- 1Paleontological Institute and Museum, University of Zurich, Zurich, Switzerland
- 2Department of Biosciences, Swansea University, Swansea, United Kingdom
- 3Smithsonian Tropical Research Institution, Panama City, Panama
- 4Royal Botanic Gardens, Kew, Richmond, United Kingdom
- 5Gothenburg Global Biodiversity Centre, Department of Biological and Environmental Sciences, University of Gothenburg, Gothenburg, Sweden
- 6Department of Biology, University of Oxford, Oxford, United Kingdom
Halting biodiversity loss under growing anthropogenic pressure is arguably the greatest environmental challenge we face. Given that not all species are equally threatened and that resources are always limited, establishing robust prioritisation schemes is critical for implementing effective conservation actions. To this end, the International Union for Conservation of Nature (IUCN) Red List of Threatened Species has become a widely used source of information on species’ extinction risk. Various metrics have been proposed that combine IUCN status with different aspects of biodiversity to identify conservation priorities. However, current strategies do not take full advantage of palaeontological data, with conservation palaeobiology often focussing on the near-time fossil record (the last 2 million years). Here, we make a case for the value of the deep-time (over 2 million years ago), as it can offer tangible parallels with today’s biodiversity crisis and inform on the intrinsic traits that make species prone to extinction. As such, palaeontological data holds great predictive power, which could be harnessed to flag species likely to be threatened but that are currently too poorly known to be identified as such. Finally, we identify key IUCN-based prioritisation metrics and outline opportunities for integrating palaeontological data to validate their implementation. Although the human signal of the current extinction crisis makes direct comparisons with the geological past challenging, the deep-time fossil record has more to offer to conservation than is currently recognised.
Introduction
Anthropogenic pressures are driving a shockingly large number of species to extinction (Díaz et al., 2019a), suggesting we have entered a sixth mass extinction event, comparable in magnitude with the other five that shaped the history of life (Barnosky et al., 2011; Ceballos et al., 2015). Because resources for conservation are limited, the preservation of biodiversity requires effective strategies, including the prioritisation of species and habitats.
The International Union for Conservation of Nature (IUCN) Red List of Threatened Species1 provides a useful framework that categorises species according to their extinction risk (Mace and Lande, 1991; Betts et al., 2020). To further identify the species or areas for which extinction will result in the most significant losses, various conservation metrics have been proposed that combine IUCN categories with different prioritisation criteria. Because these tools are mostly focussed on the present state of biodiversity, current conservation strategies are generally reactive, operating under near-emergency conditions (Avise, 2005).
Another dimension, which is rarely incorporated in conservation prioritisation, is the deep geological past–“deep-time”–which, in the context of this article, contrasts with “near-time” [the last 2 million years (myrs); Dietl et al., 2015]. Logically, conservation needs to be forward-focussed but usually ignores that the vast majority of species that have ever lived–over 99%–have already gone extinct (Jablonski, 2004). Because most extinctions have taken place over geological timescales, the deep-time fossil record represents our best source of information on how, when, and why biodiversity changes through time and space. Nevertheless, the usefulness of deep-time palaeontology in conservation is limited not only by the inherent incompleteness of the fossil record, which worsens as we look deeper into geological time (Raup, 1972; but see Pimiento and Benton, 2020) but also by striking differences in pace, patterns, and scale relative to the rapid, human-driven loss of species we witness today. While these challenges call for caution in extrapolating past insights into future predictions, we argue that the potential of deep-time palaeontology to aid conservation is high and should not be readily overlooked.
Here, we make a case for the potential of the deep-time fossil record as it offers tangible parallels with today’s biodiversity crisis and can inform conservation theory, policy, and practice on species’ intrinsic vulnerability to extinction. We further list the key metrics currently used in conservation prioritisation that explicitly incorporate IUCN statuses and provide suggestions for opportunities to integrate palaeontological data into these metrics. While the implementation gap between deep-time palaeontology and conservation is significant, increasingly rich datasets and novel analytical frameworks offer exciting opportunities for bridging this divide.
The potential of deep-time palaeontology to aid conservation
Conservation palaeobiology uses geo-historical data to offer a long-term perspective on biodiversity changes in the face of human impacts (Dietl and Flessa, 2011; Dietl et al., 2015). Advances in this field have successfully informed restoration efforts of, for example, forests in Hawaii (Burney et al., 2001), the Everglades in Florida (Volety et al., 2009), and tortoise populations on islands (Hansen, 2010). However, most cases in which palaeobiology has impacted conservation, including those listed above, pertain to research focussing on near-time, with the last ∼130,000 years holding particular value and receiving most attention (e.g., Fordham et al., 2020).
As an important complement to near-time studies, the deep-time fossil record can offer informative parallels with today’s biodiversity and climate crises, not only because global temperatures are approaching the maximum experienced in the last 1.2 million years (Steffen et al., 2018) but also because many drastic environmental changes taking place today are comparable in at least one aspect with events recorded over deep timescales (Figure 1). For instance, the collision of an asteroid outside the Yucatán coast in Mexico around 66 Ma is somewhat comparable with the catastrophic effects of deforestation and depletion of natural resources caused by manifold human impacts on the environment, particularly since the Great Acceleration from the 1950s onwards (Figure 1A; Steffen et al., 2015). Similarly, anthropogenic global warming is to some extent comparable with historical “hyperthermals” in the geological record, most notably the Paleocene-Eocene Thermal Maximum (PETM) some 56 million years ago [Ma] (Zachos et al., 2008; Gingerich, 2019; Figure 1B). The extinction of around 70% of pelagic shark diversity in the early Miocene (∼19 Ma) mirrors the level of extinction they face today due to overfishing (Figure 1C; Pimiento and Pyenson, 2021; Sibert and Rubin, 2021). The wetlands that covered parts of western Amazonia in the Miocene (∼18 and ∼14 Ma; Jaramillo et al., 2017) causing pronounced biological changes in the region (Wesselingh et al., 2010; Salas-Gismondi et al., 2015), could be compared with the flooding caused by mega dams in Amazonia today (Figure 1D). The Messinian Salinity Crisis event, which resulted in the drying out of the Mediterranean Sea during the late Miocene (∼5 Ma), likely due to tectonic activity, can be compared to the drying of the Aral Sea as a consequence of cotton production (Figure 1E; Gupta, 2020). Finally, the Great American Biotic Interchange of species between South and North America following the emergence of the Panama Isthmus, which for mammals was mainly triggered at 2.5 Ma (Bacon et al., 2015; Carrillo et al., 2020), is comparable with the many recent introductions of invasive species, such as the European beaver causing an “invasive meltdown” in the Navarino Island of southern Chile (Figure 1F; Crego et al., 2016).
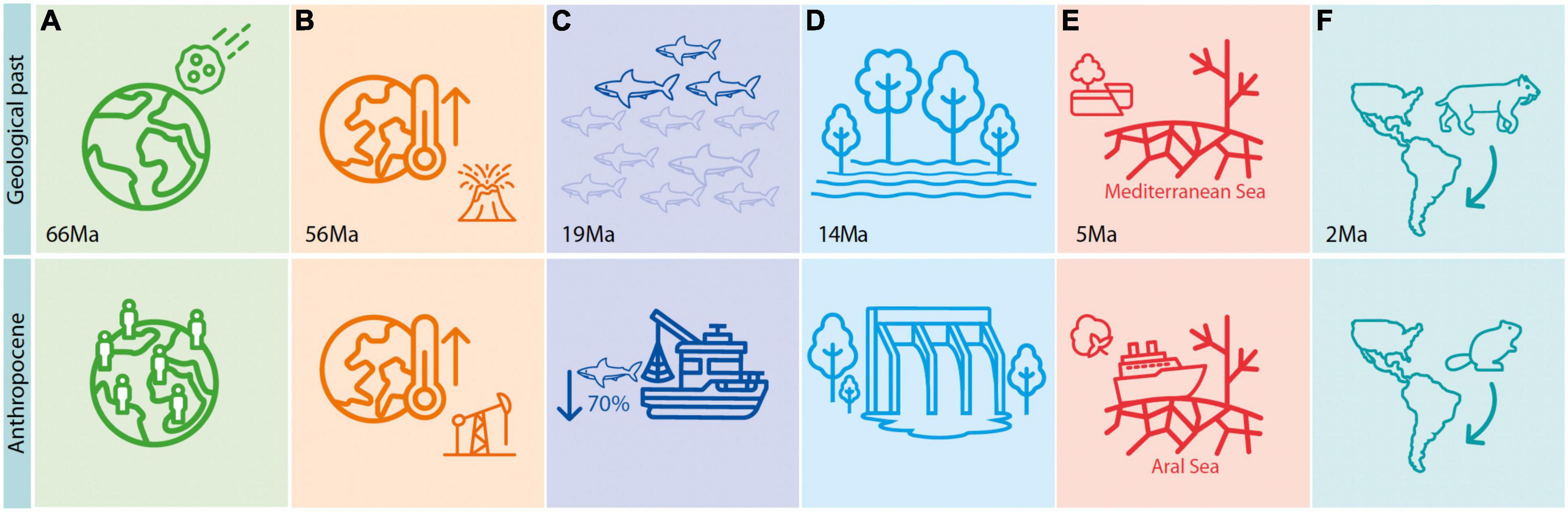
Figure 1. Parallels between deep-time events and anthropogenic changes, which are comparable in at least one aspect, such as size of the area affected or magnitude of change. (A) Asteroid collision ∼66 Ma vs. Great Acceleration from the 1950s onwards. (B) Palaeocene-Eocene Thermal Maximum vs. on-going global warming. (C) Extinction of pelagic sharks in the early Miocene vs. extinction levels today due to overfishing. (D) Wetlands covering parts of western Amazonia in the Miocene vs. flooding caused by mega dams today. (E) Drying out of the Mediterranean Sea during the late Miocene vs. drying of the Aral Sea as a consequence of cotton production. (F) Great American Biotic Interchange between South and North America ∼2.5 Ma vs. European beaver introduction in southern Chile.
Although the putative parallels mentioned above can be debated and do not represent a comprehensive list of events, they show that some deep-time events are comparable with human-driven stressors, although the pace (and sometimes geographic and taxonomic scale) at which humans have affected biodiversity is generally far greater. From a conservation management perspective, these parallels can inform the likely long-term response of species and ecosystems to abiotic stressors (Fordham et al., 2020). For example, the recovery pace, sequence, and timing of establishment for complex plant communities after the mass extinction event at 66 Ma (Figure 1A; Carvalho et al., 2021) could inform reforestation programmes in regions highly degraded by human activities. The body size reduction in mammals following the high temperatures of the PETM (Figure 1B; Secord et al., 2012) could be used to predict changes in the composition and function of future communities in response to climate change. Understanding the mechanisms of extinction of pelagic sharks in the early Miocene (Figure 1C; Sibert and Rubin, 2021) could reveal the abiotic factors that potentially interact with overfishing to cause the decline of sharks in the open ocean, thereby informing mitigation actions. The recorded drowning or draining of vast areas in Amazonia (Figure 1D; Antonelli et al., 2009) and the Mediterranean (Figure 1E; Van Der Made et al., 2006) could inform the long-term consequences of large infrastructural projects such as for energy production or agriculture; and on likely effects on the diversity, distribution, and migration of species. Finally, the general direction of biological invasions, from larger and more diverse areas to smaller and less diverse ones (Figure 1F; Beard, 1998), could help predict where invasions are likely to take place following climate-driven range shifts. The deep-time, therefore, represents a valuable source of information to anticipate the response of species to environmental conditions outside human experiences and possibly even beyond the survival of our species. Deep-time insights should, however, be interpreted with caution, since they are limited to the broad aspects of species responses and because the parallels between the deep geological past and today may only be applicable to certain clades or periods of time.
Incorporating the fossil record in conservation prioritisation metrics
The IUCN is widely influential in conservation practice today. Using the best available scientific information, standardised methods, and rigorous criteria, species are assigned to one of nine extinction risk categories for the IUCN Red List of Threatened Species: Not Evaluated (NE), Data Deficient (DD), Least Concern (LC), Near Threatened (NT), Vulnerable (VU), Endangered (EN), Critically Endangered (CR), Extinct (EX), and Extinct in the Wild (EW) (Rodrigues et al., 2006; IUCN, 2016a). The criteria used to assess the status of a species include population decline, restricted range, small population size, and quantitative extinction risk assessments (IUCN, 2016a; Rodrigues et al., 2019). Because these criteria are based on relatively short-term trends, using fossil and historical records has been proposed to shift the ecological baselines used to assess extinction risk (Rodrigues et al., 2019). Indeed, some LC species (e.g., the grey whale, Eschrichtius robustus) have shown shrinking ranges when longer-term trends are considered (Rodrigues et al., 2018). As such, historical ranges are now being accounted for in recovery assessments under the IUCN Green List (Akçakaya et al., 2018).
The fossil record could further aid conservation prioritisation schemes by providing fundamental information on extinction selectivity and predictability. Palaeontological research at different timescales, but especially over the deep-time, has shown that the extent to which species are prone to extinction is largely linked to their intrinsic traits, such as geographic range, body size, diet, and thermoregulation (Willis et al., 2008; Harnik et al., 2012; Finnegan et al., 2015; Pimiento et al., 2017; Cantalapiedra et al., 2021). For some clades, intrinsic traits can predict extinction risk in both fossil and extant taxa (Tietje and Rödel, 2018) and better than abiotic factors alone (Boyer, 2010). Although the link between intrinsic traits and extinction risk is also apparent when studying modern species (e.g., Leao et al., 2014; Ripple et al., 2017; Dulvy et al., 2021), current (neontological) data can be restricted to human scales (i.e., ecological timescales, small geographic extent, or narrow taxonomic coverage). The deep-time fossil record has the potential to complement biological studies by providing data on extinction selectivity over much longer, evolutionary scales. This broader and longer perspective on threatened clades is unique to the fossil record. As such, taking advantage of the phylogenetic conservatism seen in traits associated with extinction risk (Purvis, 2008; Roy et al., 2009), the fossil record could be used to flag species that hold traits associated with extinction risk in their clade, but that are too poorly known from neontological data to be categorised as threatened (e.g., DD or NE species). To that end, and given the urgency of the current extinction crisis, we propose that the palaeontological community engages more closely with the IUCN to discuss the possibility of a palaeontologically informed threat category, or the addition of palaeontological information into existing categories, to identify species that are potentially vulnerable in this context and therefore merit further evaluation. Although we recognise this would be a complex task to formalise and standardise across taxa and life forms, we believe that such discussions could inspire future research to enhance conservation actions.
In addition to its potential to flag species intrinsically vulnerable to extinction, a deep-time approach has the potential of validating an array of existing prioritisation metrics. There are at least six metrics that identify species for which extinction will result in irreplaceable losses, and areas holding particularly important species (Figure 2). These metrics combine IUCN statuses with different prioritisation criteria, namely evolutionary distinctiveness, ecological importance, and restricted distribution. Below we summarise them and outline opportunities to incorporate the fossil record, especially over deep timescales.
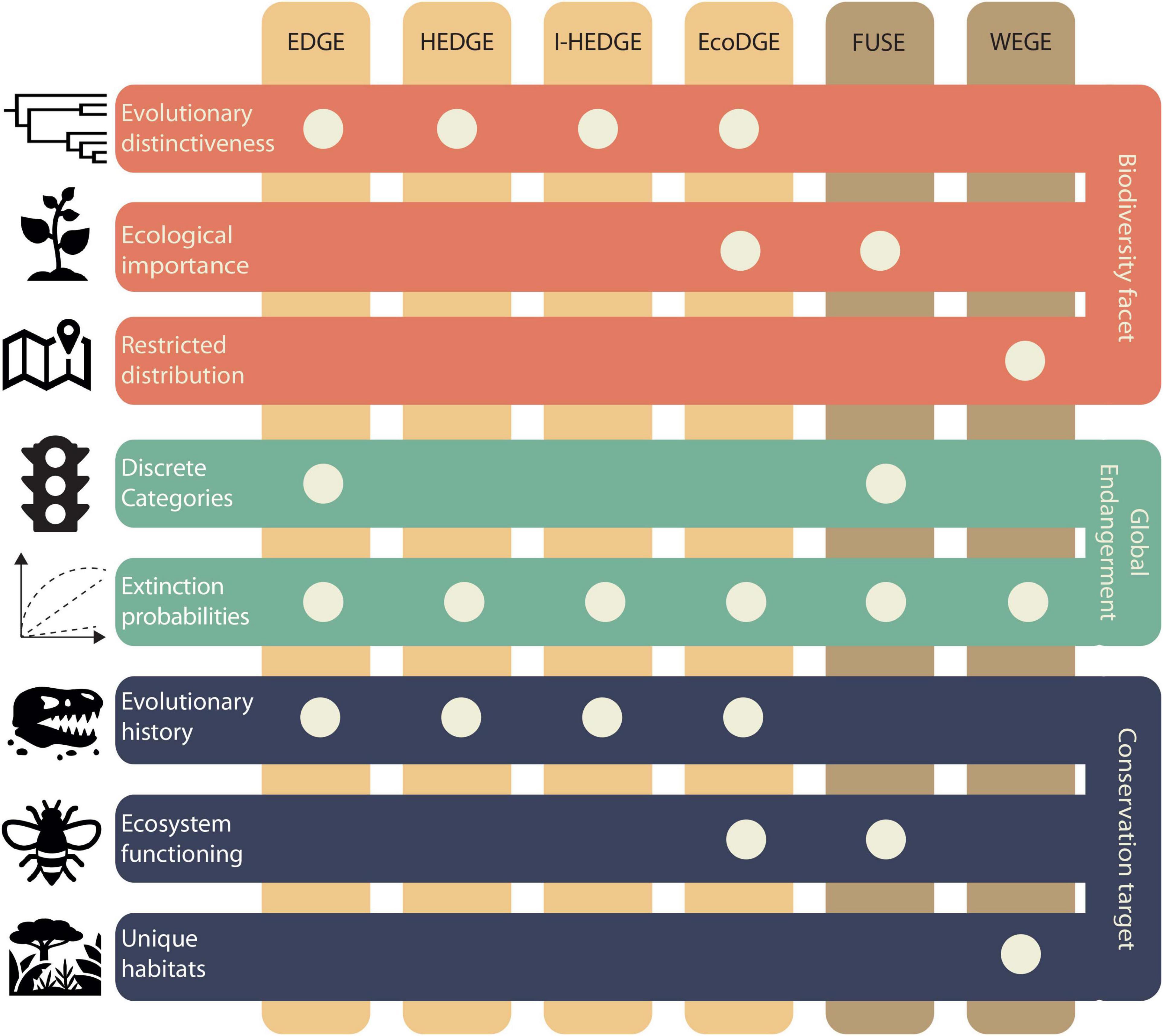
Figure 2. International Union for Conservation of Nature (IUCN)-based conservation prioritisation metrics. Orange horizontal bars show the prioritisation criteria that are combined with global endangerment (GE). Green bars show how GE is used in each metric (Table 1). Blue bars show their conservation prioritisation target.
Evolutionary distinctiveness
Isaac et al. (2007) proposed the evolutionarily distinct and globally endangered metric (EDGE; Figure 2) to identify species that disproportionately contribute to the evolutionary history of their clade. Given a phylogeny, evolutionary distinctiveness (ED) is calculated (Table 1) and then combined with IUCN statuses [also called global endangerment (GE)] in a log scale (Isaac et al., 2007; Figure 2). The EDGE metric is widely used, with the IUCN Phylogenetic Diversity Task Force2 guiding its inclusion in conservation strategies. It has been applied to a wide array of clades, including mammals (Isaac et al., 2007), amphibians (Isaac et al., 2012), birds (Jetz et al., 2014), squamates (Tonini et al., 2016), sharks, rays, chimaeras (Stein et al., 2018), and gymnosperms (Forest et al., 2018).
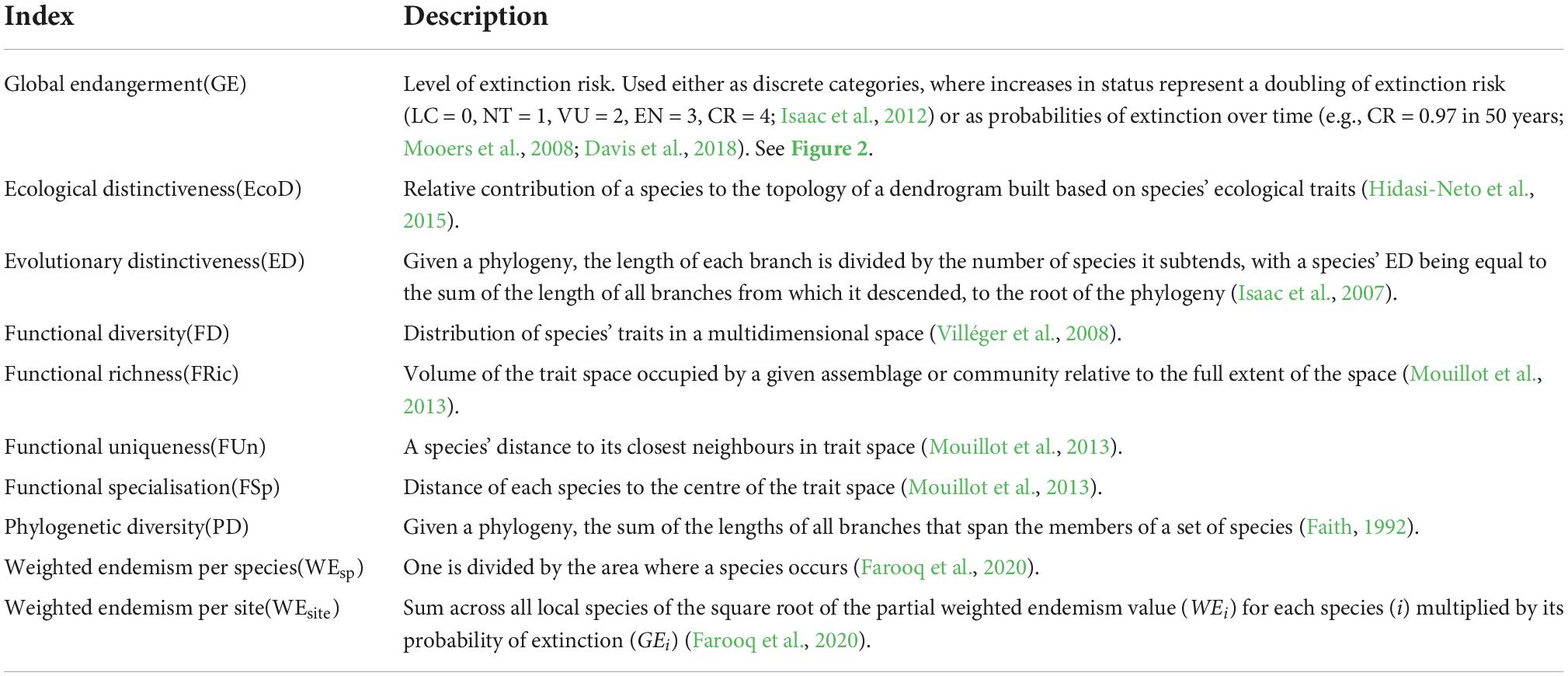
Table 1. Glossary of indices and abbreviations used in International Union for Conservation of Nature (IUCN)-based prioritisation metrics.
There are at least two other metrics that have been proposed with modifications to the EDGE index. The metric heightened evolutionary distinctiveness and globally endangered (HEDGE; Figure 2) quantifies how much a species is likely to contribute to future biodiversity (Steel et al., 2007). It consists of HED, which is the expected gain in phylogenetic diversity (PD; Table 1) if a given species is protected (Steel et al., 2007), which is then computed with GE (Table 1). Unlike EDGE, HEDGE is based on expected PD, thereby reflecting the opportunity to safeguard more evolutionary history in the future (Robuchon et al., 2021). Similarly, iteratively calculated HEDGE (I-HEDGE; Figure 2) is computed in iterative rounds where top HEDGE species are identified and “saved” by setting their extinction probability to near zero, and then recalculating HEDGE until all species have been “saved” (Jensen et al., 2016). Unlike HEDGE, I-HEDGE produces an optimal rank of species to protect while accounting for complementarity (i.e., the fact that if one species is protected, its sister should drop in value because the shared component of evolutionary diversity is now retained). Empirical applications include prosimian primates for HEDGE (Steel et al., 2007) and Galápagos tortoises for I-HEDGE (Jensen et al., 2016).
Although not widely exploited yet, the deep-time fossil record has begun to be incorporated into the ED framework. For instance, it has been shown that the inclusion of Early Cretaceous fossils (110 Ma) in the lungfish phylogeny increases the Australian lungfish’s distinctiveness (Cavin and Kemp, 2011). Furthermore, Bennett et al. (2019) tested whether the implementation of EDGE results in the preservation of “seeds” of future radiations where high ED leads to low ED (Table 1); or if, instead, it preserves the tail-ends of once-diverse clades, now “doomed” to extinction, where low ED results in high ED. They addressed this question by incorporating the mammalian fossil record into phylogenetic super-trees, computing per-clade ED across time slices, and modelling past ED as a function of future ED. They found that evolutionarily distinct taxa are neither seeds nor doomed. Instead, they are disproportionally represented by “living fossils” (with low origination and extinction rates), whereby high ED leads to even higher ED. As such, while preserving ED species today may not result in ensuring future radiations, it does preserve species doomed to extinction, at least for mammals. Applying an approach similar to that of Bennett et al. (2019) to different clades across realms would provide a more comprehensive assessment of the use of ED-based metrics, further determining if the results they found are specific for mammals, or if protecting ED taxa generally results in preserving other living fossils. The inclusion of the fossil record in phylogenetic trees can therefore allow us to determine the likely evolutionary consequences and benefits of conservation actions guided by the EDGE metric.
Palaeontological data could be further used to test how a clade’s PD changes following the extinction of species with high ED (Table 1). This could be done by building a phylogeny that includes both extant and extinct taxa, computing per-species ED and per-time PD, and quantifying the erosion of PD following the removal of extinct species with different levels of ED. This combined approach would also allow the quantification of changes in prioritisation rankings after hypothetically “saving” species with high ED. Unlike molecular trees composed of extant species only, the combination of extinct and extant taxa in phylogenies to calculate ED and PD could improve knowledge on how the extinction (and protection) of species with high ED can shape the evolutionary history of entire clades, thereby providing tools to validate or justify the use of EDGE- and HEDGE-informed conservation priorities today.
To undertake the studies envisioned above, a good understanding of the taxonomy and morphology of fossil taxa is required, which is only possible with primary palaeontological work (e.g., fieldwork, collections inspection, and comparative anatomy). Similarly, it is necessary to account for the uncertainties associated with fossil dating and taxonomic identifications. To that end, a new set of methods that rely on the fossilised date-birth process is particularly useful, which unifies neontological and palaeontological phylogenetics by combining morphology, DNA sequences, and stratigraphy (Wright et al., 2022). Therefore, the incorporation of the fossil record in the calculation of ED-based metrics, which requires primary palaeontological work and the use of analytical approaches to account for uncertainties, holds great potential to test hypotheses on the long-term implications of the extinction of species with high ED.
Ecological importance
Ecological distinctiveness is part of two additional IUCN-based metrics. The metric ecologically and evolutionarily distinct and globally endangered (EcoEDGE; Figure 2) computes ecological distinctiveness (EcoD; Table 1) using the same rationale as ED, but instead of phylogeny, it uses a functional dendrogram based on ecological traits. EcoD is then combined with ED and GE (Table 1 and Figure 2; Hidasi-Neto et al., 2015). Notably, the application of this metric has shown that EcoD is complementary to ED, rather than redundant (Hidasi-Neto et al., 2015). Functionally unique, specialised, and endangered (FUSE; Figure 2) uses a different approach. Although also inspired by EDGE, it aims to identify species disproportionately contributing to functional diversity, which is quantified based on a multidimensional trait space (i.e., defined by axes representing functional traits, along which species are placed according to their trait values; Table 1). This metric comprises two components: functional uniqueness (FUn; Table 1) thereby identifying species with low functional redundancy (ecologically dissimilar to others); and functional specialisation (FSp; Table 1), identifying species with extreme trait values, which delimit the volume of the trait space (i.e., functional richness, FRic; Table 1; Mouillot et al., 2013). In FUSE, FUn and FSp are computed per species, and combined with GE (Griffin et al., 2020; Pimiento et al., 2020a). Empirical applications of EcoEDGE and FUSE include terrestrial mammals and marine megafauna, respectively (Hidasi-Neto et al., 2015; Pimiento et al., 2020a).
The fossil record could inform ecological importance metrics in several ways. For instance, it could allow testing whether the implementation of the EcoEDGE metric results in the preservation of ecological- and/or ED over time. This could be achieved by incorporating fossils into phylogenetic trees and analogous functional dendrograms, and computing EcoD and ED (Table 1) to then quantify differences across time slices. That is, to model: (i) per-clade EcoD in ti as a function of EcoD in ti+1; (ii) EcoD in ti as a function of ED in ti+1; and (iii) ED in ti as a function of EcoD in ti+1, following Bennett et al. (2019). This approach would allow us to not only assess what facet of biodiversity (EcoD or ED; Table 1) is best preserved by implementing EcoEDGE, but also to test if the complementarity between EcoD and ED seen in some clades today (Hidasi-Neto et al., 2015; Cooke et al., 2020) is persistent over deep timescales. As such, the incorporation of fossils into phylogenetic trees and functional dendrograms can offer a framework on which to base predictions for the application of EcoEDGE to clades facing extinction.
Additionally, the fossil record could be used to assess the extent to which the preservation of ecologically distinct species results in higher functional diversity (Table 1) over time. This could be done by assembling a pool of fossil and extant taxa of different geological ages, building a trait space encompassing all species, quantifying per-species FUn and FSp, and computing functional richness (Table 1) at different time periods. This approach would account for the loss and gain of species over time, thereby testing if the implementation of the FUSE metric (which focusses on species with high FUn and FSp; Table 1) results in the preservation of a larger extent of trait values and, therefore, ecosystem functionality. Furthermore, the incorporation of fossils in the construction of functional spaces could enable testing of the order and importance of the different components of the FUSE metric. This could be done by assessing if the loss of species with high FUn (functionally redundant; Table 1) in the geological past preceded the extinction of those with high FSp. In other words, it would allow testing whether or not erosion inside the functional space affects its extent. Indeed, some extinction events have particularly affected functionally redundant species, leaving functional richness (Table 1) largely unaffected (Pimiento et al., 2020b). Understanding the response of the different components of the FUSE metric to extinctions would, in turn, allow the assessment of the role of past functional redundancy in shaping future ecological resilience. The fossil record has therefore the potential to not only help us evaluate the justification of conservation metrics focussed on ecological importance (i.e., EcoEDGE and FUSE; Figure 2), but also to test the ecological responses of communities to extinctions.
The incorporation of the fossil record into conservation metrics focussed on ecological importance (Figure 2) is, however, limited by the availability of trait data from extinct species. Assigning or measuring functional traits from incomplete or fragmentary specimens is challenging, especially when they lack extant relatives or ecological analogues. To support the studies suggested above, it is imperative that further research expands our understanding of the functional traits of fossil taxa. This will require a high level of expertise in specific clades, the cataloguing and digitisation of fossil records around the world, and authoritative and specialised taxonomic revisions that include both fossils and extant relatives. Despite these current limitations, many palaeontological studies have already compiled or analysed trait data and studied their functional diversity over deep timescales (e.g., Villéger et al., 2011; Leonard-Pingel et al., 2012; Aberhan and Kiessling, 2015; Pimiento et al., 2017, 2020b). Therefore, as fossil trait data become increasingly available (e.g., Raja et al., 2022), more opportunities will arise to include extinct taxa in the assessment of functional diversity over time, thereby allowing the implementation of EcoEDGE and FUSE metrics to be evaluated.
Geographic distribution
Species’ geographic range is considered in the metric weighted endemism and globally endangered (WEGE; Farooq et al., 2020). This metric ranks areas according to their biodiversity importance (Figure 2). It has been shown to highlight the same areas as the Key Biodiversity Areas methodology (IUCN, 2016b; KBA Standards and Appeals Committee, 2020), but in continuous scales instead of using a binary classification system, which greatly facilitates conservation decisions. Areas with narrowly distributed species and high extinction risk have the highest WEGE values. WEGE is computed by calculating the species’ weighted endemism (WE) and GE (Table 1) and is expressed per site. This metric has been applied to amphibians, mammals, and birds, thereby identifying WEGE hotspots in Central America, the Andes, West and East Africa, Eastern Madagascar, China, and Eastern Australia (Farooq et al., 2020).
The fossil record is already widely used to model past species’ geographic distributions (e.g., Varela et al., 2011). Furthermore, fossil occurrences can be combined with modern distribution data to expand the otherwise incomplete description of species’ ecological niches (e.g., Monsarrat et al., 2019), thereby enhancing predictions of range shifts in response to climate change. This application of the fossil record in biogeographic studies has important implications for conservation, including the identification of areas for reintroduction potential (Jarvie et al., 2021) and predictions of refugia (Greenstein and Pandolfi, 2008). Species distribution models using both fossil occurrences and current distributions could be used to extend the baselines to identify global and regional WEGE hotspots. Furthermore, these models can be used to test the extent to which range shifts due to climate change affect levels of endemism and therefore WEGE scores. For example, a species for which the range is expected to be reduced by half will be predicted to have a higher WE in the future relative to today (i.e., WEt+1 > WEt), even if its GE remained the same, resulting in an overall larger WEGE score. Finally, the fossil record could be used to infer WEGE hotspots across geological time periods, which in turn can allow an assessment of how conserved they are over time. The fossil record can therefore extend the baselines to identify WEGE hotspots, account for the role of climate-driven range shifts in current and future WEGE scores, and identify stable WEGE hotspots where conservation actions could be targeted.
To incorporate the fossil record into the WEGE framework, large datasets of fossil occurrences with spatial data are required. A number of databases are already available [e.g., the Palaeobiology Database3; the Neotoma Palaeoecology Database4; and PHYLACINE (Faurby et al., 2018)]. Although useful, these resources represent imperfect sets of data, with their quality and completeness varying across clades and time periods. As such, continually entering new data and critically evaluating existing datapoints, as well as using analytical approaches that account for the incompleteness and biases of the fossil record (e.g., Silvestro et al., 2016) are necessary activities. Therefore, the incorporation of the fossil record in the spatially explicit WEGE framework, which requires large amounts of palaeontological data and the use of analytical approaches to account for biases, holds great potential to extend the baselines in the identification of conservation prioritisation hotspots.
Prospects and limitations
The field of conservation palaeobiology, which includes scientists from multiple disciplines, regions, and specialisms5 has already made important advances in informing the conservation of species (Dietl and Flessa, 2011; Dietl et al., 2015; Fordham et al., 2020). However, the use of deep-time insights has remained largely hypothetical, mainly because the inherent limitations of the fossil record tend to exacerbate as we look deeper in geological time and because of the marked differences relative to the anthropogenic pressures species face today. As such, the usefulness of the deep-time in aiding conservation is restricted to coarse aspects of species responses and might be applicable to specific clades and time-periods. Despite these limitations, a deep-time approach can: (i) offer valuable parallels with today’s crisis, thereby informing on the long-term responses of species to environmental change (Figure 1); (ii) potentially allow identifying species likely to be threatened but not currently recognised as such; and (iii) enable testing current prioritisation metrics and expanding the baselines for the identification of spatial conservation priorities (Figure 2).
The integration of the deep-time into conservation, therefore, holds a largely unexplored potential to enrich and improve conservation. For this to be possible, it is critical to also advance our understanding of the fossil record of clades that are now considered to be threatened. Notwithstanding the rapid growth of palaeontological datasets, a deeper understanding of the phylogenetic relationships of extinct species, their life history, and ecological traits, as well as their distribution and climatic tolerance, is pivotal to bridging the gap between palaeontology and conservation. Further research is needed to incorporate the general directions and suggestions presented here for designing conservation prioritisation metrics that are ever more realistic, reliable, and useful.
Anthropogenic pressures, direct and indirect, are unequivocally the main drivers of biodiversity loss today (Díaz et al., 2019b). While the responsibility for this crisis lies predominantly with the wealthy nations and the burden is felt primarily by the poor (Saldanha, 2020; Gonzalez, 2021), it is of utmost urgency that all segments of society work collaboratively to safeguard the future of our planet’s rapidly disappearing species. We urge the scientific community to further consider palaeontology in this pursuit.
Data availability statement
The original contributions presented in this study are included in the article/supplementary material, further inquiries can be directed to the corresponding author.
Author contributions
CP conceived the idea and wrote the first draft with substantial input from AA. Both authors contributed intellectually and wrote the final version of this manuscript.
Funding
CP was funded by a PRIMA grant from the Swiss National Science Foundation (no. 185798) and AA acknowledges financial support from the Swedish Research Council (2019-05191), the Swedish Foundation for Strategic Environmental Research MISTRA (Project BioPath) and the Royal Botanic Gardens, Kew.
Acknowledgments
We thank Félix Forest, Ian Ondo, and Harith Farooq for providing feedback on earlier versions of the manuscript, Jeff Eden (RBG Kew) for professional support with Figure 1, Rhian Smith for science and language editing, and the editor and the reviewers for valuable feedback that helped us improve this article.
Conflict of interest
The authors declare that the research was conducted in the absence of any commercial or financial relationships that could be construed as a potential conflict of interest.
Publisher’s note
All claims expressed in this article are solely those of the authors and do not necessarily represent those of their affiliated organizations, or those of the publisher, the editors and the reviewers. Any product that may be evaluated in this article, or claim that may be made by its manufacturer, is not guaranteed or endorsed by the publisher.
Footnotes
- ^ www.iucnredlist.org
- ^ https://www.pdtf.org
- ^ https://paleobiodb.org
- ^ http://www.neotomadb.org
- ^ https://conservationpaleorcn.org/
References
Aberhan, M., and Kiessling, W. (2015). Persistent ecological shifts in marine molluscan assemblages across the end-Cretaceous mass extinction. Proc. Natl. Acad. Sci. U.S.A. 112, 7207–7212. doi: 10.1073/pnas.1422248112
Akçakaya, H. R., Bennett, E. L., Brooks, T. M., Grace, M. K., Heath, A., Hedges, S., et al. (2018). Quantifying species recovery and conservation success to develop an IUCN Green List of Species. Conserv. Biol. 32, 1128–1138. doi: 10.1111/cobi.13112
Antonelli, A., Nylander, J. A. A., Persson, C., and Sanmartín, I. (2009). Tracing the impact of the Andean uplift on Neotropical plant evolution. Proc. Natl. Acad. Sci. U.S.A. 106, 9749–9754. doi: 10.1073/pnas.0811421106
Avise, J. C. (2005). “Phylogenetic units and currencies above and below the species level,” in Phylogeny and Conservation, eds A. Purvis, J. L. Gittleman, and T. Brooks (Cambridge, MA: Cambridge University Press), 76–100. doi: 10.1017/CBO9780511614927.004
Bacon, C. D., Silvestro, D., Jaramillo, C., Smith, B. T., Chakrabarty, P., and Antonelli, A. (2015). Biological evidence supports an early and complex emergence of the Isthmus of Panama. Proc. Natl. Acad. Sci. U.S.A. 112, 6110–6115. doi: 10.1073/pnas.1423853112
Barnosky, A. D., Matzke, N., Tomiya, S., Wogan, G. O. U., Swartz, B., Quental, T. B., et al. (2011). Has the Earth’s sixth mass extinction already arrived? Nature 471, 51–57. doi: 10.1038/nature09678
Beard, K. C. (1998). East of Eden: Asia as an important center of taxonomic origination in mammalian evolution. Bull. Carnegie Museum Nat. Hist. 34, 5–39. doi: 10.5962/p.228609
Bennett, D. J., Sutton, M. D., and Turvey, S. T. (2019). How the past impacts the future: modelling the performance of evolutionarily distinct mammals through time. Philos. Trans. R. Soc. Lond. B Biol. Sci. 374:20190210. doi: 10.1098/rstb.2019.0210
Betts, J., Young, R. P., Hilton-Taylor, C., Hoffmann, M., Rodríguez, J. P., Stuart, S. N., et al. (2020). A framework for evaluating the impact of the IUCN Red List of threatened species. Conserv. Biol. 34, 632–643. doi: 10.1111/cobi.13454
Boyer, A. G. (2010). Consistent ecological selectivity through time in Pacific island avian extinctions. Conserv. Biol. 24, 511–519. doi: 10.1111/j.1523-1739.2009.01341.x
Burney, D. A., James, H. F., Burney, L. P., Olson, S. L., Kikuchi, W., Wagner, W. L., et al. (2001). Fossil evidence for a diverse biota from Kaua ‘i and its transformation since human arrival. Ecol. Monogr. 71, 615–641. doi: 10.1890/0012-9615(2001)071[0615:FEFADB]2.0.CO;2
Cantalapiedra, J. L., Sanisidro, Ó, Zhang, H., Alberdi, M. T., Prado, J. L., Blanco, F., et al. (2021). The rise and fall of proboscidean ecological diversity. Nat. Ecol. Evol. 5, 1266–1272. doi: 10.1038/s41559-021-01498-w
Carrillo, J. D., Faurby, S., Silvestro, D., Zizka, A., Jaramillo, C., Bacon, C. D., et al. (2020). Disproportionate extinction of South American mammals drove the asymmetry of the Great American Biotic Interchange. Proc. Natl. Acad. Sci. U.S.A. 117, 26281–26287. doi: 10.1073/pnas.2009397117
Carvalho, M. R., Jaramillo, C., De La Parra, F., Caballero-Rodríguez, D., Herrera, F., Wing, S., et al. (2021). Extinction at the end-Cretaceous and the origin of modern Neotropical rainforests. Science 372, 63–68. doi: 10.1126/science.abf1969
Cavin, L., and Kemp, A. (2011). The impact of fossils on the evolutionary distinctiveness and conservation status of the Australian lungfish. Biol. Conserv. 144, 3140–3142. doi: 10.1016/j.biocon.2011.08.014
Ceballos, G., Ehrlich, P. R., Barnosky, A. D., Garcia, A., Pringle, R. M., and Palmer, T. M. (2015). Accelerated modern human-induced species losses: entering the sixth mass extinction. Sci. Adv. 1:e1400253. doi: 10.1126/sciadv.1400253
Cooke, R. S., Eigenbrod, F., and Bates, A. E. (2020). Ecological distinctiveness of birds and mammals at the global scale. Glob. Ecol. Conserv. 22:e00970. doi: 10.1016/j.gecco.2020.e00970
Crego, R. D., Jiménez, J. E., and Rozzi, R. (2016). A synergistic trio of invasive mammals? Facilitative interactions among beavers, muskrats, and mink at the southern end of the Americas. Biol. Invasions 18, 1923–1938. doi: 10.1007/s10530-016-1135-0
Davis, M., Faurby, S., and Svenning, J.-C. (2018). Mammal diversity will take millions of years to recover from the current biodiversity crisis. Proc. Natl. Acad. Sci. U.S.A. 115, 11262–11267. doi: 10.1073/pnas.1804906115
Díaz, S., Settele, J., Brondízio, E. S., Ngo, H. T., Guèze, M., Agard, J., et al. (2019a). Summary for Policymakers of the Global Assessment Report on Biodiversity and Ecosystem Services of the Intergovernmental Science-Policy Platform on Biodiversity and Ecosystem Services. Bonn: Intergovernmental Science-Policy Platform on Biodiversity and Ecosystem Services.
Díaz, S., Settele, J., Brondízio, E. S., Ngo, H. T., Agard, J., Arneth, A., et al. (2019b). Pervasive human-driven decline of life on Earth points to the need for transformative change. Science 366:eaax3100. doi: 10.1126/science.aax3100
Dietl, G. P., and Flessa, K. W. (2011). Conservation paleobiology: putting the dead to work. Trends Ecol. Evol. 26, 30–37. doi: 10.1016/j.tree.2010.09.010
Dietl, G. P., Kidwell, S. M., Brenner, M., Burney, D. A., Flessa, K. W., Jackson, S. T., et al. (2015). Conservation paleobiology: leveraging knowledge of the past to inform conservation and restoration. Annu. Rev. Earth Planet. Sci. 43, 79–103. doi: 10.1146/annurev-earth-040610-133349
Dulvy, N. K., Pacoureau, N., Rigby, C. L., Pollom, R. A., Jabado, R. W., Ebert, D. A., et al. (2021). Overfishing drives over one-third of all sharks and rays toward a global extinction crisis. Curr. Biol. 31, 4773–4787. doi: 10.1016/j.cub.2021.08.062
Faith, D. P. (1992). Conservation evaluation and phylogenetic diversity. Biol. Conserv. 61, 1–10. doi: 10.1016/0006-3207(92)91201-3
Farooq, H., Azevedo, J., Belluardo, F., Nanvonamuquitxo, C., Bennett, D., Moat, J., et al. (2020). WEGE: a new metric for ranking locations for biodiversity conservation. Divers. Distrib. 26, 1456–1466. doi: 10.1111/ddi.13148
Faurby, S., Davis, M., Pedersen, R. Ø, Schowanek, S. D., Antonelli, A., and Svenning, J.-C. (2018). PHYLACINE 1.2: the phylogenetic atlas of mammal macroecology. Ecology 99:2626. doi: 10.1002/ecy.2443
Finnegan, S., Anderson, S. C., Harnik, P. G., Simpson, C., Tittensor, D. P., Byrnes, J. E., et al. (2015). Paleontological baselines for evaluating extinction risk in the modern oceans. Science 348, 567–570. doi: 10.1126/science.aaa6635
Fordham, D. A., Jackson, S. T., Brown, S. C., Huntley, B., Brook, B. W., Dahl-Jensen, D., et al. (2020). Using paleo-archives to safeguard biodiversity under climate change. Science 369:eabc5654. doi: 10.1126/science.abc5654
Forest, F., Moat, J., Baloch, E., Brummitt, N. A., Bachman, S. P., Ickert-Bond, S., et al. (2018). Gymnosperms on the EDGE. Sci. Rep. 8:6053. doi: 10.1038/s41598-018-24365-4
Gingerich, P. D. (2019). Temporal scaling of carbon emission and accumulation rates: modern anthropogenic emissions compared to estimates of PETM onset accumulation. Paleoceanogr. Paleoclimatol. 34, 329–335. doi: 10.1029/2018PA003379
Gonzalez, C. G. (2021). Racial capitalism, climate justice, and climate displacement. Oñati Socio Legal Ser. 11, 108–147. doi: 10.35295/OSLS.IISL/0000-0000-0000-1137
Greenstein, B. J., and Pandolfi, J. M. (2008). Escaping the heat: range shifts of reef coral taxa in coastal Western Australia. Glob. Change Biol. 14, 513–528. doi: 10.1111/j.1365-2486.2007.01506.x
Griffin, J. N., Leprieur, F., Silvestro, D., Lefcheck, J. S., Albouy, C., Rasher, D. B., et al. (2020). Functionally unique, specialised, and endangered (FUSE) species: towards integrated metrics for the conservation prioritisation toolbox. bioRxiv [Preprint]. doi: 10.1101/2020.05.09.084871
Gupta, A. (2020). Shrinking of Aral Sea: an environmental disaster in Central Asia. Int. J. Hum. Arts Soc. Sci. 6, 162–170. doi: 10.20469/ijhss.6.20003-4
Hansen, D. M. (2010). “On the use of taxon substitutes in rewilding projects on islands,” in Islands and Evolution, eds V. Pérez-Mellado and C. Ramon (Mahón: Institut Menorquí d’Estudis), 111–146.
Harnik, P. G., Lotze, H. K., Anderson, S. C., Finkel, Z. V., Finnegan, S., Lindberg, D. R., et al. (2012). Extinctions in ancient and modern seas. Trends Ecol. Evol. 27, 608–617. doi: 10.1016/j.tree.2012.07.010
Hidasi-Neto, J., Loyola, R., and Cianciaruso, M. V. (2015). Global and local evolutionary and ecological distinctiveness of terrestrial mammals: identifying priorities across scales. Divers. Distrib. 21, 548–559. doi: 10.1111/ddi.12320
Isaac, N. J. B., Redding, D. W., Meredith, H. M., and Safi, K. (2012). Phylogenetically-informed priorities for amphibian conservation. PLoS One 7:e43912. doi: 10.1371/journal.pone.0043912
Isaac, N. J. B., Turvey, S. T., Collen, B., Waterman, C., and Baillie, J. E. M. (2007). Mammals on the EDGE: conservation priorities based on threat and phylogeny. PLoS One 2:e000296. doi: 10.1371/journal.pone.0000296
Jaramillo, C., Romero, I., D’apolito, C., Bayona, G., Duarte, E., Louwye, S., et al. (2017). Miocene flooding events of western Amazonia. Sci. Adv. 3:e1601693. doi: 10.1126/sciadv.1601693
Jarvie, S., Worthy, T. H., Saltré, F., Scofield, R. P., Seddon, P. J., and Cree, A. (2021). Using Holocene fossils to model the future: distribution of climate suitability for tuatara, the last rhynchocephalian. J. Biogeogr. 48, 1489–1502. doi: 10.1111/jbi.14092
Jensen, E. L., Mooers, A. Ø, Caccone, A., and Russello, M. A. (2016). I-HEDGE: determining the optimum complementary sets of taxa for conservation using evolutionary isolation. PeerJ 4:e2350. doi: 10.7717/peerj.2350
Jetz, W., Thomas, G. H., Joy, J. B., Redding, D. W., Hartmann, K., and Mooers, A. O. (2014). Global distribution and conservation of evolutionary distinctness in birds. Curr. Biol. 24, 919–930. doi: 10.1016/j.cub.2014.03.011
KBA Standards and Appeals Committee (2020). Guidelines for using A Global Standard for the Identification of Key Biodiversity Areas. Version 1.1. Prepared by the KBA Standards and Appeals Committee of the IUCN Species Survival Commission and IUCN World Commission on Protected Areas. Gland: IUCN.
Leao, T. C., Fonseca, C. R., Peres, C. A., and Tabarelli, M. (2014). Predicting extinction risk of Brazilian Atlantic Forest angiosperms. Conserv. Biol. 28, 1349–1359. doi: 10.1111/cobi.12286
Leonard-Pingel, J. S., Jackson, J. B. C., and O’Dea, A. (2012). Changes in bivalve functional and assemblage ecology in response to environmental change in the Caribbean Neogene. Paleobiology 38, 509–524. doi: 10.1666/10050.1
Mace, G. M., and Lande, R. (1991). Assessing extinction threats: toward a reevaluation of IUCN threatened species categories. Conserv. Biol. 5, 148–157. doi: 10.1111/j.1523-1739.1991.tb00119.x
Monsarrat, S., Novellie, P., Rushworth, I., and Kerley, G. (2019). Shifted distribution baselines: neglecting long-term biodiversity records risks overlooking potentially suitable habitat for conservation management. Philos. Trans. R. Soc. B Biol. Sci. 374:20190215. doi: 10.1098/rstb.2019.0215
Mooers, A. O., Faith, D. P., and Maddison, W. P. (2008). Converting endangered species categories to probabilities of extinction for phylogenetic conservation prioritization. PLoS One 3:e003700. doi: 10.1371/journal.pone.0003700
Mouillot, D., Graham, N. A. J., Villeger, S., Mason, N. W. H., and Bellwood, D. R. (2013). A functional approach reveals community responses to disturbances. Trends Ecol. Evol. 28, 167–177. doi: 10.1016/j.tree.2012.10.004
Pimiento, C., and Benton, M. J. (2020). The impact of the Pull of the Recent on extant elasmobranchs. Palaeontology 63, 369–374. doi: 10.1111/pala.12478
Pimiento, C., Griffin, J. N., Clements, C. F., Silvestro, D., Varela, S., Uhen, M. D., et al. (2017). The Pliocene marine megafauna extinction and its impact on functional diversity. Nat. Ecol. Evol. 1:1100. doi: 10.1038/s41559-017-0223-6
Pimiento, C., Leprieur, F., Silvestro, D., Lefcheck, J., Albouy, C., Rasher, D., et al. (2020a). Functional diversity of marine megafauna in the Anthropocene. Sci. Adv. 6:eaay7650. doi: 10.1126/sciadv.aay7650
Pimiento, C., Bacon, C. D., Silvestro, D., Hendy, A., Jaramillo, C., Zizka, A., et al. (2020b). Selective extinction against redundant species buffers functional diversity. Proc. Biol. Sci. 287:20201162. doi: 10.1098/rspb.2020.1162
Pimiento, C., and Pyenson, N. D. (2021). When sharks nearly disappeared. Science 372, 1036–1037. doi: 10.1126/science.abj2088
Purvis, A. (2008). Phylogenetic approaches to the study of extinction. Annu. Rev. Ecol. Evol. Syst. 39, 301–319. doi: 10.1146/annurev-ecolsys-063008-102010
Raja, N. B., Dimitrijević, D., Krause, M. C., and Kiessling, W. (2022). Ancient Reef Traits, a database of trait information for reef-building organisms over the Phanerozoic. Sci. Data 9, 1–10. doi: 10.31219/osf.io/sxq7m
Raup, D. M. (1972). Taxonomic Diversity during the Phanerozoic. Science 177, 1065–1071. doi: 10.1126/science.177.4054.1065
Ripple, W. J., Wolf, C., Newsome, T. M., Hoffmann, M., Wirsing, A. J., and McCauley, D. J. (2017). Extinction risk is most acute for the world’s largest and smallest vertebrates. Proc. Natl. Acad. Sci. U.S.A. 114, 10678–10683. doi: 10.1073/pnas.1702078114
Robuchon, M., Pavoine, S., Véron, S., Delli, G., Faith, D. P., Mandrici, A., et al. (2021). Revisiting species and areas of interest for conserving global mammalian phylogenetic diversity. Nat. Commun. 12, 1–11. doi: 10.1038/s41467-021-23861-y
Rodrigues, A. S., Pilgrim, J. D., Lamoreux, J. F., Hoffmann, M., and Brooks, T. M. (2006). The value of the IUCN Red List for conservation. Trends Ecol. Evol. 21, 71–76. doi: 10.1016/j.tree.2005.10.010
Rodrigues, A. S. L., Charpentier, A., Bernal-Casasola, D., Gardeisen, A., Nores, C., Pis Millán, J. A., et al. (2018). Forgotten Mediterranean calving grounds of grey and North Atlantic right whales: evidence from Roman archaeological records. Proc. R. Soc. B Biol. Sci. 285:20180961. doi: 10.1098/rspb.2018.0961
Rodrigues, A. S. L., Monsarrat, S., Charpentier, A., Brooks, T. M., Hoffmann, M., Reeves, R., et al. (2019). Unshifting the baseline: a framework for documenting historical population changes and assessing long-term anthropogenic impacts. Philos. Trans. R. Soc. B Biol. Sci. 374:20190220. doi: 10.1098/rstb.2019.0220
Roy, K., Hunt, G., and Jablonski, D. (2009). Phylogenetic conservatism of extinctions in marine bivalves. Science 325, 733–737. doi: 10.1126/science.1173073
Salas-Gismondi, R., Flynn, J. J., Baby, P., Tejada-Lara, J. V., Wesselingh, F. P., and Antoine, P.-O. (2015). A Miocene hyperdiverse crocodylian community reveals peculiar trophic dynamics in proto-Amazonian mega-wetlands. Proc. R. Soc. Lond. B Biol. Sci. 282:20142490. doi: 10.1098/rspb.2014.2490
Saldanha, A. (2020). A date with destiny: racial capitalism and the beginnings of the Anthropocene. Environ. Plann. D Soc. Space 38, 12–34. doi: 10.1177/0263775819871964
Secord, R., Bloch, J. I., Chester, S. G., Boyer, D. M., Wood, A. R., Wing, S. L., et al. (2012). Evolution of the earliest horses driven by climate change in the paleocene-eocene thermal maximum. Science 335, 959–962. doi: 10.1126/science.1213859
Sibert, E. C., and Rubin, L. D. (2021). An early Miocene extinction in pelagic sharks. Science 372, 1105–1107. doi: 10.1126/science.aaz3549
Silvestro, D., Zizka, A., Bacon, C. D., Cascales-Miñana, B., Salamin, N., and Antonelli, A. (2016). Fossil biogeography: a new model to infer dispersal, extinction and sampling from palaeontological data. Philos. Trans. R. Soc. B Biol. Sci. 371:20150225. doi: 10.1098/rstb.2015.0225
Steel, M., Mimoto, A., and Mooers, A. Ø (2007). Hedging our bets: the expected contribution of species to future phylogenetic diversity. Evol. Bioinform. 3:117693430700300024. doi: 10.1177/117693430700300024
Steffen, W., Broadgate, W., Deutsch, L., Gaffney, O., and Ludwig, C. (2015). The trajectory of the Anthropocene: the great acceleration. Anthropocene Rev. 2, 81–98. doi: 10.1177/2053019614564785
Steffen, W., Rockström, J., Richardson, K., Lenton, T. M., Folke, C., Liverman, D., et al. (2018). Trajectories of the earth system in the anthropocene. Proc. Natl. Acad. Sci. U.S.A. 115, 8252–8259. doi: 10.1073/pnas.1810141115
Stein, R. W., Mull, C. G., Kuhn, T. S., Aschliman, N. C., Davidson, L. N. K., Joy, J. B., et al. (2018). Global priorities for conserving the evolutionary history of sharks, rays and chimaeras. Nat. Ecol. Evol. 2, 288–295. doi: 10.1038/s41559-017-0448-4
Tietje, M., and Rödel, M. O. (2018). Evaluating the predicted extinction risk of living amphibian species with the fossil record. Ecol. Lett. 21, 1135–1142. doi: 10.1111/ele.13080
Tonini, J. F. R., Beard, K. H., Ferreira, R. B., Jetz, W., and Pyron, R. A. (2016). Fully-sampled phylogenies of squamates reveal evolutionary patterns in threat status. Biol. Conserv. 204, 23–31. doi: 10.1016/j.biocon.2016.03.039
Van Der Made, J., Morales, J., and Montoya, P. (2006). Late Miocene turnover in the Spanish mammal record in relation to palaeoclimate and the Messinian Salinity Crisis. Palaeogeogr. Palaeoclimatol. Palaeoecol. 238, 228–246. doi: 10.1016/j.palaeo.2006.03.030
Varela, S., Lobo, J. M., and Hortal, J. (2011). Using species distribution models in paleobiogeography: a matter of data, predictors and concepts. Palaeogeogr. Palaeoclimatol. Palaeoecol. 310, 451–463. doi: 10.1016/j.palaeo.2011.07.021
Villéger, S., Mason, N. W., and Mouillot, D. (2008). New multidimensional functional diversity indices for a multifaceted framework in functional ecology. Ecology 89, 2290–2301. doi: 10.1890/07-1206.1
Villéger, S., Novack-Gottshall, P. M., and Mouillot, D. (2011). The multidimensionality of the niche reveals functional diversity changes in benthic marine biotas across geological time. Ecol. Lett. 14, 561–568. doi: 10.1111/j.1461-0248.2011.01618.x
Volety, A. K., Savarese, M., Hoye, B., and Loh, A. N. (2009). Landscape Pattern: Present and Past Distribution of Oysters in South Florida Coastal Complex (Whitewater Bay/Oyster Bay/Shark to Robert’s Rivers). Fort Myers, FL: Florida Gulf Coast University.
Wesselingh, F. P., Hoorn, C., Kroonenberg, S. B., Antonelli, A., Lundberg, J. G., Vonhof, H. B., et al. (2010). “On the origin of Amazonian landscapes and biodiversity: a synthesis,” in Amazonia, Landscape and Species Evolution, 1st Edn, eds C. Hoorn and F. P. Wesselingh (Hoboken, NJ: Blackwell publishing), 421–431. doi: 10.1002/9781444306408.ch26
Willis, C. G., Ruhfel, B., Primack, R. B., Miller-Rushing, A. J., and Davis, C. C. (2008). Phylogenetic patterns of species loss in Thoreau’s woods are driven by climate change. Proc. Natl. Acad. Sci. U.S.A. 105, 17029–17033. doi: 10.1073/pnas.0806446105
Wright, A. M., Bapst, D. W., Barido-Sottani, J., and Warnock, R. C. (2022). Integrating fossil observations into phylogenetics using the fossilized birth–death model. Annu. Rev. Ecol. Evol. Syst. 53:12. doi: 10.1146/annurev-ecolsys-102220-030855
Keywords: biodiversity, conservation prioritisation, extinction, fossil record, palaeontology
Citation: Pimiento C and Antonelli A (2022) Integrating deep-time palaeontology in conservation prioritisation. Front. Ecol. Evol. 10:959364. doi: 10.3389/fevo.2022.959364
Received: 01 June 2022; Accepted: 21 October 2022;
Published: 24 November 2022.
Edited by:
Damien Fordham, University of Adelaide, AustraliaReviewed by:
Jamie Wood, University of Adelaide, AustraliaCopyright © 2022 Pimiento and Antonelli. This is an open-access article distributed under the terms of the Creative Commons Attribution License (CC BY). The use, distribution or reproduction in other forums is permitted, provided the original author(s) and the copyright owner(s) are credited and that the original publication in this journal is cited, in accordance with accepted academic practice. No use, distribution or reproduction is permitted which does not comply with these terms.
*Correspondence: Catalina Pimiento, Y2F0YWxpbmEucGltaWVudG9oZXJuYW5kZXpAcGltLnV6aC5jaA==