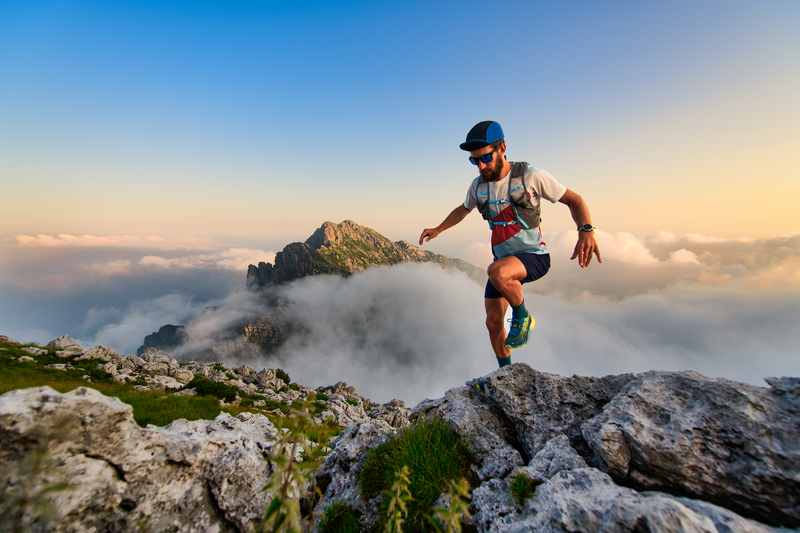
95% of researchers rate our articles as excellent or good
Learn more about the work of our research integrity team to safeguard the quality of each article we publish.
Find out more
ORIGINAL RESEARCH article
Front. Ecol. Evol. , 18 August 2022
Sec. Behavioral and Evolutionary Ecology
Volume 10 - 2022 | https://doi.org/10.3389/fevo.2022.923248
This article is part of the Research Topic Coevolution of Insect-Gut Microbiome View all 5 articles
Biological control of pests continues to become more important in agriculture as pesticides are being withdrawn. However, successful control can be compromised by contemporary evolution. Recent work in New Zealand has shown that the once-successful biological control programme of the sexually reproducing grassland weevil pest Listronotus bonariensis by the asexual parasitoid Microctonus hyperodae has now failed. To explain the mechanisms associated with this, weevil parasitism rates were intensively monitored between 1994 and 2019. Frequent sampling took place at widely dispersed New Zealand sites spanning the warmer northern regions to the cooler south. Based on elapsed heat accumulation above the parasitoid’s development temperature threshold of 10.2°C degree-day (DD), the results over c. 25 years indicated that the extent of parasitism decline at a given location was directly related to the accumulated DD. The latter, in turn, was taken to be indicative of parasitoid activity and selection pressure. Accordingly, laboratory microcosm experiments measuring the response of weevils collected from the North–South distribution to a common population of parasitoids showed that the weevils from the warmer northern region showed higher rates of avoidance of the searching parasitoids than those from the cooler south. This strongly supported the hypothesis that the weevil resistance mechanism is related to levels of parasitoid avoidance behaviour arising from long-term parasitoid selection pressure. This study of the behaviourally based acquisition of resistance to a biological control agent illustrates a general need to consider the potential capability of an exotic target host to develop resistance to imported biological control agents. This includes identifying existing host adaptations that selection pressure could potentially act upon that may compromise otherwise successful biological control programmes. Such a requirement points to the need for long-term monitoring of biological control systems and understanding of parasitoid/host dynamics.
This contribution refers to the ecology of the Argentine stem weevil, Listronotus bonariensis Kuschel (Coleoptera: Curculionidae; referred to hereafter as L. bonariensis or “the weevil”) and its response to the parthenogenetic parasitoid Microctonus hyperodae Loan (Hymenoptera: Braconidae; referred to hereafter as M. hyperodae or “the parasitoid”). The weevil is a particularly severe pest of New Zealand pasture grasses, causing c. $160M per annum damage to farming (Ferguson et al., 2019). The parasitoid was introduced into New Zealand in 1991 (Goldson et al., 1993) as an agent against the weevil, and it rapidly became effective, with parasitism rates averaging over 70% in the 1990s, but occasionally peaking at >90% (e.g., Goldson et al., 1998; Barker, 2013). An exception to this has been in southern New Zealand, where parasitism remained at c. 10–15% (Ferguson et al., 1997). While the beneficial effects of this biological control initiative were apparent (e.g., Goldson et al., 2011; Barker, 2013; Ferguson et al., 2019), Tomasetto et al. (2017) subsequently presented clear evidence that after c.14 weevil generations (7 years), national parasitism rates had declined by 44% with a consequent lack of weevil suppression (Popay et al., 2011). This was attributed to contemporary resistance by the weevil to the parasitoid (Goldson and Tomasetto, 2016; Tomasetto et al., 2017).
This paper explores the mechanism behind this first-documented example of an insect pest species hypothesised to have become resistant to its biological control agent (Goldson et al., 2014a; Mills, 2017; Pennisi, 2017; Tomasetto et al., 2017). The rareness of this event is quite different from the relatively common acquisition of pest resistance to insecticides (Sparks and Nauen, 2015). Possible reasons for the apparent ecological stability of biological control suppression elsewhere may include that biological control agents can co-evolve with a pest and thereby counteract resistance developing in the latter (Henter, 1995; Henter and Via, 1995). Furthermore, should a pest develop resistance to a control agent, it may not persist in a population because of the imposition of metabolic or other adaptive costs (e.g., Kraaijeveld and Godfray, 1997). Also, pest susceptibility to biological control agents can be maintained through either the presence of spatial (e.g., Hanski, 1981) or temporal refuges (e.g., Godfray et al., 1994). Finally, diverse agroecosystems may stabilise biological control by virtue of a wide range of natural-enemy guilds that collectively contribute to pest suppression (Tylianakis and Romo, 2010). Irrespective, it is now apparent that resistance to insect biological control agents can occur given the right circumstances. Although not an importation biological control programme per se, Pascoal et al. (2014, 2020) presented clear evidence of the contemporary evolution of an orthopteran host against its dipteran parasitoid in Hawaii. Considering the resistance of L. bonariensis to M. hyperodae, Goldson et al. (2014a,b) contended that the sexually reproducing weevil has greater adaptive capacity than the clonal M. hyperodae, resulting in an uneven evolutionary arms race (e.g., Mills, 2017). Subsequent modelling by Casanovas et al. (2018) has strongly supported this hypothesis. Furthermore, Goldson et al. (2014a,2020) have also noted that the dearth of natural enemy complexity in New Zealand’s species-poor exotic pastoral ecosystems has probably also contributed to the appearance of resistance.
While the decline of L. bonariensis parasitism is now well-documented (e.g., Goldson et al., 2014a,b, 2015, 2020; Goldson and Tomasetto, 2016; Tomasetto et al., 2017, 2018a,b; Casanovas et al., 2018), the mechanism behind this is less certain. Goldson et al. (2014b) speculated that parasitoid-exerted selection had enhanced weevil avoidance behaviours, leading to the reductions in parasitism. This idea was subsequently supported by Tomasetto et al. (2018a), who showed that the parasitoid’s searching efficiency had declined significantly between 1993 and 2018, implying increased avoidance behaviour by L. bonariensis. At the same time, ongoing research has revealed no other definitive factors that might singularly contribute to parasitism decline (Goldson et al., 2015, 2022), such as the occurrence of defensive bacterial endosymbionts (White et al., 2015).
This contribution further investigates the hypothesis that the mechanism for reduced parasitism of L. bonariensis is behaviourally based and that selection pressure for this effect is directly related to accumulated heat above the parasitoid’s development temperature threshold of 10.2°C.
New Zealand’s montane geography has a North–South orientation, with the bulk of its farmed landmass extending from c. 35.4oS to c. 46.0oS (therefore being c. 1,150 km long). Usefully, for the purposes of ecological experimentation, this offers a range of largely maritime climates extending from the almost subtropical climate in the North to the cool temperate climate in the South.
This study refers to three broad New Zealand regions. For the purposes of this contribution, these are referred to as the Northern, Central, and Southern Regions. Within these, specific localities are referred to and are at least 80 km apart. In the Northern Region, these localities are Ruakura (37°46′19.4″S 175°18′29.0″E) and Wellsford (36°14′14.1″S 174°30′48.7″E); in the Central Region, the locality is Lincoln (43°37′49.2″S 172°28′16.8″E); and in the Southern Region, the localities are Invermay (45°51′31.4″S 170°23′14.5″) and Waipiata (45°10′34.9″S 170°09′23.1″E). The use of these different localities within the regions was partly to ensure that there were no outlier locality biases. The Central Region data collection was focussed on the Lincoln locality and constituted the most thorough sampling regime in the country. The areas within each locality, where the collection of L. bonariensis and later M. hyperodae populations were made, were usually within 30 km of the coordinates listed above. These areas were intensively and repeatedly sampled in both space and time.
Stable diapausing L. bonariensis field parasitism rate data (Goldson, 1981; Goldson et al., 1993) were extracted from both published (Ferguson et al., 1997; Barker, 2013; Goldson et al., 2014a; Phillips and Kean, 2017; Tomasetto et al., 2017) and unpublished records (AgResearch). These data were collected from the Northern Region (Locality: Ruakura), Central Region (Locality: Lincoln), and the Southern Region (Locality: Invermay). Notably, Ruakura, Lincoln, and Invermay are the localities where major AgResearch science centres are located. The L. bonariensis field parasitism data were collected intensively between 1994 and 2000, between 2011 and 2019, and sporadically between 2000 and 2011.
By 2000, analysis and understanding of the parasitoid biology, ecology and impacts had been well researched in all three regions. Thereafter, sampling intensity decreased markedly until 2011, when there was evidence of parasitism decline (Popay et al., 2011). Sampling for diapausing parasitism rates ranged from May to August in the Northern Region (Locality: Ruakura) and from May to September in the Central (Locality: Lincoln) and Southern Regions (Locality: Invermay). Parasitism rates were determined by dissection based on >14 weevils per sample.
In considering the effects of regional temperature differences on the biology of M. hyperodae, extensive use was made of rates of heat accumulation. Between November to June inclusively, regional maximum and minimum air temperature data from 1993 to 2018 were collected (NIWA, 2019) when the adult parasitoids were active (i.e., non-diapausing; Phillips and Kean, 2017). Using these data, degree-day (DD) accumulation above the parasitoid’s development threshold of 10.2°C (Barlow et al., 1994) was calculated using the “4-step,” or “trapezoidal” approximation as described by Barlow et al. (1994).
The behavioural studies comprised two experiments involving early and late season L. bonariensis collected from different regions and localities. The intention was to see if the weevils from these places responded differently to the presence of the parasitoids. Both experiments were based on a common design, as mentioned below.
The experiments were maintained at 23°C, 60% humidity under a 16:8 light:dark photoperiod using a randomised block design. The weevils were starved for 3 days before the experiment but were provided with water-soaked dental wicks. The replicated arenas consisted of an upper part of a transparent polyvinyl chloride jar (230 mm × 12 mm × 8 mm), embedded in a-165 mm diameter plant pot containing moistened pasteurised soil collected from Lincoln pasture. The top of each jar was fitted with 0.1 mm mesh for ventilation, and the lower internal walls had a band of polytetrafluoroethylene applied to prevent the weevils from climbing up. Within 24 h of the experiments starting, 4-week-old nil-endophyte diploid perennial ryegrass Lolium perenne L. plants were embedded in the pots, and each plant was cropped to 150 mm and comprised 7–10 tillers. On the day of the experiments, 2 h before the onset of darkness, 10 weevils from each of the test regions and localities were added to the appropriate treatment jars. Ninety minutes later, a single parasitoid was also added to the parasitoid-present treatments; the controls had no such addition.
To obtain the parasitoids, field-collected weevils from Lincoln were maintained at room temperature pending prepupal M. hyperodae larvae emergence. After parasitism pupation and eclosion, the adults were kept in Petri dishes with dental wicks soaked with 10% honey-water (Phillips et al., 1996; Goldson and Tomasetto, 2016). In all experiments, the Lincoln parasitoids were ≤5 days old.
Once established, the following behavioural parameters were measured:
On-plant presence: This was measured as the total number of weevils on the plant. The on-plant population was taken to be at risk of parasitism, whereas the remainder were presumed less susceptible because they were often stationary or semi-buried in the soil.
Feeding: These weevils were characterised as having their heads down and their rostrums in contact with the plant. This configuration resulted in the abdomen being slanted upward, thereby exposing their inter-sternite membranes and anus to attack. In this position, the weevils are considered to be highly vulnerable (Phillips, 2002). Such weevil feeding behaviour was a subset of their on-plant presence.
These observations commenced at the onset of darkness and were conducted systematically during four consecutive 40–60 min observation periods using a red light.
The L. bonariensis populations for the early season experiment were collected from the Northern Region (Locality: Ruakura) on 8 January 2018, the Central Region (Locality: Lincoln) on 8 January 2018, and the Southern Region (Locality: Invermay) on 13 February 2018. These collections were made during the mass emergence of first summer generation adult weevils (late December to early February) when parasitism rates were low (Goldson et al., 1998; Phillips and Kean, 2017). Before the experiment, these weevils were maintained in cages for c. 3 weeks (in order to purge any parasitoids present), to which bouquets of nil-endophyte tetraploid Lolium multiflorum Lam. were added as food twice a week. As per the design, the parasitoids for the early season experiment were obtained from parasitised weevils collected in the Central Region (Locality: Lincoln) on 8 January and supplemented parasitoids from other weevils, again collected from the Central Region (Locality: Lincoln) in late February and early March 2018 when parasitism rates were relatively high (Goldson et al., 2011; Phillips and Kean, 2017).
The experiment was conducted in the abiotic conditions described above and comprised a randomised block design consisting of six treatments, viz. the three weevil populations being tested were collected from the Northern Region (Locality: Ruakura), the Central Region (Locality: Lincoln), and the Southern Region (Locality: Invermay). These were subjected to either the presence or absence (control) of the parasitoids. For reasons of space and parasitoid availability, the first six blocks were established on 9 March and the remaining five blocks on 23 March. Observational data (as above) were collected on the same dates as the blocks’ establishment.
The L. bonariensis populations for the late-season weevil experiment were collected from the Northern Region (Localities: Ruakura and Wellsford) on 19 April 2018 and the Southern Region (Locality: Waipiata) on 26 April 2018. These autumnal collections were made after the onset of diapause when parasitism rates were moderate (Goldson et al., 1998; Phillips and Kean, 2017). Before the experiment, the weevils were maintained in cages for up to 21 days, to which bouquets of nil-endophyte tetraploid L. multiflorum Lam. were added as food two times a week. The parasitoids for the late-season weevil experiment were obtained from weevils collected from the Central Region (Locality: Lincoln) in early March 2018.
The experiment was conducted in the same abiotic conditions as described above. For reasons of space and parasitoid availability, the first six blocks were established on 30 April, the next six blocks on 4 May and the last six blocks on 10 May 2018. Observational data (as above) were collected on the same dates as the blocks’ establishment.
Analyses of the field parasitism rates and accumulated DD calculations across the three regions were conducted using SAS version 9.4 (SAS Institute Inc, 2018). The parasitism rate data were analysed using an analysis of variance (ANOVA) and comprised two factors, these are the regional parasitism rates and the two sampling periods (i.e., 1994–2000 or 2011–2019). A comparison of accumulated DD across the three regions during the period of M. hyperodae activity (non-diapause) was conducted using ANOVA with locality as the only factor.
Behavioural analysis was based on two variables, the percentage of L. bonariensis showing “on-plant presence” and “feeding” (out of the 10 weevils in each replicate). The data were first analysed using R version 3.6.3 (R Core Team, 2018) with a generalised linear mixed-effects model with a penalised quasi-likelihood estimation (GLMMPQL) to indicate overall behavioural trends. The GLMMPQL was applied to all data collected in all four observation periods in each behavioural experiment and correlation was modelled based on observations first-order autoregressive random effects. The fixed effects of the GLMMPQL were sampling regions, present/absent parasitoid, and their interaction using binomial distributions through a logit link function. The GLMMPQL analysis was conducted using the glmmPQL function in the R package MASS (Venables and Ripley, 2002). Next, behavioural responses over time were investigated by fitting a Generalised Linear Model (GLM) with a binomial error term using GenStat Release 20.1 (VSN International, 2019). All GLM assumptions were met. Model terms were replicated (a blocking factor) by sampling locality, plus/minus parasitoid, and their interaction. A (log-odds) “logit” “link function” was specified, and the “dispersion parameter” was estimated rather than fixed. Each GLM gave a “prediction” of the six means and estimated a least significant difference (LSD) at P < 0.05 for comparing each of the 6C2 = 15 pairs of means; these LSDs were relatively small for comparing two small means, large for comparing two large means and intermediate for comparing a small and large mean. A GLM was independently fitted for each of the two behaviours in each of the four observation periods in the early and season experiments.
During the 1994–2000 period, mean weevil parasitism rates when in diapause were significantly different amongst all regions, and thereafter, the extent of parasitism decline that occurred from 2011 to 2019 also varied greatly amongst the regions (Figure 1).
Figure 1. Mean percent parasitism of diapausing Listronotus bonariensis (horizontal bars indicate mean during each period) in the Southern Region (Locality: Invermay), the Central Region (Locality: Lincoln), and the Northern Region (Locality: Ruakura) of New Zealand during the periods 1994–2000 and 2011–2019. Points (•) indicate annual mean overwintering parasitism rates. The slope of the dotted lines (—) indicate the Regional rates of parasitism decline. Error bars = standard error of the mean overwintering parasitism rate for each year.
In the Northern Region (Locality: Ruakua), in 1994–2000 period, the mean parasitism rate was 70.4% ± 2.4% [mean ± standard error (SE)] but reduced to 13.7% ± 2.0% (mean ± SE) in the 2011–2019 period [t = 16.6, degrees of freedom (df) = 214, P < 0.001] (Figure 1). Likewise, in the Central Region (Locality: Lincoln), during the 1994–2000 period, the mean parasitism rate was 61.6% ± 2.2% (mean ± SE) but reduced to 32.0% ± 2.1% (mean ± SE) in the 2011–2019 period (t = 10.5, df = 214, P < 0.001; Figure 1). In the Southern region (Locality: Invermay), there were no significant changes in the parasitism rates between the 1994–2000 and 2011–2019 periods (t = 0.1, df = 214, P > 0.05; Figure 1).
In the Northern Region (Locality: Ruakua), the extent of parasitism decline was significantly greater (56.6% ± 3.4%) than that in the Central Region (Locality: Lincoln; 29.6% ± 2.8%) and the Southern Region (Locality: Invermay; 1.1% ± 10.6%; t = 6.1 and 5.0, respectively, df = 214, P < 0.001 for both). Furthermore, the extent of the Central Region (Locality: Lincoln) decline (56.6% ± 3.4%) was significantly greater than that of the Southern Region (Locality: Invermay) decline (1.1% ± 10.6%; t = 2.6, df = 214, P < 0.01; Figure 1).
Measured between 1993 and 2018, the rate of November–June DD heat accumulation per 24 h period above the M. hyperodae 10.2°C development threshold was significantly different between the regions (t ≥ 9.4, df = 75, P < 0.001; Figure 2). The results followed a latitudinal gradient with the Northern Region (Locality: Ruakura) having the highest mean DD accumulation at (5.442 DD ± 0.133 DD; mean ± SE) followed by Central Region (Locality: Lincoln; 3.268 DD ± 0.106 DD) and the Southern Region (Locality: Invermay; 1.761 DD ± 0.097 DD; Figure 2).
Figure 2. Mean 24 h accumulation degree-days (DD) occurring above the 10.2°C development threshold of Microctonus hyperodae in the Southern Region (Locality: Invermay), the Central Region (Locality: Lincoln), and Northern Region (Locality: Ruakura) of New Zealand between November and June 1993–2018. Error bars represent the standard error of the mean daily DD occurring during the period of parasitoid activity.
The results of on-plant presence in the early season weevil experiment are summarised in Table 1.
The weevil feeding responses to the parasitoid were far more pronounced than the on-plant presence responses (Tables 1, 2).
In the presence of M. hyperodae, the Northern Region (Locality: Ruakura) weevils spent significantly less time on the plants than when the parasitoid was absent (t = 2.1, df = 256, P < 0.05).
The GLM analysis also suggested that the Northern Region (Locality: Ruakura) weevils that remained on the plant in the presence of the parasitoids moved to the lower parts of the plant. This displacement was observed in all four 40–60 min observation periods but was only statistically significant in observation period 2 (df = 49, P < 0.05; Table 1). Conversely, the weevils from the Central Region (Locality: Lincoln) and the Southern Region (Locality: Invermay) showed no significant on-plant responses to the presence of the parasitoid compared to when the parasitoid was absent (t = 0.1, df = 256, P > 0.05) and (t = 0.3, df = 256, P > 0.05), respectively.
When exposed to the parasitoid, the GLMMPQL analysis showed that the Northern Region (Locality: Ruakura) weevils had significantly fewer individuals on the plants than was found with weevils from the Central Region (Locality: Lincoln; t = –3.2, df = 256, P < 0.05) and Southern Region (Locality: Invermay; t = –3.1, df = 256, P < 0.05). The GLM analysis showed the same trend across each of the four observation periods (df = 49 for all, P < 0.05; Table 1). There were no significant differences in on-plant presence responses between the weevil populations of the Central and Southern Regions in the presence of the parasitoid.
The feeding responses by L. bonariensis to M. hyperodae were far more pronounced than their on-plant presence responses (Table 1).
The GLMMPQL analysis for the early season weevil experiment indicated that a lower proportion of the Northern Region (Locality: Ruakura) L. bonariensis were feeding when M. hyperodae was present compared to when it was absent (t = 3.3, df = 256, P < 0.01; Figure 3).
Figure 3. The early season weevil experiment. Mean percentages of Listronotus bonariensis feeding in the absence or presence of Microctonus hyperodae. The weevils were collected from the Southern Region (Locality: Invermay), the Central Region (Locality: Lincoln), and the Northern Region (Locality: Ruakura) of New Zealand. Rectangles show the mean (central line) and 95% CL, and tails show the range.
Similarly, the GLM analysis showed that Northern Region (Locality: Ruakura) weevils consistently reduced feeding in response to M. hyperodae across all four observation periods; the reduction was statistically significant in observation periods 2, 3, and 4 (df = 49 for all, P < 0.01, P < 0.05 and P < 0.01, respectively; Table 2).
Conversely, the GLMMPQL suggested there was no such significant feeding response to the parasitoid shown by weevils from either the Central Region (Locality: Lincoln; t = 1.5, df = 256, P > 0.05) or the Southern Region (Locality: Invermay; t = 1.4, df = 256, P > 0.05).
However, the more targeted GLM analysis showed that in observation period 3, Central Region (Locality: Lincoln) weevils had significantly reduced feeding (df = 49, P < 0.05) in response to the parasitoid (mean ± SE: 23.6% ± 4.1%) compared to when the parasitoid was absent (40.0% ± 4.7%; Table 2).
In observation period 4, the Southern Region (Locality: Invermay) weevils showed a significantly lower percentage of feeding (18.2% ± 3.7%) in response to M. hyperodae presence compared to its absence (34.9% ± 4.8%; df = 49, P < 0.05).
When exposed to the parasitoid, the GLMMPQL analysis showed that the Northern Region (Locality: Ruakura) weevils showed significantly less feeding than those from the Central Region (Locality: Lincoln; t = –2.2, df = 256, P < 0.05) or the Southern Region (Locality: Invermay; t = –3.3, df = 256, P < 0.01; Figure 3). GLM analysis also showed that in the presence of M. hyperodae significantly fewer Northern Region (Locality: Ruakura) weevils were observed to be feeding compared to those from the Southern Region (Locality: Invermay) in observation periods 2 and 3 (df = 49 for both, P < 0.001 and P < 0.05, respectively) and from the Central Region (Locality: Lincoln) in observation period 2 (df = 49, P < 0.05; Table 2).
The results of on-plant presence in the late-season weevil experiment are summarised in Table 3.
The GLMMPQL analysis revealed no significant on-plant presence responses to M. hyperodae (t ≥−0.7, df = 422, P > 0.05). However, while the results were predominantly non-significant, indications were that the Northern Region (Localities: Ruakura and Wellsford) populations of weevils responded to M. hyperodae by moving off the leaves in a way similar to how the Northern Region weevils (Ruakura) responded in the early season experiment (Table 1).
In the parasitoid’s absence, the GLMMPQL indicated there were significantly more Northern Region (Locality: Ruakura) weevils observed on the plants compared to Southern Region (Locality: Waipiata) weevils (t = 2.0, df = 422, P < 0.05). This was by supported the GLM, which showed that in observation period 4, in the parasitoid’s absence, there were significantly fewer Southern Region (Locality Waipiata) weevils on the plants compared to those from Northern Region (Locality Ruakura) weevils (df = 83, P < 0.05).
The feeding responses by L. bonariensis to M. hyperodae were far more pronounced than their on-plant presence responses.
The GLMMPQL analysis for the late-season weevil experiment indicated that the Northern region (Locality: Ruakura) weevils showed significantly reduced feeding when M. hyperodae was present compared to when it was absent (t = 4.4, df = 422, P < 0.001; Figure 4).
Figure 4. The late-season weevil experiment. Mean percentages of Listronotus bonariensis feeding in the absence or presence of Microctonus hyperodae. The weevils were collected from the Southern Region (Locality: Waipiata) and the Northern Region (Localities: Wellsford and Ruakura) of New Zealand. Rectangles show the mean (central line) and 95% CL, and tails show the range.
Similarly, the GLM analysis showed that Northern Region (Locality: Ruakura) weevils showed significantly reduced feeding in response to M. hyperodae in observation periods 1, 2, and 4 (df = 83 for all, P < 0.01, P < 0.001, and P < 0.001, respectively; Table 4).
Likewise, the other Northern Region (Locality: Wellsford) weevils also had significantly reduced feeding in response to M. hyperodae in observation periods 3 and 4 (df = 83 for both, P < 0.05 and P < 0.01, respectively). There was no significant feeding response to the parasitoid by the Southern Region (Locality: Waipiata) weevils.
When exposed to the parasitoid, the GLMMPQL analysis indicated that the Northern Region (Locality: Ruakura) weevils showed significantly reduced feeding compared to those also from Northern Region (Locality: Wellsford; t = –2.5, df = 422, P < 0.05). When using the GLM analysis, this finding was found to have occurred in observation periods 1 and 2 (df = 83 for both, P < 0.05 and P < 0.01, respectively; Table 4).
In the absence of the parasitoid, the GLMMPQL analysis indicated that there were significantly fewer Southern Region (Locality: Waipiata) weevils feeding compared to the two Northern Region populations at Locality Ruakura (t = –3.4, df = 422, P < 0.001) and Locality Wellsford (t = –3.3, df = 422, P < 0.05; Figure 4). This finding is also supported by the GLM analysis, where there were significantly fewer Southern Region (Locality Waipiata) weevils observed to be feeding than in the Northern Region weevils (Localities: Ruakura and Wellsford) during observation periods 2, 3, and 4 (df = 83 for all, P < 0.05; Table 4).
This study considered the extent of M. hyperodae parasitism decline since the 1990s which was greatest in the warmer regions of New Zealand and graded to negligible in the cooler South. Furthermore, the mechanism for this is likely to have been varied selection pressure levels for enhanced evasion by L. bonariensis.
The results have indicated significant differences (since the 1990s) in the levels of long-term field parasitism decline within each of the weevil populations from the three regions (Figure 1). These corresponded to the rates of DD accumulation. In the Central and Northern Regions, Goldson et al. (1998) indicated that, respectively, first-generation parasitoid egg and first instar development rates were 17–42% and 11–16% faster in the Northern Region (Locality: Ruakura) than in the Central Region (Locality: Lincoln). This DD effect was even more apparent in the Northern Region (Locality: Ruakura), considering the late autumn/winter third-generation development which took 130 days less than in the Central Region (Locality: Lincoln; Goldson et al., 1998). Given that the long-term regional differences in DDs (Figure 2) strongly corresponded to the extent of this regional weevil parasitism decline (Figure 1), it is reasonable to consider the cumulative extent of DD build-up as a proxy for selection pressure.
The exception here is that in the Southern Region, parasitism rates have always remained very low c. 8% (Figure 1), obviating any possible selection pressure. This is supported by the little to no avoidance behaviour exhibited by Southern Region weevil populations (Figures 3, 4 and Tables 1–4). The reason for the limited parasitism rates demands further research, but it could be related to the climatical limitation of the parasitoid’s range as suggested by the low rate of DD accumulation.
Contrary to the observed regional effect on parasitism decline discussed here, Tomasetto et al. (2018b) suggested that the differences in DD accumulation between the two regions (Northern and Central) had not brought about changes in overwintering parasitism rates and therefore contended that time was the only correlate of the appearance of resistance. However, their conclusion was based on broad national parasitism trends, and unlike in this study, no data had been collected beyond 2016. Furthermore, these workers did not have access to the additional historical raw data used in this study.
Given that the graded extent of regional weevil parasitism decline is based on the extent of parasitoid selection pressure as per the DD accumulation, this study has sought to discover how this is manifest.
The strongest observed weevil population response to the parasitoid was feeding reduction (Barratt et al., 1996a; Gerard, 2000; Phillips, 2002). Based on the arena experiments, weevil cessation-of-feeding responses were highest in those populations collected from areas with high DD accumulation (Northern Region) through to no response with low DD accumulation (Southern Region). When there was an intermediate DD accumulation (Central Region), the non-feeding responses were correspondingly less pronounced (Figures 3, 4 and Tables 2, 4). A slight anomaly to this generalisation resulted from the weevils collected from the Northern Region (Locality: Wellsford). These still showed a typically reduced feeding response, but it was delayed, albeit to a lesser extent than the weevils from regions further south (Table 4). This weaker response could possibly be explained by the weevils’ changed physiological condition, as the late season test weevils were collected during diapause by necessity (Goldson, 1981).
Linked to this, an associated response was reduced weevils on the foliage. Significantly, this reaction was strong enough in the early season weevil experiment to overcome any fitness costs associated with reduced optimal feeding and ovipositional opportunity (Goldson, 1982; Barker, 1989). Broadly, weevil numbers on the foliage in the presence of the parasitoid showed the same pattern as reduced feeding, with lower numbers amongst populations collected from the Northern Region compared to those from further south. There was no significant on-plant presence response in the late-season weevil experiment (Table 3) which again, could be explained by the weevils having been collected during diapause.
The acquired resistance of L. bonariensis to M. hyperodae is based on parasitoid evasion, which is known to have a lower reproductive cost than physiological immunity (Lefèvre et al., 2012; Lynch et al., 2016). Moreover, it is the least disruptive form of resistance that is known to rapidly generate new phenotypes (Sih et al., 2011).
With regard to the origin of the L. bonariensis avoidance of M. hyperodae, fieldwork by Barker (2013) has shown that it is possible to discern that, in the presence of the parasitoid, weevil oviposition is largely suspended until the parasitoid density has declined (e.g., via an intergenerational gap). Thereafter in the parasitoid’s absence, there is an ovipositional pulse’ by the weevil. Given the weevil’s susceptibility to M. hyperodae parasitism during oviposition and feeding (Phillips, 2002; Cournoyer and Boivin, 2005), it can be argued the selection pressure discussed here has led to an accentuation of already-existing weevil responses to the parasitoid.
Based on their population analyses, Tomasetto et al. (2017, 2018b) concluded that the genetic competency for the appearance of resistance in the weevil was likely to be evenly distributed across all locations and possibly associated with the increased prevalence of certain genotypic changes, but not spreading from a point source. Consistent with this idea, Harrop et al. (2020), in their extensive genomic survey of L. bonariensis, based on GBS analysis, found that the weevil populations showed high levels of heterozygosity and low population structure, indicating a large effective population size and frequent gene flow. They also noted that a large amount of standing genetic variation in weevil populations could be sufficient to encode phenotypic variation in parasitoid survivability and that resistance was most likely to have been through multiple genomic regions of small effect. These observations of both Tomasetto et al. (2018a) and Harrop et al. (2020) contrast strongly with the finding of Pascoal et al. (2014, 2020), who examined the rapid evolution of resistance in a Hawaiian population of field crickets [Teleogryllus oceanicus (Le Guillou; Orthoptera: Gryllidae) to the parasitoid fly, Ormia ochrea (Bigot; Diptera: Tachinidae)]. They showed that a resistance-causing point mutation in T. oceanicus spread through the cricket population, greatly reducing parasitism rates.
With regard to L. bonariensis parasitism decline, a number of alternative hypotheses have been considered. Ideas have included influences such as changes in farming practices, seasonal changes, and pasture grass endophytes (e.g., Gerard et al., 2021). However, earlier investigations into such proposed mechanisms have found that they do not accommodate aspects such as the regional patterns of parasitism decline or the different regions’ weevil populations showing varying levels of parasitoid evasion.
The loss of M. hyperodae efficacy has serious economic implications. Therefore, some consideration has been given to increasing the adaptive capacity of the parasitoid. This could include the introduction of new parasitoid strains additional to those previously introduced to New Zealand (Phillips et al., 2008). There is also the possibility of making M. hyperodae sexually reproductive, thereby permitting laboratory-based selection processes to improve the parasitoid’s adaptive potential.
The resistance of L. bonariensis to M. hyperodae, as the first documented example of resistance-based insect biological control failure (Mills, 2017; Pennisi, 2017), has implications for biological control generally. An immediate candidate for consideration would be the clover root weevil Sitona obsoletus Gmelin (Coleoptera: Curculionidae), which is a severe pest of white clover in New Zealand pasture (Ferguson et al., 2019). This weevil is controlled by another parthenogenetic congeneric wasp, Microctonus aethiopoides Loan (Hymenoptera: Braconidae; e.g., Gerard et al., 2011). Given the closeness of the system discussed here to that of S. obsoletus and M. aethiopoides, there is good reason to suppose that resistance could occur in a similar way. Therefore, consideration should be given to the genetic diversity of S. obsoletus and whether the species has any of the characteristics of L. bonariensis, including the extent of its genetic diversity. S. obsoletus was first described in New Zealand c. 1996 (Barratt et al., 1996b) but the first incursion was probably some 5 years earlier (Barker et al., 1996). Irrespective, the species’ initial limited distribution in the Auckland area (Barker et al., 1996) would suggest that only a single incursion had occurred, therefore, there may be insufficient genetic diversity for resistance compared to L. bonariensis.
More generally, it is widely recognised that many exotic pest species have established and caused problems in simplified non-complex agricultural ecosystems with limited biotic resistance and thus offer enemy release (e.g., Goldson et al., 2014b,2020; Tomasetto et al., 2017; Ferguson et al., 2019). As a result, biological control practitioners have often imported natural enemies from the pests’ native ranges for biological control purposes (e.g., Heimpel and Mills, 2017). Given that the pest and its control agent frequently originate from the same native range, it is possible that the pest may have, at least to some degree, resistance adaptations toward its co-evolved natural enemies. For example, Kraaijeveld et al. (1998) and Lapchin (2002) have pointed out that some pre-adapted defensive responses of pests to their parasitoids can provide at least some level of fitness when confronted by a wide range of natural enemies. Thus, as contended in this study, when biotic and abiotic circumstances permit, possible resistance traits in an exotic pest species can be acted on by high control agent selection pressure, thereby leading to pest resistance. This particularly applies to biological control programmes with unequal adaptive capacity in the pest’s favour, as referenced in this contribution (Casanovas et al., 2018). Speculatively, these considerations may be part of the reason for the very low success rate reported in importation biological control initiatives (e.g., Cock et al., 2016).
There may well indeed be other unpublished examples of what is discussed in this contribution. However, typically, sparse long-term attention is paid to biological control programmes after the agents’ initial introductions and sometimes, evaluations. Furthermore, if biological control failure is behaviourally based, as in this study, this indeed can be a difficult component to measure.
The original contributions presented in this study are publicly available. This data can be found here in the Lincoln University Data Repository, doi: 10.25400/lincolnuninz.20416419.v1. Any further queries should be directed to the corresponding author.
MS and SLG conceived the ideas, designed the study, and led the manuscript writing. MS conducted the experiments. MS, SW, SLG, PJG, and CBP collected the data. MS, CVK, and CBP analysed the data. All authors contributed to the drafts and gave final approval for publication.
This project was supported by the New Zealand Tertiary Education Commission CoRE grant to the Bio-Protection Research Centre (BPRC) and Lincoln University and AgResearch Ltd., New Zealand.
The authors are grateful for the collaboration from colleagues at AgResearch Ltd. and the BPRC, including Jacquelyn Bennett, Kate Scanlan, Malvika Bana, Derick Wilson, Colin Fergusson, Dave Saville, Federico Tomasetto, Louise Hennessy, Paula Casanovas, Peter Dearden, Jeanne Jacobs, Sarah Inwood, and Will Godsoe.
The authors declare that the research was conducted in the absence of any commercial or financial relationships that could be construed as a potential conflict of interest.
All claims expressed in this article are solely those of the authors and do not necessarily represent those of their affiliated organizations, or those of the publisher, the editors and the reviewers. Any product that may be evaluated in this article, or claim that may be made by its manufacturer, is not guaranteed or endorsed by the publisher.
Barker, G. M. (1989). Grass host preferences of Listronotus bonariensis (Coleoptera: Curculionidae). J. Econ. Entomol. 82, 1807–1816. doi: 10.1093/jee/82.6.1807
Barker, G. M. (2013). Biology of the introduced biocontrol agent Microctonus hyperodae (Hymenoptera: Braconidae) and its host Listronotus bonariensis (Coleoptera: Curculionidae) in Northern New Zealand. Environ. Entomol. 42, 902–914. doi: 10.1603/EN11248
Barker, G. M., Addison, P. J., Firth, A. C., and Barratt, B. I. P. (1996). Sitona lepidus Gyllenhal newly established in New Zealand: assessment of distribution in the North Island. Proc. New Zeal. Plant Prot. Conf. 49, 266–269. doi: 10.30843/nzpp.1996.49.11453
Barlow, N. D., Goldson, S. L., and McNeill, M. R. (1994). A prospective model for the phenology of Microctonus hyperodae (Hymenoptera: Braconidae), a potential biological control agent of Argentine stem weevil in New Zealand. Biocontrol Sci. Technol. 4, 375–386. doi: 10.1080/09583159409355347
Barratt, B. I. P., Evans, A. A., and Johnstone, P. D. (1996a). Effect of the ratios of Listronotus Bonariensis and Sitona Discoideus (Coleoptera: Curculionidae) to their respective parasitoids Microctonus hyperodae and M. aethiopoides (Hymenoptera: Braconidae), on parasitism, host oviposition and feeding in the laboratory. Bull. Entomol. Res. 86, 101–108. doi: 10.1017/S0007485300052329
Barratt, B. I. P., Barker, G. M., and Addison, P. J. (1996b). Sitona lepidus Gyllenhal (Coleoptera: Curculionidae), a potential clover pest new to New Zealand. New Zeal. Entomol. 19, 23–30. doi: 10.1080/00779962.1996.9722017
Casanovas, P., Goldson, S. L., and Tylianakis, J. M. (2018). Asymmetry in reproduction strategies drives evolution of resistance in biological control systems. PLoS One 13:e0207610. doi: 10.1371/journal.pone.0207610
Cock, M. J. W., Murphy, S. T., Kairo, M. T. K., Thompson, E., Murphy, R. J., and Francis, A. W. (2016). Trends in the classical biological control of insect pests by insects: an update of the biocat database. BioControl 61, 349–363. doi: 10.1007/s10526-016-9726-3
Cournoyer, M., and Boivin, G. (2005). Short distance cues used by the adult parasitoid Microctonus hyperodae Loan (Hymenoptera: Braconidae, Euphorinae) for host selection of a novel host Listronotus oregonensis (Coleoptera: Curculionidae). J. Insect Behav. 18, 577–591. doi: 10.1007/s10905-005-5614-x
Ferguson, C. M., Barratt, B. I. P., Bell, N., Goldson, S. L., Hardwick, S., Jackson, M., et al. (2019). Quantifying the economic cost of invertebrate pests to New Zealand’s pastoral industry. New Zeal. J. Agric. Res. 62, 255–315. doi: 10.1080/00288233.2018.1478860
Ferguson, C. M., Evans, A. A., Barratt, B. I. P., and Phillips, C. B. (1997). Establishment and dispersal of Microctonus hyperodae Loan (Hymenoptera: Braconidae) in Otago and Southland. Proc. New Zeal. Plant Prot. Conf. 50, 41–46. doi: 10.30843/nzpp.1997.50.11274
Gerard, P., Wilson, D., and Upsdell, M. (2021). Contrasting host: parasitoid synchrony drives differing levels of biocontrol by two introduced Microctonus spp. in northern New Zealand pastures. BioControl 66, 727–737. doi: 10.1007/s10526-021-10104-8
Gerard, P. J. (2000). Ryegrass endophyte infection affects Argentine stem weevil adult behaviour and susceptibility to parasitism. New Zeal. Plant Prot. 53, 406–409. doi: 10.30843/nzpp.2000.53.3654
Gerard, P. J., Wilson, D. J., and Eden, T. M. (2011). Field release, establishment and initial dispersal of Irish Microctonus aethiopoides in Sitona lepidus populations in northern New Zealand pastures. BioControl 56, 861–870. doi: 10.1007/s10526-011-9367-5
Godfray, H. C. J., Hassell, M. P., and Holt, R. D. (1994). The population dynamic consequences of phenological asynchrony between parasitoids and their hosts. J. Anim. Ecol. 63, 1–10. doi: 10.2307/5577
Goldson, S. L., McNeill, M. R., Bana, M., Olaniyan, O., Popay, A. J., Barratt, B. I. P., et al. (2022). Implications of sampling bias and population behaviour in the study of parasitoid-based biocontrol of Listronotus bonariensis in New Zealand’s exotic pasture ecosystems. New Zeal. J. Agric. Res. doi: 10.1080/00288233.2022.2084121
Goldson, S., Tomasetto, F., and Popay, A. (2015). Effect of Epichloë endophyte strains in Lolium spp. cultivars on Argentine stem weevil parasitism by Microctonus hyperodae. New Zeal. Plant Prot. 68, 204–211. doi: 10.30843/nzpp.2015.68.5807
Goldson, S., Wratten, S., Ferguson, C., Gerard, P., Barratt, B., Hardwick, S., et al. (2014a). If and when successful classical biological control fails. Biol. Control 72, 76–79. doi: 10.1016/j.biocontrol.2014.02.012
Goldson, S. L., Tomasetto, F., and Popay, A. J. (2014b). Biological control against invasive species in simplified ecosystems: its triumphs and emerging threats. Curr. Opin. Insect Sci. 5, 50–56. doi: 10.1016/j.cois.2014.09.003
Goldson, S. L. (1981). Reproductive diapause in the Argentine stem weevil, Listronotus bonariensis, (Kuschel) (Coleoptera: Curculionidae), in New Zealand. Bull. Entomol. Res. 71, 275–287. doi: 10.1017/S0007485300008300
Goldson, S. L. (1982). An examination of the relationship between Argentine stem weevil Listronotus bonariensis (Kuschel) and several of its host grasses. New Zeal. J. Agric. Res. 25, 395–403. doi: 10.1080/00288233.1982.10417903
Goldson, S. L., Barker, G. M., Chapman, H. M., Popay, A. J., Stewart, A. V., Caradus, J. R., et al. (2020). Severe insect pest impacts on New Zealand pasture: the plight of an ecological outlier. J. Insect Sci. 20:17. doi: 10.1093/jisesa/ieaa018
Goldson, S. L., Barron, M. C., Kean, J. M., and Van Koten, C. (2011). Argentine stem weevil (Listronotus bonariensis, Coleoptera: Curculionidae) population dynamics in Canterbury, New Zealand dryland pasture. Bull. Entomol. Res. 101, 295–303. doi: 10.1017/S0007485310000507
Goldson, S. L., Mcneill, M. R., Proffitt, J. R., Barker, G. M., Addison, P. J., Barratt, B. I. P., et al. (1993). Systematic mass rearing and release of Microctonus hyperodae (Hym.: Braconidae, Euphorinae), a parasitoid of the Argentine stem weevil Listronotus bonariensis (Col.: Curculionidae) and records of its establishment in New Zealand. Entomophaga 38, 527–536. doi: 10.1007/BF02373087
Goldson, S. L., Proffitt, J. R., and Baird, D. B. (1998). Establishment and phenology of the parasitoid Microctonus hyperodae (Hymenoptera: Braconidae) in New Zealand. Environ. Entomol. 27, 1386–1392. doi: 10.1093/ee/27.6.1386
Goldson, S. L., and Tomasetto, F. (2016). Apparent acquired resistance by a weevil to its parasitoid is influenced by host plant. Front. Plant Sci. 7:1259. doi: 10.3389/fpls.2016.01259
Hanski, I. (1981). Coexistence of competitors in patchy environments with and without predation. Oikos 37, 306–312. doi: 10.1377/hlthaff.2010.0023
Harrop, T. W. R., Le Lec, M. F., Jauregui, R., Taylor, S. E., Inwood, S. N., Van Stijn, T., et al. (2020). Genetic diversity in invasive populations of Argentine stem weevil associated with adaptation to biocontrol. Insects 11:441. doi: 10.3390/insects11070441
Heimpel, G. E., and Mills, N. J. (2017). Biological Control: Ecology and Application. Cambridge, MA: Cambridge University Press, 386. doi: 10.1017/9781139029117
Henter, H. J. (1995). The potential for coevolution in a host-parasitoid system. II. Genetic variation within a population of wasps in the ability to parasitize an aphid host. Evolution 49, 439–445. doi: 10.1111/j.1558-5646.1995.tb02276.x
Henter, H. J., and Via, S. (1995). The potential for coevolution in a host-parasitoid system. I. Genetic variation within an aphid population in susceptibility to a parasitic wasp. Evolution 49, 427–438. doi: 10.1111/j.1558-5646.1995.tb02275.x
Kraaijeveld, A. R., and Godfray, H. C. J. (1997). Trade-off between parasitoid resistance and larval competitive ability in Drosophila melanogaster. Nature 389, 278–280. doi: 10.1038/38483
Kraaijeveld, A. R., Van Alphen, J. J. M., and Godfray, H. C. J. (1998). The coevolution of host resistance and parasitoid virulence. Parasitology 116, S29–S45. doi: 10.1017/S0031182000084924
Lapchin, L. (2002). Host-parasitoid association and diffuse coevolution: When to be a generalist? Am. Nat. 160, 245–254. doi: 10.1086/341020
Lefèvre, T., De Roode, J. C., Kacsoh, B. Z., and Schlenke, T. A. (2012). Defence strategies against a parasitoid wasp in Drosophila: Fight or flight? Biol. Lett. 8, 230–233. doi: 10.1098/rsbl.2011.0725
Lynch, Z. R., Schlenke, T. A., and De Roode, J. C. (2016). Evolution of behavioural and cellular defences against parasitoid wasps in the Drosophila melanogaster subgroup. J. Evol. Biol. 29, 1016–1029. doi: 10.1111/jeb.12842
Mills, N. J. (2017). Rapid evolution of resistance to parasitism in biological control. Proc. Natl. Acad. Sci. U.S.A. 114, 3792–3794. doi: 10.1073/pnas.1702753114
NIWA (2019). The National Climate Database. Auckland, NZ: National Institute of Water and Atmospheric Research.
Pascoal, S., Cezard, T., Eik-Nes, A., Gharbi, K., Majewska, J., Payne, E., et al. (2014). Rapid convergent evolution in wild crickets. Curr. Biol. 24, 1369–1374. doi: 10.1016/j.cub.2014.04.053
Pascoal, S., Risse, J. E., Zhang, X., Blaxter, M., Cezard, T., Challis, R. J., et al. (2020). Field cricket genome reveals the footprint of recent, abrupt adaptation in the wild. Evol. Lett. 4, 19–33. doi: 10.1002/evl3.148
Pennisi, E. (2017). In a first, natural selection defeats a biocontrol insect. Science 356, 570–570. doi: 10.1126/science.356.6338.570
Phillips, C. B. (2002). Observations of oviposition behavior of Microctonus hyperodae Loan and M. aethiopoides Loan (Hymenoptera: Braconidae: Euphorinae). J. Hymenoptera Res. 11, 326–337.
Phillips, C. B., Baird, D. B., Iline, I. I., Mcneill, M. R., Proffitt, J. R., Goldson, S. L., et al. (2008). East meets west: adaptive evolution of an insect introduced for biological control. J. Appl. Ecol. 45, 948–956. doi: 10.1111/j.1365-2664.2008.01461.x
Phillips, C. B., Barker, G. M., Roberts, R. L., McNeill, M. R., and Goldson, S. L. (1996). “Fecundity of wild and laboratory reared ecotypes of Microctonus hyperodae Loan (Hymenoptera: Braconidae),” in Proceedings of the New Zealand Plant Protection Conference, Wellington. doi: 10.30843/nzpp.1996.49.11457
Phillips, C. B., and Kean, J. M. (2017). Response of parasitoid egg load to host dynamics and implications for egg load evolution. J. Evol. Biol. 30, 1313–1324. doi: 10.1111/jeb.13095
Popay, A. J., Mcneill, M. R., Goldson, S. L., and Ferguson, C. M. (2011). The current status of Argentine stem weevil (Listronotus bonariensis) as a pest in the North Island of New Zealand. New Zeal. Plant Prot. 64, 55–62. doi: 10.30843/nzpp.2011.64.5962
Sih, A., Maud, C. O., and Ferrari and Harris, D. J. (2011). Evolution and behavioural responses to human-induced rapid environmental change. Evol. Appl. 4, 367–387. doi: 10.1111/j.1752-4571.2010.00166.x
Sparks, T. C., and Nauen, R. (2015). IRAC: Mode of action classification and insecticide resistance management. Pest. Biochem. Physiol. 121, 122–128. doi: 10.1016/j.pestbp.2014.11.014
Tomasetto, F., Casanovas, P., Brandt, S. N., and Goldson, S. L. (2018a). Biological control success of a pasture pest: has its parasitoid lost its functional mojo? Front. Ecol. Evol. 6:215. doi: 10.3389/fevo.2018.00215
Tomasetto, F., Cianciullo, S., Reale, M., Attorre, F., Olaniyan, O., and Goldson, S. L. (2018b). Breakdown in classical biological control of Argentine stem weevil: a matter of time. BioControl 63, 521–531. doi: 10.1007/s10526-018-9878-4
Tomasetto, F., Tylianakis, J. M., Reale, M., Wratten, S., and Goldson, S. L. (2017). Intensified agriculture favors evolved resistance to biological control. Proc. Natl. Acad. Sci. U.S.A. 114, 3885–3890. doi: 10.1073/pnas.1618416114
Tylianakis, J. M., and Romo, C. M. (2010). Natural enemy diversity and biological control: Making sense of the context-dependency. Basic Appl. Ecol. 11, 657–668. doi: 10.1016/j.baae.2010.08.005
Venables, W. N., and Ripley, B. D. (2002). Modern Applied Statistics with S. New York, NY: Springer. doi: 10.1007/978-0-387-21706-2
Keywords: Argentine stem weevil, avoidance behaviour, biological control, contemporary evolution, Listronotus, Microctonus, parasitoid, resistance
Citation: Shields MW, Wratten SD, Van Koten C, Phillips CB, Gerard PJ and Goldson SL (2022) Behaviour drives contemporary evolution in a failing insect-parasitoid importation biological control programme. Front. Ecol. Evol. 10:923248. doi: 10.3389/fevo.2022.923248
Received: 19 April 2022; Accepted: 30 June 2022;
Published: 18 August 2022.
Edited by:
Alberto Pozzebon, University of Padua, ItalyReviewed by:
Kim Hoelmer, Beneficial Insects Introduction Research Unit, Agricultural Research Service (USDA), United StatesCopyright © 2022 Shields, Wratten, Van Koten, Phillips, Gerard and Goldson. This is an open-access article distributed under the terms of the Creative Commons Attribution License (CC BY). The use, distribution or reproduction in other forums is permitted, provided the original author(s) and the copyright owner(s) are credited and that the original publication in this journal is cited, in accordance with accepted academic practice. No use, distribution or reproduction is permitted which does not comply with these terms.
*Correspondence: Stephen L. Goldson, c3RlcGhlbi5nb2xkc29uQGFncmVzZWFyY2guY28ubno=
†Deceased
‡These authors have contributed equally to this work
Disclaimer: All claims expressed in this article are solely those of the authors and do not necessarily represent those of their affiliated organizations, or those of the publisher, the editors and the reviewers. Any product that may be evaluated in this article or claim that may be made by its manufacturer is not guaranteed or endorsed by the publisher.
Research integrity at Frontiers
Learn more about the work of our research integrity team to safeguard the quality of each article we publish.