- 1Centro de Estudios Avanzados en Zonas Áridas (CEAZA), Coquimbo, Chile
- 2Facultad de Ciencias del Mar, Departamento de Biología Marina, Universidad Católica del Norte, Coquimbo, Chile
- 3Millennium Institute of Oceanography (IMO), Universidad de Concepción, Concepción, Chile
- 4National Institute of Biology, Marine Biological Station, Piran, Slovenia
- 5Cooperative Institute for Marine Resources Studies, Oregon State University, Newport, OR, United States
Studies assessing latitudinal variations in habitat conditions and phenotypic plasticity among populations yield evidence of the mechanisms governing differentiation in the potential to adapt to current/future habitat changes. The cosmopolitan copepod species Acartia tonsa thrives across ocean clines delimiting Seasonal (30–40° S) and Permanent (10–30° S) Upwelling coastal provinces established during the middle–late Pliocene (3.6–1.8 Ma) alongshore the South East Pacific (SEP), nowadays exhibiting contrasting variability features related to several ocean drivers (temperature, salinity, pH, and food availability). Latitudinal variation across the range of environmental conditions of the coastal provinces can contribute toward shaping divergent A. tonsa’s phenotypes, for example, through specific patterns of phenotypic plasticity in morphological and physiological traits and tolerance to environmental drivers. With the aim of contributing to the understanding of these adaptive processes in a relatively little studied oceanic region, here we compared the expression of parental (i.e., adult size, egg production, and ingestion rate) and offspring (i.e., egg size) traits in relation to variation in environmental habitat conditions across different cohorts of two distant (> 15° latitude) A. tonsa populations inhabiting estuarine and upwelling habitats located in the Seasonal and Permanent Upwelling province, respectively. Mean conditions and ranges of variability in the habitat conditions and phenotypic plasticity of parental and offspring traits within and among cohorts of A. tonsa populations varied significantly across the different examined regions (i.e., Seasonal vs. Permanent). We also found significant differences in the coupling of habitat variability and trait expression, suggesting that the differences in trait expressions might be related to habitat variability. The phenotypic divergence was translated to cohort-related patterns of trait trade-offs regulating reproduction and tolerance of egg production efficiency that can jointly determine the level of plasticity, genetic structure, or local adaptation. The current findings provide novel evidence of how divergent phenotypes might sustain A. tonsa populations across variable coastal provinces of the SEP.
Introduction
The variability in morphological and physiological traits that underlie the phenotypic plasticity of individuals is a fundamental component toward understanding the dynamics and persistence of the wild populations against the fluctuating nature of the habitat (Pianka, 1976; Stearns, 1992). Trait-related phenotypic plasticity (i.e., the genotype to have a broader tolerance) in the variable habitats supports the maintenance of individual fitness despite the shifts in the mean habitat conditions and inherent variability (e.g., Schlichting and Pigliucci, 1998; Pigliucci, 2001, 2005). Phenotypic plasticity is manifested during different ontogenetic stages (Senner et al., 2015) and across multiple life history traits (Langerhans, 2018). The expression of traits can vary with that of other traits through trade-offs (Zera and Harshman, 2001; Ortega-Mayagoitia et al., 2018), which can reveal adaptive costs of plasticity (Stillman, 2003; Sasaki and Dam, 2021). In adults, phenotypic plasticity is also related to the phenotype and fitness of their offspring, through parental anticipatory effects or adaptive plasticity (Morrongiello et al., 2012 and references therein). This is mediated by genetic components contributing to the local adaptation (Sunday et al., 2011), such that genetic polymorphism may promote rapid phenotypic responses as a response to changes in the environment (Bitter et al., 2019; Brennan et al., 2019; Sasaki and Dam, 2020). Studies of the drivers and extent of intraspecific variations in phenotypic plasticity within single marine species can yield essential information in determining the mechanisms of populations resilience under current as well as the potential adaptation to future habitat changes (Porlier et al., 2012; Barley et al., 2021; Sasaki and Dam, 2021).
Climate and geographic milestones consolidated during the middle–late Pliocene (3.6–1.8 Ma) (Lindberg, 1991; Cardenas et al., 2009 and references therein) alongshore the South East Pacific (SEP) nowadays promote different climatic (Tapia et al., 2014) and ecogeographic coastal provinces (Camus, 2001; Escribano et al., 2003; Supplementary Figure 1). Each province exhibits contrasting mean conditions and variability ranges in several ocean drivers (temperature, salinity, pH, and food) (Vargas et al., 2017), likely conducting to spatial patterns of local adaptation in contemporary populations (Gaitán-Espitia et al., 2017; Vargas et al., 2022). The Seasonal Upwelling province extends from 30° S to 40° S, corresponding to > 1,000 km of coast widely interrupted by river discharges. The river influence on the coastal ocean is maximal during the rainy austral fall-winter, while an intense upwelling activity becomes more important on local hydrography during spring-summer seasons (Vargas et al., 2016). The physical–chemical gradients occurring along and across-shore in these habitats due to river and upwelling influence also experience high-frequency shifts associated with tidal cycles, yielding a temporarily predictable and spatially variable temperature, salinity, and pH mosaic over relatively small coastal areas (Aguilera et al., 2013; Vargas et al., 2016; Garcés-Vargas et al., 2020; Osma et al., 2020). More than 15° latitude toward the north, the Permanent Upwelling province (18–30° S) is located off the Atacama Desert, where nearshore habitat conditions vary in a less predictable way over the synoptic-temporal scale (García-Reyes et al., 2014; Aguilera et al., 2020). Year-round upwelling of cold (< 14°C) and salty and low pH (< 7.7) subsurface water (Torres et al., 1999; Aguilera et al., 2020) leads to synoptic changes in surface ocean conditions, though individual upwelling events are highly variable depending on wind stress and involved water masses (Song et al., 2011; Oerder et al., 2015). Additionally, El Niño (EN) Southern Oscillation can dramatically disrupt the upwelling variability pattern given its proximity to the Equatorial Pacific (Ulloa et al., 2001; Escribano et al., 2004; Aguilera et al., 2019). Empirical evidence scattered over these provinces accumulated over three decades has established strong signatures of higher variability and likely lower predictability in the upwelling habitats (Tapia et al., 2009; Aguirre et al., 2021). These in situ studies are fundamental to the ongoing debates in ecology and evolution, specifically, the extensive theoretical and emerging empirical research investigating how organisms have evolved to cope with high heterogeneous habitats (Vargas et al., 2017, 2022).
The cosmopolitan copepod species Acartia tonsa (Chaalali et al., 2013) is contemporarily distributed alongshore the SEP from 54° S in the sub-Antarctic region (Aguirre et al., 2012), to the tropical upwelling (20° S) systems (Aguilera et al., 2011; Ruz et al., 2015). In fact, A. tonsa is common and abundant enough to be considered as a foundation species in estuarine and upwelling habitats embedded in the contrasting coastal provinces of the SEP (Peterson et al., 1988; Hidalgo and Escribano, 2007; Vargas et al., 2007; Escribano et al., 2009; Aguilera et al., 2013). Latitudinal gradients occurring in the average and ranges of environmental drivers can influence the expression of traits in copepods, leading to divergent patterns of phenotypic plasticity within populations on a long-term scale (Dunson and Travis, 1991; Pörtner et al., 2004; Lercari and Defeo, 2006). Investigations of variation in physiological traits across space and time can yield evidence for latitudinal differences in phenotypic plasticity among A. tonsa populations (Sasaki and Dam, 2019), including acclimation (Stillman, 2003) and developmental phenotypic plasticity (Pereira et al., 2017), and ultimately shape populations’ tolerance to variation in environmental conditions (Dam, 2013). Phenotypic divergence can be assessed by analyzing variation in the expression of parental and offspring traits within and across cohorts of the A. tonsa populations inhabiting latitudinally distant and climate different coastal provinces. Body size (BS), egg production (EPR), egg size (ES), and ingestion rate (IR) are fundamental traits shaping phenotypic plasticity and acclimation responses outlining the fitness under variable conditions in a given habitat (Kingsolver and Huey, 1998). Recent studies investigated the factors that determine variations in the expression of the traits in heterogeneous habitats (Moran, 1992; Reed et al., 2010; Bernhardt et al., 2020; Bitter et al., 2021) and showed that more heterogeneous habitats, i.e., characterized by a greater difference in the variation, might favor alternative phenotypes, expanding the phenotypic plasticity. Phenotypic plasticity is often modeled in wild populations as the slope of the reaction norm (Stearns, 1992), which can reveal differences among populations in the tolerance to environmental changes (Sasaki and Dam, 2019). On the other hand, dominant drivers, such as temperature, might also act toward reducing phenotypic plasticity (Barley et al., 2021), determining the impact of multiple factors on the development of the population-level phenotypic plasticity. In order to understand the mechanisms that govern the variation in copepod phenotypic plasticity across latitudinal gradients, we studied variation in the expression of morphometric and physiological traits within and across three different cohorts of the two A. tonsa populations sourced from an estuarine and upwelling habitat located at the Seasonal (39.8° S) and Permanent (23.3° S) Upwelling provinces of the SEP, respectively. Our study characterized specific habitat variability and phenotypic plasticity at the inter-daily scale of a comprehensive suite of parental (BS, EPR, and IR), and offspring (ES) reproduction-related traits, established for each cohort. Our fundamental question was to investigate the linkage between environmental conditions and variation in the expression of traits within and among cohorts potentially shaping divergent patterns of phenotypic plasticity in A. tonsa populations. We found significant differences in the phenotypic plasticity of parental and offspring traits among cohorts of and between A. tonsa populations across two examined regions (i.e., Seasonal vs. Permanent). Environmental–biological coupling and cohort-related patterns of trait trade-offs regulating reproduction also diverged between populations, potentially determining the tolerance to environmental drivers. Our study provides novel evidence of how divergent phenotypes might sustain A. tonsa populations across latitudinal coastal gradients in the SEP.
Materials and methods
Two divergent study areas
The estuarine and upwelling habitat are located in two distant (>3,000 km, >15° latitude distant) and contrasting climatic-ecogeographic provinces alongshore the SEP (Camus, 2001; Escribano et al., 2003; Tapia et al., 2014; Supplementary Figure 1). The first habitat is an estuarine system in Southern Chile (Valdivia River Estuary, 39.8° S), strongly seasonal and affected year-round by river runoff discharges (average 484 ± 300 m3 s–1; Pérez et al., 2015). Nearshore, river discharges are partially mixed by coastal circulation (Pino et al., 1994), and temporarily modulated by tidal cycles. Such interaction promotes as a whole a marked alongshore gradient in the average and range of temperature (15 ± 2°C), salinity (33 ± 1 psu), and pHT (7.93 ± 0.3) (Aguilera et al., 2013; Garcés-Vargas et al., 2020; Osma et al., 2020). The second habitat is the Antofagasta upwelling center (Antofagasta, 23.3° S), situated off the most arid desert in the world, the Atacama Desert, which lacks seasonality (Tapia et al., 2014) and freshwater discharges (Hartley et al., 2005). Equatorward wind prevails year-round at this latitude (Strub et al., 1998), favoring permanent upwelling events at the coast. Depending on the intensity and extent of wind stress, the vertical advection of deep-cold (< 14°C) and low pHT (< 7.7) water masses synoptically affects the nearshore surface habitat (Torres et al., 1999; Aguilera et al., 2020), with upwelling events highly different from each other. Mean profiles of temperature and salinity illustrate vertical differences between habitats and vertical variations as well (Supplementary Figure 2). Importantly, ocean clines delimiting the aforementioned coastal provinces can act as effective dispersal barriers for populations of benthic species (Cardenas et al., 2009 and references therein).
Cohorts’ assessment and temporal habitat characterization
The copepod A. tonsa is a neritic species distributed over the upper 30 m of the water column (Paffenhöfer and Stearns, 1988). Within this range, the peak of chlorophyll-a (i.e., copepods food index) is often placed within the upper 10 m in nearshore habitats alongshore the SEP (Escribano et al., 1997, 2009; Osma et al., 2020). Depending on habitat depth, variability in environmental conditions (temperature, salinity, pH, and food) was in general characterized at 7 m in the estuarine and 10 m in the upwelling habitat (Table 1). Because of specific depth measurements, we could not consider vertical variability in the conditions, however, the large bathymetric gradient occurs at the surface (1 m) and near the bottom due to the influence of less dense and low pH freshwater lens (Osma et al., 2020) and turbulent and sediment enriched tidal currents (Pino et al., 1994; Vargas et al., 2003), respectively (see Supplementary Figure 2). In the upwelling habitat, A. tonsa displays restricted vertical migrations constrained to the upper 30 m likely due to a shallow oxygen minimum zone (Escribano et al., 2009). Habitat conditions were measured interdaily (i.e., each 4–6 days) through 30 estuarine and 28 upwelling nearshore surveys (Table 1). The occurrence and reproduction of the estuarine A. tonsa population are restricted to the austral spring–summer season (Aguilera et al., 2013). Thus, sampling distributed from 2010 to 2012 was carried out during the austral spring–summer seasons (Table 1), corresponding to the seasonal occurrence of the individuals, with no copepods found during fall–winter periods (Aguilera et al., 2013). Variations in habitat drivers (and thus related copepod traits) at the estuarine habitat were measured under the impact of the flood tides, since during ebb tides, the water column is shallower and the abundance of A. tonsa individuals was lower. Temperature (°C) and salinity (psu) were recorded at the sampling depth (± 1 m) by a CTD (Ocean Seven 305 Plus1). Discrete water samples were collected at 7 m depth with a Niskin bottle for determination of food availability and pHT. Food concentration was determined in triplicate as counts of nanoflagellates and phytoplankton cells in carbon units (μg C L–1) with their bio-volumes assessed with an inverted microscope OLYMPUS IX-51. Bio-volumes were converted to carbon units by using carbon:volume conversion factors available in the literature (e.g., Vargas and González, 2004). Within 2 h of water collection, triplicate pHT measurements were assessed in a closed 25-ml cell thermostated at 25.0°C using a Metrohm 713 pH meter, and a glass combined double junction Ag/AgCl electrode (Metrohm model 6.0219.100) calibrated with Tris buffer at 25°C. By using temperature, salinity, pH25°C, and total alkalinity (not shown) data, pH was calculated at total scale (pHT) through the CO2CYS software v.3.0 (Pierrot et al., 2021).
Reproductive copepods of A. tonsa are found throughout the year in the upwelling habitat with several season-independent recruitment episodes with generation times of two weeks to two months (Hidalgo and Escribano, 2007). Discrete samplings in this habitat were carried out throughout 2015 (Table 1). Temperature (°C) and salinity (psu) were recorded at the sampling depth (± 1 m) with a SeaBird SBE19 Plus CTD. Discrete water samples were attained at 10 m depth through oceanographic sample collections, for determinations of chlorophyll-a concentration (copepod food) and pHT. For determinations of chlorophyll-a (Chl) concentration, seawater was filtered on 200 μm mesh to remove large-sized grazers and debris while maintaining natural food assemblages. Triplicate samples (200 ml) were filtered onto a GF/F filter (nominal pore size = 0.7 μM). Chl was extracted for 24h in 90% acetone v/v and measured in a TD Turner fluorometer (Strickland and Parsons, 1972). Chl concentration (μg Chl L–1) was converted to carbon units by using a Chl:C ratio = 120 (Vargas and González, 2004). Within 2 h of collection, seawater pHT was measured in triplicate as described above in a closed 25-ml cell thermostated (25°C).
Copepod collection
Parental and offspring traits were assessed in three different cohorts of the estuarine A. tonsa population over the period of 2010–2012 (Table 1), while in the northern-upwelling habitat, these traits were assessed throughout 2015. Based on intraseasonal changes in temperature and body size observed at the upwelling habitat during sampling, three different cohorts were identified within the upwelling population (see “Results” section). Plankton samples were obtained by gently oblique hauling of a 200-μm mesh size WP2 net equipped with a closing system and non-filtering 1 L cod-end, from 7 to 12 m (estuarine) and 10–15 m (upwelling) depth strata. Each time, the content of the cod-end was gently transferred to a temperature-controlled container containing aquarium bubblers to keep containers well oxygenated over the duration of transport to the laboratory. Within 2 h of collection, mature and visibly healthy adult copepod females were sorted out under a stereomicroscope and transferred in groups of 10 females to 200 ml beakers. Copepods were incubated in natural seawater collected during sampling with the aim of conducting the experiments to estimate phenotypic plasticity in parental and offspring traits as related to the habitat-specific variations in environmental conditions (Supplementary Figure 2). Laboratory temperature was maintained at a controlled temperature (14 ± 1°C at the estuarine and 15 ± 1°C at the upwelling habitat), which corresponded to the temperature conditions experienced by the various cohorts in the natural habitats. Given that we infer that the parental and offspring traits are all based on the adult females makes a more scattered sampling in the estuarine area more acceptable.
Copepod traits
The assessed traits in the adult females were the following: body size (BS), mean egg production rate (EPR), egg size, and ingestion rate (IR). For BS estimations, up to 45 copepod females grouped in three batches were immediately preserved in 90% ethanol for further measurements of body size (cephalothorax length, mm). Body length was converted to body mass with the A. clausi length–dry weight regressions (Uye, 1982) and to body carbon (BC) assuming that the C content was 45% of dry weight (Kiørboe and Nielsen, 1994). The body mass was then considered to estimate specific reproduction rates. Mean egg production rates (EPR) were estimated based on three groups of 30–40 copepod females, individually incubated at in situ temperature for 17–20 h in 200 ml clean crystallizing dishes filled with sieved (<200 μm) natural seawater. Eggs produced over this period by each group were counted under a stereomicroscope, standardized to daily duration (24 h), with the mean EPR expressed as the egg fem–1 d–1 (± SD). Eggs (30) produced by each female group were randomly sorted and preserved (90% ethanol), and their size (i.e., diameter, μm) was measured using an Olympus IX-51 inverted microscope within two weeks of preservation to diminish ethanol effects on egg size (Moksness and Fossum, 1992). Assuming spherical-shaped eggs and a conversion factor of 0.14 × 10–6 μg C μm–3 ratio (Huntley and Lopez, 1992), the diameter of the egg size was converted to mass (μg C). Specific EPR was calculated as the EPR/BC quotient × 100% (EPR%BC).
In parallel, ingestion rates (IR) were estimated through the bottle incubation method (Kiørboe et al., 1982) and expressed in μg C fem–1 d–1. Additional copepod (4–5) females were pipetted into three 660-ml borosilicate bottles containing ambient water filled with < 200 μm natural food assemblages. These bottles alongshore another three control bottles without animals (i.e., blank bottles) were placed on a plankton wheel rotated r at 1.2 rpm for 14–16 h at the same temperature as the EPR was estimated. Subsamples were preserved at the beginning (T0) and at the end of the incubation period (Tf) from experimental and blank bottles. Ingestion rate (IR) (μg C fem–1 d–1) was calculated following Frost (1972) and modified by Marín et al. (1986). With data of EPR (μg C fem–1 d–1) and IR (μg C fem–1 d–1), the egg production efficiency (EPE) was calculated as the EPR/IR quotient.
Defining terms
Due to the abundance of data and multiple analyses across various cohorts, we provide a definition of the measurements and this related interpretation in this study. In this study, we refer to estuarine and upwelling A. tonsa populations in the absence of genetic evidence supporting the occurrence of various genotypes. Cohorts of the respective population were sampled in subsequent chronological order, which implies that the latter cohorts inherently contain genetic information in response to environmental conditions experienced by earlier cohorts. However, cohorts, especially those from the estuarine habitat but also those from the upwelling location considering habitat environmental variability, were sampled and correspond to discrete subgroups within a highest-level nominal group or population. Habitat drivers and copepod traits were characterized in terms of mean values and their variability, with reaction norms on different cohorts of both A. tonsa populations. For each cohort, habitat conditions and traits were assessed only at the adult stages, which referred to different cohorts between measurements. There are two measures of variability within and among cohorts. The first one is a measure of variations, i.e., the variability experienced by a single cohort. The second is similar to that experienced across generations or among different cohorts, ultimately characterizing each population. We also define the term “trade-off” as a significant correlation between two traits (Zera and Harshman, 2001; Ortega-Mayagoitia et al., 2018).
Data analysis
Habitat environmental and copepod data were examined for requirements of parametric tests, such as normal distribution (Lilliefors test, p < 0.01) and homogeneity of variance (Levene’s tests). Variations in nominal environmental drivers and copepod traits were assessed regarding two variability factors: habitats/populations (groups) and cohorts (subgroups). To evaluate changes in a nominal variable among subgroups in relation to a single value of the highest-level nominal variable (the groups), we utilized a nested design ANOVA (Error type III) in which cohorts were nested within their respective habitat/population. To evaluate the potential effects of plasticity on trait differences between habitats, we conducted a linear mixed effects model in which environmental drivers and habitat were considered as fixed effects and cohorts as random effects. Plasticity effects on trait variations were indicated by a significant interaction between environmental drivers and habitat. The correlation or coupling between environmental variability with identified drivers and biological variables was explored in both habitats, first through the Principal Component Analysis (PCA). The numerical relationships of specific EPR%BC and EPE with environmental drivers were explored with Pearson’s correlation tests, such as plastic responses expressed as a mean reaction norm that had a significantly non-zero slope, and were compared between populations (Stearns, 1992). Mechanistic relationships between traits and specific environmental features can yield insights into ecological speciation among populations (Chen and Hare, 2008). Ocean Data View and SURFER packages provided graphical outputs, whereas statistical analyses were performed in STATISTICA package10.
Results
Intrahabitat/population variability
The distribution of environmental conditions (temperature, salinity, pHT, and food concentration) showed normal distribution and homogeneity of variance in both habitats. Habitat drivers varied widely among cohorts of the estuarine (Figure 1) and upwelling (Figure 2) population, while also exhibiting fluctuations within cohorts (Supplementary Figure 3). The mean temperature range at the estuarine habitat was 12°C–17°C (Figure 2A), in which each cohort experienced mean thermal fluctuations of 3.5°C without significant changes among cohorts (Table 2). A warmer mean temperature range (14°C–17°C) was observed in the upwelling habitat (Figure 2A). The significant changes in temperature were observed among cohorts of the upwelling population (Table 2), which in general experienced narrower (2.6°C) thermal fluctuations. Salinity varied between 31.5 and 34 psu at the estuarine (Figure 1B) and between 34.6 and 35.1 psu at the upwelling habitat (Figure 2B). Cohorts of the estuarine population experienced larger salinity fluctuations, however, salinity varied significantly among cohorts of both populations, especially of the upwelling population (Table 2). There was a surprising similarity in the ranges of pHT variations experienced by cohorts of the estuarine (Figure 1C) and upwelling (Figure 2C) populations. The significant pHT variations occurred among cohorts of both populations (Table 2) though pHT ranges were wider for estuarine cohorts. Outliers were detected in food concentration data of the upwelling habitat (Grubbs’ test, > 400 μg C L–1). After removing outlier values, food varied between 0 and 350 μg C L–1 in the estuarine (Figure 1D) and upwelling (Figure 2D) habitat. Variations in food concentration were significant among cohorts of the upwelling population (Table 2).
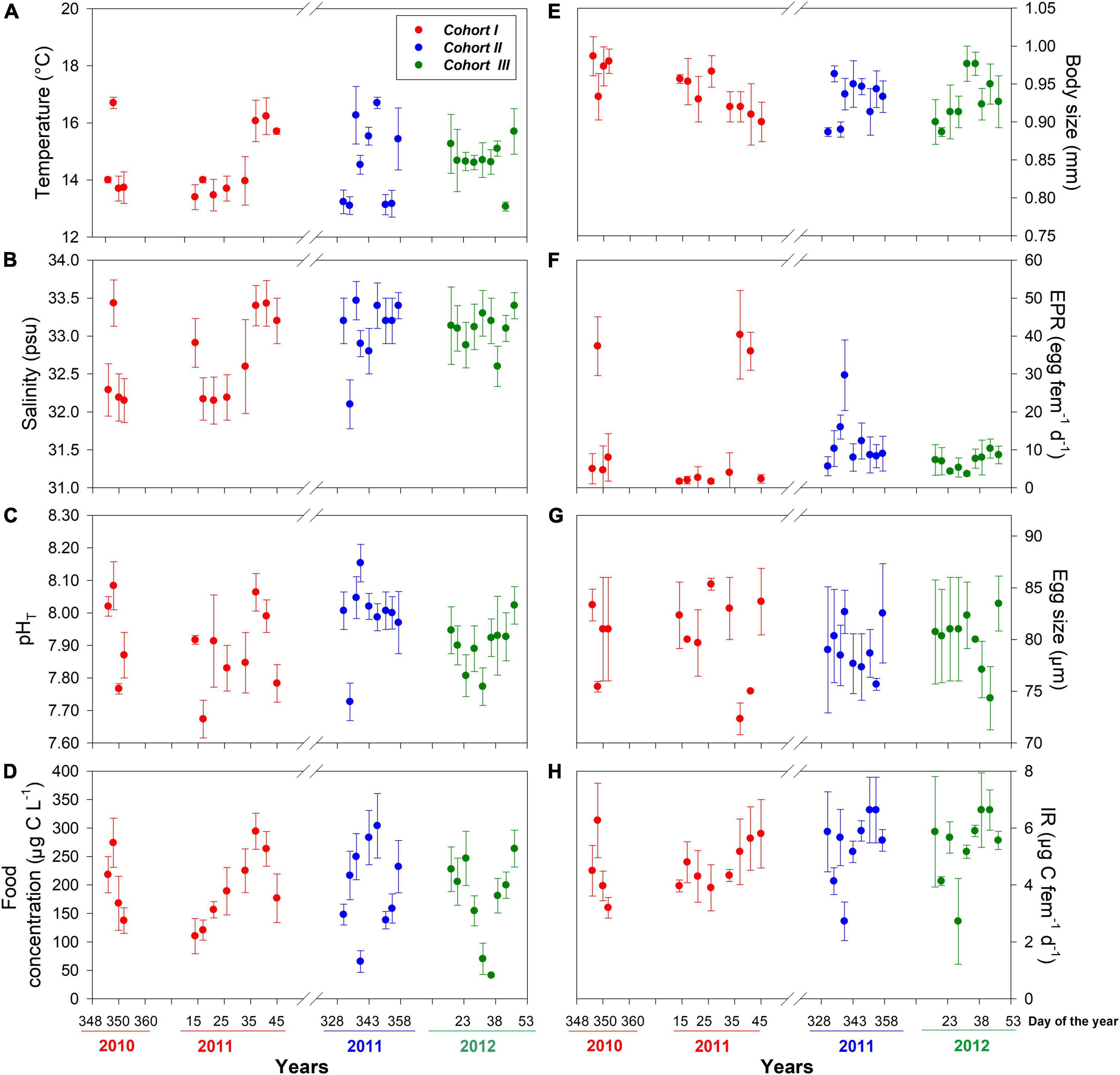
Figure 1. Interdaily mean (± SD) values of environmental drivers (A–D) and copepod traits (E–H) assessed for three different cohorts at the estuarine habitat. Sampling was conducted during the seasonal occurrence and reproductive period of the local A. tonsa population.
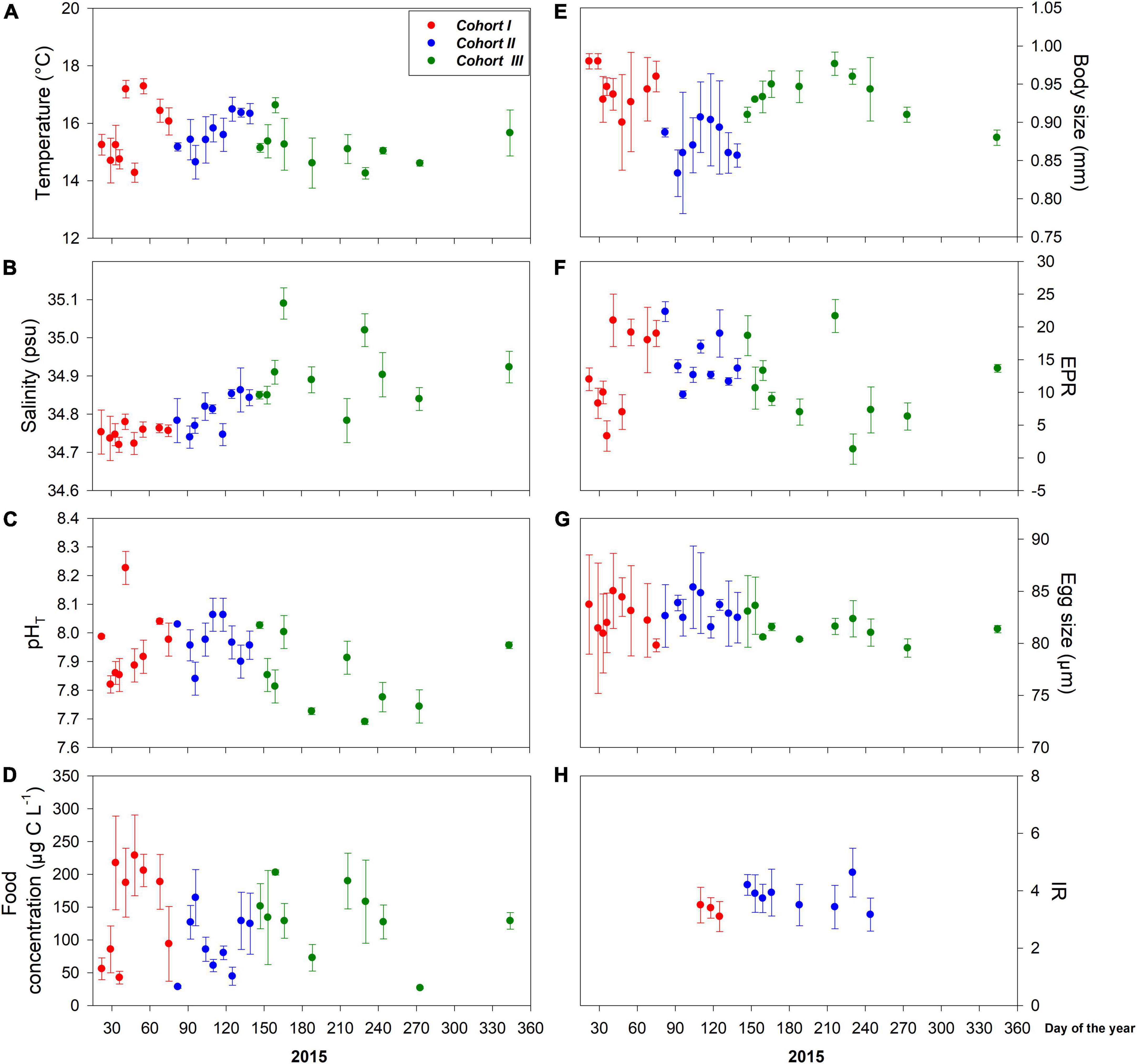
Figure 2. Interdaily mean (± SD) values of environmental drivers (A–D) and copepod traits (E–H) assessed for three different cohorts at the upwelling habitat. Local A. tonsa population reproduces year-round under upwelling-dominant environmental conditions, which were strongly disrupted during samplings due to El Niño 2015.
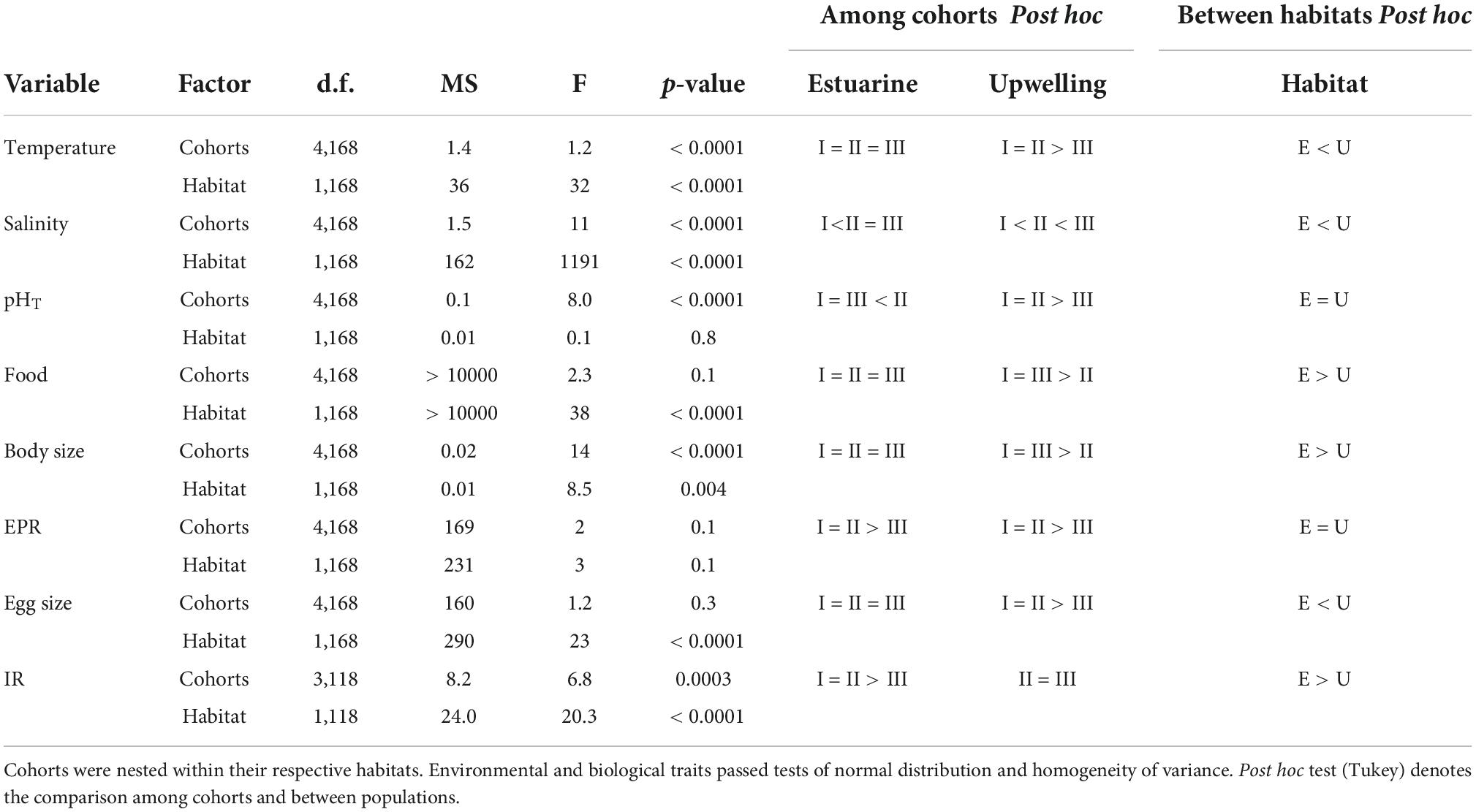
Table 2. Comparison of environmental drivers and copepod traits regarding two variability factors: cohorts (3 levels) and habitats (2 levels).
The distribution of parental and offspring traits was normally distributed, but egg size failed the test for homogeneity of variance. The expression of parental and offspring traits displayed important variations within the estuarine (Figure 1) and upwelling (Figure 2) populations. With respect to body size, it varied between 0.85 and 0.99 mm within the estuarine (Figure 1E) and between 0.80 and 0.99 mm within the upwelling (Figure 2E) population. Variations in BS were significant only among cohorts of the upwelling population (Table 2), although BS fluctuations within cohorts were similar between populations. Removing the 0 values (likely related to the incubation of non-egg producing C5 stage with a similar size range as the adults) and outliers (> 70 egg fem–1 d–1), the EPR range was 1–57 egg fem–1 d–1 in the estuarine (Figure 1F) and 2–25 egg fem–1 d–1 in the upwelling (Figure 2F) population. There were significant EPR variations among cohorts of both populations (Table 2), and larger fluctuations were observed within and among estuarine cohorts. The egg size (ES) varied between 71.3 and 86.2 μm, and 76.5 and 89.5 μm among the cohorts of the estuarine (Figure 1G), and the upwelling (Figure 2G) population, respectively. Although changes in the ES within cohorts were similar between populations, significant differences in BS were observed among cohorts of the upwelling population (Table 2). IR varied between 2 and 7 μg C fem–1 d–1 among the three cohorts assessed within the estuarine population (Figure 1H) and between 3 and 5 μg C fem–1 d–1 among two cohorts of the upwelling population (Figure 2H). IR varied significantly only among cohorts of the estuarine population (Table 2).
Significant effects of isolated environmental drivers together with interactions between environmental drivers and habitat explained a relatively lower (28%) to moderate (51%) proportion of the variations accounted for in the expression of copepod traits, according to the linear mixed-effects model (Table 3). The interactions between salinity, food, and habitat were important for changes observed in body size. With the exception of food, the interactions between habitat and assessed environmental drivers were significant for EPR variation, whereas only the interaction between habitat and salinity was relevant for changes in egg size. Significant correlations (i.e., trade-offs) between morphometric and physiological traits were detected only in the estuarine population (Figure 3). Female size was inversely related to EPR (Figure 3A), and EPR was negatively related to eggs (Figure 3B).
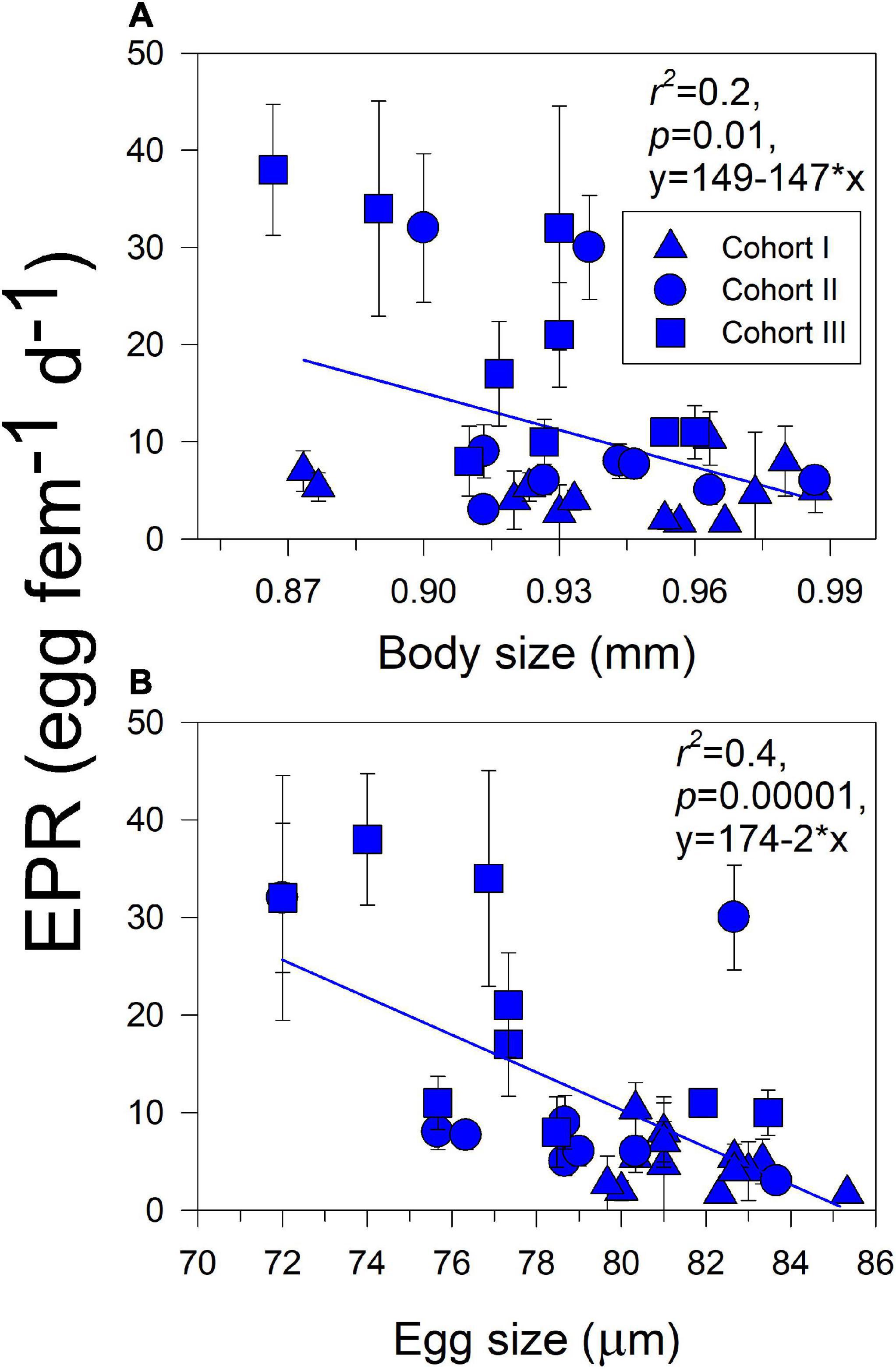
Figure 3. Correlations (i.e., trade-offs) between parental (A) and offspring (B) traits with reproductive trait in the estuarine A. tonsa population. Dots represent the average (± SD) value of each trait at a given sampling survey.
Interhabitat/population variability
An average and ranges of environmental conditions and copepod traits were plotted in box plots for comparison between habitats and populations (estuarine and upwelling) (Figure 4). There were significant differences between the two habitats in all assessed environmental conditions except for pHT (Table 2). The ranges of temperature and salinity were wider, and mean values were significantly higher in the upwelling than estuarine habitat (Figures 4A,B). In contrast to temperature and salinity, pHT ranges largely overlapped (Figure 4C) with no significant difference in mean pHT between the habitats (Table 2). Food concentration ranges also overlapped between habitats (Figure 4D), although the mean food concentration in the estuarine (< 150 μg C L–1) was lower than in the upwelling habitat (> 200 μg C L–1) (Table 2). There were significant differences in the expression of parental and offspring traits between the populations, except for EPR (Figure 4). The body size of adult estuarine females was significantly larger than that of their upwelling counterparts (Table 2), though exhibiting a narrower BS range (Figure 4E). Despite mean EPR values not varying significantly between the estuarine and upwelling populations (Table 2), the EPR range was wider within estuarine females (Figure 4F). Among similar ranges of egg size (Figure 4G), the mean value of egg size produced by upwelling females was significantly larger than that produced by estuarine females (Table 2). The IR of estuarine females was significantly higher than that of their upwelling counterparts (Table 2) also exhibiting a wider IR range (Figure 4H).
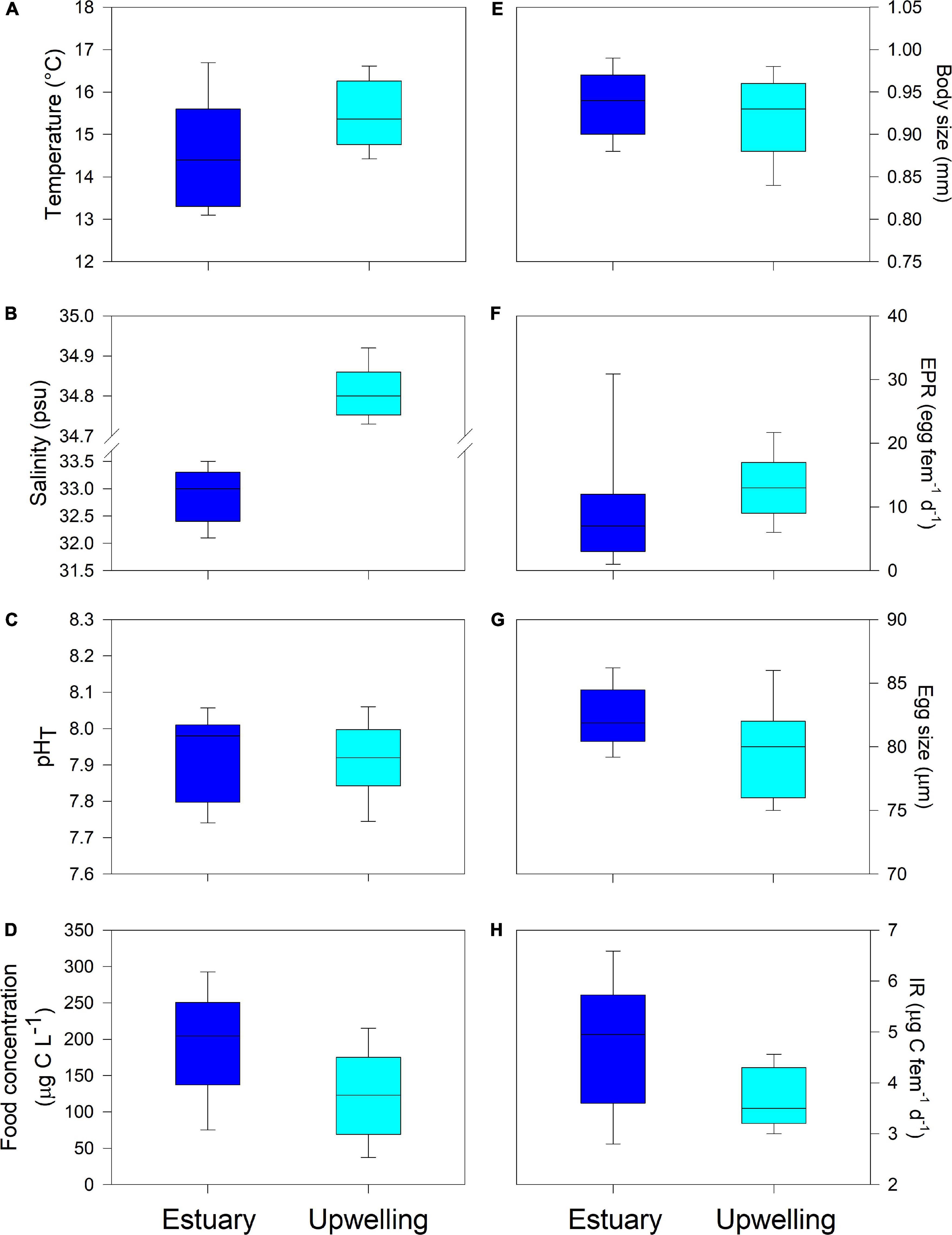
Figure 4. Comparison of box plots of environmental drivers (A–D) and copepod traits (E–H) between examined estuarine and upwelling populations. Statistical results are provided in Table 2.
The association or coupling among nominal environmental drivers and between those with copepod traits was assessed for each habitat with a Principal component analysis (PCA) (Figure 5). Upon assessed abiotic and biotic variables, this analysis accounted for a higher explained variability in the estuarine (R2 = 69) than in the upwelling (R2 = 64) habitat. This analysis also revealed a stronger coupling among environmental conditions and between environmental conditions with copepod traits in the estuarine (Figure 5A) than in the upwelling habitat (Figure 5B). Featured variables were salinity, pHT, EPR, and ES in the estuarine habitat, and salinity, pHT, BS, and EPR in the upwelling habitat (Supplementary Table 1).
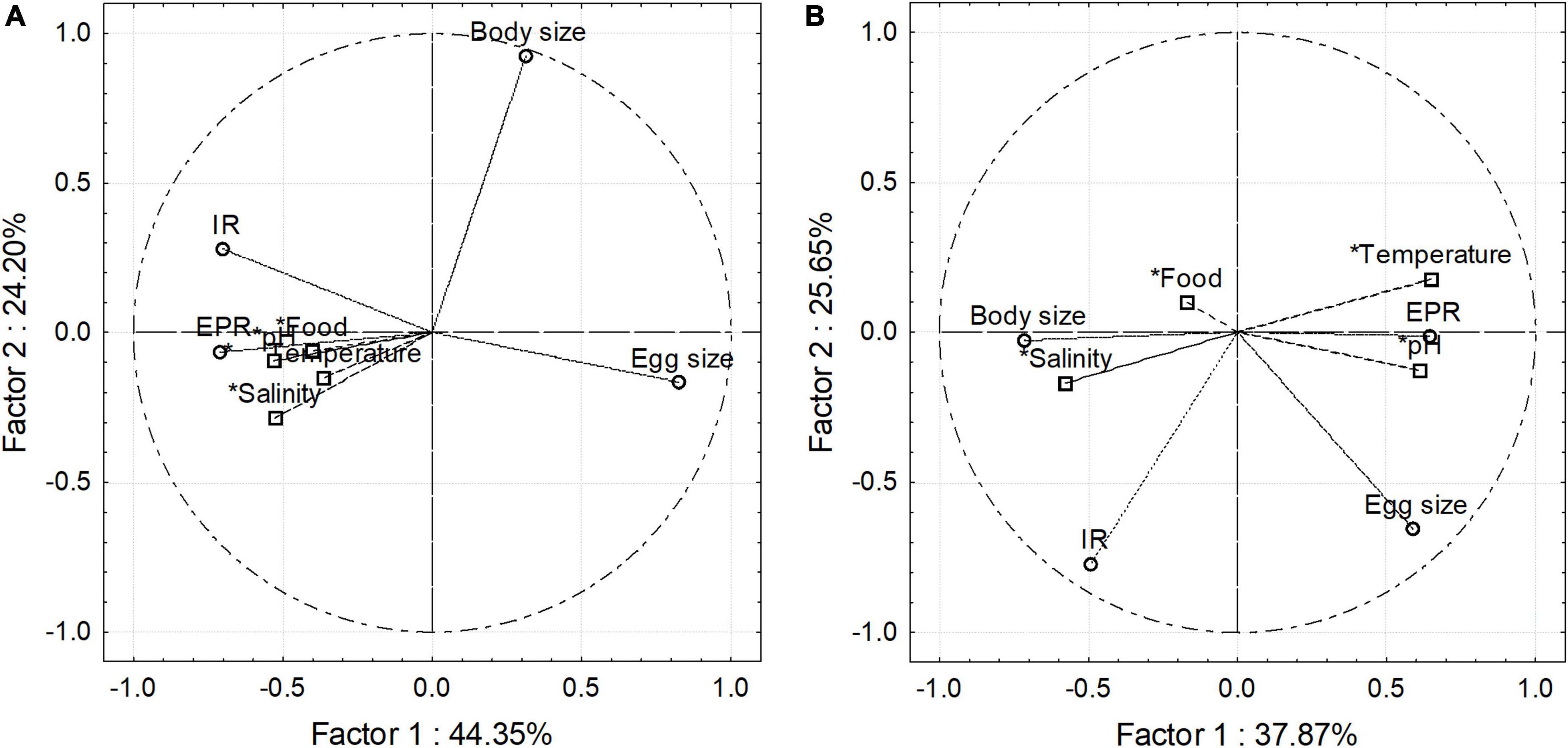
Figure 5. Environmental–biological coupling in the estuarine (A) and upwelling (B) habitat explored through Principal Component Analysis. Besides explaining a higher proportion of the observed variance, PCA in the estuarine habitat also indicates biological cues, especially physiological ones correlated among them.
Habitat-specific drivers on the reproduction
Reproduction rates (EPR) were standardized by the body mass of females in carbon units (EPR expressed as a percentage of body mass) (Figure 6). The significant changes in EPR%BC were observed among cohorts of both populations (F4,168 = 14, p < 0.0001) (Figure 6A). Such variability in the EPR%BC was higher among cohorts of the upwelling population, which produced significantly (F1,168 = 16, p < 0.0001) more carbon eggs at a similar body size than their estuarine counterparts (Figure 6B). In addition, the egg production efficiency (EPE), corresponding to the EPR/IR ratio was significantly correlated (i.e., reaction norms), with environmental conditions in both habitats (Figure 7). These correlations demonstrate the impact (i.e., mitigating/aggravating) of habitat conditions and potential differences in the tolerance (i.e., slope) to environmental drivers between populations (Table 4). Temperature exerted a mitigating (i.e., positive) effect on the EPE of both populations (Figure 7A), though the EPE of the estuarine population was less tolerant to thermal changes (Table 4). Salinity showed contrasting effects on EPE, mitigating in the estuarine, and aggravating (i.e., negative) in the upwelling population (Figure 7B). The pHT exerted mitigating effects on the EPE of both populations (Figure 7C), and the estuarine population was less tolerant (slope = 0.8) than the upwelling (slope = 0.12) population to pH changes. Food concentration was only related to EPE in the estuarine population, where it exerted a positive effect (Figure 7D).
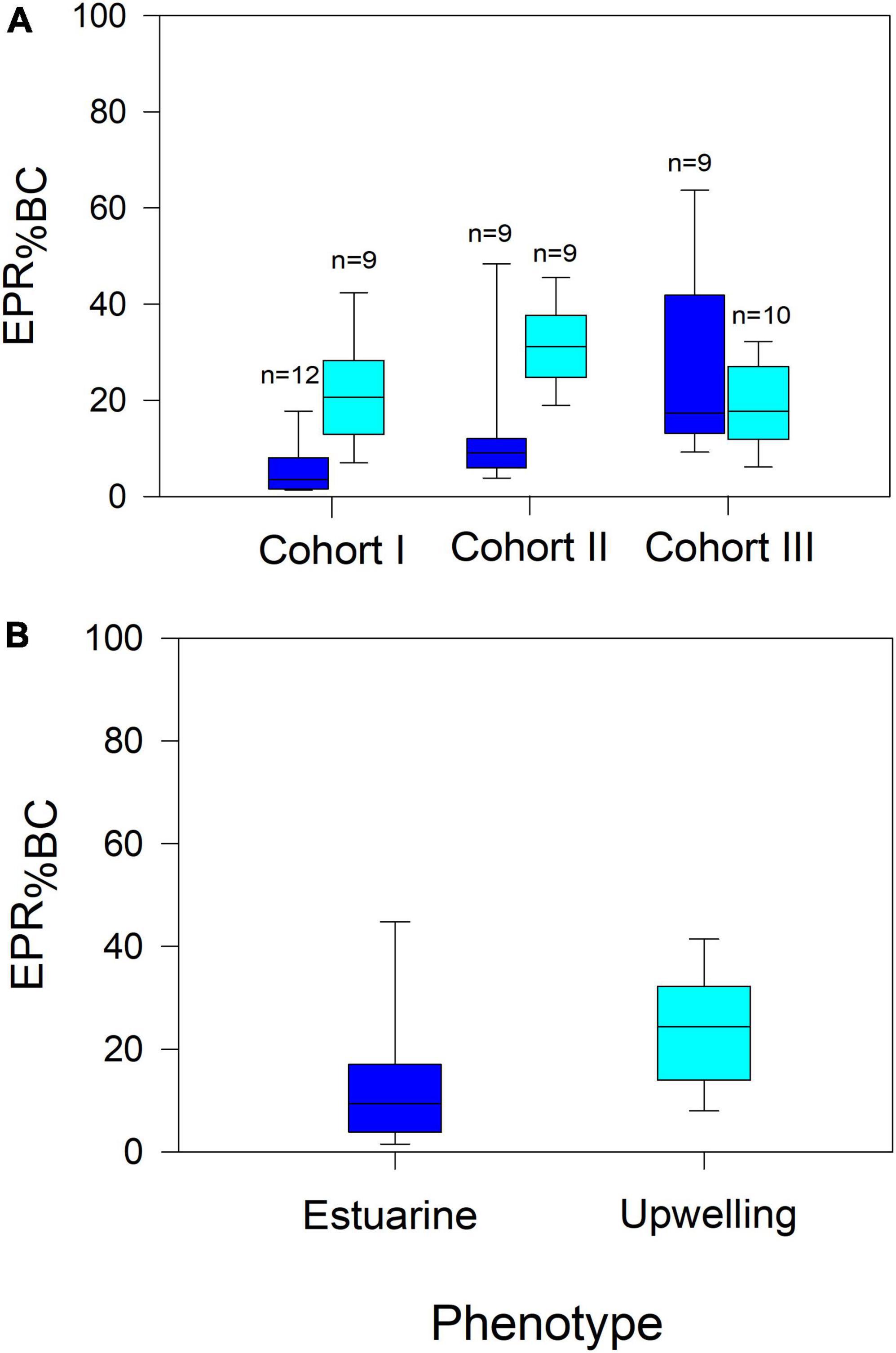
Figure 6. Among cohorts (A) and between populations (B) comparison of specific EPR (EPR%BC). Significant EPR%BC variations were found among cohorts of both populations, in which upwelling females produced comparatively more eggs per body carbon than estuarine females. n denotes the number of observations for each cohort.
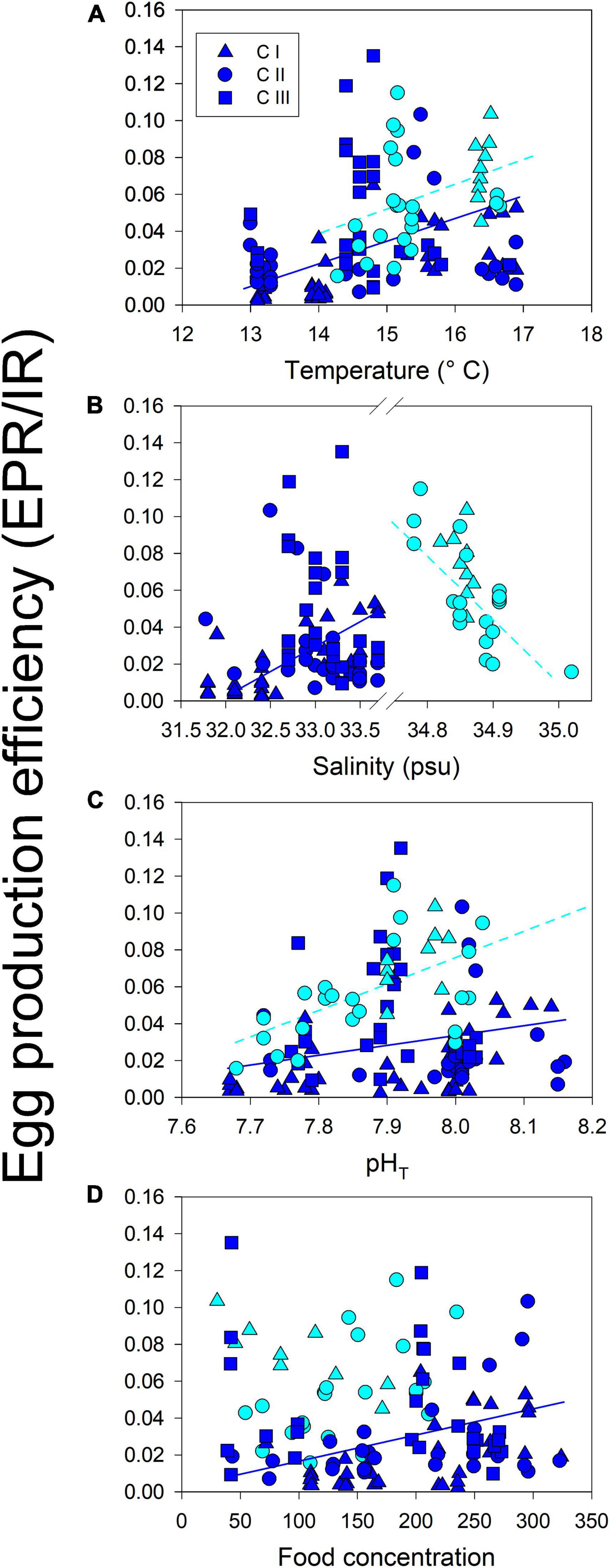
Figure 7. Phenotypic plasticity of EPE (EPR/IR quotient) to sampling temperature (A), salinity (B), pHT (C) and food (D) in each habitat. Plastic responses were expressed as a mean reaction norm that had a significantly non-zero slope. The slope denotes the EPE tolerance to the respective driver.
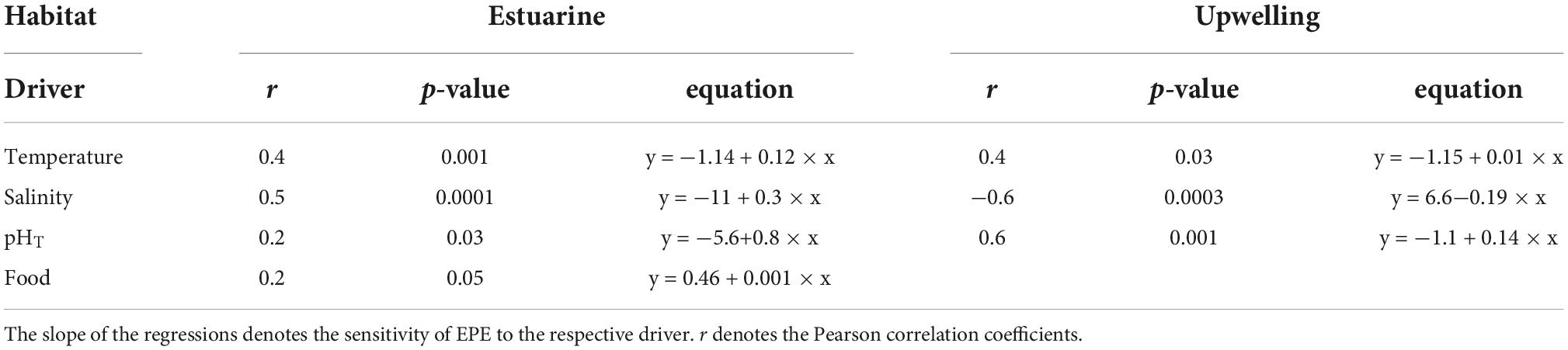
Table 4. Significant correlations (i.e., phenotypic plasticity) between EPE and environmental drivers for each population.
Discussion
This study comprehensively captured patterns of variability in the expression of parental and offspring traits within and among cohorts of A. tonsa populations sourced from latitudinal distant habitats located in contrasting climatic provinces alongshore the SEP. Understanding the variation in phenotypic plasticity within the species in the wild conferring the acclimation and adaptation to climate change provides central insights for evolutionary ecology (Porlier et al., 2012; Sasaki and Dam, 2020; Barley et al., 2021). One of the aspects is variability across different habitats as it provides cues on different habitat-specific variability that shapes different phenotypes. The information gathered in this study allowed us to compare the habitats’ variability manifested as mean values and environmental temporal heterogeneity, environmental–biological coupling, and its relationship with patterns of phenotypic plasticity in parental and offspring traits. In understanding and interpreting these results, contrasting life strategies related to the timing of occurrence to cope with seasonal (estuarine) or synoptic (upwelling) temporal variability affecting habitat conditions can be considered. Under such selective pressure(s), we focused on parental traits that largely predict tactical variation in offspring fitness among populations concerning the environmental quality or variability (Morrongiello et al., 2012 and references therein). The estuarine habitat located in the seasonal ocean seascape exhibited lower temporal variability in habitat drivers among cohorts of the local population. The relationship between habitat variability and related traits was higher in the estuarine habitat, suggesting how the extent of habitat variability impacts phenotypic plasticity. The phenotypic divergence was translated to cohort-related patterns of trait trade-offs regulating reproduction and population-specific tolerance to environmental changes. Current findings provide novel evidence of how divergent phenotypes might sustain the population of A. tonsa across variable coastal provinces of the SEP.
Characterization of cohorts and habitat variability
The assessment of discrete cohorts is well supported in the population inhabiting the seasonal estuarine habitat. Temperate populations exhibit seasonal–biological dynamics (Uye, 1982), and the estuarine population was sampled seasonally across different spring–summer periods from 2010 to 2012. At a mean habitat temperature of 14.6 ± 1.2°C, the local A. tonsa population should complete its ontogenetic development within 17–18 days and thus, several recruitment events can occur in the estuarine habitat during the seasonal reproductive cycle over approximately four months between the austral spring (September to October) and summer (December to February) period. In contrast, the upwelling population that reproduces year-round (Hidalgo and Escribano, 2007) was sampled throughout 2015. As with the most wild populations, age distribution among copepod cohorts might be stable or change over time (i.e., true cohort) (Landry, 1978; Durbin and Durbin, 1981). Copepod populations, including A. tonsa, grow in a temperature-dependent way in the permanent upwelling habitat (Hidalgo and Escribano, 2007). Furthermore, species of the genus Acartia can complete each molt-to-molt phase of the life cycle at a constant time (i.e., isochronal development), which depends on habitat temperature. At a mean temperature of 15.6 ± 0.8°C, the upwelling A. tonsa population should complete its life cycle within 17 days (Miller et al., 1977). Given the high likelihood of collecting females belonging to the same cohorts, we assigned Cohort I to those females collected at the beginning of 2015. Although upwelling-driven hydrographic variability prevailed throughout the study period, El Niño 2015 also impacted hydrographic conditions in the upwelling habitat during the fall–winter. In this sense, El Niño 2015 shifted the subsurface temperature range from 14.0°C to 15.3°C, prevailing because of the upwelling dynamics, to warmer (> 16°C) water under El Niño which separated the year into three different hydrographic regimes, each lasting around 2–3 months (Aguilera et al., 2019). By analyzing accumulated (over 4 consecutive sampling days) changes in the body size of reproducing females, we detected significant changes after periods of roughly 100 days matching the above described hydrographic regimes (Figure 2E). Although females belonging to subsequent cohorts (true cohorts) might have overlapped during consecutive samplings, the integrated analysis of changes in female body size allowed us to distinguish among different female cohorts, each exhibiting specific plasticity patterns related to a specific thermal experience. Indeed, females sampled during El Niño 2015 were relatively smaller than females sampled before and after El Niño.
Both estuarine and upwelling habitats were characterized by interdaily variability of the suite of environmental conditions, such as temperature, pH, salinity, and food availability (Henson et al., 2017). We acknowledge that our sampling might have underestimated higher frequency variability (i.e., hours-day) affecting physical–chemical conditions (see e.g., Hofmann et al., 2011. Bednarsšek et al., 2022). For short life-cycle copepods (i.e., month-months), this high-frequency variability might be critical in shaping the biological plasticity and tolerance to changing habitat conditions (Gaitán-Espitia et al., 2017; Vargas et al., 2017; Sasaki and Dam, 2020) and needs to be further assessed. In addition, there were clear differences in the temporal characterization of environmental variability between habitats. The estuarine habitat variability was characterized only during spring–summer periods, while the variability at the upwelling location was characterized throughout the annual cycle. This difference in habitat characterization might likely have underestimated the range of both environmental conditions and phenotypic plasticity within the estuarine population. However, the seasonal occurrence and biological productivity of the estuarine population are likely restricted to spring-summer periods, like the other mid-high latitude copepod species (Uye, 1982). In addition, copepod vertical migrations can extend the range of environmental conditions experienced within and among cohorts. Contrary to large-sized species, the vertical distribution of A. tonsa in coastal provinces of the SEP is restricted to the upper vertical layers. Within this range, constrained in the estuarine habitat by the surface freshwater lens (Osma et al., 2020), tidal currents (Vargas et al., 2003) in the estuarine habitats, and shallow oxygen-poor water in the upwelling habitat (Escribano et al., 2009); habitat and cohorts’ characterization were assessed in this study at a mean depth likely representing the most prevalent environmental conditions affecting cohorts’ traits (Supplementary Figure 2).
In the case of seasonal observations in the estuarine habitat, a similar thermal range (4°C) was reported during the seasonal reproductive occurrence of A. tonsa and A. hudsonica in Narragansett Bay (41° N) (Sullivan and McManus, 1986), and Oithona nana in temperate (39° S) coastal waters of Argentina (Temperoni et al., 2011). The subsurface (10 m depth) thermal range (14°C–17°C) observed in the upwelling habitat was consistent with the reported annual cycle achieved in the same site at a higher (i.e., weekly) frequency (Hidalgo et al., 2005), and a subtropical (20° S) upwelling loci in the northern Benguela upwelling system (Bode et al., 2014). In this sense, laboratory temperature was 1°C above and below the lower and upper edge, respectively, of the thermal range in the estuarine habitat, and 1°C below the upper edge in the upwelling habitat. However, these cases corresponded to only 14% (estuarine) and 6% (upwelling) of incubations, which might have introduced a negligible effect on estimations of copepod traits. All this suggests that the conducted estuarine characterization might have covered environmental variations experienced within generations. This is important when we consider the importance of fluctuating selection on genetic polymorphism of the local population (Sasaki and Dam, 2020).
Intrapopulation variability in traits and plasticity
There were significant variations in the expression of parental and offspring traits within cohorts of the estuarine population (Supplementary Figure 3) and among cohorts of the upwelling population (Table 2). Significant effects of single drivers on the expression of trait suggest there is an effect of plasticity, while the effect of habitat–environmental driver interactions indicates such plasticity diverged between populations (Table 3). In terms of environmental conditions, members of the same cohort experienced greater variations in the estuarine habitat, conditions that tended to prevail across the different cohorts. In turn, environmental conditions seem to be relatively more homogeneous during the development of a single cohort in the upwelling population, which, however, were environmentally decoupled from other cohorts. The divergent pattern in phenotypic plasticity between both A. tonsa populations can be related to genetic polymorphism among cohorts (Sasaki and Dam, 2020) and habitat variability (Pigliucci, 2005; Reed et al., 2010). For example, temperature and food abundance modulate phenotypic changes in the body size/weight of copepods (McLaren, 1963; Huntley and Lopez, 1992). Temperature and food abundance varied across the cohorts alongshore the body size of copepods in the upwelling habitat (Figure 3). In the upwelling seascape, changes in the body size of copepods have been related to temperature increases either experimentally (Escribano and McLaren, 1999) or in the field due to El Niño (Ulloa et al., 2001; Escribano et al., 2004). However, plasticity in morphometric traits was not related to habitat–temperature but habitat–salinity interaction, whereas physiological traits did relate to habitat-specific temperature conditions. Morphometric traits are settled in the early ontogeny of copepods in a tight relationship with habitat temperature (Miller et al., 1977), which might not necessarily correspond to the sampling temperature. Furthermore, the narrow temperature range associated with either seasonal thermal changes or vertical migrations over a restricted (30 m) depth range shaping the phenotypic plasticity (and genetic diversity) of the upwelling A. tonsa population was disrupted during this study by drastic thermal changes associated with El Niño 2015. Along with explaining the lack of correlation between variations in the expression of traits and habitat temperature, El Niño 2015 experienced among cohorts of the upwelling population could have changed the relative allele frequency and favored the selection of different alleles, for example, related to the phenotypic responses (Sasaki and Dam, 2020). Changes in the body size further translate to and influence the expression of reproductive traits (Durbin et al., 1983; Roff, 2002). In fact, EPR and egg size also varied significantly among cohorts in the upwelling population (Table 2). As the environment experienced by earlier generations can transgenerationally interact with the future generations, this can predetermine the phenotype, and thus related plasticity against future conditions (Donelson et al., 2018 and references therein); these findings suggest a reduced capacity in adult females for sensing habitat cues early in the season period, to better anticipate or fit their own and offspring traits through anticipatory maternal effects (Marshall et al., 2008). In the case of the estuarine population, the expression of body and egg size remained constant among cohorts while physiological EPR and IR traits varied among cohorts (Figure 2 and Table 2). The expression of these physiological traits is also strongly dependent on the food-driven phenotypic changes through food composition and quality (Kleppel and Burkart, 1995; Jónasdóttir et al., 2009). Although we did not evaluate changes in food quality, there were significant variations in food abundance among cohorts of the estuarine population, responding to the observed temporal and alongshore changes in the estuarine habitat (Aguilera et al., 2013; Osma et al., 2020). The relatively larger phenotypic plasticity observed in the expression of physiological traits might have rendered a reduced tolerance to environmental drivers (see below) in the estuarine population.
Variability in traits and plasticity between populations
The significant differences in the expression of parental and offspring traits were found between examined A. tonsa populations (Figure 4 and Table 2), overall, indicating variations in parental phenotypic plasticity (Burgess and Marshall, 2014). Adult females from the estuarine habitat were significantly larger than their upwelling counterparts, although the EPR of each population was similar. However, standardized by body size, the EPR%BC of upwelling was higher than that of estuarine females (Figure 6). This suggests that the upwelling population is allocating a greater proportion of their energy intake to reproduction. Furthermore, since female body size is positively correlated with the number of eggs produced (Durbin et al., 1983; Hopcroft and Roff, 1998), observed differences in body size and EPR%BC can have strong implications for population growth rates and ecosystem processes (Bassar et al., 2010; Gianuca et al., 2016). The slower growth in the colder estuarine environment, with no increment in fecundity related to the body size and lower gross reproductive output, might represent a handicap for the estuarine population (Stearns, 1989, 1992). This seems to be in part countered by a stronger phenological regulation manifested in higher fecundity in smaller females, as suggested by the trade-off between body size and EPR observed consistently among cohorts of the estuarine population (Figure 3A). The EPR varied within upwelling females without any obvious phenological cycles. Females from the upwelling habitat produced larger eggs (Table 2), which varied (trade-off) inversely with EPR in estuarine females (Figure 3B). Larger eggs in upwelling females cannot be explained phenologically as the upwelling females have a relatively smaller body size. Alternatively, the offspring size could evolve to reflect the optimal compromise between the positive fitness effects of increased size on offspring survival and the reduced/increased number of offspring produced (Smith and Fretwell, 1974; Brockelman, 1975). Increased EPR in smaller estuarine females might be related to elevated IR, which was phenotypically mediated by food variations in the estuarine habitat. Variation in habitat conditions can indeed determine phenotype variations among populations as demonstrated by the linear mixed-effects model (Table 3). In terms of fluctuations and correlations among points of a given variable, and between points of different variables in each habitat, the estuarine habitat seemed less variable with lower heterogeneity in the environmental conditions (Figure 5). In the estuarine habitat, the environmental drivers strongly covary, which was also reflected in strongly coupled environmental–biological variables (Figure 5), suggesting a significant role of habitat variability on phenotypic plasticity. Our current results in spatial patterns of variability in environmental conditions and reproduction-related traits agree that divergent phenotype variations, for example, parental anticipatory effects, take place in response to different environmental pressures alongshore climate-spatial gradients (Marshall et al., 2008; Burgess and Marshall, 2014).
Tolerance to habitat drivers between populations
Significant correlations found between EPE (EPR/IR quotient) and environmental conditions might correspond to the effect of simultaneous drivers on EPE (Figure 7). Building upon different and discrete cohorts of both A. tonsa populations, mechanistic relationships between EPE and environmental drivers can also reflect ecological specialization and local adaptation, for example, disentangling individual mechanisms that can be assessed later through multifactorial experimental approaches (Boyd et al., 2018). Furthermore, significant non-zero slopes indicate phenotypic plasticity in EPE and variation in the tolerance (sensitivity) to environmental changes. Both populations exhibited roughly the same tolerance to temperature which exerted positive effects on the EPE of both populations (Table 4). The mean temperature was higher at the upwelling habitat, whereas wider ranges and drastic thermal fluctuations were observed among cohorts of the estuarine population. Thermal fluctuations can strongly modulate selection on plasticity and thermal tolerance (Stillman, 2003; Sasaki and Dam, 2020), in which the mean temperature experienced by a population corresponds to tolerance, whereas phenotypic plasticity should evolve in response to temperature variability (Stevens, 1989). Salinity exerted population-specific effects on the EPE. The positive effect on the estuarine population might be related to the high natural variability of salinity in estuarine habitats (Kinne, 1966). In fact, elevated EPE levels were evident among cohorts of the local A. tonsa population when high salinity values prevailed. In contrast, the salinity range and fluctuations typically prevailing at the upwelling habitat were dramatically disrupted in timing and magnitude by the 2015 El Niño-related hydrographic shifts (Aguilera et al., 2020). Under upwelling influence, salinity in the upper water column varies over a narrow (0.01 psu) range forced by upwelling-driven replacement of water masses with slightly different salinity values (Silva et al., 2009). Larger (> 0.4 psu) salinity shifts were observed in the upwelling habitat due to El Niño (Aguilera et al., 2020) together with other unpredictable hydrographic changes significantly affecting the distribution and physiology of upwelling populations (Escribano et al., 2004; Riascos et al., 2009; Aguilera et al., 2019). Interestingly, both populations showed relatively similar tolerance to salinity and pHT. In the case of pHT, this promoted a positive effect on EPE of both populations, such that elevated EPE levels were evident when high pHT prevailed. The significant changes in pHT were observed within and among cohorts of both populations. However, phenotypic plasticity in physiological traits was higher in the estuarine population. These findings suggest that higher phenotypic plasticity can render the population less tolerant to changes in the environmental drivers (Stillman, 2003; Sasaki and Dam, 2019), which could decrease its overall adaptive variability (Leung et al., 2020).
Other potential factors structuring phenotypic plasticity
Our study is limited in the way that it only considers the impact of habitat variability in structuring phenotypic plasticity across two divergent habitats. However, we strongly emphasize that habitat-related parameters are only one aspect, while genetic structure, local adaptation, and gene flow include additional parameters that simultaneously impact the overall determination of the level of plasticity. A comprehensive interpretation of all observed parameters provides novel evidence of how divergent phenotypes might sustain the population of A. tonsa across variable coastal provinces of the SEP. Preliminary results based on (COI) mtDNA sequence data indicate the occurrence of different clades alongshore the SEP with higher haplotype diversity in the southern population, and the presence of a common haplotype with reduced frequency in the upwelling population (pers. work in progress). Ocean clines delimiting coastal provinces alongshore the SEP tend to act as effective dispersal barriers for populations of benthic species (Cardenas et al., 2009 and references therein). Comparatively, pelagic copepods exhibit higher dispersal potential that erodes ocean clines and manifested ecological speciation (Chen and Hare, 2008), preventing selection and local adaptation across large spatial scales in the presence of ocean connectivity. Despite evident differences in mean values and ranges of environmental conditions between the estuarine and upwelling habitat, patterns of phenotypic plasticity in the tolerance to environmental variations tended to overlap between populations. This might suggest there was or is a south-to-north gene flow which can posit some antagonist influence in the phenotypic plasticity and adaptive genetic differentiation between A. tonsa populations. Common garden experiments would help to clarify the role of habitat variability, genetic structure, and adaptations on phenotypic plasticity to fluctuations in other combined habitat drivers.
Conclusion
Our results underline the role of habitat-related variability between the estuarine and upwelling habitat toward shaping the distribution of the expression of trait and subsequent plasticity. The significant differences in patterns of environmental variability and phenotypic plasticity were found between both A. tonsa populations, in parental and offspring morphological and physiological traits, traits trade-offs regulating reproduction, and sensitivity to environmental drivers. Such differences in plasticity and tolerance related to temperature, pH and other ocean drivers are concerning if considering the species role in trophodynamics and biogeochemical cycles, and that both upwelling (Sydeman et al., 2014) and El Niño (Cai et al., 2021) intensity are projected to increase in the SEP due to global warming. Despite the absence of genetic evidence supporting the occurrence of various genotypes, our findings strongly indicate divergent A. tonsa phenotypes to be currently distributed across specific climate and ecogeographic provinces alongshore the SEP.
Data availability statement
Publicly available datasets were analyzed in this study. This data can be found here: doi: 10.1007/s12237-013-9615-2 and doi: 10.1594/PANGAEA.925450.
Author contributions
VA performed field campaigns and experiments. VA and NB analyzed and interpreted the data and led the writing of the manuscript. Both authors contributed critically to the drafts and gave final approval for publication.
Funding
VA was supported by the Chilean Scientific and Technologic Agency (ANID) through the Millennium Scientific Initiative Grant IC120019 and Proyecto ANILLOS ACT210071. NB was supported by the Slovene Research Agency (ARRS J1-2468 “Biomarkers of subcellular stress in the Northern Adriatic under global environmental change”).
Acknowledgments
We would like to thank Mr. Mauricio Vegas, Mr. Miguel Barrientos, Mr. Jose Martel, and Mr. Boris Aqueveque for their help during field surveys and experiments. We would also like to thank Paulo and Borja Aguilera for keeping hope of better times alive.
Conflict of interest
The authors declare that the research was conducted in the absence of any commercial or financial relationships that could be construed as a potential conflict of interest.
Publisher’s note
All claims expressed in this article are solely those of the authors and do not necessarily represent those of their affiliated organizations, or those of the publisher, the editors and the reviewers. Any product that may be evaluated in this article, or claim that may be made by its manufacturer, is not guaranteed or endorsed by the publisher.
Supplementary material
The Supplementary Material for this article can be found online at: https://www.frontiersin.org/articles/10.3389/fevo.2022.925648/full#supplementary-material
Footnotes
References
Aguilera, V. M., Donoso, K., and Escribano, R. (2011). Reproductive performance of small-sized dominant copepods with a highly variable food resource in the coastal upwelling system off the Chilean Humboldt Current. Mar. Biol. Res. 7, 235–249. doi: 10.1080/17451000.2010.499437
Aguilera, V. M., Vargas, C. A., Manríquez, P. H., Navarro, J. M., and Duarte, C. (2013). Low-pH freshwater discharges drive spatial and temporal variations in life history traits of neritic copepod Acartia tonsa. Estuaries Coasts 36, 1084–1092. doi: 10.1007/s12237-013-9615-2
Aguilera, V. M., Escribano, R., Vargas, C. A., and González, M. T. (2019). Upwelling modulation of functional traits of a dominant planktonic grazer during “warm-acid” El Niño 2015 in a year-round upwelling area of Humboldt Current. PLoS One 14:e0209823. doi: 10.1371/journal.pone.0209823
Aguilera, V. M., Vargas, C. A., and Dewitte, B. (2020). Intraseasonal hydrographic variations and nearshore carbonates system off northern Chile during the 2015 El Niño event. J. Geophys. Res. Biogeosci. 125:e2020JG005704. doi: 10.1029/2020JG005704
Aguirre, C., Garreaud, R., Belmar, L., Farías, L., Ramajo, L., and Barrera, F. (2021). High-frequency variability of the surface ocean properties off central Chile during the upwelling season. Front. Mar. Sci. 8:702051. doi: 10.3389/fmars.2021.702051
Aguirre, G. E., Capitanio, F. L., Lovrich, G. A., and Esnal, G. B. (2012). Seasonal variability of metazooplankton in coastal sub-Antarctic waters (Beagle Channel). Mar. Biol. Res. 8, 341–353. doi: 10.1080/17451000.2011.627922
Barley, J. M., Cheng, B. S., Sasaki, M., Gignoux-Wolfsohn, S., Hays, C. G., Putnam, A. B., et al. (2021). Limited plasticity in thermally tolerant ectotherm populations: evidence for a trade-off. Proc. R. Soc. B 288:20210765. doi: 10.1098/rspb.2021.0765
Bassar, R. D., Marshall, M. C., López-Sepulcre, A., Zandonà, E., Auer, S. K., Travis, J., et al. (2010). Local adaptation in Trinidadian guppies alters ecosystem processes. Proc. Natl. Acad. Sci. U S A. 107, 3616–3621. doi: 10.1073/pnas.0908023107
Bednarsšek, N., Beck, M. W., Pelletier, G., Applebaum, S. L., Feely, R. A., Butler, R., et al. (2022). Natural analogues in pH variability and predictability across the coastal pacific estuaries: extrapolation of the increased oyster dissolution under increased pH amplitude and low predictability related to ocean acidification. Environ. Sci. Technol. 56, 9015–9028. doi: 10.1021/acs.est.2c00010
Bernhardt, J. R., O’Connor, M. I., Sunday, J. M., and Gonzalez, A. (2020). Life in fluctuating environments. Philos. Trans. R. Soc. B 375:20190454. doi: 10.1098/rstb.2019.0454
Bitter, M. C., Kapsenberg, L., Gattuso, J. P., and Pfister, C. A. (2019). Standing genetic variation fuels rapid adaptation to ocean acidification. Nat. Commun. 10:5821. doi: 10.1038/s41467-019-13767-1
Bitter, M. C., Kapsenberg, L., Silliman, K., Gattuso, J. P., and Pfister, C. A. (2021). Magnitude and predictability of pH fluctuations shape plastic responses to ocean acidification. Am. Nat. 197, 486–501. doi: 10.1086/712930
Bode, M., Kreiner, A., van der Plas, A. K., Louw, D. C., Horaeb, R., Auel, H., et al. (2014). Spatio-temporal variability of copepod abundance along the 20° S monitoring transect in the northern Benguela upwelling system from 2005 to 2011. PLoS One 9:e97738. doi: 10.1371/journal.pone.0097738
Boyd, P. W., Collins, S., Dupont, S., Fabricius, K., Gattuso, J. P., Havenhand, J., et al. (2018). Experimental strategies to assess the biological ramifications of multiple drivers of global ocean change—a review. Global Change Biol. 24, 2239–2261. doi: 10.1111/gcb.14102
Brennan, R. S., Garrett, A. D., Huber, K. E., Hargarten, H., and Pespeni, M. H. (2019). Rare genetic variation and balanced polymorphisms are important for survival in global change conditions. Proc. R. Soc. B 286:20190943. doi: 10.1098/rspb.2019.0943
Brockelman, W. Y. (1975). Competition, the fitness of offspring, and optimal clutch size. Am. Nat. 109, 677–699. doi: 10.1086/283037
Burgess, S. C., and Marshall, D. J. (2014). Adaptive parental effects: the importance of estimating environmental predictability and offspring fitness appropriately. Oikos 123, 769–776. doi: 10.1111/oik.01235
Cai, W., Santoso, A., Collins, M., Dewitte, B., Karamperidou, C., Kug, J. S., et al. (2021). Changing El Niño-Southern Oscillation in a warming climate. Nat. Rev. Earth Environ. 2, 628–644. doi: 10.1038/s43017-021-00199-z
Camus, P. A. (2001). Biogeografía marina de Chile continental. Rev. Chilena Historia Nat. 74, 587–617. doi: 10.4067/S0716-078X2001000300008
Cardenas, L., Castilla, J. C., and Viard, F. (2009). A phylogeographical analysis across three biogeographical provinces of the south-eastern Pacific: the case of the marine gastropod Concholepas concholepas. J. Biogeography 36, 969–981. doi: 10.1111/j.1365-2699.2008.02056.x
Chaalali, A., Beaugrand, G., Raybaud, V., Goberville, E., David, V., Boët, P., et al. (2013). Climatic facilitation of the colonization of an estuary by Acartia tonsa. PLoS One 8:e74531. doi: 10.1371/journal.pone.0074531
Chen, G., and Hare, M. P. (2008). Cryptic ecological diversification of a planktonic estuarine copepod. Acartia tonsa. Mol. Ecol. 17, 1451–1468. doi: 10.1111/j.1365-294X.2007.03657.x
Dam, H. G. (2013). Evolutionary adaptation of marine zooplankton to global change. Ann. Rev. Mar. Sci. 5, 349–370. doi: 10.1146/annurev-marine-121211-172229
Donelson, J. M., Salinas, S., Munday, P. L., and Shama, L. (2018). Transgenerational plasticity and climate change experiments: where do we go from here? Global Change Biol. 24, 13–34. doi: 10.1111/gcb.13903
Dunson, W. A., and Travis, J. (1991). The role of abiotic factors in community organization. Am. Nat. 138, 1067–1091. doi: 10.1086/285270
Durbin, A. G., and Durbin, E. G. (1981). Standing stock and estimated production rates of phytoplankton and zooplankton in Narragansett Bay, Rhode Island. Estuaries 4, 24–41. doi: 10.2307/1351540
Durbin, E. G., Durbin, A. G., S-mayda, T. J., and Verity, P. G. (1983). Food limitation of production by adult Acartia tonsa in Narragansett Bay, Rhode Island. Limnol. Oceanography 28, 1199–1213. doi: 10.4319/lo.1983.28.6.1199
Escribano, R., Daneri, G., Farías, L., Gallardo, V. A., González, H. E., Gutiérrez, D., et al. (2004). Biological and chemical consequences of the 1997–1998 El Niño in the Chilean coastal upwelling system: a synthesis. Deep Sea Res. Part II: Top. Stud. Oceanography 51, 2389–2411. doi: 10.1016/j.dsr2.2004.08.011
Escribano, R., Fernández, M., and Aranís, A. (2003). Physical-chemical processes and patterns of diversity of the Chilean eastern boundary pelagic and benthic marine ecosystems: an overview. Gayana (Concepción) 67, 190–205. doi: 10.4067/S0717-65382003000200008
Escribano, R., Hidalgo, P., and Krautz, C. (2009). Zooplankton associated with the oxygen minimum zone system in the northern upwelling region of Chile during march 2000. Deep Sea Res. Part II: Top. Stud. Oceanography 56, 1083–1094. doi: 10.1016/j.dsr2.2008.09.009
Escribano, R., Irribarren, C., and Rodriguez, L. (1997). Influence of food quantity and temperature on development and growth of the marine copepod Calanus chilensis from northern Chile. Mar. Biol. 128, 281–288. doi: 10.1007/s002270050093
Escribano, R., and McLaren, I. (1999). Production of Calanus chilensis in the upwelling area of Antofagasta, northern Chile. Mar. Ecol. Prog. Series 177, 147–156. doi: 10.3354/meps177147
Frost, B. W. (1972). Effects of size and concentration of food particles on the feeding behavior of the marine planktonic copepod Calanus pacificus. Limnol. Oceanography 17, 805–815. doi: 10.4319/lo.1972.17.6.0805
Gaitán-Espitia, J. D., Marshall, D., Dupont, S., Bacigalupe, L. D., Bodrossy, L., and Hobday, A. J. (2017). Geographical gradients in selection can reveal genetic constraints for evolutionary responses to ocean acidification. Biol. Lett. 13:20160784. doi: 10.1098/rsbl.2016.0784
Garcés-Vargas, J., Schneider, W., Pinochet, A., Piñones, A., Olguin, F., Brieva, D., et al. (2020). Tidally forced saltwater intrusions might impact the quality of drinking water, the Valdivia River (40° S), Chile estuary case. Water 12:2387. doi: 10.3390/w12092387
García-Reyes, M., Largier, J. L., and Sydeman, W. J. (2014). Synoptic-scale upwelling indices and predictions of phyto- and zooplankton populations. Prog. Oceanography 120, 177–188. doi: 10.1016/j.pocean.2013.08.004
Gianuca, A. T., Pantel, J. H., and De Meester, L. (2016). Disentangling the effect of body size and phylogenetic distances on zooplankton top-down control of algae. Proc. R. Soc. B: Biol. Sci. 283:20160487. doi: 10.1098/rspb.2016.0487
Hartley, A. J., Chong, G., Houston, J., and Mather, A. E. (2005). 150 Million years of climatic stability: evidence from the Atacama Desert, northern Chile. J. Geol. Soc. 162, 421–424. doi: 10.1144/0016-764904-071
Henson, S. A., Beaulieu, C., Ilyina, T., John, J. G., Long, M., Séférian, R., et al. (2017). Rapid emergence of climate change in environmental drivers of marine ecosystems. Nat. Commun. 8:14682. doi: 10.1038/ncomms14682
Hidalgo, P., and Escribano, R. (2007). Coupling of life cycles of the copepods Calanus chilensis and Centropages brachiatus to upwelling induced variability in the central-southern region of Chile. Prog. Oceanography 75, 501–517. doi: 10.1016/j.pocean.2007.08.028
Hidalgo, P., Escribano, R., and Morales, C. E. (2005). Ontogenetic vertical distribution and diel migration of the copepod Eucalanus inermis in the oxygen minimum zone off northern Chile (20–21° S). J. Plankton. Res. 27, 519–529. doi: 10.1093/plankt/fbi025
Hofmann, G. E., Smith, J. E., Johnson, K. S., Send, U., Levin, L. A., Micheli, F., et al. (2011). High-frequency dynamics of ocean pH: a multi-ecosystem comparison. PLoS One 6:e28983. doi: 10.1371/journal.pone.0028983
Hopcroft, R. R., and Roff, J. C. (1998). Zooplankton growth rates: the influence of female size and resources on egg production of tropical marine copepods. Mar. Biol. 132, 79–86. doi: 10.1007/s002270050373
Huntley, M., and Lopez, M. (1992). Temperature-dependent production of marine copepods: a global synthesis. Am. Nat. 140, 201–242. doi: 10.1086/285410
Jónasdóttir, S. H., Visser, A. W., and Jespersen, C. (2009). Assessing the role of food quality in the production and hatching of Temora longicornis eggs. Mar. Ecol. Prog. Series 382, 139–150. doi: 10.3354/meps07985
Kingsolver, J. G., and Huey, R. B. (1998). Evolutionary analyses of morphological and physiological plasticity in thermally variable environments. Am. Zool. 38, 545–560. doi: 10.1093/icb/38.3.545
Kinne, O. (1966). Physiological aspects of animal life in estuaries with special reference to salinity. Netherlands J. Sea Res. 3, 222–244. doi: 10.1016/0077-7579(66)90013-5
Kiørboe, T., Møhlenberg, F., and Nicolajsen, H. (1982). Ingestion rate and gut clearance in the planktonic copepod Centropages hamatus (Lilljeborg) in relation to food concentration and temperature. Ophelia 21, 181–194. doi: 10.1080/00785326.1982.10426586
Kiørboe, T., and Nielsen, T. G. (1994). Regulation of zooplankton biomass and production in a temperate, coastal ecosystem. 1. copepods. Limnol. Oceanography 39, 493–507. doi: 10.4319/lo.1994.39.3.0493
Kleppel, G. S., and Burkart, C. A. (1995). Egg production and the nutritional environment of Acartia tonsa: the role of food quality in copepod nutrition. ICES J. Mar. Sci. 52, 297–304. doi: 10.1016/1054-3139(95)80045-X
Landry, M. R. (1978). Population dynamics and production of a planktonic marine copepod, Acartia clausii, in a small temperate lagoon on San Juan Island, Washington. Int. Rev. Gesamten Hydrobiol. Hydrographie 63, 77–119. doi: 10.1002/iroh.19780630106
Langerhans, R. B. (2018). Predictability and parallelism of multitrait adaptation. J. Heredity 109, 59–70. doi: 10.1093/jhered/esx043
Lercari, D., and Defeo, O. (2006). Large-scale diversity and abundance trends in sandy beach macrofauna along full gradients of salinity and morphodynamics. Estuarine Coastal Shelf Sci. 68, 27–35. doi: 10.1016/j.ecss.2005.12.017
Leung, C., Rescan, M., Grulois, D., and Chevin, L. M. (2020). Reduced phenotypic plasticity evolves in less predictable environments. Ecol. Lett. 23, 1664–1672. doi: 10.1111/ele.13598
Lindberg, D. R. (1991). Marine biotic interchange between the northern and southern hemispheres. Paleobiology 17, 308–324. doi: 10.1017/S0094837300010629
Marín, V., Huntley, M. E., and Frost, B. (1986). Measuring feeding rates of pelagic herbivores: analysis of experimental design and methods. Mar. Biol. 93, 49–58. doi: 10.1007/BF00428654
Marshall, D. J., Allen, R. M., and Crean, A. J. (2008). The ecological and evolutionary importance of maternal effects in the sea. Oceanography Mar. Biol. Ann. Rev. 46, 203–250. doi: 10.1201/9781420065756.ch5
McLaren, I. A. (1963). Effects of temperature on growth of zooplankton, and the adaptive value of vertical migration. J. Fisheries Board Canada 20, 685–727. doi: 10.1139/f63-046
Miller, C. B., Johnson, J. K., and Heinle, D. R. (1977). Growth rules in the marine copepod genus Acartia. Limnol. Oceanography 22, 326–335. doi: 10.4319/lo.1977.22.2.0326
Moksness, E., and Fossum, P. (1992). Daily growth rate and hatching-date distribution of Norwegian spring-spawning herring (Clupea harengus L.). ICES J. Mar. Sci. 49, 217–221. doi: 10.1093/icesjms/49.2.217
Moran, N. A. (1992). The evolutionary maintenance of alternative phenotypes. Am. Nat. 139, 971–989. doi: 10.1086/285369
Morrongiello, J. R., Bond, N. R., Crook, D. A., and Wong, B. B. (2012). Spatial variation in egg size and egg number reflects trade-offs and bet-hedging in a freshwater fish. J. Animal Ecol. 81, 806–817. doi: 10.1111/j.1365-2656.2012.01961.x
Oerder, V., Colas, F., Echevin, V., Codron, F., Tam, J., and Belmadani, A. (2015). Peru-Chile upwelling dynamics under climate change. J. Geophys. Res. Oceans 120, 1152–1172. doi: 10.1002/2014JC010299
Ortega-Mayagoitia, E., Hernández-Martínez, O., and Ciros-Pérez, J. (2018). Phenotypic plasticity of life-history traits of a calanoid copepod in a tropical lake: is the magnitude of thermal plasticity related to thermal variability? PLoS One 13:e0196496. doi: 10.1371/journal.pone.0196496
Osma, N., Latorre-Melín, L., Jacob, B., Contreras, P. Y., von Dassow, P., and Vargas, C. A. (2020). Response of phytoplankton assemblages from naturally acidic coastal ecosystems to elevated pCO2. Front. Mar. Sci. 7:323. doi: 10.3389/fmars.2020.00323
Paffenhöfer, G. A., and Stearns, D. E. (1988). Why is Acartia tonsa (Copepoda: Calanoida) restricted to nearshore environments? Mar. Ecol. Prog. Series 42, 33–38. doi: 10.3354/meps042033
Pereira, R. J., Sasaki, M. C., and Burton, R. S. (2017). Adaptation to a latitudinal thermal gradient within a widespread copepod species: the contributions of genetic divergence and phenotypic plasticity. Proc. R. Soc. B: Biol. Sci. 284:20170236. doi: 10.1098/rspb.2017.0236
Pérez, C. A., DeGrandpre, M. D., Lagos, N. A., Saldías, G. S., Cascales, E. K., and Vargas, C. A. (2015). Influence of climate and land use in carbon biogeochemistry in lower reaches of rivers in central southern Chile: implications for the carbonate system in river-influenced rocky shore environments. J. Geophys. Res. Biogeosci. 120, 673–692. doi: 10.1002/2014JG002699
Peterson, W. T., Arcos, D. F., McManus, G. B., Dam, H., Bellantoni, D., Johnson, T., et al. (1988). The nearshore zone during coastal upwelling: daily variability and coupling between primary and secondary production off central Chile. Prog. Oceanography 20, 1–40. doi: 10.1016/0079-6611(88)90052-3
Pianka, E. R. (1976). Natural selection of optimal reproductive tactics. Am. Zool. 16, 775–784. doi: 10.1093/icb/16.4.775
Pierrot, D., Epitalon, J.-M., Orr, J. C., Lewis, E., and Wallace, D. W. R. (2021). MS Excel program developed for CO2 system calculations. Available online at: https://github.com/dpierrot/co2sys_xl
Pigliucci, M. (2001). Phenotypic Plasticity: Beyond Nature and Nurture. Baltimore, MD: JHU Press. doi: 10.1093/oso/9780195131543.003.0009
Pigliucci, M. (2005). Evolution of phenotypic plasticity: Where are we going now? Trends Ecol. Evol. 20, 481–486. doi: 10.1016/j.tree.2005.06.001
Pino, M. Q., Perillo, G. M. E., and Santamarina, P. (1994). Residual fluxes in a cross-section of the Valdivia river estuary, Chile. Estuarine Coastal Shelf Sci. 38, 491–505. doi: 10.1006/ecss.1994.1034
Porlier, M., Charmantier, A., Bourgault, P., Perret, P., Blondel, J., and Garant, D. (2012). Variation in phenotypic plasticity and selection patterns in blue tit breeding time: between-and within-population comparisons. J. Animal Ecol. 81, 1041–1051. doi: 10.1111/j.1365-2656.2012.01996.x
Pörtner, H. O., Langenbuch, M., and Reipschläger, A. (2004). Biological impact of elevated ocean CO2 concentrations: lessons from animal physiology and earth history. J. Oceanography 60, 705–718. doi: 10.1007/s10872-004-5763-0
Reed, T. E., Waples, R. S., Schindler, D. E., Hard, J. J., and Kinnison, M. T. (2010). Phenotypic plasticity and population viability: the importance of environmental predictability. Proc. R. Soc. B: Biol. Sci. 277, 3391–3400. doi: 10.1098/rspb.2010.0771
Riascos, J. M., Carstensen, D., Laudien, J., Arntz, W. E., Oliva, M. E., Güntner, A., et al. (2009). Thriving and declining: climate variability shaping life-history and population persistence of Mesodesma donacium in the Humboldt Upwelling System. Mar. Ecol. Prog. Series 385, 151–163. doi: 10.3354/meps08042
Roff, D. A. (2002). Life History Evolution. Sunderland: Sinauer Associates. doi: 10.1016/B978-0-12-384719-5.00087-3
Ruz, P. M., Hidalgo, P., Yáñez, S., Escribano, R., and Keister, J. E. (2015). Egg production and hatching success of Calanus chilensis and Acartia tonsa in the northern Chile upwelling zone (23 S), Humboldt Current System. J. Mar. Systems 148, 200–212. doi: 10.1016/j.jmarsys.2015.03.007
Sasaki, M. C., and Dam, H. G. (2019). Integrating patterns of thermal tolerance and phenotypic plasticity with population genetics to improve understanding of vulnerability to warming in a widespread copepod. Global Change Biol. 25, 4147–4164. doi: 10.1111/gcb.14811
Sasaki, M. C., and Dam, H. G. (2020). Genetic differentiation underlies seasonal variation in thermal tolerance, body size, and plasticity in a short-lived copepod. Ecol. Evol. 10, 12200–12210. doi: 10.1002/ece3.6851
Sasaki, M. C., and Dam, H. G. (2021). Negative relationship between thermal tolerance and plasticity in tolerance emerges during experimental evolution in a widespread marine invertebrate. Evol. Appl. 14, 2114–2123. doi: 10.1111/eva.13270
Schlichting, C., and Pigliucci, M. (1998). Phenotypic Evolution: a Reaction Norm Perspective. Wallingford: CABI.
Senner, N. R., Conklin, J. R., and Piersma, T. (2015). An ontogenetic perspective on individual differences. Proc. R. Soc. B: Biol. Sci. 282:20151050. doi: 10.1098/rspb.2015.1050
Silva, N., Rojas, N., and Fedele, A. (2009). Water masses in the Humboldt Current System: Properties, distribution, and the nitrate deficit as a chemical water mass tracer for Equatorial Subsurface Water off Chile. Deep Sea Res. II Top. Stud. Oceanogr. 56, 1004–1020. doi: 10.1016/j.dsr2.2008.12.013
Smith, C. C., and Fretwell, S. D. (1974). The optimal balance between size and number of offspring. Am. Nat. 108, 499–506. doi: 10.1086/282929
Song, H., Miller, A. J., Cornuelle, B. D., and Di Lorenzo, E. (2011). Changes in upwelling and its water sources in the California current system driven by different wind forcing. Dyn. Atmospheres Oceans 52, 170–191.
Stearns, S. C. (1989). The evolutionary significance of phenotypic plasticity. Bioscience 39, 436–445. doi: 10.2307/1311135
Stevens, G. (1989). The latitudinal gradient in geographical range: how so many species coexist in the tropics. Am. Nat. 133, 240–256. doi: 10.1086/284913
Stillman, J. H. (2003). Acclimation capacity underlies susceptibility to climate change. Science 301:65. doi: 10.1126/science.1083073
Strickland, J. D. H., and Parsons, T. R. (1972). A Practical Handbook of Seawater Analysis. Ottawa: Fisheries Research Board of Canada.
Strub, P. T., Mesías, J. M., Montecino, V., Rutlant, J., and Salinas, S. (1998). Coastal ocean circulation off western South America. Coast. Segment 273–313.
Sullivan, B. K., and McManus, L. T. (1986). Factors controlling seasonal succession of the copepods Acartia hudsonica and A. tonsa in Narragansett Bay, Rhode Island: temperature and resting egg production. Mar. Ecol. Prog. Ser. 28, 121–128. doi: 10.3354/meps028121
Sunday, J. M., Crim, R. N., Harley, C. D., and Hart, M. W. (2011). Quantifying rates of evolutionary adaptation in response to ocean acidification. PLoS One 6:e22881. doi: 10.1371/journal.pone.0022881
Sydeman, W. J., García-Reyes, M., Schoeman, D. S., Rykaczewski, R. R., Thompson, S. A., Black, B. A., et al. (2014). Climate change and wind intensification in coastal upwelling ecosystems. Science 345, 77–80. doi: 10.1126/science.1251635
Tapia, F. J., Largier, J. L., Castillo, M., Wieters, E. A., and Navarrete, S. A. (2014). Latitudinal discontinuity in thermal conditions along the nearshore of central-northern Chile. PLoS One 9:e110841. doi: 10.1371/journal.pone.0110841
Tapia, F. J., Navarrete, S. A., Castillo, M., Menge, B. A., Castilla, J. C., Largier, J., et al. (2009). Thermal indices of upwelling effects on inner-shelf habitats. Prog. Oceanography 83, 278–287. doi: 10.1016/j.pocean.2009.07.035
Temperoni, B., Viñas, M. D., Diovisalvi, N., and Negri, R. (2011). Seasonal production of Oithona nana Giesbrecht, 1893 (Copepoda: Cyclopoida) in temperate coastal waters off Argentina. J. Plankton Res. 33, 729–740. doi: 10.1093/plankt/fbq141
Torres, R., Turner, D. R., Silva, N., and Rutllant, J. (1999). High short-term variability of CO2 fluxes during an upwelling event off the Chilean coast at 30 S. Deep Sea Res. Part I: Oceanographic Res. Papers 46, 1161–1179. doi: 10.1016/S0967-0637(99)00003-5
Ulloa, O., Escribano, R., Hormazabal, S., Quinones, R. A., González, R. R., and Ramos, M. (2001). Evolution and biological effects of the 1997–98 El Niño in the upwelling ecosystem off northern Chile. Geophys. Res. Lett. 28, 1591–1594. doi: 10.1029/2000GL011548
Uye, S. I. (1982). Length-weight relationships of important zooplankton from the Inland Sea of Japan. J. Oceanograph. Soc. Japan 38, 149–158. doi: 10.1007/BF02110286
Vargas, C. A., Araneda, S. E., and Valenzuela, G. (2003). Influence of tidal phase and circulation on larval fish distribution in a partially mixed estuary, Corral Bay, Chile. J. Mar. Biol. Assoc. U K 83, 217–222. doi: 10.1017/S0025315403006994h
Vargas, C. A., Contreras, P. Y., Pérez, C. A., Sobarzo, M., Saldías, G. S., and Salisbury, J. (2016). Influences of riverine and upwelling waters on the coastal carbonate system off central Chile and their ocean acidification implications. J. Geophys. Res. Biogeosci. 121, 1468–1483. doi: 10.1002/2015JG003213
Vargas, C. A., Cuevas, L. A., Broitman, B. R., San Martin, V. A., Lagos, N. A., Gaitán-Espitia, J. D., et al. (2022). Upper environmental pCO2 drives sensitivity to ocean acidification in marine invertebrates. Nat. Clim. Chang. 12, 200–207. doi: 10.1038/s41558-021-01269-2
Vargas, C. A., and González, H. E. (2004). Plankton community structure and carbon cycling in a coastal upwelling system. I. Bacteria, microprotozoans and phytoplankton in the diet of copepods and appendicularians. Aquatic Microbial Ecol. 34, 151–164. doi: 10.3354/ame034151
Vargas, C. A., Lagos, N. A., Lardies, M. A., Duarte, C., Manríquez, P. H., Aguilera, V. M., et al. (2017). Species-specific responses to ocean acidification should account for local adaptation and adaptive plasticity. Nat. Ecol. Evol. 1:84. doi: 10.1038/s41559-017-0084
Vargas, C. A., Martínez, R. A., Cuevas, L. A., Pavez, M. A., Cartes, C., González, H. E., et al. (2007). The relative importance of microbial and classical food webs in a highly productive coastal upwelling area. Limnol. Oceanography 52, 1495–1510. doi: 10.4319/lo.2007.52.4.1495
Keywords: coastal ocean, environmental variability, latitudinal gradient, phenotypic plasticity, copepod
Citation: Aguilera VM and Bednaršek N (2022) Variations in phenotypic plasticity in a cosmopolitan copepod species across latitudinal hydrographic gradients. Front. Ecol. Evol. 10:925648. doi: 10.3389/fevo.2022.925648
Received: 21 April 2022; Accepted: 20 September 2022;
Published: 20 October 2022.
Edited by:
Diana Sofia Madeira, University of Aveiro, PortugalReviewed by:
Matthew Sasaki, University of Connecticut, United StatesAnu Vehmaa, Åbo Akademi University, Finland
Copyright © 2022 Aguilera and Bednaršek. This is an open-access article distributed under the terms of the Creative Commons Attribution License (CC BY). The use, distribution or reproduction in other forums is permitted, provided the original author(s) and the copyright owner(s) are credited and that the original publication in this journal is cited, in accordance with accepted academic practice. No use, distribution or reproduction is permitted which does not comply with these terms.
*Correspondence: Victor M. Aguilera, dmljdG9yLmFndWlsZXJhQGNlYXphLmNs
†ORCID: Victor M. Aguilera, orcid.org/0000-0001-5791-5250; Nina Bednaršek, orcid.org/0000-0002-9177-6626