- 1Department of Ichthyology, American Museum of Natural History, New York, NY, United States
- 2Museu de Zoologia, Universidade de São Paulo, São Paulo, Brazil
- 3Programa de Coleções e Acervos Científicos, Instituto Nacional de Pesquisas da Amazônia, Manaus, Brazil
- 4Coordenação de Biodiversidade, Instituto Nacional de Pesquisas da Amazônia, Manaus, Brazil
- 5Instituto de Biociências, Universidade Estadual Paulista, Botucatu, Brazil
Tarumania walkerae is a rare fossorial freshwater fish species from the lower Rio Negro, Central Amazonia, composing the monotypic and recently described family Tarumaniidae. The family has been proposed as the sister group of Erythrinidae by both morphological and molecular studies despite distinct arrangements of the superfamily Erythrinoidea within Characiformes. Recent phylogenomic studies and time-calibrated analyses of characoid fishes have not included specimens of Tarumania in their analyses. We obtained genomic data for T. walkerae and constructed a phylogeny based on 1795 nuclear loci with 488,434 characters of ultraconserved elements (UCEs) for 108 terminals including specimens of all 22 characiform families. The phylogeny confirms the placement of Tarumaniidae as sister to Erythrinidae but differs from the morphological hypothesis in the placement of the two latter families as sister to the clade with Hemiodontidae, Cynodontidae, Serrasalmidae, Parodontidae, Anostomidae, Prochilodontidae, Chilodontidae, and Curimatidae. The phylogeny calibrated with five characoid fossils indicates that Erythrinoidea diverged from their relatives during the Late Cretaceous circa 90 Ma (108–72 Ma), and that Tarumania diverged from the most recent common ancestor of Erythrinidae during the Paleogene circa 48 Ma (66–32 Ma). The occurrence of the erythrinoid-like †Tiupampichthys in the Late Cretaceous–Paleogene formations of the El Molino Basin of Bolivia supports our hypothesis for the emergence of the modern Erythrinidae and Tarumaniidae during the Paleogene.
Introduction
The ostariophysan order Characiformes constitutes an extremely diverse assemblage with approximately 2,200 species and 22 families of freshwater characins, piranhas, tetras, and related fishes living in tropical ecosystems of Africa and the Neotropics (Nelson, 2006; Fricke et al., 2021). Phylogenetic relationships within the Characiformes have been assessed by morphological, multilocus, and phylogenomic approaches with often-conflicting arrangements among and within families (Oliveira et al., 2011; de Pinna et al., 2018; Betancur-R et al., 2019; Melo et al., 2022). One major point of incongruence is the placement of Citharinidae and Distichodontidae, also recognized as Cithariniformes (Mirande, 2017; Dornburg and Near, 2021) inside or outside Characiformes (Arcila et al., 2017; Chakrabarty et al., 2017; Betancur-R et al., 2019; Faircloth et al., 2020; Melo et al., 2022). Another major issue is the resolution of the superfamily Erythrinoidea, in which the morphological analyses often group Ctenoluciidae, Erythrinidae, Hepsetidae, and Lebiasinidae (Buckup, 1998; de Pinna et al., 2018; Pastana et al., 2020). Molecular data, however, have consistently resolved Erythrinidae as closer to Serrasalmidae, Cynodontidae, Hemiodontidae, Parodontidae, and Anostomoidea (Arcila et al., 2017; Betancur-R et al., 2019; Faircloth et al., 2020; Melo et al., 2022). The recently described family Tarumaniidae brings an additional level of complexity to characiform relationships.
Tarumania walkerae (de Pinna et al., 2018), is a recently discovered species of fossorial freshwater fish occurring in leaf-litter deposits of the riparian rainforest in the lower Rio Negro, Central Amazonia (Figure 1). It possesses extreme modifications that make it unique among characiforms and other ostariophysans, such as a swimbladder segmented in 11 compartments along the body, reverse-imbricated scales over the head, reversed pelvic fins, high number of vertebrae, ribs, and scales, among others (de Pinna et al., 2018). Phylogenetic analysis of 128 morphological characters and 35 species revealed it as a sister group of Erythrinidae (trahiras, jejus; Figure 1) in an expanded Erythrinoidea sensu lato (s.l.) (Figure 2A; de Pinna et al., 2018). Such phylogenetic position, coupled with extreme phenotypic differentiation, resulted in the recognition of a previously unknown lineage at family level, the Tarumaniidae (de Pinna et al., 2018). The position of Tarumania was subsequently tested by a molecular analysis of two mitochondrial ribosomal genes (12S and 16S) and one nuclear protein-coding gene (Rag1), with results placing Tarumaniidae as sister to Erythrinidae with high-support (98%) and defining Erythrinoidea sensu stricto (s.s.) (Figure 2B; Arcila et al., 2018). However, relationships between Erythrinidae + Tarumaniidae and remaining characoid families as well as other regions of the phylogeny had lower statistical support (Arcila et al., 2018) likely due to limited genetic data. Two recent phylogenomic studies of Characiformes using thousands of exons (Figure 2C) or ultraconserved elements (UCEs) (Figure 2D) did not include any sample of Tarumania (Betancur-R et al., 2019; Melo et al., 2022), and thus the timing of evolution of Erythrinoidea s.s. and of Tarumaniidae relative to Erythrinidae have never been a subject of proper investigation.
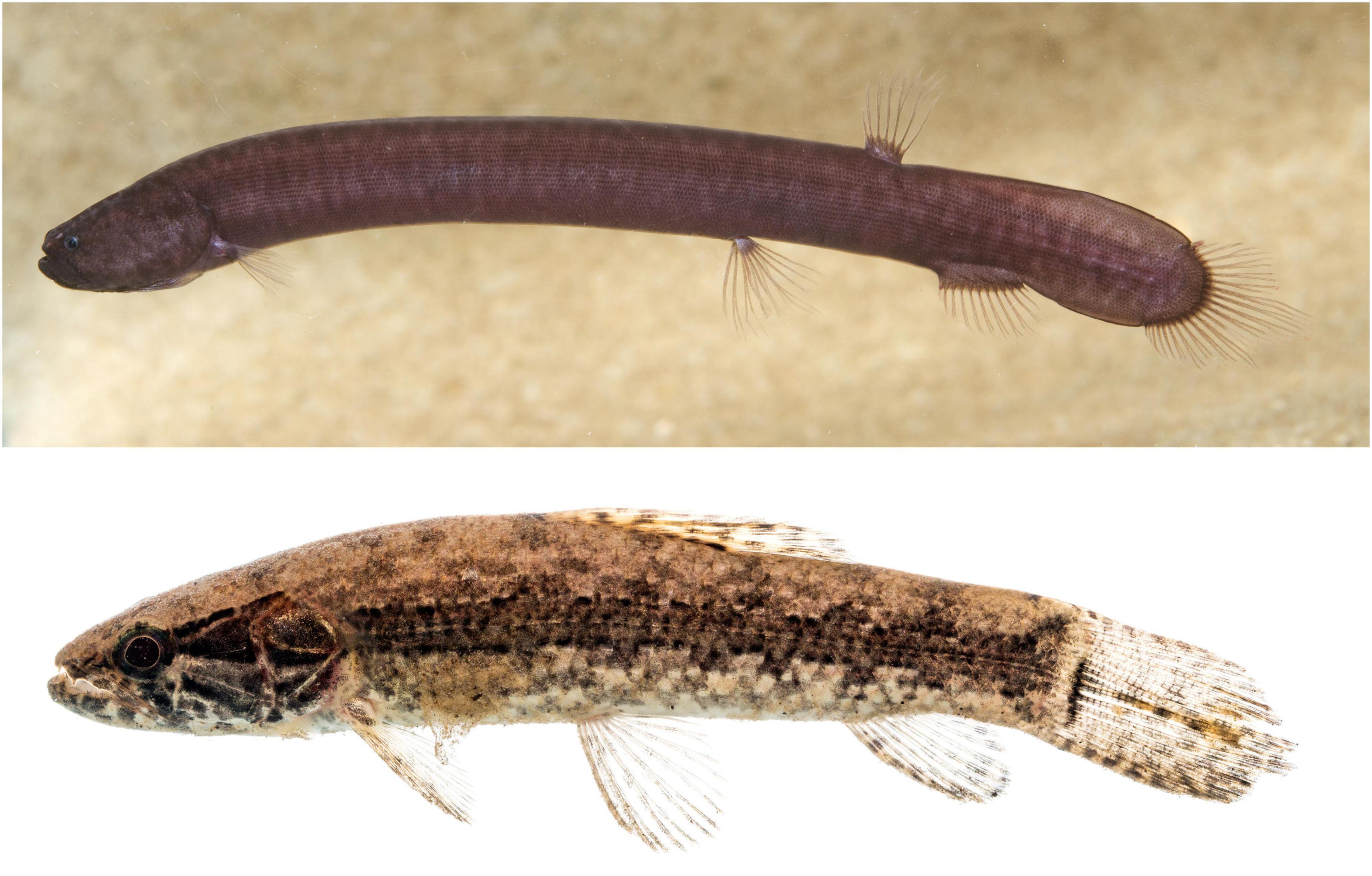
Figure 1. Live specimens of Tarumania walkerae (top), Brazil, Rio Tarumã-Mirim, lower Rio Negro, Amazon basin (photography by Douglas Bastos); Hoplias malabaricus (bottom), Colombia, Río Manacacías, Orinoco basin (photography by Jorge García-Melo, Proyeto CaVFish Colombia).
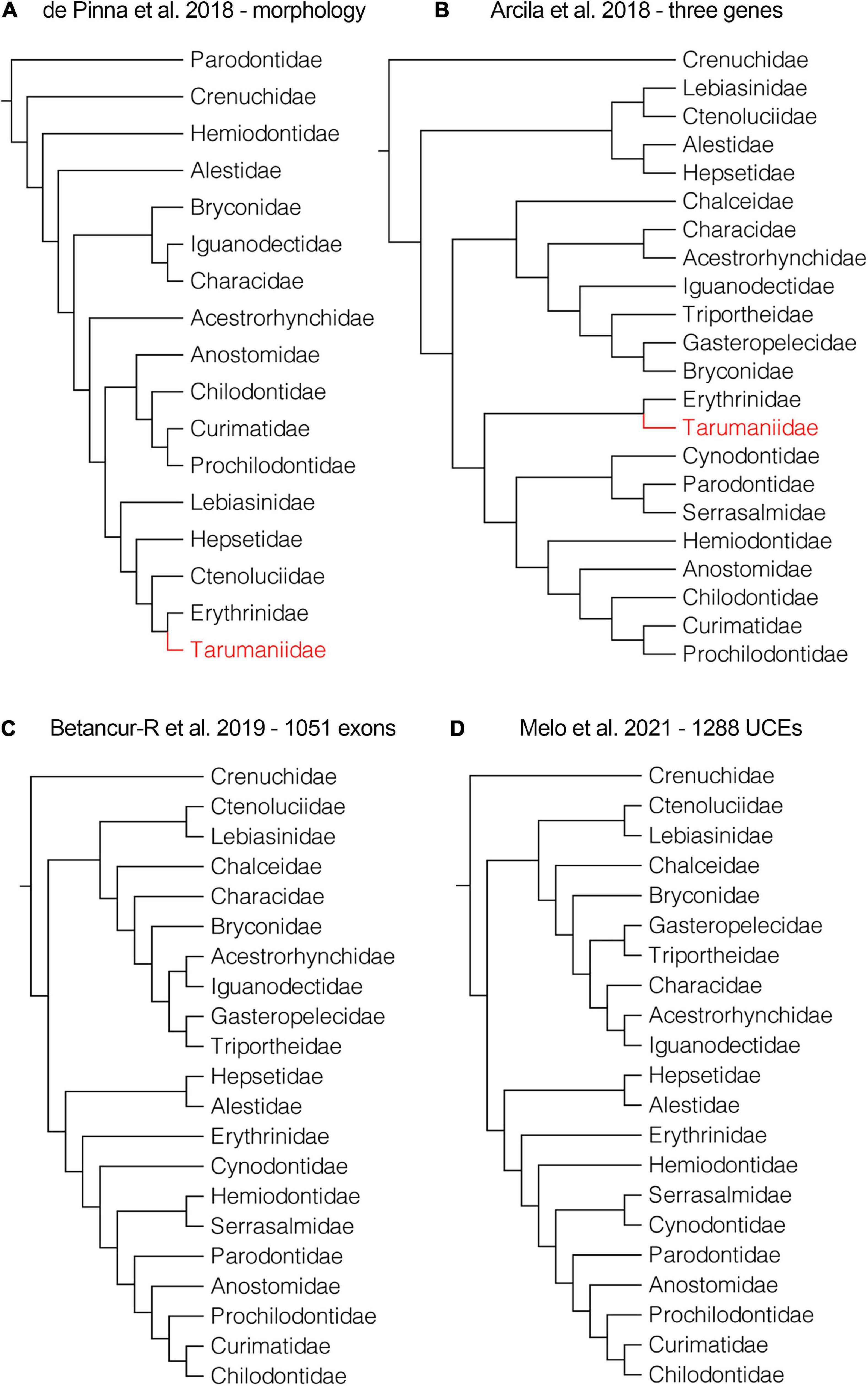
Figure 2. Alternative hypotheses for interfamilial relationships in Characiformes based on distinct sources of characters: (A) Morphological dataset with 128 characters and 33 taxa (de Pinna et al., 2018); (B) Molecular dataset of 12S, 16S, and Rag1 genes and 520 taxa (Arcila et al., 2018); (C) Molecular dataset of 1,051 exons and 321 taxa (Betancur-R et al., 2019); (D) Molecular dataset of 1,288 ultraconserved elements (UCEs) and 356 taxa (Melo et al., 2022). Note the lack of Tarumaniidae in the two phylogenomic studies (C,D).
In this study, we obtained genetic data for Tarumania and assessed its phylogenetic relationships using an expanded ultraconserved element dataset of the order Characiformes (Melo et al., 2022). UCEs were introduced as molecular markers for phylogenetic studies (Faircloth et al., 2012) and have been used to understand interrelationships of several actinopterygian groups (Faircloth et al., 2013; Harrington et al., 2016; McGee et al., 2016; Chakrabarty et al., 2017; Alfaro et al., 2018; Friedman et al., 2019; Ghezelayagh et al., 2021; Stiller et al., 2021; Tea et al., 2021). Although recent studies have found difficulties in resolving early branches of Ostariophysi and other clades using exons or UCEs (Chakrabarty et al., 2017; Betancur-R et al., 2019; Alda et al., 2021), the implementation of a method that assembles more than a thousand loci is relevant to reconstruct molecular phylogenies in many situations. More recently, a new ultraconserved element (UCE) probe set was designed to capture 2,708 UCE loci for Ostariophysi (Faircloth et al., 2020) which has been used to construct molecular supermatrices and to time-calibrate the diversification of many ostariophysan clades (Alda et al., 2018, 2021; Roxo et al., 2019; Mateussi et al., 2020; Ochoa et al., 2020; Silva et al., 2021a,b; Melo et al., 2022; Souza et al., 2022). In this context, our study aims to test the phylogenetic position of the rare and uniquely fossorial Tarumania using genomic data and estimate its timing of diversification relative to Erythrinoidea s.s. and other Characiformes. Results contribute to understand the tempo and mode of evolution of Tarumania and its highly unusual phenotypic features, as well as to have a clearer and more complete picture of the characiform radiation.
Material and methods
Taxon sampling and phylogenetic analyses
Taxon sampling was chosen based on our current phylogenetic knowledge about ostariophysan relationships (Fink and Fink, 1981, 1996; Chen et al., 2013; Arcila et al., 2017; Chakrabarty et al., 2017; Melo et al., 2022). The 108 terminals in this dataset included one specimen of Cypriniformes as root (Cyprinus), one Gymnotiformes (Steatogenys), five Siluriformes (Astroblepus, Loricaria, Pimelodella, Pimelodus, and Trichomycterus), five Cithariniformes (Citharinus, Distichodus, Nannaethyops, Paradistichodus, and Xenocharax), and 96 Characiformes. We chose a balanced subset of characiform taxa used by Melo et al. (2022) including 96 taxa representing all families: Crenuchidae (3 species: Characidium, Crenuchus, and Elachocharax), Hepsetidae (3: Hepsetus), Alestidae (4: Alestes, Alestopetersius, Bryconaethyops, and Bryconalestes), Tarumaniidae (1: Tarumania), Erythrinidae (5: Erythrinus, Hoplerythrinus, and Hoplias), Hemiodontidae (3: Anodus, Bivibranchia, and Hemiodus), Cynodontidae (3: Cynodon, Hydrolycus, and Raphiodon), Serrasalmidae (4: Colossoma, Myleus, Mylossoma, and Serrasalmus), Parodontidae (3: Apareiodon, Parodon, and Saccodon), Anostomidae (6: Abramites, Anostomus, Leporellus, Leporinus, and Megaleporinus), Prochilodontidae (3: Ichthyoelephas, Prochilodus, and Semaprochilodus), Chilodontidae (3: Caenotropus and Chilodus), Curimatidae (7: Curimata, Curimatella, Curimatopsis, and Cyphocharax), Ctenoluciidae (3: Boulengerella and Ctenolucius), Lebiasinidae (3: Copella, Lebiasina, and Nannostomus), Chalceidae (3: Chalceus), Bryconidae (6: Brycon, Chilobrycon, Henochilus, and Salminus), Acestrorhynchidae (3: Acestrorhynchus, Hoplocharax, and Lonchogenys), Iguanodectidae (3: Bryconops, Iguanodectes, and Piabucus), Gasteropelecidae (3: Carnegiella, Gasteropelecus, and Thoracocharax), Triportheidae (3: Clupeacharax, Lignobrycon, and Triportheus), and Characidae (21: Amazonspinther, Aphyocharax, Astyanax, Bryconamericus, Charax, Creagrutus, Eretmobrycon, Exodon, Glandulocauda, Gymnocorymbus, Hyphessobrycon, Knodus, Markiana, Odontostilbe, Paracheirodon, Protocheirodon, Spintherobolus, Stethaprion, and Tetragonopterus).
The tissue sample of T. walkerae corresponds to LBP 22727, collected using hand nets in the type-locality, Rio Tarumã-Mirim, lower Rio Negro, Brazil, 02.90965°S 60.22915°W, by M. de Pinna, L. Rapp Py-Daniel, and J. Zuanon, on September 2, 2006. Voucher information of all analyzed specimens is available in Supplementary Table 1. The genomic DNA of Tarumania was extracted using DNeasy tissue kit (Qiagen Inc., Germantown, MD, United States) in which resulted in 78.6 ng/μl DNA; other samples ranged 10–100 ng/μl DNA. Libraries were prepared using the Nextera kit protocol (Illumina Inc., San Diego, CA, United States) for solution-based target enrichment (Faircloth et al., 2012) and PCR-amplified using KAPA HiFi HotStart ReadyMix (Kapa Biosystems, Wilmington, MA, United States). Libraries were quantified and enriched using MYbaits Target Enrichment system (Arbor Biosciences, Ann Arbor, MI, United States) following the protocol of the ostariophysan probeset with 2,708 ultraconserved element loci (Faircloth et al., 2020). Sequencing of library pools was performed using Illumina HiSeq paired-end 100 bp lanes. Additional details of laboratory methods, library preparation, enrichment, sequencing, and data assembly are provided in Melo et al. (2022). Raw sequence reads for Tarumania and other taxa are deposited in the National Center for Biotechnology Information Sequence Read Archive (NCBI SRA) under BioProject PRJNA850628. Information about reads for each species appear in Supplementary Table 2.
Two concatenated UCE matrices were assembled for this study accounting for distinct amounts of characters and missing data. The 70% complete matrix contains only loci present in at least 70% of 108 terminals (i.e., loci present in at least 76 terminals), whereas the 90% complete matrix includes only the loci present in at least 90% of terminals (i.e., loci present in at least 98 terminals). The 70% complete matrix is consequently longer (more nucleotides/characters) but contains more missing data, while the 90% complete matrix is comparatively shorter but with fewer missing data. Matrices were aligned with MAFFT v7 (Katoh et al., 2002; Katoh and Standley, 2013), and were partitioned by Partition-UCE (Tagliacollo and Lanfear, 2018) with combination of partitions and models of nucleotide evolution subsequently chosen by PartitionFinder v2.1.1 (Lanfear et al., 2012).
For the two matrices, we used three distinct phylogenetic reconstructions. First, we ran two alternative maximum likelihood searches with identical parameters to find the maximum likelihood trees using RAxML v8 with the GTRGAMMA model (Stamatakis, 2014), and used the autoMRE function available in RAxML v8 (Stamatakis, 2014) to access non-parametric bootstrap support values. Second, we used the Bayesian framework with ExaBayes (Aberer et al., 2014) by running two independent runs (one cold and one heated chain each) for 106 generations (burn-in: 25%; thinning: 500) with default parameters. Tracer v1.6 (Rambaut et al., 2014) assessed convergence through analysis of the log files from independent runs to ensure stationary and sufficient mixing of parameters (ESS >200). We generated the most credible set of trees from the posterior distribution of possible topologies using the consensus algorithm in ExaBayes. Finally, we ran a coalescent-based analysis from individual gene trees to account for coalescent stochasticity among individual loci (Mirarab et al., 2014). For this approach, we used PHYLUCE (Faircloth, 2015) to resample the complete matrices by locus. Then, we used RAxML v8 to generate a maximum likelihood tree for each locus, and ASTRAL-II (Mirarab and Warnow, 2015) to infer species trees from the best gene trees, generating a 70% majority-rule consensus tree of the results.
Time-calibrated analysis
For the time-calibrated analysis, we used five characoid fossils as primary calibrations and one constraint on the root as secondary calibrations. The first fossil prior is represented by tooth fragments of Alestidae from the Ager basin (Early Eocene 54–49 Ma), Lérida, Spain (de la Peña Zarzuelo, 1996). That fossil has diagnostic similarities with premaxillary teeth of modern Alestes, Brycinus, and Bryconaethiops (Zanata and Vari, 2005). The node uniting those three genera is the most recent common ancestor (MRCA) of Alestidae in molecular reconstructions (Arroyave and Stiassny, 2011; Melo et al., 2022) where we calibrated the prior mean = 52.0; sigma = 2.0; 95–5% quantiles: 55.3–48.7. The second is †Leporinus scalabrinii from the Ituzaingó Formation (Late Miocene 9–6 Ma), Entre Ríos, Argentina (Bogan et al., 2012), close to Abramites and L. striatus (Bogan et al., 2012) and with similarities with Megaleporinus (Ramirez et al., 2017); we placed the fossil at the MRCA of the clade including those three taxa: mean = 7.5; sigma = 1.0; 95–5% quantiles: 9.14–5.86. The third fossil is †Cyphocharax mosesi from the Taubaté basin (Oligocene-Miocene 28.4–20.4 Ma), São Paulo, Brazil (Travassos and Santos, 1955; Malabarba, 1996), hypothesized to be closely related to the modern Cyphocharax gilbert, Cyphocharax modestus, and Cyphocharax santacatarinae (Malabarba, 1996; Melo et al., 2021). Following the phylogeny and historical biogeography of curimatids (Melo et al., 2018, 2021), we calibrated the fossil at the MRCA node of Cyphocharax and Curimatella (mean = 23.8; sigma = 3.0; 95–5% quantiles: 28.7–18.9). The fourth fossil is †Megacheirodon unicus, from Taubaté basin, São Paulo, Brazil (Bührnheim et al., 2008), and hypothesized to be sister to Amazonspinther and Spintherobolus (Bührnheim et al., 2008); we calibrated it at the node including Amazonspinther and three species of Spintherobolus (mean = 23.8; sigma = 3.0; 95–5% quantiles: 28.7–18.9). The fifth fossil was †Paleotetra entrecorregos from Aiuruoca basin (Eocene-Oligocene), Minas Gerais, Brazil (Weiss et al., 2012). †Paleotetra has been hypothesized as a putative Stevardiinae (Weiss et al., 2012) or a stem Characidae (Mirande, 2019). Considering the uncertain position of the genus, we followed the original description (Weiss et al., 2012): mean = 23.8; sigma = 3.0; 95–5% quantiles: 28.7–18.9. Finally, the root constrain is based on our current knowledge for the divergence time of Cypriniformes and remaining Otophysi estimated for the Early/Middle Jurassic c. 201–158 Ma (Near et al., 2012; Arroyave et al., 2013; Melo et al., 2022) (mean = 170.5; sigma = 10.0; 95–5% quantiles: 187–154). All prior parameters used for calibrations in this study are available in the input file (.xml) as Supplementary Material.
We preferred to not use the erythrinoids †Tiupampichthys and †Paleohoplias (Gayet et al., 2003) or fossil specimens of modern Hoplias (Lundberg, 1997; Monsch, 1998; Lundberg et al., 2009) because we aimed to compare the ages obtained from our phylogeny with the available fossil record of Erythrinoidea s.l., thus having an independent source of data for testing our estimated dating hypothesis. We used the 90% complete matrix with 108 terminals that, compared to the 70% complete matrix, has fewer missing data and we then used an uncorrelated relaxed molecular clock implemented on BEAST v2 (Drummond et al., 2006; Bouckaert et al., 2014, 2019). We tested the lognormal distribution for fossil priors using a fixed topology without success for parameter convergence in BEAST v2; thus, we used normal distributions with chosen sigma values based on the exact stratigraphy of each fossil. For example, †L. scalabrinii was found in the Late Miocene (9–6 Ma) Ituzaingó Formation of Argentina (Bogan et al., 2012), so we used a normal distribution (mean = 7.5, sigma = 1.0) that constrained the respective node ranging between 9.14 and 5.86 Ma (95–5% quantiles) in BEAUTI v2 (Bouckaert et al., 2019; see Supplementary Material).
We used a birth–death branching model and two BEAST runs for 200 million generations each with a sampling frequency of 20,000 generations (total 400 million generations). Tracer v1.6 (Rambaut et al., 2014) examined the stationarity and sufficient mixing of parameters (ESS >200), whereas TreeAnnotator v1.8.2 read the 20,002 resulting trees, discarded the first 2,000 trees as burn-in, and saved the maximum clade credibility tree. The time-calibrated phylogeny and all clade-age estimates are presented as the mean plus 95% highest posterior density values (95% HPD) in FigTree v1.4.4. All topologies are available as Supplementary Material.
Results and discussion
Phylogenetic placement and natural history of Tarumania
The first UCE dataset contained 1,795 UCE loci with 488,434 bp (70% complete matrix), and the second contained 172 UCE loci with 50,313 bp (90% complete matrix). Inside the more restricted Characiformes (i.e., exclusive of Cithariniformes), the three analyses (RAxML, ExaBayes, and ASTRAL-II) differ mainly in the placement of Bryconidae, Characidae, Hemiodontidae, and Parodontidae (Figure 3). At the family level, nodes with support values lower than 80% in the maximum likelihood analysis were Characiformes + Siluriformes, the clade [(Cynodontidae Serrasalmidae) (Parodontidae Anostomoidea)], the clade {Bryconidae [(Triportheidae Gasteropelecidae) (Iguanodectidae Acestrorhynchidae)]}, and the clade [(Triportheidae Gasteropelecidae) (Iguanodectidae Acestrorhynchidae)] (Figure 3). This is due to uncertainties in the placement of Cithariniformes, Hemiodontidae, Characidae, and Bryconidae, as previously reported in molecular phylogenies (Oliveira et al., 2011; Arcila et al., 2017; Betancur-R et al., 2019; Melo et al., 2022). In addition to those conflicting regions, the present ASTRAL-II analysis provides novel arrangements among families in the backbone of characiform phylogeny such as the Crenuchidae sister to Alestoidea, Erythrinoidea s.s., and Curimatoidea sensu Betancur-R et al. (2019) (Figures 3E,F), the close relationship between Cynodontidae, Hemiodontidae and Serrasalmidae (Figure 3E), and the unexpected non-monophyly of Triportheidae and Curimatidae (Figures 3E,F). Difficulties to resolve old early-branching nodes are likely related to the lack of phylogenetic signal in individual UCE loci (Alda et al., 2021). Nevertheless, recent order-level phylogenies of Characiformes addressed interfamilial relationships using denser matrices of exons and UCEs (Betancur-R et al., 2019; Melo et al., 2022) and this discussion lies beyond the present scope.
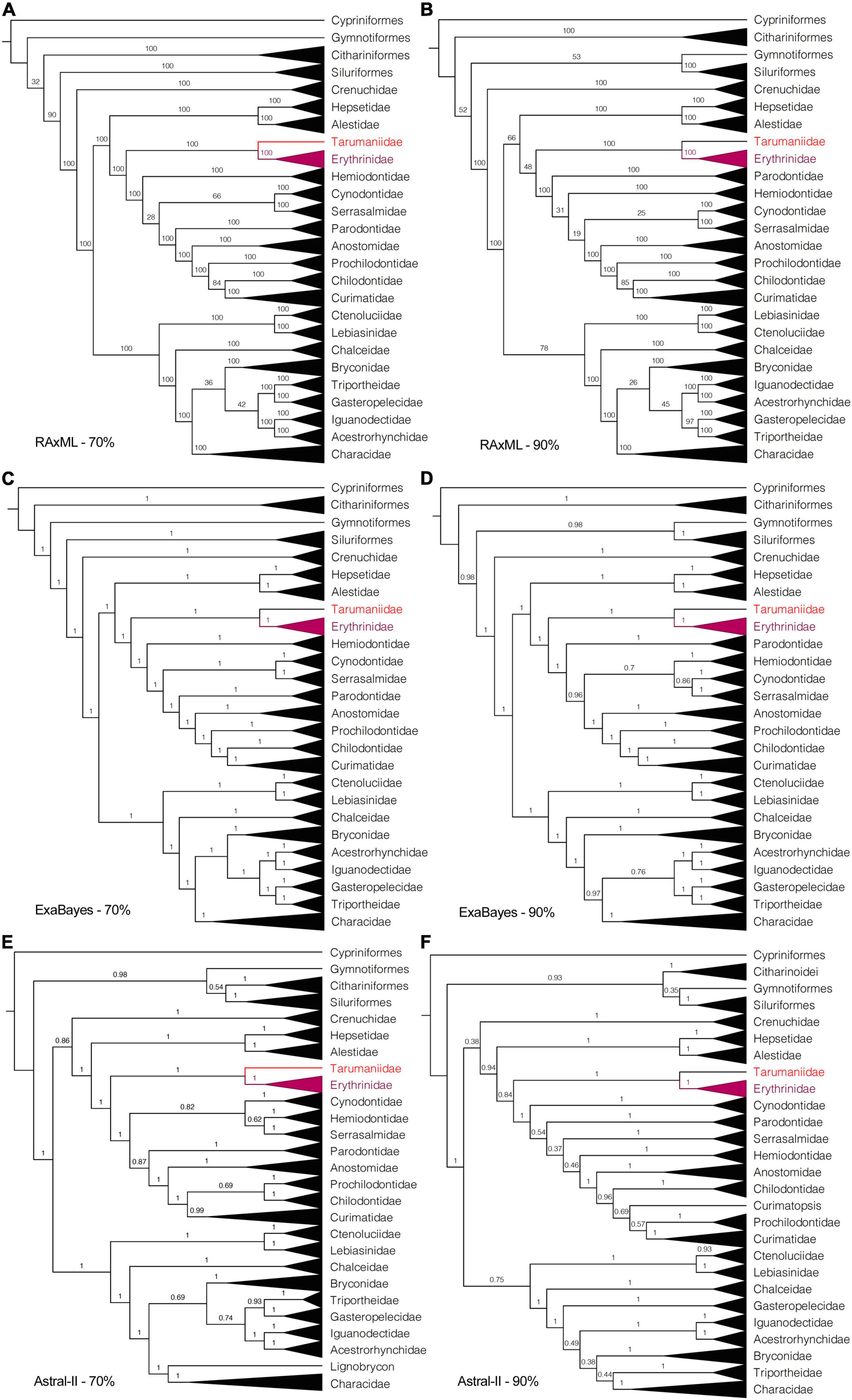
Figure 3. Phylogenetic placements of Tarumaniidae (red) and Erythrinidae (maroon) in Characiformes using the first (1795 loci; 70% complete) and second (172 loci; 90% complete) matrices of ultraconserved elements (UCEs): Maximum likelihood in RAxML (A,B), Bayesian approach in ExaBayes (C,D), Coalescent-based analysis in ASTRAL-II (E,F).
Regardless of the position of those families, all phylogenetic reconstructions agree in the placement of Tarumaniidae as the sister group of Erythrinidae (Figure 3) composing the superfamily Erythrinoidea s.s., in agreement with previous results, both morphological and molecular (Figures 2A,B; Arcila et al., 2018; de Pinna et al., 2018). Our reconstructions also place Erythrinoidea s.s. as sister of the eight-family clade with Hemiodontidae, Cynodontidae, Serrasalmidae, Parodontidae, Anostomidae, Prochilodontidae, Curimatidae, and Chilodontidae (Figure 3). The latter result also agrees with a phylogenetic study using two mitochondrial and one nuclear locus in the placement of Tarumaniidae (Arcila et al., 2018). However, our findings do not support the morphological hypothesis that Erythrinidae + Tarumaniidae are sister to Ctenoluciidae within the Erythrinoidea s.l. that also contains Hepsetidae and Lebiasinidae (de Pinna et al., 2018). Our reconstruction involving Erythrinoidea s.s. with the eight-family clade is echoed in a similar position of Erythrinidae resulting from the analysis of both exons and UCEs for Characiformes (Betancur-R et al., 2019; Melo et al., 2022).
Some hypothesized morphological synapomorphies are not congruent with our molecular hypothesis. For example, the continuous scale covering represents a synapomorphy for the Erythrinoidea s.l. (de Pinna et al., 2018) and requires convergent evolution in our phylogeny. On the other hand, morphological synapomorphies supporting the Tarumaniidae-Erythrinidae relationship are congruent with our current hypothesis, despite their lower bootstrap support cited by the authors (lower than the expanded Erythrinoidea) (de Pinna et al., 2018). Although the morphological traits of Tarumania seem to explore extreme reaches in a phenotypic landscape, such as highly reduced coracoid, exceedingly numerous scales, extreme vertebral number, reduction of suspensorium, and multiplication of air bladder chambers, the recognition of synapomorphies shared with Erythrinidae is straightforward.
Phylogenetically significant features shared between Tarumaniidae and Erythrinidae go beyond the usual molecular-phenotypic divide. The degree of fossorial adaptations in Tarumania is doubtlessly unique in characiforms. However, erythrinids also have ecological preferences which may foreshadow the extreme situation in that genus. All members of Erythrinidae are benthic or nektobenthic, favoring the bottom part of the water column, a preference unusual in characiforms. Also contrary to the majority of characiforms who spend most of the time as active swimmers, erythrinids tend to stay still in the water column or on the bottom for extended periods, a behavior associated with their predominant ambush hunting strategy. Some species of the erythrinid Hoplias has a marked preference for quiet sediment-rich areas, and species of both that genus and of Erythrinus are often found buried in leaf litter, a microhabitat similar to, yet less extreme than, that typical of Tarumania.
Another unusual trait shared by Tarumania and erythrinids is a true air-breathing capacity. Several characoids can use the air-water interface for respiration (the Aquatic Surface Respiration: ASR) as for example species of Triportheidae, Serrasalmidae, Bryconidae, and a few Characidae (Soares et al., 2006). Such strategy is associated with temporary expansion of the lower lip in species of Triportheus, Colossoma, Mylossoma, Brycon, and Salminus, increasing the intake of surface water to ventilate the gills rather than a true air-breathing ability. Among erythrinids, Hoplias can survive hypoxic conditions for long time periods by means of physiological adaptations associated to decreasing metabolic rate (Soares et al., 2006). Erythrinus and Hoplerythrinus gulp air at the water surface to withstand severe hypoxia during long periods, especially in dry seasons when they frequently get trapped in desiccating ponds. The air is pumped into the swimbladder, which in Erythrinus and Hoplerythrinus have macroscopically evident alveolations at the anterior portion of the posterior chamber (Liem, 1988; Pelster, 2021), with some species of Lebiasina and Piabucina (Lebiasinidae) also showing such adaptations (Graham et al., 1978). Species of Erythrinus can also move overland among nearby ponds and occasionally back to permanent water bodies. Tarumania also gulps air at the surface when confined to small amounts of water (de Pinna et al., 2018), but the air stays in the oral cavity and no evidence exists of it entering the swimbladder for respiratory function. Tarumania can survive deeply buried in moist leaf litter up to the next rainy season, which seems to represent an extreme adaptation to life in ephemeral water bodies of forested habitats. Despite structural differences in air-breathing mechanism, the phylogenetic proximity between Tarumania and erythrinids means that such unusual characiform traits may comprise some homologous baseline in the two lineages, with the former having developed such adaptations toward a direction allowing it to explore the inhospitable hyporheic zone. This also demonstrates that natural history traits may represent interesting sources of evidence for homologies and historically bound adaptive causation.
Evolutionary history of Erythrinoidea sensu stricto and the fossil record
Adaptations to the fossorial habitat in characiforms seem to have been present since the Mesozoic. The fossil-calibrated phylogeny indicates that the MRCA of Tarumaniidae + Erythrinidae split from other characiforms during the Late Cretaceous at around 90 Ma (108–72 Ma, 95% HPD) (Figure 4). These dates fit in with recent fossil-calibrated phylogenies for Characiformes. Using a multilocus dataset and seven fossil calibrations, Burns and Sidlauskas (2019) estimated that Erythrinus diverged from the major characoid lineage Curimatoidea at approximately 84 Ma (90–78 Ma, 95% HPD). Using a phylogenomic UCE dataset and six fossil calibrations, Melo et al. (2022) proposed that Erythrinidae diverged from other characoids during the Late Cretaceous at around 97 Ma (115–79 Ma, 95% HPD). However, other fossorial fish groups seem to have emerged later. Phreatobius has never been included in time-calibrated phylogenies, but its sister clade Pseudopimelodidae (Sullivan et al., 2006) was estimated to have diverged from Pimelodidae circa 70 Ma (Betancur-R et al., 2017). Although no study has investigated the origins and factors driving the acquisition of fossorial adaptations, the split of Erythrinoidea s.s. stands out as a particularly old event among Neotropical fish clades and represents yet another odd feature for that clade.
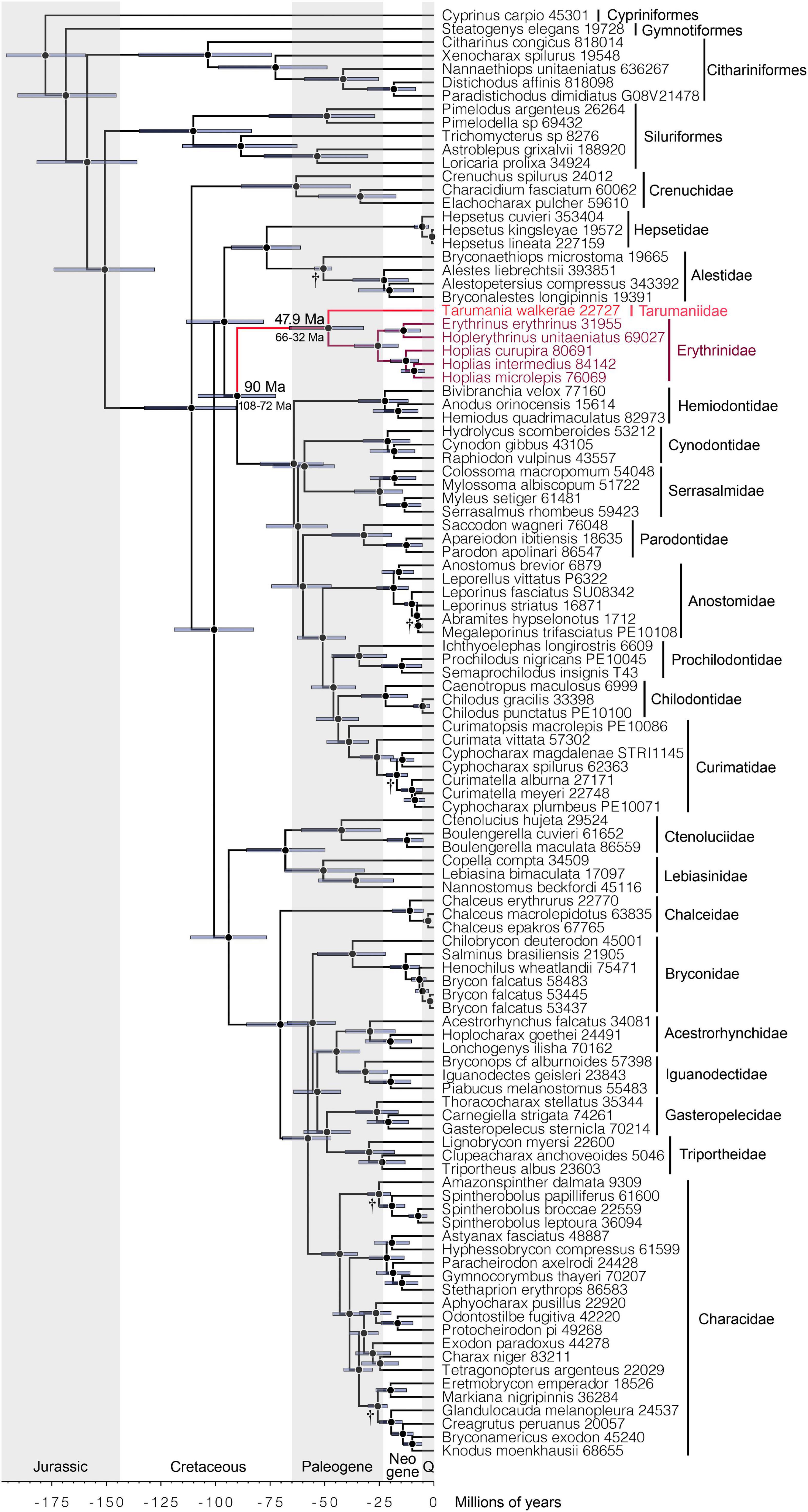
Figure 4. Fossil-calibrated phylogeny of Characiformes and related taxa based on a Bayesian analysis of ultraconserved elements (90% complete matrix; 172 loci; 50,313 bp) obtained from five fossil calibrations (†). Node ages are represented by the highest posterior density (95% HPD; purple bars). Note the divergence time between Erythrinoidea sensu stricto (s.s.) (Erythrinidae and Tarumaniidae) and related characoids during the Late Cretaceous (90 Ma; 108–72 Ma 95% HPD), and the divergence time between Erythrinidae and Tarumaniidae during the Paleogene (47.9 Ma; 66–32 Ma 95% HPD). Ma, millions of years ago; Q, quaternary.
Tarumania walkerae is the single living species of Tarumaniidae and is closely related to 20 living species of Erythrinidae known to date. No fossils of Tarumaniidae are known yet and the oldest fossils assignable to Erythrinoidea or Erythrinidae are disarticulated specimens, mostly isolated tooth fragments, found in the El Molino and Santa Lucía formations of central Bolivia dated to the Cretaceous-Paleogene boundary (Maastrichtian to Danian, 70–61 Ma) (Sempere et al., 1997; Gayet et al., 2001, 2003; Malabarba and Malabarba, 2010). One remarkable taxon particularly important to understand the evolution of Tarumaniidae is the erythrinoid-like †Tiupampichthys that possesses jaw features intermediate between modern erythrinids, cynodontids, hepsetids, and acestrorhynchids (Gayet et al., 2003). Under the three morphological hypotheses, †Tiupampichthys would be assignable either as close to the MRCA of Erythrinidae, Lebiasinidae, Hepsetidae, Ctenoluciidae, and Acestrorhynchidae (Buckup, 1998; Gayet et al., 2003), or Erythrinoidea s.l. plus Anostomoidea and Acestrorhynchidae (de Pinna et al., 2018), or Erythrinoidea s.l. plus Cynodontidae and Acestrorhynchidae (Pastana et al., 2020). Under the molecular hypothesis, †Tiupampichthys would be attributable to the MRCA of all characiforms except Crenuchidae (Figure 4). This is due to distinct positions of Hepsetidae (Alestoidea), Erythrinoidea s.s., Cynodontidae (Curimatoidea), and Acestrorhynchidae (Characoidea) (Arcila et al., 2017; Betancur-R et al., 2019; Melo et al., 2022). Although the phylogenetic placement of †Tiupampichthys is uncertain due to intermediate morphologies, the occurrence of an erythrinoid-like taxon in the Late Cretaceous suggests that derived forms of erythrinids and tarumaniids appeared later than that period (i.e., <66 Ma). This scenario supports our current hypothesis that modern Tarumania splits from erythrinids during the Paleogene c. 66–32 Ma (Figure 4).
The time-calibrated phylogeny indicates a Paleogene emergence of Tarumaniidae, splitting from Erythrinidae at approximately 47.9 Ma (66–32 Ma, 95% HPD). Available fossil evidence indicates that several modern families were present during the Paleogene (Lundberg, 1997; Malabarba and Malabarba, 2010; López-Fernández and Albert, 2011). The early diversification in 14 out of 21 modern characiform families, including highly diverse clades like Characidae, are dated to the Paleogene (66–23 Ma) (Melo et al., 2022). In fact, the Cretaceous-Paleogene El Molino Formation of Bolivia revealed the earliest fossils attributed to Characidae [Tetragonopterinae of Gayet et al. (2001)]. Paleogene formations, in turn, revealed well-preserved and phenotypically intermediate characid genera such as †Paleotetra and †Bryconetes from the Eocene-Oligocene and †Megacheirodon from the Oligocene-Miocene (Travassos and Santos, 1955; Bührnheim et al., 2008; Malabarba and Malabarba, 2010; Weiss et al., 2012, 2014). Fossils of Serrasalmidae begin to occur during the Late Cretaceous and early Paleogene (Gayet et al., 2001) and the evidence, in combination with molecular-dated phylogenies, suggests that the Paleogene was also the age for the early intrafamilial diversification (Kolmann et al., 2020; Melo et al., 2022). Therefore, the sum of evidence allows us to hypothesize that the early lineage of Tarumaniidae diverged from erythrinids in the Paleogene and colonized Central Amazonia, where inhospitable habitats favored the appearance and maintenance of extreme morphological, physiological, and ecological adaptations.
Overall, this study provides a genomic-based hypothesis for the phylogenetic placement of Tarumania and an evolutionary timeframe for the diversification of Erythrinoidea s.s. Further studies can use ancestral state reconstructions to estimate the evolution of relevant morphological characters along characiform phylogeny. For example, which characters were maintained during the evolution of Erythrinidae, and which characters were lost in Tarumania? Understanding character evolution in Erythrinoidea s.s. would be particularly important for the resolution of phylogenetic conflicts along this section of the evolutionary history of Characiformes, such as the position of Hemiodontidae, Serrasalmidae, and Cynodontidae (Betancur-R et al., 2019; Kolmann et al., 2020; Melo et al., 2022).
Data availability statement
The datasets presented in this study can be found in online repositories. The names of the repository/repositories and accession number(s) can be found in the article/Supplementary material.
Ethics statement
The animal study was reviewed and approved by CEEAA permit 3245 IB/UNESP.
Author contributions
BM, MP, and CO designed the study. MP, LR, and JZ performed the field collection. BM, CC-S, and FR collected the molecular data. BM, CC-S, and FR analyzed the data. BM, MP, LR, JZ, and CO interpreted the results. CO coordinated the research project. BM, MP, LR, and JZ contributed with writing. All authors contributed conceptually to achieve the final version of the manuscript, read, and approved the final version.
Funding
This research was funded by Fundação de Amparo à Pesquisa do Estado de São Paulo grants #16/11313-8 and #18/24040-5 (BM), #18/09767-6 and #19/16999-3 (CC-S), #14/26508-3 and #20/13433-6 (CO); Conselho Nacional de Desenvolvimento Científico e Tecnológico, grants #404991/2018-1 and #200159/2020-8 (BM), #310688/2019-1 (MP), #313183/2014-7 (JZ), #306054/2006-0 (CO), and Stiassny AMNH Axelrod Research Curatorship of the Department of Ichthyology of the American Museum of Natural History (BM).
Acknowledgments
We thank and give credits to Douglas A. Bastos for the photograph of Tarumania and to Jorge E. García-Melo (Proyeto CaVFish Colombia) for the photograph of Hoplias (Figure 1).
Conflict of interest
The authors declare that the research was conducted in the absence of any commercial or financial relationships that could be construed as a potential conflict of interest.
Publisher’s note
All claims expressed in this article are solely those of the authors and do not necessarily represent those of their affiliated organizations, or those of the publisher, the editors and the reviewers. Any product that may be evaluated in this article, or claim that may be made by its manufacturer, is not guaranteed or endorsed by the publisher.
Supplementary material
The Supplementary Material for this article can be found online at: https://www.frontiersin.org/articles/10.3389/fevo.2022.924860/full#supplementary-material
References
Aberer, A. J., Kobert, K., and Stamatakis, A. (2014). ExaBayes: Massively parallel bayesian tree inference for the whole-genome era. Mol. Biol. Evol. 31, 2553–2556. doi: 10.1093/molbev/msu236
Alda, F., Ludt, W. B., Elías, D. J., McMahan, C. D., and Chakrabarty, P. (2021). Comparing ultraconserved elements and exons for phylogenomic analyses of Middle American cichlids: When data agree to disagree. Gen. Biol. Evol. 13:evab161. doi: 10.1093/gbe/evab161
Alda, F., Tagliacollo, V. A., Bernt, M. J., Waltz, B. T., Ludt, W. B., Faircloth, B. C., et al. (2018). Resolving deep nodes in an ancient radiation of neotropical fishes in the presence of conflicting signals from incomplete lineage sorting. Syst. Biol. 68, 573–593. doi: 10.1093/sysbio/syy085
Alfaro, M. E., Faircloth, B. C., Harrington, R. C., Sorenson, L., Friedman, M., Thacker, C. E., et al. (2018). Explosive diversification of marine fishes at the Cretaceous-Palaeogene boundary. Nat. Ecol. Evol. 2:688. doi: 10.1038/s41559-018-0494-6
Arcila, D., Ortí, G., Vari, R., Armbruster, J. W., Stiassny, M. L. J., Ko, K. D., et al. (2017). Genome-wide interrogation advances resolution of recalcitrant groups in the tree of life. Nat. Ecol. Evol. 1, 1–20. doi: 10.1038/s41559-016-0020
Arcila, D., Petry, P., and Ortí, G. (2018). Phylogenetic relationships of the family Tarumaniidae (Characiformes) based on nuclear and mitochondrial data. Neotrop. Ichthyol. 16:e180016. doi: 10.1590/1982-0224-20180016
Arroyave, J., and Stiassny, M. L. J. (2011). Phylogenetic relationships and the temporal context for the diversification of African characins of the family Alestidae (Ostariophysi: Characiformes): Evidence from DNA sequence data. Mol. Phylogenet. Evol. 60, 385–397. doi: 10.1016/j.ympev.2011.04.016
Arroyave, J., Denton, J. S. S., and Stiassny, M. L. J. (2013). Are characiform fishes Gondwanan in origin? Insights from a time-scaled molecular phylogeny of the Citharinoidei (Ostariophysi: Characiformes). PLoS One 8:e77269. doi: 10.1371/journal.pone.0077269
Betancur-R, R., Arcila, D., Vari, R. P., Hughes, L. C., Oliveira, C., Sabaj, M. H., et al. (2019). Phylogenomic incongruence, hypothesis testing, and taxonomic sampling: The monophyly of characiform fishes. Evolution 73, 329–345. doi: 10.1111/evo.13666
Betancur-R, R., Wiley, E. O., Arratia, G., Acero, A., Bailly, N., Miya, M., et al. (2017). Phylogenetic classification of bony fishes. BMC Evol. Biol. 17:162. doi: 10.1186/s12862-017-0958-3
Bogan, S., Sidlauskas, B., Vari, R. P., and Agnolin, F. (2012). Arrhinolemur scalabrinii Ameghino, 1898, of the late Miocene–a taxonomic journey from the Mammalia to the Anostomidae (Ostariophysi: Characiformes). Neotrop. Ichthyol. 10, 555–560. doi: 10.1590/S1679-62252012000300008
Bouckaert, R., Heled, J., Kühnert, D., Vaughan, T., Wu, C.-H., Xie, D., et al. (2014). BEAST 2: A software platform for Bayesian evolutionary analysis. PLoS Comput. Biol. 10:e1003537. doi: 10.1371/journal.pcbi.1003537
Bouckaert, R., Vaughan, T. G., Barido-Sottani, J., Duchêne, S., Fourment, M., Gavryushkina, A., et al. (2019). BEAST 2.5: An advanced software platform for Bayesian evolutionary analysis. PLoS Comput. Biol. 15:e1006650. doi: 10.1371/journal.pcbi.1006650
Buckup, P. A. (1998). “Relationships of the Characidiinae and phylogeny of characiform fishes (Teleostei: Ostariophysi,” in Phylogeny and classification of neotropical fishes, eds L. R. Malabarba, R. E. Reis, R. P. Vari, Z. M. S. Lucena, and C. A. S. Lucena (Porto Alegre: Edipucrs).
Bührnheim, C. M., Carvalho, T. P., Malabarba, L. R., and Weitzman, S. H. (2008). A new genus and species of characid fish from the Amazon basin: The recognition of a relictual lineage of characid fishes (Ostariophysi: Cheirodontinae: Cheirodontini). Neotrop. Ichthyol. 6, 663–678. doi: 10.1590/S1679-62252008000400016
Burns, M. D., and Sidlauskas, B. L. (2019). Ancient and contingent body shape diversification in a hyperdiverse continental fish radiation. Evolution 73, 569–587. doi: 10.1111/evo.13658
Chakrabarty, P., Faircloth, B. C., Alda, F., Ludt, W. B., McMahan, C. D., Near, T. J., et al. (2017). Phylogenomic systematics of ostariophysan fishes: Ultraconserved elements support the surprising non-monophyly of characiformes. Syst. Biol. 66, 881–895. doi: 10.1093/sysbio/syx038
Chen, W.-J., Lavoué, S., and Mayden, R. L. (2013). Evolutionary origin and early biogeography of otophysan fishes (Ostariophysi: Teleostei). Evolution 67, 2218–2239. doi: 10.1111/evo.12104
de la Peña Zarzuelo, A. (1996). Characid teeth from the lower eocene of the ager basin (Lérida, Spain): Paleobiogeographical comments. Copeia 1996, 746–750. doi: 10.2307/1447544
de Pinna, M., Zuanon, J., Rapp Py-Daniel, L., and Petry, P. (2018). A new family of neotropical freshwater fishes from deep fossorial amazonian habitat, with a reappraisal of morphological characiform phylogeny (Teleostei: Ostariophysi). Zool. J. Linn. Soc. 182, 76–106. doi: 10.1093/zoolinnean/zlx028
Dornburg, A., and Near, T. J. (2021). The emerging phylogenetic perspective on the evolution of actinopterygian fishes. Annu. Rev. Ecol. Evol. Syst. 52, 427–452. doi: 10.1146/annurev-ecolsys-122120-122554
Drummond, A. J., Ho, S. Y. W., Phillips, M. J., and Rambaut, A. (2006). Relaxed phylogenetics and dating with confidence. PLoS Biol. 4:e88. doi: 10.1371/journal.pbio.0040088
Faircloth, B. C. (2015). PHYLUCE is a software package for the analysis of conserved genomic loci. Bioinformatics. 32, 786–788. doi: 10.1093/bioinformatics/btv646
Faircloth, B. C., Alda, F., Hoekzema, K., Burns, M. D., Oliveira, C., Albert, J. S., et al. (2020). A target enrichment bait set for studying relationships among ostariophysan fishes. Copeia 108, 47–60. doi: 10.1643/CG-18-139
Faircloth, B. C., McCormack, J. E., Crawford, N. G., Harvey, M. G., Brumfield, R. T., and Glenn, T. C. (2012). Ultraconserved elements anchor thousands of genetic markers spanning multiple evolutionary timescales. Syst. Biol. 61, 717–726. doi: 10.1093/sysbio/sys004
Faircloth, B. C., Sorenson, L., Santini, F., and Alfaro, M. E. (2013). A phylogenomic perspective on the radiation of ray-finned fishes based upon targeted sequencing of ultraconserved elements (UCEs). PLoS One 8:e65923. doi: 10.1371/journal.pone.0065923
Fink, S. V., and Fink, W. L. (1981). Interrelationships of the ostariophysan fishes (Teleostei). Zool. J. Lin. Soc. 72, 297–353. doi: 10.1111/j.1096-3642.1981.tb01575.x
Fink, S. V., and Fink, W. L. (1996). “Interrelationships of Ostariophysan Fishes (Teleostei),” in Interrelationships of fishes, eds M. L. J. Stiassny, L. R. Parenti, and G. D. Johnson (San Diego, CA: Academic Press), 209–249.
Fricke, R., Eschmeyer, W. N., and Fong, J. D. (2021). Species by family/subfamily. Available online at: http://researcharchive.calacademy.org/research/ichthyology/catalog/SpeciesByFamily.asp (accessed April 19, 2022).
Friedman, M., Feilich, K. L., Beckett, H. T., Alfaro, M. E., Faircloth, B. C., Èernı, D., et al. (2019). A phylogenomic framework for pelagiarian fishes (Acanthomorpha: Percomorpha) highlights mosaic radiation in the open ocean. Proc. R. Soc. B 286, 20191502. doi: 10.1098/rspb.2019.1502
Gayet, M., Jégu, M., Bocquentin, J., and Negri, F. R. (2003). New characoides from the upper cretaceous and paleocene of bolivia and the mio-pliocene of Brazil: Phylogenetic position and paleobiogeographic implications. J. Vert. Paleont. 23, 28–46.
Gayet, M., Marshall, L. G., Sempere, T., Meunier, F. J., Cappetta, H., and Rage, J.-C. (2001). Middle Maastrichtian vertebrates (fishes, amphibians, dinosaurs and other reptiles, mammals) from Pajcha Pata (Bolivia). Biostratigraphic, palaeoecologic and palaeobiogeographic implications. Palaeogeog. Palaeoclimat. Palaeoecol. 169, 39–68. doi: 10.1016/S0031-0182(01)00214-0
Ghezelayagh, A., Harrington, R. C., Burress, E. D., Campbell, M. A., Buckner, J. C., Chakrabarty, P., et al. (2021). Prolonged morphological expansion of spiny-rayed fishes following the end-Cretaceous. bioRxiv [Preprint] doi: 10.1101/2021.07.12.452083
Graham, J. B., Kramer, D. L., and Pineda, E. (1978). Comparative respiration of an air-breathing and a non-air-breathing characoid fish and the evolution of aerial respiration in characins. Physiol. Zool. 51, 279–288. doi: 10.1086/physzool.51.3.30155745
Harrington, R. C., Faircloth, B. C., Eytan, R. I., Smith, W. L., Near, T. J., Alfaro, M. E., et al. (2016). Phylogenomic analysis of carangimorph fishes reveals flatfish asymmetry arose in a blink of the evolutionary eye. BMC Evol. Biol. 16:224. doi: 10.1186/s12862-016-0786-x
Katoh, K., and Standley, D. M. (2013). MAFFT multiple sequence alignment software version 7: Improvements in performance and usability. Mol. Biol. Evol. 30, 772–780. doi: 10.1093/molbev/mst010
Katoh, K., Misawa, K., Kuma, K. I, and Miyata, T. (2002). MAFFT: A novel method for rapid multiple sequence alignment based on fast Fourier transform. Nucleic Acids Res. 30, 3059–3066. doi: 10.1093/nar/gkf436
Kolmann, M., Hughes, L., Hernandez, L., Arcila, D., Betancur-R, R., Sabaj, M., et al. (2020). Phylogenomics of piranhas and pacus (Serrasalmidae) uncovers how dietary convergence and parallelism obfuscate traditional morphological taxonomy. Syst. Biol. 70, 576–592. doi: 10.1093/sysbio/syaa065
Lanfear, R., Calcott, B., Ho, S. Y. W., and Guindon, S. (2012). PartitionFinder: Combined selection of partitioning schemes and substitution models for phylogenetic analyses. Mol. Biol. Evol. 29, 1695–1701. doi: 10.1093/molbev/mss020
Liem, K. F. (1988). Form and function of lungs: The evolution of air breathing mechanisms. Am. Zool. 28, 739–759. doi: 10.1093/icb/28.2.739
López-Fernández, H., and Albert, J. S. (2011). “Paleogene radiations,” in Historical biogeography of neotropical freshwater fishes, eds J. S. Albert and R. E. Reis (Berkeley, CA: University of California Press), 105–117.
Lundberg, J. G. (1997). “Freshwater fishes and their paleobiotic implications,” in Vertebrate paleontology in the neotropics: The miocene Fauna of La Venta, Colombia, eds R. F. Kay, R. H. Madden, R. L. Cifelli, and J. J. Flynn (Washington, DC: Smithsonian Institution), 67–91.
Lundberg, J. G., Sabaj Pérez, M. H., Dahdul, W. M., and Aguilera, O. A. (2009). “The Amazonian neogene fish fauna,” in Amazonia: Landscape and species evolution: A look into the past, eds C. Hoorn and F. P. Wesselingh (Chichester: Wiley-Blackwell Publishing Ltd), 281–301.
Malabarba, M. C. (1996). Reassessment and relationships of Curimata mosesi Travassos and Santos, a fossil fish (Teleostei: Characiformes: Curimatidae) from the Tertiary of São Paulo, Brazil. Comun. Mus. Cienc. Tecnol. Pucrs Ser. Zool. 9, 55–63.
Malabarba, M. C., and Malabarba, L. R. (2010). “Biogeography of characiformes: An evaluation of the available information of fossil and extant taxa,” in Origin and phylogenetic interrelationships of teleosts, eds J. S. Nelson, H. P. Schultze, and M. V. H. Wilson (Munich: Verlag Dr. Friedrich Pfeil), 317–336.
Mateussi, N. T., Melo, B. F., Ota, R. P., Roxo, F. F., Ochoa, L. E., Foresti, F., et al. (2020). Phylogenomics of the neotropical fish family Serrasalmidae with a novel intrafamilial classification (Teleostei: Characiformes). Mol. Phylogen. Evol. 153:106945. doi: 10.1016/j.ympev.2020.106945
McGee, M. D., Faircloth, B. C., Borstein, S. R., Zheng, J., Darrin Hulsey, C., Wainwright, P. C., et al. (2016). Replicated divergence in cichlid radiations mirrors a major vertebrate innovation. Proc. R. Soc. B Biol. Sci. 283, 20151413. doi: 10.1098/rspb.2015.1413
Melo, B. F., Albert, J. S., Dagosta, F. C. P., and Tagliacollo, V. A. (2021). Biogeography of curimatid fishes reveals multiple lowland-upland river transitions and differential diversification in the Neotropics (Teleostei, Curimatidae). Ecol. Evol. 11, 15815–15832. doi: 10.1002/ece3.8251
Melo, B. F., Sidlauskas, B. L., Hoekzema, K., Vari, R. P., Dillman, C. B., and Oliveira, C. (2018). Molecular phylogenetics of neotropical detritivorous fishes of the family Curimatidae (Teleostei: Characiformes). Mol. Phylogenet. Evol. 127, 800–812. doi: 10.1016/j.ympev.2018.06.027
Melo, B. F., Sidlauskas, B. L., Near, T. J., Roxo, F. F., Ghezelayagh, A., Ochoa, L. E., et al. (2022). Accelerated diversification explains the exceptional species richness of tropical characoid fishes. Syst. Biol. 71, 78–92. doi: 10.1093/sysbio/syab040
Mirande, J. M. (2017). Combined phylogeny of ray-finned fishes (Actinopterygii) and the use of morphological characters in large-scale analyses. Cladistics 33, 333–350. doi: 10.1111/cla.12171
Mirande, J. M. (2019). Morphology, molecules and the phylogeny of Characidae (Teleostei, Characiformes). Cladistics 35, 282–300. doi: 10.1111/cla.12345
Mirarab, S., and Warnow, T. (2015). ASTRAL-II: Coalescent-based species tree estimation with many hundreds of taxa and thousands of genes. Bioinformatics 31, i44–i52. doi: 10.1093/bioinformatics/btv234
Mirarab, S., Reaz, R., Bayzid, M. S., Zimmermann, T., Swenson, M. S., and Warnow, T. (2014). ASTRAL: Genome-scale coalescent-based species tree estimation. Bioinformatics 30, i541–i548. doi: 10.1093/bioinformatics/btu462
Monsch, K. A. (1998). Miocene fish faunas from the northwestern Amazonia basin (Colombia, Peru, Brazil) with evidence of marine incursions. Palaeogeogr. Palaeoclimatol. Palaeoecol. 143, 31–50. doi: 10.1016/S0031-0182(98)00064-9
Near, T. J., Eytan, R. I., Dornburg, A., Kuhn, K. L., Moore, J. A., Davis, M. P., et al. (2012). Resolution of ray-finned fish phylogeny and timing of diversification. Proc. Nat. Acad. Sci. U.S.A. 109, 13698–13703. doi: 10.1073/pnas.1206625109
Ochoa, L. E., Datovo, A., DoNascimiento, C., Roxo, F. F., Sabaj, M. H., Chang, J., et al. (2020). Phylogenomic analysis of trichomycterid catfishes (Teleostei: Siluriformes) inferred from ultraconserved elements. Sci. Rep. 10:2697. doi: 10.1038/s41598-020-59519-w
Oliveira, C., Avelino, G. S., Abe, K. T., Mariguela, T. C., Benine, R. C., Ortí, G., et al. (2011). Phylogenetic relationships within the speciose family Characidae (Teleostei: Ostariophysi: Characiformes) based on multilocus analysis and extensive ingroup sampling. BMC Evol. Biol. 11:275. doi: 10.1186/1471-2148-11-275
Pastana, M. N., Bockmann, F. A., and Datovo, A. (2020). The cephalic lateral-line system of Characiformes (Teleostei: Ostariophysi): Anatomy and phylogenetic implications. Zool. J. Linn. Soc. 189, 1–46. doi: 10.1093/zoolinnean/zlz105
Pelster, B. (2021). Using the swimbladder as a respiratory organ and/or a buoyancy structure – Benefits and consequences. J. Exp. Zool. Ecol. Integr. Physiol. 335, 831–842. doi: 10.1002/jez.2460
Rambaut, A., Suchard, M. A., Xie, W., and Drummond, A. J. (2014). Tracer v1.6.1. Available online at: http://beast.bio.ed.ac.uk (accessed January 1, 2022).
Ramirez, J. L., Birindelli, J. L., and Galetti, P. M. Jr. (2017). A new genus of Anostomidae (Ostariophysi: Characiformes): Diversity, phylogeny and biogeography based on cytogenetic, molecular and morphological data. Mol. Phylogenet. Evol. 107, 308–323. doi: 10.1016/j.ympev.2016.11.012
Roxo, F. F., Ochoa, L. E., Sabaj, M. H., Lujan, N. K., Covain, R., Silva, G. S. C., et al. (2019). Phylogenomic reappraisal of the Neotropical catfish family Loricariidae (Teleostei: Siluriformes) using ultraconserved elements. Mol. Phylogenet. Evol. 135, 148–165. doi: 10.1016/j.ympev.2019.02.017
Sempere, T., Butler, R., Richards, D., Marshall, L., Sharp, W., and Swisher Iii, C. (1997). Stratigraphy and chronology of upper cretaceous–lower paleogene strata in Bolivia and northwest Argentina. Geol. Soc. Am. Bull. 109, 709–727. doi: 10.1130/0016-76061997109<0709:SACOUC<2.3.CO;2
Silva, G. S., Melo, B. F., Roxo, F. F., Ochoa, L. E., Shibatta, O. A., Sabaj, M. H., et al. (2021a). Phylogenomics of the bumblebee catfishes (Siluriformes: Pseudopimelodidae) using ultraconserved elements. J. Zool. Syst. Evol. Res. 59, 1662–1672. doi: 10.1111/jzs.12513
Silva, G. S., Roxo, F. F., Melo, B. F., Ochoa, L. E., Bockmann, F. A., Sabaj, M. H., et al. (2021b). Evolutionary history of Heptapteridae catfishes using ultraconserved elements (Teleostei, Siluriformes). Zool. Scr. 50, 543–554. doi: 10.1111/zsc.12493
Soares, M. G. M., Menezes, N. A., and Junk, W. J. (2006). Adaptations of fish species to oxygen depletion in a central Amazonian floodplain lake. Hydrobiologia 568, 353–367. doi: 10.1007/s10750-006-0207-z
Souza, C. S., Melo, B. F., Mattox, G. M., and Oliveira, C. (2022). Phylogenomic analysis of the Neotropical fish subfamily Characinae using ultraconserved elements (Teleostei: Characidae). Mol. Phylogenet. Evol. 171:107462. doi: 10.1016/j.ympev.2022.107462
Stamatakis, A. (2014). RAxML version 8: A tool for phylogenetic analysis and post-analysis of large phylogenies. Bioinformatics 30, 1312–1313. doi: 10.1093/bioinformatics/btu033
Stiller, J., da Fonseca, R. R., Alfaro, M. E., Faircloth, B. C., Wilson, N. G., and Rouse, G. W. (2021). Using ultraconserved elements to track the influence of sea-level change on leafy seadragon populations. Mol. Ecol. 30, 1364–1380. doi: 10.1111/mec.15744
Sullivan, J. P., Lundberg, J. G., and Hardman, M. (2006). A phylogenetic analysis of the major groups of catfishes (Teleostei: Siluriformes) using rag1 and rag2 nuclear gene sequences. Mol. Phylogenet. Evol. 41, 636–662. doi: 10.1016/j.ympev.2006.05.044
Tagliacollo, V. A., and Lanfear, R. (2018). Estimating improved partitioning schemes for ultraconserved elements. Mol. Biol. Evol. 35, 1798–1811. doi: 10.1093/molbev/msy069
Tea, Y.-K., Xu, X., DiBattista, J. D., Lo, N., Cowman, P. F., and Ho, S. Y. (2021). Phylogenomic analysis of concatenated ultraconserved elements reveals the recent evolutionary radiation of the fairy wrasses (Teleostei: Labridae: Cirrhilabrus). Syst. Biol. 71, 1–12. doi: 10.1093/sysbio/syab012
Travassos, H., and Santos, R. S. (1955). Caracídeos fósseis da bacia do Paraíba. An. Acad. Bras. Ciênc. 27, 313–321.
Weiss, F. E., Malabarba, L. R., and Malabarba, M. C. (2012). Phylogenetic relationships of paleotetra, a new characiform fish (Ostariophysi) with two new species from the eocene-oligocene of south-eastern Brazil. J. Syst. Palaeont. 10, 73–86. doi: 10.1080/14772019.2011.565082
Weiss, F. E., Malabarba, M. C., and Malabarba, L. R. (2014). A new stem fossil characid (Teleostei: Ostariophysi) from the eocene-oligocene of southeastern Brazil. Neotrop. Ichthyol. 12, 439–450. doi: 10.1590/1982-0224-20140072
Keywords: Characiformes, Erythrinidae, Erythrinoidea, fossil calibration, Neotropics, phylogenomics, Ostariophysi, ultraconserved elements
Citation: Melo BF, de Pinna MCC, Rapp Py-Daniel LH, Zuanon J, Conde-Saldaña CC, Roxo FF and Oliveira C (2022) Paleogene emergence and evolutionary history of the Amazonian fossorial fish genus Tarumania (Teleostei: Tarumaniidae). Front. Ecol. Evol. 10:924860. doi: 10.3389/fevo.2022.924860
Received: 20 April 2022; Accepted: 20 July 2022;
Published: 16 August 2022.
Edited by:
Carolina Machado, Federal University of São Carlos, BrazilReviewed by:
Joao Filipe Tonini, University of Richmond, United StatesAnderson Oliveira Carmo, Universidade Federal de Minas Gerais, Brazil
Copyright © 2022 Melo, de Pinna, Rapp Py-Daniel, Zuanon, Conde-Saldaña, Roxo and Oliveira. This is an open-access article distributed under the terms of the Creative Commons Attribution License (CC BY). The use, distribution or reproduction in other forums is permitted, provided the original author(s) and the copyright owner(s) are credited and that the original publication in this journal is cited, in accordance with accepted academic practice. No use, distribution or reproduction is permitted which does not comply with these terms.
*Correspondence: Bruno F. Melo, Ym1lbG9AYW1uaC5vcmc=