- 1Department of Physical and Environmental Sciences, University of Toronto Scarborough, Toronto, ON, Canada
- 2Ducks Unlimited Canada, Stonewall, MB, Canada
- 3Department of Soil Science, University of Manitoba, Winnipeg, MB, Canada
- 4Department of Resource Economics and Environmental Sociology, University of Alberta, Edmonton, AB, Canada
- 5Department of Agricultural and Resource Economics, University of Saskatchewan, Saskatoon, SK, Canada
- 6Ducks Unlimited Canada, Ottawa, ON, Canada
This study advances scientific understanding of the magnitude of carbon sequestration that could be achieved through conservation (securing existing carbon stocks) and restoration (creating new carbon stocks) of freshwater mineral soil wetlands on agricultural landscapes. Within an agricultural landscape in southern Ontario (Canada), 65,261 wetlands comprising 63,135 ha were lost. Of these, 6,899 wetlands comprising 5,198 ha were “easy-to-restore” wetlands, defined as wetlands that were small (<0.5 ha), with no hydrological inflow or outflow, and that were drained by a drainage ditch and could be restored by plugging the drainage ditch. Within these easy-to-restore wetlands, a chronosequence of wetlands that covered a range of restoration ages [i.e., drained (0 years), 15 years, 25 years, 40 years, and intact marshes] was established to capture potential changes in rates of sedimentation and organic carbon (OC) sequestration with restoration age. Three sediment cores were collected at the center of the open-water portion of the wetland and segmented in the field. In the lab, each individual segment from each core was dried, sieved through a 2-mm mesh, weighed and analyzed for 137Cs and 210Pb radioisotopes and OC. OC stocks (35.60 Mg ha–1) and OC sequestration rates (0.89 Mg C ha–2 yr–1) in wetlands restored for 40 years were comparable to if not marginally larger than intact wetlands, suggesting that restoration promotes OC sequestration but that an initial recovery phase of up to 25 years or more is needed before returning to a pre-drainage equilibrium. An economic analysis to compare the costs and benefits of wetland conservation and restoration was then conducted. The benefit-cost analysis revealed that the financial benefits of carbon sequestration are greater than the financial costs over a 30-year time horizon for retaining wetlands but not for restoring wetlands. The breakeven costs such that wetland restoration is economically feasible based on current carbon price projections is estimated to be $17,173 CAD ha–1 over the 30-year time horizon; any wetland restoration project that costs this amount or less could be justified on economic grounds based solely on the carbon benefits. This study’s findings indicate that wetlands are important nature-based climate solutions, but that incentivizing their use through a carbon market will require either scientific innovations to reduce restoration costs or increase carbon sequestration rates, or stacking carbon benefits with other ecosystem service benefits into a comprehensive market for nature-based climate solutions.
Introduction
There is growing interest in applying nature-based climate solutions (NbCS) (Cohen-Shacham et al., 2016), including retention (avoided conversion) and restoration, to help countries realize and augment greenhouse gas (GHG) reduction targets (Girardin et al., 2021). Griscom et al. (2017) concluded that implementation of cost-effective NbCS – based on protection, management, and restoration actions that increase carbon storage and/or avoid GHG emissions from forests, wetlands, grasslands, and agricultural landscapes – could help meet up to one-third of the global GHG reductions required by 2030 to keep warming below 2°C. Drever et al. (2021) estimated that Canada could meet approximately 15% of its 2030 511 Tg CO2-eq yr–1 (139 Tg C yr–1) emissions target through NbCS, and that avoided conversion of natural habitats like wetlands was among the most effective NbCS actions. Drever et al. (2021) suggested that the restoration of freshwater mineral soil wetlands could reduce GHG emissions by 5.5 to 34.9 Tg CO2-eq yr–1 (1.5 to 9.5 Tg C yr–1) by 2030. While this is not a substantial contribution to the total GHG emissions reductions required nationally, it could be quite significant for helping the agricultural sector meet sector-specific GHG reduction targets.
The agricultural sector is increasingly interested in climate mitigation/adaptation strategies (Gillan et al., 2021) and many agricultural companies are aligning carbon neutrality with these strategies by working across their supply chain to reduce emissions (Santos et al., 2021). Fully understanding the carbon impact of wetland retention and restoration could help provide appropriate incentives to support agricultural producers while helping governments and companies to meet their sustainability targets. However, there remain significant uncertainties about how to account for the contributions of wetland restoration to GHG reductions in agricultural landscapes. Integral to any approaches to promote carbon sequestering practices in managed soils are reliable, accurate, and cost-effective means to quantify soil organic carbon (OC) stock changes and forecast soil OC sequestration rates to different management, climate, and edaphic conditions (Paustian et al., 2019).
This paper was motivated by the challenges in understanding, predicting, and managing wetlands in working agricultural landscapes that are increasingly impacted by humans (Golden et al., 2017). In Canada, wetland loss since the pre-settlement 1800s has been estimated at 20 million ha nationally (Environment Canada, 1991). While there is uncertainty associated with the degree of wetland loss, it is generally accepted that between 40 and 70% of historical wetlands in the agricultural landscapes of Canada has been converted to other land uses (Dahl and Watmough, 2007; Bartzen et al., 2010). These wetlands continue to be lost at alarming rates (e.g., Watmough and Schmoll, 2007; Watmough et al., 2017), despite increasing recognition of the multiple ecosystem services provided by wetlands and their value to society (de Groot et al., 2012; Creed et al., 2017; Davidson et al., 2019; Asare et al., 2022). This scale of wetland loss can have large implications for soil OC [e.g., Byun et al. (2018) estimate a loss of soil OC stocks of up to 1.58 Tg C in southern Ontario].
Here, we focus on freshwater mineral soil wetlands that provide important ecosystem services, including water purification (phosphorus and nitrogen retention), flood mitigation, and carbon sequestration (Marton et al., 2015; Cohen et al., 2016; Rains et al., 2016). More specifically we combine a biophysical approach, where we apply radiometric dating to assess carbon stocks and sequestration rates, with an economic analysis to examine the feasibility of wetlands as NbCS. To our knowledge, this type of assessment has rarely been performed for wetlands and is especially lacking for freshwater mineral soil wetlands. We show how the agricultural sector can contribute to net carbon reductions through wetland conservation and restoration in these highly degraded agricultural landscapes. The paper has two objectives: (1) to estimate the magnitude of wetland loss in southern Ontario; and (2) to estimate OC stocks and sequestration rates in natural, degraded, and restored mineral wetlands. From these estimates, we provide scientific evidence of the carbon sequestration potential of freshwater mineral soil wetlands that in turn is used to conduct an economic analysis of the feasibility of wetland restoration based on carbon values alone.
Materials and Methods
Study Area
The study area is the 87,410 km2 area of southern Ontario. The study area has a humid continental climate moderated by the Great Lakes, with warm summers and cool winters (+2.3°C average mean annual temperature for 1978–2018) and wet weather (910 mm average total annual precipitation) (McKenney et al., 2011; Canadian Forest Service [CFS], 2020). Mean annual temperatures increased 0.04°C yr–1 and total annual precipitation increased 1.5 mm yr–1 from 1978 to 2018.
The study area covers the Mixed Wood Plains ecozone (Environment Canada, 1995), which comprises temperate deciduous forest on Paleozoic rock, mostly limestone, covered with deposits of glacial till and mineral soils that are mostly leached, nutrient-poor, and calcium-rich (Soil Landscapes of Canada Working Group [SLCWG], 2010). Most original forest in the study area has been lost to urban, industrial, and agricultural land uses – only 16.5% of land area use in the study area is still forest, while 8.7% is developed, 35.5% is agriculture, and 13.8% is wetland (the remaining 24.9% of land use is classified as “undifferentiated”) (Ontario Ministry of Natural Resources and Forestry [OMNRF], 2019; Figure 1A). Of the 13.8% wetland area, 248,478 individual wetlands comprise a total area of 1,159,931 ha—of these wetlands, 20.0% in number (14.7% in area) are marshes, 79.2% in number (83.8% in area) are swamps, 0.2% in number (0.8% in area) are bogs, and 0.7% in number (0.7% in area) are fens (Figure 1B).
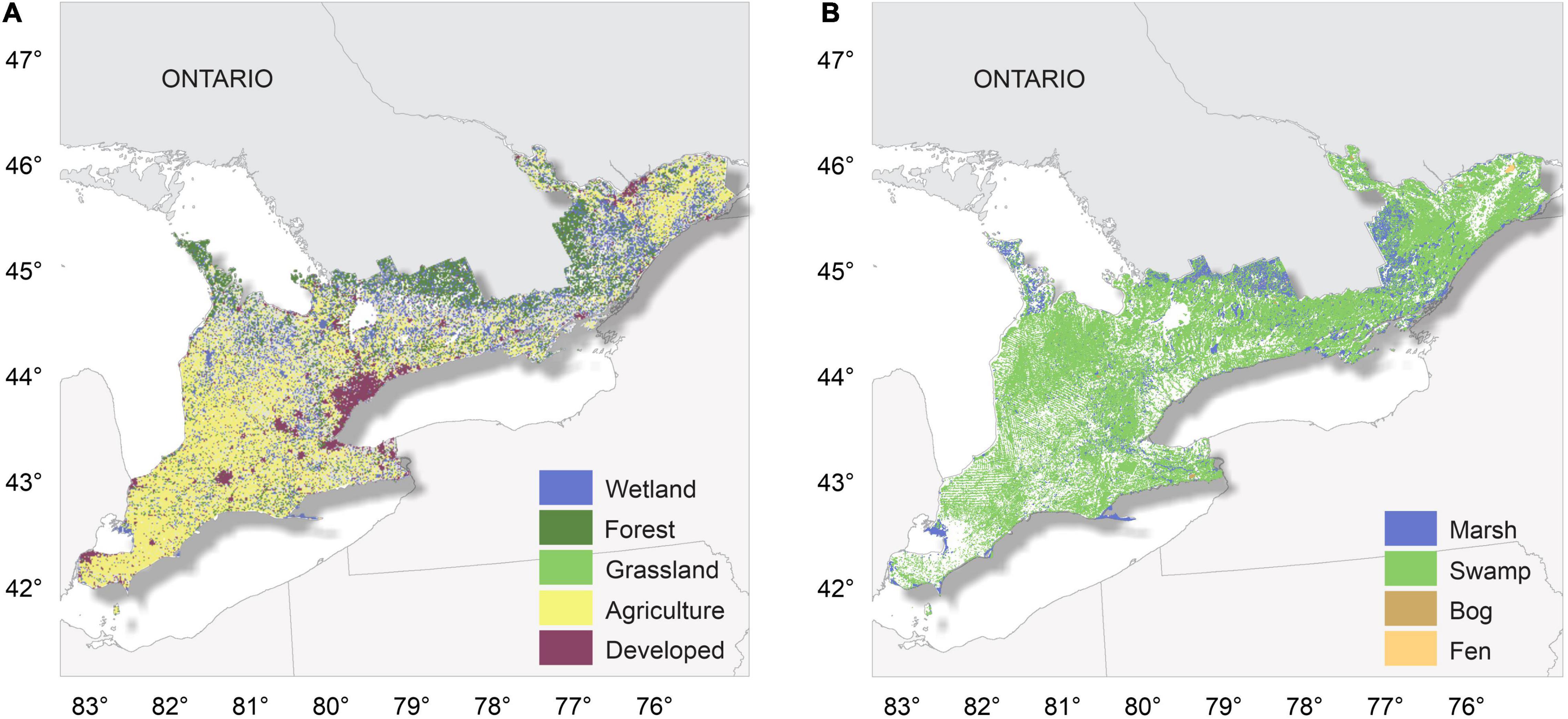
Figure 1. Southern Ontario landscape: (A) SOLRIS inventory land cover. (B) Distribution of wetland types in the SOLRIS land use inventory.
Restorable Wetlands
Easy-to-restore wetlands are small (<0.5 ha) and geographically isolated (without a hydrological inflow or outflow) marshes that can be drained by a drainage ditch and restored by plugging the drainage ditch (Tiner, 2003).
To estimate the historical loss of easy-to-restore wetlands, we conducted an analysis of the relationship between the area (x-axis) and frequency (y-axis) of the contemporary wetland inventory. Following the premise that the natural distribution of waterbodies by area is fractal (Brown et al., 2002), the relationship between the area (a) and frequency (f) of wetlands on a natural (undisturbed) landscape follows a power-law function, in which a negative linear relationship is observed between a and f when plotted on logarithmic-logarithmic axes: log10(f) = mlog10aexp, where m is the slope of the regression (Zhang et al., 2009). If the slope deviates from the power-law slope below a breakpoint, it is assumed that wetland loss has occurred. We objectively identified the breakpoint by applying (a) a three-segment piecewise linear regression to log10(f) (wetland frequency) and log10(a) (wetland area) to identify an upper (low frequency) breakpoint above which there are few or no frequency data (these data are removed), and (b) a two-segment piecewise linear regression on the remaining data to identify the lower (high frequency) breakpoint (Serran and Creed, 2016; Serran et al., 2018). Wetland loss (number and area) is estimated by the area between the power-law function extrapolated below the high-frequency breakpoint to the minimum mapping unit and the observed function below this breakpoint.
The relationship between the area and frequency of wetlands was conducted on a contemporary wetland inventory. The contemporary wetland inventory (ca. 2015) was taken from the SOLRIS Version 3.0 land use inventory derived from Landsat satellite images and provided at a 15-meter grid resolution (Ontario Ministry of Natural Resources and Forestry [OMNRF], 2019). Wetland types [swamp (treed and thicket swamps), marsh, bog, and fen] were extracted and contiguous grid cells classified as marsh and classified as any wetland type were separately converted to vector polygon marsh and wetland features, respectively. Marsh and wetland features with areas less than the reported minimum mapping unit of 0.5 ha were considered unreliable and removed. An area bin size equal to the input grid size of the contemporary wetland inventory (15 m × 15 m = 0.0225 ha) was used to calculate the frequency of marsh and wetland sizes. The analysis was performed for: (1) all wetlands up to a maximum of 100 ha; and (2) geographically isolated marshes, where isolation was established by the lack of intersection of a marsh to the surface drainage network (using the Ontario Hydro Network (OHN) – Watercourse database; Ontario Ministry of Natural Resources and Forestry [OMNRF], 2022). All GIS procedures were performed in ArcGIS 10.8.
Organic Carbon Stocks and Sequestration Rates in Restored Wetlands
Wetland Core Collection
Fifteen wetlands within the Fairchild Creek watershed near Onondaga (Figure 2) were selected for the assessment of OC stocks and sequestration rates as affected by drainage. Some of these wetlands were assumed to have never been drained, reflecting an apex natural state (Intact wetlands, OI) (average area = 0.63 ha). Some were assumed to have been drained for many decades (artificial drainage to support agricultural land use), reflecting an apex drained state (Drained wetlands, OD) (average area = 0.45 ha). The remaining wetlands were assumed to have been drained for many decades and then hydrologically restored by blocking the artificial drainage 15 years (OR15) (average area = 1.08 ha), 25 years (OR25) (average area = 1.16 ha), or 40 years (OR40) (average area = 3.73 ha) prior to the collection of sediment cores in the summer of 2018.
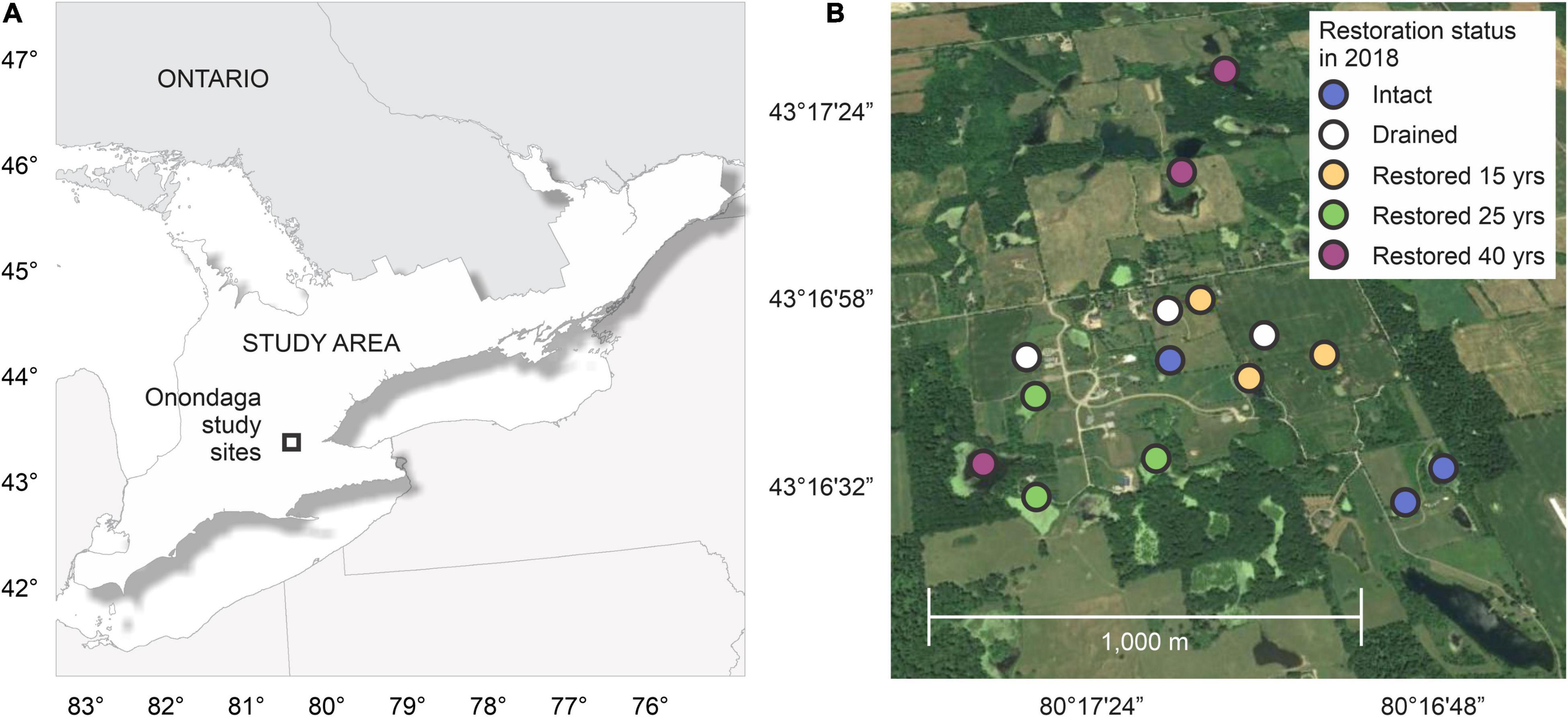
Figure 2. Study area: (A) Location of Onondaga study sites. (B) Distribution of Onondaga study sites within the Fairchild Creek watershed.
The impact of wetland restoration on OC stocks was examined by analyzing three sediment cores collected from the open-water area of each wetland. The three cores were distributed throughout the central area of the open-water area, capturing the variability in each wetland’s morphometry in the deeper areas of water (wetlands often consist of more than one sub-basin within the open-water area). Each core extended from the sediment surface down as deep as 100 cm to the underlying undeveloped geologic material, the soil parent material, capturing the full depth of organic-rich material. Each core was segmented into 1-, 2-, or 5-cm deep samples; small segments were used for shallow cores and larger segments were used for deeper cores to optimize analytical throughput and to provide adequate detail for interpretation of the core data. A Watermark Universal Corer with a polycarbonate tube (internal diameter (i.d.) = 6.8 cm) was used to collect cores that were wet and loose, and a JMP BackSaver Soil sampler (i.d. = 3.175) cm was used to collect dry cores in the drained wetlands.
Wetland Sediment Mass
The total sediment mass and total OC content was estimated for each core segment. Samples were air-dried, disaggregated using a 2-mm sieve, coarse fragments (>2 mm) were removed, and the dry masses of the fine and coarse fractions were weighed and recorded. The sample volume (product of core diameter and sample increment depth) and mass were used to calculate bulk density over the depth of the sediment cores. Coarse fragments were rarely observed within any of the wetland cores, and where they were observed, they were usually confined to a layer and were used as an indicator of a high-energy water erosion event on the surrounding land or of a disturbance event within the wetland catchment. Samples were analyzed for OC concentration using combustion in a muffle furnace (Fisher Scientific Thermolyne Largest Tabletop Muffle Furnace set at 400°C and run for 16 h). These OC concentrations and the sample bulk densities were used to calculate the OC mass over the depth of the sediment cores.
Wetland Organic Carbon Stocks and Sequestration Rates
To estimate contemporary OC stocks and sequestration rates (Mg C ha–1 yr–1), fallout radioisotopes 137Cs and 210Pb were used (Kamula et al., 2017). 137Cs provides two time-markers within sediment cores: (1) the onset of 137Cs atmospheric deposition occurred in approximately 1954; and (2) the peak atmospheric deposition of 137Cs occurred between 1958 and 1963. Since the cessation of atmospheric testing of thermonuclear weapons, the atmospheric deposition of 137Cs has greatly diminished. In sediment cores where these two time-markers were clearly observed, we were able to estimate the recently accrued stock of OC above these markers (Supplementary Table 1). We calculated the rates of sediment accumulation and OC sequestration for each core using the time frame between the year of sample collection (2018) and the year of these time markers. This was done for all study wetlands, including OD, OI, OR15, OR25, and OR40.
For OR15, OR25, and OR40, the time frame between the year of sample collection (2018) and the 1954 and 1963 137Cs time-markers represents a mix of a drained state and a restored state (e.g., for the 55-year time frame between 1963 and 2018, 15 years would be in a restored state and 40 years in a drained state in a OR15 wetland). As such, there is no clear effect of restoration on the contemporary OC stock or on the sequestration of OC. In contrast, 210Pb is continuously produced through radioactive decay at the land surface and released into the atmosphere. As with 137Cs, 210Pb is deposited on the soil/sediment surface where it binds to the mineral and organic material and acts as a tracer for soil/sediment loss or accumulation. The diminishing concentration of 210Pb with depth in the sediment profile was used with a Continuous Rate of Supply (CRS) model to estimate the accumulation of sediment (Kamula et al., 2017) and, therefore, OC sequestration over time (Supplementary Table 1). The accuracy of these estimates is a function of the number of data points available for this modeling; the estimate of OC sequestration for OR40 is more accurate than the estimate for OR25, which in turn is more accurate than for OR15. 210Pb sequestration rates of all drainage practices back to 1954 and 1963 were compared to those estimated using 137Cs to provide a measure of confidence in the use of these fallout radioisotope techniques to estimate sediment accumulation and OC sequestration (Supplementary Figures 2, 3).
The OC stock within a wetland was estimated for each core as the cumulative sum of OC (g m–2) measurements from the surface sample down to the sample depth of interest (e.g., for the 15-year restoration age, all the OC measurements in the core at and above the sample dated at 15 years were summed to determine the stock value). Unit conversion was then applied to convert from g m–2 to Mg ha–1.
The OC sequestration rates (g m–2 yr–1) were estimated by dividing the OC stocks (g m–2) by the number of years in question (e.g., for the 15-year restoration age, the stock of OC accumulated over 15 years was divided by 15 years). Unit conversion was then applied to convert from g m–2 yr–1 to Mg ha–1 yr–1.
Results
Restorable Wetlands
Based on our analyses of the power-law functions, there was an estimated loss of 65,261 wetlands (31.1% of the estimated 209,254 total historical wetland number) in the study area, comprising a loss of 63,135 ha (8.3% of the estimated 761,816 ha total historical wetland area) (Figure 3A). Based on the additional criteria of “easy-to-restore” wetlands, there was an estimated loss of 6,899 geographically isolated marshes (35.3% of the estimated 19,546 total historical geographically isolated marsh number and 10.6% of all lost wetlands) comprising a loss of 5,198 ha (14.9% of the estimated 35,002 ha total historical geographically isolated marsh area and 8.2% of all lost wetland area) (Figure 3B). A high-frequency breakpoint of 2.07 ha was identified in the two-segment piecewise linear regression of geographically isolated marsh sizes below the low-frequency breakpoint, denoting the maximum size below which the size-frequency distribution of geographically isolated marshes deviated from the “natural distribution” (i.e., the maximum size of lost and therefore restorable geographically isolated marshes).
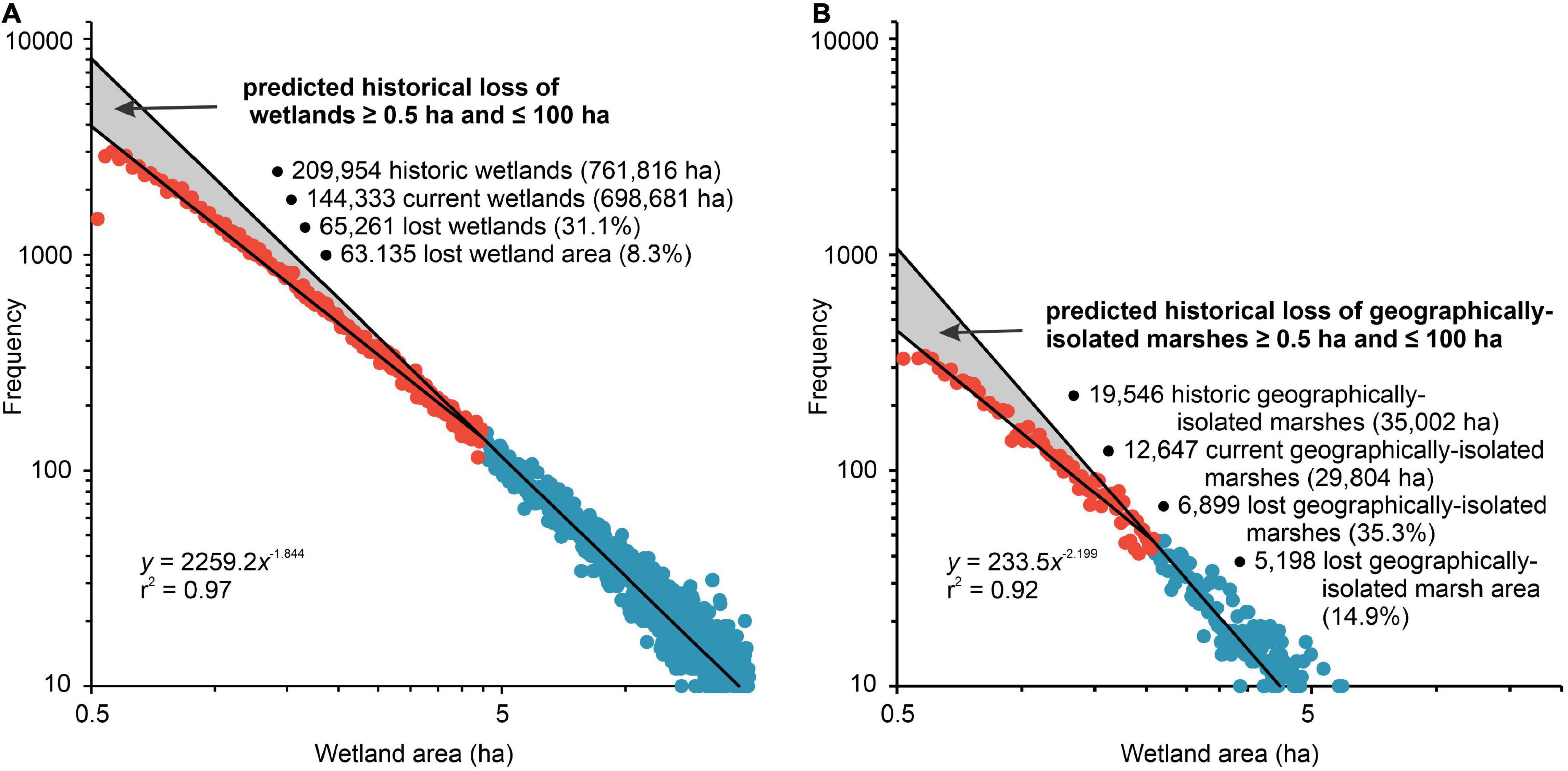
Figure 3. Relationships between area and frequency of wetlands with power-law function trendlines above (“natural distribution” in blue) and below (distribution of wetlands where loss has occurred in red) high frequency breakpoints [gray points represent wetlands above an upper (low frequency) breakpoint in which there are few or no frequency data (these data are not included in the calculation of the natural distribution trendline)]. (A) A total of 63,531 of the 209,954 (31.1%) historic wetland numbers have been lost, representing a total area of 63,135 of the 761,816 ha (8.3%) historic wetland area. (B) For the “easy-to-restore” wetlands, a total number of 6,899 of the 19,546 (35.3%) historic geographically isolated marsh wetlands have been lost comprising 5,198 of 35,002 ha (14.9%) historic geographically isolated wetland area, representing 10.6 and 8.2% of total lost wetland numbers and area, respectively (Data source: Ontario Ministry of Natural Resources and Forestry [OMNRF], 2019).
Organic Carbon Sequestration Rates and Stocks in Restored Wetlands
Core depths varied up to 100 cm, with the depths cored capturing the OC profile up to 1% OC content (Table 1; e.g., Supplementary Figure 1). In drained wetlands, sediment accumulation rates averaged 15.03 Mg ha–1 yr–1, more than double the rates in any of the restored wetlands (i.e., OR15 = 6.29 Mg ha–1 yr–1, OR25 = 2.67 Mg ha–1 yr–1, OR40 = 3.11 Mg ha–1 yr–1, OI = 5.71 Mg ha–1 yr–1) (Supplementary Figure 2). In contrast, OC sequestration rates averaged 0.47 Mg C ha–1 yr–1 in drained wetlands, lower than the rates in the restored wetlands (Table 1 and Supplementary Figure 2). Restored wetlands showed an increase in both OC sequestration stocks and rates with age since restoration; specifically, wetlands sequestered 7.65 Mg C ha–1 OC at an average rate of 0.51 Mg C ha–1 yr–1 after 15 years since restoration, 16.67 Mg C ha–1 at an average rate of 0.67 Mg C ha–1 yr–1 after 25 years, and 35.60 Mg C ha–1 at an average rate of 0.89 Mg C ha–1 yr–1 after 40 years (Table 1 and Figure 4). Given that sediment accumulation varied across the wetland ages, the ratio of OC mass to sediment mass was examined. Here, drained wetlands had less OC per unit mass of sediment than restored wetlands. Compared to the drained wetlands, there was a 4% increase in the ratio of OC to sediment masses after 15 years since restoration, a 21% increase after 25 years, and a 25% increase after 40 years. The rate of increase in the ratio was small over the first 15 years (4%), increased substantially over the next 10 years (17%), and then continued to increase but at a smaller rate over the next 15 years (4%; i.e., from 21% for OR25 wetlands to 25% for OR40 wetlands) (Figure 5). OC stocks and sequestration rates were higher in the OI wetlands than those in OD, OR15, and OR25 wetlands but were comparable to if not marginally smaller than those in OR40 wetlands. For further details on 137Cs and 210Pb data, see Supplementary Table 1.
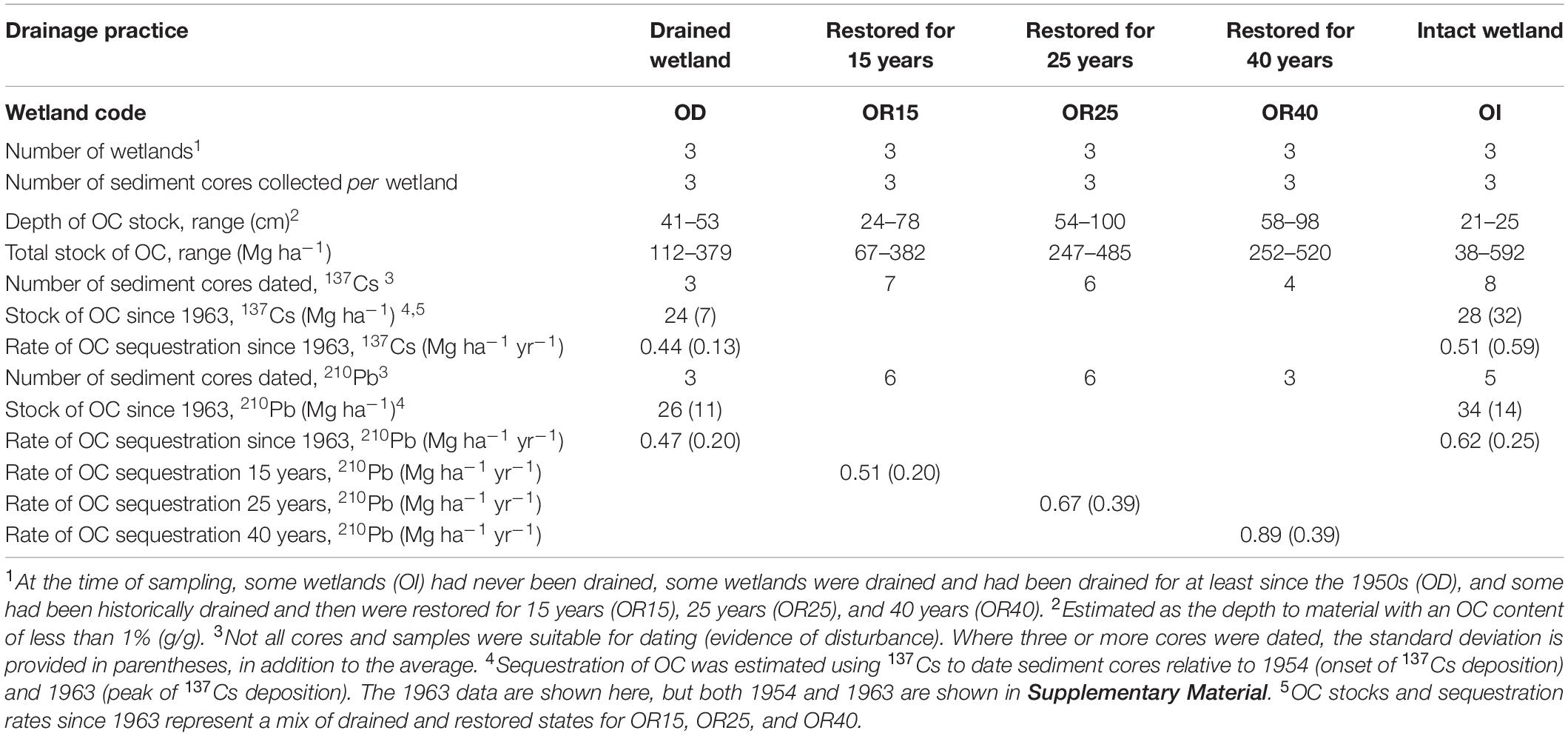
Table 1. Wetland organic carbon (OC) stocks and sequestration rates estimated from sediment cores and using fallout radionuclides for dating.
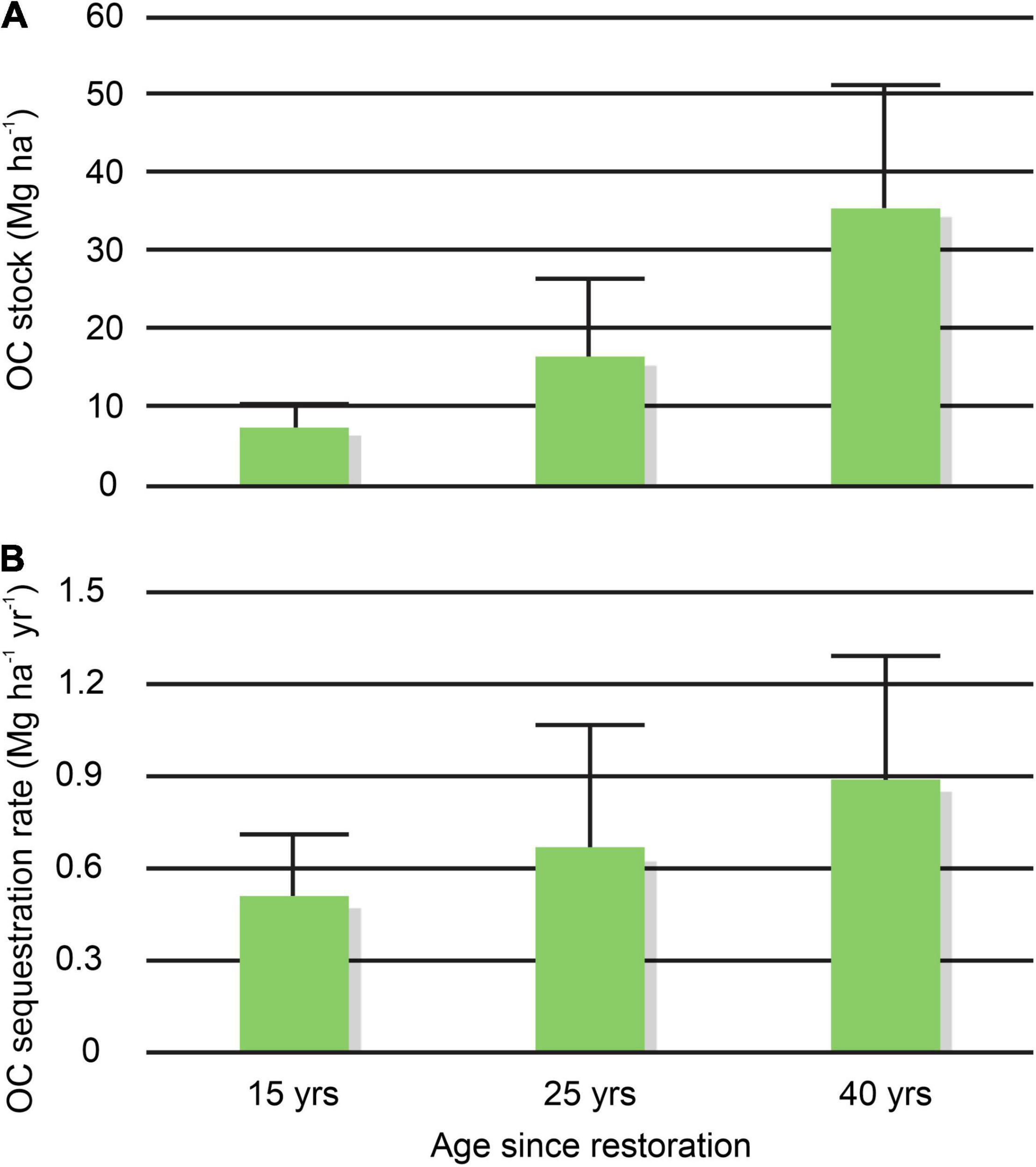
Figure 4. (A) OC stocks and (B) sequestration rates for restored wetlands after 15, 25, and 40 years based on 210Pb dating. Error bars represent standard deviations.
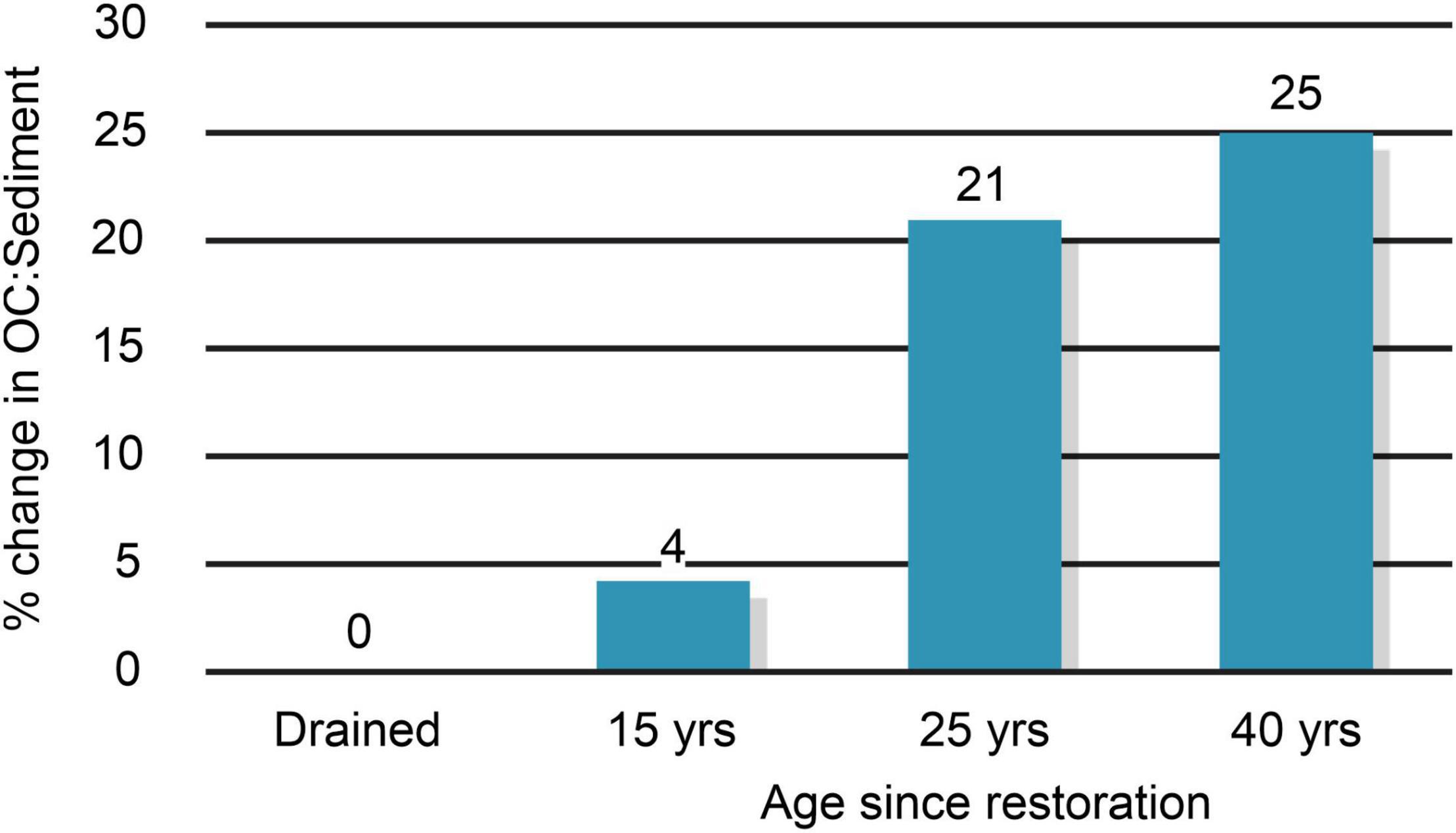
Figure 5. Percent change in the ratio of OC to sediment stocks for drained sites and restored wetlands after 15, 25, and 40 years.
Discussion
Humans have been draining and then converting wetlands for centuries, and this loss of wetlands continues (Davidson, 2014; Gardner and Finlayson, 2018). To our knowledge, this study provides the first estimates of OC sequestration rates in easy-to-restore restored freshwater mineral soil wetlands spanning a chronosequence from recently drained through restored to intact. This information, in combination with the economic assessment that follows, are important steps forward in assessing how to target wetland conservation and restoration for carbon storage. In turn, this information will help guide future research in this field, with the goal of developing wetland management programs that maximize environmental benefits while being cost-effective and providing additional revenue for agricultural producers.
Preferential Loss of Easy-to-Restore Freshwater Mineral Soil Wetlands
The restoration potential of easy-to-restore wetlands is substantial in the study area. The magnitude of total wetland area loss is estimated to be over 1.2 million ha. This estimate is derived from the difference between the total area of all wetlands (1,159,931 ha; Ontario Ministry of Natural Resources and Forestry [OMNRF], 2019) and a pre-settlement (ca. 1800) wetland inventory (2,420,694 ha; Ducks Unlimited Canada [DUC], 2010, derived from 1:50,000 soil surveys). The percentage of number of lost wetlands is larger than the percentage of area of lost wetland (Figure 3). This can likely be attributed to the fact that smaller wetlands are more vulnerable to drainage than larger wetlands – the frequency of wetland sizes was found to increase between 0.5 and 0.5625 ha (Figure 3A), contrary to an expected natural distribution.
The estimates of the number and area of wetland loss using power-law function analysis are conservative because of three assumptions. First, the minimum mapping unit of the current wetland inventory was 0.5 ha; exclusion of wetlands < 0.5 ha resulted in under-estimation of total wetland loss. Second, wetlands > 100 ha were excluded from the power-law function analyses. However, drainage of much larger wetlands has occurred in the study area since settlement. In fact, the pre-settlement wetland inventory showed 2,270 wetlands > 100 ha with a total area of 1,942,140 ha – an area larger than the total area of all wetlands in the current wetland inventory (1,159,931 ha). These very large wetlands were drained in parts, leaving larger numbers of fragments or smaller wetlands – confirming a trend identified by several researchers toward homogenization of wetland sizes through preferential loss of both small and large wetlands (Van Meter and Basu, 2015; Waz and Creed, 2017; Serran et al., 2018). Exclusion of wetlands > 100 ha resulted in under-estimation of total wetland loss. Third, power-law functions are sensitive to the accuracy (number and area) of wetland inventories. The current wetland inventory was derived through spectral analysis of Landsat satellite images that were acquired on different dates on which different climate, soil, and vegetation conditions could be found (Ontario Ministry of Natural Resources and Forestry [OMNRF], 2019), which in turn can affect spectral differentiation of different wetland types. Varying over- or under-estimations of current wetlands in different areas of the inventory would have a spatial effect on the identification of low and high frequency breakpoints and, subsequently, the “natural distribution” trendlines, resulting in over- or under-estimations of wetland loss in different areas.
Organic Carbon Sequestration Rates and Organic Carbon Stocks Increase With Age Since Restoration
With a restoration potential of easy-to-restore wetlands of over 1.2 million ha, evidence is needed on the OC sequestration potential of these wetlands.
The rates of OC sequestration (0.51–0.89 Mg C ha–1 yr–1) that we measured in the easy-to-restore wetlands were within the range of historic (0.51 Mg C ha–1 yr–1) and contemporary (1.55 Mg C ha–1 yr–1) rates reported by Loder and Finkelstein (2020).
There was heterogeneity in OC sequestration rates within and among wetlands of a given age since restoration, but a pattern emerged where the rate of sediment accumulation decreased while both the OC sequestration rates (Figure 4) and the ratio of OC to sediment masses (Figure 5) increased with age since restoration. This pattern may indicate a shift from external carbon loading (e.g., wind or water erosion from uplands to the wetland) toward internal carbon sequestration over time. This is important as it has been suggested that the redistribution of eroded soils from agricultural landscapes into wetlands may represent a significant terrestrial carbon sink (McCarty and Ritchie, 2002). In our study, wetlands were restored by simply plugging the shallow surface drainage ditch, which is a common method used to restore freshwater mineral soil wetlands (Craft, 2016). No additional measures were taken to reduce or prevent sediment loading from the surrounding uplands to the wetland. This may have contributed to the heterogeneity of OC sequestration rates. If efforts were made to filter sediment input, then the signal of the OC sequestration rate based on processes internal to the wetland could have been amplified. Alternatively, this pattern may indicate a lag between restoration and resumption of hydrological, biogeochemical, and ecological processes within the restored wetland. Drainage and restoration of wetlands alter carbon cycling, and wetlands typically undergo a recovery phase before they reach stable carbon sequestration rates (Valach et al., 2021). For example, wetland flora and fauna take time to recover from restoration before starting to sequester more carbon (Tangen and Bansal, 2020). Our data suggest that restoration promotes OC sequestration but that the restored wetlands need to go through an initial recovery phase (up to 25 + years) before fully recovering and even marginally surpassing OC sequestration rates in intact wetlands before settling into a new equilibrium.
Using Science to Better Understand the Economics of Wetland Carbon Markets
With 1.2 million ha of easy-to-restore wetlands that had relatively large (but variable) OC sequestration rates, we conducted a cost-benefit analysis of restoring these wetlands.
Wetland costs (both from avoided conversion and restoration) and associated carbon benefits were estimated using the OC sequestration rates derived in this paper to assess the feasibility of wetland carbon markets to promote their conservation and restoration using the following steps:
1. Select OC sequestration rate. There were no statistically significant differences in OC sequestration rates among OR15, OR25, and OR40 wetlands (Supplementary Table 1; p = 0.716 in a one-way analysis of variance test performed in SigmaPlot 12.0). We chose the rate for OR40 wetlands because we assumed that it was the “cleanest” rate (i.e., smallest sediment accumulation rate), the most robust rate (i.e., it would have reflected inter-annual variability in rates over multiple decades), and the most similar rate to intact wetlands. This average OC sequestration rate was 0.89 Mg C ha–1 yr–1 (Supplementary Table 1); based on this, the OC stock replenished by restored wetlands was 36 Mg C ha–1. At an average sequestration rate of 0.89 Mg C ha–1 yr–1 over the 40 years since restoration, the accrual of OC stock would equal 35.6 Mg C ha–1.
2. Select time frame for sequestering OC. We estimated the change in cumulative OC stock over a 10-year time frame (2021 to 2030) and a 30-year time frame (2021 to 2050). We chose 2030 as Canada has committed to reduce GHG emissions by 30% below 2005 levels by 2030 (Drever et al., 2021), and we chose 2050 as Canada has committed to net-zero emissions by 2050 (Environment and Climate Change Canada [ECCC], 2020a).
3. Determine the cost of restoring the wetland. Costs of wetland restoration include the direct restoration costs (e.g., cost of plugging the drain) and the opportunity costs associated with alternative uses of the land (e.g., cost of lost crop yield). Just as there is heterogeneity in OC sequestration rates across wetlands, there is heterogeneity in restoration costs of wetlands across landscapes. Direct restoration costs vary by wetland depending on factors such as local topography, the current state of the drained wetland, and the current value of the drained land. We used a restoration cost of $31,000 CAD ha–1 based on past wetland restoration projects in Ontario by Ducks Unlimited Canada [DUC] (unpublished). We assumed that drained wetlands were used for agricultural production and therefore estimated the opportunity costs of the drained wetland using annual cash rental rates for agricultural land. Deaton (2022) reported median cash rents for farmland in Ontario in 2021 of $124 to $741 CAD ha–1; we used the $432 CAD ha–1 midpoint of this range.
Several economic analyses were conducted using discounting to convert the ongoing potential costs and benefits over time into common monetary units.
We reported a cost-effectiveness metric by calculating the cost per Mg CO2-eq sequestered over a 10- and 30-year time frames. Using the average annual OC sequestration rate for OR40 wetlands (0.89 Mg C ha–1 yr–1; Supplementary Table 1) to estimate the OC stock for the specific time frames; we converted the annual OC sequestration rate from Mg C ha–1 yr–1 to Mg CO2-eq ha–1 yr–1 by multiplying Mg C by the molecular weight of CO2 (44 g) and then dividing by the atomic weight of carbon (12 g) (i.e., 0.89 Mg C ha–1 yr–1 = 2.90 Mg CO2-eq ha–1 yr–1). We calculated the present value of costs using a combination of the 3% discount rate (Treasury Board of Canada Secretariat [TBCS], 2007), the one-time $31,000 CAD ha–1 cost of wetland restoration in Ontario, and the ongoing annual $432 CAD ha–1 cost of agricultural rents in Ontario. We estimated the cost per Mg CO2-eq sequestered in restored wetlands to be $1,061 CAD and $402 CAD using the 10-year and 30-year time frames, respectively (Table 2). When we considered wetlands at risk of drainage that can be retained without expending the direct restoration cost, the cost per Mg CO2-eq sequestered decreased to $113 CAD and $86 CAD using the 10-year and 30-year time frames, respectively (Table 2). These calculations did not include other factors that could impact the costs per Mg CO2-eq including agricultural soil sequestration (↑ $ Mg CO2-eq–1) if the wetland is not restored and agricultural production continues, avoided agricultural emissions (↓ $ Mg CO2-eq–1) by converting agricultural land to wetland, potential methane emissions from wetlands (↑ $Mg CO2-eq–1), and co-benefits from other wetlands services (↓ $ Mg CO2-eq–1).

Table 2. Costs and benefits to sequester CO2 in wetlands in Southern Ontario over 10- and 30-year time frame in 2021 $ CAD.
The cost per Mg CO2-eq sequestered was compared to the monetary benefits using Canada’s carbon price schedule as determined by the federal government. We used a carbon price approach to valuation rather than using a social cost of carbon approach because the carbon price is closer to what a landowner would receive for carbon sequestration in any market. The price of carbon was $40 CAD Mg CO2-eq–1 in 2021 and $50 CAD Mg CO2-eq–1 in 2022. In 2021, Canada confirmed that the minimum nominal price on carbon pollution would increase by $15 CAD Mg CO2-eq–1 yr–1 from 2023 to 2030, with a projected nominal price of $170 CAD Mg CO2-eq–1 in 2030 (Environment and Climate Change Canada [ECCC], 2020b). If that rate of price increase were to continue, this would result in a projected nominal price of $470 CAD Mg CO2-eq–1 in 2050. The benefit-cost analysis revealed that while a financial incentive from carbon payments alone exists for the retention of wetlands, the financial incentive from carbon payments would be insufficient to cover the cost of restoration for most wetlands. For example, while the monetary benefits are greater than the costs from CO2 sequestration over a 30-year time frame for retaining wetlands (i.e., benefit to cost ratio = 1.23), the high cost of restoration results in ratios < 1; Table 2. If the conservation of existing wetlands is economically attractive as a strategy for wetlands as NbCS, then we must determine which existing wetlands are at risk of drainage and which ones are likely permanent fixtures on the landscape. Carbon payments made for existing wetlands that are not at risk of drainage likely do not result in any additional carbon reductions.
4. Breakeven costs. Due to the substantial heterogeneity of wetland conservation and restoration costs, we also calculated the breakeven costs (Table 2). The breakeven cost level is equal to the carbon benefits of the project such that wetland conservation or restoration is economically feasible based on current carbon price projections (Boardman et al., 2018). The present value of carbon benefits is estimated to be $10,452 CAD ha–1 over the 30-year time frame; any wetland conservation or restoration project that costs this amount or less could be justified on economic grounds based solely on the carbon benefits.
If we were to scale up our economic analysis to the regional scale, with 1.2 million hectares of easy-to-restore wetlands available, a total of 156,640,000 Mg-CO2 eq would be sequestered at a cost of $37.22 trillion dollars (calculations based on the wetland restored 40 years ago carbon stock of 35.60 Mg ha–1 or annual rate of 0.89 Mg ha–1 yr–1 and a restoration cost of $31,000 ha–1).
Restoring Wetlands as Nature-Based Climate Solutions: Scientific Challenges and Opportunities
The successful use of wetlands as NbCS requires establishing their carbon benefits as a basis for wetland payment programs. However, more research is needed to reduce uncertainty in estimates of carbon stocks and sequestration rates by sampling additional wetlands and factoring in the landscape matrix which determines the potential carbon loading to the wetlands. Currently, carbon payment programs often reduce payments to account for the large uncertainty in carbon sequestration rates (Angelsen, 2017; Yanai et al., 2020) as well as to address the risk of reversal or failure associated with NbCS projects. Further, carbon discount rates have been included in carbon payment programs to account for the urgency placed on reducing carbon emissions (Pearce, 2003). The current analysis uses a constant sequestration rate per year and an improved understanding of the time-varying dynamics of carbon sequestration rates in wetlands can inform the appropriate carbon discount rate that could be applied for these projects.
More research is also needed on how to target wetland management efforts in areas where CO2 sinks are maximized and CH4 sources are minimized. Wetlands can be a net carbon sink while having a net warming effect at the 100-year time-frame (Taillardat et al., 2020). This situation arises due to the favorable conditions for methane production in wetlands and its higher global warming potential in shorter timeframes relative to CO2. The balance between acting as a carbon sink while contributing to net warming can be further complicated by lateral flows, particularly in flow-through wetland systems (Chu et al., 2015). In order to tackle this situation, wetland managers could: (1) target restoration efforts in areas rich in alternative electron acceptor pools like sulfur- or iron-rich soils that inhibit CH4 fluxes by preferential sulfate or iron reduction or occasionally lower water tables to reduce CH4 production (Hemes et al., 2018); (2) plant species around the wetland that limit agricultural runoff into wetlands (Pasut et al., 2021), given the evidence that elevated nutrient levels in wetlands can lead to higher GHG emissions (Bridgham et al., 2013; Beaulieu et al., 2019); (3) plant specific species of emergent vegetation, given the evidence that GHG emissions vary substantially across different species of emergent vegetation (Laanbroek, 2010; Lawrence et al., 2017; Villa et al., 2020); (4) cut or graze plants to reduce GHG emissions (Herbst et al., 2013), although cutting at the wrong time or overgrazing can lead to greater GHG emissions; and (5) amend soils/sediments to reduce GHG emissions (e.g., Ali et al., 2007; Han et al., 2016). More research is also needed on the GWP used for methane emissions. Our study used the standard GWP100 recommended by the International Panel on Climate Change (IPCC); however, there is a growing body of evidence that demonstrates that this metric is not ideal for modeling actual global temperature change (Allen et al., 2016). GWP* is an alternate to GWP100 that better captures the different behaviors of long-lived climate pollutants like CO2 and short-lived climate pollutants like CH4 (Cain et al., 2019), and may be important to consider in assessing the climate impacts of using wetlands as NbCS given that these systems can be important sources of CH4.
The successful use of wetlands as NbCS also requires exploring the feasibility of stacking the multiple benefits of restored wetlands and incorporating them into wetland payment programs. This stacking of multiple benefits must consider the different ways that wetlands act as climate solutions, including their cooling effect (Zhang et al., 2022). It must also consider the other-than-climate benefits of wetlands, including flood/drought mitigation, water purification, and biodiversity (Cohen et al., 2016). While markets focused on wetland conservation and restoration for carbon alone may not currently be viable, markets that stack multiple benefits may be (Creed et al., 2017) [e.g., a recent study estimated the flooding damages avoided by wetlands in the United States to be $1,400 USD ha–1 yr–1 with substantially larger values near developed areas (Taylor and Druckenmiller, 2022)].
Stacking ecosystem services can provide financial incentives that may be more effective in achieving broad conservation goals, including enhancing fish and wildlife habitat, improving watershed health. sequestering carbon to mitigate climate change and providing ecosystem services at ecologically relevant scales (Deal et al., 2012). However, stacking of ecosystems services needs to be approached with caution as it can lead to net ecosystem service losses if not properly implemented due to the interdependence of many ecosystem functions (Robertson et al., 2014). Since wetlands are one of the most threatened ecosystems on the planet (Gardner and Finlayson, 2018), we should follow a hierarchy of protect (avoid conversion), manage, and then restore (Cook-Patton et al., 2021). According to Drever et al. (2021), GHG reductions through avoided conversion of wetlands can be achieved for $50 CAD Mg CO2-eq–1 ($13.62 CAD Mg C–1) or less, while those reductions through restoration of wetlands is significantly more costly [$300 CAD Mg CO2-eq –1 ($81.74 CAD Mg C –1)], and our results support these findings. Given the cost differential between avoided conversion and restoration of wetlands, the ongoing loss of wetlands must be addressed. This will be challenging as there is a preference for implementing restoration projects as opposed to avoided conversion projects, as highlighted in the Government of Canada’s recent investment in the Nature Smart Climate Solutions Fund where approximately 80% of the program funding is directed to restoration activities (Environment and Climate Change Canada [ECCC], 2021). Finding ways to incentivize agricultural landowners to maintain the remaining intact wetlands on their landscapes – such as through markets – will be paramount to helping the agricultural sector meet its climate mitigation targets.
Conclusion
The conservation and restoration of wetlands embedded in working agricultural landscapes is an important nature-based climate solution. This study provides scientific evidence that freshwater mineral soil wetlands sequester carbon but that the rates are highly variable. Despite the variability of rate estimates, the benefit-cost analysis indicates that the use of wetlands as nature-based climate mitigation strategies must focus both on their conservation and restoration. Over a 30-year time frame, the avoided emissions associated with the protection of existing wetlands offset the cost of conservation, but the cost of wetland restoration cannot currently be recovered on a carbon basis alone over the same time frame. While the protection of wetlands leads to immediate benefits, restoration of wetlands is a longer-term investment requiring 30 + years to achieve benefits based on the current (and predicted) carbon values. However, if the multiple benefits of wetland ecosystem services are stacked (i.e., considering the benefits of wetlands for carbon sequestration but also the benefits of wetlands for flood and drought mitigation, water purification, and biodiversity enhancement) including the non-tangible aesthetic value, then the immediate benefits of both protected and restored wetlands are compelling. This is of particular importance given that many of these co-benefits help mitigate the impacts of climate change such as increased eutrophication and biodiversity loss. A limitation of this study is that it covers a relatively narrow portion of the climate gradient where freshwater mineral wetlands exist. Future work is focused on extending the geographic scope to include a more complete climate gradient where freshwater mineral soil wetlands exist. Given that wetlands as nature-based climate solutions have the potential of becoming a viable market, policies and programs should be established to inform these markets and incentivize agricultural producers to adopt wetland conservation and restoration practices on marginal agricultural areas to improve the economic performance and environmental sustainability of agriculture.
Data Availability Statement
The raw data supporting the conclusions of this article will be made available by the authors, without undue reservation.
Author Contributions
MG conceived the study. IC and PB designed the study. IC, DL, and EE implemented the study. JP-W and PL-S conducted the economic analyses. All authors contributed to the writing of the manuscript.
Funding
This work was supported by a Strategic Partnership for Projects Grant to IC and DL from the Natural Sciences and Engineering Research Council of Canada (STPGP 506809), and a grant to IC and PB from Environment and Climate Change Canada (GCXE21S045).
Conflict of Interest
The authors declare that the research was conducted in the absence of any commercial or financial relationships that could be construed as a potential conflict of interest.
Publisher’s Note
All claims expressed in this article are solely those of the authors and do not necessarily represent those of their affiliated organizations, or those of the publisher, the editors and the reviewers. Any product that may be evaluated in this article, or claim that may be made by its manufacturer, is not guaranteed or endorsed by the publisher.
Acknowledgments
We thank David Aldred for his excellent assistance in generating the figures and in editing the manuscript. We also thank the many field and laboratory assistants (Eva Slavicek, Anthony Buckley, Nicole Larsen, Aleksey Paltsev, Michael Steiff, Nico Trick, Naomi Trick, and Tong Zou). We would like to thank Dave McLachlin for assistance in selecting the experimental wetlands and providing data and access to these wetlands.
Supplementary Material
The Supplementary Material for this article can be found online at: https://www.frontiersin.org/articles/10.3389/fevo.2022.932415/full#supplementary-material
References
Ali, M. A., Lee, C. H., and Kim, P. J. (2007). Effect of Phospho-gypsum on reduction of methane emission from rice paddy soil. Korean J. Environ. Agric. 26, 131–140. doi: 10.5338/KJEA.2007.26.2.131
Allen, M. R., Fuglestvedt, J. S., Shine, K. P., Reisinger, A., Pierrehumbert, R. T., and Forster, P. M. (2016). New use of global warming potentials to compare cumulative and short-lived climate pollutants. Nat. Clim. Change 6, 773–776. doi: 10.1038/nclimate2998
Angelsen, A. (2017). REDD+ as result-based aid: general lessons and bilateral agreements of Norway. Rev. Dev. Econ. 21, 237–264. doi: 10.1111/rode.12271
Asare, E., Mantyka-Pringle, C., Anderson, E., Belcher, K., and Clark, R. (2022). Evaluating ecosystem services for agricultural wetlands: a systematic review and meta-analysis. Wetl. Ecol. Manage. 2022, 1–21. doi: 10.1007/s11273-022-09857-5
Bartzen, B. A., Dufour, K. W., Clark, R. G., and Caswell, F. D. (2010). Trends in agricultural impact and recovery of wetlands in prairie Canada. Ecol. Appl. 20, 525–538. doi: 10.1890/08-1650.1
Beaulieu, J. J., DelSontro, T., and Downing, J. A. (2019). Eutrophication will increase methane emissions from lakes and impoundments during the 21st century. Nat. Commun. 10:1375. doi: 10.1038/s41467-019-09100-5
Boardman, A. E., Greenberg, D. H., Vining, A. R., and Weimer, D. L. (2018). Cost-Benefit Analysis: Concepts and Practice, 5th Edn. Cambridge: Cambridge University Press. doi: 10.1017/9781108235594
Bridgham, S. D., Cadillo-Quiroz, H., Keller, J. K., and Zhuang, Q. (2013). Methane emissions from wetlands: biogeochemical, microbial, and modeling perspectives from local to global scales. Glob. Change Biol. 19, 1325–1346. doi: 10.1111/gcb.12131
Brown, J. H., Gupta, V. K., Li, B. L., Milne, B. T., Resterpo, C., and West, G. B. (2002). The fractal nature of nature: power-laws, ecological complexity and biodiversity. Philos. Trans. R. Soc. Lond. B. Biol. Sci. 357, 619–626. doi: 10.1098/rstb.2001.0993
Byun, E., Finkelstein, S. A., Cowling, S. A., and Badiou, P. (2018). Potential carbon loss associated with post-settlement wetland conversion in southern Ontario, Canada. Carbon Balance Manage. 13:6. doi: 10.1186/s13021-018-0094-4
Cain, M., Lynch, J., Allen, M. R., Fuglestvedt, J. S., Frame, D. J., and Macey, A. H. (2019). Improved calculation of warming-equivalent emissions for short-lived climate pollutants. NPJ Clim. Atmos. Sci. 2:29. doi: 10.1038/s41612-019-0086-4
Canadian Forest Service [CFS] (2020). Historical Monthly Climate Grids For North America. Available online at: https://cfs.nrcan.gc.ca/projects/3/3 (accessed February 17, 2022).
Chu, H., Gottgens, J. F., Chen, J., Sun, G., Desai, A. R., Ouyang, Z., et al. (2015). Climatic variability, hydrologic anomaly, and methane emission can turn productive freshwater marshes into net carbon sources. Glob. Change Biol. 21, 1165–1181. doi: 10.1111/gcb.12760
Cohen, M. J., Creed, I. F., Alexander, L., Basu, N., Calhoun, A., Craft, C., et al. (2016). Do geographically isolated wetlands influence landscape functions? Proc. Natl. Acad. Sci. U.S.A. 113, 1978–1986. doi: 10.1073/pnas.1512650113
Cohen-Shacham, E., Walters, G., Janzen, C., and Maginnis, S. (eds) (2016). Nature-based Solutions to Address Global Societal Challenges. Gland: IUCN. doi: 10.2305/IUCN.CH.2016.13.en
Cook-Patton, S. C., Drever, C. R., Griscom, B. W., Hamrick, K., Hardman, H., Kroeger, T., et al. (2021). Protect, manage and then restore lands for climate mitigation. Nat. Clim. Change 11, 1027–1034. doi: 10.1038/s41558-021-01198-0
Creed, I. F., Lane, C. R., Serran, J. N., Alexander, L., Basu, N. B., Calhoun, A., et al. (2017). Enhancing protection for vulnerable waters. Nat. Geosci. 10, 809–815. doi: 10.1038/ngeo3041
Dahl, T. E., and Watmough, M. D. (2007). Current approaches to wetland status and trends monitoring in prairie Canada and the continental United States of America. Can. J. Remote Sens. 33, S17–27. doi: 10.5589/m07-050
Davidson, N. C. (2014). How much wetland has the world lost? Long-term and recent trends in global wetland area. Mar. Freshw. Res. 65, 934–941. doi: 10.1071/MF14173
Davidson, N. C., Van Dam, A. A., Finlayson, C. M., and McInnes, R. J. (2019). Worth of wetlands: revised global monetary values of coastal and inland wetland ecosystem services. Mar. Freshw. Res. 70, 1189–1194. doi: 10.1071/MF18391
de Groot, R., Brander, L., Van Der Ploeg, S., Costanza, R., Bernard, F., and Braat, L. (2012). Global estimates of the value of ecosystems and their services in monetary units. Ecosys. Serv. 1, 50–61. doi: 10.1016/j.ecoser.2012.07.005
Deal, R. L., Cochran, B., and LaRocco, G. (2012). Bundling of ecosystem services to increase forestland value and enhance sustainable forest management. Forest Policy Econ. 17, 69–76. doi: 10.1016/j.forpol.2011.12.007
Deaton, B. (2022). 2021 Farmland Value and Rental Value Survey. Department of Food, Agricultural and Resource Economics Report. Available online at: https://www.onfarmlandsurvey.com/ (accessed April 21, 2022).
Drever, C. R., Cook-Patton, S. C., Akhter, F., Badiou, P. H., Chmura, G. L., Davidson, S. J., et al. (2021). Natural climate solutions for Canada. Sci. Adv. 7:eabd6034. doi: 10.1126/sciadv.abd6034
Ducks Unlimited Canada [DUC] (2010). Southern Ontario Wetland Conversion Analysis. Final Report. (unpublished data). Available online at: https://uwaterloo.ca/ecohydrology/sites/ca.ecohydrology/files/uploads/files/ducks-unlimited-report.pdf (accessed February 17, 2022).
Environment and Climate Change Canada [ECCC] (2020a). Canadian Environmental Sustainability Indicators: Progress Towards Canada’s Greenhouse Gas Emissions Reduction Target. Available online at: https://www.canada.ca/content/dam/eccc/documents/pdf/cesindicators/progress-towards-canada-greenhouse-gas-reduction-target/2020/progress-ghg-emissions-reduction-target.pdf (accessed March 25, 2022).
Environment and Climate Change Canada [ECCC] (2020b). A Healthy Environment and a Healthy Economy. Available online at: https://www.canada.ca/content/dam/eccc/documents/pdf/climate-change/climate-plan/healthy_environment_healthy_economy_plan.pdf (accessed July 6, 2022).
Environment and Climate Change Canada [ECCC] (2021). Nature Smart Climate Solutions Fund. Available online at: https://www.canada.ca/en/environment-climate-change/services/environmental-funding/programs/nature-smart-climate-solutions-fund.html (accessed February 18, 2022).
Environment Canada (1991). The Federal Policy on Wetland Conservation. Ottawa, ON: Government of Canada.
Environment Canada (1995). National Ecological Framework for Canada. Available online at: https://sis.agr.gc.ca/cansis/nsdb/ecostrat/index.html (accessed March 11, 2022).
Gardner, R. C., and Finlayson, C. (2018). Global Wetland Outlook: State of the World’s Wetlands and Their Services to People. Gland: Ramsar Convention Secretariat.
Gillan, S. L., Koch, A., and Starks, L. T. (2021). Firms and social responsibility: a review of ESG and CSR research in corporate finance. J. Corp. Finance 66:101889. doi: 10.1016/j.jcorpfin.2021.101889
Girardin, C. A., Jenkins, S., Seddon, N., Allen, M., Lewis, S. L., Wheeler, C. E., et al. (2021). Nature-based solutions can help cool the planet – if we act now. Nature 593, 191–194. doi: 10.1038/d41586-021-01241-2
Golden, H. E., Creed, I. F., Ali, G., Basu, N., Neff, B., Rains, M., et al. (2017). Integrating geographically isolated wetlands into land management decisions. Front. Ecol. Environ. 15, 319–327. doi: 10.1002/fee.1504
Griscom, B. W., Adams, J., Ellis, P. W., Houghton, R. A., Lomax, G., Miteva, D. A., et al. (2017). Natural climate solutions. Proc. Natl. Acad. Sci. U.S.A. 114, 11645–11650. doi: 10.1073/pnas.1710465114
Han, X., Sun, X., Wang, C., Wu, M., Dong, D., Zhong, T., et al. (2016). Mitigating methane emission from paddy soil with rice-straw biochar amendment under projected climate change. Sci. Rep. 6:24731. doi: 10.1038/srep24731
Hemes, K. S., Chamberlain, S. D., Eichelmann, E., Knox, S. H., and Baldocchi, D. D. (2018). A biogeochemical compromise: the high methane cost of sequestering carbon in restored wetlands. Geophys. Res. Lett. 45, 6081–6091. doi: 10.1029/2018GL077747
Herbst, M., Friborg, T., Schelde, K., Jensen, R., Ringgaard, R., Vasquez, V., et al. (2013). Climate and site management as driving factors for the atmospheric greenhouse gas exchange of a restored wetland. Biogeosciences 10, 39–52. doi: 10.5194/bg-10-39-2013
Kamula, C. M., Zuzyk, Z. A., Lobb, D. A., and Macdonald, R. W. (2017). Sources and accumulation of sediment and particulate organic carbon in a subarctic fjard estuary: 210Pb, 137Cs, and δ13C records from Lake Melville, Labrador. Can. J. Earth Sci. 54, 993–1006. doi: 10.1139/cjes-2016-0167
Laanbroek, H. J. (2010). Methane emission from natural wetlands: interplay between emergent macrophytes and soil microbial processes. A mini-review. Ann. Bot. 105, 141–153. doi: 10.1093/aob/mcp201
Lawrence, B. A., Lishawa, S. C., Hurst, N., Castillo, B. T., and Tuchman, N. C. (2017). Wetland invasion by Typha× glauca increases soil methane emissions. Aquat. Bot. 137, 80–87. doi: 10.1016/j.aquabot.2016.11.012
Loder, A. L., and Finkelstein, S. A. (2020). Carbon accumulation in freshwater marsh soils: a synthesis for temperate North America. Wetlands 40, 1173–1187. doi: 10.1007/s13157-019-01264-6
Marton, J. M., Creed, I. F., Lewis, D. B., Lane, C., Basu, N., Cohen, M. J., et al. (2015). Geographically isolated wetlands are important biogeochemical reactors on the landscape. Bioscience 65, 408–418. doi: 10.1093/biosci/biv009
McCarty, G. W., and Ritchie, J. C. (2002). Impact of soil movement on carbon sequestration in agricultural ecosystems. Environ. Pollut. 116, 423–430. doi: 10.1016/S0269-7491(01)00219-6
McKenney, D. W., Hutchinson, M. F., Papadopol, P., Lawrence, K., Pedlar, J., Campbell, K., et al. (2011). Customized spatial climate models for North America. Bull. Am. Meteorol. Soc. 92, 611–622. doi: 10.1175/2011BAMS3132.1
Ontario Ministry of Natural Resources and Forestry [OMNRF] (2019). Southern Ontario Land Resource Information System Version 3.0 (SOLRIS 3.0). Available online at: https://geohub.lio.gov.on.ca/documents/lio::southern-ontario-land-resource-information-system-solris-3-0/about (accessed February 17, 2022).
Ontario Ministry of Natural Resources and Forestry [OMNRF] (2022). Ontario Hydro Network (OHN)-Watercourse. Available online at: https://geohub.lio.gov.on.ca/datasets/mnrf::ontario-hydro-network-ohn-watercourse/about (accessed February 17, 2022).
Pasut, C., Tang, F. H., Hamilton, D., Riley, W. J., and Maggi, F. (2021). Spatiotemporal assessment of GHG emissions and nutrient sequestration linked to agronutrient runoff in global wetlands. Glob. Biogeochem. Cycles 35:e2020GB006816. doi: 10.1029/2020GB006816
Paustian, K., Collier, S., Baldock, J., Burgess, R., Creque, J., DeLonge, M., et al. (2019). Quantifying carbon for agricultural soil management: from the current status toward a global soil information system. Carbon Manag. 10, 567–587. doi: 10.1080/17583004.2019.1633231
Pearce, D. (2003). The social cost of carbon and its policy implications. Oxford Rev. Econ. Pol. 19, 362–384. doi: 10.1093/oxrep/19.3.362
Rains, M. C., Creed, I. F., Golden, H. E., Jawitz, J. W., Kalla, P., Lane, C. R., et al. (2016). Geographically isolated wetlands are part of the hydrological landscape. Hydrol. Process. 30, 153–160. doi: 10.1002/hyp.10610
Robertson, M., BenDor, T. K., Lave, R., Riggsbee, A., Ruhl, J. B., and Doyle, M. (2014). Stacking ecosystem services. Front. Ecol. Environ. 12, 186–193. doi: 10.1890/110292
Santos, N., Monzini, J., Pedersen, E., and Borgomeo, E. (2021). The Shortest Path: Accelerating Investment Towards Carbon-Neutral Agrifood Systems. Rome: FAO. doi: 10.4060/cb7278en
Serran, J. N., and Creed, I. F. (2016). New mapping techniques to estimate the preferential loss of small wetlands on prairie landscapes. Hydrol. Process. 30, 396–409. doi: 10.1002/hyp.10582
Serran, J. N., Creed, I. F., Ameli, A. A., and Aldred, D. A. (2018). Estimating rates of wetland loss using power-law functions. Wetlands 38, 109–120. doi: 10.1007/s13157-017-0960-y
Soil Landscapes of Canada Working Group [SLCWG] (2010). Soil Landscapes of Canada version 3.2. Available online at: https://sis.agr.gc.ca/cansis/nsdb/slc/v3.2/index.html (accessed February 18, 2022).
Taillardat, P., Thompson, B. S., Garneau, M., Trottier, K., and Friess, D. A. (2020). Climate change mitigation potential of wetlands and the cost-effectiveness of their restoration. Interface Focus 10:20190129. doi: 10.1098/rsfs.2019.0129
Tangen, B. A., and Bansal, S. (2020). Soil organic carbon stocks and sequestration rates of inland, freshwater wetlands: sources of variability and uncertainty. Sci. Total Environ. 749:141444. doi: 10.1016/j.scitotenv.2020.141444
Taylor, C. A., and Druckenmiller, H. (2022). Wetlands, flooding, and the clean water act. Am. Econ. Rev. 112, 1334–1363. doi: 10.1257/aer.20210497
Treasury Board of Canada Secretariat [TBCS] (2007). Canadian Cost-Benefit Analysis Guide: Regulatory Proposals. Available online at: http://publications.gc.ca/site/eng/456648/publication.html (accessed April 21, 2022).
Valach, A. C., Kasak, K., Hemes, K. S., Anthony, T. L., Dronova, I., Taddeo, S., et al. (2021). Productive wetlands restored for carbon sequestration quickly become net CO2 sinks with site level factors driving uptake variability. PLoS One 16:e0248398. doi: 10.1371/journal.pone.0248398
Van Meter, K. J., and Basu, N. B. (2015). Signatures of human impact: size distributions and spatial organization of wetlands in the Prairie Pothole landscape. Ecol. Appl. 25, 451–465. doi: 10.1890/14-0662.1
Villa, J. A., Ju, Y., Stephen, T., Rey-Sanchez, C., Wrighton, K. C., and Bohrer, G. (2020). Plant-mediated methane transport in emergent and floating-leaved species of a temperate freshwater mineral-soil wetland. Limnol. Oceanogr. 65, 1635–1650. doi: 10.1002/lno.11467
Watmough, M. D., and Schmoll, J. (2007). Environment Canada’s Prairie and Northern Region Habitat Monitoring Program Phase II: Recent Habitat Trends in the Prairie Habitat Joint Venture. Technical Report Series No. 493. Edmonton, AB: Canadian Wildlife Service.
Watmough, M. D., Li, Z., and Beck, E. M. (2017). Canadian Prairie Wetland and Upland Status and Trends 2001–2011. Edmonton, AB: Canadian Wildlife Service.
Waz, A., and Creed, I. F. (2017). Automated techniques to identify lost and restorable wetlands in the Prairie Pothole Region. Wetlands 37, 1079–1091. doi: 10.1007/s13157-017-0942-0
Yanai, R. D., Wayson, C., Lee, D., Espejo, A. B., Campbell, J. L., Green, M. B., et al. (2020). Improving uncertainty in forest carbon accounting for REDD+ mitigation efforts. Environ. Res. Lett. 15:124002. doi: 10.1088/1748-9326/abb96f
Zhang, B., Schwartz, F. W., and Liu, G. (2009). Systematics in the size structure of prairie pothole lakes through drought and deluge. Water Resourc. Res. 45:WR006878. doi: 10.1029/2008WR006878
Keywords: nature-based climate solutions, wetland, conservation, restoration, carbon sequestration, economic analysis, cost, benefit
Citation: Creed IF, Badiou P, Enanga E, Lobb DA, Pattison-Williams JK, Lloyd-Smith P and Gloutney M (2022) Can Restoration of Freshwater Mineral Soil Wetlands Deliver Nature-Based Climate Solutions to Agricultural Landscapes? Front. Ecol. Evol. 10:932415. doi: 10.3389/fevo.2022.932415
Received: 29 April 2022; Accepted: 10 June 2022;
Published: 18 July 2022.
Edited by:
Laurie Alexander, United States Environmental Protection Agency (EPA), United StatesReviewed by:
Swapan Talukdar, Jamia Millia Islamia, IndiaKelly Hondula, Arizona State University, United States
Copyright © 2022 Creed, Badiou, Enanga, Lobb, Pattison-Williams, Lloyd-Smith and Gloutney. This is an open-access article distributed under the terms of the Creative Commons Attribution License (CC BY). The use, distribution or reproduction in other forums is permitted, provided the original author(s) and the copyright owner(s) are credited and that the original publication in this journal is cited, in accordance with accepted academic practice. No use, distribution or reproduction is permitted which does not comply with these terms.
*Correspondence: Irena F. Creed, aXJlbmEuY3JlZWRAdXRvcm9udG8uY2E=