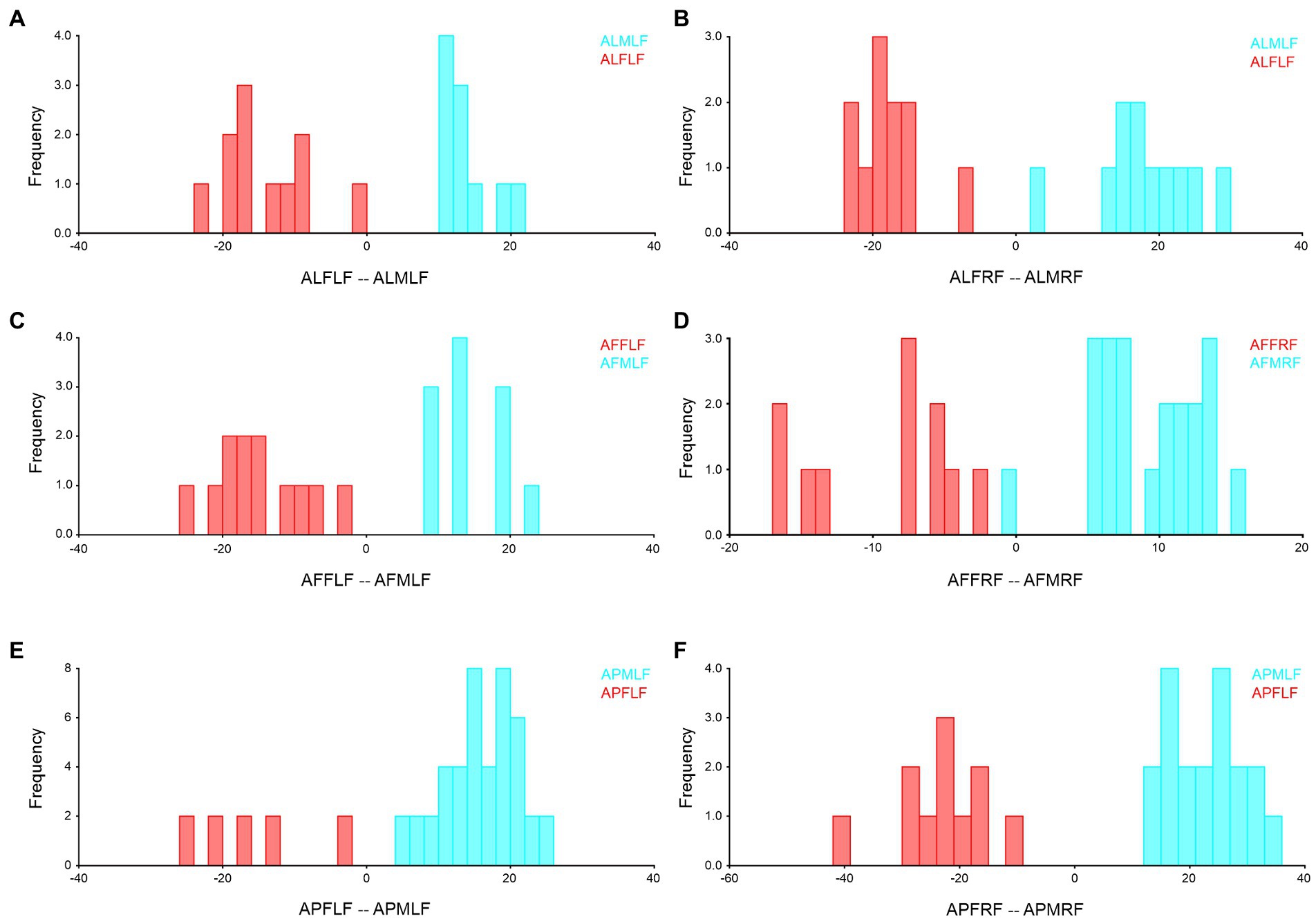
Figure 6. Forewing sexual asymmetry of Antodynerus species were verified by DFA. (A) A. limbatus female left and male left forewing, (B) A. limbatus female right and male right forewing, (C) A. flavescens female left and male left forewing, (D) A. flavescens female right and male right forewing, (E) A. punctatipennis female left and male left forewing, and (F) A. punctatipennis female right and male right forewing. Alphabet code used in figures, first alphabet indicated as the genus name, second letter as species name, third letter as sex (male or female), fourth letter as wing side (left or right), and fifth letter as wing position (fore or hindwing). ALFLF, A. limbatus female left forewing.
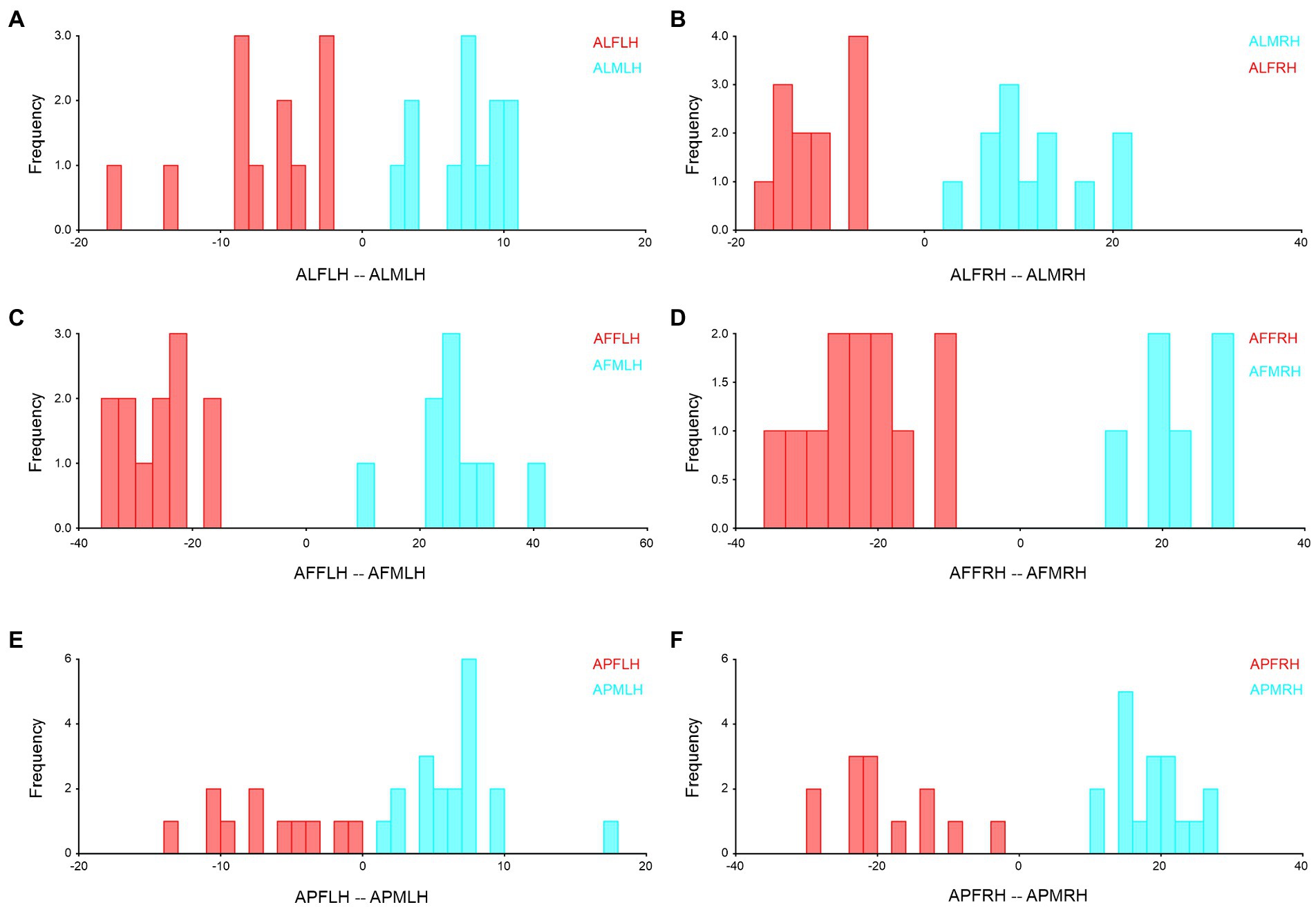
Figure 7. Forewing sexual asymmetry of Antodynerus species were verified by DFA. (A) A. limbatus female left, and male left hindwing, (B) A. limbatus female right and male right hindwing, (C) A. flavescens female left, and male left hindwing, (D) A. flavescens female right and male right hindwing, (E) A. punctatipennis female left and male left hindwing, and (F) A. punctatipennis female right and male right hindwing. Alphabet code used in figures, first alphabet indicated as the genus name, second letter as species name, third letter as sex (male or female), fourth letter as wing side (left or right), and fifth letter as wing position (fore or hindwing). ALFLH, A. limbatus female left hindwing.
Trait-based morphospace distribution analysis of Antodynerus species
PCs and CVA were used to verify the similarity and dissimilarity of Antodynerus species based on wing-specific traits (i.e., right–left asymmetry and sexual asymmetry). When compared to PCs morphospace analysis, CVs analysis distinguished Antodynerus species based on wing traits. The first two PCs in forewing PCs morphospace analysis represent less than 40% of population characteristic features (Figure 8), whereas the first two PCs in hindwing analysis represent more than 65% of population characteristic features (Figure 9). However, in the analysis of CVs, the first two CVs explained more than 65% of characteristic features. The morphospace analysis of wings revealed that approximately 30%–50% of wing traits are highly symmetric in nature. However, if the population represents less than 50% of characters, they cannot be distinguished in trait-based morphospace because the congeneric species share similar wing traits, i.e., similar morphospace.
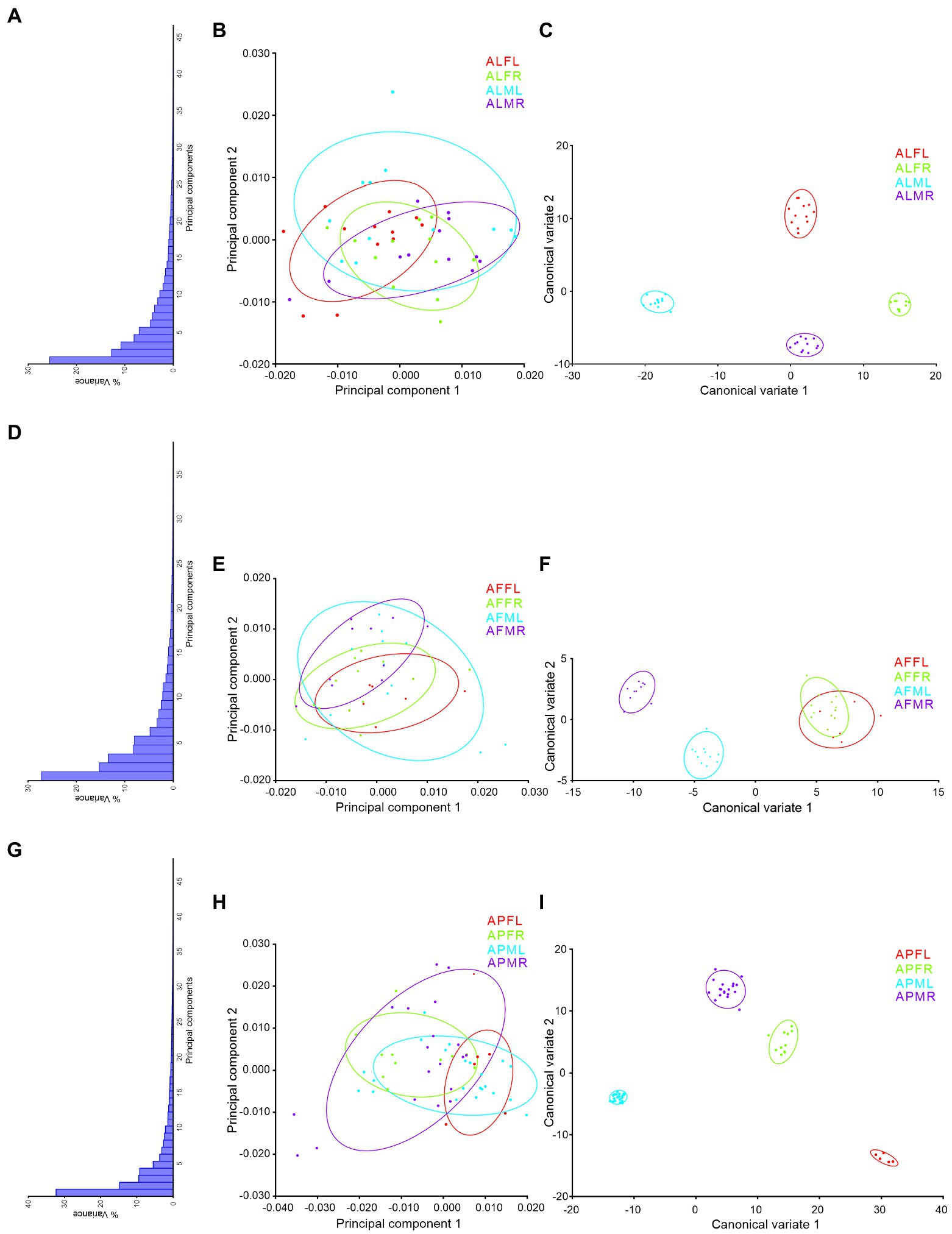
Figure 8. wing-specific morphospace trait distribution of Antodynerus species forewing. (A) % of variance, (B) PC1 vs. PC2, (C) CV1 vs. CV2 of A. limbatus forewing; (D) % of variance, (E) PC1 vs. PC2, (F) CV1 vs. CV2 of A. flavescens forewing; (G) % of variance, (H) PC1 vs. PC2, (I) CV1 vs. CV2 of A. punctatipennis forewing. Alphabet code used in figures, first alphabet indicated as the genus name, second letter as species name, third letter as sex (male or female), and fourth letter as wing side (left or right). ALFL, A. limbatus female left.
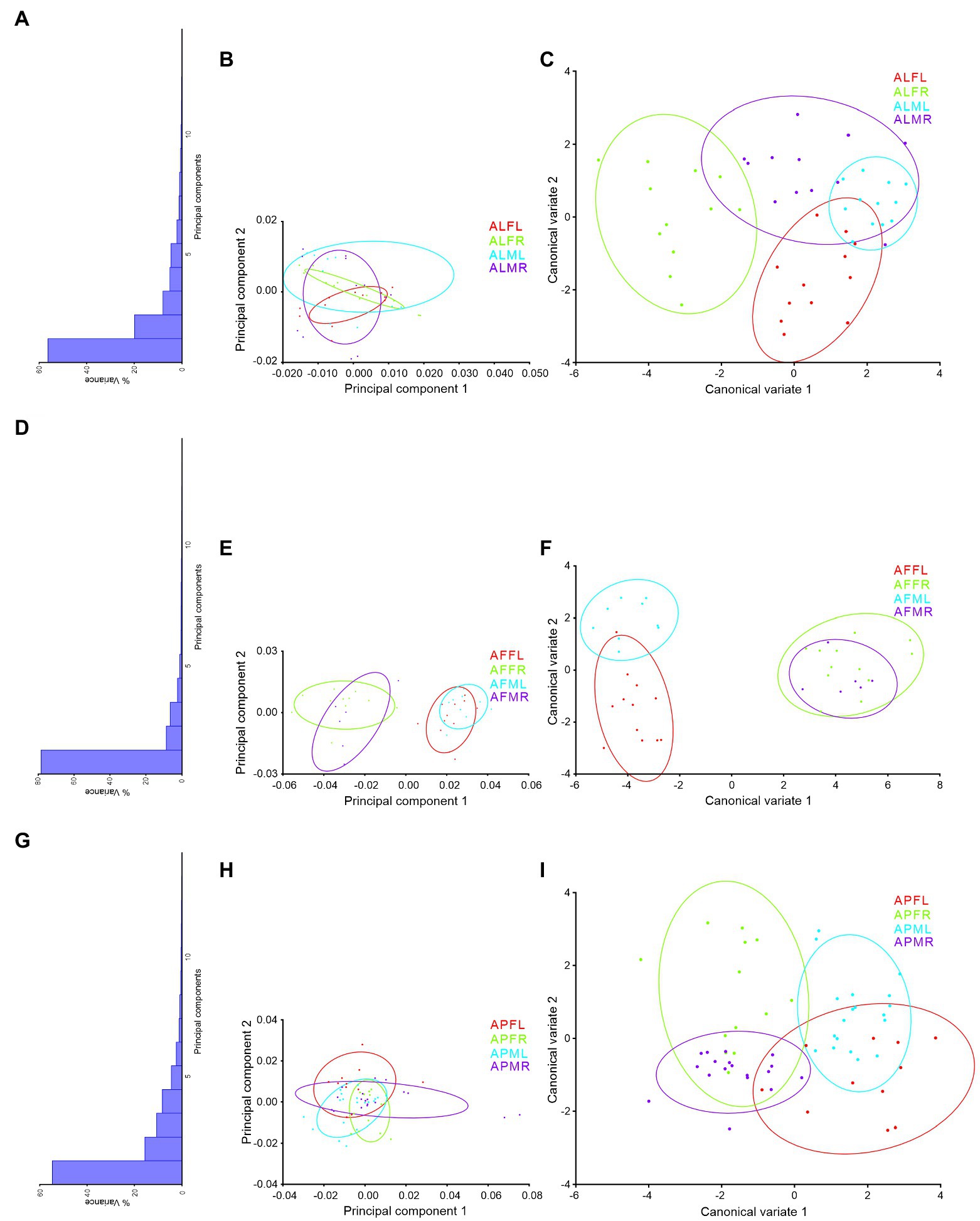
Figure 9. Wing-specific morphospace trait distribution of Antodynerus species hindwing. (A) % of variance, (B) PC1 vs. PC2, (C) CV1 vs. CV2 of A. limbatus hindwing; (D) % of variance, (E) PC1 vs. PC2, (F) CV1 vs. CV2 of A. flavescens hindwing; (G) % of variance, (H) PC1 vs. PC2, (I) CV1 vs. CV2 of A. punctatipennis hindwing. Alphabet code used in figures, first alphabet indicated as the genus name, second letter as species name, third letter as sex (male or female), and fourth letter as wing side (left or right). ALFL, A. limbatus female left.
Species-specific trait-based morphospace distribution analysis, in PCs morphospace distribution analysis, a total of 48 PCs were present in the forewing (Figure 10A) and 15 PCs present in the hindwing (Figure 10C) of Antodynerus species. In forewing PCs morphospace distribution analysis, 33.81% of variations were explained by PC1 vs. PC2 (PC1 covered 20.43% and PC2 covered 13.37% of variations; Figure 10A). These variations are not enough to distinguish the shape and size of the Antodynerus species (Figure 10B). The first two PC axes describe 76.90% of the total variance in hindwings shape (PC1 = 49.12%, PC2 = 26.78%; Figure 10C). These variations are enough to explain the inter-species shape and size variations between Antodynerus species (Figure 10D). Compared to other species, PCs analysis confirmed that A. flavescens is a highly asymmetric species, as well as that the three species share around 30%–50% of similar wing shape and size characteristics.
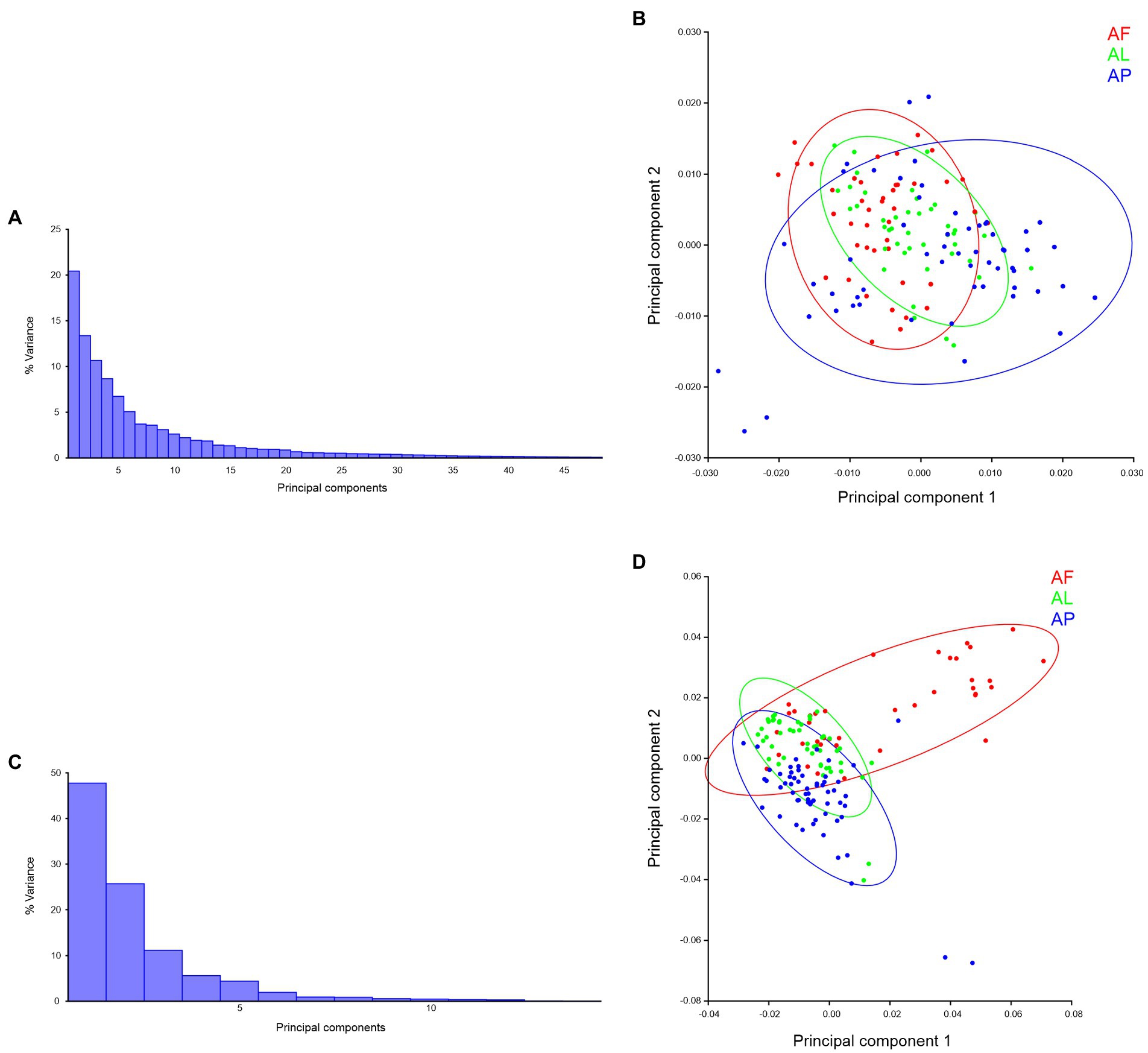
Figure 10. Species-specific wing trait morphospace distribution of Antodynerus species—PCs-based analysis. (A) % variance, (B) PC1 vs. PC2 of Antodynerus species forewing. (C) % of variance, (D) PC1 vs. PC2 of Antodynerus species hindwing. AF, A. flavescens; AP, A. punctatipennis; AL, A. limbatus.
In a CVA morphospace distribution analysis, the first two CVs explained 67.05% of the total variance in forewing shape (CV1 = 39.91% and CV2 = 27.13%) of Antodynerus species. These variations significantly contribute to the discrimination of the three species in a morphospace, i.e., we did not find their overlapping in 90% of confidence ellipses (Figure 11A). However, we found that the first two CV axes describe 81.09% variations of the total variance in hindwing shape (CV1 = 61.26% and CV2 = 19.82%). We found that A. flavescens and A. punctatipennis occupy a distinct morphospace, as well as that A. limbatus is closely associated with A. flavescens (Figure 11C).
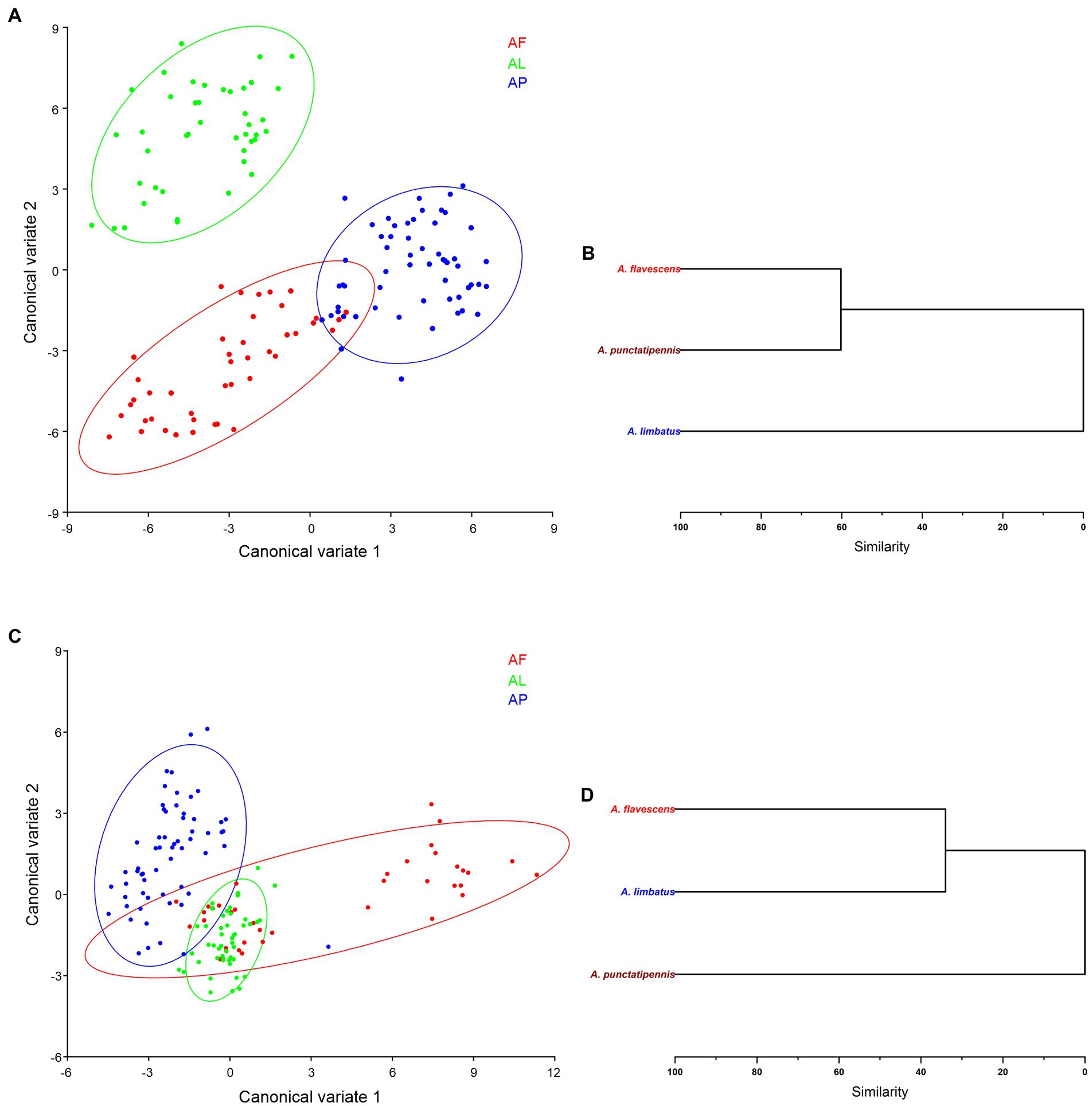
Figure 11. Species-specific wing trait morphospace distribution of Antodynerus species—CVA-based analysis. (A) CV1 vs. CV2 of Antodynerus species forewing, (B) hierarchical cluster dendrogram of Antodynerus species forewing. (C) CV1 vs. CV2 of Antodynerus species hindwing, and (D) hierarchical cluster dendrogram of Antodynerus species hindwing. AF, A. flavescens; AP, A. punctatipennis; AL, A. limbatus.
All Antodynerus species had a significant Mahalanobis distance. Each species Mahalanobis distance was used to generate a hierarchical cluster dendrogram. Based on the obtained values of Mahalanobis distance for their forewings (Mahalanobis distance = 5.0494, p < 0.001), we found that A. flavescens and A. punctatipennis diverged from the same lineage and then separated at 60% similarity, while A. limbatus represents an entirely distinct clade (Figure 11B; Supplementary Table S4). Contrary to previous results and based on the obtained values of Mahalanobis distance for their hindwings (Mahalanobis distance = 2.9259, p < 0.001), we found that A. flavescens and A. limbatus diverged from the same clade and then separated at 40% of similarity, while A. punctatipennis represents an entirely distinct clade (Figure 11D; Supplementary Table S5).
To provide additional support, species-specific DFA was employed to assess the similarity and differences between each species. This analysis confirmed that every species has a unique shape and size, which was statistically significant (p < 0.05; Figure 12). However, we found that A. flavescens and A. limbatus are significantly distinguished in their forewings (Figure 12A), as well as both species share around 20% of similar wing venation, shape, and size patterns in hindwings (Figure 12D). Overall, based on DFA trait-based analysis, we found that A. punctatipennis and A. limbatus forewings and hindwings do not share any similar characteristic features (Figures 12C, F).
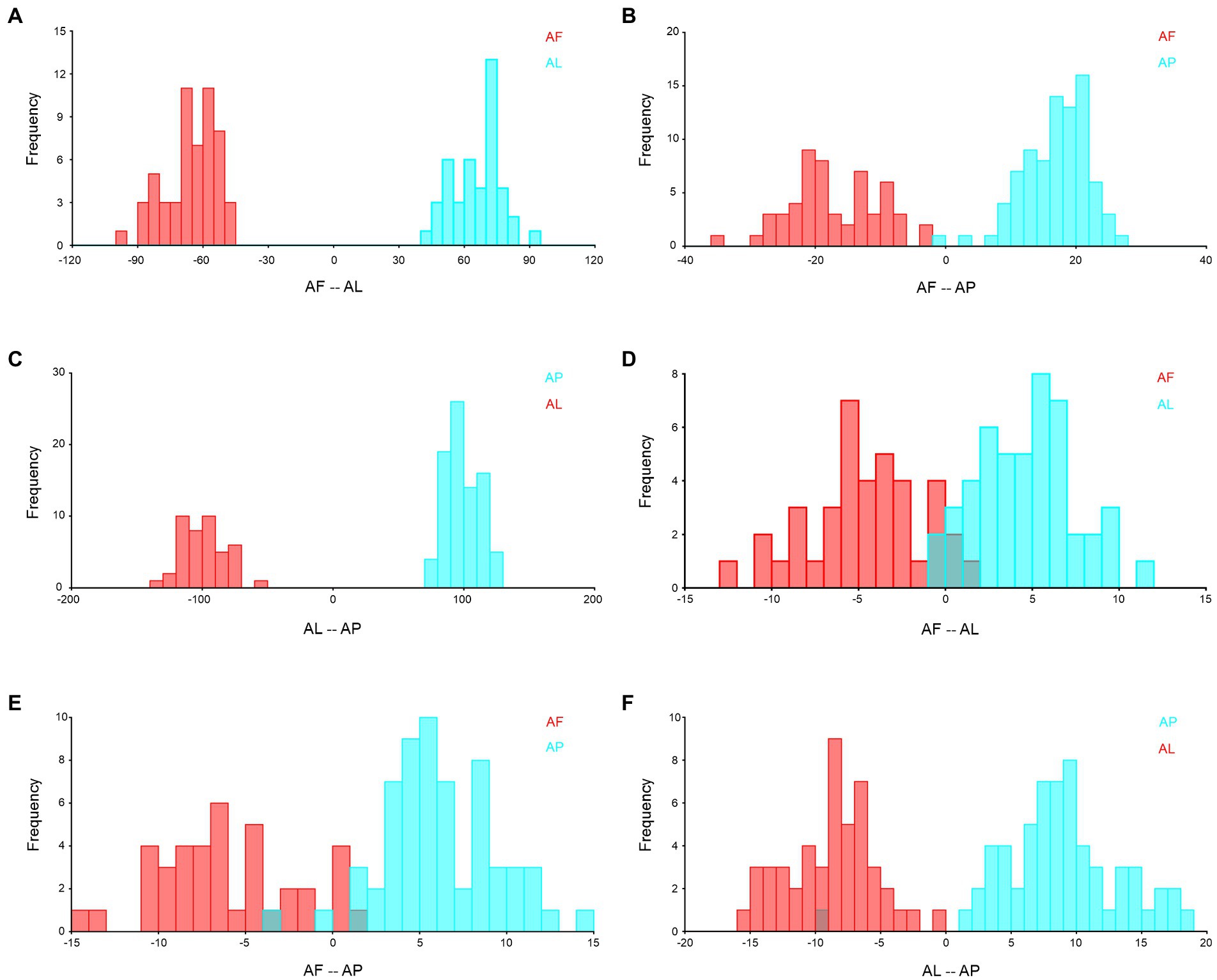
Figure 12. Species-specific wing variation validated by using DFA with 10,000 permutational analyses, and all are statistically significant at p < 0.01. (A) forewing of A. flavescens and A. limbatus, (B) forewing of A. flavescens and A. punctatipennis, (C) forewing of A. limbatus and A. punctatipennis, (D) hindwing of A. flavescens and A. limbatus, (E) hindwing of A. flavescens and A. punctatipennis, and (F) hindwing of A. limbatus and A. punctatipennis. AF, A. flavescens; AP, A. punctatipennis; AL, A. limbatus.
Size and shape similarity and difference across Antodynerus species
2B-PLS analysis was used to validate shape and size similarities and differences among the Antodynerus species. In forewing size analysis (block 1; Figure 13A), we found that A. punctatipennis significantly differs from the other two species, while in shape analysis (block 2; Figure 13B), A. punctatipennis and A. flavescens vary significantly, and A. limbatus shares 20%–30% of the shape character with A. punctatipennis. In hindwing size analysis, we found that A. flavescens is marginally delineated from A. punctatipennis and A. limbatus (Figure 13C). Based on the hindwing shape similarities and differences across Antodynerus species, A. flavescens is distinguishable from A. limbatus and A. punctatipennis (Figure 13D). Results of this analysis indicated that A. punctatipennis, with different shape and size features, share similar traits more with A. limbatus than with A. flavescens. A. flavescens showed a more similar shape and size with A. limbatus than with A. punctatipennis.
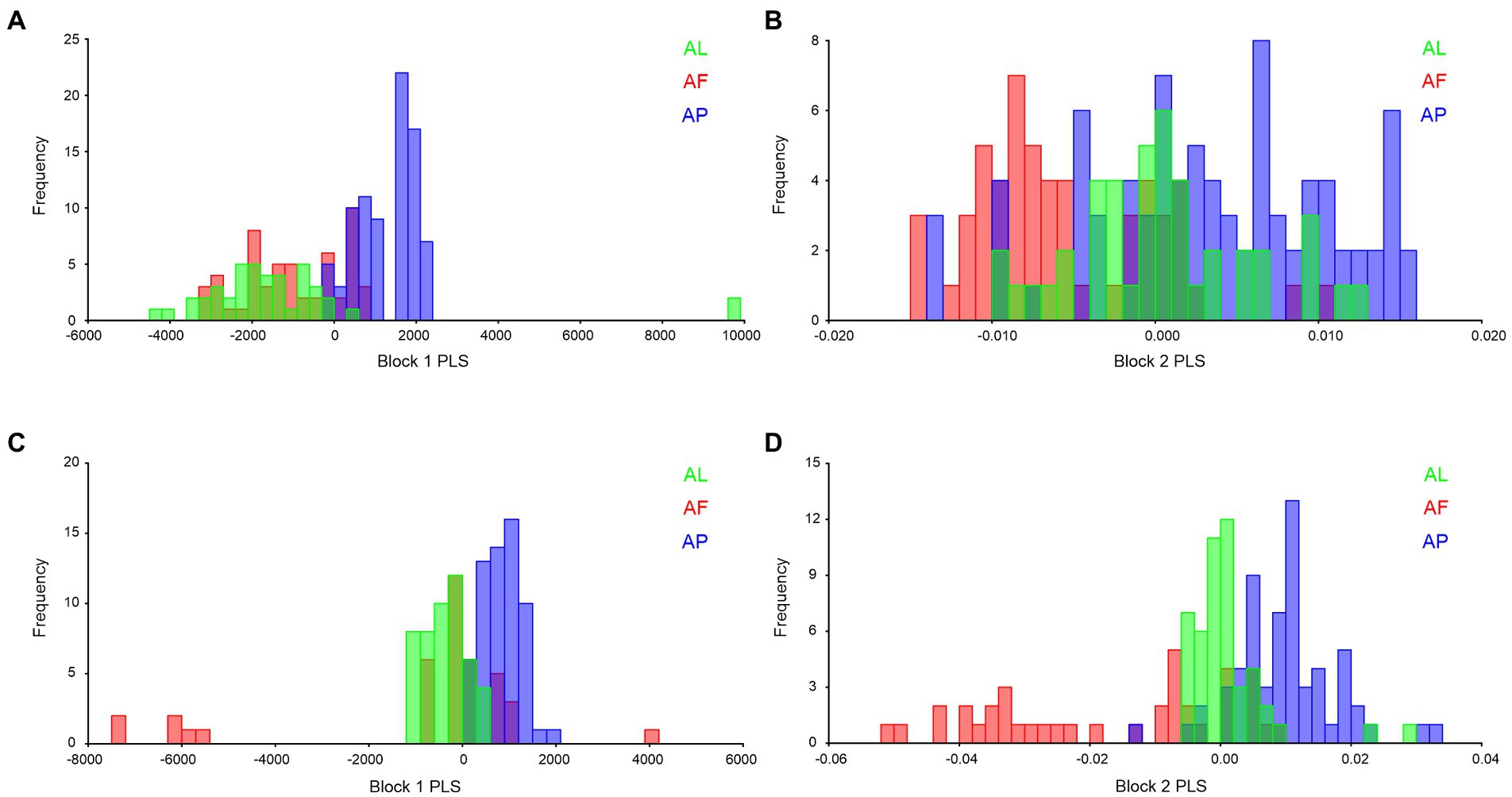
Figure 13. Species-specific wing similarities and differences, validated by using 2B-PLS with 10,000 permutational analyses, and all are statistically significant at p < 0.01. (A) block 1 (size) of Antodynerus species forewing, (B) block 2 (shape) of Antodynerus species forewing, (C) block 1 (size) of Antodynerus species hindwing, and (D) block 2 (shape) of Antodynerus species hindwing. AF, A. flavescens; AP, A. punctatipennis; AL, A. limbatus.
Wing covariation/integration analysis in Antodynerus species
The RV coefficients of the hypothesis—AP and PD covariation were insignificant (RV > 0.5) for all the components of forewing and hindwing of both sexes of Antodynerus species. This confirmed that there was no subsets/blocks level organization in the forewing and hindwing of Antodynerus species male and female. The wing was considered the single integrated unit, and it was verified by permutational analysis; the integration was statistically significant at p < 0.05 (Supplementary Table S6).
Phylogenetic signal analysis of Antodynerus species
The first two PC axes explain the 33.810% of Antodynerus species forewings variance. We found that statistically significant phylogenetic signal in the shape of the forewing (tree length = 0.00025533, p ≤ 0.0001) and we noticed two lineages from the hypothetical phylogenetic root, one lineage for A. punctatipennis and other lineage for A. limbatus and A. flavescens (this suggested that A. limbatus and A. flavescens evolved from a common hypothetical ancestor). A. punctatipennis is highly influenced by PC1 and A. flavescens is highly influenced by PC2. A. limbatus and A. flavescens originated from the same hypothetical ancestor root, but they are positioned in a different part of phylomorphospace (Figure 14A). There is no statistically significant phylogenetic signal for forewing size (tree length = 2836258.20624680, p = 0.3252). In contrast to the A. punctatipennis, A. limbatus is characterized by a higher centroid size value. Similar to the results for the forewings, we noticed two lineages from the common hypothetical ancestor, one lineage for A. punctatipennis and the other lineage for A. limbatus and A. flavescens, as well as that all three species are positioned in a different centroid size (Figure 14C).
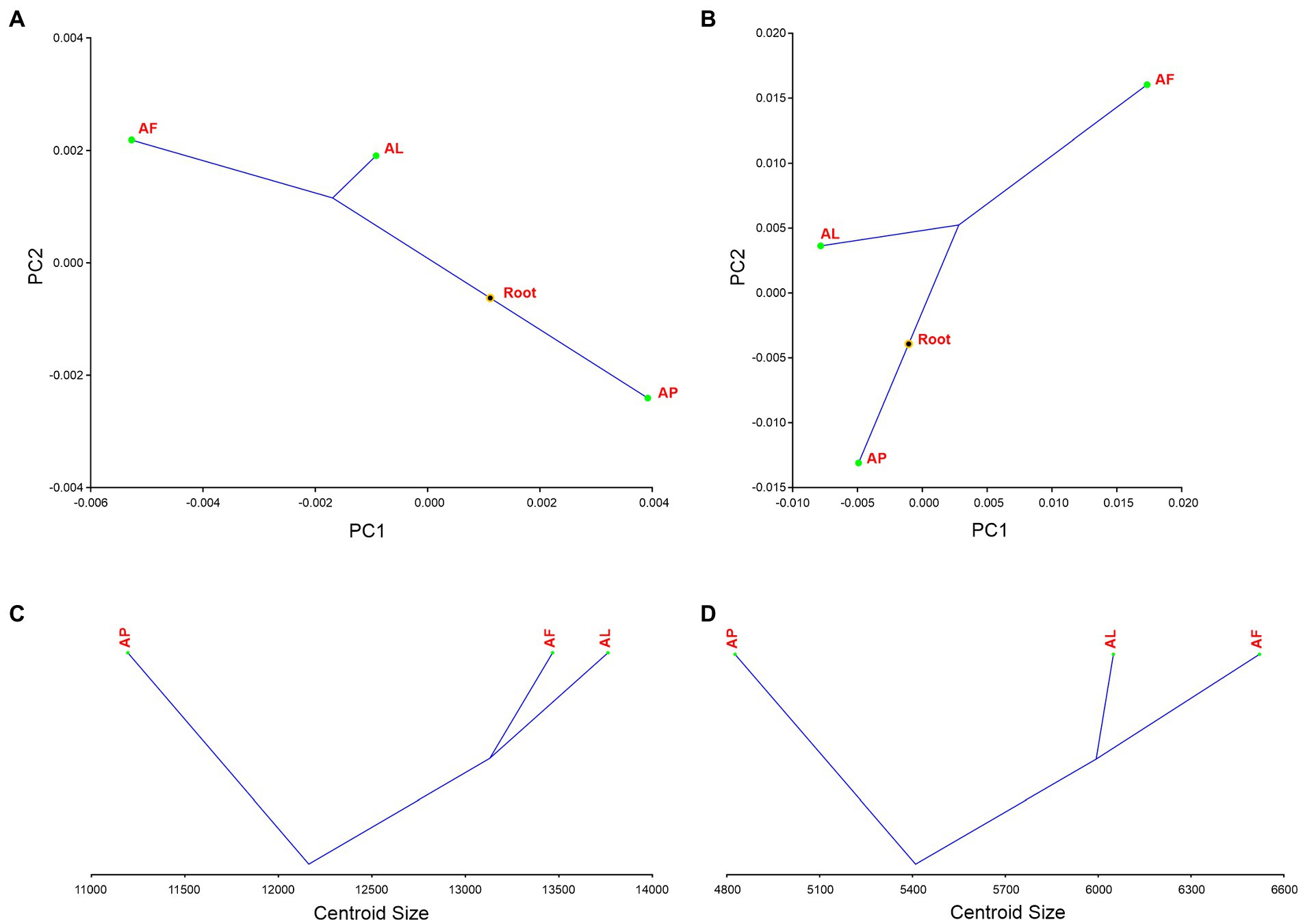
Figure 14. Phylogenetic signal analysis of Antodynerus species (A) PCs forewing – shape phylogenetic analysis, (B) PCs hindwing—shape phylogenetic analysis, (C) forewing centroid size analysis, and (D) hindwing centroid size analysis. AF, A. flavescens; AP, A. punctatipennis; AL, A. limbatus.
We did not find a statistically significant phylogenetic signal in the hindwing shape (tree length = 0.00436403, p = 0.2304; Figure 14B) and size (tree length = 962559.2316856, p = 0.3320; Figure 14D) of Antodynerus species. Same as in forewing phylogenetic signal analysis, phylomorphospace distribution analysis of hindwing clearly proved that A. punctatipennis is entirely a distinct species compared to A. limbatus and A. flavescens. Shape phylogenetic analysis PC1 vs. PC2 explained 76.90% of the total variance in wing shape. The first two PCs clearly separated the congeneric species of Antodynerus. In contrast to the A. punctatipennis, A. flavescens is characterized by a higher centroid size value.
In the multivariate regression analysis, allometry of independent contrast of wing size and dependent contrast of wing shape (i.e., venation pattern) analysis, there is no significant relationship (p > 0.05) between the size and venation pattern of wings; however, some exceptional cases are always present in this congeneric species. In the allometry analysis, significant (p < 0.05) shape-related size variations were observed only in A. flavescens female left forewings and female right hindwings; A. limbatus female left forewings and right hindwings, male left hindwings, and male right forewings and hindwings; and A. punctatipennis female left and right forewings, and male left forewings, and male right hindwings.
Discussion
Morphological characteristics like wing shape, size, and venation patterns are important traits for distinguishing taxa with unclear status (Stary, 1970; Godfray, 1994). GM has proven to resolve taxonomic issues in closely related species effectively, such as cryptic species and species complexes (Baylac and Daufresne, 1996; Kos et al., 2011; Mitrovski-Bogdanovic et al., 2013; Tomanović et al., 2013). This study explored the wing morphology of solitary wasp, genus Antodynerus de Saussure, 1855 from the Indian subcontinent. Antodynerus species separation was based on the morphological architectural variation present in the body rather than the wing morphology (Girish Kumar and Carpenter, 2013). Using several multivariate taxonomic approaches, we demonstrated that wing morphology could be employed as a key diagnostic tool for species identification in the genus of Antodynerus because each species has its own trait-based morphospace delimitation boundary.
Wing asymmetry
The overall wing size difference among Antodynerus species was measured using centroid size variations. Among the three species, the large-sized wing was observed in A. flavescens, followed by A. limbatus and A. punctatipennis. Significant wing size dimorphism (sexual dimorphism) existed in all three Antodynerus species, i.e., female populations have larger size wings than males. The wing size variations suggested the presence of species-specific evolutionary allometry of Antodynerus species. Compared to size variations, shape variations provide more reliable information about phenotypic variations and population stability (Klingenberg, 2003; Moraes et al., 2004; Dujardin, 2008). Among the three species, A. limbatus has a highly heterogeneous population structure because of the current high level of size variation compared to the other species and followed by A. flavescens. In A. limbatus and A. flavescens, female populations are more heterogenous than male. In terms of wing centroid size, A. punctatipennis is a highly stable population compared to other species.
Right- and left-wing asymmetry analysis is directly correlated with the flying ability of the insects. Less right- and left-wing asymmetry suggested a higher level of maneuverability (Thornhill, 1992). Compared to size asymmetry, significant (p < 0.05) shape asymmetry was observed in the forewings and hindwings of both sexes of A. limbatus and A. flavescens. Among the Antodynerus species, females have a high degree of right–left wing asymmetry in both wings (forewing and hindwing), as well as the existence of shape- and size-related FA and DA. We believed that high levels of FA and DA were the major reasons for the heterogenous population structure of A. flavescens and A. limbatus. The FA was considered an effective method for detecting developmental instability (DI) and represents the organism’s inability to cope with stress factors and the disturbances that arise from development (Palmer and Strobeck, 1986; Palmer, 1994; Klingenberg and McIntyre, 1998). In the case of DA, the shape-related DA was found in all three species. DA is very common in insects’ taxa. The genetic basis of DA was evolutionarily preserved due to the existence of the left–right body axis, which determined the position of the wing imaginal disk (Klingenberg et al., 1998). The higher degree of FA, the higher level of interspecific competition within and between species, which affects an organism’s mating success and life span (Thornhill, 1992). Supporting the study by Thornhill (1992), in our study, we also observed higher wing asymmetry in A. flavescens and A. limbatus. Based on the findings, we suspected that a higher degree of wing asymmetry in the female sex of A. flavescens and A. limbatus might affect the mating success, flying ability, and life span.
Sexual dimorphism in wings
Sexual dimorphism is one of the most intriguing sources of phenotypic variations, and it is recognized as a critical area of evolutionary biology research (Benitez et al., 2011). Many species of the orders Hymenoptera, Diptera, Lepidoptera, and Coleoptera species are characterized by the presence of sexual dimorphism, which was validated and verified using the GM tool (Bonduriansky, 2006; Gidaszewski et al., 2009; Marsteller et al., 2009; Benitez et al., 2011, 2013; Lemic et al., 2014). In wasps, females are often larger than males, and females have several adaptive advantages over males, including greater fecundity and parental care (Forrest, 1987; Moller and Zamora-Munoz, 1997; Reeve and Fairbairn, 1999). However, in some species, males are longer but have less mass than females (Cepeda-Pizarro et al., 1996). It is understood, rather than assessing the size of the wing, a more comprehensive morphological tool is necessary to infer the sexual dimorphism of species (Gidaszewski et al., 2009; Benitez et al., 2010). Using GM analysis, we observed significant sexual dimorphism in the three Antodynerus species. The presence of FA and DA is masking the sexual dimorphism traits in PC morphospace analysis, which was verified and visualized in discriminant analysis (i.e., CVA and DFA; Figures 6–9). Interesting to note that the forewings were more sexually asymmetric than the hindwings. Wasps exhibit shape- and size-related sexual dimorphism, as well as most of the phenotypic variations, are impacted by the natural selection pressure (Shreeves and Field, 2008; Mitrovski Bogdanović et al., 2009; Zikic et al., 2009); so, we can use these features in GM and delimit sex. The main explanation for sex dimorphism in insect wings is the different developmental life strategies of males and females (Richards, 1927; McLachlan, 1986; Svensson and Petersson, 1987; Sivinski and Dodson, 1992). Different developmental strategies could be the reason behind the sex-specific wing morphology of potter wasps. So far, it has been found that there are only a few studies that have researched wing dimorphism in wasps. Pretorius (2005) investigated the existence of sexual dimorphism in 24 species in the genus Tachysphex (Hymenoptera, Crabronidae) using GM. Based on this study, it could be concluded that GM is an efficient tool in discriminating the sex of wasps. The phenotypic response of wings has a highly adaptive significance and could be linked to different environmental functions as well as to the physiological constraints of flight (Berwaerts et al., 2002; Mitrovski Bogdanović et al., 2009).
Previously, most of the GM analysis in the wasp group was conducted on the forewing shape and size variations, but in this study, we included both forewings and hindwings. In fact, the forewing shape is becoming more popular in systematics since it can help to resolve many taxonomic problems (Dehon et al., 2014; Perrard et al., 2014). In previous GM analyses of wasps (Pretorius, 2005; Mitrovski Bogdanović et al., 2009; Baracchi et al., 2011; Perrard et al., 2016; Žikić et al., 2017; dos Santos et al., 2019), less than 20 landmarks in the forewing were identified and employed for GM analysis. In our studies, we used the maximum number of landmarks (forewing with 26 landmarks and hindwing with nine landmarks), which represents a large number of shape variables. Thus, the use of a large number of landmarks helps in unraveling the minute morphological changes in the wings of Antodynerus species. In general, congeneric species clustered together, i.e., closely related species are expected to show high morphological similarity than unrelated taxa, which was confirmed in forewing PCs morphospace analysis (Figure 10). GM tools allow us to discriminate the minute morphological differences between the taxa (Moraes et al., 2004). From the overall analysis (PCs, CVA, and DFA), we clearly observed that morphological wing-specific species delimitation was observed in Antodynerus species. In our study, we proved that wing characters could be used as a prominent tool for distinguishing the congeneric species. In support of our findings, Villemant et al. (2007) used GM to distinguish the four species of the genus Eubazus (Hymenoptera: Braconidae). In that analysis, four species separated in a distinct morphospace, with a reliability of 98.6% for well-classified females and 93.1% for males. Villemant et al. (2007) used GM to evaluate the relationship between the effect of host species on the wing shape of parasitoids, concluding that wing shape differences are correlated with the host species.
Wing covariations
Longitudinal wing folding is a common diagnostic trait of the Vespidae, which are exclusively found in subfamilies Eumeninae, Polistinae, and Vespinae (Hines et al., 2007). Wing venation, folding patterns, and other wing characters have traditionally been regarded as relevant in taxonomic and phylogenetic research (Forbes, 1922; Crowson, 1961, 1973; Dolin, 1975; Scholtz, 1990; Anand et al., 2022; Karthika et al., 2022). However, whether wing traits are relevant for phylogenetic analyses and how wing traits have developed throughout time remain mainly unknown (Bai et al., 2011). Due to the presence of wing folding, we speculated that the wings of the Antodynerus species are not a single integrated structure, but rather a combination of modules or covariation blocks. For the wing covariation analysis, we selected two alternative hypotheses for wing covariation analysis: AP (anterior–posterior) and PD (proximal-distal) wing modular organizations. Both covariation modular hypothesis was rejected at RV > 0.5 and statistically significant at p < 0.05, confirming that there is no modular organization structure in Antodynerus species wings by using two blocks. This indicated that the potter wasp wing is a highly integrated structure with a high degree of covariation across the structure, i.e., any alterations that occur in any region of the wings will have a direct effect on the corresponding regions of the wings. We have found no primary data supporting testing the division of the Eumeninae wing and its modules or covariation blocks. However, since modules are hierarchically nested units (Wagner et al., 2007), the presence of any major modules does not discard such modules that could be subdivided further (Suzuki, 2013; Munoz et al., 2016). In insects, the forewings and hindwings develop from distinct imaginal discs and they might be considered independent modules (Klingenberg, 2003). If each wing is the product of identical ontogenic processes, the variation should be homogenous and similar for each wing data (forewings and hindwings/right and left wings), and the results obtained from those data would be similar (Klingenberg, 2003). We suspected that the presence of FA, as well as wing size and shape allometry, was the reason for the highly integrated/covariate wing structure of Antodynerus species. More research is needed in the future to understand the longitudinal folding of Vespidae and its significance in the morphological evolution of wings. To the best of our knowledge, no one has investigated the morphological integration of wasp wings. Perrard (2019) used computed tomography and GM to investigate the morphological integration of 22 Vespidae species’ forewings and hindwings, thorax, and petiole. Perrard (2019) proved that there is a clear relationship between the petiole and wings or thorax shape but not between wings and thorax. The morpho-functional integration is directly correlated with the flying ability of the wasps. Wasps with elongated bodies have pointed wings, which are thought to aid flying maneuverability. In contrast, stouter species have rounded wings, which may allow for faster flight speeds (Perrard, 2019).
Phylogenetic signal
The phenotypic plastic response of the wing determines the insect’s maneuverability, long-distance flight capabilities, and chances of escaping predators and finding food, which means that these attributes may be particularly susceptible to evolutionary changes (Chursina and Negrobov, 2018). Recently, several studies have demonstrated that insect wing shape is modulated by a wide range of variables, including ecology (Chazot et al., 2015), behavioral patterns (Johansson et al., 2009), and the geographic position of their habitats (Hoffmann and Shirriffs, 2002). It is critical to determine the morphometric plasticity of wing properties while investigating the evolutionary patterns of a species. Furthermore, from the perspective of systematics, assessing the influence of convergent evolution is necessary, because homologous wing shapes may arise not in closely related species but in those occupying similar habitats (Chursina and Negrobov, 2018). Recently, many attempts have been made to assess how much the morphometric similarity of wings in various species is due to their shared origin (Neustupa and Skaloud, 2007; Klingenberg and Gidaszewski, 2010; Chazot et al., 2015); but no definitive solution has been established. In our investigation, A. flavescens and A. punctatipennis have similar geographical distribution patterns; however, trait-based morphospace analysis and phylogenetic signal analysis proved that A. limbatus and A. flavescens shared similar trait-morphospace features than A. punctatipennis (Supplementary file S1; Supplementary Figures S1–S3). Insect wing venation pattern, size, and shape are strongly conserved in insect taxa, so these features have a vital role in phylogenetic analysis (Klingenberg and Gidaszewski, 2010; Bai et al., 2011; Perrard et al., 2014). Venation divergence is characterized as the behavioral adaptation in Vespidae, i.e., as a nocturnal or diurnal habit (Perrard et al., 2014). Finally, we can explain that the wing represents a basic functional unit, while its venation has a functional importance (e.g., Mountcastle and Combes, 2013).
The evolutionary mechanisms and processes underlying the diversification of wing shape continue to be the source of unresolved questions for evolutionary biologists (Le Roy et al., 2019). In addition to the miniaturization process, both natural and sexual selection could lead to the evolution of wing shape via their involvement in migration and reproduction (Stary, 1970). However, no research has been conducted to determine the phylogenetic relationship between the size and shape of the potter wasp wings. It is hypothesized that if there is no character displacement, homologous features of closely related species will be more identical to each other than the distantly related species. Hence, if the Antodynerus species’ wing size and shape follow this pattern, there should be a strong phylogenetic signal in these morphological attributes. In GM, phylogenetic signals have been used to investigate whether the size and shape of morphological structures of taxa originated as a result of shared evolutionary history or as a result of environmental features (Sidlauskas, 2008; Klingenberg and Gidaszewski, 2010; Monteiro, 2013). Phylogenetic signals suggest phenotypic similarities across phylogenetically related species are due to a shared genetic basis and developmental programs inherited from a common ancestor. The phylomorphospace is a useful approach because it allows us to track the history of a clade’s morphological diversification and infer the magnitude and direction of shape change along phylogenetic branches (Sidlauskas, 2008; Klingenberg and Gidaszewski, 2010; Monteiro, 2013). As a result, if a phylogenetic signal in wing shape/size is absent or weak, closely related species tend to be separated in phylomorphometric space (Sidlauskas, 2008; Klingenberg and Gidaszewski, 2010; Monteiro, 2013). Perrard et al. (2016) studied the morphological (GM-based) and molecular phylogenetic analysis of social wasps, revealing the shape phylogeny of 50 Vespinae species. Based on this study, it could be concluded that unstable relationships among genera indicated that fast radiations occurred in Vespinae’s early history. However, in our phylogenetic signal analysis, we found that shape-related phylogeny was observed in the forewing of Antodynerus species and that there is no phylogenetic signal in forewing size, hindwing size, and shape. In contrast to A. punctatipennis and A. flavescens, which were distributed in low-altitude geographical areas (Supplementary Figures S1, S2), A. limbatus distribution was mainly recorded in high-altitude geographical areas (Himalayan regions; Supplementary Figure S3; Girish Kumar and Carpenter, 2013). One of the most notable findings in GM analysis is that A. limbatus and A. flavescens shared a common hypothetical ancestor; however, they were distributed in distinct geographical regions and had a distinct morphospace when compared to A. punctatipennis. This phylogenetic analysis proved that the shape of the forewing is evolutionarily highly constrained and has a high level of heritability in nature, while the size of the forewing is not evolutionarily constrained and is highly influenced by natural selection pressure. In contrast to the forewing, the shape and size of the hindwings are independently evolved based on the selection pressure (i.e., environmental conditional selective pressure). Our findings confirmed that, for the morphometric evolutionarily analysis, the hindwing can be used as a prominent marker over the forewing. Despite the fact that we used a low number of shape variables (nine landmarks) in hindwings, the three species of Antodynerus occupied a distinct phylomorphospace. Phylogenetic relationships among the major lineages of Eumeninae have been poorly investigated (Hermes et al., 2013). Schmitz and Moritz (1998) studied the molecular phylogeny and evolution of social wasps, using mitochondrial and nuclear molecular markers. From this analysis, the authors proved that solitary Eumeninae was a sister taxon to the Polistinae + Vespinae cluster, and the phylogenetic analysis proved that sociality has independently evolved twice in the Vespidae. Further morphological phylogenetic analysis is required to comprehend the morphological evolution of solitary vs. social Vespidae.
Phylogenetic signal analysis is very rare in hymenopteran groups, so this is the first study report on Eumeninae phylogenetic signal analysis. dos Santos et al. (2019) used the GM to decipher the taxonomic relationship and phylogenetic signal of six diapausing stingless bee species (Plebeia) occurring in southern Brazil. The authors discovered no phylogenetic signal in forewing centroid size, as well as the existence of a significantly strong phylogenetic signal in the forewing shape of Plebeia species. On the contrary, the strong phylogenetic signal in the forewing suggests that sister species like P. nigriceps and P. wittmanni share the same hypothetical ancestor which may be due to sharing the same evolutionary history rather than environmental influences. As support to this finding, a shape-related strong phylogenetic signal (p < 0.05) was observed in the forewing of Antodynerus species, which suggested to us that the A. limbatus and A. flavescens share a similar evolutionary history rather than environmental conditions. This might be the reason for these two species’ divergence from the same hypothetical ancestor; however, these species are distributed in the high- and low-altitude geographical area of India. Our findings were corroborated by the research of Mitrovski-Bogdanović et al. (2021) who studied the molecular and morphological similarities and differences across the European species of the genus Aphidius Nees. A statistically significant shape phylogenetic signal was observed among the Aphidius species forewing. Nonetheless, there is no phylogenetic signal in Aphidius species forewing size. These results confirmed that, in parasitoid wasps (Braconidae, Aphidiinae), the forewing shape is phylogenetically well constrained than the wing size. Based on our findings, we propose that if we want to track the evolutionary history of hymenopteran taxa, we should focus on the shape phylomorphospace analysis of the forewing, and if we want to focus on natural selection adaptive response, we should use the hindwing as the prominent marker.
The data processing and the mathematical algorithm used in phylomorphospace and cluster dendrogram analysis are completely different; phylomorphospace is based on the Browninan motion model with a known topology (see Perrard et al., 2016), and cluster analysis is based on the Mahalanobis (D2) distance (see Cooke and Terhune, 2014). According to Cooke and Terhune (2014), Mahalanobis distance-based cluster dendrogram showed a high level of resemblance with the molecular phylogenetic tree. However, we cannot precisely determine which is the perfect cladogram that represents the molecular phylogeny or evolutionary feature of Antodynerus species; because our cladogram construction is based on forewings and hindwing traits, and each wing represented a distinct type of shape and size variables, eventually the output also different. In our forewing dendrogram analysis, A. limbatus is in a completely separated clade from A. flavescens and A. punctatipennis (sharing 60% of similar traits). However, in hindwing analysis, A. punctatipennis is occupied in a distinct clade compared to A. flavescens and A. limbatus (sharing 40% of similar traits). Nonetheless, the hindwing dendrogram exactly matches the shape and size phylogeny of Antodynerus species. Currently, no one has investigated the molecular phylogenetics analysis of Antodynerus species. So, multigene molecular marker-based phylogenetic analysis is required for understanding the evolution of Antodynerus species. Besides, more research is needed to fully comprehend the significance of environmental and evolutionary modulatory character and their impact on phylomorphospace and cladogram analysis.
Wing allometry
Closely related species may exhibit comparable allometry traits; however, this is not always true in various taxa due to their complex morphological diversity, ecology, and niche preference (Dujardin et al., 2003). In contrast to the size and shape of the hindwings, Belyaev and Farisenkov (2018) indicated that the size and shape of the forewing are correlated with the body size of hymenopteran insects. These kinds of incredible variations were observed in the Antodynerus species. In the allometry analysis, significant (p < 0.05) shape-related size variations were observed only in A. flavescens female left forewings and right hindwings; A. limbatus female left forewings and right hindwings, and male left hindwings and right forewings and hindwings; and A. punctatipennis female left and right forewings, and male left forewings and right hindwings. The shape and size allometry correlation of other wings in each species of Antodynerus was insignificant. We suspected that this kind of allometry may play important role in the evolution and the diversification of Antodynerus species. Diversification in body shape, or the shape of any particular morphological structure, may originate from allometric shape changes as well as from unrelated size related to shape variations (Bookstein, 1991; Klingenberg, 1996). Empirical studies proved that allometry evolved differently in different environmental conditions (Shingleton et al., 2009). Except for A. limbatus, the other two species share similar environmental conditions, but the allometry is completely distinct, i.e., each species has its own distinct allometry. One of the most critical aerodynamic characteristic features of the wing is the length-to-width ratio. The length and width of the wing frequently increase during the adaptation process, because a greater wing surface is conducive to saving more energy during flight (McLachlan, 1986; Sivinski and Dodson, 1992). Long narrow, apically pointed wings increase flight speed (Coombes and Daniel, 2001) and may be an adaptation to long-distance flights (Moore, 1990; Johansson et al., 2009). On the contrary, shorter and more rounded wings are more efficient for short flights requiring greater maneuverability (Chazot et al., 2015).
Geometric morphometrics in species delimitation
Uncertainties in the tribal and generic classification of the Eumeninae are primarily due to the morphological complexity of the taxa (Carpenter and Cumming, 1985; Carpenter and Garcete-Barrett, 2002; Hermes et al., 2013), so the use of GM will help to unravel the morphological complexity of the Eumeninae and it will help in systematic and phylomorphological classification of potter wasps. The use of GM in insect morphological research has increased in recent years, and it is important to note that GM is based on geometric Cartesian coordinates rather than linear measurements (Tatsuta et al., 2018). GM approaches have been successfully applied in evolutionary biology, physical anthropology, palaeontology, and systematics (Corti et al., 1998; Marcus, 1998; Monteiro et al., 2002; Dujardin et al., 2003; Friess and Baylac, 2003; Pretorius, 2005; Shipunov and Bateman, 2005). This technique is powerful enough to solve the complex issue in taxonomy, especially in species-level taxonomic classification (Martias et al., 2001; Gumiel et al., 2003; Shipunov and Bateman, 2005). An intriguing example of GM is used on the wing venation to successfully discriminate between two braconid species that were not clearly distinguished by the classical morphological approach (Baylac et al., 2003). We successfully discriminated three Antodynerus species from the Indian subcontinent in a trait-based morphospace. Each species has a distinct wing shape, size, vein junction, and vein length, so the length and dimension of each vein junction and the angle between the vein junctions could be used as taxonomic features to distinguish the Antodynerus species. The use of GM highlighted significant differences in the wing shape of the Antodynerus species. This insight opens the door to further research into the environmental, developmental, behavioral, and genetic factors contributing to such disparities (Villemant et al., 2007). The landmarks (used in our study to distinguish potter wasps) that successfully differentiated the closely related taxonomic group (i.e., congeneric species) could be incorporated into dichotomous keys to help in the identification of clades of complex resolution (dos Santos et al., 2019).
Data availability statement
The original contributions presented in the study are included in the article/Supplementary material, further inquiries can be directed to the corresponding authors.
Author contributions
PA and YS: conceptualization, conceived, and designed the experiment, software analysis. PG: data collection. SS: digitalization and landmarking of specimens. PA, YS, SS, and PG: analyzation and interpretation of the data. PA: wrote the main manuscript. YS: drawing and photographic plate preparation and supervision. PG and YS: reviewing and editing the manuscript. All authors contributed to the article and approved the submitted version.
Funding
This study was financially supported by UGC-SAP (F.3-6/2012 (SAP-II) dated 10.10.2012).
Acknowledgments
The authors are grateful to the authorities at the Department of Zoology, the University of Calicut for providing an infrastructural facility. PG is grateful to the Director (Zoological Survey of India, Kolkata, India) and P. M. Sureshan, Officer-in-Charge (Western Ghat Regional Centre, Zoological Survey of India, Kozhikode, India) for providing facilities and encouragement.
Conflict of interest
The authors declare that the research was conducted in the absence of any commercial or financial relationships that could be construed as a potential conflict of interest.
Publisher’s note
All claims expressed in this article are solely those of the authors and do not necessarily represent those of their affiliated organizations, or those of the publisher, the editors and the reviewers. Any product that may be evaluated in this article, or claim that may be made by its manufacturer, is not guaranteed or endorsed by the publisher.
Supplementary material
The Supplementary material for this article can be found online at: https://www.frontiersin.org/articles/10.3389/fevo.2022.965577/full#supplementary-material
References
Adams, D. C., Slice, D. E., and Rohlf, F. J. (2004). Geometric morphometrics: ten years of progress following the ‘revolution’. Italian J. Zool. 71, 5–16. doi: 10.1080/11250000409356545
Anand, P. P., Seena, S., Peter, J., and Shibu Vardhanan, Y. (2022). Detection of geographical specific plasticity and the effect of natural selection pressure on the wing size and shape of Bactrocera dorsalis (Diptera: Tephritidae). Biologia 77, 1347–1371. doi: 10.1007/s11756-022-01059-x
Aytekin, A. M., Terzo, M., Rasmont, P., and Cagatay, N. (2007). Landmark based geometric morphometric analysis of wing shape in Sibiricobombus Vogt (Hymenoptera: Apidae: Bombus Laterille). Ann. Soc. Entomol. Fr. 43:95. doi: 10.1080/00379271.2007.10697499
Bai, M., Mc Cullough, E., Song, K. Q., Liu, W. G., and Yang, X. K. (2011). Evolutionary constraints in hindwing shape in Chinese dung beetles (coleoptera: Scarabaeinae). PLoS One 6:e21600. doi: 10.1371/journal.pone.0021600
Baracchi, D., Dapporto, L., and Turillazzi, S. (2011). Relevance of wing morphology in distinguishing and classifying general and species of Stenogastrinae wasps. Contrib. Zool. 80, 191–199. doi: 10.1163/18759866-08003003
Baylac, M., and Daufresne, T. (1996). “Wing venation variability in Monarthropalpus buxi (Diptera, Cecidomyiidae) and the quaternary coevolution of box (Buxus sempervirens L.) and its midge: a geometrical morphometrical analysis” in Advances in morphometrics. ed. L. Marcus (New York, NY: Plenum Press), 285–302.
Baylac, M., Villemant, C., and Simbolotti, G. (2003). Combining geometric morphometrics with pattern recognition for the investigation of species complexes. Biol. J. Linn. Soc. 80, 89–98. doi: 10.1046/j.1095-8312.2003.00221.x
Belyaev, O. A., and Farisenkov, S. E. (2018). A study on allometry of wing shape and venation in insects. Part 1. Hymenoptera. Moscow Univ. Biol. Sci. Bull. 73, 229–235. doi: 10.3103/S0096392518040028
Benitez, H. A., Bravi, R., Parra, L. E., Sanzana, M. J., and Sepulveda-Zuniga, E. (2013). Allometric and non-allometric pattern in sexual dimorphism discrimination of wing shape in the parasitoid, Ophion intricatusm: do two male morphotype coexist? J. Insect Sci. 13, 1–10. doi: 10.1673/031.013.14301
Benitez, H., Parra, L. E., Sepulveda, E., and Sanzana, M. J. (2011). Geometric perspectives of sexual dimorphism in the wing shape of lepidoptera: the case of Synneuria sp. (lepidoptera: Geometridae). J. Entomol. Res. Soc. 13, 53–60.
Benitez, H., Vidal, M., Briones, R., and Jerez, V. (2010). Sexual dimorphism variation of Ceroglossus chilensis (Eschscholtz) (coleoptera, Carabidae). J. Entomol. Res. Soc. 12, 87–95.
Berwaerts, K., Dyck, H., and Aerts, P. (2002). Does flight morphology relate to flight performance? An experimental test with the butterfly Pararge aegeria. Funct. Ecol. 16, 484–491. doi: 10.1046/j.1365-2435.2002.00650.x
Bickford, D., Lohman, D. J., Sodhi, N. S., Ng, P. K., Meier, R., Winker, K., et al. (2007). Cryptic species as a window on diversity and conservation. Trends Ecol. Evol. 22, 148–155. doi: 10.1016/j.tree.2006.11.004
Bonduriansky, R. (2006). Convergent evolution of sexual shape dimorphism in Diptera. J. Morphol. 267, 602–611. doi: 10.1002/jmor.10426
Bookstein, F. L. (1991). Morphometrics tools for landmark data: Geometry and biology. Cambridge: Cambridge University Press.
Carpenter, J. M., and Cumming, J. M. (1985). A character analysis of the north American potter wasps (Hymenoptera: Vespidae: Eumeninae). J. Nat. Hist. 19, 877–916. doi: 10.1080/00222938500770551
Carpenter, J. M., and Garcete-Barrett, B. R. (2002). A key to the neotropical genera of Eumeninae (Hymenoptera: Vespidae). Boletin Del Museo Nacional de Historia Natural Del Paraguay 14, 52–73.
Carpenter, J. M., Gusenleitner, J., and Madl, M. (2009). A catalogue of the Eumeninae (Hymenoptera: Vespidae) of the Ethiopian region excluding Malagasy subregion. Part 1: introduction, key to genera, genera Aethiopicodynerus Gusenleitner 1997 to Cyrtolabulus van der Vecht 1969. Linzer Biologische Beitrage 41, 513–638.
Cepeda-Pizarro, J., Vasquez, H., Veas, H., and Colon, G. (1996). Relationship between size and body biomass in adults of steppe Tenebrionidae (coleoptera) coastal southern margin of the desert Chilean. Revista Chileana de Historia Natural 69, 67–76.
Chazot, N., Panara, S., Zilbermann, N., Blandin, P., Poul, Y. L., Cornette, R., et al. (2015). Morpho morphometrics; shared ancestry and selection drive the evolution of wing size and shape in morpho butterflies. Evolution 70, 181–194. doi: 10.1111/evo.12842
Chursina, M. A., and Negrobov, O. P. (2018). Phylogenetic signal in the wing shape in the subfamily Dolichopodinae (Diptera, Dolichopodidae). Entomol. Rev. 98, 515–527. doi: 10.1134/S0013873818050019
Comstock, J. H., and Needham, J. G. (1899). The wings of insects. Am. Nat. 33, 845–860. doi: 10.1086/277462
Cooke, S. B., and Terhune, C. E. (2014). Form, function and geometric morphometrics. Anat. Rec. 298, 5–28. doi: 10.1002/ar.23065
Coombes, S. A., and Daniel, T. L. (2001). Shape, flapping and flexion: wing and fin design for forward flight. J. Exp. Biol. 204, 2073–2085. doi: 10.1242/jeb.204.12.2073
Corti, M., Aguilera, M., and Capanna, E. (1998). Phylogeny and size and shape changes in the skull of the south American rodent Proechimys. Acta Zoologica Academiae Scientiarum Hungarica 44, 139–150.
Crowson, R. A. (1961). On some new characters of classificatory importance in adults of Elateridae (coleoptera). Ent. Mon. Mag. 96, 158–161.
Crowson, R. A. (1973). New superfamily Artematopoidea of Polyphagan beetles, with definition of 2 new fossil genera from Baltic amber. J. Nat. Hist. 7, 225–238.
De la Rua, P., Galian, J., Serrano, J., and Moritz, R. F. A. (2001). Genetic structure and distinctness of Apis mellifera L. populations from the Canary Islands. Mol. Ecol. 10, 1733–1742. doi: 10.1046/j.1365-294X.2001.01303.x
De Meulemeester, T., Michez, D., Aytekin, A. M., and Danforth, B. N. (2012). Taxonomic affinity of halictid bee fossils (Hymenoptera: Anthophila) based on geometric morphometrics analyses of wing shape. J. Syst.Palaeontol. 10, 755–764. doi: 10.1080/14772019.2011.628701
Dehon, M., Michez, D., Nel, A., Engel, M. S., and De Leulemeester, T. (2014). Wing shape of four new bee fossils (Hymenoptera: Anthophila) provides insights to bee evolution. PLoS One 9:e108865. doi: 10.1371/journal.pone.0108865
Dolin, V. G. (1975). Wing venation of click beetles (coleoptera, Elateridae) and its importance for taxonomy of the family. Zoologicheskii Zn 54, 1618–1633.
dos Santos, C. F., dos Santos, P. D. S., Marques, D. M., Da-Costa, T., and Blochtein, B. (2019). Geometric morphometrics of the forewing shape and size discriminate Plebeia species (Hymenoptera: Apidae) nesting in different substrates. Syst. Entomol. 44, 787–796. doi: 10.1111/syen.12354
Dujardin, J. P. (2008). Morphometrics applied to medical entomology. Infect. Genet. Evol. 8, 875–890. doi: 10.1016/j.meegid.2008.07.011
Dujardin, J. P., Le Pont, F., and Baylac, M. (2003). Geographical versus interspecific differentiation of sand flies (Diptera: Psychodidae): a landmark data analysis. Bull. Entomol. Res. 93, 87–90. doi: 10.1079/BER2002206
Escoufier, Y. (1973). Le traitement des variables vectorielles. Biometrics 29, 751–760. doi: 10.2307/2529140
Forbes, W. T. M. (1922). The wing venation of coleoptera. Ann. Ent. Soc. Amer. Columbus Ohio. 15, 328–345. doi: 10.1093/aesa/15.4.328
Forrest, T. (1987). Insect size tactics and developmental strategies. Oecologia 73, 178–184. doi: 10.1007/BF00377505
Francuski, L., Ludoški, J., Vujić, A., and Milankov, V. (2009). Wing geometric morphometric inferences on species delimitation and intraspecific divergent units in the Merodon ruficornis group (Diptera, Syrphidae) from the Balkan Peninsula. Zoolog. Sci. 26, 301–308. doi: 10.2108/zsj.26.301
Friess, M., and Baylac, M. (2003). Exploring artificial cranial deformation using elliptic fourier analysis of Procrustes aligned outlines. Am. J. Phys. Anthropol. 122, 11–22. doi: 10.1002/ajpa.10286
Fruciano, C. (2016). Measurement error in geometric morphometrics. Dev. Genes Evol. 226, 139–158. doi: 10.1007/s00427-016-0537-4
Gaston, K. J., and O’Neill, M. A. (2004). Automated species identification: why not? Philos. Trans. R. Soc. Lond. B Biol. Sci. 359, 655–667. doi: 10.1098/rstb.2003.1442
Gidaszewski, N. A., Baylac, M., and Klingenberg, C. P. (2009). Evolution of sexual dimorphism of wing shape in the Drosophila melanogaster subgroup. BMC Evol. Biol. 9:110. doi: 10.1186/1471-2148-9-110
Girish Kumar, P., and Carpenter, J. M. (2013). A taxonomic review of the genus Antodynerus de Saussure, 1855 (Hymenoptera; Vespidae: Eumeninae) from the Indian subcontinent. Zootaxa 3731, 267–278. doi: 10.11646/zootaxa.3731.2.7
Godfray, H. C. J. (1994). Parasitoids: Behavioral and evolutionary ecology. Princeton University Press, Princeton, NJ.
Grandinete, Y. C., Hermes, M. G., and Noll, F. B. (2015). Systematics and phylogeny of the neotropical Pachymenes de Saussure and Santamenes Giordani Soika (Hymenoptera, Vespidae, Eumeninae). Syst. Entoml. 40, 365–384. doi: 10.1111/syen.12105
Gumiel, M., Catala, S., Noireau, F., Rojas de Arias, A., Garcia, A., and Dujardin, J. P. (2003). Wing geometry in Triatoma infestans (Klug) and T. melanosome Martinez, Olmedo & Carcavallo (hemiptera: Reduviidae). Syst. Entomol. 28, 173–180. doi: 10.1046/j.1365-3113.2003.00206.x
Gusenleitner, J. (2010). Uber bemerkenswerte Faltenwespen aus der athiopischen Region Teil 6 (Hymenoptera: Vespidae: Eumeninae). Linzer Biologische Beitrage 42, 1323–1346.
Hermes, G. M., Melo, G. A. R., and Carpenter, J. M. (2013). The higher-level phylogenetic relationships of the Eumeninae (Insecta, Hymenoptera, Vespidae), with emphasis on Eumenes Sensu lato. Cladistics 30, 453–484. doi: 10.1111/cla.12059
Hines, H. M., Hunt, J. H., O’Connor, T. K., Gillespie, J. J., and Cameron, S. A. (2007). Multigene phylogeny reveals eusociality evolved twice in vespid wasps. PNAS 104, 3295–3299. doi: 10.1073/pnas.0610140104
Hoffmann, A. A., and Shirriffs, J. (2002). Geographic variation for wing shape in Drosophila serrata. Evolution 56, 1068–1073. doi: 10.1111/j.0014-3820.2002.tb01418.x
Johansson, F., Soderquist, M., and Bokma, F. (2009). Insect wing shape evolution: independent effects of migratory and mate guarding flight on dragonfly wings. Biol. J. Linn. Soc. 97, 362–372. doi: 10.1111/j.1095-8312.2009.01211.x
Jouault, C., and Nel, A. (2021). A new species of Prosyntexis Sharkey, 1990 (Hymenoptera: Sepulcidae) from the lower cretaceous Crato formation of Brazil confirmed by geometric morphometric analysis. Palaeoentomology 4, 171–177. doi: 10.11646/palaeoentomology.4.2.6
Karthika, K., Anand, P. P., Seena, S., and Shibu Vardhanan, Y. (2022). Wing phenotypic plasticity, quantitative genetics, modularity, and phylogenetic signal analysis revealed the niche portioning in two fruit fly species, Bactrocera dorsalis and Zeugodacus cucurbitae. Int. J. Trop. Insect. Sci. 42, 1487–1504. doi: 10.1007/s42690-021-00668-4
Kendall, D. G., Barden, D., Carne, T. K., and Le, H. (1999). Shape and shape theory. Chichester. John Wiley & Sons,.
Klingenberg, C. P. (1996). “Multivariate allometry” in Advances in morphometrics. eds. L. F. Marcus, M. Corti, A. Loy, G. J. P. Naylor, and D. E. Slice (New York: Plenum), 23–49.
Klingenberg, C. P. (2003). “Developmental instability as a research tool: using patterns of fluctuating asymmetry to infer the developmental origins of morphological integration” in Developmental instability, causes and consequences. ed. M. Polak (Oxford University press), 427–442.
Klingenberg, C. P. (2008). Novelty and “homology-free” morphometrics: whats’s in a name? iEvol. Biol. 2008, 186–190. doi: 10.1007/s11692-008-9029-4
Klingenberg, C. P. (2009). Morphometric integration and modularity in configurations of land-marks: tools for evaluating a priori hypothesis. Evol. Dev. 11, 405–421. doi: 10.1111/j.1525-142X.2009.00347.x
Klingenberg, C. P. (2011). Morpho J: an integrated software package for geometric morphometrics. Mol. Ecol. Resour. 11, 353–357. doi: 10.1111/j.1755-0998.2010.02924.x
Klingenberg, C. P. (2016). Size, shape, and form: concepts of allometry in geometric morphometrics. Dev. Genes Evol. 226, 113–137. doi: 10.1007/s00427-016-0539-2
Klingenberg, C. P., and Gidaszewski, N. A. (2010). Testing and quantifying phylogenetic signals and homoplasy in morphometric data. Syst. Biol. 59, 245–261. doi: 10.1093/sysbio/syp106
Klingenberg, C. P., and McIntyre, G. S. (1998). Geometric morphometric of developmental instability: analyzing patterns of fluctuating asymmetry with Procrustes methods. Evolution 52, 1363–1375. doi: 10.1111/j.1558-5646.1998.tb02018.x
Klingenberg, C. P., McIntyre, G. S., and Zaklan, S. D. (1998). Left-right asymmetry of fly wings and the evolution of body axes. Proc. R. Soc. B 265, 1255–1259. doi: 10.1098/rspb.1998.0427
Klingenberg, C. P., Mebus, K., and Auffray, J. C. (2003). Developmental integration in a complex morphological structure: how distinct are the modules in the mouse mandible? Evol. Dev. 5, 522–531. doi: 10.1046/j.1525-142X.2003.03057.x
Kos, K., Petrović, A., Starý, P., Kavallieratos, N. G., Ivanović, A., Toševski, I., et al. (2011). On the identity of cereal aphid parasitoid wasps Aphidius uzbekistanicus, A. rhopalosiphi and A. avenaphis (Hymenoptera: Braconidae: Aphidiinae) by examination of COI mitochondrial gene, geometric morphometrics and morphology. Am. Entomol. Soc. Am. 104, 1221–1232. doi: 10.1603/AN11055
Laurin, M. (2004). The evolution of body size, Cope’s rule and the origin of amniotes. Syst. Biol. 53, 594–622. doi: 10.1080/10635150490445706
Le Roy, C., Debat, V., and Llaurens, V. (2019). Adaptive evolution of butterfly wing shape: from morphology to behaviour. Biol. Rev. 94, 1261–1281. doi: 10.1111/brv.12500
Lemic, D., Benitez, H., and Bazok, R. (2014). Intercontinental effect on sexual shape dimorphism and allometric relationships in the beetle pest Diabrotica virgifera virgifera LeConte (coleoptera: Chrysomelidae). Zool. Anz. Comp. Zool. 253, 203–206. doi: 10.1016/j.jcz.2014.01.001
Maddison, W. P. (1991). Squared-change parsiomony reconstructions of ancestral states for continuous-valued characters on a phylogenetic tree. Syst. Zool. 40, 304–314. doi: 10.2307/2992324
Maddison, W. P., and Maddison, D. R. (2016). Mesquite a: modular system for evolutionary analysis. Version 3.10. Available at: http://mesquiteproject.org.
Mahima, K. V., Anand, P. P., Seena, S., Shameema, K., Manogem, E. M., and Shibu Vardhanan, Y. (2021). Caste-specific phenotypic plasticity of Asian weaver ants: revealing the allometric and non-allometric component of female caste system of Oecophylla smaragdina (Hymenoptera: Formicidae) by using geometric morphometrics. Sociobiology 68:e5941. doi: 10.13102/sociobiology.v68i2.5941
Marcus, L. F. (1998). Variation in selected skeletal elements of fossil remains of Myotragus balearicus, a Pleistocene bovid from Mallorca. Acta Zool. Acad. Sci. Hungarica 44, 113–137.
Marsteller, S., Adams, D. C., Collyer, M. L., and Condon, M. (2009). Six cryptic species on a single species of host plant: morphometric evidence for possible reproductive character displacement. Ecol. Entomol. 34, 66–73. doi: 10.1111/j.1365-2311.2008.01047.x
Martias, A., de la Riva, J. X., Torrez, M., and Dujardin, J. P. (2001). Rhodnius robustus in Bolivia identified by its wings. Mem. Inst. Oswaldo Cruz 96, 974–950. doi: 10.1590/s0074-02762001000700010
McLachlan, A. J. (1986). Sexual dimorphism in midges: strategies for flight in the rain pool dweller Chironomus imicola (Diptera: Chironmidae). J. Anim. Ecol. 55, 261–267. doi: 10.2307/4706
Meier, R., Shiyang, K., Vaidya, G., and Ng, P. K. (2006). DNA barcoding and taxonomy of Diptera: a tale of high intraspecific variability and low identification success. Syt. Biol. 55, 715–728. doi: 10.1080/10635150600969864
Mitrovski Bogdanović, A., Ivanović, A., Tomanović, Ž., Žikić, V., Starý, P., and Kavallieratos, N. G. (2009). Sexual dimorphism in Ephedrus persicae (Hymenoptera: Braconidae: Aphidiinae): intraspecific variation in size and shape. Can. Entomol. 141, 550–560. doi: 10.4039/n09-029
Mitrovski-Bogdanović, A., Mitrović, M., Milosević, M. I., Žikić, V., Jamhour, A., Ivanović, A., et al. (2021). Molecular and morphological variation among the European species of the genus Aphidius Nees (Hymenoptera: Braconidae: Aphidiinae). Organ. Div. Evol. 21, 421–436. doi: 10.1007/s13127-021-00489-w
Mitrovski-Bogdanovic, A., Petrovic, A., Mitrovic, M., Ivanović, A., Zikic, V., Stary, P., et al. (2013). Identification of two cryptic species within the Praon abjectum group (Hymenoptera: Braconidae: Aphidiinae) using molecular markers and geometric morphometrics. Ann. Entool. Soc. Am. 106, 170–180. doi: 10.1603/AN12100
Moller, A., and Zamora-Munoz, C. (1997). Antennal asymmetry and sexual selection in a cerambycid beetle. Anim. Behav. 54, 1509–1515. doi: 10.1006/anbe.1997.0565
Monteiro, I. R. (1999). Multivariate regression models and geometric morphometrics: the search for casual factors in the analysis of shape. Syst. Biol. 48, 192–199. doi: 10.1080/106351599260526
Monteiro, L. R. (2013). Morphometrics and the comparative method: studying the evolution of biological shape. Hystrix 24, 25–32. doi: 10.4404/hystrix-24.1-6282
Monteiro, L. R., Diniz-Filho, J. A. F., Dos Resis, S. F., and Araujo, E. D. (2002). Geometric estimates of heritability in biological shape. Evolution 56, 563–572. doi: 10.1554/0014-3820(2002)056[0563:GEOHIB]2.0.CO;2
Moore, A. J. (1990). The evolution of sexual dimorphism by sexual selection; the separate effects of intrasexual selection and intersexual selection. Evolution 44, 315–331. doi: 10.1111/j.1558-5646.1990.tb05201.x
Moraes, E. M., Manfrin, M. H., Laus, A. C., Rosada, R. S., Bomfin, S. C., and Sene, F. M. (2004). Wing shape heritability and morphological divergence of the sibling species Drosophila mercatorum and Drosophila paranaensis. Heredity 92, 466–473. doi: 10.1038/sj.hdy.6800442
Moraes, E. M., Spressola, V. L., Prado, P. R. R., Costa, L. F., and Sene, F. M. (2004). Divergence in wing morphology among sibling species of the drosophila buzzattii cluster. J. Zoo. Syst. Evol. Res. 42:154158, 154–158. doi: 10.1111/j.1439-0469.2004.00256.x
Mountcastle, A. M., and Combes, S. A. (2013). Wing flexibility enhances laod-lifting capacity in bumblebees. Proc. R. Soc. B 280:20130531. doi: 10.1098/rspb.2013.0531
Munoz, F. M., Carreira, V. P., Abadias, N. M., Ortiz, V., Jose, R. G., and Soto, I. M. (2016). Drosophila wing modularity revisited through a quantitative genetic approach. Evolution 70, 1530–1541. doi: 10.1111/evo.12975
Neustupa, J., and Skaloud, P. (2007). Geometric morphometrics and quantitative patterns in the morphological variation of five species of Micrasterias (Zygnemophyceae, Viridiplantae). Preslia 79, 401–417.
Outomuro, D., Adams, D. C., and Johansson, F. (2013). The evolution of wing shape in ornamented-winged damselflies (Calopterygidae, Odonata). Evol. Biol. 40, 300–309. doi: 10.1007/s11692-012-9214-3
Palmer, A. R. (1994). “Fluctuating asymmetry analysis: a primer” in Development instability: Its origins and evolutionary implications. ed. T. A. Markow (Academic Publishers, Dordrecht: Kluwer), 335–364.
Palmer, A. R., and Strobeck, C. (1986). Fluctuating asymmetry: measurement, analysis, patterns. Annu. Rev. Ecol. Syst. 17, 391–421. doi: 10.1146/annurev.es.17.110186.002135
Pan, P. L., Shen, Z. R., Gao, L. W., and Yang, H. Z. (2008). Development of the technology for auto-extracting venation of insects. Entomotaxonomia 24, 8–14. doi: 10.1016/S1005-9040(08)60003-3
Pannure, A., Belavadi, V. V., and Carpenter, J. M. (2016). Taxonomic studies on potter wasps (Hymenoptera: Vespidae: Eumeninae) of South India. Zootaxa 4171, 1–050. doi: 10.11646/zootaxa.4171.1.1
Pelabon, C., Firmat, C., Bolstad, H. G., Voje, L. K., Houle, D., Cassara, J., et al. (2014). Evolution of morphological allometry. Ann. New York Acad. Sci. 1320, 58–75. doi: 10.1111/nyas.12470
Perrard, A. (2019). Wasp waist and flight: convergent evolution in wasps reveals a link between wings and body shapes. Am. Nat. 195, 181–191. doi: 10.1086/706914
Perrard, A., Baylac, M., Carpenter, J. M., and Villemant, C. (2014). Evolution of wing shape in hornets: why is the wing venation efficient for species identification? J. Evol. Biol. 27, 2665–2675. doi: 10.1111/jeb.12523
Perrard, A., Grimaldi, D., and Carpenter, J. M. (2017). Early lineages of Vespidae (Hymenoptera) in cretaceous amber. Syst. Entomol. 42, 379–386. doi: 10.1111/syen.12222
Perrard, A., Lopez-Osorio, F., and Carpenter, J. M. (2016). Phylogeny, landmark analysis and the use of wing venation to study the evolution of social wasps (Hymenoptera: Vespidae: Vespinae). Cladistics 32, 406–425. doi: 10.1111/cla.12138
Perrard, A., Villemant, C., Carpenter, J. M., and Baylac, M. (2012). Differences in caste dimorphism among three hornet species (Hymenoptera: Vespidae); forewing size, shape and allometry. J. Evol. Biol. 25, 1389–1398. doi: 10.1111/j.1420-9101.2012.02527.x
Pickett, K. M., and Carpenter, J. M. (2010). Simultaneous analysis and the origin of eusociality in the Vespidae (Insecta; Hymenoptera). Arthropod Syst. Phylo. 68, 3–33.
Pretorius, E. (2005). Using geometric morphometrics to investigate wing dimorphism in males and females of Hyemnoptera – a case study based on the genus Tachysphex kohl (Hymenoptera; Sphecidae: Larrinae). Australian J. Ento. 44, 113–121. doi: 10.1111/j.1440-6055.2005.00464.x
Reeve, J., and Fairbairn, D. (1999). Changes in sexual size dimorphism as a correlated response to selection on fecundity. Heredity 83, 697–706. doi: 10.1046/j.1365-2540.1999.00616.x
Richards, M. A. (1927). Sexual selection and applied problems in the insects. Biol. Rev. 2, 298–364. doi: 10.1111/j.1469-185X.1927.tb01401.x
Rohlf, F. J. (2001). Comparative methods for the analysis of continuous variables: geometric interpretations. Evolution 55, 2143–2160. doi: 10.1111/j.0014-3820.2001.tb00731.x
Rohlf, F. J. (2003). Tps small: Calculation of shape variation, Version 1.34. Stony Brook, NY: Department of Ecology and Evolution, State University of New York at Stony Brook.
Rohlf, F. J. (2015). The tps series of software. Hystrix. Ital. J. Mammal. 26, 9–12. doi: 10.4404/hystrix-26.1-11264
Rohlf, F. J., and Marcus, L. F. (1993). A revolution in morphometrics. Trends Ecol. Evol. 8, 129–132. doi: 10.1016/0169-5347(93)90024-J
Rohlf, F. J., and Slice, D. (1990). Extensions of Procrustes method for the optimal superimposition of landmarks. Syst. Biol. 39, 40–59. doi: 10.2307/2992207
Schmitz, J., and Moritz, R. F. A. (1998). Molecular phylogeny of Vespidae (Hymenoptera) and the evolution of sociality in wasps. Mol. Phylogenet. Evol. 9, 183–191. doi: 10.1006/mpev.1997.0460
Scholtz, C. H. (1990). Phylogenetic trends in the Scarabaeoidea. J. Nat. Hist. 24, 1027–1066. doi: 10.1080/00222939000770631
Schwarzfeld, M. D., and Sperling, F. A. H. (2014). Species delimitation using morphology, morphometrics, and molecules: definition of the Ophion scutellaris Thomson species group, with descriptions of six new species (hymenopter, Ichneumonidae). Zookeys 462, 56–114. doi: 10.3897/zookeys.462.8229
Shih, P. J. M., Li, L., Li, D., and Ren, D. (2020). Application of geometric morphometric analyses to confirm three new wasps of Evaniidae (Hymenoptera: Evanioidae) from mid-cretaceous Myanmar amber. Cretac. Res. 109:104249. doi: 10.1016/j.cretres.2019.104249
Shingleton, W. A., Estep, C. M., Driscoll, M. V., and Dworkin, I. (2009). Many ways to be small: different environmental regulators of size generate distinct scaling relationships in Drosophila melanogaster. Proc. R. Soc. B Biol. Sci. 276, 2625–2633. doi: 10.1098/rspb.2008.1796
Shipunov, A. B., and Bateman, R. M. (2005). Geometric morphometrics as a tool for understanding Dactylorhiza (Orchidaceae) diversity in European Russia. Biol. J. Linn. Soc. 85, 1–12. doi: 10.1111/j.1095-8312.2005.00468.x
Shreeves, G., and Field, J. (2008). Parental care and sexual size dimorphism in wasps and bees. Behav. Ecol. Sociobiol. 62, 843–852. doi: 10.1007/s00265-007-0510-3
Sidlauskas, B. (2008). Continuous and arrested morphological diversification in sister clades of characiform fishes: a phylomorphospace approach. Evolution 62, 3135–3156. doi: 10.1111/j.1558-5646.2008.00519.x
Sivinski, J. M., and Dodson, G. (1992). Sexual dimorphism in Anastrepha suspense (Loew) and other Tephritid fruit flies (Diptera: Tephritidae): possible roles of developmental rate, fecundity, and dispersal. J. Insect Behav. 5, 491–506. doi: 10.1007/BF01058194
Stary, P. (1970). Biology of aphid parasites (Hymenoptera: Aphididae) with respect to integrated control. Series entomological. (Vol 6). The Netherlands: Junk Publishers, Hague.
Suzuki, T. K. (2013). Modularity of a leaf moth-wing pattern and a versatile characteristics of wing pattern ground plan. BMC Evol. Biol. 13:158. doi: 10.1186/1471-2148-13-158
Svensson, G., and Petersson, E. (1987). Sex role reversed courtship behavior, sexual dimorphism and nuptial gifts in the dance fly, Empis borealis (L.). Ann. Zool. Fenn. 24, 323–334.
Tatsuta, H., Takahashi, K. H., and Sakamaki, Y. (2018). Geometric morphometric in entomology: basics and applications. Entomol. Sci. 21, 164–184. doi: 10.1111/ens.12293
Thornhill, R. (1992). Fluctuating asymmetry and the mating system of the Japanese scorpion fly, Panorpa Japonica. Am. Behav. 44, 867–879. doi: 10.1016/s0003-3472(05)80583-4
Tofilski, A. (2008). Using geometric morphometrics and standard morphometry to discriminate three honeybee subspecies. Apidologie 39, 558–563. doi: 10.1051/apido:2008037
Tomanović, Ž., Kos, K., Petrović, A., Straý, P., Kavallieratos, N. G., Žikić, V., et al. (2013). The relationship between molecular variation and variation in the wing shape of three aphid parasitoid species: Aphidius uzbekistanicus Luzhetzki, Aphidius rhopalosiphi De Stefani Perez and Aphidius avenaphis (fitch) (Hymenoptera: Braconidae: Aphidiinae). Zool. Anz. 252, 41–47. doi: 10.1016/j.jcz.2012.03.003
Tuzun, A. (2009). Significance of wing morphometry in distinguishing some of the Hymenoptera species. African J. Biotech. 8, 3353–3363.
Villemant, C., Simbolotti, G., and Kenis, M. (2007). Discrimination of Eubazus (Hymenoptera, Braconidae) sibling species using geometric morphometrics analysis of wing venation. Syst. Entomol. 32, 625–634. doi: 10.1111/j.1365-3113.2007.00389.x
Wagner, G. P., Pavlicev, M., and Cheverud, J. M. (2007). The road to modularity. Nat. Rev. Genet. 8, 921–931. doi: 10.1038/nrg2267
Wappler, T., Meulemeester, T., Aytekin, A. M., Michez, D., and Engel, M. S. (2012). Geometric morphometric analysis of a new Miocene bumble bee from the Randeck maar of southwestern Germany (Hymenoptera: Apidae). Syst. Entomol. 37, 784–792. doi: 10.1111/j.1365-3113.2012.00642.x
Yamane, S. K. (1990). A revision of the Japanese Eumenidae (Hymenoptera, Vespoidea). Insecta mastsumurana. Series Entomol. New Series 43, 1–189.
Žikić, V., Stanković, S. S., Petrović, A., Milošević, M., Tomanović, Ž., Klingenberg, C. P., et al. (2017). Evolutionary relationship of wing venation and wing size and shape in Aphidiinae (Hymenoptera: Braconidae). Org. Divers. Evol. 17, 607–617. doi: 10.1007/s13127-017-0338-2
Keywords: Antodynerus , geometric morphometrics, phylomorphospace, phylogenetic signal, solitary wasps, trait-morphospace
Citation: Anand PP, Seena S, Girish Kumar P and Shibu Vardhanan Y (2023) Species morphospace boundary revisited through wing phenotypic variations of Antodynerus species (Hymenoptera: Vespidae: Eumeninae) from the Indian subcontinent. Front. Ecol. Evol. 10:965577. doi: 10.3389/fevo.2022.965577
Edited by:
Renata Bažok, University of Zagreb, CroatiaReviewed by:
Jiufeng Wei, Shanxi Agricultural University, ChinaCintia Akemi Oi, University College London, United Kingdom
Darija Lemić, University of Zagreb, Croatia
Copyright © 2023 Anand, Seena, Girish Kumar and Shibu Vardhanan. This is an open-access article distributed under the terms of the Creative Commons Attribution License (CC BY). The use, distribution or reproduction in other forums is permitted, provided the original author(s) and the copyright owner(s) are credited and that the original publication in this journal is cited, in accordance with accepted academic practice. No use, distribution or reproduction is permitted which does not comply with these terms.
*Correspondence: P. P. Anand, ✉ YW5hbmRwcDYzM0BnbWFpbC5jb20=; Y. Shibu Vardhanan, ✉ c3ZhcmRoYW5hbkBnbWFpbC5jb20=
†ORCID: P. P. Anand, https://orcid.org/0000-0002-2400-2823
P. Girish Kumar, https://orcid.org/0000-0003-2121-0165
Y. Shibu Vardhanan, https://orcid.org/0000-0001-9820-886X