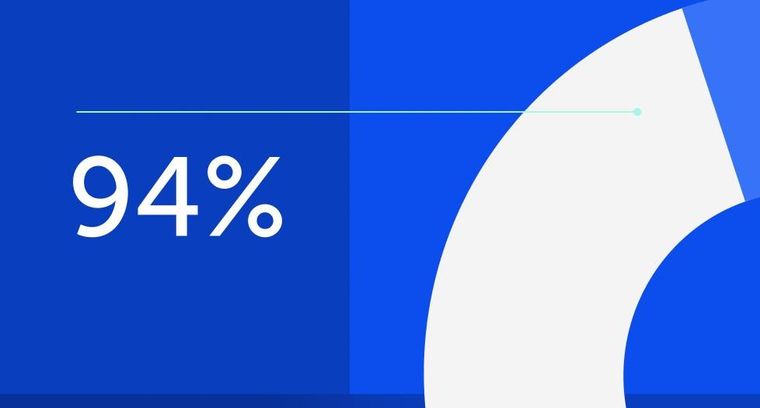
94% of researchers rate our articles as excellent or good
Learn more about the work of our research integrity team to safeguard the quality of each article we publish.
Find out more
ORIGINAL RESEARCH article
Front. Ecol. Evol., 25 August 2022
Sec. Population, Community, and Ecosystem Dynamics
Volume 10 - 2022 | https://doi.org/10.3389/fevo.2022.956819
This article is part of the Research TopicFood Webs and Stable Isotopes, volume IIView all 13 articles
Terrestrial organic matter (t-OM) has been recognized as an important cross-boundary subsidy to aquatic ecosystems. However, recent evidence has shown that t-OM contributes little to promote secondary production in lakes because it is a low-quality food for aquatic consumers. To resolve this conflict, we performed a field experiment using leaf litter as t-OM. In the experiment, we monitored zooplankton biomass in enclosures with and without addition of leaf litter under shaded and unshaded conditions and assessed food web changes with stable isotope analyses. We then examined whether or not leaf litter indeed stimulates lake secondary production and, if it does, which food chain, the detritus-originated food chain (“brown” food chain) or the algae-originated food chain (“green” food chain), contributes more to this increase. Analyses with stable isotopes showed the importance of t-OM in supporting secondary production under ambient lake conditions. However, the addition of the leaf litter increased the zooplankton biomass under unshaded conditions but not under shaded conditions. We found that phosphorus was leached from leaf litter at much faster rate than organic carbon and nitrogen despite its low content in the leaf litter. These results showed that leaf litter stimulated the increase in zooplankton biomass mainly through the green food chain rather than the brown food chain because the leaf litter supplied limiting nutrients (i.e., phosphorus) for primary producers.Our results indicate that the functional stoichiometry of the subsidized organic matter plays a crucial role in determining the relative importance of brown and green food chains in promoting production at higher trophic levels in recipient ecosystems.
Ecosystems are not necessarily isolated from each other, and the food webs therein are often sustained by subsidies of energy and organic matter transferred from adjacent ecosystems (Lindeman, 1942; Polis et al., 1997; Nakano and Murakami, 2001). An example of such subsidies across an ecosystem boundary is terrestrial organic matter (t-OM) that enters into food webs in rivers and lakes (Gasith and Hosler, 1976; Doucett et al., 2007; Cole et al., 2011; Carpenter et al., 2016; Brett et al., 2017). Several studies reported that t-OM is an important energy source for sustaining production at higher trophic levels in lakes (Doucett et al., 2007; Cole et al., 2011; Cole, 2013; Tanentzap et al., 2017). Although the role of t-OM in supporting the production of higher trophic levels in aquatic ecosystems is well-recognized (Carpenter et al., 2016), some studies have questioned the generality and magnitude of this impact on lake ecosystems (Brett et al., 2009; Kelly et al., 2014; Taipale et al., 2014). For example, recent studies showed that the growth rate of zooplankton decreased with an increasing proportion of t-OM relative to phytoplankton in the diets because t-OM is a poor-quality resource for aquatic consumers (Brett et al., 2009; Taipale et al., 2014). In addition, increased dissolved organic carbon (DOC), the dissolved fraction of t-OM, reduces light penetration into waters and thus reduces algal production (Ask et al., 2009; Karlsson et al., 2015). These studies suggest that a positive impact of t-OM on secondary production in lakes may not be a general phenomenon, since the input of t-OM does not necessarily promote zooplankton production in lakes (Carpenter et al., 2016; Brett et al., 2017).
However, some studies have shown that the input of leaf litter, a major component of t-OM, increased zooplankton production (Cottingham and Narayan, 2013; Fey et al., 2015). As a source of carbon and energy, t-OM is consumed by bacteria that in turn are consumed by protozoans such as heterotrophic nanoflagellates (HNF) that are edible food for most zooplankton (Tranvik, 1992; Jansson et al., 2007). This material pathway is often referred to as the brown food chain (Wolkovich et al., 2014) since the chain transfers organic carbon originating from terrigenous organic carbon to higher trophic levels. During this process, consumption of bacteria by HNF may mineralize nitrogen (N) and phosphorus (P) within t-OM to inorganic forms that are available to algae (Bloem et al., 1989). More importantly, leaching rates of P from the leaf litter are often higher than those of C and N (Baldwin, 1999; Schreeg et al., 2013), suggesting that t-OM directly supplies growth-limiting P for phytoplankton production. If this were the case, t-OM would support production at higher trophic levels through the “green food chain” that originates from organic carbon fixed by primary producers. Thus, the input of t-OM can promote zooplankton production through (1) material transfer along with the food chains originating from the organic carbon in the t-OM (brown food chain) and (2) material transfer along with the food chains originating from phytoplankton whose production is promoted by nutrients released from t-OM (green food chain). However, no study has yet examined the relative importance of brown and green food chains in mediating the impact of t-OM on zooplankton production.
Therefore, in this study, we examined (1) whether or not t-OM indeed stimulates production at higher trophic levels, and, if that were the case, (2) which food chain, brown or green, contributed more in increasing zooplankton production. We focused on zooplankton as secondary producers since they prey not only on phytoplankton but also on bacteria and heterotrophic protozoans (Adrian et al., 2001; Yoshida et al., 2001; Wolkovich et al., 2014) and are consumed by carnivores such as fish (McQueen et al., 1989; Carpenter and Kitchell, 1996), which integrates the brown and green food chains in lake ecosystems. We performed a field experiment using enclosures to manipulate the supply of t-OM and the rate of primary production and analyzed food sources of zooplankton with stable isotopes. We used mechanically ground leaf litter as a t-OM source since leaf litter is a quantitatively important terrestrial subsidy to many aquatic ecosystems (Gasith and Hosler, 1976; Rau, 1976; Hanlon, 1981; Wallace et al., 1997). We manipulated primary production by shading the enclosures to reduce the penetration of sunlight.
Fallen leaves and needles used in this study were composed mainly of Paper Birch (Betula papyrifera), Black Cottonwood (Populus balsamifera), and Ponderosa Pine trees (Pinus ponderosa) with some of the other taxa, and collected in a forest at the Flathead Lake Biological Station (FLBS), University of Montana, (Montana, United States), in November 2016. These leaves were dried and stored in plastic bags at room temperature for 7 months until the performance of the experiment. To physically promote the decomposition process of t-OM, 3 kg of these leaves were chopped using a large hand immersion blender and mixed in 100 L of distilled water and then stirred for 3 days in a room held at ∼20°C. After 3 days, the mixed leaf water was filtered sequentially through 3-mm mesh to a final 35-μm mesh and stored overnight at room temperature. Hereafter, this t-OM mixture is denoted as leaf homogenate. Before its use in the experiment, the leaf homogenate was subsampled to determine concentrations of total phosphorus (P), total nitrogen (N), and organic carbon (C).
The field experiment was conducted from 12 June 2017 to 14 August 2017 in Lost Lake located within the Flathead Valley of western Montana, United States (47.6729685 N, −114.066614 W). Lost Lake lies just east of the Mission Mountain Range at an elevation of 938 m. The lake’s maximum depth is 11 m with a surface area of 3 hectares. Four days before initiating the experiment, we installed 12 enclosures with 1-m diameter and 2-m depth that consisted of clear polyethylene tubes with closed bottoms (Figure 1A). We enveloped six out of 12 enclosures with black shade cloth to reduce the penetration of sunlight to 10% of ambient level (D: shaded treatment). The rest of enclosures were left unwrapped (L: unshaded treatment). Each enclosure was fixed to a floating deck anchored at the center of the lake: positions of the enclosures with different treatments at the deck were randomly assigned. Then, the enclosures were filled with 1,000 L of lake water, which was collected from a depth of 1–2 m, and passed through 100-μm plankton net to remove the large zooplankton species. On the following day, we collected zooplankton from Lost Lake by vertically towing a 100-μm mesh plankton net and added live zooplankton equivalent to 1,000 L in abundance to each enclosure. In addition, 200 individuals of Daphnia pulicaria collected at a nearby lake were added to each of the enclosures, as large zooplankton typical of small lakes in Montana were not abundant in Lost Lake when the experiment was initiated. Since animals contained in the shallow 2-m enclosures were unable to migrate to a deep depth and thus faced potentially harmful ultraviolet (UV) exposure, each enclosure was then covered by a Plexiglas plate to reduce UV exposure. On 12 June 2017, we initiated the enclosure experiment by adding 10 L of the leaf homogenate to a half of the unshaded and shaded enclosures, resulting in a total of four treatments each with three replicates; LA: unshaded with leaf homogenate, LB: unshaded without leaf homogenate, DA: shaded with leaf homogenate, and DB: shaded without leaf homogenate. During the experimental period, we added an additional 5 L of the leaf homogenate to enclosures with LA and DA treatments on both 3 July and 24 July 2017 using fresh leaf homogenate prepared as above.
Figure 1. (A) Photo showing enclosures used in the experiment. (B) Schemes showing the contribution of brown and green food chains for mass transfer to zooplankton under shaded and unshaded conditions with and without leaf homogenate. Light green arrows represent the effect of ambient nutrients on the green food chain (GBase), light brown arrows represent the effect of ambient organic matter on the brown food chain (BBase), dark green arrows represent the effect of leaf homogenate on the green food chain (GAdd), and dark brown arrows represent the effects of leaf homogenate on the brown food chain (BAdd).
We sampled water and plankton in each enclosure weekly from 12 June to 14 August. Before the sampling, we measured water temperature (°C), pH, and dissolved oxygen (mg O2/L) using a multiprobe sonde (Hydrolab® MS5, OTT HydroMet, CO., United States). Then, enclosures were gently mixed by raising and lowering a PVC disk (60 cm in diameter) several times. We then sampled 2 L of the water from the bottom to the surface using an integrated water column sampler to obtain samples for chemistry, chlorophyll a, microbes (bacteria and flagellates), and microzooplankton (ciliates, amoebas, rotifers, and small crustaceans such as copepod nauplii) and 13 L of water for examining mesozooplankton (cladocerans and copepods). The mesozooplankton samples were concentrated by passing the 13 L of water through a 100-μm mesh net and were then placed in sample jars. Screened water was returned to the enclosure.
We also routinely measured concentrations of pCO2 at the surface of the enclosures using a bottle head space method 3 days after the sampling. For measuring pCO2, we collected the surface water from each of the enclosures with two sets of 1.1-L High-Density Polyethylene (HDPE) Nalgene® bottles. We made a headspace by putting 50 mL of ambient air into each bottle using a syringe and then shook the bottle for at least 3 min by hand to equilibrate the CO2 between air and water. The air in the headspace was then collected by a syringe and used to measure CO2 with a portable infrared gas analyzer (EGM-4, PP Systems, MA, United States). After the measurement of pCO2, we homogenized the enclosures as above.
The leaf homogenate was subsampled prior to being used in the experiment and subsamples were concentrated onto GF/F filters. Filters were analyzed for seston particulate phosphorus (P), nitrogen (N), and organic carbon (C) concentrations. Water samples from the enclosures were also filtered onto the GF/F filters for chemical and chlorophyll a analyses. The filtrates of the leaf homogenate and the enclosure water samples were used for measuring dissolved P, dissolved N, and dissolved organic C concentrations. When zooplankton became abundant after 3 weeks (3 July 2017), the water samples were passed through a 100-μm mesh net to eliminate mesozooplankton and used for analyses. In addition, we occasionally concentrated water samples passed through a 30-μm mesh net onto the GF/F filters for measuring chlorophyll a concentration of small algae. Filters for chlorophyll a analysis were stored at −20°C and those for sestonic C, N, and P were dried at 60°C for 48 h and stored in a desiccator. Filtrates for measuring dissolved N, P, and organic C concentrations were stored at −20°C until analysis.
Chlorophyll a was measured using a fluorometer. Filters for seston C and N were measured using a carbon, hydrogen, and nitrogen (CHN) analyzer (Perkin Elmer 2400 series II; Perkin Elmer Inc., MA, United States). Seston P was measured using a spectrophotometer according to molybdenum blue method after digesting the filter samples with potassium persulfate at 121°C for 30 min. Dissolved N and dissolved organic C were measured using a total organic carbon and nitrogen analyzer (multi N/C 3100; Analytik Jena AG, Jana, Germany). Dissolved P was measured using the same method as seston P. We also measured organic C, total N, and total P contents of fallen leaves and leaf homogenate as above.
Samples for mesozooplankton were fixed with ethanol immediately after collection and preserved in 99% ethanol. Samples for bacteria and HNF were fixed with glutaraldehyde (1% final concentration) and stored at 4 °C in the dark. For examining microzooplankton, 500 ml of sample water was fixed with a Lugol’s solution (5% final concentration), and all organisms in the sample were concentrated down to 50 ml by gravity. Bacteria and HNF were quantitatively counted under an epifluorescence microscope at 1,000 × magnification. Mesozooplankton (cladocerans and copepods) and microzooplankton (rotifers, ciliates, and amoebas) were enumerated according to genus or finest taxonomic level under microscopes at 25–400 × magnification with the measurements of their body or cell sizes. The biomasses of these plankters were estimated with the appropriate conversion factors based on the body sizes. Details of these methods are described in Supplementary Methods.
In addition to the weekly sampling described, we sampled mesozooplankton for isotopic analysis on 3 and 17 August by vertical tows of 100-μm plankton net from the bottom to the surface of the enclosures. We also sampled water from the bottom to the surface of the enclosures using an integrating water column sampler. Particulate organic matter (POM) in the enclosures was concentrated onto GF/F filters by filtering an aliquot of the water samples. For measuring δ2H of the water, 100-mL of surface water was collected from the enclosures using a plastic bottle.
We taxonomically sorted the dominant cladocerans and copepods, and then 10–100 individuals of each taxon were placed in tin cups for carbon isotope analysis and in a silver cup for hydrogen isotope analysis. We also collected filamentous algae (mainly Zygnematophyceae) that were found in the mesozooplankton samples and placed these in cups. These were used as green food sources. The tin and silver cups with zooplankton and algae were dried at 60°C for 48 h and stored in desiccators until isotope analysis. Samples of POM were obtained by filtering 500 ml of surface lake water onto Whatman GF/F glass fiber filters (pre-combusted at 530 °C for 2 h). For brown food sources, we analyzed mixed leaves and needles that were used in making the leaf homogenate as well as five leaves from Poaceae plants surrounding the lake. The leaf samples were grounded by a homogenizer. The filters and well-mixed leaf samples were placed both in tin and silver cups.
The C isotope ratios of the samples were measured using a continuous-flow isotope mass spectrometer (Thermo Delta V Advantage, Thermo Fisher, MA, United States) interfaced with an elemental analyzer (NC2500, CE Instruments, Wigan, England) in the Cornell University Stable Isotope Laboratory (COIL). We expressed δ13C values using notation relative to Vienna Pee Dee Belemnite. The precision of δ13C values estimated by several internal organic standards was < ± 0.5 (/‰). We also analyzed samples for δ2H using a Thermo Delta V isotope mass spectrometer interfaced with a Temperature Conversion Elemental Analyzer (TC/EA, Thermo Fisher). The δ2H of water samples were analyzed by a GasBench II (Thermo Fisher) connected to a DELTA V (Thermo Fisher), which offered precision comparable to dual-inlet methods for H2 and CO2 water equilibration. In this analysis, non-exchangeable δ2H values were equilibrated for isotope exchange and normalized using the same procedure and standards as those in the previous studies (Wassenaar and Hobson, 2003; Doucett et al., 2007). All δ2H values are expressed as ordinal notation relative to the international standard, Vienna Standard Mean Ocean Water (VSMOW). All isotope analyses were performed by COIL.
We analyzed the initial difference in organic carbon and nutrient levels among enclosures with and without leaf homogenate using t-tests. For the main data, we performed a generalized linear mixed model (GLMM) to examine the effects of the leaf homogenate and light manipulation on water chemistry and biomass values of plankton consumers. In this analysis, we excluded data of the first three sampling dates to remove effects of initial conditions commonly among the enclosures and used data obtained from 3 July to 14 August. In the GLMM, the addition of leaf homogenate, light manipulation, and their interactions was set as fixed factors, and sampling date and enclosure were used as random factors. Before the analysis, chlorophyll a concentration, cell abundances of HNF and bacteria, and biomasses of zooplankters were log (n+1) transformed. The significance of the fixed effects was determined by type II ANOVA with F-tests of Kenward-Roger approximation. The analysis was done using “lmer” function of the “lme4” package and “car” function of the “car” package in R 3.4.0 (R Core Team, 2016).
We estimated the average biomass of zooplankton during the period from 3 July to 14 August for each treatment (ZBDB, ZBLB, ZBDA, and ZBLA). Then, by assuming that mass flow along with the brown food chain was not affected by light condition, the contributions of the brown and green food chains to zooplankton production were separated as follows:
where BBase and GBase are the fractions of zooplankton biomass produced by ambient organic matter and nutrients through brown and green chains, respectively, and BAdd and GAdd are the fractions of zooplankton biomass promoted by the addition of leaf homogenate through brown and green food chains, respectively (Figure 1B).
The statistical significance of differences among BAdd, GAdd, BBase, and GBase was assessed by comparing the 95% confidence limits that were estimated with a bootstrap method. In this study, we had four treatments with three replications (a total of 12 enclosures). Therefore, we randomly selected zooplankton biomass data in three enclosures from the 12 enclosers and assigned these for each treatment with repetition. Then, we estimated the average biomass for treatment (ZBDB, ZBLB, ZBDA, and ZBLA) and calculated values in Equations (1a–d). We repeated this procedure 999 times, estimated upper and lower 2.5% values for the 999 resampling values plus the original value and used these as a 95% confidence interval. If the 95% confidence intervals of a given contribution did not overlap with that of another contribution, we concluded that these contributions differed from each other. All analyses were performed in R 3.4.0 (R Core Team, 2016).
We also estimated the contributions of brown and green sources to zooplankton production using a Bayesian stable isotope mixing model, “MixSIAR” ver. 3.1.10 package (Stock et al., 2018) with JAGS ver. 4.3.0 connected with “rjags” package ver. 4–8 in R 3.4.0 (R Core Team, 2016). The “MixSIAR” model is a Bayesian stable isotope mixing model with unifying multiple error structures, including isotopes of consumers and sources, trophic enrichment of consumers. The model equations and details are in Stock et al. (2018). Before developing the mixing model, we bi-plotted the stable isotope values of all the samples (Supplementary Figure 1) to check that those consumers fell within the proper mixing polygon considering the trophic discrimination and food resources. Then, we excluded some outlier data (n = 7) from the polygon in the ensuing mixing model analysis. To confirm the validity of the result, we also analyzed with all of the data. In this analysis, we used filamentous algae and the leaf litter that was used for leaf homogenate as the potential food sources of autochthonous (green) and allochthonous origin (brown), respectively. We estimated the contribution of those two sources to the food that zooplankton assimilated. We assumed that the carbon and hydrogen isotope values of filamentous algae were the same for edible and filamentous algae as suggested by the previous studies (France, 1995; Hondula et al., 2014; Grosbois et al., 2017). In this analysis, we did not include POM since its stable isotope values were found between those of the algae and the leaf homogenate that themselves were similar to the values of leaf litter collected around the lake (Supplementary Figure 1).
Conventional trophic enrichment factors were used for zooplankton; +0.5 for δ13C (Post, 1997), and ± 0.0 for δ2H with 1.3 standard deviation in all the values (Post, 1997). We performed the mixing model of δ2H; δ2Hconsumer = [(1-ωcompound) × δ2H diet] + (ωcompound× δ2Hwater) with surface water values (δ2Hwater = -119.4 ± 3.6, mean ± SD, N = 24). Although the environmental water correction for the consumer (ωcompound) is known to vary depending on consumers (Wilkinson et al., 2015), Solomon et al. (2009) showed that it was around 0.20 ± 0.04 (mean ± SD) for zooplankton in freshwater systems. We used this value for ωcompound in our analysis. We did not use δ15N values in the analysis because the trophic levels of zooplankton taxa feeding on both brown and green foods were uncertain.
We ran the model with Markov chain Monte Carlo (MCMC) parameters that were set for “short” runs as defined in MixSIAR (Chain length = 50,000, Burn-in = 25,000, thin = 25, number of MCMC chains = 3) and evaluated the degree of convergence by the Gelman–Rubin test. We also assessed the correlations of posterior values for each final model to determine its ability to isolate contributions from different food sources–strong negative correlations between diets in close proximity in isotopic space indicate problems. We set the threshold correlation coefficient value, for a “strong” correlation at 0.7. We tested the appropriateness of the mixing model using two fictitious discrimination-corrected consumers with 100% of brown (allochthonous) and green (autochthonous) resource uses (Brett, 2014) and confirmed that the model output could provide reasonable resource contributions with ± SD = 0.103 (100% of allochthonous: allochthonous = 0.814 ± 0.130, 100% of autochthonous: autochthonous = 0.761 ± 0.103).
Total organic carbon content (TOC, as percent of dry mass) of the fallen leaves themselves was 35 and 300 times higher than total nitrogen (TN) and total phosphorus contents (TP), respectively (Table 1). Elemental analyses showed that TOC relative to TN and TP in the leaf homogenate was much lower than in the fallen leaves, indicating that leaching rates from the fallen leaves differed among the three elements. Our calculations indicated that 60% of P in the fallen leaves was leached into the leaf homogenate, but only 10% of N and organic C in the fallen leaves was leached (Table 1). In addition, in the leaf homogenate, 83% of P in the leaf homogenate was in the dissolved fraction, whereas 58 and 30% in C and N were in dissolved form, respectively.
Table 1. Mean and standard deviation (SD) of total organic carbon, total nitrogen, and total phosphorus contents of leaf litter (fallen leaves) and leaf homogenate used in the experiment, and concentrations of these elements in enclosures at the beginning of experiment.
Water samples collected just after the addition of the leaf homogenate showed that the concentrations of TOC and TN were significantly increased by three to four times in enclosures with the leaf homogenate compared with those without it (Table 1; p < 0.001, Supplementary Table 1 and Supplementary Figures 2A,B). Addition of the leaf homogenate also significantly elevated the concentration of TP by 10-fold (Table 1; p < 0.001, Supplementary Table 1 and Supplementary Figure 2C). The concentrations of these elements also tended to increase after the second and third additions of the leaf homogenate in the LA and DA treatments (Supplementary Figure 2).
In enclosures with leaf homogenate (LA and DA), seston C, N, and P concentrations varied temporally and reached high levels during the last half of the experimental period (Supplementary Figures 2D–F). In enclosures without leaf homogenate (LB and DB), however, seston C, N, and P concentrations were stable at low levels. Accordingly, seston C, N, and P concentrations were, on average, significantly different between enclosures with and without leaf homogenate (p < 0.001, Supplementary Table 2) but were not affected by light conditions (p > 0.1, Supplementary Table 2). Seston C: N ratio tended to increase in all the treatments (Figure 2B), but this trend was not statistically significant among the treatments (p > 0.05, Supplementary Table 2). Seston C: P ratio temporally varied depending on treatments (Figure 2A). In both the LA and DA treatments, the C: P ratio gradually decreased and was stabilized at a level < 200 (atomic). Seston C: P ratio was significantly higher in enclosures without leaf homogenate than in enclosure with leaf homogenate (p < 0.01, Supplementary Table 2) and was often > 300 especially in the LB treatment (Figure 2A), although no significant difference was detected between the unshaded and shaded treatments (p > 0.1, Supplementary Table 2).
Figure 2. Changes in (A) Seston C: P atomic ratio, (B) seston C: N atomic ratio, (C) water temperature during the experiment from 12 June to 14 August 2017, and (D) pCO2 that was measured beginning on 15th June. White squares indicate the LA treatment (unshaded, with leaf homogenate), brown squares indicate the DA treatment (shaded, with leaf homogenate), white circles indicate the LB treatment (unshaded, without leaf homogenate), and blue circles indicate the DB treatment (shaded, without leaf homogenate). Vertical bar on each data point indicates ± SD on the mean (3 replicates). The black arrows at the bottom of the graph are the dates when leaf homogenate was added to LA and DA treatments.
During the experiment, water temperature varied from 18°C in mid-June to 22°C in early August (Figure 2C) and was significantly higher in the unshaded enclosures with leaf homogenate (p < 0.001, Supplementary Table 1), although the difference was always less than 0.5°C. In enclosures with leaf homogenate, pCO2 reached levels > 1,500 ppm at the beginning of the experiment (Figure 2D). However, while remaining at high levels throughout the experiment in the shaded treatment (DA), pCO2 gradually decreased and stabilized at a low level in the unshaded treatment (LA). In enclosures without the leaf homogenate, pCO2 was consistently and significantly lower than in enclosures with the homogenate (p < 0.001, Supplementary Table 2). In enclosures without leaf homogenate, dissolved oxygen (DO) concentration was temporally stable and similar between the unshaded (LB) and shaded enclosures (DB). In enclosures with leaf homogenate, DO concentration was ∼5 mg/L at the beginning of the experiment but gradually increased to a level > 8 mg/L (Supplementary Figure 2G). On average, DO was significantly lower in the shaded treatments than in the unshaded treatments (p < 0.001, Supplementary Table 1) but not affected by the addition of the leaf homogenate (p > 0.05).
Chlorophyll a concentration varied over time (Figure 3A) and increased toward the end of the experiments, especially in enclosures with leaf homogenate (LA and DA). More than 60% of chlorophyll a was smaller than 30 μm in all the enclosures except for the last 2 weeks when chlorophyll a concentration increased due to an increase in abundance of filamentous algae (Supplementary Figure 3). On average, chlorophyll a concentration was significantly higher in enclosures with leaf homogenate (LA and DA) than those without (LB and DB). However, chlorophyll a concentration was not affected by light conditions (Supplementary Table 2). Bacterial abundance increased when leaf homogenate was added but always decreased rapidly to a level similar to the enclosures without the homogenate (Figure 3B). On average, bacterial abundance did not differ among experimental treatments (Supplementary Table 2). The abundance of HNF was also not affected by light conditions but did vary over time and was higher in enclosures with leaf homogenate compared with those without (Figure 3C and Supplementary Table 2).
Figure 3. Abundances of (A) chlorophyll a (<100 μm), (B) bacteria, (C) HNF, and biomasses of (D) ciliates, (E) amoebas, (F) rotifers, (G) cladocerans, (H) copepods, and (I) sum of this zooplankton during the experiment. White squares indicate the LA treatment (unshaded, with leaf homogenate), brown squares indicate the DA treatment (shaded, with leaf homogenate), white circles indicate the LB treatment (unshaded, without leaf homogenate), and blue circles indicate the DB treatment (shaded, without leaf homogenate). Vertical bars on each data point indicate ± SD on the mean (3 replicates). The black arrows at the bottom of the graph are the dates when leaf homogenate was added to LA and DA treatments.
During the study period, a variety of zooplankton taxa were observed (Supplementary Tables 3–5). Among the enclosures, cladocerans were the most abundant taxa, followed by copepods (Supplementary Figure 4). Except for the first several weeks, cladocerans were consistently abundant throughout the experiment in the LA treatment compared with other treatments, as evidenced by significant interaction effects of light and leaf homogenate on their abundance (Figure 3G and Supplementary Table 2). Copepod biomass was significantly higher in enclosures with leaf homogenate than in those without, regardless of light condition (Figure 3H and Supplementary Table 2). During the study period, rotifer biomass was low (Figure 3F) and did not differ among treatments (Supplementary Table 2). The biomass of ciliates and amoebas was significantly affected by the addition of leaf homogenate but not by light conditions (Supplementary Table 2). Ciliates increased their biomass from late-July to early-August in both the LA and DA treatments (Figure 3D), while the biomass of amoebas was especially high in late-August in the DA treatment (Figure 3E).
Although responses to the addition of leaf homogenate and light manipulation differed somewhat among taxonomic groups, as shown above, the sum of their biomass was significantly higher in enclosures with leaf homogenate compared with those without but was not consistently affected by light conditions (Supplementary Table 2). Reflecting the predominance of cladocerans in the zooplankton communities (Supplementary Figure 4), total zooplankton biomass (ZB: crustaceans, rotifers, and ciliated and amoeba protozoans) increased in the LA treatment and was consistently higher than those in other treatments (Figure 3I). Total zooplankton biomass in the DA treatment was similar to biomass in the LB and DB treatments in the first half of experimental period but gradually increased to a higher level in the last half of experiment, mainly to increased amoeba biomass. Total zooplankton biomass in the LB and DB treatments was stable at a low level throughout the experimental period.
Using Equations (1a)–(1d), we estimated zooplankton biomass produced by brown food and green food chains (Figure 4). In enclosures without leaf homogenate, the contribution of zooplankton biomass produced by ambient nutrients and organic matter through green food chain (GBase) was 0–25 μg C/L and, as evidenced by a large overlap of the 95% cl, did not significantly differ from the contribution generated through brown food chains (BBase). The contribution of zooplankton biomass produced by the addition of leaf homogenate through the brown chain (BAdd) was similar to that produced by ambient nutrients and organic matter (GBase and BBase). However, the contribution to zooplankton biomass produced by the leaf homogenate through the green chain (GAdd) was as high as 90 μg C/L and its 95% cl did not overlap with other contributions, indicating that the addition of leaf homogenate significantly increased zooplankton production and that the role of the green food chain in supporting zooplankton production was 3–4 times larger than that of the brown food chain.
Figure 4. Contribution to zooplankton biomass by ambient organic matter and nutrients through the green chain (GBase) and the brown food chain (BBase) and those by leaf homogenate through green food chain (GAdd) and brown food chain (BAdd). Filled areas indicate the frequency of distributions of bootstrap data, boxplots represent the median and the first and third quantiles, and vertical bars are ± 95% CI on the mean.
The relative contribution of autochthonous materials to food sources that zooplankton assimilated (estimated from stable isotope analyses, excluding data that were outside of the range polygon in the ensuing mixing model) varied from 20 to 80% depending on experimental treatment (Figure 5), but was similar in each treatment between 3 and 17 August. The relative contribution was only 20% in shaded enclosures with added t-OM (DA treatment) on average but was higher than 40% in other treatments (Figure 5). Using a GLMM analysis with ANOVA and Tukey post-hoc comparison tests, we confirmed that the relative contribution of the green food chain was significantly lower in the DA treatment and higher in the LA treatment when compared to the rest of treatments. In the LB and DB treatment, the mean autochthonous contribution ranged from 40 to 55%, indicating that half of the zooplankton biomass was produced by food derived from autochthonous materials. Almost the same results were obtained when we made the analysis with minimum (lower 2.5%) and maximum (upper 2.5%) contributions of autochthonous sources to zooplankton using all of the stable isotope data (Supplementary Figures 5, 6). We also assessed if the relative contribution of the green food chain was significantly different among zooplankton taxa using GLMM (Supplementary Table 6). The result showed the contribution did not differ among the zooplankton taxa (GLMM, p = 0.108).
Figure 5. Contributions of autochthonous sources to zooplankton production. The bold line in the box indicates the median value. The upper and lower limits of the box, and the whisker plots indicate the first and third quartiles, and ± 1.5 × interquartile range, respectively. Significant differences among treatments are denoted by different letters. Points with different colors represent values for different zooplankton taxa.
Since the pioneering work by Lindeman (1942), a number of studies have examined the importance of t-OM in supporting the production of higher trophic levels in lake ecosystems (Gasith and Hosler, 1976; Tranvik, 1992; Doucett et al., 2007; Ask et al., 2009; Brett et al., 2009; Cole et al., 2011). However, an overall view of the contribution of t-OM to lake food webs has been elusive and even controversial (Doucett et al., 2007; Carpenter et al., 2016; Brett et al., 2017). Carpenter et al. (2016) stated that zooplankton biomass is limited even when allochthony (consumption and assimilation of allochthonous material) is high because allochthonous organic matter is low-quality food, while zooplankton biomass would show wide variations depending on P supply rate when allochthony is low. Our study showed that t-OM can be an important P source, implying that low allochthony does not mean that t-OM contributes less to zooplankton production. Indeed, this study clearly showed that t-OM promoted a 4- to 5-fold increase in zooplankton biomass under ambient light conditions by stimulating mass transfer through the green food chain.
In our experiment, bacterial density increased only during the period immediately after leaf homogenate was added on 12 June, 3 July, and 24 July (Figure 3B) and did not show significant differences among enclosures with and without leaf homogenate. However, abundances of HNF were significantly increased in enclosures with the leaf homogenate. This result suggests that, although bacteria likely consumed and respired the leaf homogenate, their biomass did not accumulate due to increased predation by bacterivores. Supporting this inference, pCO2 at the surface was high in enclosures with leaf homogenate in the shaded treatments, indicating that leaf homogenate was respired and mineralized, and promoted mass transfer along with the brown food chain. However, even in the enclosures with leaf homogenate pCO2 was much lower under the unshaded conditions relative to the shaded condition. These results indicate that much more CO2 was consumed for photosynthesis by algae. Nonetheless, the abundance of chlorophyll a in these enclosures was similar between shaded and unshaded conditions. The results suggest that primary production in the unshaded enclosures was harvested for secondary production by the zooplankton. Indeed, zooplankton biomass increased in the unshaded enclosures with leaf homogenates.
According to the stable isotope analyses, half of the zooplankton biomass was produced directly or indirectly by allochthonous materials in the enclosures without leaf homogenate. The result indicates the importance of the terrigenous organic matter in sustaining the lake community and that the brown food chain plays a substantial role in supporting secondary production under ambient lake conditions (Doucett et al., 2007; Cole et al., 2011; Cole, 2013, Tanentzap et al., 2017). However, leaf homogenates did not increase the zooplankton biomass in the shaded enclosures, although they assimilated more materials from the brown food chain. These results imply that mass transfer along with the microbial chain extends less into zooplankton production. These findings confirm previous experimental studies that both terrigenously derived organic matter and heterotrophic microbes fueled by these are low-quality food for most herbivorous zooplankton (Brett et al., 2009; Kelly et al., 2014; Taipale et al., 2014). It should be noted that in the shaded enclosures with leaf homogenate, total zooplankton biomass gradually increased toward the end of the experiment. This increase was mainly due to an increase in the abundance of amoebas (Figure 3E) that are known to consume diverse organisms including algae, bacteria, and other micro heterotrophs (Mieczan, 2007).
Substantial amounts of leaf litter have been reported to enter into small lakes, such as Lost Lake (Gasith and Hosler, 1976; Rau, 1976; Hanlon, 1981). However, t-OM has often been viewed primarily as an energy or carbon source to aquatic ecosystems (e.g., Polis et al., 1997; Doucett et al., 2007; Cole et al., 2011; Carpenter et al., 2016; Brett et al., 2017) and not as a P source presumably due to its relatively high C to P ratio. Our study showed that P was leached from leaf litter much faster rate than organic carbon and nitrogen, despite its low content in the leaf litter. In freshwater ecosystems, P is often limiting for algal growth (Elser et al., 2007). Thus, the input of leaf litter can be an important P source for stimulating lake primary production. Studies examining leaf litter of various tree species have also shown that, among C, N, and P, P is the most efficiently leached across all litter types (Baldwin, 1999; Schreeg et al., 2013).
Some studies suggest that dissolved t-OM may decrease primary production by reducing light penetration into lakes (Carpenter et al., 1998; Ask et al., 2009; Karlsson et al., 2015). In this study, such a negative effect of t-OM was not detected. Rather, t-OM stimulated primary production by supplying limited nutrients. It should be noted that in this study, we used shallow enclosures where sufficient light was provided throughout the water column. However, in lakes where epilimnion extends into a deep depth, it is likely that dissolved t-OM reduces the light availability for phytoplankton (Carpenter et al., 1998; Karlsson et al., 2015). In such lakes, the positive effects of t-OM found in this study may be undermined by the negative effect. In other words, the relative importance of negative (light limitation) and positive effects (P supply) of t-OM on primary producers may vary depending on the lake depth.
Cottingham and Narayan (2013) and Fey et al. (2015) have shown that the addition of tree leaves to lake water elevated TP relative to organic C and TN and increased zooplankton production. However, it was not clear that which food chains, brown or green, contributed more to increased zooplankton production when t-OM was amended. In this study, therefore, we tried to separate the contributions of brown and green food chains to raising zooplankton production using the Equations (1a)–(1d), which assume that mass flow along with the brown food chains was not affected by light conditions. However, this assumption may not be correct if the majority of the DOC in our t-OM amendment was refractory but was photochemically transformed to labile forms, promoting bacterial production (Tranvik and Bertilsson, 2001). Moreover, increased primary production under the unshaded conditions may have facilitated bacterial production through the supply of autochthonous DOC (Karlsson et al., 2002; Kritzberg et al., 2005). In this case, our calculation of the contribution from the green food chains to zooplankton production may be overestimated. However, some primary production did occur in the shaded treatments since there was 10% irradiance, likely resulting in an overestimate of the flow of the brown food chains in the shaded treatments. To solve these uncertainties, we examined the diets of zooplankton using stable isotopes. The analysis showed that 50–75% of food sources assimilated by zooplankton were autochthonous in the unshaded enclosures even when leaf homogenate was added (the LA treatment). These results suggest that the addition of leaf homogenate promoted zooplankton production mainly through green food chains.
Zooplankton growth is often limited by food quality especially when the C: P ratio of phytoplankton food is > 200 (e.g., Frost et al., 2006; Urabe et al., 2018). In the unshaded enclosures without leaf homogenate, the seston C: P ratio was > 300 for most of the dates, although the seston C: N ratio did not differ substantially among the enclosures with different treatments. Therefore, phytoplankton may have been stoichiometrically low-quality food for zooplankton under ambient lake conditions, suggesting that even if the primary production rate is substantial, the contribution of the green food chain to production at a higher trophic level can be limited relative to that of the brown food chain in P deficient lakes. However, in enclosures with leaf homogenate, seston C: P ratio was lower and close to Redfield ratio in the unshaded condition (LA) than in the shaded condition (DA) especially during the last several weeks of the experiment. This result suggests that the leaf homogenate provided sufficient amounts of P to primary producers and improved the stoichiometric quality of phytoplankton as food for zooplankton.
The effects of C, N, and P in t-OM on aquatic ecosystems may depend on the timescale considered because of differences among these elements in rates of leaching. According to Schreeg et al. (2013), > 50% of P in the leaf litter was released in inorganic forms within only 4 h when these were soaked in water, but immediate release rates of DOC and TN from the leaf litter were < 10% and varied depending on tree species. Although we artificially processed leaf litters by mechanical shredding, the release rates of these elements from the leaf litter to leaf homogenate (Table 1) produced similar results. Thus, P leached from leaf litter can affect the aquatic food web on short time scales in nature. However, since the majority of C and N in t-OM is less efficiently leached over short time scales and decomposes slowly through shredding and crushing processes by benthic organisms, its effects may be more modest but temporally prolonged. Hence, we need to examine the long-term effects of t-OM on the aquatic food web and production (Fey et al., 2015).
Although fallen leaves entering a lake provide POC and DOC every year, the effects of t-OM on aquatic food web may change depending on the timing of input. Although this study was made in summer, the majority of litterfall occurs in the fall in north temperate areas (e.g., Gasith and Hosler, 1976). Since the water temperatures are relatively low in fall, the input of leaf litter into lakes in the fall may have little impact on aquatic food webs. However, nutrients leached from leaf litter in fall may be left unused in winter and be able to support aquatic production in the following spring. More importantly, the seasonality of leaf litter production differs depending on region and vegetation type in watersheds (e.g., evergreen vs. deciduous species: Gasith and Hosler, 1976; Alhamd et al., 2004). For example, although the production of litterfall is generally high in the fall in north temperate areas, summer peaks of the litterfall are often found even in deciduous forests such as that composed of Alnus (Kikuzawa et al., 1984). Moreover, the stoichiometry and leaching rates of nutrients will differ among leaf litter from different tree species. Thus, the effects of t-OM on green and brown food chains likely differ depending on the timing of the litter input and the types of vegetation in the watershed.
In this study, amendment of leaf homogenate increased DOC concentrations by 3 times and TP by 10 times when compared with the ambient levels. Although the TP level was lower than those in plankton culture media used for experimental studies (e.g., Lindström, 1983; Kilham et al., 1998), this study may have exaggerated the effects of leaf litters on zooplankton production. In addition, this study removed a large size fraction of leaf litter by screening. This large size fraction may be an important resource for benthic invertebrates (Batt et al., 2015). Shredding and crushing leaf litter by benthic invertebrates can promote brown food chains and nutrient cycling (Covich et al., 1999; Cross et al., 2005). This suggests that, in addition to research examining the contributions of green vs. brown food chains to higher trophic levels, benthic–pelagic coupling also requires further attention to more fully understand how t-OM affects aquatic food webs.
Although t-OM is rich in C, most of C in leaf litter is refractory while P is easily leached. Classically, t-OM has been viewed as a carbon or energy source for aquatic ecosystems. However, it can also be an important P source for primary producers as suggested by Cottingham and Narayan (2013) and thus promote mass transfer to higher trophic levels along with green food chain. As shown in this study, the stoichiometry of materials leached from t-OM is not necessarily the same as that of the t-OM itself. To understand the roles of trophic subsidies to ecosystems, therefore, we need to consider stoichiometric functions of the subsidized materials for green and brown food chains.
The datasets presented in this study can be found in the online Dryad Repository, Dataset, https://doi.org/10.5061/dryad.8pk0p2nqv.
JU, FH, and JE planned and designed the enclosure experiment. JE and TT arranged access to Lost Lake for the experiment. FH, JU, HD, TK, TN, TT, IK, MY, TY, and JE contributed to the experimental setup and carried out the experiment. FH, TK, TN, and TT performed chemical analyses and enumerated plankton. HD and IK performed stable isotope analysis. JU, FH, and HD performed statistical analyses. JU and FH wrote the draft. All authors contributed to the article and approved the submitted version.
This study was financially supported by the Japan Society for the Promotion of Science (JSPS) Grant-in-Aid for Scientific Research (KAKENHI) (16H02522 and 20H03315).
We appreciate Adam Bauman, Matthew Church, and staff at the FLBS for their help in lab work and Tamaki Doi and Tamon Doi for their assistance with field works during the experiment. We also thank the Crecelius and Sailors families for permission to work on their lake and conduct our enclosure experiment.
The authors declare that the research was conducted in the absence of any commercial or financial relationships that could be construed as a potential conflict of interest.
All claims expressed in this article are solely those of the authors and do not necessarily represent those of their affiliated organizations, or those of the publisher, the editors and the reviewers. Any product that may be evaluated in this article, or claim that may be made by its manufacturer, is not guaranteed or endorsed by the publisher.
The Supplementary Material for this article can be found online at: https://www.frontiersin.org/articles/10.3389/fevo.2022.956819/full#supplementary-material
Alhamd, L., Arakaki, S., and Hagihara, A. (2004). Decomposition of leaf litter of four tree species in a subtropical evergreen broad-leaved forest, Okinawa Island, Japan. For. Ecol. Manage. 202, 1–11. doi: 10.1016/j.foreco.2004.02.062
Adrian, R., Wickham, S. A., and Butler, N. M. (2001). Trophic interactions between zooplankton and the microbial community in contrasting food webs: The epilimnion and deep chlorophyll maximum of a mesotrophic lake. Aquat. Microb. Ecol. 24, 83–97. doi: 10.3354/ame024083
Ask, J., Karlsson, J., Persson, L., Askm, P., Byström, P., and Jansson, M. (2009). Terrestrial organic matter and light penetration: Effects on bacterial and primary production in lakes. Limnol. Oceanogr. 54, 2034–2040. doi: 10.4319/lo.2009.54.6.2034
Baldwin, D. S. (1999). Dissolved organic matter and phosphorus leached from fresh and ‘terrestrially’ aged river red gum leaves: Implications for assessing river–floodplain interactions. Freshw. Biol. 41, 675–685. doi: 10.1046/j.1365-2427.1999.00404.x
Batt, R. D., Carpenter, S. R., Cole, J. J., Pace, M. L., Johnson, R. A., Kurtzweil, J. T., et al. (2015). Altered energy flow in the food web of an experimentally darkened lake. Ecosphere 6, 1–23. doi: 10.1890/ES14-00241.1
Bloem, J., Albert, C., Bar-Gillissen, M.-J. B., Berman, T., and Cappenberg, T. E. (1989). Nutrient cycling through phytoplankton, bacteria and protozoa, inselectively filtered Lake Vechten water. J. Plankton Res. 11, 119–131. doi: 10.1093/plankt/11.1.119
Brett, M. T. (2014). Resource polygon geometry predicts Bayesian stable isotope mixing model bias. Mar. Ecol. Prog. Ser. 514, 1–12. doi: 10.3354/meps11017
Brett, M. T., Kainz, M. J., Taipale, S. J., and Seshan, H. (2009). Phytoplankton not allochthonous carbon, sustains herbivorous zooplankton production. Proc. Natl. Acad. Sci. U.S.A. 106, 21197–21201. doi: 10.1073/pnas.0904129106
Brett, M. T., Bunn, S. E., Chandra, S., Galloway, A. W., et al. (2017). How important are terrestrial organic carbon inputs for secondary production in freshwater ecosystems? Freshw. Biol. 62, 833–853. doi: 10.1111/fwb.12909
Carpenter, S. R., and Kitchell, J. F. (1996). The trophic cascade in lakes. Cambridge: Cambridge University Press.
Carpenter, S. R., Cole, J. J., Pace, M. L., and Wilkinson, G. B. (2016). Response of plankton to nutrients, planktivory and terrestrial organic matter: A model analysis of whole-lake experiments. Ecol. Lett. 19, 230–239. doi: 10.1111/ele.12558
Carpenter, S. R., Cole, J. J., Kitchell, J. F., and Pace, M. L. (1998). Impact of dissolved organic carbon, phosphorus, and grazing on phytoplankton biomass and production in experimental lakes. Limnol. Oceanogr. 43, 73–80. doi: 10.4319/lo.1998.43.1.0073
Cole, J. J., Carpenter, S. R., Kitchell, J., Pace, M. L., Solomon, C. T., and Weidel, B. (2011). Strong evidence for terrestrial support of zooplankton in small lakes based on stable isotopes of carbon, nitrogen, and hydrogen. Proc. Natl. Acad. Sci. U.S.A. 108, 175–1980. doi: 10.1073/pnas.1012807108
Cole, J. J. (2013). “Freshwater ecosystems and the carbon cycle,” in Excellence in ecology, Vol. 18, ed. O. Kinne (Oldendorf: International Ecology Institute), 146.
Cottingham, K. L., and Narayan, L. (2013). Subsidy quantity and recipient community structure mediate plankton responses to autumn leaf drop. Ecosphere 4, 1–18. doi: 10.1890/ES13-00128.1
Covich, A. P., Palmer, M. A., and Crowl, T. A. (1999). The role of benthic invertebrate species in freshwater ecosystems: Zoobenthic species influence energy flows and nutrient cycling. BioScience 49, 119–127. doi: 10.2307/1313537
Cross, W. F., Benstead, J. P., Frost, P. C., and Thomas, S. A. (2005). Ecological stoichiometry in freshwater benthic systems: Recent progress and perspectives. Freshw. Biol. 50, 1895–1912. doi: 10.1111/j.1365-2427.2005.01458.x
Doucett, R. R., Marks, J. C., Blinn, D. W., Caron, M., and Hungate, B. A. (2007). Measuring terrestrial subsidies to aquatic food webs using stable isotopes of hydrogen. Ecology 88, 1587–1592. doi: 10.1890/06-1184
Elser, J. J., Matthew, M. E. S., Cleland, E. E., Gruner, D. S., Harpole, W. S., Hillebtand, H., et al. (2007). Global analysis of nitrogen and phosphorus limitation of primary producers in freshwater, marine and terrestrial ecosystems. Ecol. Lett. 10, 1135–1142. doi: 10.1111/j.1461-0248.2007.01113.x
Fey, S. B., Mertens, A. N., and Cottingham, K. L. (2015). Autumn leaf subsidies influence spring dynamics of freshwater plankton communities. Oecologia 178, 875–885. doi: 10.1007/s00442-015-3279-5
France, R. L. (1995). Differentiation between littoral and pelagic food webs in lakes using stable carbon isotopes. Limnol. Oceanogr. 40, 1310–1313. doi: 10.4319/lo.1995.40.7.1310
Frost, P. C., Benstead, J. P., Cross, W. F., James, H. H., Marguerite, H. L., Xenopoulos, A., et al. (2006). Threshold elemental ratios of carbon and phosphorus in aquatic consumers. Ecol. Lett. 9, 774–779. doi: 10.1111/j.1461-0248.2006.00919.x
Gasith, A., and Hosler, A. D. (1976). Airborne litterfall as a source of organic matter in lakes. Limnol. Oceanogr. 21, 253–258. doi: 10.4319/lo.1976.21.2.0253
Grosbois, G., Del Giorgio, P. A., and Rautio, M. (2017). Zooplankton allochthony is spatially heterogeneous in a boreal lake. Freshw. Biol. 62, 474–490. doi: 10.1111/fwb.12879
Hanlon, R. D. G. (1981). Allochthonous plant litter as a source of organic material in an oligotrophic lake (Llyn Frongoch). Hydrobiologia 80, 257–261. doi: 10.1007/BF00018365
Hondula, K. L., Pace, M. L., Cole, J. J., and Batt, R. D. (2014). Hydrogen isotope discrimination in aquatic primary producers: Implications for aquatic food web studies. Aquat. Sci. 76, 217–229. doi: 10.1007/s00027-013-0331-6
Jansson, M., Persson, L., De Roos, A. M., Jones, R. I., and Tranvik, L. J. (2007). Terrestrial carbon and intraspecific size-variation shape lake ecosystems. Trends Ecol. Evol. 22, 316–322. doi: 10.1016/j.tree.2007.02.015
Karlsson, J., Bergstom, A. K., Bystrom, P., Gudasz, C., Rodriguez, P., and Hein, C. (2015). Terrestrial organic matter input suppresses biomass production in lake ecosystems. Ecology 96, 2870–2876. doi: 10.1890/15-0515.1
Karlsson, J., Jansson, M., and Jonsson, A. (2002). Similar relationships between pelagic primary and bacterial production in clearwater and humic lakes. Ecology 83, 2902–2910. doi: 10.1890/0012-9658(2002)083[2902:SRBPPA]2.0.CO;2
Kelly, P. T., Solomon, C. T., Weidel, B. C., and Jones, S. E. (2014). Terrestrial carbon is a resource, but not a subsidy, for lake zooplankton. Ecology 95, 1236–1242. doi: 10.1890/13-1586.1
Kikuzawa, K., Asai, T., and Fukuchi, M. (1984). Leaf-litter production in a plantation of Alnus inokumae. J. Ecol. 72, 993–999. doi: 10.2307/2259546
Kilham, S. S., Kreeger, D. A., Lynn, S. G., Goulden, C. E., and Herrera, L. (1998). COMBO: A defined freshwater culture medium for algae and zooplankton. Hydrobiologia 377, 147–159. doi: 10.1023/A:1003231628456
Kritzberg, E. S., Cole, J. J., Pace, M. M., and Granéli, W. (2005). Does autochthonous primary production drive variability in bacterial metabolism and growth efficiency in lakes dominated by terrestrial C inputs? Aquat. Microb. Ecol. 38, 103–111. doi: 10.3354/ame038103
Lindeman, R. L. (1942). The trophic-dynamic aspect of ecology. Ecology 23, 399–417. doi: 10.2307/1930126
Lindström, K. (1983). Selenium as a growth factor for plankton algae in laboratory experiments and in some Swedish lakes. Hydrobiologia 101, 35–48. doi: 10.1007/BF00008655
McQueen, D. J., Johannes, M. R. S., Post, J. R., Steward, T. J., and Lean, D. R. S. (1989). Bottom–up and top–down impacts on freshwater pelagic community structure. Ecol. Monogr. 59, 289–310. doi: 10.2307/1942603
Mieczan, T. (2007). Seasonal patterns of testate amoebae and ciliates in three peatbogs: Relationship to bacteria and flagellates (Poleski National Park, Eastern Poland). Ecohydrol. Hydrobiol. 7, 77–89. doi: 10.1016/S1642-3593(07)70191-X
Nakano, S., and Murakami, M. (2001). Reciprocal subsidies: Dynamic interdependence between terrestrial and aquatic food webs. Proc. Natl. Acad. Sci. U.S.A. 98, 166–170. doi: 10.1073/pnas.98.1.166
Polis, G. A., Anderson, W. B., and Holt, R. D. (1997). Toward an integration of landscape and food web ecology: The dynamics of spatially subsidized food webs. Annu. Rev. Ecol. Syst. 28, 289–316. doi: 10.1146/annurev.ecolsys.28.1.289
Post, D. M. (2002). Using stable isotopes to estimate trophic position: models, methods, and assumptions. Ecology, 83, 703–718. doi: 10.1890/0012-9658(2002)083[0703:USITET]2.0.CO;2
R Core Team (2016). R: A language and environment for statistical computing. Vienna: R Foundation for Statistical Computing.
Rau, G. H. (1976). Dispersal of terrestrial plant litter into a subalpine lake. Oikos 27, 153–160. doi: 10.2307/3543445
Schreeg, L. A., Mack, M. C., and Turner, B. L. (2013). Nutrient-specific solubility patterns of leaf litter across 41lowland tropical woody species. Ecology 94, 94–105. doi: 10.1890/11-1958.1
Solomon, C. T., Cole, J. J., Doucett, R. R., Pace, M. L., Preston, N. D., Smith, L. E., et al. (2009). The influence of environmental water on the hydrogen stable isotope ratio in aquatic consumers. Oecologia 161, 313–324. doi: 10.1007/s00442-009-1370-5
Stock, B. C., Jackson, A. L., Ward, E. J., Parnell, A. C., Phillips, D. L., and Semmens, B. X. (2018). Analyzing mixing systems using a new generation of Bayesian tracer mixing models. PeerJ 6:e5096. doi: 10.7717/peerj.5096
Taipale, S. T., Brett, M. T., Hahn, M. W., Martin-Creuzburg, D., Yeung, S., Hiltunen, M., et al. (2014). Differing Daphnia magna assimilation efficiencies for terrestrial, bacterial, and algal carbon and fatty acids. Ecology 95, 563–576. doi: 10.1890/13-0650.1
Tanentzap, A. J., Kielstra, B. W., Wilkinson, G. M., Berggren, M., Craig, N., Del Giorgio, P. A., et al. (2017). Terrestrial support of lake food webs: Synthesis reveals controls over cross-ecosystem resource use. Sci. Adv. 3:e1601765. doi: 10.1126/sciadv.1601765
Tranvik, L. J., and Bertilsson, S. (2001). Contrasting effects of solar UV radiation on dissolved organic sources for bacterial growth. Ecol. Lett. 4, 458–463. doi: 10.1046/j.1461-0248.2001.00245.x
Tranvik, L. J. (1992). Allochthonous dissolved organic matter as an energy source for pelagic bacteria and the concept of the microbial loop. Dissolved Org. Matter Lacustrine Ecosyst. 229, 107–114. doi: 10.1007/BF00006994
Urabe, J., Shimizu, Y., and Yamaguchi, T. (2018). Understanding the stoichiometric limitation of herbivore growth: The importance of feeding and assimilation flexibilities. Ecol. Lett. 21, 197–206. doi: 10.1111/ele.12882
Wallace, J. B., Eggert, S. L., Meyer, J. L., and Webster, J. R. (1997). Multiple trophic levels of a forest stream linked to terrestrial litter inputs. Science 522, 102–104. doi: 10.1126/science.277.5322.102
Wassenaar, L. I., and Hobson, K. A. (2003). Comparative equilibration and online technique for determination of non-exchangeable hydrogen of keratins for use in animal migration studies. Isotopes Environ. Health Stud. 39, 211–217. doi: 10.1080/1025601031000096781
Wilkinson, G. M., Cole, J. J., and Pace, M. L. (2015). Deuterium as a food source tracer: Sensitivity to environmental water, lipid content, and hydrogen exchange. Limnol. Oceanogr. Methods 13, 213–223. doi: 10.1002/lom3.10019
Wolkovich, E. M., Allesina, S., Cottingham, K. L., Moore, J. C., Sandin, S. A., and de Mazancourt, C. (2014). Linking the green and brown worlds: The prevalence and effect of multichannel feeding in food webs. Ecology 95, 3376–3386. doi: 10.1890/13-1721.1
Keywords: ecological stoichiometry, lake ecosystems, leaf litter, phosphorus, stable isotope analysis, terrestrial subsidy, secondary production
Citation: Hirama F, Urabe J, Doi H, Kazama T, Noguchi T, Tappenbeck TH, Katano I, Yamamichi M, Yoshida T and Elser JJ (2022) Terrigenous subsidies in lakes support zooplankton production mainly via a green food chain and not the brown food chain. Front. Ecol. Evol. 10:956819. doi: 10.3389/fevo.2022.956819
Received: 30 May 2022; Accepted: 25 July 2022;
Published: 25 August 2022.
Edited by:
Rona A. R. McGill, University of Glasgow, United KingdomReviewed by:
Mojmir Vasek, Biology Centre of the Czech Academy of Sciences, CzechiaCopyright © 2022 Hirama, Urabe, Doi, Kazama, Noguchi, Tappenbeck, Katano, Yamamichi, Yoshida and Elser. This is an open-access article distributed under the terms of the Creative Commons Attribution License (CC BY). The use, distribution or reproduction in other forums is permitted, provided the original author(s) and the copyright owner(s) are credited and that the original publication in this journal is cited, in accordance with accepted academic practice. No use, distribution or reproduction is permitted which does not comply with these terms.
*Correspondence: Jotaro Urabe, dXJhYmVAdG9ob2t1LmFjLmpw
†Present Addresses: Takehiro Kazama, Graduate School of Human Development and Environment, Kobe University, Kobe, Japan; Masato Yamamichi, School of Biological Sciences, The University of Queensland, Brisbane, QLD, Australia
Disclaimer: All claims expressed in this article are solely those of the authors and do not necessarily represent those of their affiliated organizations, or those of the publisher, the editors and the reviewers. Any product that may be evaluated in this article or claim that may be made by its manufacturer is not guaranteed or endorsed by the publisher.
Research integrity at Frontiers
Learn more about the work of our research integrity team to safeguard the quality of each article we publish.