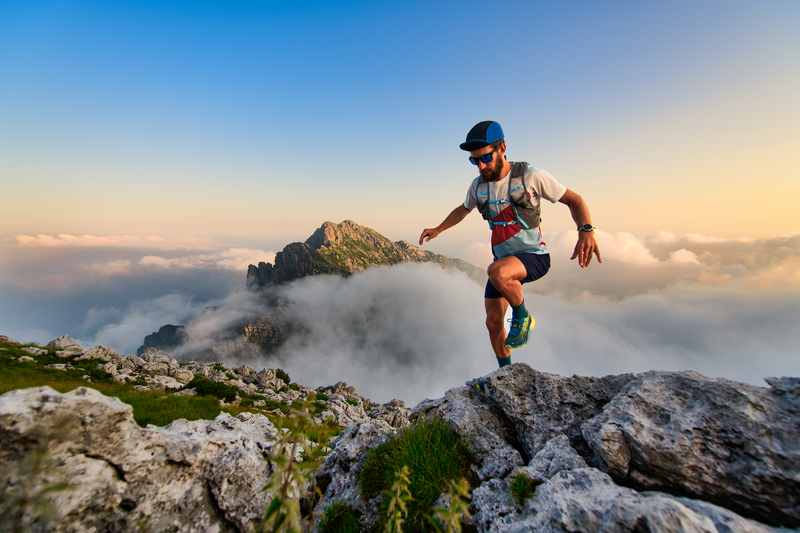
95% of researchers rate our articles as excellent or good
Learn more about the work of our research integrity team to safeguard the quality of each article we publish.
Find out more
ORIGINAL RESEARCH article
Front. Ecol. Evol. , 08 August 2022
Sec. Chemical Ecology
Volume 10 - 2022 | https://doi.org/10.3389/fevo.2022.960850
This article is part of the Research Topic Women in Chemical Ecology 2022 View all 8 articles
A correction has been applied to this article in:
Corrigendum: Development of a polyphagous leaf beetle on different host plant species and its detoxification of glucosinolates
Herbivores face a broad range of defences when feeding on plants. By mixing diets, polyphagous herbivores are assumed to benefit during their development by gaining a better nutritional balance and reducing the intake of toxic compounds from individual plant species. Nevertheless, they also show strategies to metabolically cope with plant defences. In this study, we investigated the development of the polyphagous tansy leaf beetle, Galeruca tanaceti (Coleoptera: Chrysomelidae), on mono diets consisting of one plant species [cabbage (Brassica rapa), Brassicaceae; lettuce (Lactuca sativa), or tansy (Tanacetum vulgare), Asteraceae] vs. two mixed diets, both containing tansy. Leaves of the three species were analysed for contents of water, carbon and nitrogen, the specific leaf area (SLA) and trichome density. Furthermore, we studied the insect metabolism of two glucosinolates, characteristic defences of Brassicaceae. Individuals reared on cabbage mono diet developed fastest and showed the highest survival, while the development was slowest for individuals kept on tansy mono diet. Lettuce had the highest water content and SLA but the lowest C/N ratio and no trichomes. In contrast, tansy had the lowest water content and SLA but the highest C/N ratio and trichome density. Cabbage was intermediate in these traits. Analysis of insect samples with UHPLC-DAD-QTOF-MS/MS revealed that benzyl glucosinolate was metabolised to N-benzoylglycine, N-benzoylalanine and N-benzoylserine. MALDI-Orbitrap-MS imaging revealed the localisation of these metabolites in the larval hindgut region. 4-Hydroxybenzyl glucosinolate was metabolised to N-(4-hydroxybenzoyl)glycine. Our results highlight that G. tanaceti deals with toxic hydrolysis products of glucosinolates by conjugation with different amino acids, which may enable this species to develop well on cabbage. The high trichome density and/or specific plant chemistry may lower the accessibility and/or digestibility of tansy leaves, leading to a poorer beetle development on pure tansy diet or diet mixes containing tansy. Thus, diet mixing is not necessarily beneficial, if one of the plant species is strongly defended.
Herbivores are confronted with various challenges when feeding on plants. Morphological structures such as spines, trichomes, tough leaves, or granular minerals may hamper the access to or digestion of plant tissues (Fürstenberg-Hägg et al., 2013; Kant et al., 2015). In addition, a broad range of natural products that are specific for certain plant taxa act as repellents, deterrents or toxins and impede food digestion (Singh and Singh, 2021). Mono- and oligophagous herbivores face a low diversity of defensive compounds contained in their host plants and evolved specific adaptations to cope with these. In contrast, polyphagous herbivores have to deal with a broad range of defences of plants belonging to different taxa (Halon et al., 2015; Katsanis et al., 2016). The nutritional quality and composition of natural products of the host plants influence the development and population dynamics of herbivores (Mason et al., 2022). Their behavioural, physiological and biochemical adaptations determine how herbivores develop on these plants and how they deal with the plant chemistry (Després et al., 2007; Beran and Petschenka, 2022). However, less is known about the development of polyphagous herbivores on different pure vs. mixed diets and which adaptations they have evolved to cope with certain plant defences.
Generally, the metabolism of polyphagous herbivores is less optimised for dealing with particular plant species than that of mono-/oligophagous herbivores (Pentzold et al., 2014a). However, by consuming different host plant species, polyphagous herbivores can benefit from a higher nutritional balance and greater resource availability and they can dilute specific defence compounds of the plants (Franzke et al., 2010; Pentzold et al., 2014b). As a prerequisite, they need to be able to tolerate or detoxify metabolites of different plant species at least for certain periods of time (Ali and Agrawal, 2012; Pentzold et al., 2014b). Even within a host plant individual, the diet quality often differs, with young leaves usually showing higher nutritional values but also higher concentrations of defence compounds than old leaves. Alternating feeding of young and old leaves can thus also help balancing the ingestion of nutrients vs. defences (Tremmel and Müller, 2013). Feeding on mixed diets comprising different plant species has been shown to enhance the performance of different polyphagous but also oligophagous species (Mody et al., 2007; Unsicker et al., 2008; Karban et al., 2010; Malinga et al., 2018). For example, caterpillars of the polyphagous species Platyprepia virginalis (Lepidoptera: Erebidae) have a higher growth rate and survival (Karban et al., 2010), while the oligophagous grasshopper Ruspolia differens (Orthoptera: Tettigoniidae) shows a faster development, higher body mass and higher survival on mixed artificial diets compared to an artificial diet consisting of just one food source (i.e., mono diet) (Malinga et al., 2018). However, metabolic resistance to different plant defences can imply energetic costs for herbivores (Després et al., 2007). Therefore, there may be a trade-off for herbivores that feed on mixed plant diets between nutritional benefits and costs of coping with different plant defences.
One specific defensive system herbivores need to cope with when feeding on plants of the Brassicales is the glucosinolate-myrosinase system. Glucosinolates are non-toxic thiohydroxymate anions with an S-linked β-glucopyranosyl residue, an O-linked sulphate residue and a variable amino acid-derived side chain (Halkier, 2016; Blažević et al., 2020). When plant tissues are disrupted due to insect feeding, glucosinolates are hydrolysed by myrosinases and toxic products such as isothiocyanates, thiocyanates, or nitriles are formed (Winde and Wittstock, 2011; Wittstock et al., 2016). Insects feeding on these plants have evolved different mechanisms to cope with this dual defence. Some species sequester the glucosinolates; others possess enzymes such as nitrile specifier proteins, which redirect the hydrolysis to less toxic products, or sulfatases that convert glucosinolates faster than the myrosinases and thus prevent the formation of toxic products (Müller et al., 2001; Ratzka et al., 2002; Wittstock et al., 2004; Falk and Gershenzon, 2007; Malka et al., 2016; Beran et al., 2018). Another mechanism of dealing with the binary defence system is the conjugation of the toxic hydrolysis products with ascorbate, glutathione or single amino acids (proline, isoleucine, leucine, ascorbate, cysteine, glycine, or aspartic acid) (Wadleigh and Yu, 1988; Kim et al., 2008; Schramm et al., 2012; Friedrichs et al., 2020). However, for many insect species it is still unknown how they cope with the glucosinolate-myrosinase system.
In the present study, the development of a polyphagous insect species on different mono and mixed plant diets as well as the metabolism of glucosinolates by this herbivore were investigated. We used larvae and adults of the tansy leaf beetle Galeruca tanaceti (Coleoptera: Chrysomelidae), which feed on plants of various families, including Asteraceae, to which tansy (Tanacetum vulgare) belongs, and Brassicaeae (Obermaier et al., 2008). To examine the development on different diets, neonate larvae of G. tanaceti were reared on leaves of Chinese cabbage (Brassica rapa spp. pekinensis, Brassicaceae), lettuce (Lactuca sativa, Asteraceae) or tansy or they received mixed diets (cabbage and tansy; lettuce and tansy). Tansy was chosen, because we found different developmental stages, including neonates of G. tanaceti, on this plant in nature. As G. tanaceti can also occur as a pest on cultivated species such as oregano (Origanum vulgare) (Roditakis and Roditakis, 2006), we used lettuce as cultivated Asteraceae for comparison with tansy and Chinese cabbage was used, as we knew from previous studies that the insect can develop very well on this species (Tremmel and Müller, 2014). The development time until reaching adulthood, the adult body mass and the survival were recorded. In addition, as leaf traits the contents of water, carbon (C) and nitrogen (N) and the C/N ratio, specific leaf area (SLA) and trichome density were measured. To investigate whether and how glucosinolates are metabolised by this beetle species, metabolites resulting from feeding experiments with benzyl glucosinolate or 4-hydroxybenzyl glucosinolate were analysed in insect bodies and their faeces. Glucosinolates were applied on leaves of cabbage or lettuce to analyse their metabolism in presence and absence of plant myrosinases, respectively. In line with common findings for polyphagous herbivores we expected that G. tanaceti develops faster, gains a higher body mass and survives better on a mixed diet than when feeding just on one plant species (mono diet). In addition, we expected that this leaf beetle conjugates metabolites derived from glucosinolate hydrolysis rather than using enzymes that redirect the myrosinase activity or circumvent the myrosinase-mediated reactions directly.
Egg clutches of G. tanaceti were collected on a grass-rich landscape near the nature protection area “Moosheide” in late March 2018 (51°84′72″ N, 8°67′28″ E) and on fields consisting of different grasses (Poaceae), tansy (∼20% coverage), ribwort plantain (Plantago lanceolata, Plantaginaceae), yarrow (Achillea millefolium, Asteraceae), and other species from January until March 2020 and 2021 in Germany (52°26′57.08″ N, 9°4′40.69″ E). The eggs are laid from September to December on grasses and other herbs and remain in a diapause over the winter (Meiners et al., 2006). The eggs were kept at 4°C until they were needed for the experiments. To induce the hatching of larvae, twelve to fifty egg clutches were transferred to a climate chamber (18°C, 70% r.h., 16:8 h light:dark) and sprayed with water. For the development experiment (see below), larvae were reared from hatching onwards on leaves of 5–6 weeks old non-flowering Chinese cabbage (Brassica rapa L. spp. pekinensis “Michihili,” seeds from Kiepenkerl, Bruno Nebelung GmbH, Konken, Germany, in the following called “cabbage” for simplicity), lettuce (Lactuca sativa spp. capitata “Maikönig,” seeds from Kiepenkerl) and/or tansy (seeds collected in Bielefeld-Ummeln, Germany; N 51°58.980′, E 008°27.115′,11 m a.s.l.) plants, which were grown in pots (12 cm diameter) with composted soil in a greenhouse (60% r.h., 16:8 h light:dark). The glucosinolate profile of the greenhouse-grown cabbage consists of benzyl glucosinolate [116 nmol/g dry weight (DW); ∼ 8%], indol-3-ylmethyl glucosinolate (718 nmol/g DW; ∼50%), 4-methoxyl-indol-3-ylmethyl glucosinolate (172 nmol/g DW; ∼12%), and 1-methoxyl-indol-3-ylmethyl glucosinolate (428 nmol/g DW; ∼ 30%). For the glucosinolate feeding experiments (see below), larvae and adults were kept in plastic boxes (20 × 20 × 6.5 cm) with ventilated lids, with 50–80 individuals per box, and were provided ad libitum with leaves of about 6–8 weeks old non-flowering cabbage plants until the start of the experiments.
To compare the development of G. tanaceti on different diets, neonate larvae hatching from egg clutches were randomly assigned to the different treatments and were supplied with pieces of leaves of intermediate age of either cabbage, lettuce or tansy (mono diets) or with the mixed diets cabbage and tansy or lettuce and tansy, all offered ad libitum. The diet mixes thus included the natural host tansy and one other cultivated crop plant species. After hatching, the larvae were randomly placed in small Petri dishes (55 mm diameter) with wet filter paper and assigned to one of the diet treatment groups. Initially, the larvae were kept in groups of five individuals due to their gregarious behaviour, which they show in the first larval instar. After 5 days larvae were separated into individual Petri dishes. Every other day individuals were supplied with fresh food and, if necessary, fresh filter paper. Individuals of the mixed diet treatments received leaves of the respective two host plants in alternating order, changing the plant species every other day to ensure that they had to feed on both and could not just select one species. In addition, half of these larvae started with one of the two plant species, while the other half started with the other species, to analyse whether the initial food plant has an effect on the development. In total, 200 larvae were tested, i.e., 40 larvae (replicates) per diet treatment. Individuals were weighed (Sartorius CPA224S-0CE, Sartorius AG, Göttingen, Germany) directly after hatching of larvae from eggs, then again at the separation event (5th day), after reaching the pupal and finally when reaching the adult life stage. The developmental time from neonate until reaching adulthood was assessed for each individual. In addition, the relative growth rate (RGR) was calculated as RGR = (adult body mass − larval body mass at day 5)/(mean body mass * days) (Tremmel and Müller, 2014). Body mass at day 5 was chosen as initial body mass, because this was the day from which on larvae were kept separately. Furthermore, the survival was assessed daily for each individual, starting at the day of larval hatching until the corresponding individual reached adulthood.
To compare quality-related traits of the host plants, leaf material was analysed for contents of water carbon and nitrogen (C and N), the specific leaf area (SLA) and the trichome density. From a separate batch of plants of comparable age (not the ones used for bioassays), 22 discs (6 mm diameter, around 100 mg fresh weight) were taken from leaves of intermediate age and pooled for one replicate (15 replicates per plant species). Fresh leaf material was weighed with a fine scale (Sartorius ME36S, Sartorius AG), freeze-dried and weighed again to calculate the water content. The SLA was calculated by dividing the leaf area (mm2) by the dry mass (mg). The dried leaf material was homogenised, and C and N contents were analysed from a subsample of about 3 mg with a micro-elemental analyser (CN-vario MICRO cube, Elementar Analysesysteme GmbH, Hanau, Germany), using acetanilide as calibration standard (Merck KGaA, Darmstadt, Germany). The data were normalised using the exact dry weight. On one leaf disc per replicate, trichomes (glandular and non-glandular) were counted on the upper and lower leaf surface with a binocular, summed up for both sides and divided by the leaf area (both sides together 0.57 cm2) to obtain the trichome density per cm2 (stereosystem-microscope SZX2-ILLT, Olympus Corporation, Tokyo, Japan; n = 15 per plant species).
Groups of two 3rd instar larvae (around 15 days old, body length: 7–9 mm) or single adults (around 40 days old), kept until the start of the experiment in groups on cabbage leaves, were placed in Petri dishes (55 mm diameter) and supplied with either cabbage or lettuce leaf discs (22 mm diameter) treated with glucosinolates to determine their glucosinolate metabolism in presence (cabbage) and absence (lettuce) of plant myrosinases, respectively. Individuals were starved for at least 3 h and then offered a single treated leaf disc for 23 h on moistened filter paper (two to four replicates per plant species and treatment group). The leaf discs had been treated each with 25 μl of 40 mM solutions of benzyl glucosinolate or 4-hydroxybenzyl glucosinolate (>99%, Phytoplan GmbH, Heidelberg, Germany) dissolved in a mixture of methanol, millipore water and dichloromethane (v:v:v; 64:30:6; methanol LC-MS grade, dichloromethane HPLC grade, Fisher Scientific UK Ltd., Loughborough, United Kingdom), resulting in 1 μmol glucosinolate per leaf disc, or the discs had been treated with the corresponding solvent mixture as control. Using the same leaf treatment, a recovery of at least 80% of the applied glucosinolates after 23 h had been found in a previous study (Friedrichs et al., 2020). The solutions or solvents were applied on the leaf surface and allowed to evaporate for 40 min before offering the leaf discs to the insects. After the feeding time, the groups of two larvae and the individual adults were transferred to 2 ml Eppendorf tubes without food to collect their faeces for 3 h. Then, the individuals (“bodies”) were transferred to new 2 ml Eppendorf tubes. In addition, cabbage and lettuce leaf discs treated with benzyl glucosinolate or 4-hydroxybenzyl glucosinolate solution or the solvent control (n = 3 per treatment combination) were kept without insects and collected after the experimental period (23 h). All samples were frozen in liquid N2 and stored at −80°C until lyophilisation and further processing (see Section “Metabolite identification in larvae, adults, and faeces”).
To identify the glucosinolates and their potential breakdown metabolites in G. tanaceti, the freeze-dried samples were extracted on ice with 300 μl ice-cooled 90% methanol (LC-MS grade, Fisher Scientific UK Ltd.) containing hydrocortisone (>98%, Sigma Aldrich Chemie GmbH, Steinheim, Germany) as internal standard. Samples were macerated with a glass rod and centrifuged at 4°C. Supernatants were filtered with 0.2 μm polytetrafluoroethylene membrane filters (Phenomenex Inc., Torrance, CA, United States) and stored at −80°C until measurement. Samples were analysed using an ultra-high performance liquid chromatograph with a diode array detector, connected to a quadrupole time of flight mass spectrometer (UHPLC-DAD-QTOF-MS/MS; UHPLC: Dionex UltiMate 3000, Thermo Fisher Scientific, San José, CA, United States; Q-TOF: compact, Bruker Daltonics GmbH & Co. KG, Bremen, Germany). Samples (3 μl) were injected and compounds separated on a Kinetex XB-C18 column (1.7 μm, 150 × 2.1 mm, with guard column, Phenomenex) with a gradient of water (eluent A) to acetonitrile (LC-MS grade, Th. Geyer GmbH & Co. KG, Renningen, Germany, eluent B), both with 0.1% (v:v) formic acid (eluent additive for LC-MS, ∼98%, Sigma-Aldrich) at 45°C and a flow rate of 0.5 ml min–1. The gradient started with a ramp from 2% B to 30% B within 20 min, rising to 75% B within 9 min, followed by column cleaning and equilibration. Ultraviolet/visible (UV/VIS) spectra were recorded per DAD at 210–400 nm. MS and MS/MS line spectra [mass-to-charge ratios (m/z) of 50–1300] were acquired in negative electrospray ionisation (ESI–) mode at 8 Hz with the following parameter settings: end plate offset 500 V, capillary voltage 3000 V, nebuliser (N2) pressure 3 bar, dry gas (N2) flow of 12 l min–1 at 275°C, quadrupole ion energy 4 eV, low mass 90 m/z, collision energy in MS mode 7 eV, transfer time 75 μs and pre-pulse storage 6 μs. N2 was used as collision gas in Auto-MS/MS mode to produce fragments of those ions with the highest intensities by ramping the collision energy and isolation width with increasing m/z. A sodium formate solution, which passed through the ESI sprayer before each sample, was used for recalibration of the mass axis. Fifteen blanks were prepared and measured in the same way.
In Compass DataAnalysis 4.4 (Bruker Daltonics GmbH & Co. KG), the mass axis of each sample was recalibrated and molecular features (characteristic retention time and m/z) were picked with the Find Molecular Features algorithm which included spectral background subtraction. The following settings were used: signal-to-noise threshold 3, correlation coefficient threshold 0.75, minimum compound length 20 spectra, smoothing width 7. Ion types used to sort molecular features belonging to the same metabolite together in buckets were: [M-H]–, [M-H2O-H]–, [M+Cl]–, [M+HCOOH-H]–, [M+CH3COOH-H]–, [2M-H]–, [2M+HCOOH-H]–, [2M+CH3COOH-H]–, [3M-H]–. The bucket alignment over the samples was performed in Compass ProfileAnalysis 2.3 (Bruker Daltonics GmbH & Co. KG) by allowing deviations of 0.1 min (retention time) and 6 mDa (m/z), respectively. For semi-quantification, the intensities (peak heights) of the most intense metabolic features (one per bucket) were divided by the intensities of the hydrocortisone [M+HCOOH-H]– ion in the corresponding sample. Fold changes were calculated for these features by dividing their mean intensity in the glucosinolate-fed individuals (each treatment) by the mean intensity in the solvent-fed individuals. Features that may represent metabolites of the glucosinolate breakdown were filtered and retained in the insect dataset [as in Friedrichs et al. (2020)]. Only those features of the different treatment groups (plant type * leaf treatment * sample type) that fulfilled all of the following criteria remained in the data set: (1) in at least one treatment group, the mean intensity of the features should be at least 50 times higher than in the blank samples, (2) the features should occur in at least two of the three replicate samples in at least one treatment group, (3) their fold change should be >2 or the feature should occur only in samples of insects fed glucosinolate-treated leaves (not in control samples), (4) and the mean intensity should have > 1600 counts in at least one sample group (of larvae and adults fed glucosinolate-treated leaves). In the same data set, we also searched for the hydrolysis products such as benzyl isothiocyanate, 4-hydroxybenzyl-isothiocyanate, benzyl cyanide, 4-hydroxybenzyl cyanide, benzyl amine, and 4-hydroxybenzyl amine, as well as already known degradation products from other insect species. To identify the corresponding metabolites, the samples with the highest feature intensities were measured again with a lower spectra rate (1 or 2 Hz) and/or multiple reaction monitoring (with calculated collision energies and isolation widths for the corresponding m/z) in negative (parameters see above) and/or positive (capillary voltage: 4500 V) ESI mode. In DataAnalysis the Smart Formula (3D) function was used to generate molecular formulas of the parent and daughter ions and to rank them based on the m/z deviation and the fit of the isotopic patterns. To obtain further information on the structural formulas, in silico fragmentation (compound lists from PubChem) and spectra matching against the MassBank of North America1 were performed with MetFrag (Ruttkies et al., 2016). In addition, retention times, ion types and spectra (UV/VIS, MS, MS/MS) of the putative breakdown metabolites found in insect samples were compared directly with purchased reference standards by consecutive measurements with UHPLC-QTOF-MS/MS in ESI– and ESI+ mode, which were also recorded in an in-house database. The obtained reference standards were N-benzoyl-D,L-alanine (≥97%, Alpha Aesar, Thermo Fisher GmbH, Kandel, Germany), 3-hydroxy-2-(phenylformamido)propanoic acid (=N-benzoyl-D,L-serine; 95%, Enamine Ltd., NJ, United States), hippuric acid (=N-benzoylglycine; 98%, Sigma-Aldrich Chemie GmbH, Steinheim, Germany) and 2-[(4-hydroxyphenyl)formamido]acetic acid [=N-(4-hydroxybenzoyl)-glycine; 95%, Life Chemicals Inc., Kyiv, Ukraine].
To analyse the localisation of metabolites potentially involved in glucosinolate metabolism in larvae, 3rd instar larvae were starved for 3 h and then either transferred to 2 ml Eppendorf tubes and immediately frozen in liquid N2 (n = 3) or fed with a cabbage leaf disc to which 50 μl of the 40 mM benzyl glucosinolate solution (2 μmol per leaf disc) were applied. The feeding was stopped after 10 min and 3 h, respectively (n = 3 per time point) and the larvae were transferred to 2 ml Eppendorf tubes and immediately frozen in liquid N2. The samples were stored at −80°C until further processing.
To prepare the larval samples for the cryostat, the frozen samples were placed on a small plate, water was added and then frozen again so that the larvae ended up embedded in frozen Millipore water. Tissue sections (40 μm) of frozen larval samples were prepared at −20°C with a cryostat (Leica CM 1950, Leica Biosystems Nussloch GmbH 2021, Nußloch, Germany), transferred with tape (tesa, Norderstedt, Germany) to an indium tin oxide-coated conductive glass slide (Bruker Daltonics GmbH & Co. KG) and fixed with conductive tape (Mouser Electronics Inc., München, Germany). Afterwards, the tissue sections were dried in a vacuum desiccator and coated with an N-(1-naphthyl)-ethylenediamine dihydrochloride matrix (≥99%, Carl Roth GmbH + Co. KG; 7 mg ml–1 in 70% methanol). The matrix was applied using a TM-Sprayer (HTX Technologies, LLC, Chapel Hill, NC, United States), with a flow rate of 0.12 ml min–1, a velocity of 1200 mm min–1, and 3 mm track spacing for 28 passes at a nozzle temperature of 70°C (Neumann et al., 2021). Separate cross sections of larvae were stained with Mayer’s hematoxylin and eosin Y stain (Carl Roth GmbH+Co. KG) and scanned with a MIRAX DESK slide scanner (ZEISS, Carl Zeiss MicroImaging GmbH, Jena, Germany).
Matrix-assisted laser desorption/ionisation mass spectrometry imaging (MALDI-MSI) measurements of larval tissues were carried out on a Spectroglyph MALDI/ESI Injector (Spectroglyph, LLC, Kennewick, WA, United States) connected with a Q Exactive Plus Orbitrap (Thermo Fisher Scientific Inc., Waltham, MA, United States). External mass calibration was done with Pierce Negative Ion Calibration Solution (Thermo Fisher Scientific Inc.) and internal calibration using a matrix signal. The measurement settings were m/z range of 85–1000, maximum injection time of 250 ms and mass resolution of 70,000 in negative ion mode. The laser settings were a diode laser current of 2.20 Amps and a laser repetition rate of 500 Hz for the MSI measurements with a lateral resolution of 100 μm. MALDI-Orbitrap-MSI data were analysed using SCiLS Lab MVS Version 2022a Pro (Bruker Daltonics GmbH & Co. KG). Peaks were extracted manually by calculating the expected m/z of the [M-H]– ions of the target metabolites with the Isotope Pattern tool of DataAnalysis and adding them to the peak list.
All data were analysed using R (R Core Team, 2021, version 4.1.2) and RStudio (RStudio, version 2021.09.2-382), using the packages car (Fox and Weisberg, 2019), ggplot2 (Wickham, 2016), remotes (Csárdi et al., 2022), ggpattern (FC et al., 2022), stringr (Wickham, 2022), survival (Therneau and Grambsch, 2000), and survminer (Kassambara, 2016). A significance threshold of α = 0.05 was set for all statistical tests. To test whether the starting food plant (mixed diets) has an effect on the insects, the development time from neonate until adulthood, time in the pupal stage and the RGR of individuals were first compared within each of the two mixed diet treatments between those that started with tansy or the corresponding other food plant species with Mann–Whitney U-tests (in case of non-normally distributed data) or Student’s t-tests (normally distributed data based on Shapiro–Wilk tests and variance homogeneous based on Levene-tests). In addition, the influence of the initial food plant on the survival until day 38 was examined using log-rank tests. Since the order of the food plants had no significant effect on any of the measured traits, the data were pooled within each of the mixed diet treatments. Subsequently, because none of the data sets (mono and mixed diets) were normally distributed (Shapiro–Wilk tests), Kruskal–Wallis tests followed by pairwise Mann–Whitney U-tests with Bonferroni–Holm correction of p values were performed to test for differences in development time from neonate until adulthood, time in the pupal stage and the RGR between the treatment groups. Leaf quality traits were statistically compared between the plant species in the same way. Survival of insects on the different diet treatments was compared with an overall log-rank test followed by pairwise log-rank tests with Bonferroni-Holm correction of p-values and was illustrated with Kaplan–Meier curves; for these analyses, the data until day 38, when the first individual reached adulthood, were included.
The development time of G. tanaceti from larval hatch until adulthood was significantly different between individuals reared on the different diets (X2 = 67.73, df = 4, p < 0.001); individuals developed fastest on the cabbage mono diet, while the few individuals that survived until adulthood on tansy took on average 1.7 times as long (Figure 1A). The development time on lettuce and the two mixed diets was intermediate. The time in the pupal stage was between 7 and 11 days and similar for the individuals kept on the different diets (X2 = 10.51, df = 4, p > 0.05). In line with the findings for the development time, the RGR of the insects was significantly different between the treatment groups (X2 = 68.48, df = 4; p < 0.001); individuals showed the highest RGR on the cabbage mono diet and the lowest one on the tansy mono diet (Figure 1B).
Figure 1. Development and survival of Galeruca tanaceti on different diets with the (A) development time from larval hatch until adulthood, (B) relative growth rate (RGR), and (C) survival probability until day 38 [initially n = 40 per dietary treatment; n = 7–33 per dietary treatment in panels (A,B) as indicated below the x-axis, because the parameters could only be calculated for individuals that reached adulthood]. Individuals were either reared on mono diets of cabbage (Brassica rapa), lettuce (Lactuca sativa), or tansy (Tanacetum vulgare) or received mixed diets (host plant change every other day) of cabbage and tansy or lettuce and tansy. Box plots (A,B) show the means (as crosses), medians (horizontal lines), and the interquartile ranges (IQR; boxes), the whiskers extend to the most extreme values with max. 1.5 times the IQR. Individual values are plotted as dark circles. Survival (C) is depicted as Kaplan–Meier curves, including data until day 38 when the first individual reached adulthood; crosses at day 38 indicate right censoring (i.e., individuals were still alive at this time point). Statistical analyses were done with Kruskal–Wallis tests followed by pairwise Mann–Whitney U-tests (A,B) and an overall log-rank test followed by pairwise log-rank tests (C), respectively. Significant differences (p < 0.05) are indicated by different lowercase letters.
More than 80% (33 out of 40) of the individuals reared on cabbage reached adulthood, whereas only 18% of the individuals on tansy reached adulthood; on cabbage and tansy, lettuce and lettuce and tansy 22, 16, and 16 (out of 40) individuals reached adulthood, respectively. The survival of the individuals until day 38 differed significantly between the individuals kept on the different diets (X2 = 18.5, df = 4, p = 0.001), with the survival on the cabbage mono diet being significantly higher than that on lettuce, lettuce and tansy and the tansy diets, while the survival on the cabbage and tansy diet was intermediate (Figure 1C).
The water content differed significantly between the leaves of the three host plant species (X2 = 39.13, df = 2, p < 0.001), being highest for lettuce and lowest for tansy (Figure 2A). The C/N ratios of the leaves also differed significantly (X2 = 39.13, df = 2, p < 0.001), with lettuce showing the lowest and tansy the highest C/N ratio, being on average more than three times higher in tansy compared to the other two plant species (Figure 2B). The contents of nitrogen (X2 = 38.96, df = 2, p < 0.001) and carbon (X2 = 31.90, df = 2, p < 0.001) were significantly different between the three plant species, whereby lettuce leaves had the highest nitrogen and lowest carbon content (N: 5.76% ± 0.28, C: 40.54% ± 0.58, mean ± SD), for tansy it was the opposite (N: 1.48% ± 0.25, C: 45.38% ± 0.57) and cabbage was in between (N: 4.56% ± 0.36, C: 41.04% ± 0.40). Lettuce leaves had the highest and tansy leaves the lowest SLA, while the SLA of cabbage leaves was in between (X2 = 39.13, df = 2, p < 0.001; Figure 2C). The density of leaf trichomes was also significantly different between the three plant species (X2 = 40.72, df = 2, p < 0.001). Only few trichomes per leaf area were found on cabbage leaves (7.3 ± 3.2 per cm2, mean ± SD), none on lettuce, while the leaves of tansy showed by far the highest trichome density (629.2 ± 111.6 per cm2). The trichomes were more or less equally spread on the lower and upper leaf surface.
Figure 2. Leaf quality traits of different food plant species (cabbage: Brassica rapa; lettuce: Lactuca sativa; tansy: Tanacetum vulgare) with (A) water content, (B) carbon-to-nitrogen (C/N) ratio, and (C) specific leaf area (SLA; n = 15 per plant species). Box plots show the means (as crosses), medians (horizontal lines) and interquartile ranges (IQR; boxes), the whiskers extend to the most extreme values with max. 1.5 times the IQR. Individual values are plotted as dark circles. Statistical analyses were done with Kruskal–Wallis tests followed by pairwise Mann–Whitney U-tests. Significant differences (p < 0.05) are indicated by different lowercase letters.
The glucosinolate feeding experiment revealed eight metabolites that were of interest, because they only or mainly occurred in samples (bodies, faeces) of individuals fed with glucosinolate-treated leaves but were not present or occurred in much lower concentrations in individuals fed with solvent-treated controls. All metabolites could be putatively identified using reference standards (1–8, Tables 1, 2; Supplementary Table 1). Both glucosinolates (benzyl glucosinolate: 1, 5.00 min, ESI– m/z 408.0437; 4-hydroxybenzyl glucosinolate: 5, 2.20 min, ESI– m/z 424.0381) were detected in body and faecal samples of larvae and adults fed with the corresponding glucosinolate-treated cabbage and lettuce leaves as well as in the corresponding leaves (Tables 1, 2). They showed characteristic fragments ([SO3]–, [SO4]–, and [HSO4]–) and rearrangement structures (Table 1; Supplementary Table 1; Cataldi et al., 2007; Cataldi et al., 2010; Friedrichs et al., 2020).
Table 1. Glucosinolates (1, 5; in bold) and their putative breakdown metabolites (2–4, 6–8) detected in bodies and/or faeces of larvae and adults of Galeruca tanaceti with molecular formulas, average retention times (RT), ion types, observed masses as mass-to-charge ratios (m/z), calculated (calc.) m/z [IsotopPattern] and error [ppm] as well as ion formulas for the negative (ESI–) and positive (ESI+) electrospray ionisation mode measured by UHPLC-DAD-QTOF-MS/MS.
Table 2. Numbers of insect body and faecal samples from larvae and adults of Galeruca tanaceti (out of 2–4 replicates) and leaf samples in which the glucosinolates (highlighted in bold) and their putative breakdown metabolites were found by UHPLC-DAD-QTOF-MS/MS.
After feeding on leaves treated with benzyl glucosinolate (1), three putative breakdown metabolites of this glucosinolate with m/z values (ESI– mode) of 178.0516 (5.40 min, 2; Table 1), 192.0672 (7.83 min, 3) and 208.0619 (4.70 min, 4) were found in body and faecal samples of both larvae and adults (Table 2). The metabolite (2) with an m/z of 178.0516 was putatively identified as N-benzoylglycine based on the characteristic fragments with m/z 77 ([C6H5]–), 132 ([C8H6NO]–), and 134 ([C8H8NO]–) (Table 1; Supplementary Table 1). The characteristic fragments of the metabolite with an m/z of 192.0672 (3) were m/z 77 ([C6H5]–), 120 ([C7H6NO]–), and 148 ([C9H10NO]–) and the metabolite was putatively identified as N-benzoylalanine. Metabolite (4) with an m/z of 208.0619 was putatively identified as N-benzoylserine due to the characteristic fragments with m/z 134 ([C8H8NO]–), 146 ([C9H8NO]–), and 178 ([C9H8NO3]–). These breakdown metabolites (2–4) were putatively identified by direct comparison with reference standards measured by UHPLC-QTOF-MS/MS, showing matching retention times, ion types and spectra (UV/VIS, MS, MS/MS).
In body and faecal samples of larvae and in body samples of adults which were fed with leaves treated with 4-hydroxybenzyl glucosinolate (5), three putative breakdown metabolites (6–8) of this glucosinolate were detected. The metabolite with an m/z (ESI– mode) of 194.0458 (3.10 min, 6) and the characteristic fragments with m/z 93 ([C6H5O]–) and 100 ([C3H2NO3]–) was putatively identified as N-(4-hydroxybenzoyl)glycine. The metabolite with an m/z of 121.0297 (5.50 min, 7) and a fragment with an m/z of 92 ([C6H4O]–) was putatively identified as 4-hydroxybenzaldehyde, while the metabolite with an m/z of 137.0246 (4.20 min, 8) and a fragment with an m/z of 93 ([C6H5O]–), was putatively identified as 4-hydroxybenzoic acid. The main putative breakdown metabolites (2–4, 6) were only detected in insect samples but not in plant samples, while 4-hydroxybenzaldehyde (7) and 4-hydroxybenzoic acid (8) were also found in samples of leaves treated with 4-hydroxybenzyl glucosinolate, but mostly only in traces (Table 2). None of the potential hydrolysis products such as isothiocyanates, nitriles and amines, as well as the already known degradation products of other insect species that might be involved in the glucosinolate degradation of benzyl glucosinolate and 4-hydroxybenzyl glucosinolate could be found in our dataset.
The putative breakdown metabolites of benzyl glucosinolate (1) with calculated m/z values for [M-H]– ions of 178.0498 for N-benzoylglycine (2), of 192.0655 for N-benzoylalanine, (3) and of 208.0604 for N-benzoylserine (4), respectively, were found by MALDI-Orbitrap-MSI in larval tissues (Figure 3). They showed increasing concentrations (represented as colour gradients) from the anterior to the posterior region of the larvae (Figure 3C). The posterior region with the highest metabolite concentrations (Figure 3C) probably represented the Malpighian tubules and the hindgut (Figures 3A,B). The breakdown metabolites were detected in low concentrations in the hindgut already in larvae that had fed on treated leaves for 10 min, but showed a high concentration in insects fed for 3 h.
Figure 3. Schematic representation of a Galeruca tanaceti 3rd instar larva showing a (A) stained tissue cross-section, (B) dissected gut, and (C) MALDI-Orbitrap MSI of tissue cross-sections. The larva was fed for 3 h with a cabbage leaf disc treated with benzyl glucosinolate. The abundance of the putative breakdown metabolites (m/z of 178.0498: putatively [M-H]– ions of N-benzoylglycine; m/z of 192.0655: putatively [M-H]– ions of N-benzoylalanine; m/z of 208.0604: putatively [M-H]– ions of N-benzoylserine) is highlighted in colour. The more intense the colour, the more ions of the putative breakdown metabolites could be found.
The consumption of different food plants is considered to be beneficial for polyphagous insects, because it enables them to balance their supply with nutrients, which they need for growth, development, and reproduction (Simpson et al., 2015; Wang et al., 2021). In addition, individuals may profit from diet mixing, because in that way toxic plant compounds get diluted (Singer and Bernays, 2003; Mody et al., 2007; Unsicker et al., 2008; Malinga et al., 2018). Based on these potential advantages of diet mixing, we expected G. tanaceti to develop better on mixed diets than on mono diets. However, the results revealed a different pattern, as individuals reared on cabbage mono diet had a significantly faster development and a higher RGR and survival than individuals reared on the two mixed diets, cabbage and tansy (not significantly different for survival) and lettuce and tansy, as well as on the lettuce mono diet. On a tansy mono diet individuals developed very poorly and only few individuals reached adulthood. These findings may be explained by a combination of factors including plant nutritional quality, morphological and chemical plant defences as well as the metabolic ability of G. tanaceti to deal with certain plant defences.
As nutritional components of leaves, nitrogen and, to a lesser extent, water content are crucial for herbivores (Coley et al., 2006). Both diminish with leaf age, but in our experiment we used leaves of standardised intermediate age for all host plants. The water content differed between the species, but at least for lettuce and cabbage it was around 90% and can thus be considered as sufficient for herbivores (Scriber and Slansky, 1981). However, the N content was much higher in cabbage and lettuce leaves, probably due to the fact that these are cultivated crop plants, compared to the ruderal species tansy. Plant tissue containing more nitrogen usually results in a better nutrition for herbivores and thus better growth and development (Coley et al., 2006; Carmona et al., 2011; Franzke and Reinhold, 2011; Duan et al., 2021). Moreover, a protein-rich diet may be beneficial to gain the necessary amino acids for detoxification processes (Jeschke et al., 2021). In line with the N content, the leaf C/N ratio was lowest in lettuce, a bit higher in cabbage and highest in tansy. C and N not only form the basis of essential food components, such as carbohydrates and proteins, for herbivores, but are also the structural components of specialised metabolites. The high C/N ratio in tansy suggests lower nutritional value for herbivorous insects. The high C content is probably partly due to the high concentrations of terpenoids present in tansy (Jakobs and Müller, 2019).
Apart from nutritional traits, the SLA and trichome density are important determinants of plant quality by influencing the digestibility and potentially acting as mechanical barriers (Dalin et al., 2008; Arora and Malik, 2021; Tiku, 2021). Moreover, SLA is a good indicator of leaf toughness (DeLucia et al., 2012). In our study, leaves of tansy had the lowest SLA, indicating high toughness, and the highest trichome density, both providing effective mechanical defences. In addition, the oil reservoirs of the glandular trichomes of tansy contain several terpenoids (Jakobs and Müller, 2019). Terpenoids have adverse effects on various organisms, potentially due to their lipophilic nature that causes loss of chemiosmotic control of cell membranes (Gershenzon and Dudareva, 2007). Thus, the trichomes of tansy may not only be a mechanical barrier but also be involved in chemical defence. Although the tansy leaf beetle G. tanaceti is named after tansy, on which it can be found in nature, diets containing tansy are surprisingly not very beneficial for this species, potentially due to the low water content, low SLA and high trichome density. Since we did not observe the feeding behaviour, we cannot conclude whether the insects got intoxicated from feeding high amounts of tansy or whether they started to avoid feeding on tansy at all at some point and starved to death. The combination of nutritional parameters as well as morphological and chemical defences appear to play a critical role for the performance of the leaf beetle G. tanaceti, with chemical defences possibly having the greatest impact due to their potential toxicity. In contrast, G. tanaceti obviously was able to deal with the chemical defences of Brassicaceae, as it developed so well on cabbage. Chinese cabbage, as used here, may be in particular a suitable food source for the beetles, as it mainly forms epithionitriles rather than toxic isothiocyanates during glucosinolate hydrolysis (Geilfus et al., 2016). Thus, we explored how this insect species metabolises glucosinolates and their hydrolysis products.
In line with our expectation, we found evidence that G. tanaceti larvae and adults conjugate metabolites from glucosinolate hydrolysis, leading to specific breakdown metabolites. The main metabolites found in body and faecal samples of G. tanaceti after feeding on leaves treated with benzyl glucosinolate were putatively identified as N-benzoylglycine, N-benzoylalanine, and N-benzoylserine, whereas the main metabolite after feeding on 4-hydroxybenzyl glucosinolate-treated leaves was identified as N-(4-hydroxybenzyl)glycine. Thus, larvae and adult G. tanaceti seem to use amino acid conjugation, to cope with plant toxins released by glucosinolate hydrolysis. Phase I and II metabolism of lipophilic compounds is a general strategy of herbivores to cope with toxins, turning the toxic compounds more polar and facilitating excretion (Yu, 2008). Ubiquitous enzymes that are often involved in these reactions are cytochrome P450s or UDP-glycosyltransferases (Heidel-Fischer and Vogel, 2015). For example, three classes of general detoxification enzymes, namely esterases, glutathione S-transferases, and cytochrome P450 monooxygenases, showed enhanced activities in adults of the polyphagous species Helopeltis theivora (Hemiptera: Miridae) when feeding on certain host plants (Saha et al., 2012). Individuals of G. tanaceti may likewise use such general enzymes to cope with the glucosinolate-myrosinase system and other plant toxins. The conjugation with amino acids may be catalysed by amino acid transferases and facilitate the excretion of metabolites.
When offering 4-hydroxybenzyl glucosinolate to G. tanaceti, we additionally detected 4-hydroxybenzaldehyde and 4-hydroxybenzoic acid, which were also present in the treated leaf samples. These metabolites were likewise found in larvae and adults of the oligophagous species Phaedon cochleariae (Coleoptera: Chrysomelidae) after feeding on 4-hydroxybenzyl glucosinolate-treated leaves (Table 1; Friedrichs et al., 2020, 2022). Thus, the glucosinolate metabolism in G. tanaceti may be similar to that suggested for P. cochleariae (Figure 4; Friedrichs et al., 2020, 2022). In a first step, benzenic glucosinolates may be hydrolysed by plant myrosinases and/or gut microbiota of the insects (see below) to isothiocyanates and/or nitriles. The isothiocyanates then react to alcohols or amines, which are oxidised to aldehydes, and further react to carboxylic acids, while the nitriles are either converted directly to the carboxylic acids or also oxidised first to an alcohol, then to an aldehyde and then to a carboxylic acid (Buskov et al., 2000; Heckel, 2014; Jeschke et al., 2016; Welte et al., 2016b).
Figure 4. Postulated metabolism of benzyl glucosinolate and 4-hydroxybenzyl glucosinolate in Galeruca tanaceti. The reaction pathways are based on metabolites found in samples (bodies, faeces) of larvae and adults fed with glucosinolate-treated cabbage or lettuce leaves. Glucosinolates are probably first hydrolysed to isothiocyanates and/or nitriles. The isothiocyanates then react to alcohols or amines (both not shown), which are oxidised to aldehydes, and further react to carboxylic acids. The nitriles are either converted directly to the carboxylic acids or also oxidised first to an alcohol, then to an aldehyde and then to a carboxylic acid. The carboxylic acids are probably metabolised to carbonyl-coenzyme A (CoA)-complexes or other activated carboxylic acids (not shown) and further conjugated with different amino acids based on the benzenic side chain. The putative intermediate derived from benzyl glucosinolate (1) is then conjugated with glycine, alanine or serine leading to N-benzoylglycine (2), N-benzoylalanine (3), and N-benzoylserine (4), respectively, while for the intermediate derived from 4-hydroxybenzyl glucosinolate (5) conjugation with glycine leads to N-(4-hydroxybenzoyl)glycine (6). The intermediates 4-hydroxybenzaldehyde (7) and 4-hydroxybenzoic acid (8) were detected in individuals fed with 4-hydroxybenzyl glucosinolate; the corresponding intermediates for the benzyl glucosinolate treatment were not detectable. Putative intermediates are shown in grey, if they were not detectable in insect samples but are probably part of the putative metabolism in G. tanaceti.
Surprisingly, the same breakdown metabolites were also detected in individuals of G. tanaceti fed with glucosinolate-treated lettuce, which does not contain myrosinases. Moreover, no myrosinase activity was detectable in larvae that had freshly moulted or only fed lettuce as revealed by photometric assays with larvae [data not shown, experiments performed as in Friedrichs et al. (2020)]. These findings suggest that plant myrosinases are not necessarily involved in the detoxification mechanism. Gut microbiota may play a role in the formation of isothiocyanates, nitriles, and amines independent of plant myrosinases, as known for humans, other vertebrates, and insects (Mutlib et al., 2002; Angelino et al., 2015; Welte et al., 2016a, b; Liu et al., 2017; Liou et al., 2020; Sikorska-Zimny and Beneduce, 2021) and also suggested for P. cochleariae (Friedrichs et al., 2020, 2022).
Part of the glucosinolate metabolism may predominantly take place in G. tanaceti in the Malpighian tubules. These are known to be involved in osmoregulation and removal of metabolic waste as well as xenobiotic substances in insects (Rossi et al., 2020). The breakdown metabolites were already detected after 10 min in the corresponding region of the larvae, indicating a rapid detoxification. In adult Phyllotreta armoraciae (Coleoptera: Chrysomelidae) a selective and active reabsorption of glucosinolates takes place in the Malpighian tubules, which seems to be important for glucosinolate sequestration into the haemolymph (Yang et al., 2021). In larvae and adults of Helicoverpa armigera (Lepidoptera: Noctuidae) some detoxification-related enzymes were found in the Malpighian tubules (Yuan et al., 2018). After being processed and/or stored in the Malpighian tubules, the detoxification metabolites may then be excreted.
The question arises why G. tanaceti uses these specific amino acids for conjugation of metabolites derived from glucosinolate metabolism. Glycine, alanine, and serine are non-essential amino acids (Brodbeck and Strong, 1987) that are synthesised in plant species in different concentrations (Kumar et al., 2017) and are thus readily available to herbivores. Pieris rapae (Lepidoptera: Pieridae) also uses glycine to conjugate metabolites derived from glucosinolates, among other detoxification mechanisms, while insect species such as Bemisia tabaci (Hemiptera: Aleyrodoidae) and P. armoraciae use conjugation with glutathione, which contains glycine (Vergara et al., 2006; Stauber et al., 2012; Malka et al., 2020; Sporer et al., 2021). Alanine and serine are not yet known to be used by other insects to cope with the glucosinolate-myrosinase system [for review see Friedrichs et al. (2022)]. However, in Spodoptera littoralis (Lepidoptera: Noctuidae) these amino acids are involved in detoxification of 3-nitropropanoic acid, an irreversible inhibitor of mitochondrial succinate dehydrogenase (Novoselov et al., 2015). Moreover, it is puzzling that we found conjugation products with three amino acids derived from benzyl glucosinolate, but only one amino acid conjugate derived from 4-hydroxybenzyl glucosinolate. The structural difference between these glucosinolates, i.e., the additional hydroxy group, together with enzyme substrate specificities, may influence the enzymatic pathways involved in glucosinolate metabolism. Similarly, differences in the metabolism of benzyl glucosinolate vs. 4-hydroxybenzyl glucosinolate were found in P. rapae, where both glucosinolates are first converted to nitriles, but phenylacetonitrile is then converted and conjugated with glycine, while the phenolic moiety of 4-hydroxyphenylacetonitrile is sulphated (Jeschke et al., 2015).
In summary, herbivores feeding on mixed plant species may face a trade-off between a more balanced nutrient uptake and minimising or regulating negative effects of plant toxins (Behmer, 2009; Nersesian et al., 2012). In addition, they have to cope with different morphological defences. Thus, food mixing is not necessarily beneficial when one of the plant species is strongly defended, such as tansy. The fact that individuals of G. tanaceti performed best on the cabbage mono-diet may be attributed to the high N content and low morphological resistance of this plant species, as well as to the ability of G. tanaceti to metabolise glucosinolate hydrolysis products. A conjugation with glycine to deal with the glucosinolate-myrosinase system is already known, but conjugation with alanine and serine seems to be more unique to G. tanaceti. The question remains whether this insect species may use similar amino acid conjugation processes to deal with other defences than glucosinolates.
The original contributions presented in this study are included in the article/Supplementary material, further inquiries can be directed to the corresponding author.
JF, SG, and CM contributed to the conception and design of the study. JF and SG carried out the experiments. JF, RS, and SJMS accomplished the metabolome analysis and identification of metabolites. JF, JMN, and KN performed the metabolite localisation. JF, RS, and CM interpreted the data. JF wrote the first draft of the manuscript and RS and CM revised it. All authors contributed to manuscript revision, read, and approved the submitted version.
This work was funded by the grant MU 1829/20-1 to CM of the Deutsche Forschungsgemeinschaft. We acknowledged support regarding the publication costs by the Deutsche Forschungsgemeinschaft and the Open Access Publication Fund of Bielefeld University.
We thank the gardeners of Bielefeld University for help in plant rearing, Karin Djendouci for help with the C/N-analysis, and Saskia Wall and Christine Recker for support in performing the experiments.
The authors declare that the research was conducted in the absence of any commercial or financial relationships that could be construed as a potential conflict of interest.
All claims expressed in this article are solely those of the authors and do not necessarily represent those of their affiliated organizations, or those of the publisher, the editors and the reviewers. Any product that may be evaluated in this article, or claim that may be made by its manufacturer, is not guaranteed or endorsed by the publisher.
The Supplementary Material for this article can be found online at: https://www.frontiersin.org/articles/10.3389/fevo.2022.960850/full#supplementary-material
Ali, J. G., and Agrawal, A. A. (2012). Specialist versus generalist insect herbivores and plant defense. Trends Plant Sci. 17, 293–302. doi: 10.1016/j.tplants.2012.02.006
Angelino, D., Dosz, E. B., Sun, J., Hoeflinger, J. L., Van Tassell, M. L., Chen, P., et al. (2015). Myrosinase-dependent and -independent formation and control of isothiocyanate products of glucosinolate hydrolysis. Front. Plant Sci. 6:831. doi: 10.3389/fpls.2015.00831
Arora, R., and Malik, G. (2021). “Microbe-plant-insect interactions: A comparative dissection of interactome,” in Plant-Pest Interactions: From Molecular Mechanisms To Chemical Ecology, eds I. K. Singh and A. Singh (Berlin: Springer), 365–398.
Behmer, S. T. (2009). Insect herbivore nutrient regulation. Annu. Rev. Entomol. 54, 165–187. doi: 10.1146/annurev.ento.54.110807.090537
Beran, F., and Petschenka, G. (2022). Sequestration of plant defense compounds by insects: From mechanisms to insect–plant coevolution. Annu. Rev. Entomol. 67, 163–180. doi: 10.1146/annurev-ento-062821-062319
Beran, F., Sporer, T., Paetz, C., Ahn, S. J., Betzin, F., Kunert, G., et al. (2018). One pathway is not enough: The cabbage stem flea beetle Psylliodes chrysocephala uses multiple strategies to overcome the glucosinolate-myrosinase defense in its host plants. Front. Plant Sci. 9:1754. doi: 10.3389/fpls.2018.01754
Blažević, I., Montaut, S., Burčul, F., Olsen, C. E., Burow, M., Rollin, P., et al. (2020). Glucosinolate structural diversity, identification, chemical synthesis and metabolism in plants. Phytochemistry 169:112100. doi: 10.1016/j.phytochem.2019.112100
Brodbeck, B., and Strong, D. (1987). “Amino acid nutrition of herbivorous insects and stress to host plants,” in Insect Outbreaks, eds P. Barbosa and J. C. Schultz (San Diego: Academic Press, INC), 347–363. doi: 10.1016/b978-0-12-078148-5.50018-x
Buskov, S., Hasselstrøm, J., Olsen, C. E., Sørensen, H., Sørensen, J. C., and Sørensen, S. (2000). Supercritical fluid chromatography as a method of analysis for the determination of 4-hydroxybenzylglucosinolate degradation products. J. Biochem. Biophys. Methods 43, 157–174. doi: 10.1016/s0165-022x(00)00081-6
Carmona, D., Lajeunesse, M. J., and Johnson, M. T. J. (2011). Plant traits that predict resistance to herbivores. Funct. Ecol. 25, 358–367. doi: 10.1111/j.1365-2435.2010.01794.x
Cataldi, T. R. I., Lelario, F., Orlando, D., and Bufo, S. A. (2010). Collision-induced dissociation of the A+2 isotope ion facilitates glucosinolates structure elucidation by electrospray ionization-tandem mass spectrometry with a linear quadrupole ion trap. Anal. Chem. 82, 5686–5696. doi: 10.1021/ac100703w
Cataldi, T. R. I., Rubino, A., Lelario, F., and Bufo, S. A. (2007). Naturally occuring glucosinolates in plant extracts of rocket salad (Eruca sativa L.) identified by liquid chromatography coupled with negative ion electrospray ionization and quadrupole ion-trap mass spectrometry. Rapid Commun. Mass Spectrom. 21, 2374–2388. doi: 10.1002/rcm.3101
Coley, P. D., Bateman, M. L., and Kursar, T. A. (2006). The effects of plant quality on caterpillar growth and defense against natural enemies. Oikos 115, 219–228. doi: 10.1111/j.2006.0030-1299.14928.x
Csárdi, G., Hester, J., Wickham, H., Chang, W., Morgan, M., and Tenenbaum, D. (2022). Remotes: R Package Installation from Remote Repositories, Including ‘GitHub’. Available online at: https://remotes.r-lib.org, https://github.com/r-lib/remotes (accessed February 28, 2022).
Dalin, P., Ågren, J., Björkman, C., Huttunen, P., and Kärkkäinen, K. (2008). “Leaf trichome formation and plant resistance to herbivory,” in Induced Plant Resistance to Herbivory, ed. A. Schaller (Dordrecht: Springer), 89–105. doi: 10.1007/978-1-4020-8182-8_4
DeLucia, E. H., Nabity, P. D., Zavala, J. A., and Berenbaum, M. R. (2012). Climate change: Resetting plant-insect interactions. Plant Physiol. 160, 1677–1685. doi: 10.1104/pp.112.204750
Després, L., David, J. P., and Gallet, C. (2007). The evolutionary ecology of insect resistance to plant chemicals. Trends Ecol. Evol. 22, 298–307. doi: 10.1016/j.tree.2007.02.010
Duan, M. Y., Zhu, H., Wang, H., Guo, S. Y., Li, H., Jiang, L. L., et al. (2021). Effects of water deficiency on preference and performance of an insect herbivore Ostrinia furnacalis. Bull. Entomol. Res. 111, 595–604. doi: 10.1017/s0007485321000407
Falk, K. L., and Gershenzon, J. (2007). The desert locust. Schistocerca gregaria, detoxifies the glucosinolates of Schouwia purpurea by desulfation. J. Chem. Ecol. 33, 1542–1555. doi: 10.1007/s10886-007-9331-0
FC, M., and Davis, T. ggplot2 authors. (2022). Ggpattern: ‘Ggplot2’ Pattern Geoms. Available online at: https://github.com/coolbutuseless/ggpattern (accessed February 28, 2022).
Fox, J., and Weisberg, S. (2019). An R Companion to Applied Regression, Third edition. Thousand Oaks CA: Sage.
Franzke, A., and Reinhold, K. (2011). Stressing food plants by altering water availability affects grasshopper performance. Ecosphere 2:art85. doi: 10.1890/es11-00095.1
Franzke, A., Unsicker, S. B., Specht, J., Kohler, G., and Weisser, W. W. (2010). Being a generalist herbivore in a diverse world: How do diets from different grasslands influence food plant selection and fitness of the grasshopper Chorthippus parallelus? Ecol. Entomol. 35, 126–138. doi: 10.1111/j.1365-2311.2009.01168.x
Friedrichs, J., Schweiger, R., Geisler, S., Mix, A., Wittstock, U., and Müller, C. (2020). Novel glucosinolate metabolism in larvae of the leaf beetle Phaedon cochleariae. Insect Biochem. Mol. Biol. 124:103431. doi: 10.1016/j.ibmb.2020.103431
Friedrichs, J., Schweiger, R., and Müller, C. (2022). Unique metabolism of different glucosinolates in larvae and adults of a leaf beetle specialised on Brassicaceae. Sci. Rep. 12:10905. doi: 10.1038/s41598-022-14636-6
Fürstenberg-Hägg, J., Zagrobelny, M., and Bak, S. (2013). Plant defense against insect herbivores. Int. J. Mol. Sci. 14, 10242–10297. doi: 10.3390/ijms140510242
Geilfus, C. M., Hasler, K., Witzel, K., Gerendas, J., and Muhling, K. H. (2016). Interactive effects of genotype and N/S-supply on glucosinolates and glucosinolate breakdown products in Chinese cabbage (Brassica rapa L. ssp pekinensis). J. Appl. Bot. Food Qual. 89, 279–286. doi: 10.5073/jabfq.2016.089.036
Gershenzon, J., and Dudareva, N. (2007). The function of terpene natural products in the natural world. Nat. Chem. Biol. 3, 408–414. doi: 10.1038/nchembio.2007.5
Halkier, B. A. (2016). “General introduction to glucosinolates,” in Advances in Botanical Research, ed. S. Kopriva (Cambridge, MA: Academic Press), 1–14.
Halon, E., Eakteiman, G., Moshitzky, P., Elbaz, M., Alon, M., Pavlidi, N., et al. (2015). Only a minority of broad-range detoxification genes respond to a variety of phytotoxins in generalist Bemisia tabaci species. Sci. Rep. 5:17975. doi: 10.1038/srep17975
Heckel, D. G. (2014). Insect detoxification and sequestration strategies. Annu. Plant Rev. 47, 77–114. doi: 10.1002/9781118829783.ch3
Heidel-Fischer, H. M., and Vogel, H. (2015). Molecular mechanisms of insect adaptation to plant secondary compounds. Curr. Opin. Insect Sci. 8, 8–14. doi: 10.1016/j.cois.2015.02.004
Jakobs, R., and Müller, C. (2019). Volatile, stored and phloem exudate-located compounds represent different appearance levels affecting aphid niche choice. Phytochemistry 159, 1–10. doi: 10.1016/j.phytochem.2018.11.018
Jeschke, V., Gershenzon, J., and Giddings Vassão, D. (2015). Metabolism of glucosinolates and their hydrolysis products in insect herbivores, in The Formation, Structure and Activity of Phytochemicals. Recent Adv. Phytochem. 45, 163–194. doi: 10.1007/978-3-319-20397-3_7
Jeschke, V., Gershenzon, J., and Vassão, D. G. (2016). Glucosinolates. Chapter 8 - Insect detoxification of glucosinolates and their hydrolysis products. Adv. Bot. Res. 80, 199–245.
Jeschke, V., Zalucki, J. M., Raguschke, B., Gershenzon, J., Heckel, D. G., Zalucki, M. P., et al. (2021). So much for glucosinolates: A generalist does survive and develop on Brassicas, but at what cost? Plants 10:962. doi: 10.3390/plants10050962
Kant, M. R., Jonckheere, W., Knegt, B., Lemos, F., Liu, J., Schimmel, B. C. J., et al. (2015). Mechanisms and ecological consequences of plant defence induction and suppression in herbivore communities. Ann. Bot. 115, 1015–1051. doi: 10.1093/aob/mcv054
Karban, R., Karban, C., Huntzinger, M., Pearse, I., and Crutsinger, G. (2010). Diet mixing enhances the performance of a generalist caterpillar, Platyprepia virginalis. Ecol. Entomol. 35, 92–99. doi: 10.1111/j.1365-2311.2009.01162.x
Kassambara, A. (2016). Survminer: Drawing Survival Curves Using ‘Ggplot’. Available online at: http://www.sthda.com/english/wiki/survminer (accessed February 28, 2022).
Katsanis, A., Rasmann, S., and Mooney, K. A. (2016). Herbivore diet breadth and host plant defense mediate the tri-trophic effects of plant toxins on multiple coccinellid predators. PLoS One 11:e0155716. doi: 10.1371/journal.pone.0155716
Kim, J. H., Lee, B. W., Schroeder, F. C., and Jander, G. (2008). Identification of indole glucosinolate breakdown products with antifeedant effects on Myzus persicae (green peach aphid). Plant J. 54, 1015–1026. doi: 10.1111/j.1365-313X.2008.03476.x
Kumar, V., Sharma, A., Kaur, R., Thukral, A. K., Bhardwaj, R., and Ahmad, P. (2017). Differential distribution of amino acids in plants. Amino Acids 49, 821–869. doi: 10.1007/s00726-017-2401-x
Liou, C. S., Sirk, S. J., Diaz, C. A. C., Klein, A. P., Fischer, C. R., Higginbottom, S. K., et al. (2020). A metabolic pathway for activation of dietary glucosinolates by a human gut symbiont. Cell 180, 717–729. doi: 10.1016/j.cell.2020.01.023
Liu, X. J., Wang, Y. L., Hoeflinger, J. L., Neme, B. P., Jeffery, E. H., and Miller, M. J. (2017). Dietary broccoli alters rat cecal microbiota to improve glucoraphanin hydrolysis to bioactive isothiocyanates. Nutrients 9:262. doi: 10.3390/nu9030262
Malinga, G. M., Valtonen, A., Lehtovaara, V. J., Rutaro, K., Opoke, R., Nyeko, P., et al. (2018). Mixed artificial diets enhance the developmental and reproductive performance of the edible grasshopper. Ruspolia differens (Orthoptera: Tettigoniidae). Appl. Entomol. Zool. 53, 237–242. doi: 10.1007/s13355-018-0548-x
Malka, O., Easson, M., Paetz, C., Gotz, M., Reichelt, M., Stein, B., et al. (2020). Glucosylation prevents plant defense activation in phloem-feeding insects. Nat. Chem. Biol. 16, 1420–1426. doi: 10.1038/s41589-020-00658-6
Malka, O., Shekhov, A., Reichelt, M., Gershenzon, J., Vassão, D. G., and Morin, S. (2016). Glucosinolate desulfation by the phloem-feeding insect Bemisia tabaci. J. Chem. Ecol. 42, 230–235. doi: 10.1007/s10886-016-0675-1
Mason, C. J. J., Ray, S., Davidson-Lowe, E., Ali, J., Luthe, D. S. S., and Felton, G. (2022). Plant nutrition influences resistant maize defense responses to the fall armyworm (Spodoptera frugiperda). Front. Ecol. Evol. 10:844274. doi: 10.3389/fevo.2022.844274
Meiners, T., Randlkofer, B., and Obermaier, E. (2006). Oviposition at low temperatures - late season negatively affects the leaf beetle Galeruca tanaceti (Coleoptera: Galerucinae) but not its specialised egg parasitoid Oomyzus galerucivorus (Hymenoptera: Eulophidae). Eur. J. Entomol. 103, 765–770. doi: 10.14411/eje.2006.103
Mody, K., Unsicker, S. B., and Linsenmair, K. E. (2007). Fitness related diet-mixing by intraspecific host-plant-switching of specialist insect herbivores. Ecology 88, 1012–1020. doi: 10.1890/06-1338
Müller, C., Agerbirk, N., Olsen, C. E., Boevé, J.-L., Schaffner, U., and Brakefield, P. M. (2001). Sequestration of host plant glucosinolates in the defensive hemolymph of the sawfly Athalia rosae. J. Chem. Ecol. 27, 2505–2516. doi: 10.1023/a:1013631616141
Mutlib, A. E., Dickenson, P., Chen, S. Y., Espina, R. J., Daniels, J. S., and Gan, L. S. (2002). Bioactivation of benzylamine to reactive intermediates in rodents: Formation of glutathione, glutamate, and peptide conjugates. Chem. Res. Toxicol. 15, 1190–1207. doi: 10.1021/tx020063q
Nersesian, C. L., Banks, P. B., Simpson, S. J., and McArthur, C. (2012). Mixing nutrients mitigates the intake constraints of a plant toxin in a generalist herbivore. Behav. Ecol. 23, 879–888. doi: 10.1093/beheco/ars049
Neumann, J. M., Niehaus, K., Neumann, N., Knobloch, H. C., Bremmer, F., Krafft, U., et al. (2021). A new technological approach in diagnostic pathology: Mass spectrometry imaging-based metabolomics for biomarker detection in urachal cancer. Laborat. Invest. 101, 1281–1288. doi: 10.1038/s41374-021-00612-7
Novoselov, A., Becker, T., Pauls, G., von Reuss, S. H., and Boland, W. (2015). Spodoptera littoralis detoxifies neurotoxic 3-nitropropanoic acid by conjugation with amino acids. Insect Biochem. Mol. Biol. 63, 97–103. doi: 10.1016/j.ibmb.2015.05.013
Obermaier, E., Heisswolf, A., Pöthke, H. J., Randlkofer, B., and Meiners, T. (2008). Plant architecture and vegetation structure: Two ways for insect herbivores to escape parasitism. Eur. J. Entomol. 105, 233–240. doi: 10.14411/eje.2008.033
Pentzold, S., Zagrobelny, M., Roelsgaard, P. S., Møller, B. L., and Bak, S. (2014a). The multiple strategies of an insect herbivore to overcome plant cyanogenic glucoside defence. PLoS One 9:e91337. doi: 10.1371/journal.pone.0091337
Pentzold, S., Zagrobelny, M., Rook, F., and Bak, S. (2014b). How insects overcome two-component plant chemical defence: Plant beta-glucosidases as the main target for herbivore adaptation. Biol. Rev. 89, 531–551. doi: 10.1111/brv.12066
Ratzka, A., Vogel, H., Kliebenstein, D. J., Mitchell-Olds, T., and Kroymann, J. (2002). Disarming the mustard oil bomb. Proc. Natl. Acad. Sci. U.S.A. 99, 11223–11228. doi: 10.1073/pnas.172112899
R Core Team (2021). R: a Language and Environment for Statistical Computing. Vienna: R Foundation for statistical computing. Available online at: https://www.R-project.org/
Roditakis, E., and Roditakis, N. E. (2006). First record of Galeruca tanaceti in organic Origanum vulgare in Crete. Phytoparasitica 34, 486–487. doi: 10.1007/BF02981203
Rossi, M., Ott, S. R., and Niven, J. E. (2020). Malpighamoeba infection compromises fluid secretion and P-glycoprotein detoxification in Malpighian tubules. Sci. Rep. 10:15953. doi: 10.1038/s41598-020-72598-z
Ruttkies, C., Schymanski, E. L., Wolf, S., Hollender, J., and Neumann, S. (2016). MetFrag relaunched: Incorporating strategies beyond in silico fragmentation. J. Cheminform. 8:3. doi: 10.1186/s13321-016-0115-9
Saha, D., Mukhopadhyay, A., and Bahadur, M. (2012). Effect of host plants on fitness traits and detoxifying enzymes activity of Helopeltis theivora, a major sucking insect pest of tea. Phytoparasitica 40, 433–444. doi: 10.1007/s12600-012-0244-2
Schramm, K., Vassão, D. G., Reichelt, M., Gershenzon, J., and Wittstock, U. (2012). Metabolism of glucosinolate-derived isothiocyanates to glutathione conjugates in generalist lepidopteran herbivores. Insect Biochem. Mol. Biol 42, 174–182. doi: 10.1016/j.ibmb.2011.12.002
Scriber, J. M., and Slansky, F. (1981). The nutritional ecology of immature insects. Ann. Rev. Entomol. 26, 183–211. doi: 10.1146/annurev.en.26.010181.001151
Sikorska-Zimny, K., and Beneduce, L. (2021). The metabolism of glucosinolates by gut microbiota. Nutrients 13:2750. doi: 10.3390/nu13082750
Simpson, S. J., Clissold, F. J., Lihoreau, M., Ponton, F., Wilder, S. M., and Raubenheimer, D. (2015). Recent advances in the integrative nutrition of arthropods. Ann. Rev. Entomol. 60, 293–311. doi: 10.1146/annurev-ento-010814-020917
Singer, M. S., and Bernays, E. A. (2003). Understanding omnivory needs a behavioral perspective. Ecology 84, 2532–2537. doi: 10.1890/02-0397
Singh, K. I., and Singh, A. (2021). Plant-Pest Interactions: From Molecular Mechanisms To Chemical Ecology. London: Springer Nature Singapore Pte Ltd, doi: 10.1007/978-981-15-2467-7
Sporer, T., Kornig, J., Wielsch, N., Gebauer-Jung, S., Reichelt, M., Hupfer, Y., et al. (2021). Hijacking the mustard-oil bomb: How a glucosinolate-sequestering flea beetle copes with plant myrosinases. Front. Plant Sci. 12:645030. doi: 10.3389/fpls.2021.645030
Stauber, E. J., Kuczka, P., van Ohlen, M., Vogt, B., Janowitz, T., Piotrowski, M., et al. (2012). Turning the ‘mustard oil bomb’ into a ‘cyanide bomb’: Aromatic glucosinolate metabolism in a specialist insect herbivore. PLoS One 7:e35545. doi: 10.1371/journal.pone.0035545
Therneau, T. M., and Grambsch, P. M. (2000). Modeling Survival Data: Extending the Cox Model. New York, NY: Springer.
Tiku, A. R. (2021). “Direct and indirect defence against insects,” in Plant-Pest Interactions: From Molecular Mechanisms To Chemical Ecology, eds I. K. Singh and A. Singh (Berlin: Springer), 157–192.
Tremmel, M., and Müller, C. (2013). The consequences of alternating diet on performance and food preferences of a specialist leaf beetle. J. Insect Physiol. 59, 840–847. doi: 10.1016/j.jinsphys.2013.05.009
Tremmel, M., and Müller, C. (2014). Diet dependent experience and physiological state shape the behavior of a generalist herbivore. Physiol. Behav. 129, 95–103. doi: 10.1016/j.physbeh.2014.02.030
Unsicker, S. B., Oswald, A., Kohler, G., and Weisser, W. W. (2008). Complementarity effects through dietary mixing enhance the performance of a generalist insect herbivore. Oecologia 156, 313–324. doi: 10.1007/s00442-008-0973-6
Vergara, F., Svatos, A., Schneider, B., Reichelt, M., Gershenzon, J., and Wittstock, U. (2006). Glycine conjugates in a lepidopteran insect herbivore - The metabolism of benzylglucosinolate in the cabbage white butterfly, Pieris rapae. ChemBioChem 7, 1982–1989. doi: 10.1002/cbic.200600280
Wadleigh, R. W., and Yu, S. J. (1988). Detoxification of isothiocynante allelochemicals by glutathione transferase in three lepidopterous species. J. Chem. Ecol. 14, 1279–1288. doi: 10.1007/bf01019352
Wang, P., Vassao, D. G., Raguschke, B., Furlong, M. J., and Zalucki, M. P. (2021). Balancing nutrients in a toxic environment: The challenge of eating. Insect Sci. 29, 289–303. doi: 10.1111/1744-7917.12923
Welte, C. U., Rosengarten, J. F., de Graaf, R. M., and Jetten, M. S. M. (2016b). SaxA-mediated isothiocyanate metabolism in phytopathogenic pectobacteria. Appl. Environ. Microbiol. 82, 2372–2379. doi: 10.1128/aem.04054-15
Welte, C. U., de Graaf, R. M., van den Bosch, T. J. M., Op den Camp, H. J. M., van Dam, N. M., and Jetten, M. S. M. (2016a). Plasmids from the gut microbiome of cabbage root fly larvae encode SaxA that catalyses the conversion of the plant toxin 2-phenylethyl isothiocyanate. Environ. Microbiol. 18, 1379–1390. doi: 10.1111/1462-2920.12997
Wickham, H. (2022). Stringr: Simple, Consistent Wrappers for Common String Operations. Available online at: http://stringr.tidyverse.org, https://github.com/tidyverse/stringr (accessed February 28, 2022).
Winde, I., and Wittstock, U. (2011). Insect herbivore counteradaptations to the plant glucosinolate-myrosinase system. Phytochemistry 72, 1566–1575. doi: 10.1016/j.phytochem.2011.01.016
Wittstock, U., Agerbirk, N., Stauber, E. J., Olsen, C. E., Hippler, M., Mitchell-Olds, T., et al. (2004). Successful herbivore attack due to metabolic diversion of a plant chemical defense. Proc. Natl. Acad. Sci. U.S.A. 101, 4859–4864. doi: 10.1073/pnas.030800710
Wittstock, U., Kurzbach, E., Herfurth, A. M., and Stauber, E. J. (2016). Glucosinolate breakdown. Adv. Bot. Res. 80, 125–169. doi: 10.1016/bs.abr.2016.06.006
Yang, Z. L., Nour-Eldin, H. H., Hanniger, S., Reichelt, M., Crocoll, C., Seitz, F., et al. (2021). Sugar transporters enable a leaf beetle to accumulate plant defense compounds. Nat. Commun. 12:2658. doi: 10.1038/s41467-021-22982-8
Yu, S. J. (2008). “Detoxification mechanisms in insects,” in Encyclopedia of Entomology, ed. J. L. Capinera (Dordrecht: Springer), 1187–1201. doi: 10.1007/978-1-4020-6359-6
Keywords: glucosinolate-myrosinase system, metabolism, polyphagous herbivore, performance, detoxification
Citation: Friedrichs J, Schweiger R, Geisler S, Neumann JM, Sadzik SJM, Niehaus K and Müller C (2022) Development of a polyphagous leaf beetle on different host plant species and its detoxification of glucosinolates. Front. Ecol. Evol. 10:960850. doi: 10.3389/fevo.2022.960850
Received: 03 June 2022; Accepted: 22 July 2022;
Published: 08 August 2022.
Edited by:
Dani Lucas-Barbosa, Research Institute of Organic Agriculture (FiBL), SwitzerlandReviewed by:
Franziska S. Hanschen, Leibniz Institute of Vegetable and Ornamental Crops, GermanyCopyright © 2022 Friedrichs, Schweiger, Geisler, Neumann, Sadzik, Niehaus and Müller. This is an open-access article distributed under the terms of the Creative Commons Attribution License (CC BY). The use, distribution or reproduction in other forums is permitted, provided the original author(s) and the copyright owner(s) are credited and that the original publication in this journal is cited, in accordance with accepted academic practice. No use, distribution or reproduction is permitted which does not comply with these terms.
*Correspondence: Caroline Müller, Y2Fyb2xpbmUubXVlbGxlckB1bmktYmllbGVmZWxkLmRl
Disclaimer: All claims expressed in this article are solely those of the authors and do not necessarily represent those of their affiliated organizations, or those of the publisher, the editors and the reviewers. Any product that may be evaluated in this article or claim that may be made by its manufacturer is not guaranteed or endorsed by the publisher.
Research integrity at Frontiers
Learn more about the work of our research integrity team to safeguard the quality of each article we publish.