- 1CEFE, Univ. Montpellier, CNRS, EPHE, IRD, Montpellier, France
- 2Norwegian Polar Institute, FRAM Centre, Tromsø, Norway
- 3CEFE, Univ. Montpellier, CNRS, EPHE-PSL University, IRD, Montpellier, France
Reproductive senescence is ubiquitous in mammals. However, patterns of senescence vary across reproductive traits, even within populations, perhaps because of differences in selection pressures, physiological constraints, and responses to environmental conditions. We investigated reproductive senescence in wild female polar bears (Ursus maritimus), using 31 years of capture-recapture data from the Svalbard area. We studied the influence of environmental conditions on age-specific litter production and litter size using generalized linear mixed models. Further, using a capture-recapture model that handles the dependency between vital rates of individuals belonging to the same family unit, we assessed maternal-age-related changes in first year cub and litter survival. We provide clear evidence for reproductive senescence in female polar bears. Litter production and litter size peaked in middle-aged females and declined sharply afterward. By contrast cub and litter survival did not decline after prime age. We found no evidence of terminal investment. The reproductive output of all females was affected by sea-ice conditions during the previous year and the Arctic Oscillation, with some effects differing greatly between age groups. Old females were affected the most by environmental conditions. Our results suggest that the decline in reproductive output is a combination of fertility and body-condition senescence, with a weak contribution of maternal-effect senescence, possibly due to benefits of experience. Further, as predicted by evolutionary theory, senescence appears to be a consequence of failures in early stages of the reproductive cycle rather than in late stages, and environmental variation affected old females more than prime-aged females. Our study emphasizes the need to study several reproductive traits and account for environmental variation when investigating reproductive senescence. Differences in senescence patterns across reproductive traits should be interpreted in light of evolutionary theory and while considering underlying physiological drivers.
Introduction
Reproductive senescence, the decline in reproductive success with increasing age, is nearly ubiquitous in wild populations of vertebrates (Lemaître and Gaillard, 2017; Lemaître et al., 2020). However, the age at onset and the rate of senescence vary considerably across species (Jones et al., 2014; Lemaître et al., 2020) and populations (Lemaître and Gaillard, 2017). Furthermore, various indicators of reproductive performance, such as conception rate, fecundity, litter size and offspring survival often display contrasted and asynchronous patterns of senescence within populations (Nussey et al., 2009; Massot et al., 2011; Hayward et al., 2013, 2015; Berger et al., 2015). These reproductive traits are likely governed by distinct physiological mechanisms and may be under different selection pressures. Therefore, they may vary differently with age and in response to environmental conditions, potentially explaining the heterogenous patterns of senescence observed across traits and/or populations within the same species. Considering reproductive senescence as the result of senescence of several processes which vary in their physiological drivers and in the timing at which they intervene in the reproductive cycle, while taking into account the influence of environmental conditions, may help gain a better understanding of senescence patterns.
First, reproductive senescence can be decomposed into fertility senescence and maternal-effect senescence (Moorad and Nussey, 2016; Karniski et al., 2018). Fertility senescence corresponds to the aging of the reproductive physiology resulting in lower fertility. A major mechanism underpinning fertility senescence is the progressive depletion of the finite pool of primary oocytes in mammals and birds, resulting in lower pregnancy rates (Lemaître and Gaillard, 2017). Maternal-effect senescence, on the other hand, corresponds to a declining in the ability to provide for offspring (both pre- and postnatally) with increasing age, resulting in lower reproductive success in late-life. Maternal-effect senescence is caused by somatic senescence impairing functions (e.g., resource acquisition, lactation, immunity) that have an influence on offspring traits such as body mass and viability (Karniski et al., 2018). For instance, reduced predatory performance at old age as documented in wolves (MacNulty et al., 2009) may lead to lower energy intake which may hinder lactation, resulting in low body mass of offspring born to old females. In capital breeders, senescence in body condition, a consequence of somatic senescence, may be an additional cause of reproductive decline as it may prevent old females from undertaking reproduction due to insufficient energy stores (Derocher et al., 1992; Nussey et al., 2011).
Second, reproduction can be considered as a sequence of stages, any of which may fail in a given breeding attempt, with reproductive senescence increasing the overall chances of failure. Late stages of the reproductive cycle (potentially mediating maternal-effect senescence, e.g., lactation) contribute more to the fitness cost of overall reproduction (that is, reduced survival and future reproduction) than do earlier, typically less energy demanding, stages (potentially mediating fertility or maternal-effect senescence, e.g., pregnancy) (Clutton-Brock et al., 1989). Losing offspring in early stages of the reproductive cycle should therefore be less costly than losing offspring in late stages. In addition, in species providing parental care over several breeding season, losing offspring tardively entails lost breeding opportunities. Therefore, senescence of traits impacting late stages of the reproductive cycle should be counter-selected more strongly than traits impacting earlier stages of the reproductive cycle (Lemaître and Gaillard, 2017) [but see Nussey et al. (2009)].
The way and extent to which environmental conditions interact with reproductive senescence–through either of its components or any of its stages, also remains poorly understood (Lemaître and Gaillard, 2017; Gaillard and Lemaître, 2020). Early life is the most critical period during which the available resources must be partitioned between somatic maintenance, growth, and first reproductive events. Good environmental conditions during early life (e.g., high resource availability) can have positive impacts later in life, including at old age (Cooper and Kruuk, 2018). Such “silver-spoon” effects have been shown in mammals, e.g., red deer born during a year with high intra-specific competition exhibited higher rates of reproductive senescence (Nussey et al., 2007). Less is known about the effect of environmental conditions faced during adult life (Lemaître and Gaillard, 2017). Based on resource acquisition and allocation theory, age-related differences in reproductive performance are expected to be minimal under favorable environmental conditions and maximum under adverse environmental conditions. These predictions have mostly been tested in seabirds (Ratcliffe et al., 1998; Bunce et al., 2005; Nevoux et al., 2007; Vieyra et al., 2009; Pardo et al., 2013; Oro et al., 2014), showing contrasted results. In Australasian gannets (Bunce et al., 2005) and black-browed albatrosses (Pardo et al., 2013) results were consistent with theoretical predictions. By contrast, differences in breeding performance were highest during years of high food availability in Audouin’s gulls (Oro et al., 2014), and only detected in years of intermediate environmental conditions in great skuas (Ratcliffe et al., 1998). Heterogeneity among individuals unaccounted for may explain these differences (Nussey et al., 2008; Gimenez et al., 2018). In mammals, one study on lemurs showed that in years of low rainfall during the lactation period, old females had reduced offspring survival but not younger females, because of tooth wear (King et al., 2005). Similarly, weaning success of young and old female chamois was reduced in poor years while that of prime-aged females remained unchanged (Morin et al., 2016). These findings are in line with theoretical predictions. To our knowledge, the interactive effect of maternal age and environmental variation on reproductive output has not been studied in other mammal species.
Here we investigate age-related patterns of four indicators of reproductive success (litter production, litter size at capture, and cub and litter survival during the first year) in interaction with environmental conditions in female polar bears (Ursus maritimus) in Svalbard, Norwegian Arctic, using 31 years (1992–2019, 2021–2022) of capture-recapture (CR) data. Our aim is twofold. (i) We analyze and contrast age-related changes in several indicators of reproductive success to get insight into the contribution of fertility senescence and maternal senescence to overall reproductive senescence. Cub and litter survival fall within the scope of maternal-effect senescence, whereas litter production and litter size may reflect body-condition, fertility and maternal effect senescence (Derocher et al., 1992). (ii) Evaluate the age-specific impacts of environmental variation on our first two indicators of reproductive success to understand the role of environmental conditions in shaping senescence patterns in the wild. Polar bears live in a challenging habitat, where availability of food resources varies greatly seasonally and annually, due to the dynamic nature of sea-ice habitat. These challenges have been aggravated by climate warming, which has progressively led to earlier sea-ice break-up in spring, later freeze-up in autumn, lower extent, and altered sea-ice characteristics. These changes in sea-ice reduce foraging opportunities for polar bears in several areas (Stirling and Derocher, 2012 and Derocher, 2012), with cascading effects on body condition and reproduction (Obbard et al., 2006, 2016; Stirling and Derocher, 2012). Additionally, contrary to other well-studied polar bear populations, Svalbard polar bears are not hunted, following a ban in the 1970’. This context provides us with an ideal setting to investigate how age and a broad range of environmental conditions influence reproductive output.
Female polar bears are capital breeders for the first part of their reproductive cycle. They must acquire extensive fat reserves before entering their den, where they give birth and nurse their young while fasting (Atkinson and Ramsay, 1995). Given the high cost of reproduction, we expect body-condition senescence and maternal-effect senescence to play a major role in polar bears, with strong declines in litter production, litter size, and cub and litter survival in late life. Alternatively, since late stages of the reproductive cycle should be under stronger selection than early stages (Lemaître and Gaillard, 2017), we may observe that cub and litter survival display slower senescence than litter production and litter size. Regarding the effect of environmental conditions, we predict an influence of sea-ice dynamics and the Artic Oscillation index in the year prior to capture, during which pregnant females acquire fat reserves for maternity denning, on reproductive performance. We expect lower reproductive performance for all females following years with a lack of sea-ice, although this effect could be amplified for certain age groups (Gaillard and Yoccoz, 2003). Young females and senescent females could be affected the most (e.g., Morin et al., 2016). Alternatively, additional experience acquired with age may ease the effect of environmental conditions on old females through better foraging ability [e.g., better knowledge of resource distribution, higher hunting success (Daunt et al., 2007; Nisbet et al., 2020)] or higher quality of maternal care (Weladji et al., 2006; Limmer and Becker, 2009).
Overall, our long-term data provides us with an opportunity to study senescence patterns in several indices of reproductive success in a long-lived capital breeder species, under a wide range of environmental conditions.
Materials and methods
Study species and data
Biology of polar bears
The polar bear is a long-lived species, with a maximum life span slightly above 30 in the wild (Weigl, 2005). Polar bears mate in spring, but females delay implantation until autumn (Lønø, 1970; Ramsay and Stirling, 1988). If females’ energy stores are too low at this time, they may abort (Atkinson and Ramsay, 1995). Otherwise, they enter a den where they give birth between November and January, most often to two cubs, occasionally to one, or rarely to triplets (Stirling, 2011). Cubs are very small at birth, weighing around 600 g. In the Barents Sea area, females emerge from the den in March–April when their cubs are 3–4 months old (Wiig, 1998). Between den entry and den emergence, females fast, loosing on average 40% of their body mass, which means they must have extensive fat stores at den entry to sustain lactation and maintenance (Atkinson and Ramsay, 1995). For a few days or occasionally weeks after den emergence, families remain close to their den (Hansson and Thomassen, 1983). During their first year, cubs have a high mortality rate (Amstrup and Durner, 1995; Derocher and Stirling, 1996; Cubaynes et al., 2021) and depend entirely on their mother. Singleton litters may result from pre-natal or neonatal mortality occurring in what was initially a twin (or more rarely a triplet) litter. Cubs remain with their mother, depending on her for food and protection, until age 2–2.5, with most departures occurring in late winter and spring (Amstrup, 2003). In Svalbard, most females have their first litter at age 6, although some females can have their first litter at age 5 (Derocher, 2005). Polar bears depend strongly on sea-ice for hunting seals, their main prey (Stirling and Archibald, 1977), for traveling, and for access to denning areas (Derocher et al., 2011). Contrary to other well-studied populations, Svalbard polar bears are not subjected to indigenous subsistence harvest or sport hunting. Since the ban on polar bear hunting in 1973, an average of 2.9 bears are killed by humans each year, mainly because they pose a danger to life or property.1
Longitudinal data on polar bears
We live-captured and marked polar bears around Svalbard (Norway, Figure 1) in 1992–2019 and 2021–2022 using methods described in Stirling et al. (1989). Captures occurred between late March and early May when most females with cubs-of-the-year (hereafter “cubs”), have emerged from maternity dens (Wiig, 1998). Age of cubs, yearlings and 2-year-olds was determined with certainty based on size. Age of bears captured for the first time as sub-adults or adults was estimated using an extracted vestigial premolar tooth (Calvert and Ramsay, 1998; Christensen-Dalsgaard et al., 2010). Mother’s straight body length (cm) (hereafter “size”), was measured as the straight-line distance between the tip of the nose and the caudal end of the tail bone of bears laying in sternal recumbency (Derocher, 2005). For mothers with cubs, litter size was recorded at capture. Females captured alone were considered to have no dependent offspring alive. This could be so if (i) they did not mate, (ii) they mated but did not get pregnant or aborted, (iii) they lost their cub, yearling, or 2-year-old offspring prior to capture, or (iv) they parted from their 2-year-old offspring prior to capture. Apart from (iv), these situations all correspond to a reproductive failure.
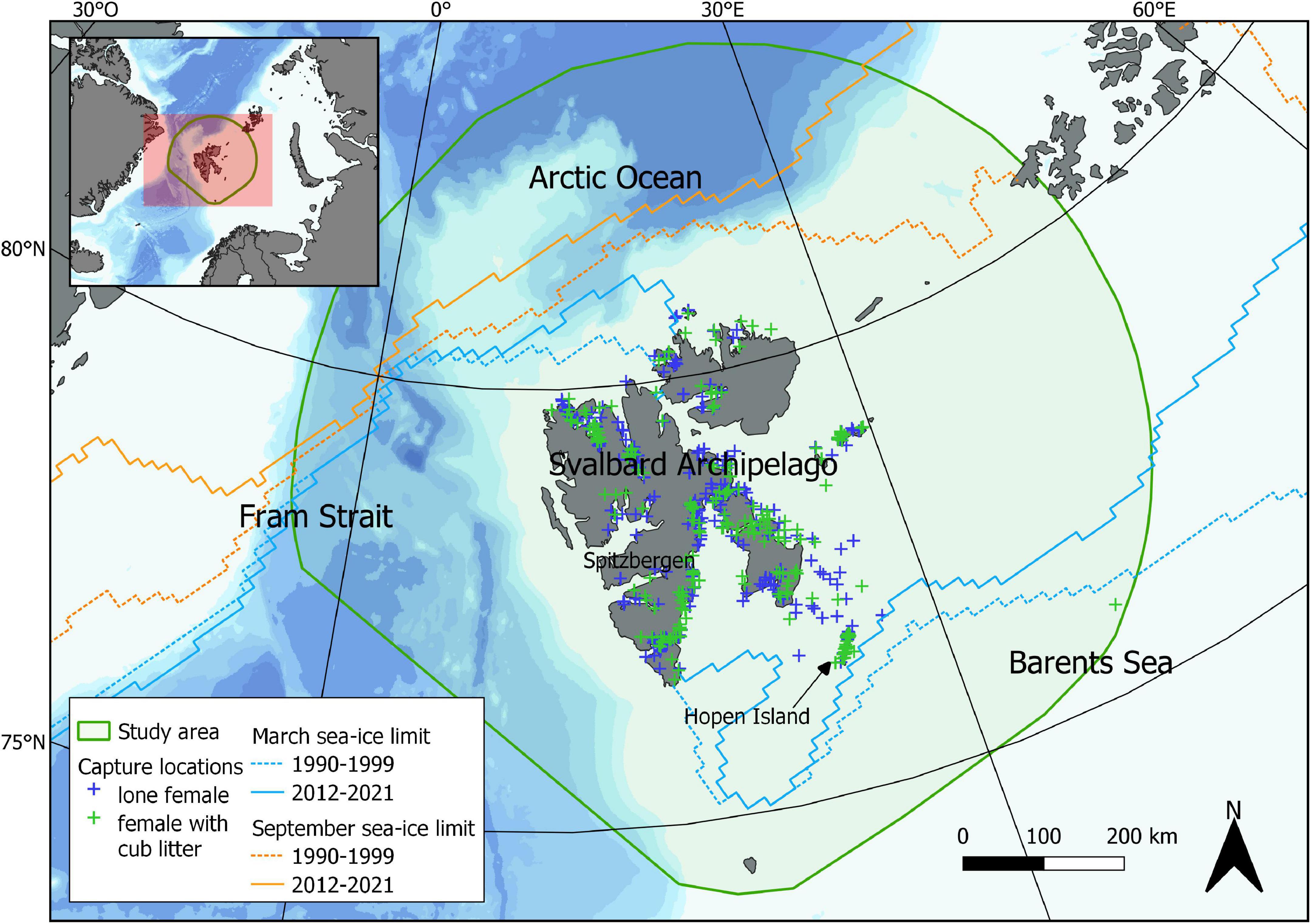
Figure 1. Capture locations of adult female polar bears in the Svalbard area from 1992 to 2019 and 2021 to 2022. Captures of lone females from 1992 to 1999 were excluded from our analysis and are not represented. The lines represent the mean maximum (March) and minimum (September) sea-ice extents for the periods 1990–1999 and 2012–2021. The study area corresponds to the 95% minimum convex polygon of telemetry locations of 135 adult females recorded between 1989 and 2021.
In the 1990s, most of the sampling was conducted using a base station located on Hopen Island (in the south-east of the Svalbard archipelago, Figure 1), an important denning area at that time (Derocher et al., 2011). Many females with cubs of the year were thus captured close to the base station just after they had left their maternity den. From 2000 onward, a much larger area of Svalbard was covered, using as a base station either a boat with a helicopter deck or a cabin located in Spitsbergen. We thus think that the probability of capturing females with cubs was higher in 1992–1999 than from 2000 onward. Consequently, in the analysis including both lone females and females with cubs, we only considered captures that occurred in year 2000 or later.
Environmental conditions
Sea-ice
We downloaded daily sea-ice concentration grids made available by the university of Hamburg for year 1992–2019 (Kern et al., 2020). These grids are derived from radiance temperature data measured remotely using the 85 GHz Special Sensor Microwave/Imagers (SSM/Is) and Special Sensor Microwave Imager/sounder (SSMIS) channels and processed using the ARTIST Sea Ice algorithm (Kern et al., 2020). The data has a resolution of 12.5 × 12.5 km. Nonetheless, as the Hamburg University timeseries does not extend further back than December 1991, we retrieved daily sea-ice concentration grids (with a 25 × 25 km gird cell size) for 1990 and 1991 from the National Snow and Ice Data Center (Cavalieri et al., 1996).
To define the study area, over which sea-ice covariates were calculated, we used telemetry data from 135 adult female polar bears captured between 1989 through 2021 and fitted with a satellite-linked collar. Polar bear locations were obtained using Argos (CLS, Toulouse, France) or Global Positioning System (GPS) systems. Location estimates became more accurate over the years with a gradual transition from using mainly Argos location systems to GPS with the first GPS deployments occurring in 2000. To account for the larger spatial errors of the Argos system, all Argos locations were processed through a speed, distance, and angle filter (Freitas et al., 2008) which removes all positions deemed unrealistic given a maximum speed and a maximum deviation from the track. In addition, the sampling regime varied between the tags from one position every 2 h to one position every 6 day. Hence, we retained one GPS position every 6th day to match the early Argos sampling regime and not introduce a bias. The tracking duration was at least 1 year. The study area was then defined as the 95% minimum convex polygon (MCP) (Mohr, 1947). This method was selected because it is simple, non-parametric and creates the unique smallest polygon encompassing a preset proportion of locations (here 95%, Figure 1).
Following (Cherry et al., 2013; Molnár et al., 2020), we considered a grid cell to be ice-covered if sea-ice concentration >30%. We then generated a 1990–2021 time series at daily resolution of the surface covered by sea-ice (hereafter “sea-ice extent”) by multiplying the number of ice-covered grid cells in our study area by the appropriate area of an individual grid cell (252 and 12.52 km2 for years 1990–1991 and 1992–2021, respectively). We adopted an extent-based rather than concentration-based approach following the recommendations of Molnár et al. (2020). Missing and aberrant values were interpolated using the closest available previous and following days. We defined the transition sea-ice extent as the extent halfway between the 30-day minimum (September) and maximum (March) extent over 1990–1999. For each year, the date of sea-ice break-up (respectively of freeze-up) was defined as the date when sea-ice extent falls below (respectively rises above) the transition sea-ice extent for ≥5 consecutive days. Considering that cells were ice-covered if sea-ice concentration >15% (instead of >30%) as done in Galicia et al. (2020) and Rode et al. (2021) yielded very similar dates of sea-ice break-up (mean difference −0.2 ± 0.04 days, Kendall’s τ = 0.98) and freeze-up (mean difference −0.6 ± 0.2 days, Kendall’s τ = 0.96).
We expected early break-up and late freeze-up to reduce foraging opportunities and fat storage before denning, as well as access to denning areas, thereby negatively affecting reproductive performance in the following year (Derocher et al., 2011; Molnár et al., 2011; Stirling and Derocher, 2012). We thus considered the date of sea-ice break-up (DateBreakUp) and freeze-up (DateFreezeUp) in the year prior to capture as covariates.
Arctic oscillation
The Arctic oscillation (AO) is a large-scale climate index calculated based on the sea-level pressure anomalies north of 20°N. The AO determines the wind regimes around the Arctic and thereby influences sea-ice conditions. When the AO is low, more sea-ice is trapped in the middle of the Arctic by the winds resulting in greater area of multilayer sea-ice (Rigor et al., 2002). When the AO is high, more sea-ice drifts out of the arctic through Fram Strait (Rigor et al., 2002). Nonetheless, the effect of the AO does not appear to be captured by satellite-derived sea-ice metrics, likely because it impacts characteristics of sea-ice other than extent (e.g., thickness, presence of rafter ice, presence of leads) that may affect polar and their prey (Derocher, 2005; Ferguson et al., 2005; Pilfold et al., 2015; Rode et al., 2018b,2021).
We retrieved a monthly arctic oscillation time series from the NOAA website.2 We define winter as the period January–March and spring April–June. We calculated the winter and spring AO by averaging the monthly AO index over the corresponding period for each year in the study period.
We expected a high AO index in the winter and the spring of the year preceding capture (PriorWinterAO, PriorSpringAO), and in the winter of the year of capture (WinterAO), to be associated with lower reproductive performance.
Statistical analyses
We performed all analyses in R version 4.1.2 (R Core Team, 2021) and Rstudio (RStudio Team, 2021). All the figures were made using the ggplot2 (Wickham et al., 2020) and patchwork (Pedersen, 2020) packages.
Analysis of litter production and litter size
Modeling approach
First, we used a binomial Generalized Linear Mixed Model (GLMM) to examine the-effect of individual and environmental covariates on the probability of litter production (i.e., of being with at least one cub at capture) in interaction with age. The covariates were size (Size, with a quadratic effect Size2), DayBreakup, DayFreezeUp, WinterAO, PriorWinterAO, and PriorSpringAO. We also controlled for the date of capture (DateCapture). We included data on n1 = 441 captures of females that were alone or with ≥1 cub in 2000–2019 and 2021–2022, corresponding to 289 distinct females. Females with yearlings or 2-year-olds were not included in this analysis. We did not take into account the reproductive status of females in the years prior to capture, meaning we considered that females who were alone in spring t-1 and those who had a litter but lost it have the same probability of producing a litter. Including only females for which past reproductive history was known would have greatly reduced sample size (n = 68 instead of 441). Second, we built another binomial GLMM to investigate the effect of the same individual and environmental covariates on the probability of having a twin or triplet litter, given a litter. In this analysis, we included data on n2 = 251 captures of females with ≥1 cub in 1992–2019 and 2021–2022 corresponding to 204 distinct females. One female was captured once outside of the study area and was excluded from this analysis. We grouped twin and triplet litters because only 3% of females in our dataset were captured with triplets. Hereafter, we refer to both twin and triplet litters as “large litters.” We accounted for environmental variation not captured by our covariates using a yearly random effect. We did not include individual identity as a random effect because of the low recapture rate (25% of females captured more than once, mean number of captures per female 1.53 ± 1.16 for the analysis of litter production; 17% and 1.22 ± 0.57 for the analysis of litter size).
We included the effect of the date of capture for three reasons. (i) To account for the departure of 2-year-old from their mother over the fieldwork season that spanned more than a month, which results in an overestimation of reproductive failures if not accounted for in our analysis of litter production (Cubaynes et al., 2021), (ii) to account for den emergence throughout the field season in our analysis of litter production (Wiig, 1998), and (iii) to account for potential offspring loss since cub mortality rate is high (Wiig, 1998; Folio et al., 2019).
We tested for potential dependences between environmental covariates. As our covariates were not normally distributed, we used Kendall rank correlation tests. DateBreakUp and DateFreezeUp were correlated with one another with τ = 0.4. Consequently, we did not include them in the same model. All other covariates included were correlated with τ < 0.4.
In both models, we grouped females into age groups because (i) we did not want to impose the shape of the relationship between age and reproductive output and therefore did not consider age as a continuous variable using for example a quadratic function; (ii) we did not want to use non-linear models [e.g., generalized additive model (GAM)] as this would have made exploring the interaction between environmental covariates and age, and interpreting the results in terms of senescence patterns difficult (iii) sample size was insufficient to consider one category per age (as done in Hayward et al., 2013 for instance). In order to determine the appropriate number of age groups and cut-offs between them, we (1) ran GAMs while controlling for the date of capture and (2) since we were particularly interested in senescence, we ran a segmented regression with two segments to estimate the age at onset of senescence (see Appendix 1, Supplementary Figures 1–4). Results from these preliminary analyses yielded the following age categories: 5–9, 10–15, 16–20, and ≥21 years for the analysis of litter production and 5–9, 10–15, and ≥16 years for the analysis of litter size. We grouped females aged 16–20 and ≥21 years in the analysis of litter size because of the low number of females ≥21 years (n = 11, Supplementary Figure 5). We estimated age-group-specific effects of all covariates.
Implementation
We fitted all models in a Bayesian framework using the nimble package (de Valpine et al., 2021). Both GLMMs were ran simultaneously as parts of a single nimble model. All continuous covariates were standardized. We used non-informative normal prior distributions for the regression coefficients and a uniform prior distribution for the standard deviation of the random effect. We ran two MCMC in parallel with different initial values, 135,000 iterations and a burn-in of 10,000 iterations. We kept one out of 10 values from each chain. We used the Gelman and Rubin R-hat diagnostic [R-hat < 1.1, (Gelman and Rubin, 1992)] and visual inspection to assess convergence.
Variable selection procedure
We considered age-specific covariates to be significant if the 89% credible interval (CRI) of their posterior distribution did not overlap with 0. We used 89% CRI rather than 95% CRI following recent recommendations (Kruschke, 2015; McElreath, 2020). Only age-specific covariates that were significant were retained in the final model. Because our dataset was already divided in three or four categories according to female’s age with a limited sample size in each category, we did not investigate additional interactions.
Calculation of additional quantities
Based on the posterior distributions of parameters obtained from the best models for litter production and litter size, we calculated the absolute probability of having a singleton litter and of having a large litter. It was simply obtained as the product of the probability of litter production and the probability of a large litter. This was possible for females aged ≥16 because the two age categories 16–20 and ≥21 years used in the analysis of litter production are nested within the age category ≥16 years used in the analysis of litter size (Appendix 1).
To get insight into the age-related changes in susceptibility of litter production and litter size to environmental conditions, we calculated the age-specific mean effect size of environmental covariates. To do so, we averaged, for each age class, the values from the posterior distributions of all environmental variables, iteration by iteration.
Analysis of cub and litter survival
Capture-recapture model
We estimated individual cub and litter survival in singleton and large litters using CR data collected between 1992 and 2019. We assessed cub and litter survival between the age of 3–4 months and 15–16 months (i.e., between the cubs’ first spring and the following one) depending on maternal age. Due to the limited sample size, we could not determine the optimal number of age groups and cut-offs. We therefore used the same age groups as in the analysis of litter size (5–9, 10–15, and ≥16 years) as they require fewer old individuals than those used in the analysis of litter production. To perform this analysis, we adapted a CR model which accounts for the multiple-year dependency between the demographic parameters of individuals belonging to the same family unit as well as the influence of past-reproductive history on female survival and reproductive performance (see Cubaynes et al., 2021).
Model assumptions
Polar bears captured in Svalbard are a mixture of resident bears, who stay in the Svalbard coastal area, and pelagic bears who follow the marginal-ice-zone as it retreats and advances (Mauritzen et al., 2001). Pelagic bears have a low recapture probability because they mostly remain outside of our study area, as indicated by extensive telemetry records (Norwegian Polar Institute, unpublished data). As recommended by Cubaynes et al. (2021) to avoid underestimating the survival rates of all bears, we only considered resident individuals captured at least twice between 1992 and 2019 in the analysis of cub and litter survival.
Because of identifiability issues due to the relatively low sample size, we could not estimate the probability of litter production for each age class using the CR model. For the same reason, we did not investigate the effect of environmental covariates on cub and litter survival.
Implementation
We fitted the model in a Bayesian framework using the R2Jags package (Su and Yajima, 2020). We ran two MCMC chains in parallel with different initial values, over 20,000 iterations discarding the first 9,000 iterations. We kept one out of 5 values from each chain. We used the R-hat diagnostic and visual inspection to assess convergence.
Results
Litter production and litter size
Our dataset for the analysis of litter production included 441 captures (of 289 females), among which 167 (38%) were females with cubs. Of those 441 captures, 170 (38%) were females aged 5–9 (mean age ± SD: 6.96 ± 1.42 years), 172 (39%) were females aged 10–15 ( = 12.3 ± 1.70 years), 63 (14%) were females aged 16–20 ( = 17.8 ± 1.44 years), and 36 (8%) were females aged ≥21 ( = 23.4 ± 2.38 years). Our dataset for the analysis of litter size consisted in 251 captures (of 201 females), 167 (66%) of which were females with a large litter. Among these 251 captures of mothers, 99 (39%) were females aged 5–9 ( = 7.25 ± 1.18 years), 107 (43%) were females aged 10–15 ( = 12.4 ± 1.75 years), and 45 (18%) were females aged ≥16 ( = 19 ± 2.23 years). None of the 10 females aged 25 or more were accompanied with cubs at capture. The oldest female in our dataset was 29 years old. A more detailed overview of the dataset is provided in Appendix 2 (Supplementary Table 1; Supplementary Figure 5).
Below we present the results from the models that included the date of sea-ice break-up in the year prior to capture (DateBreakUp) as the sea-ice covariate. A full account of the variables included in the final models is provided in Supplementary Table 2. The models that included the date of sea-ice freeze-up in the year prior to capture (DateFreezeUp) produced similar results that we report in Appendix 3.
Effect of age
The probability of producing a litter varied with female age (Figure 2A). It increased 1.8 folds from age 5–9 to age 15–10. Then it decreased slightly at age 16–20 before declining more sharply after age 20, with females aged ≥21 having less than half as many chances of producing a litter as females aged 10–15 (Figure 3). The probability of having a large litter, given a litter, also varied with age, with the same pattern as litter production although the difference between age groups was lower (Figure 2B). Females aged 10–15 had 1.2 and 1.4 times as many chances of having a large litter, given a litter, compared to females aged 5–9 and ≥16, respectively (Supplementary Figure 6). The absolute probability of having a large litter (i.e., the product of the probability of producing a litter and the conditional probability of having a large litter) peaked at age 10–15 (Figure 3). Females aged 16–20 had a slightly lower probability of producing a litter than females aged 10–15, but almost half of their litters were singleton litters.
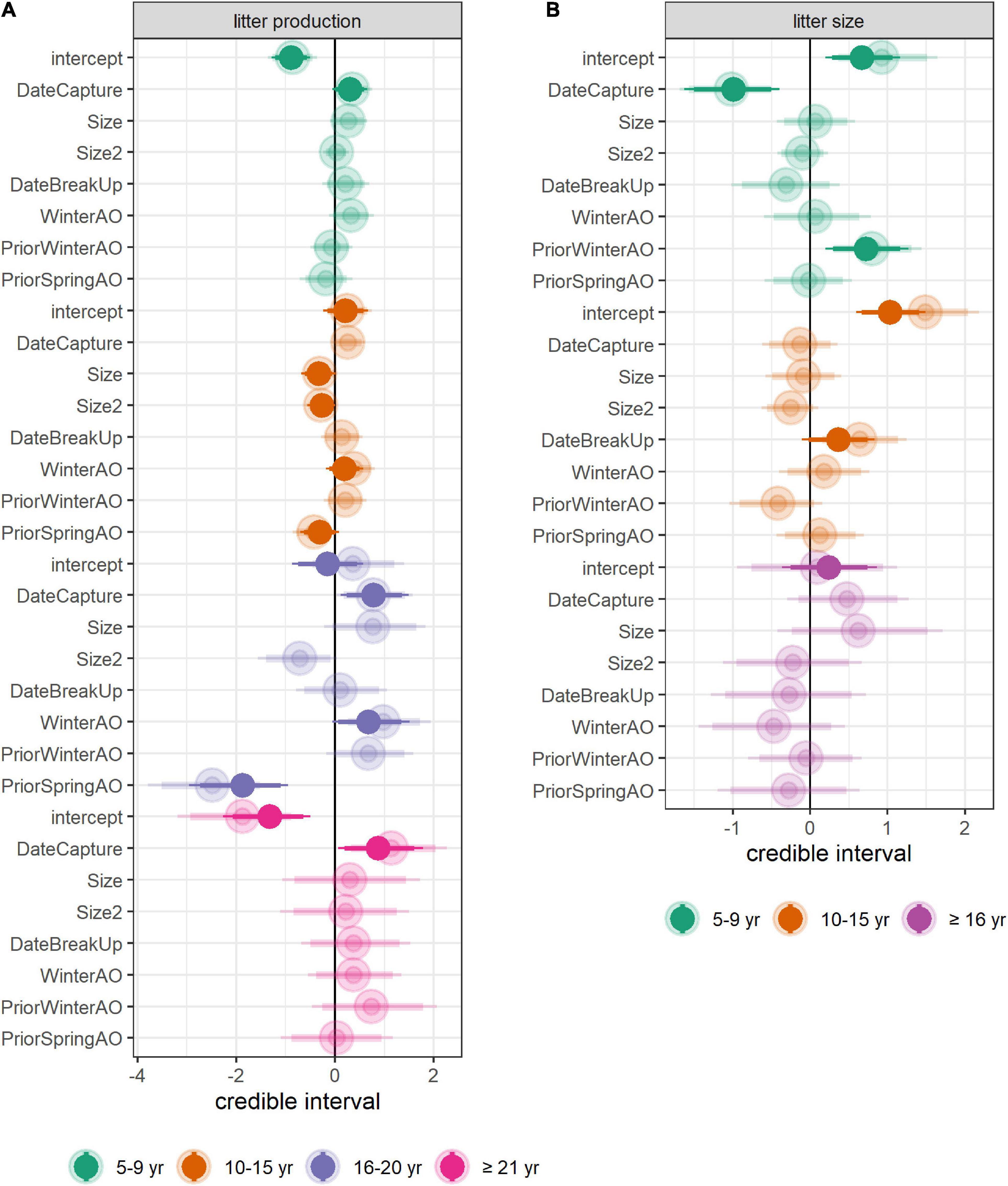
Figure 2. Output of the models of litter production and litter size. Posterior distributions of the coefficients in the full model (transparent colors) and in the final model (solid colors) of (A) litter production and (B) litter size. The mean (dot), 89% CRI (thick line), and 95% CRI (thin line) are provided. Results are on the logit scale. DateBreakUp refers to the date of sea-ice break-up in the year prior to capture (see Appendix 3, Supplementary Figure 8 for the version of this figure obtained when using DateFreezeUp as the sea-ice covariate), WinterAO, PriorWinterAO, and PriorSpringAO, respectively refer to the mean Arctic Oscillation index over the winter of the year of capture, over the winter of the year preceding capture and over the spring of the year preceding capture.
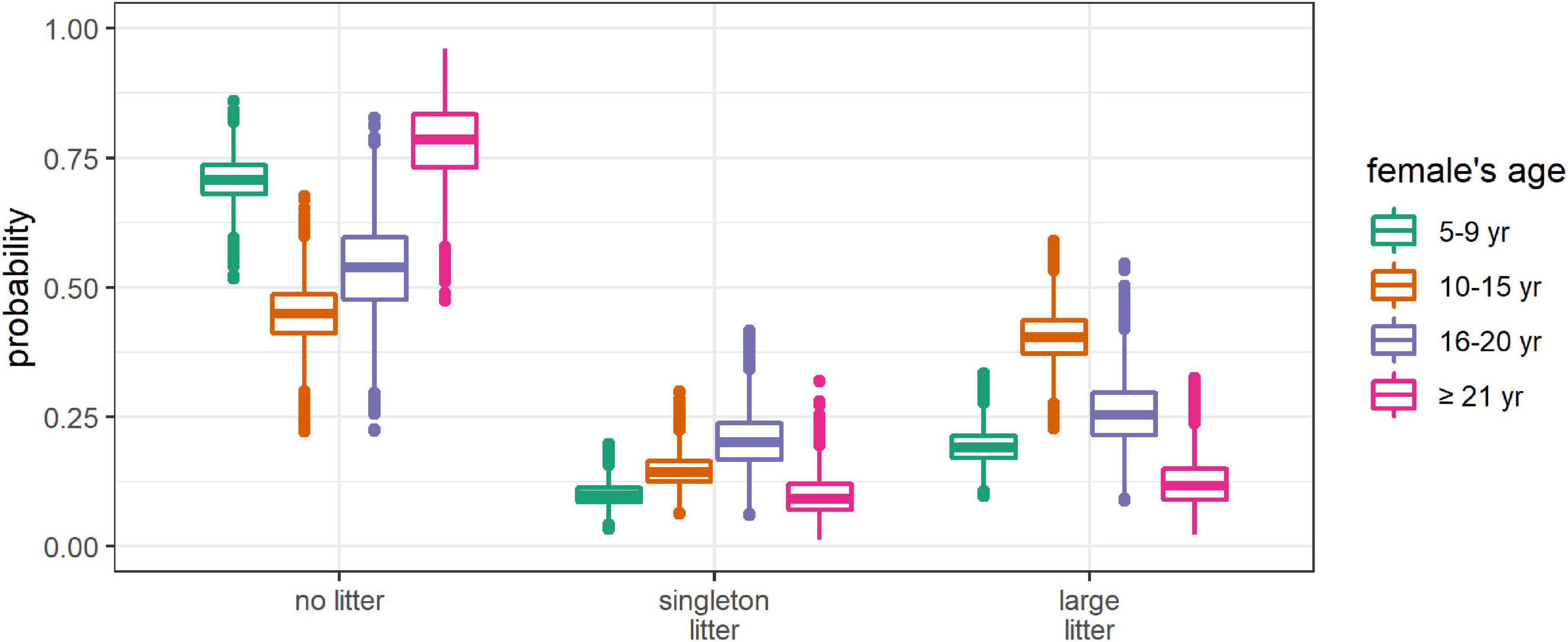
Figure 3. Posterior distributions of the absolute probabilities of having no litter, a singleton litter, or a large litter, depending on female’s age. All the other covariates were set at their mean value (DateCapture = 104, i.e., April 14, Size = 195 cm, DateBreakUp = 174, i.e., June 23, WinterAO = –0.06, PriorWinterAO = 0.01, PriorSpringAO = 0.12).
Effect of the date of capture
The date of capture influenced both the probability of litter production and the probability of having a large litter (Figure 2). The probability of litter production increased over the field season for all females except females aged 10–15 (Figure 4). However, for females aged 5–9 (but not for older females), the probability of a large litter decreased over the field season (Supplementary Figure 7). Consequently, the absolute probability of having a large litter had a parabolic shape for females aged 5–9, peaking at mid-season (Figure 4). By contrast, for females aged 16–20 and ≥21, the absolute probability of having a large litter increased monotonously throughout the season.
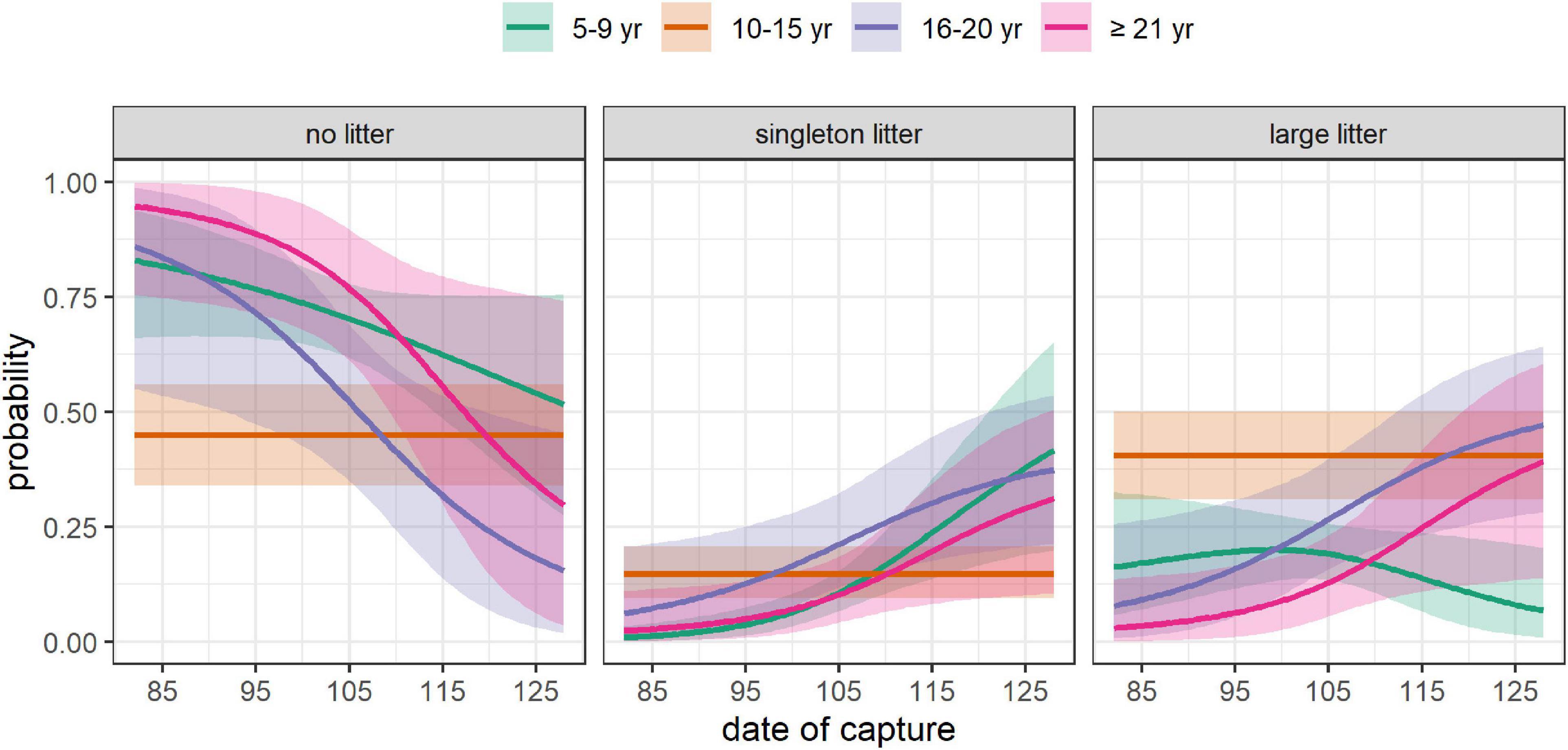
Figure 4. Effect of date of capture on the absolute probability of having no litter, a singleton litter, or a large litter, depending on female’s age. The mean (solid line) and 95% CRI (shading) are provided. All the covariates other than DateCapture were set at their mean value (Size = 195 cm, DateBreakUp = 174, i.e., June 23, WinterAO = –0.06, PriorWinterAO = 0.01, PriorSpringAO = 0.12).
Effect of environmental conditions
Litter production
A high winter AO index in year t was associated with a higher probability of litter production for females aged 10–15 and 16–20 (Figure 2A). A high spring AO index in year t-1 was also associated with a lower probability of litter production for females aged 10–15 and 16–20. The effect was particularly strong for the latter age group (Figure 2A). Dates of sea-ice break-up and freeze-up in year t-1 had no significant effect on the probability of litter production although there was a trend for a positive effect of the date of break-up, and a negative effect of the date of freeze-up for all aged age groups (Figure 2A; Supplementary Figure 8A). Overall, the mean effect size of environmental covariates (including non-significant ones) was comparable for females aged 5–9 and 10–15 but was higher for older females, particularly females aged 16–20 (Figure 5A; Supplementary Figure 9A).
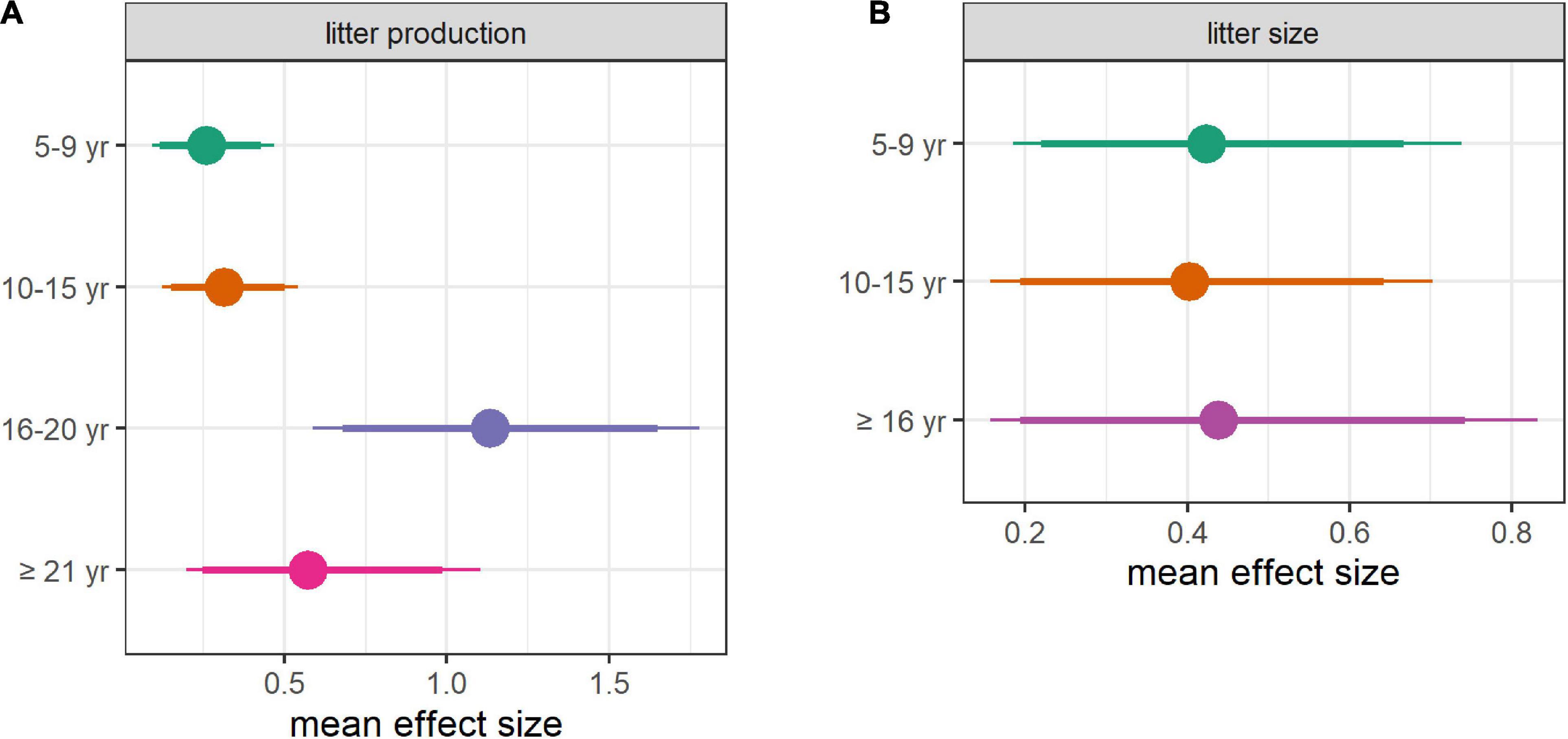
Figure 5. Mean effect size of all environmental covariates. Mean effect size of environmental covariates for (A) probability of litter production and (B) probability of a large litter, depending on female’s age. The mean (dot) with 89% CRI (thick line), and 95% CRI (thin line) are provided. Each distribution was obtained by averaging values in the posterior distributions across environmental covariates, iteration by iteration and for each age groups. Posteriors were on the logit scale. The models used for this figure included DateBreakUp as the sea-ice covariate (see Appendix 3, Supplementary Figure 9 for the version obtained when including DateFreezeUp).
Litter size
The date of sea-ice break-up in year t-1 was positively correlated to the probability of a large litter for females aged 10–15 (that is, the later the sea-ice retreated in the year prior to capture, the larger the litter, Figure 2B). By contrast, the date of sea-ice break-up in year t-1 had a non-significant negative effect on the probability of a large litter for females aged 5–9 and ≥16 (Figure 2B). There was a trend for a negative effect of the date of sea-ice freeze-up in year t-1 (Supplementary Figure 8B). The probability of a large litter increased with the winter AO index in year t-1 for females aged 5–9 (Figure 2B). Overall, the mean effect size of environmental covariates (including non-significant ones) was comparable across age groups (Figure 5B; Supplementary Figure 9B).
Since none of the environmental covariates were selected in both binomial regressions for a given age group, we obtained straightforward patterns of absolute probabilities (i.e., no environmental covariate increased the probability of litter production while reducing the probability of a large litter, or vice versa) (Figures 6, 7).
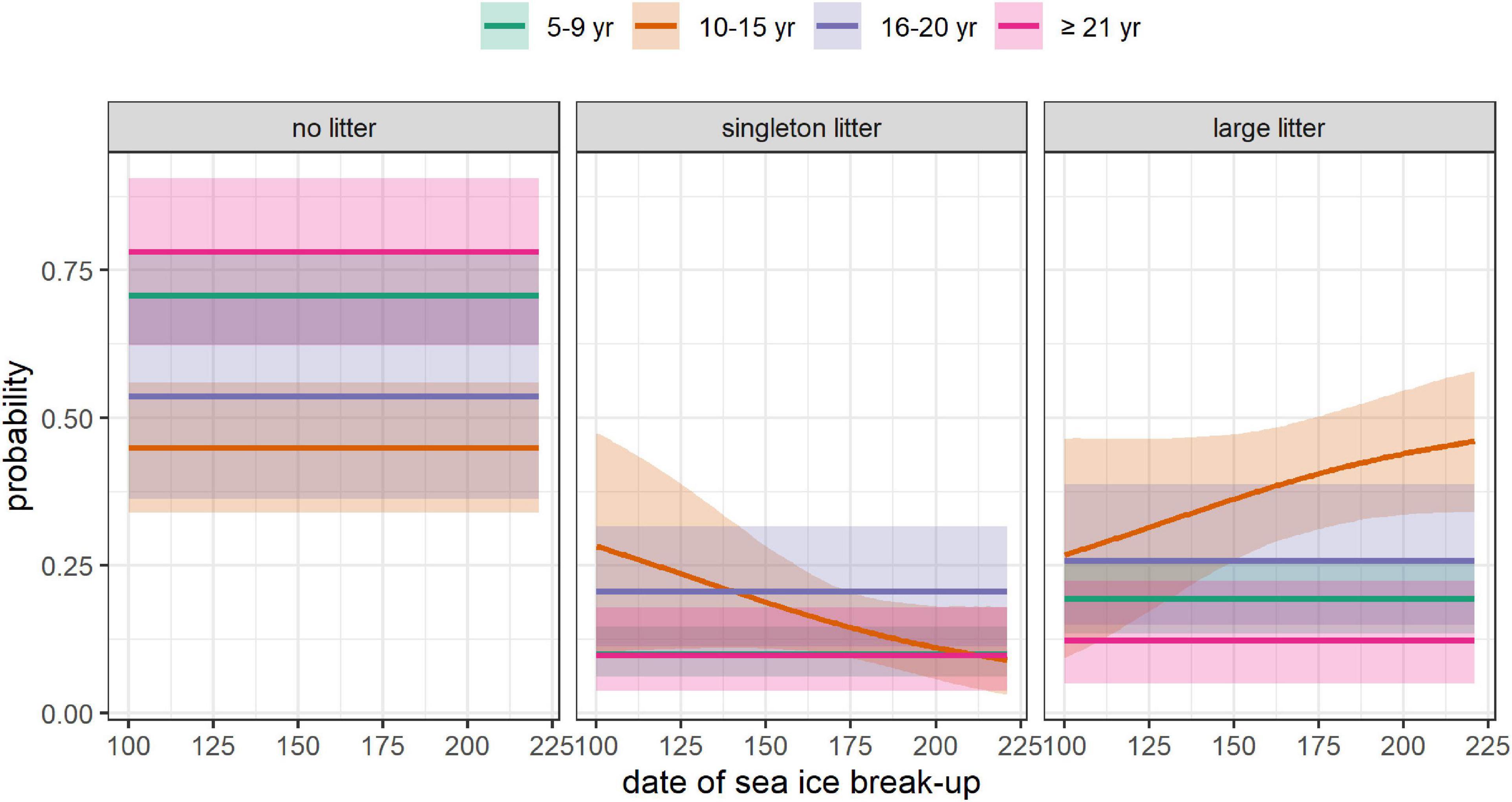
Figure 6. Effect of the date of sea-ice break-up in the year prior to capture on the absolute probability of having no litter, a singleton litter, or a large litter, depending on female’s age. The mean (solid line) and 95% CRI (shading) are provided. All the covariates other than DateBreakUp were set at their mean value (DateCapture = 104, i.e., April 14, Size = 195 cm, WinterAO = –0.06, PriorWinterAO = 0.01, PriorSpringAO = 0.12).
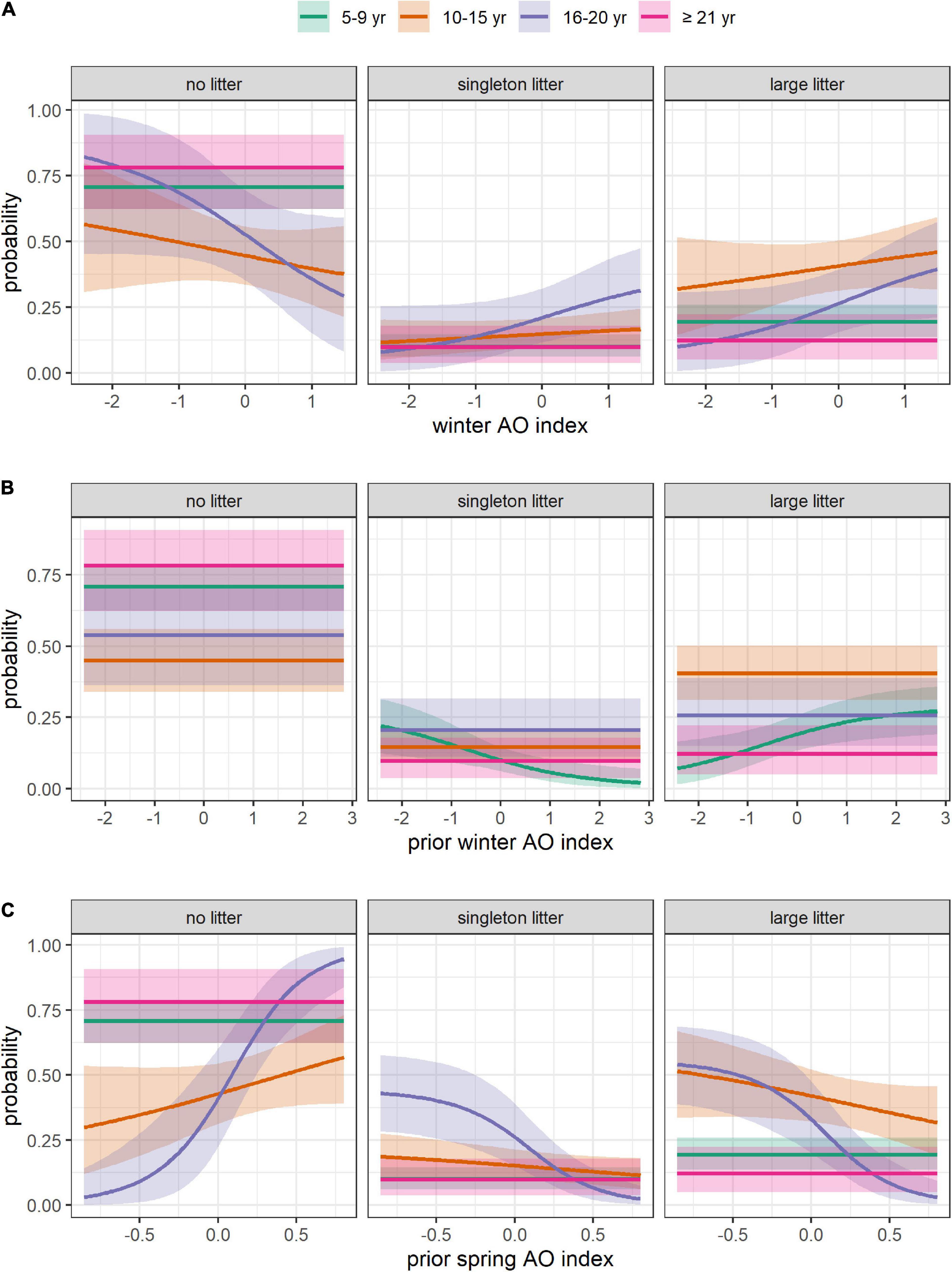
Figure 7. Effect of Arctic oscillation on the absolute probability of having no litter, a singleton litter, or a large litter, depending on female’s age. (A) Effect of the mean AO index over the winter of the year of capture. (B) Effect of the mean AO index over the winter of the year preceding capture. (C) Effect of the mean AO index over the spring of the year preceding capture. The mean (solid line) and 95% CRI (shading) are provided. All the covariates other than the one represented in each panel were set at their mean value (DateCapture = 104, i.e., April 14, Size = 195 cm, DateBreakUp = 174, i.e., June 23, WinterAO = –0.06, PriorWinterAO = 0.01, PriorSpringAO = 0.12).
Cub and litter survival
Our dataset consisted in 158 capture histories corresponding to 57 captures of independent juveniles and subadults, 444 captures of adult females, 63 singleton cub litters and 84 large cub litters, 73 yearling litters and 19 2-year-old litters.
Cub survival (from the cubs’ first spring to the following one) in singleton litters increased with mother’s age with a mean of 0.24, 0.58, and 0.75 for females aged 5–9, 10–15, and ≥16, respectively (Figure 8). Cub survival in twin litters also increased with mother age from a mean of 0.43 for females aged 5–9, to 0.54 for females aged 10–15, before reaching a plateau at 0.56 for females aged ≥16. Litter survival followed a similar pattern.
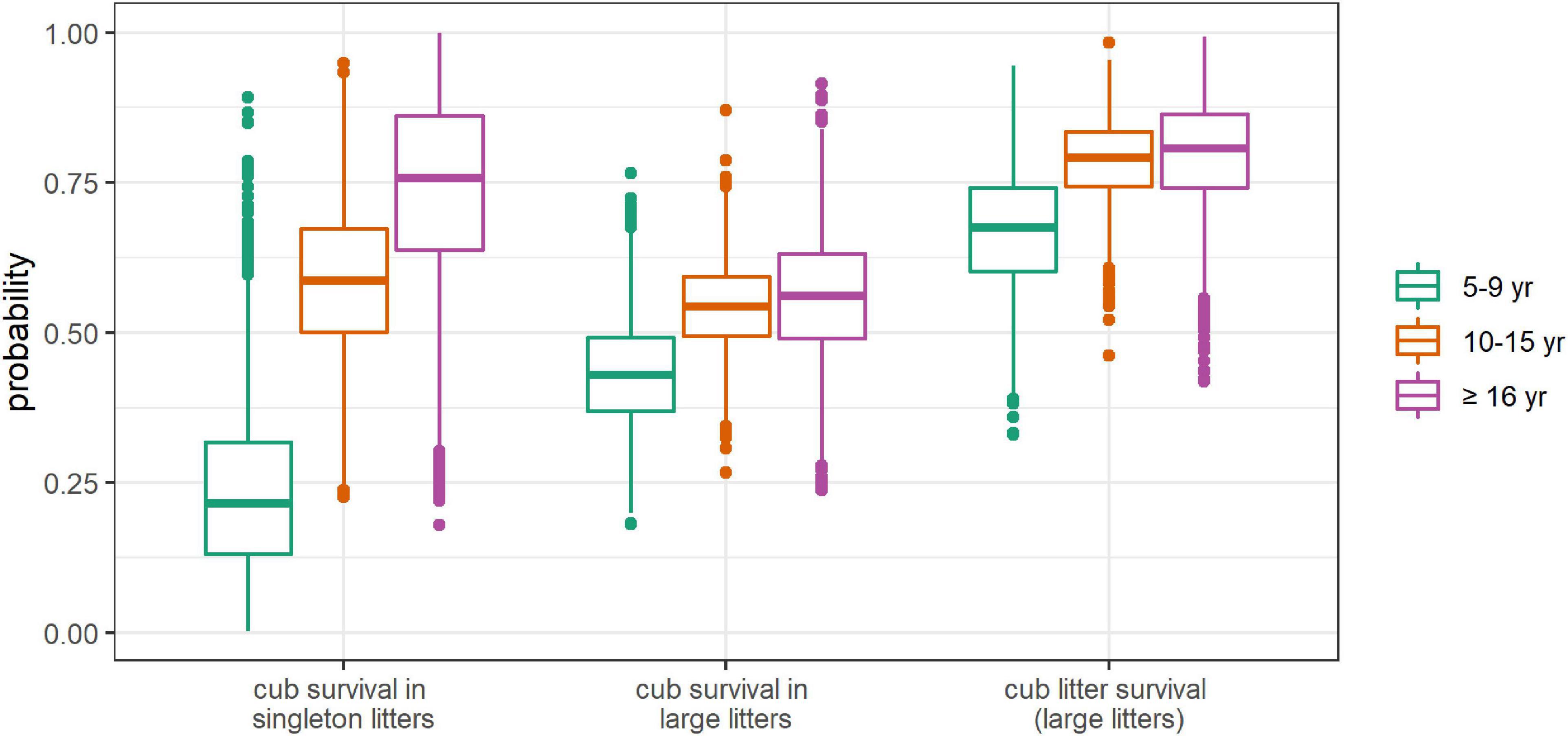
Figure 8. Posterior distributions of first-year cub and litter survival probabilities depending on mother’s age. First-year survival corresponds to survival between the age of 3–4 months and the age of 15–16 months (i.e., between the cubs’ first spring and the following one). First-year litter survival (as opposed to whole litter loss) refers to at least one cub of a large litter surviving.
Discussion
Overall, we found contrasted senescence patterns in the four indicators of reproductive performance of female polar bears. Females aged ≥16 had a lower probability of producing a litter and produced smaller litters, but their offspring first-year survival was higher than that of females aged 10–15. Young females had a lower probability of producing a litter and produced smaller litters with reduced cub survival chances over the field season and over their first year. We also showed that environmental conditions influenced reproductive output of all females, and more so for old females.
Contrasted patterns of senescence across the four reproductive traits
Senescence in litter production and litter size
We found a reduction in litter production and litter size for females ≥16 (Figure 3), in accordance with previous studies on this subpopulation (Folio et al., 2019) and Hudson Bay bears (Lunn et al., 2016). Senescence in litter size has been reported in other polytocous mammals [in carnivores: e.g., lions (Packer et al., 1998), red fox (Lieury et al., 2017), American minks (Melero et al., 2015), meerkats (Sharp and Clutton-Brock, 2010), in ungulates: e.g., moose (Ericsson et al., 2001), Soay sheep (Hayward et al., 2013)]. Similarly, senescence in litter production has been reported in several mammal species (Packer et al., 1998; Bowen et al., 2006; Sharp and Clutton-Brock, 2010; Melero et al., 2015), including brown bears (Schwartz et al., 2003). Interestingly, females aged 16–20 had only a slightly lower probability of producing a litter compared to females aged 10–15, but this was at the expense of litter size, with a greater proportion of their litters being singletons.
Senescence in litter production and litter size are thought to be a result of lower conception rates, implantation rates and/or higher rates of resorption/miscarriage in old females (Hewison and Gaillard, 2001; Melero et al., 2015; Lieury et al., 2017). In Hudson Bay polar bears, pregnancy rates are highest in young females then decline with age, with the sharpest decline occurring after age 20 (Derocher et al., 1992). Failure to enter estrous, to produce viable follicle or to implant embryos leading to lower pregnancy rates may account for the lower litter production and size of females in our study, as found in roe deer (Hewison and Gaillard, 2001). Even if old females mate and their eggs implant, they may be more prone to fetal loss (Melero et al., 2015; Lieury et al., 2017). These failures may be driven by the aging of the reproductive physiology, i.e., fertility senescence (Ellis et al., 2018). Alternatively, they may be mediated by senescence in body condition (Nussey et al., 2011). The amount of fat reserves females can accumulate before denning is known to influence reproductive performance, with fatter females being more likely to have cubs in the following spring (Derocher et al., 1992). In southern Hudson bay, old pregnant females are known to be lighter than prime-aged females at den entrance and at den emergence (Derocher et al., 1992; Derocher and Stirling, 1994), suggesting reduced body condition at old age. If such a decline occurs in Svalbard polar bears, it could reduce the ability of old females to conceive or to undergo denning, thereby reducing pregnancy rates and in turn litter production and litter size. In species such as polar bears for which litter size right after parturition is not known, postnatal mortality can lower apparent litter size, and even litter production rate in case of whole litter loss (Derocher et al., 1992). However, we found that in females aged ≥16, litter size did not decline over the field season (Figure 2B; Supplementary Figure 7). This suggests that old females are capable of providing adequate care to their offspring after den emergence, thereby avoiding litter size reduction and whole litter loss. Alternatively, females aged ≥16 with large litters may emerge from den later, masking litter losses of mothers who emerged early. This would explain the non-significant increase in litter size over the field season we observed (Figure 2B). In this case, the effect of the date of capture would reflect the ability of some old females to stay longer in den while keeping both (or all three) of their cubs alive. By contrast, our results suggest that young females struggle to keep all cubs in a large litter alive after den emergence (or to stay in den for longer while keeping their cubs alive), as indicated by the negative relationship between date of capture and litter size (Supplementary Figure 7).
Collectively, these findings suggest that lower reproductive output in old females is due to failure in early stages of the reproductive cycle, driven either by fertility or body condition senescence.
There are a few limits to our analysis. First, we did not consider the influence of reproductive status in year t-1 on the probability of producing a litter because including only females for which past reproductive history was known would have greatly reduced sample size. This may have introduced a bias because compared to successful breeders or previously lone females, females who lost a litter in year t-1 are less likely to produce a litter in year t (Cubaynes et al., 2021). While the probability of losing a yearling litter is low, the probability of losing a litter-of-the-year varies between 0.24 (twin litters) and 0.46 (singleton litters) (Cubaynes et al., 2021). Thus the presence of females who lost their litter in year t-1 in our dataset may have led to underestimating the probability of producing a litter. According to our findings, young females were more likely to lose their litter (Figure 8), suggesting their probability of producing a litter could have been biased low. However, young females may also be more likely to be nulliparous in year t-1, so it is unclear whether overall, their probability of producing a litter was under- or overestimated. Second, since the reproductive cycle of polar bears extends over several years, the number of females who are available to breed (including those who lose their offspring in time to breed) is influenced by environmental conditions experienced over the previous years. It is unclear in what directions these delayed effects of the environment could have biased our results, but they may have obscured the relationships between environmental conditions in year t-1 and the probability of producing a litter. Avoiding these biases would have required using a CR model with memory effects, which is much more complex than our models and requires more data, to be able to investigate litter production while accounting for past reproductive history, especially while controlling for the effect of the environment.
Absence of senescence in cub and litter survival
We did not detect any decline in offspring first-year survival with maternal age (Figure 8). This finding is consistent with the absence of a negative effect of the date of capture on litter size in females aged ≥16, further supporting the idea that older females produce fewer offspring or lose them soon after birth but can keep them alive after den emergence. This finding also suggests a weak contribution of maternal-effect senescence in polar bears.
However, the absence of decline in cub survival could result from methodological issues. Firstly, viability selection (or selective disappearance), that is the death of frail, low-quality individuals at an early age leaving only high-quality individuals in old age groups, is known to alter or even mask senescence (Nussey et al., 2008). Due to the relatively low recapture rate in our dataset, we could not include individual identity as a random effect in the GLMMs nor investigate the covariation between survival and parameters related to reproduction in each female in the CR model. However, we found evidence of strong senescence in litter production and litter size, which suggests that viability selection might not be strong enough to mask senescence in our dataset. In addition, we included female structural size in the GLMMs to account for individual quality and longevity as larger individuals usually live longer (Gaillard et al., 2000a) and have higher reproductive success (Derocher and Wiig, 2002; Folio et al., 2019). Importantly, not including size in the GLMMs yielded very similar results (see Appendix 4 for the model without size), further suggesting that the influence of selective disappearance on the observed senescence patterns is limited. Still, we acknowledge the possibility that our approach underestimates the magnitude of the decline in reproductive performance that occurs at old age in polar bears. Secondly, the age of females in the ≥16 age group ranges from 16 to 28, meaning there may be a lot of within-age-group heterogeneity. If only females slightly older than 15 reproduce while older females do not, the high cub survival rate could reflect the performance of the former, instead of the performance of the whole age class. However, very few females live past the age of 20, and those who did continued to produce cubs at least until age 24 (Supplementary Figure 5). This indicates that the high cub and litter survival rates do reflect the reproductive performance of the vast majority of females classified as old. There may nonetheless be a decline in cub survival at very old age although detecting it would be challenging given the very few females that reach that age.
Several studies have reported increasing or stable offspring survival or correlates of offspring survival with maternal age [e.g., in mammals (Cameron et al., 2000; Weladji et al., 2006; Hadley et al., 2007; Berger et al., 2015; Oosthuizen et al., 2015), and in birds (Mauck et al., 2012; Ivimey-Cook and Moorad, 2020; Nisbet et al., 2020)]. This pattern can be attributed to three biological processes. (i) terminal investment or allocation, (ii) an increasingly conservative tactic, and (iii) increased experience.
Under the terminal investment hypothesis, reproductive investment (and thereby reproductive cost) should increase with age as an individual’s residual reproductive value decreases (Clutton-Brock et al., 1989). For instance, female bottlenose dolphins delay weaning of their last offspring (Karniski et al., 2018), moose give birth to heavier offspring as they age (Ericsson et al., 2001), and old female North American red squirrels attempt a second reproduction in a given breeding season more often than young females (Descamps et al., 2007). In Svalbard polar bears, direct measures of reproductive allocation are difficult to acquire (e.g., rate of milk and energy transfer to cubs, mother weight loss), and some life-history traits indicative of reproductive allocation in other species show little or no variability (e.g., lactation length, offspring age at weaning, number of breeding attempts per season) (Derocher, 2012). In western Hudson Bay polar bears, Derocher and Stirling (1994) used the ratio of litter mass on mother mass as an indicator of maternal allocation and reported a concave-down relationship with mother’s age. This suggests that old female polar bears do not increase allocation-in reproduction to mitigate reproductive senescence.
Another possibility is that old females adopt a conservative reproductive tactic to lower the cost of reproduction by lengthening the interbirth interval and undertaking reproduction only if they have high chances of successfully raising offspring (i.e., if they are in good condition), as do Alpine ibex and southern elephant seals (Rughetti et al., 2015; Desprez et al., 2018). Under this hypothesis, and consistent with our results, litter production and litter size would be lower for old females than for prime-aged females, whereas offspring survival over their first few years would not necessarily be lower.
Finally, old females may benefit from additional experience, simply because they have had more time to accumulate experience (Komdeur, 1996; Mauck et al., 2012) and/or because they have had more breeding attempts (Broussard et al., 2008; Limmer and Becker, 2009; Desprez et al., 2011), which could offset the deleterious effects of maternal-effect senescence on offspring survival (Weladji et al., 2006). A skill that could improve with experience even in late life is habitat selection (Allen et al., 2022). For instance, females with cubs spend less time on active ice and more time on land fast ice compared to lone females (Stirling et al., 1993; Freitas et al., 2012). This is because swimming may be necessary on active ice, putting cubs at risk of hypothermia (Aars and Plumb, 2010), and cub mortality is consequently estimated to be 3.5 times higher on active ice (Reimer et al., 2019). But prey density and vulnerability are thought to be greater on active ice, meaning that females with cubs must weigh the prospect of increased energy intake and transfer to their offspring against the prospect of increased offspring mortality (Reimer et al., 2019). Older, more experienced females may be better able to make the optimal decision.
Overall reproductive senescence
Regardless of the mechanism underpinning the marked reduction of litter production and litter size in old females and the lack of decline in cub and litter survival, our findings are consistent with Lemaître and Gaillard’s prediction whereby senescence of traits involved in late stages of the reproductive cycle should be counter-selected more strongly than senescence in traits involved in early stages (Lemaître and Gaillard, 2017). In polar bears, implantation occurs months after mating, and gestation is thought to have a very small energetic cost as cubs are very small at birth (Derocher, 2012). Losing a cub before birth or after a relatively short period of nursing therefore doesn’t represent a major energetic cost. By contrast, losing a cub after a prolonged period of costly lactation likely entails a fitness cost, as it reduces prospects of future reproduction (Cubaynes et al., 2021). This fitness cost could be sizeable even for females aged ≥16 because the residual reproductive value of females aged 16 is substantial. Indeed, if a female survived to 24 years of age (our dataset includes 3% of adult females aged ≥24), she could wean up to six litters, three of which after age 16.
Nonetheless, according to our findings, the mean probability of a female producing a litter in a given year and of at least one cub reaching its second spring was 0.16 for females aged 5–9, 0.41 for females aged 10–15, 0.37 for females aged 16–20 and 0.18 for females aged ≥21. Thus, the increased survival of cubs of females aged ≥16 did not offset their reduced litter production and litter size, and reproductive output did decline at old age.
Effect of environmental conditions
We found that environmental covariates influenced reproductive success in all females (Figures 2, 5–7), in accordance with our expectations (Gaillard et al., 2000b). Indeed, long-lived species are expected to reduce their investment in current reproductive effort or even skip reproduction when environmental conditions are too harsh, in favor of survival and later reproduction (Clutton-Brock et al., 1983; Cubaynes et al., 2011; Lemaître et al., 2015; Desprez et al., 2018).
We found an effect of sea-ice dynamics on the reproductive output of females aged 10–15, with smaller litters following years with early spring break-up (Figure 6). Although the effects were not significant, we also found a trend for a positive effect the date of break-up and for a negative effect of the date of freeze-up on litter production (Figure 2A). Low sample size associated with wide CRI or the biases mentioned above caused by ignoring potential delayed effects of climate and past reproductive status may have reduced our ability to detect an effect of these covariates. These findings are consistent with previous mechanistic and empirical studies linking reductions in sea-ice availability to declines in reproductive output in other subpopulations (e.g., Rode et al., 2010; Molnár et al., 2011; Lunn et al., 2016; Reimer et al., 2019; Laidre et al., 2020). In the Barents Sea, sea-ice now breaks up in spring 40 days earlier than at the beginning of our study period and freezes up in autumn 55 days later (Stern and Laidre, 2016). Nonetheless, the negative effect of sea-ice loss on population size were not yet perceptible as of 2015 (Aars et al., 2017), perhaps because the population growth rate has not fallen below one yet.
We found that the AO had contrasting effect depending on the season and the time-lag considered (Figure 7). Notably, the winter AO in year t and t-1 was positively correlated with litter production and/or size for some age groups. These positive relationships are in contradiction with previous studies conducted in North America showing that a low winter AO index in year t and/or t-1 was associated with higher frequency of predation events by polar bears (Pilfold et al., 2015; Rode et al., 2018b), and higher reproductive output and body condition of females and dependent young (Rode et al., 2021). It’s unclear, however, whether the AO has the same effects in Svalbard, although a study conducted in East Greenland found high winter North Atlantic Oscillation (a close relative of the AO) index in year t to be associated with high hair cortisol concentration, an indicator of chronic stress and fasting state (Bechshøft et al., 2013).
We found that spring AO in year t-1 was negatively correlated with litter production of females aged 10–20, in accordance with a previous study conducted in Svalbard (Derocher, 2005). The effect of the AO on polar bears may be mediated by prey availability, as suggested by low ringed seal densities and body condition during or following years with a high NAO index in western Hudson Bay and Svalbard (Ferguson et al., 2005, 2020).
Interactive effect of age group and environmental conditions
We found that environmental conditions had different effects depending on the age group. A striking example is the effect size of the spring AO index in year t-1, which was six times higher for females aged 16–20 than it was for females aged 10–15. Such results highlight the need to account for potential interaction between age and environmental conditions when investigating age-related variation in demographic traits. While litter size was affected by environmental covariates at a similar level across age groups, litter production of females aged ≥16 was affected more strongly than that of younger females (Figure 5; Supplementary Figure 9). The reproductive success of old females was thus more sensitive to environmental conditions than that of younger females. From an evolutionary perspective, this finding is in accordance with the canalization theory whereby demographic parameters of individuals contributing less to the population growth rate should exhibit higher variability (Gaillard and Yoccoz, 2003). From an ecological point of view, senescent females are expected to be more vulnerable to environmental harshness (Bunce et al., 2005; King et al., 2005; Pardo et al., 2013). This is supported by the reduced body mass of old females in Western Hudson Bay (Derocher et al., 1992; Derocher and Stirling, 1994). Additional environmental hurdles such as low prey availability may compromise reproduction, lowering the probability of litter production in old females. A conservative tactic whereby old females attempt reproduction only if environmental conditions are favorable may also account for the high susceptibility of litter production to environmental variables. Interestingly, females aged ≥21 appeared to be less susceptible to environmental conditions than females aged 16–20, perhaps because at very old age, reproductive output decline regardless of environmental conditions, potentially as a result of fertility senescence.
It is surprising that we found only a weak impact of environmental conditions on litter production for young females (Gaillard et al., 2000b). Very few females have their first litter at age 5 (i.e., mate at age 4), perhaps because of preferential allocation to growth (Larue et al., 2021), which is still ongoing at that age (Derocher and Wiig, 2002). This allocation tactic may operate independently of environmental conditions, partly accounting for our finding. The reproduction strategy of females aged 6–9 may also be largely independent of environmental conditions, perhaps because of low-quality females delaying reproduction, regardless of environmental conditions (Fay et al., 2016). Another possibility is that the environmental covariates included in our analysis do not adequately represent the environmental conditions that influence litter production for young females. Such factor may operate at spatial and temporal scales that we could not examine in this study [e.g., wind speed over a few days (Pilfold et al., 2015)].
Perspectives
All females captured in Svalbard since 2011 have been equipped with light and temperature sensors, allowing to detect den entrance and parturition (Friebe et al., 2014; Olson et al., 2017). Further, many adult females have had collars that also provides accurate data on denning (Andersen et al., 2012). Future analyses using these data will allow to differentiate between females who entered den but did not give birth, females who entered den and gave birth but lost their litter, and females who didn’t go into denning at all, providing further insight into the drivers of reproductive senescence in female polar bears. These data will also allow to explore potential relationships between denning phenology and litter size and survival (Rode et al., 2018a). Studying age-related changes in female, litter and family mass will also likely improve our understanding of senescence. Finally, data from captive animals may also help assess the timing of senescence in some reproductive traits (e.g., litter size) as well as distinguish between the contribution of body-condition senescence and fertility senescence to reproductive senescence (Henriksen et al., 2005; Roof et al., 2005).
Our study of the aging of several reproductive traits demonstrates differences in the patterns of senescence across traits, emphasizing the need to investigate several indicators of reproductive output to grasp the overall pattern of reproductive senescence (Massot et al., 2011; Hayward et al., 2013). These differences may arise notably because the strength of natural selection varies depending on when each trait intervenes in the reproductive cycle (Lemaître and Gaillard, 2017), and because of distinct physiological mechanisms driving age-related changes in reproductive performance (i.e., fertility senescence and somatic senescence). Interpreting differences in patterns of senescence across traits in light of evolutionary theory and while considering underlying drivers may foster a clearer understanding of reproductive senescence as a whole. Finally, our study provides insight into how age and environmental conditions interactively determine reproductive output in capital-breeding mammals, highlighting the need to take them into account when investigating reproductive senescence. Comparing senescence patterns in subpopulations differentially affected by climate change may further unravel the interaction between environment and senescence (Kroeger et al., 2018). In turn, a thorough understanding of the age-specific effect of environmental variation will help to accurately forecast demographic responses to climate change.
Data availability statement
The datasets used in this study are available online at https://doi.org/10.6084/m9.figshare.20749141. R scripts used to generate results and create figures presented in the main text are freely available at https://github.com/MarwanNaciri/Reproductive_senescence_in_polar_bears_in_a_variable_environment.
Ethics statement
The animal study was reviewed and approved by Norwegian Food Safety Authority and the Governor of Svalbard.
Author contributions
JA conducted the fieldwork and curated the data. SC and MN designed the study with insight from JA, M-AB, and OG. MN ran the analyses under the supervision of SC and wrote the first draft. All authors contributed to manuscript revisions, read, and approved the submitted version.
Funding
This study was supported by World Wildlife Fund. MN and SC were supported by a grant from the Agence Nationale de la Recherche (ANR-18-CE02-0011, MathKinD).
Acknowledgments
We thank Magnus Andersen and Øystein Wiig for their participation to fieldwork and Thor Larsen for initiating the monitoring. We are grateful to Clément Brun who provided the MCP. We thank MF-B, AP, and one reviewer for their helpful comments.
Conflict of interest
The authors declare that the research was conducted in the absence of any commercial or financial relationships that could be construed as a potential conflict of interest.
The reviewer AP declared a past co-authorship with one of the authors JA to the handling editor.
Publisher’s note
All claims expressed in this article are solely those of the authors and do not necessarily represent those of their affiliated organizations, or those of the publisher, the editors and the reviewers. Any product that may be evaluated in this article, or claim that may be made by its manufacturer, is not guaranteed or endorsed by the publisher.
Supplementary material
The Supplementary Material for this article can be found online at: https://www.frontiersin.org/articles/10.3389/fevo.2022.920481/full#supplementary-material
Footnotes
- ^ https://www.mosj.no/en/influence/hunting-trapping/polar-bear-bag.html
- ^ https://www.climate.gov/news-features/understanding-climate/climate-variability-arctic-oscillation
References
Aars, J., and Plumb, A. (2010). Polar bear cubs may reduce chilling from icy water by sitting on mother’s back. Polar Biol. 33, 557–559. doi: 10.1007/s00300-009-0721-3
Aars, J., Marques, T. A., Lone, K., Andersen, M., Wiig, Ø., Bardalen Fløystad, I. M., et al. (2017). The number and distribution of polar bears in the western Barents Sea. Polar Res. 36:1374125. doi: 10.1080/17518369.2017.1374125
Allen, S. J. J., Bowen, W. D., and den Heyer, C. E. (2022). Birth-site habitat selection in gray seals (Halichoerus grypus): Effects of maternal age and parity and association with offspring weaning mass. Mar. Mamm. Sci. 38, 349–363. doi: 10.1111/mms.12867
Amstrup, S. C. (2003). “Polar bear, Ursus maritimus,” in Wild mammals of North America: Biology, management, and conservation, eds G. A. Feldhamer, B. C. Thompson, and J. A. Chapman (Baltimore, MD: The Johns Hopkins University Press), 587–610.
Amstrup, S. C., and Durner, G. M. (1995). Survival rates of radio-collared female polar bears and their dependent young. Can. J. Zool. 73, 1312–1322. doi: 10.1139/z95-155
Andersen, M., Derocher, A. E., Wiig, Ø., and Aars, J. (2012). Polar bear (Ursus maritimus) maternity den distribution in Svalbard, Norway. Polar Biol. 35, 499–508. doi: 10.1007/s00300-011-1094-y
Atkinson, S., and Ramsay, M. (1995). The Effects of Prolonged Fasting of the Body Composition and Reproductive Success of Female Polar Bears (Ursus maritimus). Funct. Ecol. 9:559. doi: 10.2307/2390145
Bechshøft, T. Ø., Sonne, C., Rigét, F. F., Letcher, R. J., Novak, M. A., Henchey, E., et al. (2013). Polar bear stress hormone cortisol fluctuates with the North Atlantic Oscillation climate index. Polar Biol. 36, 1525–1529. doi: 10.1007/s00300-013-1364-y
Berger, V., Lemaître, J. F., Gaillard, J. M., and Cohas, A. (2015). How do animals optimize the size–number trade-off when aging? Insights from reproductive senescence patterns in marmots. Ecology 96, 46–53. doi: 10.1890/14-0774.1
Bowen, W. D., Iverson, S. J., Mcmillan, J. I., and Boness, D. J. (2006). Reproductive performance in grey seals: Age-related improvement and senescence in a capital breeder. J. Anim. Ecol. 75, 1340–1351. doi: 10.1111/j.1365-2656.2006.01157.x
Broussard, D. R., Dobson, F. S., and Murie, J. O. (2008). Previous Experience and Reproductive Investment of Female Columbian Ground Squirrels. J. Mamm. 89, 145–152. doi: 10.1644/06-MAMM-A-357.1
Bunce, A., Ward, S. J., and Norman, F. I. (2005). Are age-related variations in breeding performance greatest when food availability is limited? J. Zool. 266, 163–169. doi: 10.1017/S0952836905006734
Calvert, W., and Ramsay, M. (1998). Evaluation of Age Determination of Polar Bears by Counts of Cementum Growth Layer Groups. Ursus 10, 449–453.
Cameron, E. Z., Linklater, W. L., Stafford, K. J., and Minot, E. O. (2000). Aging and improving reproductive success in horses: Declining residual reproductive value or just older and wiser? Behav. Ecol. Sociobiol. 47, 243–249. doi: 10.1007/s002650050661
Cavalieri, D. J., Parkinson, C. L., Gloersen, P., and Zwally, H. J. (1996). Sea Ice Concentrations from Nimbus-7 SMMR and DMSP SSM/I-SSMIS Passive Microwave Data, Version 1. Boulder: NASA National Snow and Ice Data Center Distributed Active Archive Center, doi: 10.5067/8GQ8LZQVL0VL
Cherry, S. G., Derocher, A. E., Thiemann, G. W., and Lunn, N. J. (2013). Migration phenology and seasonal fidelity of an Arctic marine predator in relation to sea ice dynamics. J. Anim. Ecol. 82, 912–921. doi: 10.1111/1365-2656.12050
Christensen-Dalsgaard, S. N., Aars, J., Andersen, M., Lockyer, C., and Yoccoz, N. G. (2010). Accuracy and precision in estimation of age of Norwegian Arctic polar bears (Ursus maritimus) using dental cementum layers from known-age individuals. Polar Biol. 33, 589–597. doi: 10.1007/s00300-009-0734-y
Clutton-Brock, T. H., Albon, S. D., and Guinness, F. E. (1989). Fitness costs of gestation and lactation in wild mammals. Nature 337, 260–262. doi: 10.1038/337260a0
Clutton-Brock, T. H., Guinness, F. E., and Albon, S. D. (1983). The Costs of Reproduction to Red Deer Hinds. J. Anim. Ecol. 52, 367–383. doi: 10.2307/4560
Cooper, E. B., and Kruuk, L. E. B. (2018). Ageing with a silver-spoon: A meta-analysis of the effect of developmental environment on senescence. Evol. Lett. 2, 460–471. doi: 10.1002/evl3.79
Cubaynes, S., Aars, J., Yoccoz, N. G., Pradel, R., Wiig, Ø., Ims, R. A., et al. (2021). Modeling the demography of species providing extended parental care: A capture–recapture multievent model with a case study on polar bears (Ursus maritimus). Ecol. Evol. 11, 3380–3392. doi: 10.1002/ece3.7296
Cubaynes, S., Doherty, P. F., Schreiber, E. A., and Gimenez, O. (2011). To breed or not to breed: A seabird’s response to extreme climatic events. Biol. Lett. 7, 303–306. doi: 10.1098/rsbl.2010.0778
Daunt, F., Wanless, S., Harris, M. P., Money, L., and Monaghan, P. (2007). Older and wiser: Improvements in breeding success are linked to better foraging performance in European shags. Funct. Ecol. 21, 561–567. doi: 10.1111/j.1365-2435.2007.01260.x
de Valpine, P., Paciorek, C., Turek, D., Michaud, N., Anderson-Bergman, C., Obermeyer, F., et al. (2021). nimble: MCMC, Particle Filtering, and Programmable Hierarchical Modeling. Available online at: https://CRAN.R-project.org/package=nimble (accessed on Jan 12, 2022).
Derocher, A. E. (2005). Population ecology of polar bears at Svalbard. Norway. Popul. Ecol. 47, 267–275. doi: 10.1007/s10144-005-0231-2
Derocher, A. E. (2012). Polar bears: A complete guide to their biology and behavior. Baltimore: Hopkins university press.
Derocher, A. E., and Stirling, I. (1994). Age-specific reproductive performance of female polar bears (Ursus maritimus). J. Zool. 234, 527–536. doi: 10.1111/j.1469-7998.1994.tb04863.x
Derocher, A. E., and Wiig, Ø. (2002). Postnatal growth in body length and mass of polar bears (Ursus maritimus) at Svalbard. J. Zool. 256, 343–349. doi: 10.1017/S0952836902000377
Derocher, A. E., Stirling, I., and Andriashek, D. (1992). Pregnancy rates and serum progesterone levels of polar bears in western Hudson Bay. Can. J. Zool. 70, 561–566. doi: 10.1139/z92-084
Derocher, A., and Stirling, I. (1996). Aspects of survival in juvenile polar bears. Can. J. Zool. 74, 1246–1252. doi: 10.1139/z96-138
Derocher, A., Andersen, M., Wiig, Ø., Aars, J., Hansen, E., and Biuw, M. (2011). Sea ice and polar bear den ecology at Hopen Island, Svalbard. Mar. Ecol. Prog. Ser. 441, 273–279. doi: 10.3354/meps09406
Descamps, S., Boutin, S., Berteaux, D., and Gaillard, J. M. (2007). Female red squirrels fit Williams’ hypothesis of increasing reproductive effort with increasing age. J. Anim. Ecol. 76, 1192–1201. doi: 10.1111/j.1365-2656.2007.01301.x
Desprez, M., Gimenez, O., McMahon, C. R., Hindell, M. A., and Harcourt, R. G. (2018). Optimizing lifetime reproductive output: Intermittent breeding as a tactic for females in a long-lived, multiparous mammal. J. Anim. Ecol. 87, 199–211. doi: 10.1111/1365-2656.12775
Desprez, M., Pradel, R., Cam, E., Monnat, J. Y., and Gimenez, O. (2011). Now you see him, now you don’t: Experience, not age, is related to reproduction in kittiwakes. Proc. R. Soc. B Biol. Sci. 278, 3060–3066. doi: 10.1098/rspb.2011.0189
Ellis, S., Franks, D. W., Nattrass, S., Currie, T. E., Cant, M. A., Giles, D., et al. (2018). Analyses of ovarian activity reveal repeated evolution of post-reproductive lifespans in toothed whales. Sci. Rep. 8:12833. doi: 10.1038/s41598-018-31047-8
Ericsson, G., Wallin, K., Ball, J. P., and Broberg, M. (2001). ). Age-Related Reproductive Effort and Senescence in Free-Ranging Moose, Alces Alces. Ecology 82, 1613–1620.
Fay, R., Barbraud, C., Delord, K., and Weimerskirch, H. (2016). Variation in the age of first reproduction: Different strategies or individual quality? Ecology 97, 1842–1851. doi: 10.1890/15-1485.1
Ferguson, S. H., Stirling, I., and McLoughlin, P. (2005). Climate Change and Ringed Seal (Phoca hispida) Recruitment in Western Hudson Bay. Mar. Mamm. Sci. 21, 121–135. doi: 10.1111/j.1748-7692.2005.tb01212.x
Ferguson, S. H., Yurkowski, D. J., Young, B. G., Fisk, A. T., Muir, D. C. G., Zhu, X., et al. (2020). Comparing temporal patterns in body condition of ringed seals living within their core geographic range with those living at the edge. Ecography 43, 1521–1535. doi: 10.1111/ecog.04988
Folio, D. M., Aars, J., Gimenez, O., Derocher, A. E., Wiig, Ø., and Cubaynes, S. (2019). How many cubs can a mum nurse? Maternal age and size influence litter size in polar bears. Biol. Lett. 15:20190070. doi: 10.1098/rsbl.2019.0070
Freitas, C., Kovacs, K. M., Andersen, M., Aars, J., Sandven, S., Skern-Mauritzen, M., et al. (2012). Importance of fast ice and glacier fronts for female polar bears and their cubs during spring in Svalbard. Norway. Mar. Ecol. Prog. Ser. 447, 289–304. doi: 10.3354/meps09516
Freitas, C., Lydersen, C., Fedak, M. A., and Kovacs, K. M. (2008). A simple new algorithm to filter marine mammal Argos locations. Mar. Mamm. Sci. 24, 315–325. doi: 10.1111/j.1748-7692.2007.00180.x
Friebe, A., Evans, A. L., Arnemo, J. M., Blanc, S., Brunberg, S., Fleissner, G., et al. (2014). Factors Affecting Date of Implantation, Parturition, and Den Entry Estimated from Activity and Body Temperature in Free-Ranging Brown Bears. PLoS One 9:e101410. doi: 10.1371/journal.pone.0101410
Gaillard, J. M., and Lemaître, J. F. (2020). An integrative view of senescence in nature. Funct. Ecol. 34, 4–16. doi: 10.1111/1365-2435.13506
Gaillard, J. M., and Yoccoz, N. (2003). Temporal variation in survival of mammals: A case of environmental canalization? Ecology 84, 3294–3306. doi: 10.1890/02-0409
Gaillard, J. M., Festa-Bianchet, M., Delorme, D., and Jorgenson, J. (2000a). Body mass and individual fitness in female ungulates: Bigger is not always better. Proc. R. Soc. Lond. Ser. B Biol. Sci. 267, 471–477. doi: 10.1098/rspb.2000.1024
Gaillard, J. M., Festa-Bianchet, M., Yoccoz, N. G., Loison, A., and Toïgo, C. (2000b). Temporal Variation in Fitness Components and Population Dynamics of Large Herbivores. Annu. Rev. Ecol. Syst. 31, 367–393. doi: 10.1146/annurev.ecolsys.31.1.367
Galicia, M. P., Thiemann, G. W., and Dyck, M. G. (2020). Correlates of seasonal change in the body condition of an Arctic top predator. Glob. Change Biol. 26, 840–850. doi: 10.1111/gcb.14817
Gelman, A., and Rubin, D. B. (1992). Inference from Iterative Simulation Using Multiple Sequences. Statist. Sci. 7, 457–472. doi: 10.1214/ss/1177011136
Gimenez, O., Cam, E., and Gaillard, J. M. (2018). Individual heterogeneity and capture–recapture models: What, why and how? Oikos 127, 664–686. doi: 10.1111/oik.04532
Hadley, G. L., Rotella, J. J., and Garrott, R. A. (2007). Influence of maternal characteristics and oceanographic conditions on survival and recruitment probabilities of Weddell seals. Oikos 116, 601–613. doi: 10.1111/j.0030-1299.2007.15528.x
Hansson, R., and Thomassen, J. (1983). Behavior of Polar Bears with Cubs in the Denning Area. Bears Their Biol. Manag. 5:246. doi: 10.2307/3872544
Hayward, A. D., Moorad, J., Regan, C. E., Berenos, C., Pilkington, J. G., Pemberton, J. M., et al. (2015). Asynchrony of senescence among phenotypic traits in a wild mammal population. Exp. Gerontol. 71, 56–68. doi: 10.1016/j.exger.2015.08.003
Hayward, A. D., Wilson, A. J., Pilkington, J. G., Clutton-Brock, T. H., Pemberton, J. M., and Kruuk, L. E. B. (2013). Reproductive senescence in female Soay sheep: Variation across traits and contributions of individual ageing and selective disappearance. Funct. Ecol. 27, 184–195. doi: 10.1111/1365-2435.12029
Henriksen, H. B., Andersen, R., Hewison, A. J. M., Gaillard, J. M., Bronndal, M., Jonsson, S., et al. (2005). Reproductive biology of captive female Eurasian lynx, Lynx lynx. Eur. J. Wildl. Res. 51, 151–156. doi: 10.1007/s10344-005-0104-1
Hewison, A. J. M., and Gaillard, J. M. (2001). Phenotypic quality and senescence affect different components of reproductive output in roe deer. J. Anim. Ecol. 70, 600–608. doi: 10.1046/j.1365-2656.2001.00528.x
Ivimey-Cook, E., and Moorad, J. (2020). The diversity of maternal-age effects upon pre-adult survival across animal species. Proc. R. Soc. B Biol. Sci. 287:20200972. doi: 10.1098/rspb.2020.0972
Jones, O. R., Scheuerlein, A., Salguero-Gómez, R., Camarda, C. G., Schaible, R., Casper, B. B., et al. (2014). Diversity of ageing across the tree of life. Nature 505, 169–173. doi: 10.1038/nature12789
Karniski, C., Krzyszczyk, E., and Mann, J. (2018). Senescence impacts reproduction and maternal investment in bottlenose dolphins. Proc. R. Soc. B 285:20181123. doi: 10.1098/rspb.2018.1123
Kern, S., Kaleschke, L., Girard-Ardhuin, F., Spreen, G., and Beitsch, A. (2020). Global daily gridded 5-day median-filtered, gap-filled ASI Algorithm SSMI-SSMIS sea ice concentration data, Integrated Climate Date Center (ICDC), CEN, University of Hamburg, Germany. Available online at: https://www.cen.uni-hamburg.de/en/icdc/data/cryosphere/seaiceconcentration-asi-ssmi.html (accessed on Jan 19, 2022).
King, S. J., Arrigo-Nelson, S. J., Pochron, S. T., Semprebon, G. M., Godfrey, L. R., Wright, P. C., et al. (2005). Dental senescence in a long-lived primate links infant survival to rainfall. PNAS 102, 16579–16583. doi: 10.1073/pnas.0508377102
Komdeur, J. (1996). Influence of age on reproductive performance in the Seychelles warbler. Behav. Ecol. 7, 417–425. doi: 10.1093/beheco/7.4.417
Kroeger, S. B., Blumstein, D. T., Armitage, K. B., Reid, J. M., and Martin, J. G. A. (2018). Age, state, environment, and season dependence of senescence in body mass. Ecol. Evol. 8, 2050–2061. doi: 10.1002/ece3.3787
Kruschke, J. (2015). Doing Bayesian Data Analysis: A Tutorial with R, JAGS, and Stan, (2nd Edn). Amsterdam: Elsevier, doi: 10.1016/B978-0-12-405888-0.09999-2
Laidre, K. L., Atkinson, S., Regehr, E. V., Stern, H. L., Born, E. W., Wiig, Ø., et al. (2020). Interrelated ecological impacts of climate change on an apex predator. Ecol. Appl. 30:e02071. doi: 10.1002/eap.2071
Larue, B., Pelletier, F., Côté, S. D., Hamel, S., and Festa-Bianchet, M. (2021). Growth and reproduction trade-offs can estimate previous reproductive history in alpine ungulates. J. Appl. Ecol. 58, 869–878. doi: 10.1111/1365-2664.13840
Lemaître, J. F., and Gaillard, J. M. (2017). Reproductive senescence: New perspectives in the wild. Biol. Rev. 92, 2182–2199. doi: 10.1111/brv.12328
Lemaître, J. F., Ronget, V., and Gaillard, J. M. (2020). Female reproductive senescence across mammals: A high diversity of patterns modulated by life history and mating traits. Mech. Ageing Dev. 192:111377. doi: 10.1016/j.mad.2020.111377
Lemaître, J.-F., Berger, V., Bonenfant, C., Douhard, M., Gamelon, M., Plard, F., et al. (2015). Early-late life trade-offs and the evolution of ageing in the wild. Proc. R. Soc. B Biol. Sci. 282:20150209. doi: 10.1098/rspb.2015.0209
Lieury, N., Drouet-Hoguet, N., Ruette, S., Devillard, S., Albaret, M., and Millon, A. (2017). Rural populations of the red fox Vulpes vulpes show little evidence of reproductive senescence. Mamm. Biol. 87, 146–151. doi: 10.1016/j.mambio.2017.08.008
Limmer, B., and Becker, P. H. (2009). Improvement in chick provisioning with parental experience in a seabird. Anim. Behav. 77, 1095–1101. doi: 10.1016/j.anbehav.2009.01.015
Lønø, O. (1970). The Polar bear (Ursus maritimus Phipps) in the Svalbard area. 118. Available online at: https://brage.npolar.no/npolar-xmlui/handle/11250/173883 (accessed on Feb 11, 2021).
Lunn, N. J., Servanty, S., Regehr, E. V., Converse, S. J., Richardson, E., and Stirling, I. (2016). Demography of an apex predator at the edge of its range: Impacts of changing sea ice on polar bears in Hudson Bay. Ecol. Appl. 26, 1302–1320. doi: 10.1890/15-1256
MacNulty, D. R., Smith, D. W., Vucetich, J. A., Mech, L. D., Stahler, D. R., and Packer, C. (2009). Predatory senescence in ageing wolves. Ecol. Lett. 12, 1347–1356. doi: 10.1111/j.1461-0248.2009.01385.x
Massot, M., Clobert, J., Montes-Poloni, L., Haussy, C., Cubo, J., and Meylan, S. (2011). An integrative study of ageing in a wild population of common lizards. Funct. Ecol. 25, 848–858. doi: 10.1111/j.1365-2435.2011.01837.x
Mauck, R. A., Huntington, C. E., and Doherty, P. F. Jr. (2012). Experience versus effort: What explains dynamic heterogeneity with respect to age? Oikos 121, 1379–1390. doi: 10.1111/j.1600-0706.2012.20271.x
Mauritzen, M., Derocher, A. E., and Wiig, Ø. (2001). Space-use strategies of female polar bears in a dynamic sea ice habitat. Can. J. Zool. 79, 1704–1713. doi: 10.1139/z01-126
McElreath, R. (2020). Statistical rethinking: A Bayesian course with examples in R and Stan, (2nd Edn). Boca Raton: Taylor and Francis, CRC Press.
Melero, Y., Robinson, E., and Lambin, X. (2015). Density- and age-dependent reproduction partially compensates culling efforts of invasive non-native American mink. Biol. Invasions 17, 2645–2657. doi: 10.1007/s10530-015-0902-7
Mohr, C. O. (1947). Table of Equivalent Populations of North American Small Mammals. Am. Midl. Nat. 37, 223–249. doi: 10.2307/2421652
Molnár, P. K., Bitz, C. M., Holland, M. M., Kay, J. E., Penk, S. R., and Amstrup, S. C. (2020). Fasting season length sets temporal limits for global polar bear persistence. Nat. Clim. Change 10, 732–738. doi: 10.1038/s41558-020-0818-9
Molnár, P. K., Derocher, A. E., Klanjscek, T., and Lewis, M. A. (2011). Predicting climate change impacts on polar bear litter size. Nat. Commun. 2:186. doi: 10.1038/ncomms1183
Moorad, J. A., and Nussey, D. H. (2016). Evolution of maternal effect senescence. Proc. Natl. Acad. Sci. U. S. A. 113, 362–367. doi: 10.1073/pnas.1520494113
Morin, A., Rughetti, M., Rioux-Paquette, S., and Festa-Bianchet, M. (2016). Older conservatives: Reproduction in female Alpine chamois (Rupicapra rupicapra) is increasingly risk-averse with age. Can. J. Zool. 94, 311–321. doi: 10.1139/cjz-2015-0153
Nevoux, M., Weimerskirch, H., and Barbraud, C. (2007). Environmental variation and experience-related differences in the demography of the long-lived black-browed albatross. J. Anim. Ecol. 76, 159–167. doi: 10.1111/j.1365-2656.2006.01191.x
Nisbet, I. C. T., Iles, D., Kaneb, A., Mostello, C. S., and Jenouvrier, S. (2020). Breeding performance of Common Terns (Sterna hirundo) does not decline among older age classes. Auk 137:ukaa022. doi: 10.1093/auk/ukaa022
Nussey, D. H., Coulson, T., Delorme, D., Clutton-Brock, T. H., Pemberton, J. M., Festa-Bianchet, M., et al. (2011). Patterns of body mass senescence and selective disappearance differ among three species of free-living ungulates. Ecology 92, 1936–1947. doi: 10.1890/11-0308.1
Nussey, D. H., Coulson, T., Festa-Bianchet, M., and Gaillard, J. M. (2008). Measuring senescence in wild animal populations: Towards a longitudinal approach. Funct. Ecol. 22, 393–406. doi: 10.1111/j.1365-2435.2008.01408.x
Nussey, D. H., Kruuk, L. E. B., Morris, A., and Clutton-Brock, T. H. (2007). Environmental conditions in early life influence ageing rates in a wild population of red deer. Curr. Biol. 17, R1000–R1001. doi: 10.1016/j.cub.2007.10.005
Nussey, D. H., Kruuk, L. E. B., Morris, A., Clements, M. N., Pemberton, J. M., and Clutton-Brock, T. H. (2009). Inter- and Intrasexual Variation in Aging Patterns across Reproductive Traits in a Wild Red Deer Population. Am. Nat. 174, 342–357. doi: 10.1086/603615
Obbard, M. E., Cattet, M. R. L., Howe, E. J., Middel, K. R., Newton, E. J., Kolenosky, G. B., et al. (2016). Trends in body condition in polar bears (Ursus maritimus) from the Southern Hudson Bay subpopulation in relation to changes in sea ice. Arct. Sci. 2, 15–32. doi: 10.1139/as-2015-0027
Obbard, M., Cattet, M., Moody, T., Walton, L., Potter, D., Inglis, J., et al. (2006). Temporal Trends in the Body Condition of Southern Hudson Bay Polar Bears. Climate Change Research Information Note, No. 3. Ontario: Ontario Ministry of Natural Resources.
Olson, J. W., Rode, K. D., Eggett, D., Smith, T. S., Wilson, R. R., Durner, G. M., et al. (2017). Collar temperature sensor data reveal long-term patterns in southern Beaufort Sea polar bear den distribution on pack ice and land. Mar. Ecol. Prog. Ser. 564, 211–224. doi: 10.3354/meps12000
Oosthuizen, W. C., Bester, M. N., Altwegg, R., McIntyre, T., and de Bruyn, P. J. N. (2015). Decomposing the variance in southern elephant seal weaning mass: Partitioning environmental signals and maternal effects. Ecosphere 6:art139. doi: 10.1890/ES14-00508.1
Oro, D., Hernández, N., Jover, L., and Genovart, M. (2014). From recruitment to senescence: Food shapes the age-dependent pattern of breeding performance in a long-lived bird. Ecology 95, 446–457. doi: 10.1890/13-0331.1
Packer, C., Tatar, M., and Collins, A. (1998). Reproductive cessation in female mammals. Nature 392, 807–811. doi: 10.1038/33910
Pardo, D., Barbraud, C., Authier, M., and Weimerskirch, H. (2013). Evidence for an age-dependent influence of environmental variations on a long-lived seabird’s life-history traits. Ecology 94, 208–220. doi: 10.1890/12-0215.1
Pedersen, T. L. (2020). patchwork: The Composer of Plots. Available online at: https://CRAN.R-project.org/package=patchwork (accessed March 15, 2022).
Pilfold, N. W., Derocher, A. E., Stirling, I., and Richardson, E. (2015). Multi-temporal factors influence predation for polar bears in a changing climate. Oikos 124, 1098–1107. doi: 10.1111/oik.02000
Ramsay, M., and Stirling, I. (1988). Reproductive biology and ecology of female polar bears (Ursus maritimus). J. Zool. 214, 601–634. doi: 10.1111/j.1469-7998.1988.tb03762.x
Ratcliffe, N., Furness, R. W., and Hamer, K. C. (1998). The interactive effects of age and food supply on the breeding ecology of great skuas. J. Anim. Ecol. 67, 853–862. doi: 10.1046/j.1365-2656.1998.6760853.x
Reimer, J. R., Mangel, M., Derocher, A. E., and Lewis, M. A. (2019). Modeling optimal responses and fitness consequences in a changing Arctic. Glob. Change Biol. 25, 3450–3461. doi: 10.1111/gcb.14681
Rigor, I. G., Wallace, J. M., and Colony, R. L. (2002). Response of Sea Ice to the Arctic Oscillation. J. Clim. 15, 2648–2663. doi: 10.1175/1520-04422002015<2648:ROSITT<2.0.CO;2
Rode, K. D., Amstrup, S. C., and Regehr, E. V. (2010). Reduced body size and cub recruitment in polar bears associated with sea ice decline. Ecol. Appl. 20, 768–782.
Rode, K. D., Wilson, R. R., Douglas, D. C., Muhlenbruch, V., Atwood, T. C., Regehr, E. V., et al. (2018b). Spring fasting behavior in a marine apex predator provides an index of ecosystem productivity. Glob. Change Biol. 24, 410–423.
Rode, K. D., Olson, J., Eggett, D., Douglas, D. C., Durner, G. M., Atwood, T. C., et al. (2018a). Den phenology and reproductive success of polar bears in a changing climate. J. Mamm. 99, 16–26. doi: 10.1093/jmammal/gyx181
Rode, K. D., Regehr, E. V., Bromaghin, J. F., Wilson, R. R., St. Martin, M., Crawford, J. A., et al. (2021). Seal body condition and atmospheric circulation patterns influence polar bear body condition, recruitment, and feeding ecology in the Chukchi Sea. Glob. Change Biol. 27, 2684–2701. doi: 10.1111/gcb.15572
Roof, K. A., Hopkins, W. D., Izard, M. K., Hook, M., and Schapiro, S. J. (2005). Maternal age, parity, and reproductive outcome in captive chimpanzees (Pan troglodytes). Am. J. Primatol. 67, 199–207. doi: 10.1002/ajp.20177
RStudio Team (2021). RStudio: Integrated Development Environment for R. Available online at: http://www.rstudio.com/ (accessed March 15, 2022).
Rughetti, M., Dematteis, A., Meneguz, P. G., and Festa-Bianchet, M. (2015). Age-specific reproductive success and cost in female Alpine ibex. Oecologia 178, 197–205. doi: 10.1007/s00442-014-3192-3
Schwartz, C. C., Keating, K. A., Reynolds, H. V., Barnes, V. G., Sellers, R. A., Swenson, J. E., et al. (2003). Reproductive Maturation and Senescence in the Female Brown Bear. Ursus 14, 109–119.
Sharp, S. P., and Clutton-Brock, T. H. (2010). Reproductive senescence in a cooperatively breeding mammal. J. Anim. Ecol. 79, 176–183. doi: 10.1111/j.1365-2656.2009.01616.x
Stern, H. L., and Laidre, K. L. (2016). Sea-ice indicators of polar bear habitat. Cryosphere 10, 2027–2041. doi: 10.5194/tc-10-2027-2016
Stirling, I. (2011). Polar bears: The natural history of a threatened species. Markham, ONT: Fitzhenry & Whiteside.
Stirling, I., and Archibald, W. R. (1977). Aspects of Predation of Seals by Polar Bears. J. Fish. Res. Bd. Can. 34, 1126–1129. doi: 10.1139/f77-169
Stirling, I., and Derocher, A. E. (2012). Effects of climate warming on polar bears: A review of the evidence. Glob. Change Biol. 18, 2694–2706. doi: 10.1111/j.1365-2486.2012.02753.x
Stirling, I., Andriashek, D., and Calvert, W. (1993). Habitat preferences of polar bears in the western Canadian Arctic in late winter and spring. Polar Record 29, 13–24. doi: 10.1017/S0032247400023172
Stirling, I., Spencer, C., and Andriashek, D. (1989). Immobilization of polar bears (Ursus maritimus) with Telazol in the Canadian Arctic. J. Wildl. Dis. 25, 159–168. doi: 10.7589/0090-3558-25.2.159
Su, Y. S., and Yajima, M. (2020). R2jags: Using R to Run “JAGS.”. Available online at: https://CRAN.R-project.org/package=R2jags (accessed on Mar 8, 2022).
Vieyra, L., Velarde, E., and Ezcurra, E. (2009). Effects of parental age and food availability on the reproductive success of Heermann’s Gulls in the Gulf of California. Ecology 90, 1084–1094. doi: 10.1890/07-2009.1
Weigl, R. (2005). Longevity of mammals in captivity: From the living collections of the world: A list of mammalian longevity in captivity. Stuttgart: E. Schweizerbart’sche.
Weladji, R. B., Gaillard, J. M., Yoccoz, N. G., Holand, Ø., Mysterud, A., Loison, A., et al. (2006). Good reindeer mothers live longer and become better in raising offspring. Proc. R. Soc. B 273, 1239–1244. doi: 10.1098/rspb.2005.3393
Wickham, H., Chang, W., Henry, L., Pedersen, T. L., Takahashi, K., Wilke, C., et al. (2020). ggplot2: Create Elegant Data Visualisations Using the Grammar of Graphics. Available online at: https://CRAN.R-project.org/package=ggplot2 (accessed on Sep 1, 2020).
Keywords: polar bears, sea ice, fertility, reproductive senescence, litter size, offspring survival, environment, litter production
Citation: Naciri M, Aars J, Blanchet M-A, Gimenez O and Cubaynes S (2022) Reproductive senescence in polar bears in a variable environment. Front. Ecol. Evol. 10:920481. doi: 10.3389/fevo.2022.920481
Received: 14 April 2022; Accepted: 05 September 2022;
Published: 05 October 2022.
Edited by:
Jean-Michel Gaillard, Université Claude Bernard Lyon 1, FranceReviewed by:
Marco Festa-Bianchet, Université de Sherbrooke, CanadaAnthony Michael Pagano, United States Geological Survey (USGS), United States
Copyright © 2022 Naciri, Aars, Blanchet, Gimenez and Cubaynes. This is an open-access article distributed under the terms of the Creative Commons Attribution License (CC BY). The use, distribution or reproduction in other forums is permitted, provided the original author(s) and the copyright owner(s) are credited and that the original publication in this journal is cited, in accordance with accepted academic practice. No use, distribution or reproduction is permitted which does not comply with these terms.
*Correspondence: Marwan Naciri, bWFyd2FuLm5hY2lyaUBjZWZlLmNucnMuZnI=