- 1Organic Isotope Geochemistry Group, Max Planck Institute for Chemistry, Mainz, Germany
- 2Emmy Noether Group for Hominin Meat Consumption, Max Planck Institute for Chemistry, Mainz, Germany
- 3Primate Models for Behavioural Evolution, Institute of Human Sciences, University of Oxford, Oxford, United Kingdom
- 4Senckenberg Biodiversity and Climate Research Centre, Frankfurt, Germany
- 5Institute of Geosciences, Johannes Gutenberg University, Mainz, Germany
- 6Interdisciplinary Center for Archaeology and Evolution of Human Behaviour, Universidade do Algarve, Faro, Portugal
- 7Evolutionary Studies Institute, University of the Witwatersrand, Johannesburg, South Africa
- 8Department of Zoology, University of Oxford, Oxford, United Kingdom
- 9Department of Brain and Cognitive Sciences, University of Rochester, Rochester, NY, United States
- 10Technological Primates Group, Max Planck Institute for Evolutionary Anthropology, Leipzig, Germany
- 11Center for the Advanced Study of Human Paleobiology, George Washington University, Washington, DC, United States
- 12Department of Chemistry, Life Sciences and Environmental Sustainability, University of Parma, Parma, Italy
- 13Smithsonian Tropical Research Institute, Balboa, Panama
- 14Graduate School of Oceanography, University of Rhode Island, Narragansett, RI, United States
- 15Centro de Investigação em Biodiversidade e Recursos Genéticos, InBIO Laboratório Associado, Universidade do Porto, Vairão, Portugal
- 16BIOPOLIS Program in Genomics, Biodiversity and Land Planning, Centro de Investigação em Biodiversidade e Recursos Genéticos, Vairão, Portugal
- 17Organisms and Environment Division, School of Biosciences, Cardiff University, Cardiff, United Kingdom
- 18GeoZentrum Nordbayern, Friedrich-Alexander-Universität Erlangen-Nürnberg, Erlangen, Germany
- 19Department of Climate Geochemistry, Max Planck Institute for Chemistry, Mainz, Germany
- 20Escuela de Antropología, Facultad de Ciencias Sociales, Pontificia Universidad Católica de Chile, Santiago, Chile
- 21Gorongosa National Park, Sofala, Mozambique
- 22Institute of Geosciences, Goethe University Frankfurt, Frankfurt, Germany
- 23Department of Geosciences, Princeton University, Princeton, NJ, United States
- 24Inorganic Gas Isotope Geochemistry Group, Max Planck Institute for Chemistry, Mainz, Germany
- 25Centre for Functional Ecology, University of Coimbra, Coimbra, Portugal
The analyses of the stable isotope ratios of carbon (δ13C), nitrogen (δ15N), and oxygen (δ18O) in animal tissues are powerful tools for reconstructing the feeding behavior of individual animals and characterizing trophic interactions in food webs. Of these biomaterials, tooth enamel is the hardest, most mineralized vertebrate tissue and therefore least likely to be affected by chemical alteration (i.e., its isotopic composition can be preserved over millions of years), making it an important and widely available archive for biologists and paleontologists. Here, we present the first combined measurements of δ13C, δ15N, and δ18O in enamel from the teeth of modern fauna (herbivores, carnivores, and omnivores) from the well-studied ecosystem of Gorongosa National Park (GNP) in central Mozambique. We use two novel methods to produce high-precision stable isotope enamel data: (i) the “oxidation-denitrification method,” which permits the measurement of mineral-bound organic nitrogen in tooth enamel (δ15Nenamel), which until now, has not been possible due to enamel’s low organic content, and (ii) the “cold trap method,” which greatly reduces the sample size required for traditional measurements of inorganic δ13Cenamel and δ18Oenamel (from ≥0.5 to ≤0.1 mg), permitting analysis of small or valuable teeth and high-resolution serial sampling of enamel. The stable isotope results for GNP fauna reveal important ecological information about the trophic level, dietary niche, and resource consumption. δ15Nenamel values clearly differentiate trophic level (i.e., carnivore δ15Nenamel values are 4.0‰ higher, on average, than herbivores), δ13Cenamel values distinguish C3 and/or C4 biomass consumption, and δ18Oenamel values reflect local meteoric water (δ18Owater) in the park. Analysis of combined carbon, nitrogen, and oxygen stable isotope data permits geochemical separation of grazers, browsers, omnivores, and carnivores according to their isotopic niche, while mixed-feeding herbivores cannot be clearly distinguished from other dietary groups. These results confirm that combined C, N, and O isotope analyses of a single aliquot of tooth enamel can be used to reconstruct diet and trophic niches. Given its resistance to chemical alteration, the analysis of these three isotopes in tooth enamel has a high potential to open new avenues of research in (paleo)ecology and paleontology.
Introduction
In modern ecosystems, stable isotope geochemistry can complement traditional ecological approaches (e.g., field observations and behavioral studies) and help researchers to better understand the dietary niche and habitat use of animals in the wild. The stable carbon (δ13C), nitrogen (δ15N), and oxygen (δ18O) isotope compositions of body tissues can provide information about an individual’s metabolism and feeding behavior, trophic interactions, and even record aspects of the (paleo)environment such as aridity, seasonality, and vegetation composition (e.g., DeNiro and Epstein, 1981; Ambrose, 1986; Cerling et al., 1997; Bocherens and Drucker, 2003; Kingston and Harrison, 2007; Segalen et al., 2007; Bocherens, 2009; Lüdecke et al., 2016, 2018).
In modern fauna, isotopic measurements are routinely conducted on a variety of biological materials such as collagen (from bone or dentin), soft tissues (e.g., muscle), and body fluids (e.g., blood and urea). Paleontologists have long sought a reliably preserved tissue (i.e., without diagenetic alteration) in which to measure isotope ratios of all three elements—carbon, nitrogen, and oxygen—in deep time contexts. Tooth enamel, as the densest and most mineralized vertebrate tissue, has great potential in this respect (Leichliter et al., 2022). Hydroxyapatite content is about 95% wt. in mature enamel (Sakae et al., 1997; Passey and Cerling, 2002; Lacruz et al., 2017; Gil-Bona and Bidlack, 2020), which makes it more resistant to diagenetic alteration during fossilization than more porous and poorly mineralized tissues such as bone or dentin (mineralization ca. 70% wt.; Goldberg et al., 2011).
As such, the inorganic mineral phase of tooth enamel has long been the focus of carbon (δ13Cenamel) and oxygen (δ18Oenamel) stable isotope analyses for the reconstruction of the diet of extinct and extant species (Ambrose and Norr, 1993).
Typically, 500–1,000 μg of tooth enamel, which contains less than 5% structural carbonate, is needed for precise δ13Cenamel and δ18Oenamel analysis with traditional continuous-flow isotope ratio mass spectrometry. The “cold trap method” presented in this study permits high-precision δ13Cenamel and δ18Oenamel analysis of as little as 50 μg tooth enamel. The method employs a cryofocusing step during which the sample gas is collected in a liquid N2 trap (Vonhof et al., 2020a,b). This results in >80% reduction in the sample size typically required for conventional analyses (refer to e.g., Merceron et al., 2021; Jaouen et al., 2022).
Recently, Leichliter et al. (2021) showed that δ15Nenamel records the nitrogen isotopic composition of an animal’s diet under controlled conditions in a feeding experiment with rodents. The significant advantage of measuring δ15N in tooth enamel (δ15Nenamel) instead of (bone/dentin) collagen is that enamel is more resistant to digenesis (Lee-Thorp and Van der Merwe, 1987; Wang and Cerling, 1994; Koch et al., 1997; Koch, 2007). The dense mineralization of enamel thus has a high potential to protect inorganic components from isotopic alteration during fossilization (Leichliter et al., 2022). In fact, the tooth enamel biomineral matrix appears to act as a closed system during oxidative attack, dissolution, and thermal alteration, leaving the δ15Nenamel value of a fossil unchanged (Martinez-Garcia et al., 2022), demonstrating the potential utility of δ15Nenamel as a new trophic proxy in paleoecological studies. However, high-precision analysis of δ15Nenamel in tooth enamel-bound nitrogen has been hampered by its low nitrogen content (about 0.5–2.5 g N/100 g in mature mammalian enamel; Teruel et al., 2015). To fill this gap in our isotopic toolbox, we recently developed a method to determine the nitrogen isotopic composition of the organic matter preserved in tooth enamel (Leichliter et al., 2021). This method, adapted from studies of marine microfossils (i.e., diatoms and foraminifera; Sigman et al., 2001; Robinson et al., 2004; Ren et al., 2009) and macrofossils (Wang et al., 2014, 2015, 2017; Lueders-Dumont et al., 2018; Kast et al., 2022), involves the oxidation of nitrogen in enamel-bound organic matter to nitrate, followed by bacterial conversion of nitrate to N2O. This “oxidation-denitrification method” requires 5 nmol of N (i.e., 5 mg of enamel; Leichliter et al., 2021), which reflects ca. 1% of the material needed for conventional combustion measurements and ca. half the material used for nano-elemental analyzer measurements (e.g., Polissar et al., 2009; Fulton et al., 2018). Importantly, the “oxidation-denitrification method” drastically improves analytical precision from ∼1.0‰ 1σ standard deviation for nano-EA measurements at 8 nmol of N (Fulton et al., 2018) to <0.2‰ at 5 nmol of N. This precision is more than sufficient to resolve the 3–5‰ trophic level enrichment in δ15N observed in large-scale ecological studies (Schoeninger and DeNiro, 1984; Bocherens and Drucker, 2003; Caut et al., 2009).
Here, we present the first combined δ13Cenamel, δ15Nenamel, and δ18Oenamel isotope data measured in the same aliquot of tooth enamel. We analyzed the tooth enamel of modern mammalian fauna (17 taxa; bovids, equids, suids, elephants, hippos, primates, felids) and one reptile (crocodiles) from Gorongosa National Park (GNP), a well-studied ecosystem in central Mozambique (refer to e.g., Wilson, 2014; Correia et al., 2017; Atkins et al., 2019; Martinez et al., 2019; Pansu et al., 2019; Stalmans et al., 2019; Bobe et al., 2020; Guyton et al., 2020). In addition, we analyzed δ18Owater of (permanent and ephemeral) lakes, ponds, streams, floodplains, rain-, and groundwater to evaluate isotope patterns of available drinking water, which are the main determinants of δ18Oenamel in large mammals (Kohn and Cerling, 2002). With this dataset, we test two novel methods, as well as gain new insight into wild animal foraging behavior and the food web dynamics of GNP.
Tooth enamel as dietary proxy material
Dental material chronologically records the diet of an individual during a distinct time period in their life. During enamel maturation, the organic matrix is removed and replaced with inorganic minerals over a period of weeks, months, or years, depending on taxon, rate of wear, and tooth size (Ungar, 2010). Once mature enamel has fully mineralized, it has no regenerative capacity, and thus preserves an animal’s isotopic composition during the time of tooth formation. This differentiates enamel from other tissues (e.g., soft tissues, but also bone or dentin) that undergo continuous remodeling (e.g., Balasse et al., 1999; Kohn and Cerling, 2002; Passey and Cerling, 2002; Zazzo et al., 2005; Abou Neel et al., 2016; Yang et al., 2020). Tooth enamel is, therefore, one of the only archives in the vertebrate body that records dietary information from early life stages (i.e., infant to young-adult) and that is also preserved in the fossil record. To reconstruct the adult diet and avoid the isotopic effect of breast milk consumption (Fuller et al., 2006; Tsutaya and Yoneda, 2015; Dailey-Chwalibóg et al., 2020; Chinique de Armas et al., 2022), we targeted the latest-forming permanent tooth (i.e., usually M3) in each specimen (for details refer to Supplementary Data Sheet 1 and Supplementary Table 1).
For this study, we sampled bulk enamel, meaning that the resulting isotope data are time-averaged and record several months or even years depending on tooth mineralization rate (which can vary between taxa), as well as sampling strategy.
Isotope ratios for tooth enamel are reported using per mil (‰) notation relative to VPDB (Vienna Pee Dee Belemnite) for carbon, AIR for nitrogen, or VSMOW (Vienna Standard Mean Ocean Water) for oxygen, where aX is the heavier and bX is the lighter isotope (13C/12C, 15N/14N, or 18O/16O) for δ13Cenamel, δ15Nenamel, δ18Oenamel, and δ18Owater, respectively:
δ18Oenamel values were converted from VPDB into VSMOW after Coplen (1988).
Nitrogen isotopes in tooth enamel
All living organisms require nitrogen as a major nutrient, which animals acquire from the food they consume (Ambrose and Norr, 1993). Due to isotopic fractionation during metabolism and subsequent excretion of waste, a consumer’s 15N/14N ratio is elevated compared to their diet. As a result, animals typically have δ15N tissue values that are ca. 3–5‰ higher than the foods they consume (Figure 1; Schoeninger and DeNiro, 1984; Bocherens and Drucker, 2003; Fox-Dobbs et al., 2007; Krajcarz et al., 2018; Leichliter et al., 2021).
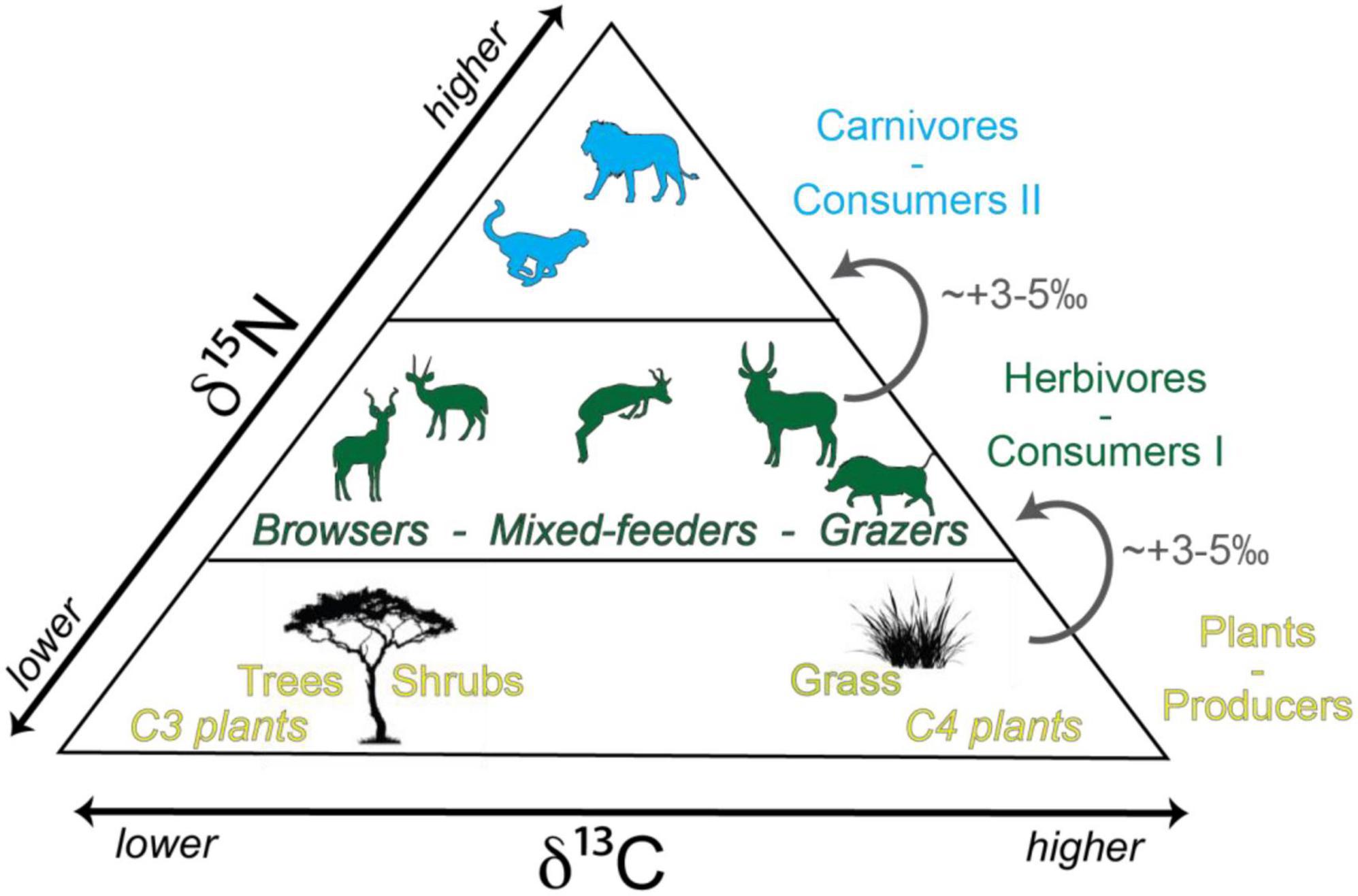
Figure 1. Simplified summary of variations in δ13C and δ15N values in an African terrestrial food web. Modified after Bocherens (2009).
The living part of any terrestrial food web starts with plants (which in turn use energy from the sun). Most plants obtain nitrogen from the soil, and soil δ15N systematically decreases with increasing mean annual precipitation and decreasing mean annual temperature (Evans, 2001; Robinson, 2001; Amundson et al., 2003). Soil δ15N has an effect on the δ15N values of plants (Codron et al., 2005) which in turn determines the δ15N tissue values of primary and secondary consumers. Thus, climate influences the nitrogen isotope composition of animals living in a given ecosystem, which can vary across space and time (Ambrose, 1986, 1991; Ambrose and DeNiro, 1986). To avoid the confounding effects of regional baseline variability in nitrogen, we focus on the vertebrate community living in GNP. Our aim is to characterize the δ15Nenamel values of herbivores (i.e., grazers, mixed-feeders, and browsers), omnivores, and carnivores living within this single and well-constrained ecosystem (Figure 2).
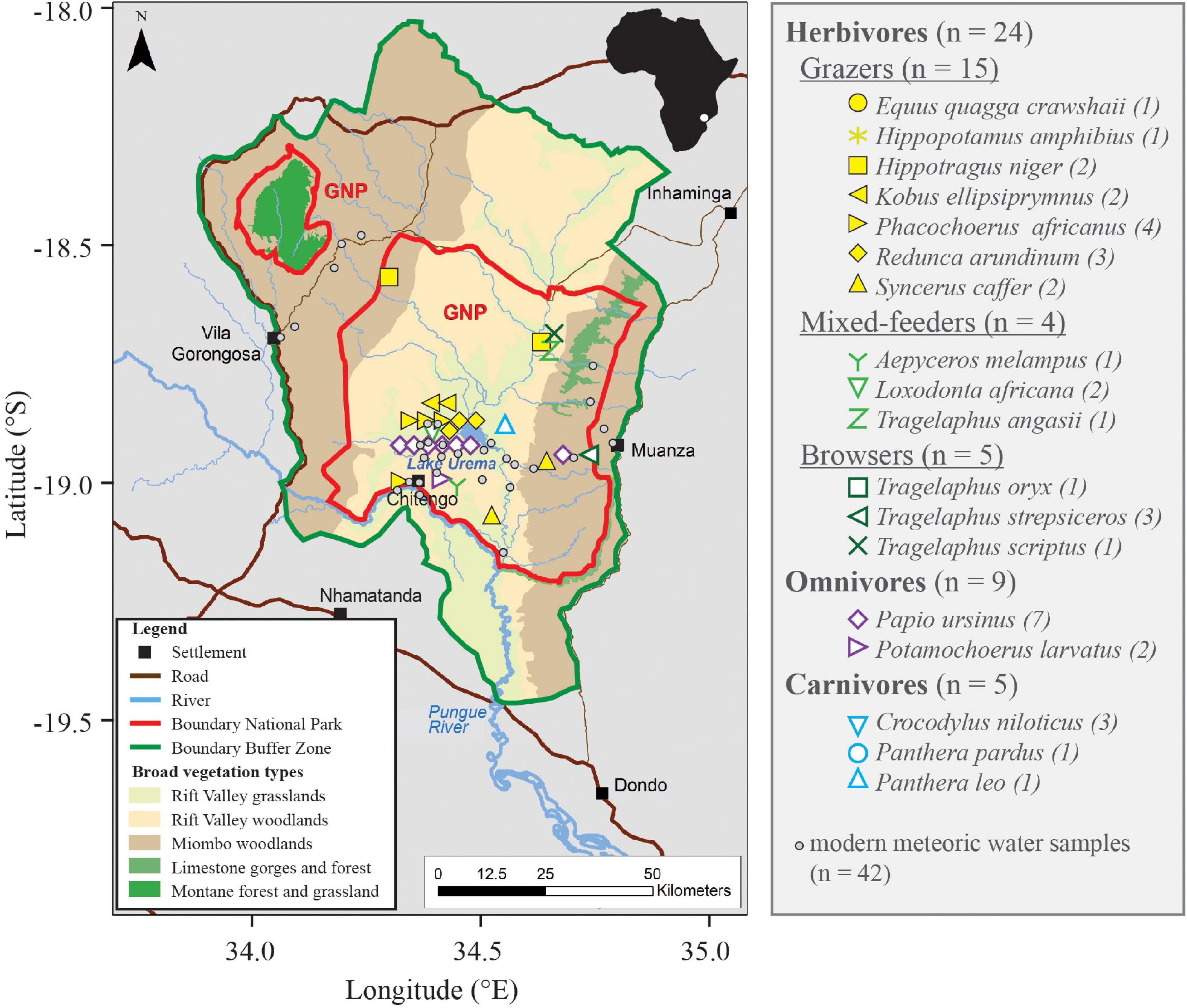
Figure 2. Map of Gorongosa National Park (red outline) and the Buffer Zone (green outline) indicating main vegetation types (modified after Stalmans and Beilfuss, 2008) with faunal and meteoric water collection sites. GPS coordinates for fauna specimens and water samples can be found in Supplementary Tables 1, 2. Note that the exact location of nine faunal specimens is not known, because they were collected before the start of the PPPG, but all specimens are from inside the park or Buffer Zone. A black line drawing of Africa shows the position of GNP (white circle).
In addition to climate and habitat-driven baseline variation, digestive physiology and water dependence have also been proposed to affect the δ15N of animals’ tissues (Sealy et al., 1987; Ambrose, 1991; Hartman, 2010). For instance, studies suggest that the δ15N values of ruminant herbivores differ from non-ruminants as the result of the incorporation of 15N-enriched microbes community in their hindgut (Steinhour et al., 1982; Sutoh et al., 1987). Additionally, herbivore species with physiological adaptations for water conservation, such as the excretion of concentrated urine, are proposed to have higher δ15N values than water-dependent animals (Ambrose, 1991 and references therein). However, the effects of digestive physiology and water dependence are still poorly understood and not well-tested (Ambrose, 1991; Cantalapiedra-Hijar et al., 2015). In this study, herbivorous ruminants and non-ruminants, as well as obligate drinkers and non-obligate drinkers were analyzed to evaluate these hypotheses.
Carbon isotopes in tooth enamel
In contrast to δ15N, δ13C of animal tissues increases only slightly with each step in the food chain (ca. 1‰ per trophic level; DeNiro and Epstein, 1981; Schoeniger and DeNiro, 1984; Bocherens and Drucker, 2003; O’Connell et al., 2012). In Africa, this signal is usually overprinted by the larger δ13C differences between plants using different photosynthetic pathways; therefore, δ13Cenamel cannot be reliably used for trophic-level reconstructions. However, δ13Cenamel is a robust and well-established tool for reconstructing the plant-based diet of an animal (Figure 1; e.g., Cerling et al., 2015).
Dicots (trees, bushes, and herbs) use the C3 photosynthetic pathway, whereas most tropical grasses and sedges use the C4 photosynthetic pathway (e.g., Pearcy and Ehleringer, 1984). C4 photosynthesis is advantageous in warm and seasonally dry, open environments with high light intensity, whereas the C3 pathway is typically prevalent under low water stress and high-pCO2 conditions (Kohn, 2010). Another pathway (Crassulacean Acid Metabolism; Wolf, 1960; Lüttge, 2004) is used by very arid-adapted plants like succulents, but these are rare at GNP and do not contribute significantly to the diet of the studied animals.
As a result of differential discrimination against 13CO2 during photosynthesis, C3 and C4 types can be distinguished based on their δ13C values (Figure 1). The δ13C values of African C4 plants range from –19‰ to –9‰, while those of C3 plants lie between –29‰ and –25‰, resulting in bimodal and non-overlapping δ13C values (Smith and Epstein, 1971; Pearcy and Ehleringer, 1984; Cerling et al., 2003; Kohn, 2010). Plant δ13C values are reflected in the tissues of the animals that consume them, such that C4 grazing (>70% C4 grass consumption), mixed-feeding (>30% C4 grass and >30% C3 browse), and browsing (>70% C3 browse) taxa can be differentiated (Cerling et al., 2003). Isotopic fractionation from diet to tooth takes place during enamel biomineralization. Large herbivore δ13Cenamel values are enriched by ∼14.5 to 12.0 ± 1.0‰ compared to the plants that they consume, depending on their digestive strategies (Cerling and Harris, 1999; Tejada-Lara et al., 2018, Cerling et al., 2021). Generally, browsers have δ13Cenamel values lower than –8‰, grazers have values above –2‰, and values in between are typical for mixed-feeders (Cerling and Harris, 1999; Uno et al., 2018). Carnivore δ13Cenamel values are determined by the isotopic composition of their prey, with negligible isotopic fractionation (Bocherens and Drucker, 2003).
Stable oxygen isotopes in tooth enamel and drinking water
The oxygen isotope composition of tooth enamel can be measured in either structural carbonate (CO3) or phosphate (PO4). Here, we report measurements on structural carbonate. For mammals, the δ18O values of both components can be converted with the equation δ18OPO4 ≈ 0.98 * δ18OCO3 – 8.5 after Iacumin et al. (1996). While reptiles may have a slightly different PO4 to CO3 relationship than mammals due to physiological effects (Stanton and Carlson, 2004), this has not been adequately studied to establish a separate equation for this group.
The oxygen isotope composition of tooth enamel is directly linked to the δ18O values of body water which itself is a complex function of (micro)habitat, climate, diet, drinking behavior, and physiology (e.g., Bryant and Froelich, 1995; Kohn, 1996; Pederzani and Britton, 2019). The body’s main oxygen sources are drinking water, food, and atmospheric O2.
In sub-Saharan Africa, meteoric water (i.e., available drinking water) is often strongly influenced by the composition of the source water and evaporation processes (for details regarding GNP meteoric water, refer to the following section and Steinbruch, 2010; Steinbruch and Weise, 2014). To interpret variability in δ18Oenamel in the GNP fauna, we measured δ18O of potential drinking water (i.e., permanent and ephemeral lakes and streams, rain, and groundwater, in the park and surrounding areas).
In warm-blooded mammals with an internally regulated body temperature (ca. 37°C for most mammals larger than 1 kg), biogenic hydroxyapatite is precipitated at a constant temperature, and thus the oxygen isotopic signature of ingested food and water is recorded without temperature-dependent fractionation. For large herbivores, digested water is assumed to be equivalent to surficial water (Bryant and Froelich, 1995). There is an offset between δ18O of body water and the δ18O of the bioapatite (Bryant et al., 1996; Iacumin et al., 1996) according to the generalized equation for mammalian taxa of δ18Owater ≈ (δ18Oenamel(PO4) –23)/0.9 (Kohn and Cerling, 2002); taxon-specific variations (refer to e.g., Ayliffe et al., 1992), relative humidity, and water temperature can, however, change this correlation (Kohn, 1996). The oxygen isotope compositions of Crocodylus correlate with those of ambient water according to the equation δ18Oenamel(PO4) ≈ (δ18Owater + 19.13)/0.82 (Amiot et al., 2007).
In additional consideration, African mammals can be divided into obligate and non-obligate drinkers. δ18Oenamel of large-bodied (>100 kg), obligate drinkers have been shown to primarily reflect the δ18Owater values of consumed water, and hence their δ18Oenamel is closely related to the isotopic composition of their ambient environment (Bryant and Froelich, 1995; Kohn, 1996; Hoppe, 2006). However, non-obligate drinking animals, such as drought-adapted herbivores, obtain large proportions (or even all) of their water from the plant foods they consume (e.g., Nicholson, 1985). This behavior can lead to an increase in δ18Oenamel values because leaf water is sensitive to evaporation, resulting in high δ18O values in this part of the plant (Levin et al., 2006).
Additionally, plant δ18O composition can vary as the result of differences in feeding behavior (e.g., δ18Oenamel values typically decrease with increasing fraction of C3 diet, because C3 plants have lower δ18O values than coexisting C4 plants) (Bocherens et al., 1996).
At GNP, both obligate drinkers, which mostly include grazers and omnivores (e.g., hippos, equids, elephants, primates, suids), as well as non-obligate drinkers, mainly browsers and mixed-feeders (e.g., eland, bushbuck, kudu, nyala, and sable, refer to Cain et al., 2012), were sampled (refer to Table 1).
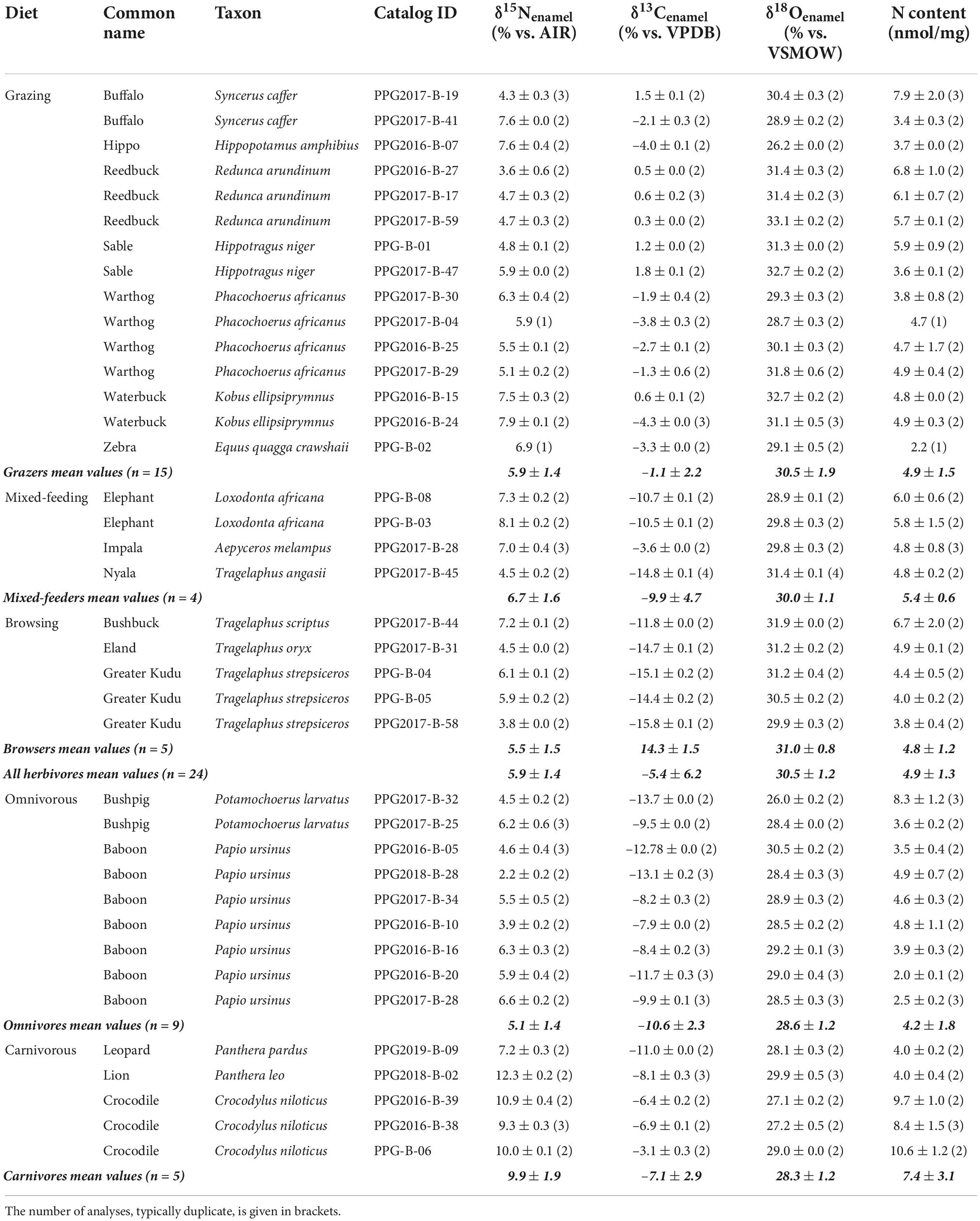
Table 1. List of all 38 analyzed specimens including diet, common and Latin name, catalog ID, and stable nitrogen, carbon, and oxygen values in % and nitrogen content. Mean values for each dietary group are shown in bold.
Materials and methods
Gorongosa National Park
Gorongosa National Park is located in the Sofala Province in central Mozambique (Figure 2) in the Urema Rift, the southernmost part of the East African Rift System. The unfenced park encompasses a 3,688 km2 mosaic of diverse habitats and is surrounded by an inhabited “Buffer Zone” consisting mostly of agricultural lands (Figure 2). The valley floor is ca. 40 km wide and flanked to the east and west by hilly terrain rising to 400 m (Stalmans and Beilfuss, 2008; Stalmans et al., 2019). The region’s climate is influenced by the migration of the Intertropical Convergence Zone and is dominated by hot wet summers and cooler dry winters with a mean annual rainfall of 700–900 mm. Over 80% of the annual rainfall occurs between November and March and is derived from the Indian Ocean, although some rainfall can also occur in the dry months as a result of the inflow of sub-polar mist cold air and continental compression (Steinbruch and Weise, 2014; Stalmans et al., 2019; Ma et al., 2021).
A central feature of the park is Lake Urema, which is on average only 2 m deep (Böhme, 2005) and is drained through a floodplain and the Urema River into the Pungwe River (Figure 2). The lake is fed by several ephemeral rivers that flow only in the wet season. In the dry season, the lake water infiltrates aquifer systems at the transition of the escarpments of the Urema Graben (Steinbruch, 2010; Arvidsson et al., 2011). The Lake Urema floodplain extends for >300 km2 and floods annually (Steinbruch and Merkel, 2008), becoming uninhabitable for most terrestrial animals during the wet season. Stalmans and Beilfuss (2008) identified and mapped five major habitat types within GNP (Figure 2). From west to east, these are (i) Midlands miombo woodland (Brachystegia and Julbernardia sp.) on the western rim of the Rift Valley (331 km2); (ii) Alluvial Fan Acacia, Combretum, and palm savannas (1,265 km2); (iii) floodplain grasslands (759 km2) around Lake Urema; (iv) Colluvial Fan savannas (326 km2) within the Rift Valley; and (v) Cheringoma Plateau miombo woodlands and forested limestone gorges on the eastern rim of the Rift Valley (938 km2).
These landscapes have an overall proportional tree cover of 0.39 (Daskin et al., 2016) and support a large diversity of herbivores, including grazers, browsers, and mixed-feeders (Tinley, 1977; Stalmans and Beilfuss, 2008; Daskin et al., 2016; Stalmans et al., 2019; Gaynor et al., 2021). However, while Gorongosa was once renowned for its large mammal population (Tinley, 1977), the ecosystem experienced severe perturbation during 15 years of civil war (1977–1992), from which it is still recovering today. Most apex predators were extirpated from the park during this time, including leopards, African wild dogs, and spotted hyenas. Of these taxa, leopards have recently re-colonized the Buffer Zone by migrating from surrounding areas, and five additional adult leopards as well as two founding packs of wild dogs have been re-introduced from different regions in South Africa (Bouley et al., 2021). Lions and crocodiles are the only apex predators that persisted throughout the periods of war and recovery; however, the lions’ abundance was greatly reduced (Pringle, 2017; Bouley et al., 2018). Herbivore populations were similarly decimated. Before the war, elephants, hippos, buffalo, zebra, and wildebeest dominated the herbivore fauna but today are outnumbered by waterbuck and other small to mid-size antelopes (Stalmans et al., 2019).
The Gorongosa Restoration Project, established in 2006, is focused on long-term biodiversity conservation, sustainable development of the neighboring Buffer Zone, training farmers in new practices to improve crop yields, and improving the health and education of communities in the greater Gorongosa region. Due to these efforts, the GNP ecosystem is slowly recovering and offers a unique setting in which to study the adaptation of mammals to dynamic and complex environments. Today, numerous projects are underway in GNP. These studies use a combination of methods including motion-triggered cameras (Gaynor et al., 2018, 2021; Easter et al., 2019), biologgers (often including Global Positioning System units, triaxial accelerometers, and sometimes video recorders; e.g., Branco et al., 2019a,b; Becker et al., 2021; Bouley et al., 2021), aerial counts (Cumming et al., 1994; Dutton and Carvalho, 2002; Dunham, 2004; Stalmans, 2012; Stalmans et al., 2019), animal follows (Hammond et al., 2022), molecular studies (Martinez et al., 2019; Santander et al., 2022), and other field observation tools (e.g., Muschinski et al., 2019) to gain insights in the park’s ecology. The δ13Cenamel, δ15Nenamel, and δ18Oenamel datasets of Gorongosa’s fauna presented here—the first stable isotopes result for any terrestrial fauna in Mozambique—will help us to better understand dietary patterns of the large-bodied animals which roam GNP today.
Material and sampling protocol
Tooth enamel
Since 2016, remains of modern fauna have been collected from different regions of the park and the surrounding Buffer Zone (Figure 2 and Supplementary Table 1) by members of the Paleo-Primate Project Gorongosa (PPPG). We selected as many different vertebrate taxa as possible and analyzed the tooth enamel of 38 adult individuals (17 mammalian taxa and one reptile; refer to Table 1) for organic δ15Nenamel as well as inorganic δ13Cenamel and δ18Oenamel. These include 15 grazers, four mixed-feeders, five browsers, nine omnivores, and five carnivores. The bone weathering stage was 0–1, suggesting that all animals died within the last few years prior to field collection (after Behrensmeyer, 1978).
During sampling, the topmost ca. 0.2 mm of enamel was discarded to avoid contamination by any adherent sediment. Tooth enamel powder was sampled using a Dremel handheld drill with a diamond ball head drill tip (0.9 mm diameter) at low to medium speed (1,000–2,000 RMP). For herbivore specimens, which generally have thick enamel, the sampling depth was 0.5–1 mm. For carnivores and some omnivores which have only a thin layer of enamel, great care was taken to avoid the underlying dentin, and sampling was conducted to a shallower depth, over a larger area of the tooth. Bulk enamel samples were usually taken longitudinally, between the cusp and the cervix, integrating at least 3 months of the dietary signal. We collected 10–50 mg of enamel for carbon, nitrogen, and oxygen isotope analyses, which were measured in duplicate or triplicate whenever possible.
Meteoric water
Water samples from the lake (n = 15), river (n = 18), rain (n = 4), and groundwater (n = 5) sources were collected from GNP and the surrounding Buffer Zone between 2016 and 2019 (Figure 2 and Supplementary Table 2) in both dry seasons (May to October; n = 32; mostly permanent lakes and rivers, groundwater) and wet season (November to April; n = 10; rainwater, ephemeral and permanent lakes/water holes, floodplain). These water sources represent potential drinking water for the Gorongosa fauna.
At each sampling site, 30 ml of unfiltered water was collected with as little air volume as possible in high-density polyethylene bottles. Rainwater was captured directly from the runoff of an aluminum roof at Camp Chitengo (Figure 2). Groundwater was sampled from water pumps which were run for at least 3 min prior to sampling. Samples were stored at room temperature and in the dark until returned to the laboratory for refrigeration and subsequent analysis.
Analyses
Nitrogen isotope analysis of tooth enamel using a novel “oxidation-denitrification method”
We use the “oxidation-denitrification method” to measure the δ15Nenamel of mineral-bound nitrogen in tooth enamel at the Max Planck Institute for Chemistry (MPIC). The “oxidation-denitrification method” was first used for marine-dissolved organic nitrogen (Knapp et al., 2005) and marine microfossil-bound nitrogen (Robinson et al., 2004; Ren et al., 2009). The protocol used here for enamel-bound nitrogen follows Leichliter et al. (2021). It includes reductive-oxidative cleaning of enamel powder followed by oxidation of enamel-bound organic matter to nitrate using a basic solution of potassium peroxydisulfate in a specially designed clean room. Nitrate is subsequently converted to N2O using the bacteria Pseudomonas chlororaphis, grown, cultured, and harvested at the MPIC following the methods outlined by Sigman et al. (2001) and Weigand et al. (2016). The isotopic composition of the N2O is extracted, purified, and analyzed by an automated purge-trap, gas chromatography-isotope ratio mass spectrometry, in this case by a custom-built system online to a Thermo Scientific MAT253-Plus isotope ratio mass spectrometer (Weigand et al., 2016). Coupled with the denitrification step for N2O production, this system results in high-precision measurements (1σ < 0.1‰) of nitrogen isotopes of nitrate down to 5 nmol N (Weigand et al., 2016).
We cleaned and measured each sample (5–7 mg of enamel powder) in duplicate or triplicate (exceptions are PPG-B-02 and PPG2017-B-04 due to limited sample amounts) in different batches, resulting in 78 individual measurements in five different batches.
Individual nitrate isotopic analyses are referenced to injections of N2O and standardized using international nitrate reference materials IAEA-NO3 and USGS34. Additionally, sample data were corrected for the contribution of the blank using the nitrogen content and δ15N values of oxidation blanks after Leichliter et al. (2021). Blank N content was between 0.3 and 0.4 nmol/ml, resulting in an average blank contribution of 3% or less. Inter-batch precision (±1σ) in δ15N for international standards is <0.2‰ for USGS65 (glycine; n = 7); <0.3‰ for USGS 40 (L-glutamic acid; n = 16); and <0.4‰ for USGS41 (L-glutamic acid; n = 11; Supplementary Table 3). For in-house standards (refer to Leichliter et al., 2021), this precision is <0.4‰ for coral standard PO-1 (Porites sp.; n = 18) and LO-1 (Lophelia pertusa; n = 17) and <0.5‰ for tooth enamel standards AG-Lox (modern Loxodonta africana; n = 18) and Noto-1 (Late-Pleistocene Notochoerus scotti; n = 17), across all analytical batches (Supplementary Table 4).
Stable carbon and oxygen isotope analyses of tooth enamel
High-precision stable carbon and oxygen isotope analysis of small sample amounts (∼50–100 μg of untreated enamel) was performed using the “cold trap method” (Vonhof et al., 2020a) at the laboratories of the Climate Geochemistry Department at MPIC in Mainz, Germany. We used a Thermo Delta-V mass spectrometer in continuous flow configuration, directly interfaced with a GasBench II gas preparation unit with an integrated pneumatically operated cold trap system. In automated mode, digestion of enamel occurs in 12 ml He-flushed exetainer vials with >99% H3PO4 at 70°C for 90 min. Then, the CO2 sample is carried with ultrapure He to the cold trap where it is cryogenically focused for 6–7 min by cooling the trap with liquid N2. After lifting the trap out of the liquid N2, the carrier gas with the sample CO2 passes through a standard Poraplot-Q Gas Chromatography (GC) column where the entire CO2 sample is delivered to the mass spectrometer for carbon and oxygen isotope analyses in a single peak, preceded by five reference gas peaks. Calculation of isotope values follows Vonhof et al., 2020a,b with the international standards IAEA-603, NBS18, and/or NBS120c in addition to two internal house standards: a carbonate standard (VICS) and tooth enamel standard AG-Lox, the latter was also used as a standard for δ15Nenamel for reference (Supplementary Table 5). Overall analytical uncertainties are better than 0.09‰ for δ13Cenamel and 0.14‰ for δ18Oenamel (1σ standard deviation of AG-Lox within batches). Carbonate contents were derived from standard vs. sample total peak area ratios (7.5% structural carbonate content for AG-Lox; after Vonhof et al., 2020b).
Oxygen isotope analyses of meteoric waters
Oxygen isotope ratios were measured on 1 ml aliquots using an LGR 24d liquid water isotope analyzer at the Goethe University-Senckenberg BiK-F Joint Stable Isotope Facility, Frankfurt, Germany (Schemmel et al., 2013). The δ18Owater values are calibrated and reported against VSMOW, with an analytical precision of <0.2‰ (2σ).
Statistical analyses
Statistical analyses of elemental content and univariate isotope values were performed using Paleontological Statistics (PAST4) version 4 (Hammer et al., 2001). Statistical significance between isotopic groups was determined using one-way ANOVA with a Tukey–Kramer HSD post hoc test if not stated otherwise with a level of significance of p = 0.050. Pearson correlation coefficient (r) is given for correlations.
Statistical analyses of multivariate isotope comparisons were performed in R (version 4.2.0; R Core Team, 2022). Isotopic niches were analyzed using the Stable Isotopes Bayesian Ellipses (SIBER) package (version 2.1.6; Jackson et al., 2011) and the Turner et al. (2010) statistical code. Data normality was first checked using the Shapiro–Wilk test for both individual groups by element using the nor.test function from the onewaytests package (Dag et al., 2018), and with a multivariate Shapiro–Wilk test for the entire dataset using the mshapiro_test function from the rstatix package (Kassambra, 2021). Data normality was assumed if p > 0.050 for all tests. The isotopic niches of the four different a priori dietary groups with n ≥ 5 were determined by fitting their distribution of δ13Cenamel, δ15Nenamel, and δ18Oenamel isotope values with estimated standard ellipse areas corrected for sample size (SEAC; Jackson et al., 2011). The SEAC contains 40% of the variation of a group and was chosen over SEA as it limits calculation biases due to small and unbalanced sample sizes and is appropriate when the analyzed groups contain fewer than 30 individuals (Syväranta et al., 2013; Pinzone et al., 2019). However, the mixed feeding group was not included in the multivariate analyses because of its sample size (n = 4) which does not meet the minimum sampling requirements for SEAC as recommended by Jackson et al. (2011). Its mean value, standard deviation, and convex hull were still calculated and plotted for visual comparisons.
Geometric overlap between ellipses was calculated and compared between analyzed groups, both in total overlap (in ‰2) and in proportional overlap (Jackson et al., 2011; refer to Supplementary Tables 6–8). Niche standard ellipse areas for each group were further explored using Bayesian modeling (SEAB) and 50, 75, and 95% credible intervals were calculated and compared across groups. Finally, to determine whether the location of each group’s niche differed in isotopic space, the Euclidean distance between the centroids of each group was calculated and compared in pairs. A residual permutation procedure and Hotelling T2 test were used to evaluate significance, with p < 0.050 indicating that the two compared niches occupy significantly different areas in isotopic space (Turner et al., 2010).
Results
We report δ15Nenamel, δ13Cenamel, δ18Oenamel, and N content data from 35 mammals and three reptiles sampled in GNP, and the results are given in Table 1. For calculated δ18Odrinking–water, carbonate contents, tooth position, sex, GPS coordinates, and collection date and locality (habitat), refer to Supplementary Table 1. Additionally, we report δ18Owater data from 42 meteoric water samples, results with water type (rain, river, lake, and groundwater), GPS coordinates, elevation, and collection date are given in Supplementary Table 2.
Nitrogen isotope values
δ15Nenamel values of all 38 specimens range from 2.2 to 12.3‰, with mean δ15Nenamel values of 5.9 ± 1.4‰ (n = 24) for herbivores, 5.1 ± 1.4‰ (n = 9) for omnivores, and 9.9 ± 1.9‰ (n = 5) for carnivores (Figure 3A). Carnivore nitrogen isotope ratios differ significantly from herbivores and omnivores (p < 0.001), while the δ15Nenamel values of omnivores (bushpigs and baboons) do not differ significantly from herbivores (p = 0.324). Grazers (6.9 ± 1.4‰, n = 15), mixed-feeders (6.7 ± 1.6‰, n = 4), and browsers (5.5 ± 1.3‰, n = 5) show no significant difference in their δ15Nenamel values (p = 0.428). Herbivore taxa do not display significantly different δ15Nenamel values when grouped according to digestive physiology (p = 0.104) or water dependency (p = 0.128; Figure 4A).
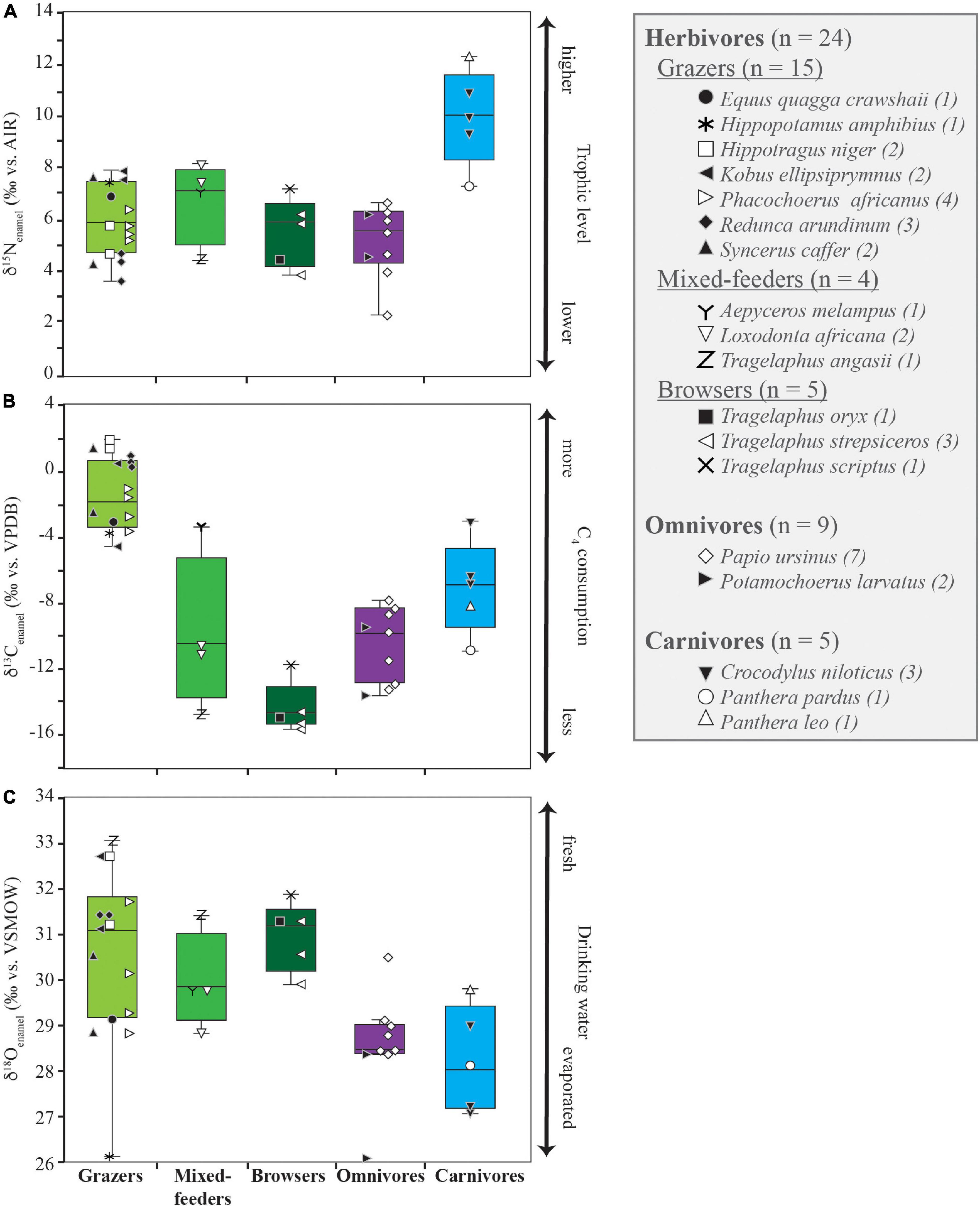
Figure 3. δ15Nenamel (A), δ13Cenamel (B), and δ18Oenamel (C) values for Gorongosa fauna by diet. Boxplots show the interquartile range (note outliers in omnivore δ18Oenamel dataset), with the median indicated by the solid line. Symbols correspond to individual specimens.
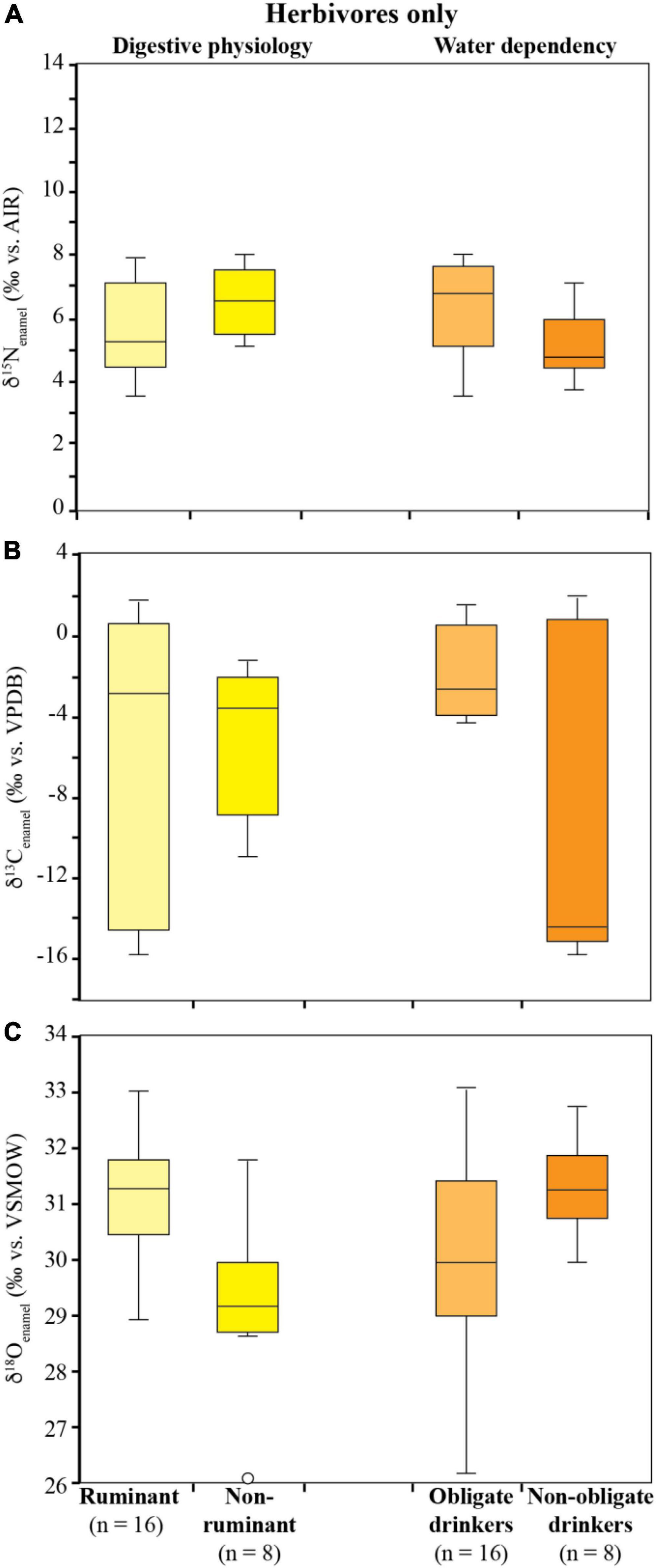
Figure 4. δ15Nenamel (A), δ13Cenamel (B), and δ18Oenamel (C) values for Gorongosa herbivores grouped by digestive physiology and water dependency. Boxplots show the interquartile range, with the median indicated by solid lines and outliers by open circles. Water dependency after Hamilton (1986), Bothma (2005), Wilson and Mittermeier (2009), and Hempson et al. (2015); see Supplementary Table 1.
Nitrogen content in tooth enamel
The nitrogen content of clean tooth enamel ranges from 2.0 to 10.6 nmol/mg with an average of 5.1 ± 1.9 nmol/mg (Figure 5 and Table 1). No significant correlation is observed between δ15Nenamel and nitrogen content (r = 0.285; p = 0.083; Figure 5A). Carnivores have slightly higher N contents compared to the other dietary groups (p = 0.008). However, this difference is entirely driven by the high nitrogen content of crocodile enamel, and crocodiles were the only reptiles sampled (Figure 5B). No other differences in the N content of tooth enamel were observed between dietary groups.
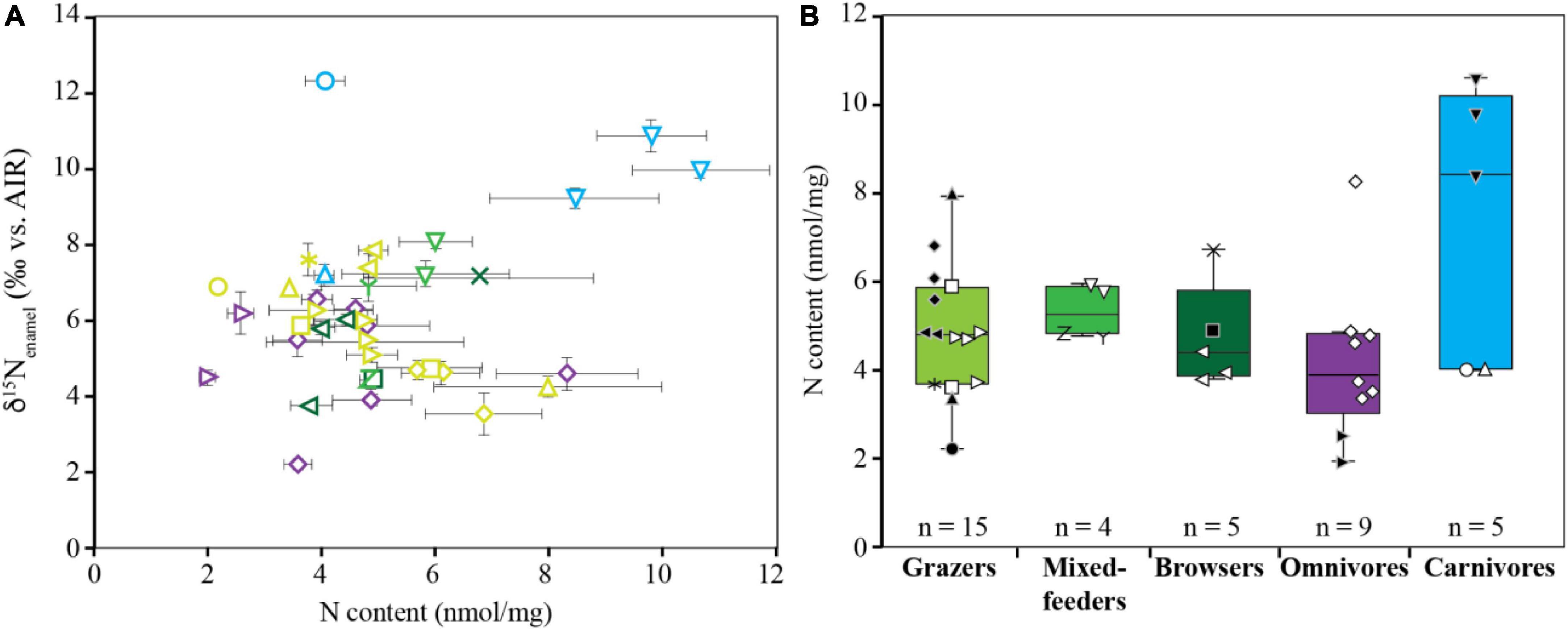
Figure 5. Biplot of δ15Nenamel values vs. N content of modern tooth enamel from GNP fauna (A) and N content grouped according to diet (B). For taxon-specific symbols, refer to Figures 2, 3.
Carbon isotope values
The complete range of C3 to C4 δ13C values is represented in the Gorongosa fauna. δ13Cenamel values range from –15.7 to 1.8‰, with mean δ13Cenamel values for herbivores of –5.4 ± 6.2‰ (n = 24), –10.6 ± 21.4‰ (n = 9) for omnivores, and –7.1 ± 2.9‰ (n = 5) for carnivores. δ13Cenamel values for grazing herbivores (mean = –1.1 ± 2.2‰; n = 15), mixed-feeders (–9.9 ± 4.7‰, n = 4), and browsers (–14.3 ± 1.5‰; n = 5) are significantly different (p < 0.001). Herbivores do not display significant differences in δ13Cenamel values when grouped according to digestive physiology [ruminant (n = 16) vs. non-ruminant (n = 8); p = 0.761]. Herbivorous obligate drinkers (n = 16) have higher δ13Cenamel values compared to non-obligate drinkers (n = 8; p = 0.002; Figure 4B).
The structural carbonate component of enamel powder ranges from 3 to 9% (Supplementary Table 1) with a mean of 6 ± 1%. Herbivores have slightly higher carbonate content than omnivores (p < 0.001), while the other dietary groups do not differ (p > 0.050).
Oxygen isotope values
The δ18Oenamel values for the Gorongosa fauna range from 26.0 to 33.1‰ with mean values of 30.5 ± 1.6‰ (n = 24) for herbivores, 28.6 ± 1.2‰ (n = 9) for omnivores, and 28.3 ± 1.2‰ for carnivores (n = 5). Herbivores differ statistically from other dietary groups (p < 0.008), while carnivores and omnivores are similar (p = 0.907). Ruminant δ18Oenamel values are significantly higher than those of non-ruminants (p < 0.001). δ18Oenamel values for herbivorous non-obligate drinkers are not significantly different than those of obligate drinkers (p = 0.108; Figure 4C).
The δ18Owater values of drinking water range from –6.2 to 13.1‰ with a mean value of –0.8 ± 5.3‰ (n = 42; Figure 6 and Supplementary Table 2). Groundwaters have the lowest δ18Owater values with an average of –5.2 ± 0.6‰ (n = 5), followed by waters sampled from rivers (–3.4 ± 1.8‰; n = 18), rainfall (–2.5 ± 1.3‰; n = 4), and lakes (4.1 ± 6.1‰; n = 15); this difference is statistically significant only when comparing lake water to samples from other reservoirs (p < 0.020). Lake δ18Owater values sampled in the wet season (–4.9 to –1.5‰; mean = –3.2 ± 1.4‰, n = 5) are significantly (p < 0.001) lower than dry season data (2.9–13.1‰; mean = 7.8 ± 3.4‰; n = 10), and there is no overlap in these two datasets.
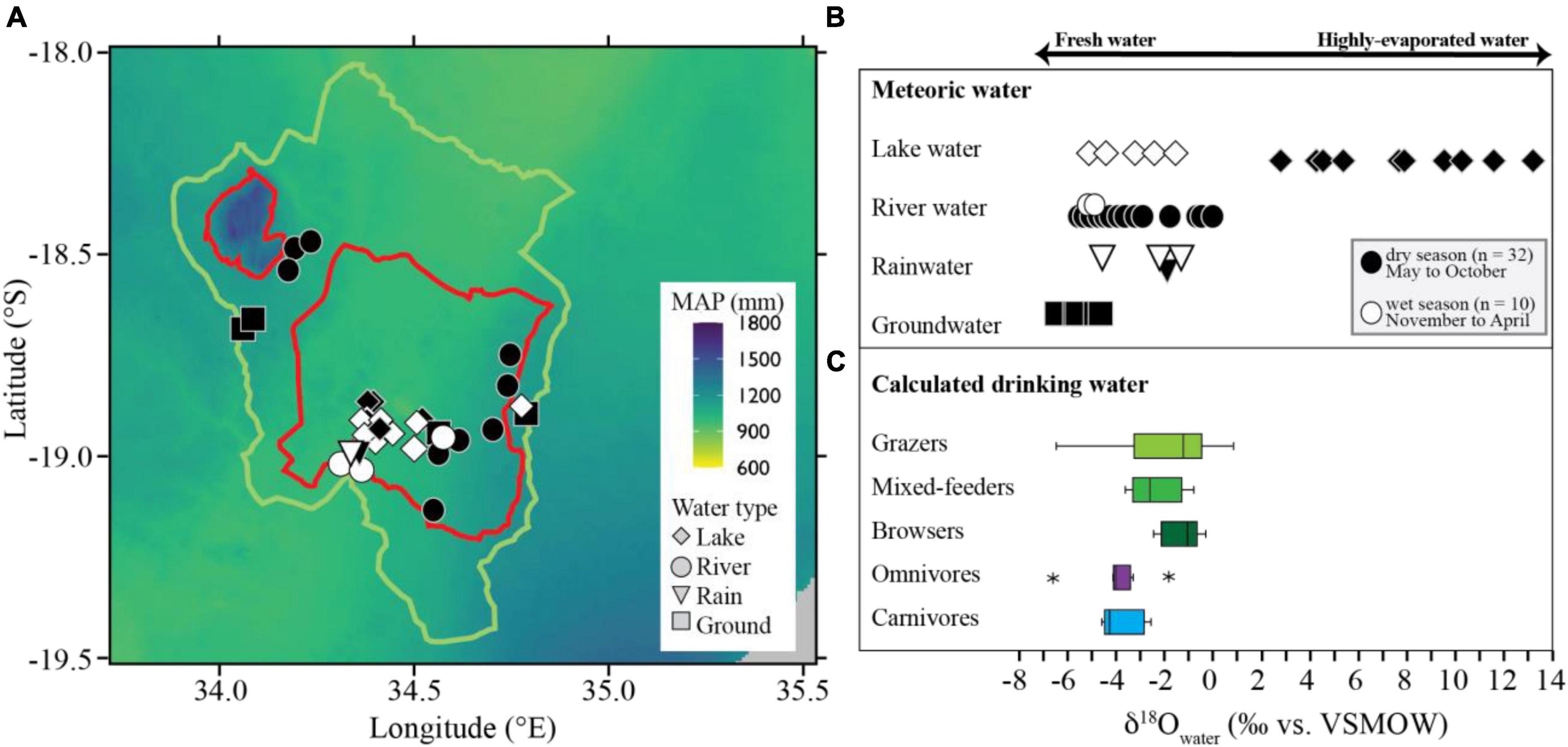
Figure 6. Map of mean annual precipitation (MAP) for Gorongosa National Park (red line), Buffer Zone (green line), and the surrounding region indicating the locations of water sampling sites included in this study (A). Mean annual precipitation data were generated using WorldClim version 2.1 bioclimatic variables (1970–2000) at a resolution of ∼1 km2 (Fick and Hijmans, 2017). δ18Owater values of meteoric water sampled in and around GNP during the dry (open symbols) and wet (closed symbols) seasons from 2016 to 2019 (B). Colored boxplots show the range of possible drinking water for each dietary group when bioapatite δ18Oenamel to is converted to δ18Owater (after Kohn and Cerling, 2002); stars indicate outliers (C).
Isotope niche analyses
Isotopic niche overlap, niche area, and distinction of niche space differed depending on the pairing of isotope values analyzed (Figure 7 and Supplementary Tables 6–8). We could not reject the null hypotheses for all Shapiro–Wilks tests indicating data were normal for both the individual feeding group by element (all groups p > 0.050) as well as the entire multivariate dataset (p = 0.212).
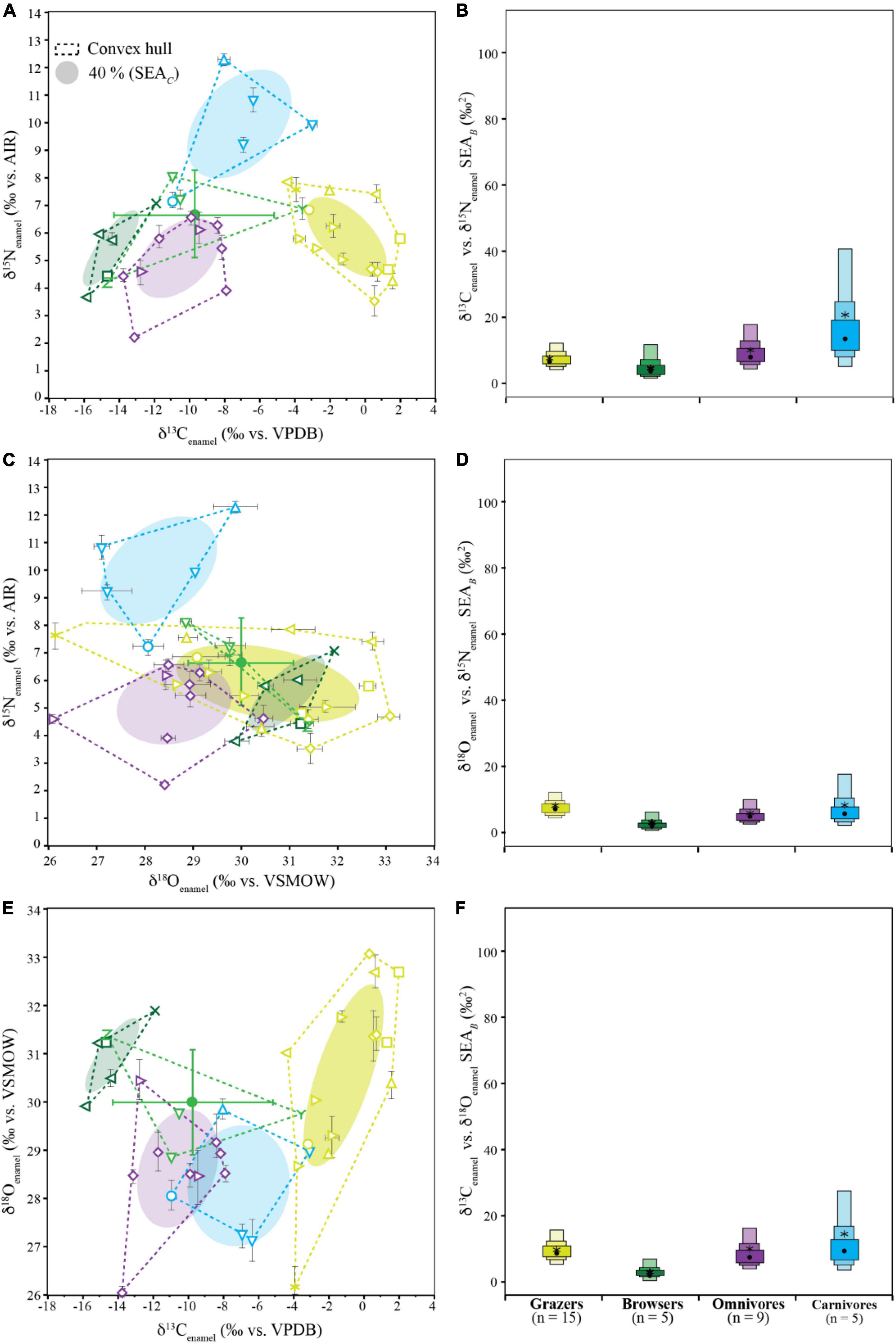
Figure 7. Biplots (A,C,E) and calculated SEAB boxplots (B,D,F) for δ13Cenamel vs. δ15Nenamel (A,B), δ18Oenamel vs. δ15Nenamel (C,D), and δ13Cenamel vs. δ18Oenamel (E,F). Biplots show raw isotope values with 1σ standard deviation, convex hulls encompass the full variation in the data, and ellipses indicate 40% estimated SEAC for Gorongosa fauna grouped by diet (light green: grazers; bright green: mixed-feeders; dark green: browsers; purple: omnivores; blue: carnivores; for taxon-specific symbols refer to Figure 2). Boxplots show the variability of SEAB, with 50, 75, and 95% credible intervals represented by light, medium, and dark colored boxes, respectively. Black dots are the SEAB mode and stars are the calculated SEAc, which correspond to biplot ellipses. Box plots and SEAC of mixed-feeders are not shown due to their small sample size (n = 4) instead, their mean values and 1σ standard deviation are given (green cycle with error bar).
For δ13Cenamel vs. δ15Nenamel, there is no SEAC overlap present (Figure 7A), and the Bayesian modes of niche size ranged from 4.0 to 13.1‰2 with no statistical differences based on 95% credible intervals (Figure 7B). All dietary groups were statistically distinct in isotopic space (p < 0.035).
For δ18Oenamel vs δ15Nenamel, SEAC overlap ranged from 0 to 78%, and the Bayesian modes of niche size ranged from 2.2 to 7.2‰2 with no statistical differences based on 95% credible intervals (Figure 7C). Isotopic niches were indistinct between grazers and browsers (p = 0.790) and were nearly indistinct between browsers and omnivores (p = 0.048). All other dietary groups were statistically distinct in isotopic space when compared to one another (p < 0.010).
For δ13Cenamel vs. δ18Oenamel, SEAC overlap ranged from 0 to 30% (Figure 7E), and the Bayesian modes of niche size ranged from 2.4 to 9.1‰2 with no statistical differences based on 95% credible intervals (Figure 7F). All dietary groups were statistically distinct in δ13Cenamel vs δ18Oenamel space when compared to one another (p < 0.020).
Sample sizes for some groups analyzed could affect calculated niche values. Small sample sizes (i.e., ∼5) have been shown to underrepresent ellipse area (Jackson et al., 2011) and increase niche size uncertainty (Syväranta et al., 2013), although SEAC aims to minimize this (Jackson et al., 2011). Increased replication could possibly change the niche overlap, shape, and areas of dietary groups. However, SEAB accounts for this variability in its credible intervals, and the Turner et al. (2010) analysis of centroid position remains statistically valid.
Discussion
We use stable isotope values in the tooth enamel of modern fauna living in and around GNP to (a) understand food web dynamics and dietary behavior in this well-constrained faunal community, (b) provide the first test of the suitability of using δ15Nenamel data in a natural ecosystem to reconstruct trophic level, (c) apply a new method for measuring δ13Cenamel and δ18Oenamel of ≤100 μg of tooth enamel, and (d) investigate isotopic niche overlap, size, and separation within and between groups by analyzing the three isotope systems (δ13Cenamel, δ15Nenamel, and δ18Oenamel).
Reconstructing trophic levels using δ15Nenamel
Gorongosa carnivores have an average of 4.0‰ higher δ15Nenamel values and occupy distinct isotopic niche space compared to herbivores (Figures 3, 7). This trophic enrichment agrees well with a documented 3–5‰ increase in δ15N between diet and consumer reported by numerous large-scale ecological studies using other biological tissues (e.g., Schoeninger and DeNiro, 1984; Bocherens and Drucker, 2003; Caut et al., 2009). These results clearly show that δ15Nenamel values obtained with the “oxidation-denitrification method” reflect expected patterns in natural settings and have great potential for reconstructing (paleo)food webs.
Herbivore δ15Nenamel
In our dataset, herbivore δ15Nenamel values vary by 4.3‰, with values ranging from 3.8 to 8.1‰ (Figure 3A). Similar variability has been reported for herbivores in studies using bone collagen and is believed to be related to differences in feeding strategies (e.g., grazing vs. browsing), digestive physiology (e.g., ruminant vs. non-ruminant), the nutritional value of the consumed plant material (e.g., low vs. high protein content), foraging habitat (e.g., savanna vs. forest) (Ambrose, 1991; Robbins et al., 2005), and location (Leichliter et al., 2022). However, we do not observe any significant differences in δ15Nenamel between herbivores grouped according to grazing, browsing, or mixed-feeding (Figure 3A), ruminant vs. non-ruminant, or water dependency (Figure 4A).
These results suggest that local variation in plant δ15N values (likely associated with variation in soil δ15N) appears to be the principal mechanism influencing herbivore δ15N values. The observed 4.3‰ variation in δ15Nenamel within the herbivore trophic level agrees well with the reported 4‰ baseline variation in δ15N of savanna plants from different microhabitats of Kruger National Park in South Africa (Codron et al., 2005). This dry savanna biome lies ca. 650 km southwest of GNP and is similar in size to GNP and its Buffer Zone. In Kruger, some deciduous trees (e.g., Cassia abbreviate) and tussock-forming grasses (e.g., Heteropogon contortus and Themeda triandra) have low δ15N values (≤1.5‰), while other trees (e.g., Ficus sycomorus, Grewia sp., and Ziziphus mucronate) and grasses (e.g., Dactyloctenium australe) have much higher δ15N values (>5.0‰). All these plant taxa are present in GNP and, therefore, available as a food resource for the studied herbivores. Different ungulate individuals occupy different habitats within GNP (e.g., floodplain vs. savanna), where they are observed to eat radically different diets (Becker et al., 2021), and therefore possibly consume plants with varying nitrogen isotope values.
Therefore, the large variety of herbivore δ15Nenamel is expected and probably reflects variations in plant δ15N values in GNP with its diverse microhabitats, soil types, plant taxa, and water availability, even if faunal migration ranges are relatively small (Stalmans and Beilfuss, 2008).
Omnivore δ15Nenamel
The δ15Nenamel values for omnivores are significantly different from those of carnivores, but not herbivores (Figure 3A and Table 1). Our omnivore dataset includes seven baboons (Papio ursinus with genetic variants of P. cynocephalus, Santander et al., 2022) and two bushpigs (Potamochoerus larvatus); the two taxa are indistinguishable in their δ15Nenamel values.
Plant material consumed by baboons mainly consists of specific plant parts such as fruits, drupes, tubers, and other underground materials, depending on the habitat and foraging season (Nowak, 1999). The baboons sampled for this study have δ15Nenamel values between 2.2 and 6.6‰, a large range of variation in δ15Nenamel despite the fact that six of the seven specimens were collected within a small geographic area (0.6 km2) of open C4 grasslands with some isolated acacia trees and could possibly have even belonged to the same troop (Figure 2 and Supplementary Table 2). This large intra-taxon variation indicates a diverse and flexible diet and possibly distinct individual dietary preferences.
Baboons and bushpigs are omnivorous generalists, their diet often consisting of up to 30% of animal resources (e.g., insects, small mammals, small reptiles, eggs, and nestlings). GNP baboons have been observed to consume mussels and snails, as well as to hunt small mammals (e.g., warthog, reedbuck or bushbuck infants, and ducklings) on rare occasions, usually during the birthing season (L. Lewis-Bevan, personal communication). However, only adults (usually dominant males) have been directly observed consuming meat, although high-ranking adult females occasionally consume scraps discarded by the males. Young, low-ranking troop members, however, whose teeth are still mineralizing, rarely have access to these protein-rich resources before vultures claim leftovers. The teeth sampled for this study would have formed during this juvenile period, which likely explains the low δ15Nenamel values observed for the GNP baboons. Moreover, while occasional meat consumption should increase omnivore δ15Nenamel values, available data on bone collagen and fecal samples show that chacma baboons tend to have low δ15N values compared to sympatric herbivores (Ambrose and DeNiro, 1986; Codron et al., 2006). This pattern is possibly related to the consumption of N2-fixing plants, underground storage organs, fruits, roots, and termites, all of which have relatively low δ15N values (Codron et al., 2005, 2007).
Despite the fact that the two sampled bushpigs (Potamochoerus larvatus) were found over 120 km apart, they have similar δ15Nenamel values, which are comparable to those of the baboons (Figure 3A). While baboons are not observed to scavenge, bushpigs do eat carrion, and their opportunistic diet includes roots, crops, bulbs, insects, and fallen fruit (e.g., discarded by baboons; Ghiglieri et al., 2008).
Carnivore δ15Nenamel
Carnivore δ15Nenamel values are significantly higher than the values of the other dietary groups (p < 0.001; Figure 3A). Compared to their potential prey the GNP carnivores had, on average 4.0‰ higher δ15Nenamel values. This trophic enrichment falls well within the expected range of 3–5‰ between trophic levels (Figure 1). While our carnivore dataset is small, niche separation between carnivore taxa is observed. The lion (Panthera leo) has the highest δ15Nenamel value (12.3‰) recorded in the GNP dataset, indicating that it fed on prey with high δ15Nenamel values. In contrast, the only other felid, a single leopard (Panthera pardus), has the lowest δ15Nenamel value of all the carnivores and is the only individual that overlaps with herbivores (but not omnivores). Its δ15Nenamel value of 7.2‰ is several permil lower than the other analyzed carnivores. δ15Nenamel values lower than coexisting carnivores have also been reported from two leopards from Angola (Leichliter et al., 2022). This is probably the result of different habitat use and prey preference, which is also apparent in the leopard’s more negative δ13Cenamel values compared to the GNP lion and crocodiles (refer to δ13Cenamel section; Figure 3B). Accordingly, our bulk δ15Nenamel data possibly reflect a time in the animal’s life where it fed on prey with low δ15N tissue values.
Only one apex predator, the Nile crocodile (Crocodylus niloticus), sustains a stable population in GNP (Stalmans et al., 2014). In this study, only fully grown C. niloticus were sampled, as physiological and ontogenetic factors such as body size can influence their δ15N values (Villamarin et al., 2018). GNP crocodiles are reported to be highly generalized predators, feeding on carnivorous and herbivorous fish species (i.e., Siluriformes) as well as mammalian prey (Wilson, 2014). However, crocodilians which consume a large variety of prey from tropical coastal floodplains derive the majority of their nutrition from the consumption of (occasional) terrestrial prey (Adame et al., 2018). Thus, while the aquatic prey consumed by GNP crocodiles may influence the δ15N values of the crocodiles to some degree (these resources derive from the aquatic food chain with a possibly different N isotope baseline), the nitrogen isotope values of the crocodilians should reflect mostly their terrestrial prey. The GNP crocodilian’s δ15Nenamel values fall well between those of the two felids, suggesting that both groups share isotopic niche space.
We analyzed all carnivore specimens available from GNP at the time of this study. The lion died naturally within the park’s boundaries (Figure 1) and the leopard was poached within the Buffer Zone (the body was later confiscated by park rangers, exact coordinates were not reported). Planned analyses will incorporate additional GNP predators as well as aquatic resources for their isotopic composition once they become available, to fully understand the observed patterns. However, even our small dataset shows promising results from an ecological perspective, and more importantly, shows expected δ15N patterns in enamel.
Nitrogen contents in tooth enamel
Nitrogen contents of mammalian tooth enamel from GNP fauna have a relatively small range (2.0–8.3 nmol/mg) and do not show any correlation with δ15Nenamel. Moreover, N contents are remarkably similar across different individuals and dietary groups (p = 0.357) and are in good agreement with those previously reported for rodents in a controlled feeding experiment (Leichliter et al., 2021) and other mammals from different sites across Africa (Leichliter et al., 2022). These findings suggest that mammalian tooth enamel N content is relatively consistent regardless of feeding strategy.
Interestingly, the nitrogen content of crocodile tooth enamel is, on average, nearly 5 nmol/mg higher than any of the mammalian taxa [9.6 ± 1.1 nmol/mg for crocodiles (n = 3) vs. 4.7 ± 1.4 nmol/mg for mammalians (n = 35); Figure 5 and Table 1]. While the structure of reptilian enamel is reported to be generally similar to mammalian enamel (Dauphin and Williams, 2008), we were unable to find any information regarding the N content of reptilian tooth enamel. Our results suggest that reptilian tooth enamel contains more nitrogen than mammalian tooth enamel, possibly as the result of differences in their respective physiology or mineralization mechanisms. We considered the possibility that the higher N content of the reptilian specimens was caused by contamination with dentin during sampling. Measurements of dentin N content of in-house standard AG-Lox (n = 3) indicate that the nitrogen content is substantially lower in dentin than in enamel after the reductive-oxidative cleaning (Supplementary Table 9). This is not surprising because dentin has a lower proportion of mineral-bound organic material that can survive the cleaning treatment. Therefore, possible contamination with dentin cannot be the cause of the elevated N contents of the analyzed crocodile enamel.
Overall, the fact that mammalian tooth enamel N content is relatively consistent and is not correlated with δ15Nenamel suggests that N content could be used in paleodietary studies as a diagnostic tool to asses potential signs of diagenetic alteration or contamination by exogenous organic N, as it has been suggested for invertebrate organisms, such as foraminifera and corals (Ren et al., 2009; Straub et al., 2013; Martinez-Garcia et al., 2014; Ren et al., 2017; Wang et al., 2017; Kast et al., 2019, Auderset et al., 2022).
Reconstructing plant-based diet using δ13Cenamel
Our δ13Cenamel data reflects the niche partitioning that is present between taxa feeding on different plants, and animals consuming prey with different feeding strategies (Figures 3B, 7).
Herbivore δ13Cenamel
Herbivore δ13Cenamel data from GNP indicate a wide range of foraging strategies, including mixed-feeders, browsers, and grazers (Figure 3B), reflecting the wide range of ecosystems present in the park (Figure 2).
Within the GNP herbivores, browsers have δ13Cenamel values of –11.8‰ to –15.8‰, reflecting diets consisting almost exclusively of C3 plants (≥93%; calculated after Cerling et al., 2011). Most grazers, in contrast, have δ13Cenamel values > –2‰ which reflect at least 70% of C4 consumption. Three individuals are hypergrazers with >95% C4 grass consumption (one buffalo and two sable antelopes). However, five individuals (two warthogs and one zebra, waterbuck, and hippo) that are typically considered to be grazing species have somewhat low δ13Cenamel value (–2.7‰ to –4.3‰), reflecting up to 45% of C3 intake (refer to Figure 3B). This unusually high C3 consumption may be the result of grazing on C3 grasses (Oryza longistaminata), which grow in the floodplain grasslands near Lake Urema, or feeding on forbs and other C3 understory vegetation. For example, hippos are reported to feed on Oryza (Noirard et al., 2008), and we observed warthogs in GNP digging up the underground rhizomes of Oryza and sedges.
Mixed-feeders usually have a diet of >30% C4 grass and >30% C3 browse, resulting in δ13Cenamel values between –2‰ and –8‰. In the GNP dataset, only the impala (Aepyceros melampus) has a mixed-feeder δ13Cenamel value (Figure 3B). Impalas are known to exhibit dietary flexibility and rely on browse in some areas and graze in others, sometimes on a seasonal basis which can result in a large range of δ13Cenamel values (Monro, 1980; Sponheimer et al., 2003). The single impala specimen from GNP has a δ13Cenamel value of –3.6‰ corresponding to ca. 37% C3 consumption. In contrast, the analyzed nyala (Tragelaphus angasii) and the two elephants (Loxodonta africana) primarily browsed (≥85% C3 biomass). African elephants are also mixed-feeding generalists, but with a diet that consists largely of C3 browse (Codron et al., 2012), agreeing well with our data. Gorongosa elephants have been regularly observed raiding nutritious crops (e.g., fruits, maize, and tubers) in the Buffer Zone when the quality and abundance of natural forages are low within the park (Branco et al., 2019b). The majority of these crops are C3 plants (e.g., banana, tomato, papaya, peas, sweet potato, pumpkin, and sorghum; Sage and Zhu, 2011); only maize and sugar cane are C4. Hence, crop raiding probably contributed to the low δ13Cenamel values observed for the elephants.
Herbivore δ13Cenamel values do not differ when grouped by digestive physiology (Figure 4B), as ruminants include bovids with diverse feeding behaviors (grazers, mixed-feeders, and browsers) and have a large range in δ13Cenamel values which overlap with the non-ruminants. In our dataset, the non-ruminants are mostly grazers with high δ13Cenamel values, but also include elephants that ate C3 plants. Obligate drinkers have statistically higher δ13Cenamel values compared to non-obligate drinkers, likely reflecting the higher water content of browse compared to graze (i.e., mostly C4 grasses) which must be supplemented with drinking water.
Overall, δ13Cenamel data reflects the diversity of GNP habitats, providing niches for herbivores with different feeding behaviors, ranging from hyperbrowsers to hypergrazers.
Omnivore δ13Cenamel
Omnivore δ13Cenamel values (–13.7 to –8.2‰) indicate a predominantly C3 diet for Gorongosa baboons and bushpigs (Figure 3B). Grasses and other C4-based foods comprise less than a third of the bulk diet of all sampled individuals, and three individuals (two primates and one suid) consumed an exclusively C3 diet (Figure 3C and Supplementary Table 1). One of the bushpigs, which are typically forest-dwelling, was collected in a C4-dominated grassland (Digitaria swaziensis; Supplementary Table 1), yet its low δ13Cenamel value (–13.7‰) reflects pure browsing, suggesting that dietary preference rather than the locally dominant vegetation drove the feeding behavior in this individual. Overall, our findings are consistent with previously published δ13C data for baboons and bushpigs which also indicate a diet consisting largely of C3 foods (e.g., Ambrose and DeNiro, 1986; Thackeray et al., 1996; Codron et al., 2006; Venter and Kalule-Sabiti, 2016 and references therein).
Carnivore δ13Cenamel
Few studies have investigated the δ13C (or δ18O) ecology of carnivores in Africa (Codron et al., 2007, 2016, 2018; Voigt et al., 2018; Hopley et al., 2022), which makes interpretation of the GNP dataset challenging. The GNP carnivores have δ13Cenamel values which fall between those of herbivores and omnivores, indicating that grazers, browsers, mixed-feeders, and/or omnivores were all potentially consumed (Figure 3B). The three crocodiles have the highest δ13Cenamel values (between –6.9‰ and –3.1‰), which could be the result of the consumption of aquatic resources, or grazers and mixed-feeders, while the single leopard individual’s low δ13Cenamel value (–11.0‰) indicates that it was feeding almost exclusively on browsing taxa. The lion’s higher δ13Cenamel (–8.1‰) indicates that this individual preyed on more grazers compared to the leopard. According to field observations (Bouley et al., 2018), waterbuck (δ13Cenamel = –4.3 to 0.6‰) make up ca. 60% of the prey biomass consumed by Gorongosa’s lions. The lion’s intermediate δ13Cenamel value indicates that it must have also consumed some prey with lower δ13C values such as browsers (e.g., kudu, eland, and bushbuck) or mixed-feeders with a C3-dominated diet (e.g., nyala). The specimen for this study was collected in the tall-grass margins that border the savanna woodlands, which is a preferred hunting habitat for lions as it provides access to abundant grazing, browsing, and mixed-feeding prey. The lion’s δ13Cenamel is nearly 3‰ higher than that of the leopard, indicating that it focused more on grazing prey while the leopard consumed more browsers. A similar difference in δ13Cenamel between leopard and lion is observed in a modern felid dataset from Turkana Basin (Kenya; Hopley et al., 2022).
Reconstructing drinking behavior using δ18Oenamel and δ18Owater
δ18Owater of meteoric water sampled between 2016 and 2019 within GNP agree well with data reported from meteoric water stations of the Global Network of Isotopes (GNI) in Precipitation (sampled in Chitengo in 2010 to 2011; IAEA/WISER, 2020a) and GNI in Rivers (Pungwe River in 2009; IAEA/WISER, 2020b). Moreover, data fall on the Local Meteoric Water Line (LMWL) and Local Evaporation Line (LEL) established with δ18Owater and deuterium (δ2Hwater) data of samples from springs, boreholes, river, and Lake Urema taken in the Urema catchment in the period 2006–2010 (Steinbruch and Weise, 2014). Note that the δ2Hwater values are reported in Supplementary Table 2 but are not discussed in this study.
δ18Owater of available drinking water reported in this study has a range of >20‰ (Figure 6), and varies much more than the δ18Oenamel values measured for the fauna (i.e., ∼7‰, Figure 3C). After converting bioapatite δ18Oenamel to δ18Owater (using equations by Kohn and Cerling, 2002), the measured range of 26.0 to 33.1‰ in δ18Oenamel translates into the intake of water with δ18Owater values between –6.6‰ and 1.0‰, which falls, with the exception of a hippo and a baboon specimen, in low to medium range of measured meteoric waters (–6.2 to 13.1‰; Figure 6). This indicates that the animals in this study consumed primarily fresh water that was only moderately influenced by evaporative enrichment. Time-averaging of temporal signals during the progressive mineralization of tooth enamel could dampen the variation in δ18Owater ingested by a single individual through time (i.e., information about seasonal variability of the drinking water is lost).
Meteoric water δ18Owater
Potentially available drinking water for animals living in GNP overlaps greatly in their δ18Owater values and falls generally between –6.2 and 0.8‰, with the exception of dry season lake waters, which range from 2.9‰ up to 13.1‰ (Figure 6). Therefore, dry season lake water displays significantly higher δ18Owater values, compared to all other types of water (p < 0.001; there is no overlap with other δ18Owater datasets; Figure 6). During the dry season, standing water in lakes or ponds is most strongly influenced by evaporation (Clark and Fritz, 1997; Kendall and McDonnell, 1998). δ18Owater of rainwater sampled during the wet season and rain samples were taken from the end of the dry season overlap. This agrees well with previously published isotope data from Gorongosa meteoric waters, which indicate that wet season rainfall was formed over the Indian Ocean without undergoing major fractionation, while dry season rainfall comes partly from the same source, and also indicates locally evaporated or recycled waters from the floodplains of the Urema Graben (Steinbruch and Weise, 2014).
Herbivore δ18Oenamel
With a mean of 30.6 ± 1.6‰ (n = 24) herbivores have the highest δ18Oenamel values compared to other dietary groups (Figure 3C), but no differences are observed for browsing, mixed-feeding, or grazing taxa, indicating similar drinking behaviors, from a mix of sources, among these groups. Ranging from 26.2 to 33.1‰, δ18Oenamel values of grazers have the largest spread of any dietary group. In herbivores, ruminants have significantly higher δ18Oenamel values compared to non-ruminants, but obligate drinkers do not have significantly different δ18Oenamel values compared to non-obligate drinkers (Figure 4C). This indicates that animals had regular access to fresh water that has experienced only limited evaporation, or to leaf water and/or parts of plants that are not affected much by evaporation (e.g., stems, roots, bark, and fruits) in mesic environments.
No significant correlation was observed between δ13Cenamel and δ18Oenamel for most groups; however, a strong and significant positive relationship exists between δ13Cenamel and δ18Oenamel in grazers and obligate drinkers (r = 0.700; p = 0.004 for grazers; r = 0.493; p = 0.052 for obligate drinkers), indicating increased intake of 18O-depleted water (e.g., from Lake Urema or waterholes) associated with greater C4 plant consumption (e.g., grasses from the floodplain) for these groups.
Omnivore δ18Oenamel
Omnivore δ18Oenamel values are significantly lower than those of herbivores but overlap with those of carnivores. The two bushpigs show slightly lower δ18Oenamel values compared to the seven baboons. As obligate drinkers, both δ18Oenamel values in both taxa reflect the oxygen isotope composition of local meteoric waters as expected (Moritz et al., 2012; Steinbruch and Weise, 2014). Their relatively low δ18Oenamel values point toward a regular recharge of their body water by drinking from meteoric sources which are only moderately influenced by evaporation (Fricke and O’Neil, 1996; Levin et al., 2006; Blumenthal et al., 2017), limited intake of evaporated plant tissues or animal fats (refer to nitrogen section; Crowley, 2012; Nelson, 2013; Carter and Bradbury, 2016), and little sweating or panting (thermoregulatory processes which induce evaporative fractionation; Kohn et al., 1996; Sponheimer and Lee-Thorp, 1999).
Carnivore δ18Oenamel
Studies about the water requirements of African savanna carnivores are rare and observations of drinking behavior in Gorongosa’s felids are anecdotal; thus, oxygen stable isotope data can provide valuable additional information regarding water intake in predators.
Most African felids have low water needs and receive their moisture from the metabolic water of their prey (Bothma and Walker, 2013), but drink water from waterholes when it is available (Wilson and Mittermeier, 2009; Hayward and Hayward, 2012). Through this intake of prey body fluids and standing water, δ18Oenamel data of lions and leopards from eastern Africa are strongly influenced by local meteoric δ18Owater, similar to what has been documented for herbivores (Kohn, 1996; Hopley et al., 2022). Gorongosa carnivores have, on average, ca. 2‰ lower δ18Oenamel values compared to their prey (Figure 3C), and therefore relatively low calculated δ18Odrinking–water. This suggests that the GNP carnivores had access to freshwater and drank regularly. While the lion’s δ18Oenamel value overlaps with those of the herbivores, the leopard’s does not, possibly indicating that the leopard consumed more fresh river water rather than evaporated lake water.
A rough linear correlation is reported between the oxygen isotopic ratios of crocodiles’ phosphate tooth enamel and ambient water which is influenced by mean air temperature, diet, and physiology (Amiot et al., 2007). GNP crocodile carbonate δ18Oenamel values are generally low, indicating that the sampled individuals lived in relatively fresh water that was rarely influenced by evaporation.
Isotopic niches and inferred ecology from paired δ13Cenamel, δ15Nenamel, and δ18Oenamel data
Traditionally, paired carbon and nitrogen isotope values are analyzed in ecological studies to reconstruct isotopic niches and infer some trophic information (Newsome et al., 2007). Until recently, this type of reconstruction using tooth enamel has not been possible due to the methodological limitations of measuring δ15Nenamel (Leichliter et al., 2021). This study is the first to combine nitrogen, carbon, and oxygen stable isotope values obtained from diagenetically robust tooth enamel, permitting ecological interpretations based on the combination of these three isotopes. We compare the different pairs of isotope values (δ13Cenamel-δ15Nenamel, δ15Nenamel-δ18Oenamel, and δ13Cenamel-δ18Oenamel; Figure 7) to investigate niche overlap, size, and separation within and between dietary groups of modern fauna from GNP. Isotopic niches calculated in this way can be used to infer information about trophic ecology and resources used by determined groups (Layman et al., 2007; Newsome et al., 2007; Layman and Allgeier, 2012). Given the small sample size of the mixed-feeder (n = 4; not included in niche analysis), browser (n = 5), and carnivore (n = 5) groups, we consider our statistical conclusions to be preliminary but promising. Our isotopic niche results are best interpreted as (1) relative but not absolute values for niche overlap; (2) SEAB is variable and increased sample size would likely decrease the uncertainty around the estimates and lead to a more robust analysis resolving potential size differences, but generalities are valid; and (3) unique locations in isotopic space are statistically accurate, and small changes to niche size from additional sampling would likely not move centroids enough to effect centroid distance. Additional Bayesian analyses are becoming increasingly popular in ecological studies and could also be included in future multi-isotope tooth enamel studies if sample sizes are increased. For example, 3-dimensional isotopic analysis using SIBER has proven useful for determining isotopic niches of complex trophic systems such as coral reef atolls (Cybulski et al., 2022), and other analyses such as nicheROVER allow for incorporation of additional dimensions of isotopes or other continuous ecological indicators (Swanson et al., 2015). Even with uncertainties, isotopic investigations coupled with Bayesian statistics have significant implications for future (paleo)ecological reconstructions.
In the GNP fauna, the niches of different dietary groups are completely distinct in δ13Cenamel-δ15Nenamel space (Figure 7A), with no SEAC overlap between any dietary groups (Supplementary Table 6). Thus, animals with these diets can be classified into distinct isotopic niches based on their carbon and nitrogen isotope values in our dataset. Although we cannot quantify the niche of the mixed-feeders due to limited sampling, we can explore potential overlap with other groups. For example, even with only four samples, mixed-feeders convex-hull overlaps with the omnivores niche in δ13Cenamel-δ15Nenamel space. This overlap would be expected, as it occurs around typical δ13Cenamel values for C3 dominated diets (Cerling et al., 2003), on which GNP omnivores and some of the analyzed mixed-feeders (nyala and elephants) rely (Figure 3B and Supplementary Table 1). We would expect that even with additional sampling, isotopic niche overlap between these two groups would remain.
δ18O data are not frequently used in ecological studies; however, some research has shown that δ18O can complement the information provided by carbon and nitrogen (e.g., Crowley et al., 2015; Roberts, 2017). Gorongosa grazers exhibit a wide range of δ18Oenamel values and share δ15Nenamel-δ18Oenamel niche space with browsers (Figures 7B,E). Omnivores tend to have lower δ18Oenamel values and only overlap slightly with grazers in δ15Nenamel-δ18Oenamel niche space. Due to the trophic enrichment in δ15Nenamel and relatively low δ18Oenamel values, carnivores do not overlap with any other group in δ15Nenamel-δ18Oenamel space. This shows that animals with a plant-dominated diet (i.e., herbivores and omnivores) cannot be distinguished in δ15Nenamel-δ18Oenamel alone. In contrast, carnivores with their specialized diet occupy a distinct isotopic space and can be clearly distinguished from other groups, even though terrestrial mammalian and aquatic reptilian carnivores were combined in this group. While δ15Nenamel vs. δ18Oenamel comparisons can potentially reveal isotope niche distinction between some dietary groups, niche separation is mostly driven by trophic elevation in δ15Nenamel in this dataset.
In δ13Cenamel–δ18Oenamel space (Figure 7C), the SEAC of the grazers and browsers are well separated, while omnivores and carnivores overlap by 20–30%. The convex hull of the four sampled mixed-feeders overlaps with all isotopic niches except the grazers, indicating that further sampling may lead to significant niche overlaps. In the δ13Cenamel vs. δ18Oenamel biplot, most of the dietary information is derived from carbon. The small trophic enrichment of 1% sometimes observed in δ13C of tissues (DeNiro and Epstein, 1981; Schoeniger and DeNiro, 1984; Bocherens and Drucker, 2003; O’Connell et al., 2012) is obscured by differences in δ13C between consumed C3 and C4 plants (or prey that consumed those plants; Cerling and Harris, 1999; Hopley et al., 2022), while δ18Oenamel cannot serve as a trophic proxy (but can reveal information about drinking behavior and source waters instead). This shows that there is a minimal ecologically expected organization of isotopic niches without the information gained from δ15Nenamel data.
In conclusion, analysis of combined carbon, nitrogen, and oxygen stable isotope values leads to the isotopic separation of grazers, browsers, omnivores, and carnivores dietary groups, with significant niche overlap of mixed-feeding herbivores with all groups expected upon further sampling. Niche separation is clearest in δ13Cenamel-δ15Nenamel space with no overlap between any of the dietary groups. This illustrates the high potential of δ15Nenamel combined with δ13Cenamel and δ18Oenamel measured from a single aliquot of tooth enamel for reconstructing isotopic niches and inferring dietary and trophic information. Carnivores—arguably the most isotopically distinguishable group—provide a useful example of the benefits of this type of combined isotopic approach. Typical and recent studies of trophic behavior using stable isotope data from teeth and/or bone have been limited to δ13C and δ18O (e.g., Domingo et al., 2020), though zinc (Bourgon et al., 2020, 2021; McCormack et al., 2021; Jaouen et al., 2022) and calcium (Martin et al., 2015, 2020, 2022) are also used for trophic studies. If we consider only δ13Cenamel vs. δ18Oenamel, carnivores would not occupy a distinct isotopic niche space since they would overlap with omnivores (20%) and with the mixed-feeders convex hull. Ecologically, however, carnivores occupy a distinct dietary niche, as they consume animal resources almost exclusively. In contrast, GNP omnivores (baboons and bushpigs) consume meat only occasionally while mixed-feeders rely exclusively on plant biomass. Thus, carnivores should not overlap with either group unless there is wide variation in baseline δ15N (refer e.g., Schmidt and Stewart, 2003; Codron et al., 2005). The addition of δ15Nenamel to both δ13Cenamel and δ18Oenamel analysis clearly shows that carnivores do occupy a statistically significant isotopic niche space, with no SEAC overlap with any other groups’ niche.
Conclusion
We present the first stable carbon, nitrogen, and oxygen isotope data from tooth enamel for fauna from GNP. We validate two novel geochemical methods and draw conclusions about the dietary patterns of animals living within this well-constrained and well-studied African ecosystem. While field observations are extremely useful for understanding the dietary ecology of modern fauna, they are very labor intensive, requiring following an individual (or a group of animals) for days or months, or the deployment and evaluation of hours of camera trap footage. Moreover, such studies often only record a snapshot in time (e.g., one single feeding event) rather than capturing long-term behavior. In contrast, our bulk stable isotope data of herbivores (including grazers, mixed-feeders, and browsers), omnivores, and carnivores, reflect δ13C, δ15N, and δ18O of consumed food and water averaged over the course of tooth enamel mineralization.
Our results generally exhibit robust isotopic patterns and therefore support ecological information about the trophic level, dietary niche, and resource consumption. We show that δ15Nenamel analyzed with the “oxidation-denitrification method” records the trophic position of an individual within its local food web. This is evidenced by a trophic enrichment of 4.0‰ between herbivores and carnivores in the GNP dataset. This method applies not only to a wide range of sample-limited ecosystems but also has potential applications in paleoecology. δ13Cenamel analysis using the “cold trap method,” tailored to measure small sample sizes (down to 50 μg tooth enamel), distinguishes C3 and/or C4 biomass consumption, while δ18Oenamel values reflect drinking water.
This first tri-isotope approach conducted on the tooth enamel of fauna from a single, well-constrained ecosystem indicates that combined C, N, and O isotope data analyses permit the separation of grazers, browsers, omnivores, and carnivores according to their isotopic niche, while preliminary analysis of mixed-feeding herbivores indicate that they cannot be clearly distinguished from other groups. This illustrates the high potential of our multi-isotope approach for paleontological applications using diagenetically robust tooth enamel. We plan to apply this novel multi-isotope approach to recently discovered vertebrate fossils from GNP, which represent the only Miocene fossil locality in the southern East African Rift (Habermann et al., 2019; Bobe et al., 2021). Thus, the datasets presented here will serve as an excellent comparison for the interpretation of fossil stable isotope data.
Data availability statement
The original contributions presented in this study are included in the article/Supplementary material, further inquiries can be directed to the corresponding authors.
Ethics statement
Ethical review and approval was not required for the animal study because samples were taken from specimens collected after natural death and curated by the faunal bone collection of Gorongosa National Park.
Author contributions
TL and JL: conceptualization and analysis. TL, JL, AF, ND, HV, DS, and AM-G: methodology. RB and SC: Paleo-Primate Project Gorongosa direction. TL, VA, MB, DB, DRB, CC, MF, JH, FM, JM, RB, and SC: fieldwork. JM and RB: curating of PPPG collection. TL, JL, and JC: statistical analysis. TL, JL, and AM-G: writing—original draft. All authors contributed to the article and approved the submitted version.
Funding
This study was funded by the Deutsche Forschungsgemeinschaft (DFG) grant LU 2199/1-1 and Emmy Noether Fellowship LU 2199/2-1 to TL, the Max Planck Society to GH, HV, and AM-G, and the National Geographic Society grants NGS-51478R to TL and JH, NGS-57285R to SC, and NGS-51140R-18 to RB. MF worked under an FCT-funded associate researcher contract (CEECIND/01937/2017), AM-G received funding from DFG grant MA 8270/1-1, and ND from the Paul Crutzen Nobel Prize fellowship of the Max Planck Society. The Paleo-Primate-Project Gorongosa was funded by the Gorongosa Restoration Project, the Leverhulme Trust (Philip Leverhulme Prize 114 to SC); the John Fell Fund, Oxford, and the St Hugh’s College, University of Oxford.
Acknowledgments
We are grateful to the Gorongosa Restoration Project and especially Greg Carr for their vital support of the Paleo-Primate Project Gorongosa. Research and export permits were granted by the Direcção Nacional do Património Cultural, Mozambique, with the support of professors H. Madiquida and S. Macamo of Eduardo Mondlane University. We thank the dedicated staff from Gorongosa National Park, the fiscais, our students, and colleagues across many institutions who have been very enthusiastic about this project. Special thanks to P. Hammond, L. Lewis-Bevan (University of Oxford), and R. A. Farassi (Universidade Eduardo Mondlane) for first-hand observational data on Gorongosa baboons, and E. W. Negash (George Washington University) for helpful comments on the manuscript. We thank J. Delinger and M. Stalmans for their vital support and assistance with data collection throughout the years. We also thank F. Rubach, S. Brömme, M. Schmitt, B. Hinnenberg (Max Planck Institute for Chemistry, Germany), and U. Treffert (Senckenberg Biodiversity and Climate Research Centre, Frankfurt, Germany) for technical support, and I. Conti-Jerpe (UC Berkley) for the SIBER advice. This manuscript was greatly improved by the thoughtful comments of VB, JR, LK, and the editor.
Conflict of interest
The authors declare that the research was conducted in the absence of any commercial or financial relationships that could be construed as a potential conflict of interest.
Publisher’s note
All claims expressed in this article are solely those of the authors and do not necessarily represent those of their affiliated organizations, or those of the publisher, the editors and the reviewers. Any product that may be evaluated in this article, or claim that may be made by its manufacturer, is not guaranteed or endorsed by the publisher.
Supplementary material
The Supplementary Material for this article can be found online at: https://www.frontiersin.org/articles/10.3389/fevo.2022.958032/full#supplementary-material
References
Abou Neel, E. A., Aljabo, A., Strange, A., Ibrahim, S., Coathup, M., Young, A. M., et al. (2016). Demineralization-remineralization dynamics in teeth and bone. Int. J. Nanomed. 11, 4743–4763. doi: 10.2147/IJN.S107624
Adame, F., Jardine, T., Fry, B., Valdez, D., Lindner, G., Nadji, J., et al. (2018). Estuarine crocodiles in a tropical coastal floodplain obtain nutrition from terrestrial prey. PLoS One 13:e0197159. doi: 10.1371/journal.pone.0197159
Ambrose, S. H. (1986). Stable carbon and nitrogen isotope analysis of human and animal diet in Africa. J. Hum. Evol. 15, 707–731. doi: 10.1016/S0047-2484(86)80006-9
Ambrose, S. H. (1991). Effects of diet, climate and physiology on nitrogen isotope abundances in terrestrial foodwebs. J. Archaeol. Sci. 18, 293–317. doi: 10.1016/0305-4403(91)90067-Y
Ambrose, S. H., and DeNiro, M. J. (1986). The isotopic ecology of East-African mammals. Oecologia 69, 395–406. doi: 10.1007/bf00377062
Ambrose, S. H., and Norr, L. (1993). “Experimental Evidence for the Relationship of the Carbon Isotope Ratios of Whole Diet and Dietary Protein to Those of Bone Collagen and Carbonate,” in Prehistoric Human Bone: Archaeology at the Molecular Level, eds J. B. Lambert and G. Grupe (Berlin: Springer Berlin Heidelberg), 1–37.
Amiot, R., Lécuyer, C., Escarguel, G., Billon-Bruyat, J. P., Buffetaut, E., Langlois, C., et al. (2007). Oxygen isotope fractionation between crocodilian phosphate and water. Palaeogeogr. Palaeoclimatol. Palaeoecol. 243, 412–420. doi: 10.1016/j.palaeo.2006.08.013
Amundson, R., Austin, A. T., Schuur, E. A. G., Yoo, K., Matzek, V., Kendall, C., et al. (2003). Global patterns of the isotopic composition of soil and plant nitrogen. Glob. Biogeochem. Cycles 17:31. doi: 10.1029/2002gb001903
Arvidsson, K., Stenberg, L., Chirindja, F., Dahlin, T., Owen, R., and Steinbruch, F. (2011). A hydrogeological study of the Nhandugue River, Mozambique – A major groundwater recharge zone. Phys. Chem. Earth 36, 789–797. doi: 10.1016/j.pce.2011.07.036
Atkins, J. L., Long, R. A., Pansu, J., Daskin, J. H., Potter, A. B., Stalmans, M. E., et al. (2019). Cascading impacts of large-carnivore extirpation in an African ecosystem. Science 364, 173–177. doi: 10.1126/science.aau3561
Auderset, A., Moretti, S., Taphorn, B., Ebner, P. R., Kast, E., Wang, X. T., et al. (2022). Enhanced ocean oxygenation during Cenozoic warm periods. Nature 609, 77–82. doi: 10.1038/s41586-022-05017-0
Ayliffe, L. K., Lister, A. M., and Chivas, A. R. (1992). The preservation of glacial-interglacial climatic signatures in the oxygen isotopes of elephant skeletal phosphate. Palaeogeogr. Palaeoclimatol. Palaeoecol. 99, 179–191.
Balasse, M., Bocherens, H., and Mariotti, A. (1999). Intra-bone variability of collagen and apatite isotopic composition used as evidence of a change of diet. J. Archaeol. Sci. 26, 593–598.
Becker, J. A., Hutchinson, M., Potter, A., Park, S., Guyton, J., Abernathy, K., et al. (2021). Ecological and behavioral mechanisms of density-dependent habitat expansion in a recovering African ungulate population. Ecol. Monogr. 91:e01476. doi: 10.1002/ECM.1476
Behrensmeyer, A. K. (1978). Taphonomic and Ecologic Information from Bone Weathering. Paleobiology 4, 150–162.
Blumenthal, S. A., Levin, N. E., Brown, F. H., Brugal, J. P., Chritz, K. L., Harris, J. M., et al. (2017). Aridity and hominin environments. Proc. Natl. Acad. Sci. U. S. A. 114, 7331–7336. doi: 10.1073/pnas.1700597114
Bobe, R., Aldeias, V., Alemseged, Z., Archer, W., Aumaître, G., Bamford, M. K., et al. (2021). The first Miocene fossils from coastal woodlands in the southern East African Rift. Biorxiv [Preprint]. doi: 10.1101/2021.12.16.472914
Bobe, R., Martínez, F. I., and Carvalho, S. (2020). Primate adaptations and evolution in the Southern African Rift Valley. Evol. Anthropol. 29, 94–101. doi: 10.1002/evan.21826
Bocherens, H. (2009). “Neanderthal Dietary Habits: Review of the Isotopic Evidence,” in The Evolution of Hominin Diets: Integrating Approaches to the Study of Palaeolithic Subsistence, eds J. J. Hublin and M. P. Richards (Dordrecht: Springer Netherlands), 241–250.
Bocherens, H., and Drucker, D. (2003). Trophic level isotopic enrichment of carbon and nitrogen in bone collagen: Case studies from recent and ancient terrestrial ecosystems. Int. J. Osteoarchaeol. 13, 46–53.
Bocherens, H., Koch, P. L., Mariotti, A., Geraads, D., and Jaeger, J. J. (1996). Isotopic biogeochemistry (13C, 18O) of mammalian enamel from African Pleistocene hominid sites. Palaios 11, 306–318. doi: 10.2307/3515241
Böhme, B. (2005). Geo ecology of the Lake Urema / Central Mozambique. Freiberg Online Geosci. 14:2005. doi: 10.23689/fidgeo-881
Bothma, J. (2005). Water-use by southern Kalahari leopards. S. Afr. J. Wildl. Res. 35, 131–137. doi: 10.10520/EJC117220
Bouley, P., Paulo, A., Angela, M., Du Plessis, C., and Marneweck, D. G. (2021). The successful reintroduction of African wild dogs (Lycaon pictus) to Gorongosa National Park, Mozambique. PLoS One 16:e0249860. doi: 10.1371/journal.pone.0249860
Bouley, P., Poulos, M., Branco, R., and Carter, N. H. (2018). Post-war recovery of the African lion in response to large-scale ecosystem restoration. Biol. Conserv. 227, 233–242. doi: 10.1016/j.biocon.2018.08.024
Bourgon, N., Jaouen, K., Bacon, A. M., Dufour, E., McCormack, J., Tran, N. H., et al. (2021). Trophic ecology of a Late Pleistocene early modern human from tropical Southeast Asia inferred from zinc isotopes. J. Hum. Evol. 161:103075. doi: 10.1016/j.jhevol.2021.103075
Bourgon, N., Jaouen, K., Bacon, A. M., Jochum, K. P., Dufour, E., Duringer, P., et al. (2020). Zinc isotopes in Late Pleistocene fossil teeth from a Southeast Asian cave setting preserve paleodietary information. Proc. Natl. Acad. Sci. U. S. A. 117, 4675–4681. doi: 10.1073/pnas.1911744117
Branco, P., Merkle, J., Pringle, R., King, L., Tindall, T., Stalmans, M., et al. (2019a). An experimental test of community-based strategies for mitigating human-wildlife conflict around protected areas. Conserv. Lett. 13:e12679. doi: 10.1111/conl.12679
Branco, P., Merkle, J., Pringle, R., Pansu, J., Potter, A., Reynolds, A., et al. (2019b). Determinants of elephant foraging behaviour in a coupled human-natural system: Is brown the new green? J. Anim. Ecol. 88, 780–792. doi: 10.1111/1365-2656.12971
Bryant, D. J., and Froelich, P. N. (1995). A model of oxygen isotope fractionation in body water of large mammals. Geochim. Cosmochim. Acta 59, 4523–4537. doi: 10.1016/0016-7037(95)00250-4
Bryant, D. J., Koch, P. L., Froelich, P. N., Showers, W. J., and Genna, B. J. (1996). Oxygen isotope partitioning between phosphate and carbonate in mammalian apatite. Geochim. Cosmochim. Acta 60, 5145–5148. doi: 10.1016/S0016-7037(96)00308-0
Cain, J. III, Owen-Smith, N., and Macandza, V. (2012). The costs of drinking: Comparative water dependency of sable antelope and zebra. J. Zool. 286, 58–67. doi: 10.1111/j.1469-7998.2011.00848.x
Cantalapiedra-Hijar, G., Ortigues-Marty, I., Sepchat, B., Agabriel, J., Huneau, J. F., and Fouillet, H. (2015). Diet–animal fractionation of nitrogen stable isotopes reflects the efficiency of nitrogen assimilation in ruminants. Br. J. Nutr. 113, 1158–1169. doi: 10.1017/S0007114514004449
Carter, M. L., and Bradbury, M. W. (2016). Oxygen isotope ratios in primate bone carbonate reflect amount of leaves and vertical stratification in the diet. Am. J. Primatol. 78, 1086–1097. doi: 10.1002/ajp.22432
Caut, S., Angulo, E., and Courchamp, F. (2009). Variation in discrimination factors (Δ15N and Δ13C): The effect of diet isotopic values and applications for diet reconstruction. J. Appl. Ecol. 46, 443–453. doi: 10.1111/j.1365-2664.2009.01620.x
Cerling, E., Harris, J. M., and Passey, B. H. (2003). Diets of East African bovidae based on stable isotope analysis. J. Mammal. 84, 456–470. doi: 10.2307/1383890
Cerling, T., Andanje, S., Blumenthal, S., Brown, F., Chritz, K., Harris, J., et al. (2015). Dietary changes of large herbivores in the Turkana Basin, Kenya from 4 to 1 Ma. Proc. Natl. Acad. Sci. U. S. A. 112:201513075. doi: 10.1073/pnas.1513075112
Cerling, T. E., Bernasconi, S. M., Hofstetter, L. S., Jaggi, M., Wyss, F., Rudolf von Rohr, C., et al. (2021). CH4/CO2 ratios and carbon isotope enrichment between diet and breath in herbivorous mammals. Front. Ecol. Evol. 9:638568. doi: 10.3389/fevo.2021.638568
Cerling, T. E., and Harris, J. M. (1999). Carbon isotope fractionation between diet and bioapatite in ungulate mammals and implications for ecological and paleoecological studies. Oecologia 120, 347–363. doi: 10.1007/s004420050868
Cerling, T. E., Harris, J. M., Ambrose, S. H., Leakey, M. G., and Solounias, N. (1997). Dietary and environmental reconstruction with stable isotope analyses of herbivore tooth enamel from the Miocene locality of Fort Ternan, Kenya. J. Hum. Evol. 33, 635–650. doi: 10.1006/jhev.1997.0151
Cerling, T. E., Wynn, J. G., Andanje, S. A., Bird, M. I., Korir, D. K., Levin, N. E., et al. (2011). Woody cover and hominin environments in the past 6 million years. Nature 476, 51–56. doi: 10.1038/nature10306
Chinique de Armas, Y., Mavridou, A. M., Garcell Domínguez, J., Hanson, K., and Laffoon, J. (2022). Tracking breastfeeding and weaning practices in ancient populations by combining carbon, nitrogen and oxygen stable isotopes from multiple non-adult tissues. PLoS One 17:e0262435. doi: 10.1371/journal.pone.0262435
Codron, D., Codron, J., Lee-Thorp, J. A., Sponheimer, M., de Ruiter, D., and Brink, J. S. (2007). Stable isotope characterization of mammalian predator–prey relationships in a South African savanna. Eur. J. Wildl. Res. 53, 161–170. doi: 10.1007/s10344-006-0075-x
Codron, D., Codron, J., Sponheimer, M., and Clauss, M. (2016). Within-population isotopic niche variability in savanna mammals: Disparity between carnivores and herbivores. Front. Ecol. Evol. 4:15. doi: 10.3389/fevo.2016.00015
Codron, D., Lee-Thorp, J. A., Sponheimer, M., de Ruiter, D., and Codron, J. (2006). Inter- and intrahabitat dietary variability of chacma baboons (Papio ursinus) in South African savannas based on fecal δ13C, δ15N, and %N. Am. J. Phys. Anthropol. 129, 204–214. doi: 10.1002/ajpa.20253
Codron, D., Radloff, F. G., Codron, J., Kerley, G. I., and Tambling, C. J. (2018). Meso-carnivore niche expansion in response to an apex predator’s reintroduction–a stable isotope approach. Afr. J. Wildl. Res. 48, 1–16. doi: 10.3957/056.048.013004
Codron, J., Codron, D., Lee-Thorp, J. A., Sponheimer, M., Bond, W. J., de Ruiter, D., et al. (2005). Taxonomic, anatomical, and spatio-temporal variations in the stable carbon and nitrogen isotopic compositions of plants from an African savanna. J. Archaeol. Sci. 32, 1757–1772. doi: 10.1016/j.jas.2005.06.006
Codron, J., Codron, D., Sponheimer, M., Kirkman, K., Duffy, K. J., Raubenheimer, E. J., et al. (2012). Stable isotope series from elephant ivory reveal lifetime histories of a true dietary generalist. Proc. R. Soc. B Biol. Sci. 279, 2433–2441. doi: 10.1098/rspb.2011.2472
Coplen, T. B. (1988). Normalization of oxygen and hydrogen isotope data. Chem. Geol. 72, 293–297. doi: 10.1016/0168-9622(88)90042-5
Correia, M., Timóteo, S., Rodríguez-Echeverría, S., Mazars-Simon, A., and Heleno, R. (2017). Refaunation and the reinstatement of the seed-dispersal function in Gorongosa National Park. Conserv. Biol. 31, 76–85. doi: 10.1111/cobi.12782
Crowley, B. (2012). Stable Isotope Techniques and Applications for Primatologists. Int. J. Primatol. 33, 673–701. doi: 10.1007/s10764-012-9582-7
Crowley, B. E., Melin, A. D., Yeakel, J. D., and Dominy, N. J. (2015). Do oxygen isotope values in collagen reflect the ecology and physiology of neotropical mammals? Front. Ecol. Evol. 3:127. doi: 10.3389/fevo.2015.00127
Cumming, D., Mackie, C., Magane, S., and Taylor, R. (1994). Aerial census of large herbivores in the Gorongosa National Park and the Marromeu Area of the Zambezi Delta in Mozambique: June 1994. Harare, ZW: IUCN ROSA.
Cybulski, J. D., Skinner, C., Wan, Z., Wong, C. K. M., Toonen, R. J., Gaither, M. R., et al. (2022). Improving stable isotope assessments of inter- and intra-species variation in coral reef fish trophic strategies. Ecol. Evol. 12:e9221. doi: 10.1002/ece3.9221
Dag, O., Dolgun, A., and Konar, N. M. (2018). onewaytests: An R Package for One-Way Tests in Independent Groups Designs. R J. 10, 175–199.
Dailey-Chwalibóg, T., Huneau, J. F., Mathé, V., Kolsteren, P., Mariotti, F., Mostak, M. R., et al. (2020). Weaning and stunting affect nitrogen and carbon stable isotope natural abundances in the hair of young children. Sci. Rep. 10:2522. doi: 10.1038/s41598-020-59402-8
Daskin, J. H., Stalmans, M., and Pringle, R. M. (2016). Ecological legacies of civil war: 35-year increase in savanna tree cover following wholesale large-mammal declines. J. Ecol. 104, 79–89. doi: 10.1111/1365-2745.12483
Dauphin, Y., and Williams, C. (2008). Chemical composition of enamel and dentine in modern reptile teeth. Mineral. Mag. 72, 247–250. doi: 10.1180/minmag.2008.072.1.247
DeNiro, M. J., and Epstein, S. (1981). Influence of diet on the distribution of nitrogen isotopes in animals. Geochim. Cosmochim. Acta 45, 341–351. doi: 10.1016/0016-7037(81)90244-1
Domingo, L., Tomassini, R. L., Montalvo, C. I., Sanz-Pérez, D., and Alberdi, M. T. (2020). The Great American Biotic Interchange revisited: A new perspective from the stable isotope record of Argentine Pampas fossil mammals. Sci. Rep. 10:1608. doi: 10.1038/s41598-020-58575-6
Dunham, K. M. (2004). Aerial Survey of Large Herbivores in Gorongosa National Park, Mozambique: 2004. Cambridge MA: The Gregory C. Carr Foundation.
Dutton, P., and Carvalho, F. (2002). Final Report for the GERFFA Project on the Status of Fauna in the Sofala Province 1990–2001, with Reference to Previous Data. Unpublished consultancy report. Maputo, Mozambique.
Easter, T., Bouley, P., and Carter, N. (2019). Opportunities for biodiversity conservation outside of Gorongosa National Park, Mozambique: A multispecies approach. Biol. Conserv. 232, 217–227. doi: 10.1016/j.biocon.2019.02.007
Evans, R. D. (2001). Physiological mechanisms influencing plant nitrogen isotope composition. Trends Plant Sci. 6, 121–126. doi: 10.1016/S1360-1385(01)01889-1
Fick, S. E., and Hijmans, R. J. (2017). WorldClim 2: New 1-km spatial resolution climate surfaces for global land areas. Int. J. Climatol. 37, 4302–4315. doi: 10.1002/joc.5086
Fox-Dobbs, K., Bump, J. K., Peterson, R. O., Fox, D. L., and Koch, P. (2007). Carnivore-specific stable isotope variables and variation in the foraging ecology of modern and ancient wolf populations: Case studies from Isle Royale, Minnesota, and La Brea. Can. J. Zool. 85, 458–471. doi: 10.1139/Z07-018
Fricke, H. C., and O’Neil, J. R. (1996). Inter- and intra-tooth variation in the oxygen isotope composition of mammalian tooth enamel phosphate: Implications for palaeoclimatological and palaeobiological research. Palaeogeogr. Palaeoclimatol. Palaeoecol. 126, 91–99. doi: 10.1016/S0031-0182(96)00072-7
Fuller, B. T., Fuller, J. L., Harris, D. A., and Hedges, R. E. M. (2006). Detection of breastfeeding and weaning in modern human infants with carbon and nitrogen stable isotope ratios. Am. J. Phys. Anthropol. 129, 279–293. doi: 10.1002/ajpa.20249
Fulton, J., Arthur, M., Thomas, R., and Freeman, K. (2018). Pigment carbon and nitrogen isotopic signatures in euxinic basins. Geobiology 16, 429–445. doi: 10.1111/gbi.12285
Gaynor, K., Branco, P., Long, R., Gonçalves, D., Granli, P., and Poole, J. (2018). Effects of human settlement and roads on diel activity patterns of elephants (Loxodonta africana). Afr. J. Ecol. 56, 872–881. doi: 10.1111/aje.12552
Gaynor, K. M., Daskin, J. H., Rich, L. N., and Brashares, J. S. (2021). Postwar wildlife recovery in an African savanna: Evaluating patterns and drivers of species occupancy and richness. Anim. Conserv. 24, 510–511. doi: 10.1111/acv.12661
Ghiglieri, M., Butynski, T., Struhsaker, T., Leland, L., Wallis, S., and Waser, P. (2008). Bush pig (Potamochoerus porcus) polychromatism and ecology in Kibale Forest, Uganda. Afr. J. Ecol. 20, 231–236. doi: 10.1111/j.1365-2028.1982.tb00298.x
Gil-Bona, A., and Bidlack, F. B. (2020). Tooth enamel and its dynamic protein matrix. Int. J. Mol. Sci. 21:4458. doi: 10.3390/ijms21124458
Goldberg, M., Kulkarni, A. B., Young, M., and Boskey, A. (2011). Dentin: Structure, composition and mineralization. Front. Biosci. 3:711–735. doi: 10.2741/e281
Guyton, J. A., Pansu, J., Hutchinson, M. C., Kartzinel, T. R., Potter, A. B., Coverdale, T. C., et al. (2020). Trophic rewilding revives biotic resistance to shrub invasion. Nat. Ecol. Evol. 4, 712–724. doi: 10.1038/s41559-019-1068-y
Habermann, J. M., Alberti, M., Aldeias, V., Alemseged, Z., Archer, W., Bamford, M., et al. (2019). Gorongosa by the sea: First Miocene fossil sites from the Urema Rift, central Mozambique, and their coastal paleoenvironmental and paleoecological contexts. Palaeogeogr. Palaeoclimatol. Palaeoecol. 514, 723–738. doi: 10.1016/j.palaeo.2018.09.032
Hamilton, W. J. III (1986). Namib desert chacma baboon (Papio ursinus) use of food and water resources during a food shortage. Madoqua 1986, 397–407. doi: 10.10520/AJA10115498_477
Hammer, Ø., Harper, D. A., and Ryan, P. D. (2001). PAST: Paleontological statistics software package for education and data analysis. Palaeontol. Electron. 4, 1–9.
Hammond, P., Lewis-Bevan, L., Biro, D., and Carvalho, S. (2022). Risk perception and terrestriality in primates: A quasi-experiment through habituation of chacma baboons (Papio ursinus) in Gorongosa National Park, Mozambique. Am. J. Biol. Anthropol. 179, 48–59. doi: 10.1002/ajpa.24567
Hartman, G. (2010). Are elevated δ15N values in herbivores in hot and arid environments caused by diet or animal physiology? Funct. Ecol. 25, 122–131. doi: 10.1111/j.1365-2435.2010.01782.x
Hayward, M. W., and Hayward, M. D. (2012). Waterhole use by African Fauna. S. Afr. J. Wildl. Res. 42, 117–127.
Hempson, G. P., Archibald, S., and Bond, W. J. (2015). A continent-wide assessment of the form and intensity of large mammal herbivory in Africa. Science 350, 1056–1061. doi: 10.1126/science.aac7978
Hopley, P. J., Cerling, T. E., Crété, L., Werdelin, L., Mwebi, O., Manthi, F. K., et al. (2022). Stable isotope analysis of carnivores from the Turkana Basin, Kenya: Evidence for temporally-mixed fossil assemblages. Quat. Int. doi: 10.1016/j.quaint.2022.04.004
Hoppe, K. A. (2006). Correlation between the oxygen isotope ratio of North American bison teeth and local waters: Implication for paleoclimatic reconstructions. Earth Planet. Sci. Lett. 244, 408–417. doi: 10.1016/j.epsl.2006.01.062
Iacumin, P., Bocherens, H., Mariotti, A., and Longinelli, A. (1996). Oxygen isotope analyses of co-existing carbonate and phosphate in biogenic apatite: A way to monitor diagenetic alteration of bone phosphate? Earth Planet. Sci. Lett. 142, 1–6. doi: 10.1016/0012-821X(96)00093-3
IAEA/WISER (2020a). Global Network of Isotopes in Precipitation. Available online at: https://www.iaea.org/services/networks/gnip (accessed on Mar 11, 2022).
IAEA/WISER (2020b). Global Network of Isotopes in Rivers. Available online at: https://www.iaea.org/services/networks/gnir (accessed on Mar 11, 2022).
Jackson, A., Inger, R., Parnell, A., and Bearhop, S. (2011). Comparing isotopic niche width among and within communities: SIBER – Stable Isotope Bayesian Ellipses in R. J. Anim. Ecol. 80, 595–602. doi: 10.1111/j.1365-2656.2011.01806.x
Jaouen, K., Villalba-Mouco, V., Smith, G. M., Trost, M., Leichliter, J., Lüdecke, T., et al. (2022). A Neandertal dietary conundrum: New insights provided by tooth enamel Zn isotopes from Gabasa, Spain. Proc. Natl. Acad. Sci. U. S. A. 119:e2109315119. doi: 10.1073/pnas.2109315119
Kassambra, A. (2021). rstatix: Pipe-friendly framework for basic statistical tests. R package version 0.7.0. Available online at: https://CRAN.R-project.org/package=rstatix (accessed September 29, 2022).
Kast, E. R., Griffiths, M. L., Kim, S. L., Rao, Z. C., Shimada, K., Becker, M. A., et al. (2022). Cenozoic megatooth sharks occupied extremely high trophic positions. Sci. Adv. 8:eabl6529. doi: 10.1126/sciadv.abl6529
Kast, E. R., Stolper, D. A., Auderset, A., Higgins, J. A., Ren, H., Wang, X. T., et al. (2019). Nitrogen isotope evidence for expanded ocean suboxia in the early Cenozoic. Science 364, 386–389. doi: 10.1126/science.aau5784
Kendall, C., and McDonnell, J. J. (1998). Isotope tracers in catchment hydrology. Amsterdam: Elsevier.
Kingston, J. D., and Harrison, T. (2007). Isotopic dietary reconstructions of Pliocene herbivores at Laetoli: Implications for early hominin paleoecology. Palaeogeogr. Palaeoclimatol. Palaeoecol. 243, 272–306. doi: 10.1016/j.palaeo.2006.08.002
Knapp, A., Sigman, D., and Lipschultz, F. (2005). N isotopic composition of dissolved organic nitrogen and nitrate at the Bermuda Atlantic time-series study site. Glob. Biogeochem. Cycles 19. doi: 10.1029/2004GB002320
Koch, P. L. (2007). “Isotopic Study of the Biology of Modern and Fossil Vertebrates,” in Stable Isotopes in Ecology and Environmental Science, eds R. Michener and K. Lajtha (Hoboken, NJ: Wiley), 99–154.
Koch, P. L., Tuross, N., and Fogel, M. L. (1997). The effects of sample treatment and diagenesis on the isotopic integrity of carbonate in biogenic hydroxylapatite. J. Archaeol. Sci. 24, 417–429. doi: 10.1006/jasc.1996.0126
Kohn, M. J. (1996). Predicting animal δ18O: Accounting for diet and physiological adaptation. Geochim. Cosmochim. Acta 60, 4811–4829. doi: 10.1016/S0016-7037(96)00240-2
Kohn, M. J. (2010). Carbon isotope compositions of terrestrial C3 plants as indicators of (paleo)ecology and (paleo)climate. Proc. Natl. Acad. Sci. U. S. A. 107:19691. doi: 10.1073/pnas.1004933107
Kohn, M. J., and Cerling, T. E. (2002). “Stable Isotope Compositions of Biological Apatite,” in Phosphates. Geochemical, Geobiological, and Materials Importance, eds M. J. Kohn, J. Rakovan, and J. M. Hughes (Washington, DC: Mineralogical Society of America), 455–488.
Kohn, M. J., Schoeninger, M. J., and Valley, J. W. (1996). Herbivore tooth oxygen isotope compositions: Effects of diet and physiology. Geochim. Cosmochim. Acta 60, 3889–3896. doi: 10.1016/0016-7037(96)00248-7
Krajcarz, M. T., Krajcarz, M., and Bocherens, H. (2018). Collagen-to-collagen prey-predator isotopic enrichment (Δ13C, Δ15N) in terrestrial mammals - a case study of a subfossil red fox den. Palaeogeogr. Palaeoclimatol. Palaeoecol. 490, 563–570. doi: 10.1016/j.palaeo.2017.11.044
Lacruz, R. S., Habelitz, S., Wright, J. T., and Paine, M. L. (2017). Dental enamel formation and implications for oral health and disease. Physiol. Rev. 97, 939–993. doi: 10.1152/physrev.00030.2016
Layman, C., and Allgeier, J. (2012). Characterizing trophic ecology of generalist consumers: A case study of the invasive lionfish in The Bahamas. Mar. Ecol. Progress Ser. 448, 131–141. doi: 10.3354/meps09511
Layman, C. A., Arrington, D. A., Montaña, C. G., and Post, D. M. (2007). Can stable isotope ratios provide for community-wide measures of trophic structure? Ecology 88, 42–48. doi: 10.1890/0012-9658200788[42:csirpf]2.0.co;2
Lee-Thorp, J., and Van der Merwe, N. J. (1987). Carbon isotope analysis of fossil bone apatite. S. Afr. J. Sci. 83, 712–715.
Leichliter, J. N., Lüdecke, T., Foreman, A., Bourgon, N., Vonhof, H., Souksavatdy, V., et al. (2022). Nitrogen isotopic composition of tooth enamel organic matter records trophic position in modern and fossil ecosystems. Res. Square [Preprint]. doi: 10.21203/rs.3.rs-1942250/v1
Leichliter, J. N., Lüdecke, T., Foreman, A. D., Duprey, N. N., Winkler, D. E., Kast, E. R., et al. (2021). Nitrogen isotopes in tooth enamel record diet and trophic level enrichment: Results from a controlled feeding experiment. Chem. Geol. 563:120047. doi: 10.1016/j.chemgeo.2020.120047
Levin, N. E., Cerling, T. E., Passey, B. H., Harris, J. M., and Ehleringer, J. R. (2006). A stable isotope aridity index for terrestrial environments. Proc. Natl. Acad. Sci. U. S. A. 103, 11201–11205. doi: 10.1073/pnas.0604719103
Lüdecke, T., Kullmer, O., Wacker, U., Sandrock, O., Fiebig, J., Schrenk, F., et al. (2018). Dietary versatility of Early Pleistocene hominins. Proc. Natl. Acad. Sci. U. S. A. 115, 13330–13335. doi: 10.1073/pnas.1809439115
Lüdecke, T., Mulch, A., Kullmer, O., Sandrock, O., Thiemeyer, H., Fiebig, J., et al. (2016). Stable isotope dietary reconstructions of herbivore enamel reveal heterogeneous wooded savanna ecosystems in the Plio-Pleistocene Malawi Rift. Palaeogeogr. Palaeoclimatol. Palaeoecol. 459, 170–181. doi: 10.1016/j.palaeo.2016.07.010
Lueders-Dumont, J. A., Wang, X. T., Jensen, O. P., Sigman, D. M., and Ward, B. B. (2018). Nitrogen isotopic analysis of carbonate-bound organic matter in modern and fossil fish otoliths. Geochim. Cosmochim. Acta 224, 200–222. doi: 10.1016/j.gca.2018.01.001
Lüttge, U. (2004). Ecophysiology of Crassulacean Acid Metabolism (CAM). Ann. Bot. 93, 629–652. doi: 10.1093/aob/mch087
Ma, Y., Weldeab, S., Schneider, R. R., Andersen, N., Garbe-Schönberg, D., and Friedrich, T. (2021). Strong Southern African Monsoon and weak Mozambique Channel throughflow during Heinrich events: Implication for Agulhas leakage. Earth Planet. Sci. Lett. 574:117148. doi: 10.1016/j.epsl.2021.117148
Martin, J. E., Hassler, A., Montagnac, G., Therrien, F., and Balter, V. (2022). The stability of dinosaur communities before the K-Pg boundary: A perspective from southern Alberta using calcium isotopes as a dietary proxy. GSA Bull. 134, 2548–2560. doi: 10.1130/b36222.1
Martin, J. E., Tacail, T., Adnet, S., Girard, C., and Balter, V. (2015). Calcium isotopes reveal the trophic position of extant and fossil elasmobranchs. Chem. Geol. 415, 118–125. doi: 10.1016/j.chemgeo.2015.09.011
Martin, J. E., Tacail, T., Braga, J., Cerling, T. E., and Balter, V. (2020). Calcium isotopic ecology of Turkana Basin hominins. Nat. Commun. 11:3587. doi: 10.1038/s41467-020-17427-7
Martinez, F. I., Capelli, C., Ferreira da Silva, M. J., Aldeias, V., Alemseged, Z., Archer, W., et al. (2019). A missing piece of the Papio puzzle: Gorongosa baboon phenostructure and intrageneric relationships. J. Hum. Evol. 130, 1–20. doi: 10.1016/j.jhevol.2019.01.007
Martinez-Garcia, A., Jung, J., Ai, X. E., Sigman, D. M., Auderset, A., Duprey, N. N., et al. (2022). Laboratory Assessment of the Impact of Chemical Oxidation, Mineral Dissolution, and Heating on the Nitrogen Isotopic Composition of Fossil-bound Organic Matter. Geochem. Geophys. Geosyst. 23:e2022GC010396. doi: 10.1029/2022GC010396
Martinez-Garcia, A., Sigman, D. M., Ren, H., Anderson, R. F., Straub, M., Hoderll, D. A., et al. (2014). Iron Fertilization of the Subantartic Ocean During the Last Ice Age. Science 343, 1347–1350.
McCormack, J., Szpak, P., Bourgon, N., Richards, M., Hyland, C., Méjean, P., et al. (2021). Zinc isotopes from archaeological bones provide reliable trophic level information for marine mammals. Commun. Biol. 4:683. doi: 10.1038/s42003-021-02212-z
Merceron, G., Berlioz, E., Vonhof, H., Green, D., Garel, M., and Tütken, T. (2021). Tooth tales told by dental diet proxies: An alpine community of sympatric ruminants as a model to decipher the ecology of fossil fauna. Palaeogeogr. Palaeoclimatol. Palaeoecol. 562:110077. doi: 10.1016/j.palaeo.2020.110077
Monro, R. H. (1980). Observations on the Feeding Ecology of Impala. S. Afr. J. Zool. 15, 107–110. doi: 10.1080/02541858.1980.11447695
Moritz, G. L., Fourie, N., Yeakel, J. D., Phillips-Conroy, J. E., Jolly, C. J., Koch, P. L., et al. (2012). Baboons, Water, and the Ecology of Oxygen Stable Isotopes in an Arid Hybrid Zone. Physiol. Biochem. Zool. 85, 421–430. doi: 10.1086/667533
Muschinski, J., Biro, D., Lewis-Bevan, L., and Carvalho, S. (2019). Could it be culture? An inter-troop comparison of baboon behaviour in Gorongosa National Park, Mozambique. Neuroscience 91, 350–351.
Nelson, S. (2013). Chimpanzee fauna isotopes provide new interpretations of fossil ape and hominin ecologies. Proc. Biol. Sci. R. Soc. 280:20132324. doi: 10.1098/rspb.2013.2324
Newsome, S., Rio, C., Bearhop, S., and Phillips, D. (2007). A niche for isotopic ecology. Front. Ecol. Environ. 5:429–436. doi: 10.1890/060150.1
Nicholson, M. J. (1985). The water requirements of livestock in Africa. Outl. Agric. 14, 156–164. doi: 10.1177/003072708501400401
Noirard, C., Le Berre, M., Ramousse, R., and Lena, J. P. (2008). Seasonal variation of thermoregulatory behaviour in the Hippopotamus (Hippopotamus amphibius). J. Ethol. 26, 191–193. doi: 10.1007/s10164-007-0052-1
O’Connell, T. C., Kneale, C. J., Tasevska, N., and Kuhnle, G. G. C. (2012). The diet-body offset in human nitrogen isotopic values: A controlled dietary study. Am. J. Phys. Anthropol. 149, 426–434. doi: 10.1002/ajpa.22140
Pansu, J., Guyton, J. A., Potter, A. B., Atkins, J. L., Daskin, J. H., Wursten, B., et al. (2019). Trophic ecology of large herbivores in a reassembling African ecosystem. J. Ecol. 107, 1355–1376. doi: 10.1111/1365-2745.13113
Passey, B. H., and Cerling, T. E. (2002). Tooth enamel mineralization in ungulates: Implications for recovering a primary isotopic time-series. Geochim. Cosmochim. Acta 66, 3225–3234. doi: 10.1016/S0016-7037(02)00933-X
Pearcy, R. W., and Ehleringer, J. (1984). Comparative ecophysiology of C3 and C4 plants. Plant Cell Environ. 7, 1–13. doi: 10.1111/j.1365-3040.1984.tb01194.x
Pederzani, S., and Britton, K. (2019). Oxygen isotopes in bioarchaeology: Principles and applications, challenges and opportunities. Earth Sci. Rev. 188, 77–107. doi: 10.1016/j.earscirev.2018.11.005
Pinzone, M., Damseaux, F., Michel, L. N., and Das, K. (2019). Stable isotope ratios of carbon, nitrogen and sulphur and mercury concentrations as descriptors of trophic ecology and contamination sources of Mediterranean whales. Chemosphere 237:124448. doi: 10.1016/j.chemosphere.2019.124448
Polissar, P. J., Fulton, J. M., Junium, C. K., Turich, C. C., and Freeman, K. H. (2009). Measurement of 13C and 15N Isotopic Composition on Nanomolar Quantities of C and N. Anal. Chem. 81, 755–763. doi: 10.1021/ac801370c
Pringle, R. M. (2017). Upgrading protected areas to conserve wild biodiversity. Nature 546, 91–99. doi: 10.1038/nature22902
R Core Team (2022). R: A language and environment for statistical computing. Vienna: R Foundation for Statistical Computing.
Ren, H., Sigman, D. M., Martínez-García, A., Anderson, R. F., Chen, M. T., and Ravelo, A. C. (2017). Impact of glacial/interglacial sea level change on the ocean nitrogen cycle. Proc. Natl. Acad. Sci. U. S. A. 114, E6759–E6766. doi: 10.1073/pnas.1701315114
Ren, H., Sigman, D. M., Meckler, A. N., Plessen, B., Robinson, R. S., Rosenthal, Y., et al. (2009). Foraminiferal Isotope Evidence of Reduced Nitrogen Fixation in the Ice Age Atlantic Ocean. Science 323, 244–248. doi: 10.1126/science.1165787
Robbins, C. T., Felicetti, L. A., and Sponheimer, M. (2005). The effect of dietary protein quality on nitrogen isotope discrimination in mammals and birds. Oecologia 144, 534–540. doi: 10.1007/s00442-005-0021-8
Roberts, P. (2017). Stable carbon, oxygen, and nitrogen, isotope analysis of plants from a South Asian tropical forest: Implications for primatology. Am. J. Primatol. 79. doi: 10.1002/ajp.22656
Robinson, D. (2001). δ15N as an integrator of the nitrogen cycle. Trends Ecol. Evol. 16, 153–162. doi: 10.1016/S0169-5347(00)02098-X
Robinson, R. S., Brunelle, B. G., and Sigman, D. M. (2004). Revisiting nutrient utilization in the glacial Antarctic: Evidence from a new method for diatom-bound N isotopic analysis. Paleoceanography 19:A3001. doi: 10.1029/2003pa000996
Sage, R. F., and Zhu, X.-G. (2011). Exploiting the engine of C4 photosynthesis. J. Exp. Bot. 62, 2989–3000. doi: 10.1093/jxb/err179
Sakae, T., Suzuki, K., and Kozawa, Y. (1997). “A short review of studies on chemical and physical properties of enamel crystallites,” in Tooth Enamel Microstructure: Proceedings of the enamel microstructure workshop, (Boca Raton, FL: CRC Press).
Santander, C., Molinaro, L., Mutti, G., Martínez, F. I., Mathe, J., Ferreira da Silva, M. J., et al. (2022). Genomic variation in baboons from central Mozambique unveils complex evolutionary relationships with other Papio species. BMC Ecol. Evol. 22:44. doi: 10.1186/s12862-022-01999-7
Schemmel, F., Mikes, T., Rojay, B., and Mulch, A. (2013). The impact of topography on isotopes in precipitation across the Central Anatolian Plateau (Turkey). Am. J. Sci. 313, 61–80. doi: 10.2475/02.2013.01
Schmidt, S., and Stewart, G. R. (2003). δ15N values of tropical savanna and monsoon forest species reflect root specialisations and soil nitrogen status. Oecologia 134, 569–577. doi: 10.1007/s00442-002-1150-y
Schoeniger, M. J., and DeNiro, M. J. (1984). 15N/14N ratios of bone collagen reflect marine and terrestrial components of prehistoric diets. Geochim. Cosmochim. Acta 48, 625–639. doi: 10.1126/science.6344217
Schoeninger, M. J., and DeNiro, M. J. (1984). Nitrogen and carbon isotopic composition of bone collagen from marine and terrestrial animals. Geochim. Cosmochim. Acta 48, 625–639. doi: 10.1016/0016-7037(84)90091-7
Sealy, J. C., van der Merwe, N. J., Thorp, J. A. L., and Lanham, J. L. (1987). Nitrogen isotopic ecology in southern Africa: Implications for environmental and dietary tracing. Geochim. Cosmochim. Acta 51, 2707–2717. doi: 10.1016/0016-7037(87)90151-7
Segalen, L., Lee-Thorp, J. A., and Cerling, T. (2007). Timing of C4 grass expansion across sub-Saharan Africa. J. Hum. Evol. 53, 549–559. doi: 10.1016/j.jhevol.2006.12.010
Sigman, D. M., Casciotti, K. L., Andreani, M., Barford, C., Galanter, M., and Böhlke, J. K. (2001). A bacterial method for the nitrogen isotopic analysis of nitrate in seawater and freshwater. Anal. Chem. 73, 4145–4153.
Smith, B. N., and Epstein, S. (1971). Two categories of c/c ratios for higher plants. Plant Physiol. 47, 380–384. doi: 10.1104/pp.47.3.380
Sponheimer, M., Grant, R., Ruiter, D., Lee-Thorp, J., Codron, D., and Codron, J. (2003). Diets of impala from Kruger National Park: Evidence from stable carbon isotopes. Koedoe 46, 101–106. doi: 10.4102/koedoe.v46i1.43
Sponheimer, M., and Lee-Thorp, J. A. (1999). Oxygen Isotopes in Enamel Carbonate and their Ecological Significance. J. Archaeol. Sci. 26, 723–728. doi: 10.1006/jasc.1998.0388
Stalmans, M. (2012). Monitoring the recovery of wildlife in the Parque Nacional da Gorongosa through aerial surveys. A preliminary analysis. Sofala: Parque Nacional da Gorongosa.
Stalmans, M., and Beilfuss, R. (2008). Landscapes of the gorongosa national park. Available online at: https://www.researchgate.net/publication/314878798_Landscapes_of_the_Gorongosa_National_Park
Stalmans, M., Peel, M., and Massad, T. (2014). Aerial Wildlife Count of the Parque Nacional Da Gorongosa, Mozambique, October 2014. Sofala: Parque Nacional da Gorongosa.
Stalmans, M. E., Massad, T. J., Peel, M. J. S., Tarnita, C. E., and Pringle, R. M. (2019). War-induced collapse and asymmetric recovery of large-mammal populations in Gorongosa National Park, Mozambique. PLoS One 14:e0212864. doi: 10.1371/journal.pone.0212864
Stanton, K., and Carlson, S. (2004). Microscale δ18O and δ13C isotopic analysis of an ontogenetic series of the hadrosaurid dinosaur Edmontosaurus: Implications for physiology and ecology. Palaeogeogr. Palaeoclimatol. Palaeoecol. 206, 257–287. doi: 10.1016/j.palaeo.2004.01.007
Steinbruch, F. (2010). Geology and geomorphology of the Urema Graben with emphasis on the evolution of Lake Urema. J. Afr. Earth Sci. 58, 272–284. doi: 10.1016/j.jafrearsci.2010.03.007
Steinbruch, F., and Merkel, B. (2008). Characterization of a Pleistocene thermal spring in Mozambique. Hydrogeol. J. 16, 1655–1668. doi: 10.1007/s10040-008-0343-9
Steinbruch, F., and Weise, S. (2014). Analysis of Water Stable Isotopes fingerprinting to inform conservation management : Lake Urema Wetland System, Mozambique. Phys. Chem. Earth 72–75, 13–23. doi: 10.1016/j.pce.2014.09.007
Steinhour, W. D., Stokes, M. R., Clark, J. H., Rogers, J. A., Davis, C. L., and Nelson, D. R. (1982). Estimation of the proportion of non-ammonia-nitrogen reaching the lower gut of the ruminant derived from bacterial and protozoal nitrogen. Br. J. Nutr. 48, 417–431. doi: 10.1079/BJN19820124
Straub, M., Sigman, D. M., Ren, H., Martínez-García, A., Meckler, A. N., Hain, M. P., et al. (2013). Changes in North Atlantic nitrogen fixation controlled by ocean circulation. Nature 501, 200–203. doi: 10.1038/nature12397
Sutoh, M., Koyama, T., and Yoneyama, T. (1987). Variations of natural 15N abundances in the tissues and digesta of domestic animals. Radioisotopes 36, 74–77. doi: 10.3769/radioisotopes.36.2_74
Swanson, H. K., Lysy, M., Power, M., Stasko, A. D., Johnson, J. D., and Reist, J. D. (2015). A new probabilistic method for quantifying n-dimensional ecological niches and niche overlap. Ecology 96, 318–324. doi: 10.1890/14-0235.1
Syväranta, J., Lensu, A., Marjomäki, T. J., Oksanen, S., and Jones, R. I. (2013). An Empirical Evaluation of the Utility of Convex Hull and Standard Ellipse Areas for Assessing Population Niche Widths from Stable Isotope Data. PLoS One 8:e56094. doi: 10.1371/journal.pone.0056094
Tejada-Lara, J. V., MacFadden, B. J., Bermudez, L., Rojas, G., Salas-Gismondi, R., and Flynn, J. J. (2018). Body mass predicts isotope enrichment in herbivorous mammals. Proc. R. Soc. B Biol. Sci. 285:20181020. doi: 10.1098/rspb.2018.1020
Teruel, J. D. D., Alcolea, A., Hernández, A., and Ruiz, A. J. O. (2015). Comparison of chemical composition of enamel and dentine in human, bovine, porcine and ovine teeth. Arch. Oral Biol. 60, 768–775. doi: 10.1016/j.archoralbio.2015.01.014
Thackeray, J. F., Henzi, S. P., and Brain, C. (1996). Stable carbon and nitrogen isotope analysis of bone collagen in Papio cynocephalus ursinus: Comparison with ungulates and Homo sapiens from southern and East African environments. S. Afr. J. Sci. 92, 209–213.
Tsutaya, T., and Yoneda, M. (2015). Reconstruction of breastfeeding and weaning practices using stable isotope and trace element analyses: A review. Am. J. Phys. Anthropol. 156, 2–21. doi: 10.1002/ajpa.22657
Turner, T. F., Collyer, M. L., and Krabbenhoft, T. J. (2010). A general hypothesis-testing framework for stable isotope ratios in ecological studies. Ecology 91, 2227–2233. doi: 10.1890/09-1454.1
Ungar, P. S. (2010). Mammal Teeth: Origin, Evolution, and Diversity. Baltimore, MD: Johns Hopkins University Press.
Uno, K. T., Rivals, F., Bibi, F., Pante, M., Njau, J., and de la Torre, I. (2018). Large mammal diets and paleoecology across the Oldowan–Acheulean transition at Olduvai Gorge, Tanzania from stable isotope and tooth wear analyses. J. Hum. Evol. 120, 76–91. doi: 10.1016/j.jhevol.2018.01.002
Venter, J., and Kalule-Sabiti, M. (2016). Diet Composition of the Large Herbivores in Mkambati Nature Reserve, Eastern Cape, South Africa. Afr. J. Wildl. Res. 46, 49–56. doi: 10.3957/056.046.0049
Villamarin, F., Jardine, T. D., Bun, S. E., Marioni, B., and Magnusson, W. E. (2018). Body size is more important than diet in determining stable-isotope estimates of trophic position in crocodilians. Sci. Rep. 8:2020. doi: 10.1038/s41598-018-19918-6
Voigt, C. C., Krofel, M., Menges, V., Wachter, B., and Melzheimer, J. (2018). Sex-specific dietary specialization in a terrestrial apex predator, the leopard, revealed by stable isotope analysis. J. Zool. 306, 1–7. doi: 10.1111/jzo.12566
Vonhof, H., De Graaf, S., Spero, H., Schiebel, R., Verdegaal, S., Metcalfe, B., et al. (2020a). High-precision stable isotope analysis of <5 μg CaCO3 samples by continuous-flow mass spectrometry. Rapid Commun. Mass Spectrom. 34:e8878. doi: 10.1002/rcm.8878
Vonhof, H., Tütken, T., Leichiter, J. N., Lüdecke, T., and Haug, G. H. (2020b). “High-precision stable isotope analysis of structural carbonate in < 100 μ g tooth enamel samples by continuous-flow mass spectrometry,” in The Society of Vertebrate Paleontology 80th Annual Meeting. Available online at: https://vertpaleo.org/wp-content/uploads/2021/03/SVP_2020_Program-Abstracts-Volume-FINAL-for-Publishing-1.27.2021.pdf
Wang, X. T., Prokopenko, M. G., Sigman, D. M., Adkins, J. F., Robinson, L. F., Ren, H., et al. (2014). Isotopic composition of carbonate-bound organic nitrogen in deep-sea scleractinian corals: A new window into past biogeochemical change. Earth Planet. Sci. Lett. 400, 243–250.
Wang, X. T., Sigman, D. M., Cohen, A. L., Sinclair, D. J., Sherrell, R. M., Weigand, M. A., et al. (2015). Isotopic composition of skeleton-bound organic nitrogen in reef-building symbiotic corals: A new method and proxy evaluation at Bermuda. Geochim. Cosmochim. Acta 148, 179–190.
Wang, X. T., Sigman, D. M., Prokopenko, M. G., Adkins, J. F., Robinson, L. F., Hines, S. K., et al. (2017). Deep-sea coral evidence for lower Southern Ocean surface nitrate concentrations during the last ice age. Proc. Natl. Acad. Sci. U. S. A. 114, 3352–3347. doi: 10.1073/pnas.1615718114
Wang, Y., and Cerling, T. E. (1994). A model of fossil tooth and bone diagenesis: Implications for paleodiet reconstruction from stable isotopes. Palaeogeogr. Palaeoclimatol. Palaeoecol. 107, 281–289. doi: 10.1016/0031-0182(94)90100-7
Weigand, M. A., Foriel, J., Barnett, B., Oleynik, S., and Sigman, D. M. (2016). Updates to instrumentation and protocols for isotopic analysis of nitrate by the denitrifier method. Rapid Commun. Mass Spectrom. 30, 1365–1383.
Wilson, D. E., and Mittermeier, R. A. (2009). Handbook of the Mammals of the World. Barcelona: Lynx Wsicions.
Wilson, E. O. (2014). A window on eternity: A biologist’s walk through Gorongosa National Park. New York, NY: Simon and Schuster.
Wolf, J. (1960). “Der diurnale Säurerhythmus,” in Plant Respiration Inclusive Fermentations and Acid Metabolism/Pflanzenatmung Einschliesslich Gärungen und Säurestoffwechsel. Encyclopedia of Plant Physiology / Handbuch der Pflanzenphysiologie, ed. J. Wolf (Berlin: Springer), 1930–2010.
Yang, D., Uno, K. T., Souron, A., McGrath, K., Pubert, É., and Cerling, T. E. (2020). Intra-tooth stable isotope profiles in warthog canines and third molars: Implications for paleoenvironmental reconstructions. Chem. Geol. 554:119799. doi: 10.1016/j.chemgeo.2020.119799
Keywords: diet, ecology, trophic level reconstruction, food webs, vertebrate, savanna
Citation: Lüdecke T, Leichliter JN, Aldeias V, Bamford MK, Biro D, Braun DR, Capelli C, Cybulski JD, Duprey NN, Ferreira da Silva MJ, Foreman AD, Habermann JM, Haug GH, Martínez FI, Mathe J, Mulch A, Sigman DM, Vonhof H, Bobe R, Carvalho S and Martínez-García A (2022) Carbon, nitrogen, and oxygen stable isotopes in modern tooth enamel: A case study from Gorongosa National Park, central Mozambique. Front. Ecol. Evol. 10:958032. doi: 10.3389/fevo.2022.958032
Received: 31 May 2022; Accepted: 27 October 2022;
Published: 24 November 2022.
Edited by:
Rona A. R. McGill, University of Glasgow, United KingdomReviewed by:
Vincent Balter, Centre National de la Recherche Scientifique (CNRS), FranceJoshua Robinson, Boston University, United States
Laszlo Kocsis, Université de Lausanne, Switzerland
Copyright © 2022 Lüdecke, Leichliter, Aldeias, Bamford, Biro, Braun, Capelli, Cybulski, Duprey, Ferreira da Silva, Foreman, Habermann, Haug, Martínez, Mathe, Mulch, Sigman, Vonhof, Bobe, Carvalho and Martínez-García. This is an open-access article distributed under the terms of the Creative Commons Attribution License (CC BY). The use, distribution or reproduction in other forums is permitted, provided the original author(s) and the copyright owner(s) are credited and that the original publication in this journal is cited, in accordance with accepted academic practice. No use, distribution or reproduction is permitted which does not comply with these terms.
*Correspondence: Tina Lüdecke, dGluYS5sdWVkZWNrZUBtcGljLmRl; Jennifer N. Leichliter, amVubmlmZXIubGVpY2hsaXRlckBtcGljLmRl
†These authors have contributed equally to this work and share first authorship
‡These authors share senior authorship