- 1Department of Evolutionary Studies of Biosystems, The Graduate University for Advanced Studies (SOKENDAI), Hayama, Japan
- 2Department of General Systems Studies, Graduate School of Arts and Sciences, The University of Tokyo, Tokyo, Japan
In addition to habitat loss and fragmentation, behavioral traits and the deterioration of water environments also contribute to the local extinction of amphibians. Abundant microflora in urban ponds may cause fatal diseases, whereas symbiotic bacteria may protect the host from pathogens; these effects may vary with group size. In this study, I monitored the growth of Japanese common toad (Bufo japonicus) larvae in Tokyo using three different group sizes: 1 (solitary), 2 (pair), and 15. Although there was no genetic bias in the major histocompatibility complex (MHC) class II genes or microsatellite loci to the survival of the larvae, the mortality risk of the larvae reared in pond water was higher than that of those reared in tap water. According to the survival analysis, the risk was more significant when the group size was 15. This result would be unwelcomed for the B. japonicus tadpoles, which have habits of social aggregation. Furthermore, larval metamorphosis took longer to complete in pond than tap water without any difference in body length or mass. These findings provide fundamental insight into the impact of the aquatic environment and the effect of the group size on animal health and conservation.
Introduction
Wildlife disease management is an important global issue. Two-thirds of mammals, birds, reptiles, and fish, and amphibians face global declines, driven by habitat loss and infectious diseases (WWF, 2020). Local extinctions of wild animals alter ecosystems; this is linked to human activity, animal health, and interactions with microorganisms (Fisher et al., 2012; Rabinowitz and Conti, 2013; Mills et al., 2019; Ode Sang et al., 2022).
In amphibians, fungal pathogens can cause disease-driven extinction; for example, Batrachochytrium dendrobatidis has caused or threatened the local extinctions of more than 200 species (Daszak et al., 1999; Skerratt et al., 2007). This has encouraged research on disease management and microorganism diversity in amphibians (Harris et al., 2009; Mcfall-Ngai et al., 2013; Walke et al., 2014; Bataille et al., 2016; Rebollar et al., 2016; Walke and Belden, 2016; Bletz et al., 2017; Jani et al., 2021), where the composition of microbial communities is linked to host resistance to fungal pathogens (Harris et al., 2009; Becker et al., 2015; Rebollar et al., 2016; Bates et al., 2018). Symbiotic bacteria vary by host species, host age, and site (Kueneman et al., 2014; Walke et al., 2014; Hernández-Gómez et al., 2017; Jiménez and Sommer, 2017). In addition, temperature, humidity, water characteristics, and landscape influence host–microbiota interactions, i.e., the spread of fungal pathogens is related to both biotic and abiotic factors (Beyer et al., 2015; Krynak et al., 2016; Bernardo-Cravo et al., 2020). Environmental well-being is important for wild animal health. The aquatic environment is critical for the growth of aquatic organisms, such as amphibian larvae. Moreover, ponds are crucial for aquatic biodiversity, and urban ponds can maintain the same level of biodiversity as rural ponds (Hill et al., 2017). Finally, managing urban ponds is essential for public health (Lambert and Donihue, 2020) and studying amphibians is necessary to understand pond environments (Menetrey et al., 2005).
The larvae of the Japanese common toad, Bufo japonicus Temminck and Schlegel, 1838, exhibit a strong schooling tendency, often aggregating cohesively (Wells, 2010). Aggregation is beneficial in the presence of predators (Watt et al., 1997), whereas it has costs when infectious diseases occur because it prevents social distancing (Sandel et al., 2020; Stockmaier et al., 2021). Generally, grouping increases the risk of transmission of infectious disease through social interactions (Kappeler et al., 2015; Pinter-Wollman et al., 2018; Stockmaier et al., 2021). Furthermore, highly transmissible pathogens can spread more easily, and over longer periods, in gregarious than socially hierarchical species (Sah et al., 2018).
Ponds that are home to B. japonicus larvae contain many microorganisms, some of which are pathogenic. This supports the hypothesis that growing in pond water would be more costly for larvae than growing in water without microorganisms. The risk of mortality due to infection may also be greater for larger than smaller groups. To test this, I conducted a rearing experiment using three different group sizes of B. japonicus larvae from an urban pond, Ichiniro-ike (at The University of Tokyo). The number of B. japonicus in the Ichiniro pond population has decreased—10 years ago, 120 mating pairs were observed (Hase and Shimada, 2014), compared to fewer than 10 in the last few years (Hase, personal observation). I investigated the influence of water environment and group size on the decline in this local population. In addition, to ascertain whether the genetic background contributed to differences in larval viability, I proceeded with population genetic analyses using the major histocompatibility complex (MHC) class II gene (as an assessment for the immunity against pathogens) and microsatellite loci (as a neutral marker).
Methods
Rearing Experiment
The rearing experiment was conducted using B. japonicus larvae from a field-captured egg string laid by one female in Ichiniro-ike at The University of Tokyo in February, 2021. Only one egg string was available at the time of sampling, which limited replication to the rearing experiment of the present study. Two types of water were used for rearing: dechlorinated tap water and pond water. Figure 1 shows the rearing experiment. To measure the effect of group size in a limited sample size, I focused on three levels: 1 (solitary, no interaction), 2 (pair, smallest size in which interaction can occur), and 15 (large enough to observe group effects). Bufo japonicus larvae were raised in three groups at 18°C with a 12:12 h dark:light cycle. Larval development was monitored for 50 days: survival, hindlimb emergence [developmental stage 38 (Gosner, 1960)], forelimb emergence [stage 42 (Gosner, 1960)], and completion of metamorphosis [i.e., toadlet, stage 46 (Gosner, 1960)] were recorded. Finally, the snout-vent lengths (hereafter, SVL) and body masses of the toadlets were measured. The daily records and developmental data on the reared larvae are listed in Supplementary Tables 1, 2, respectively.
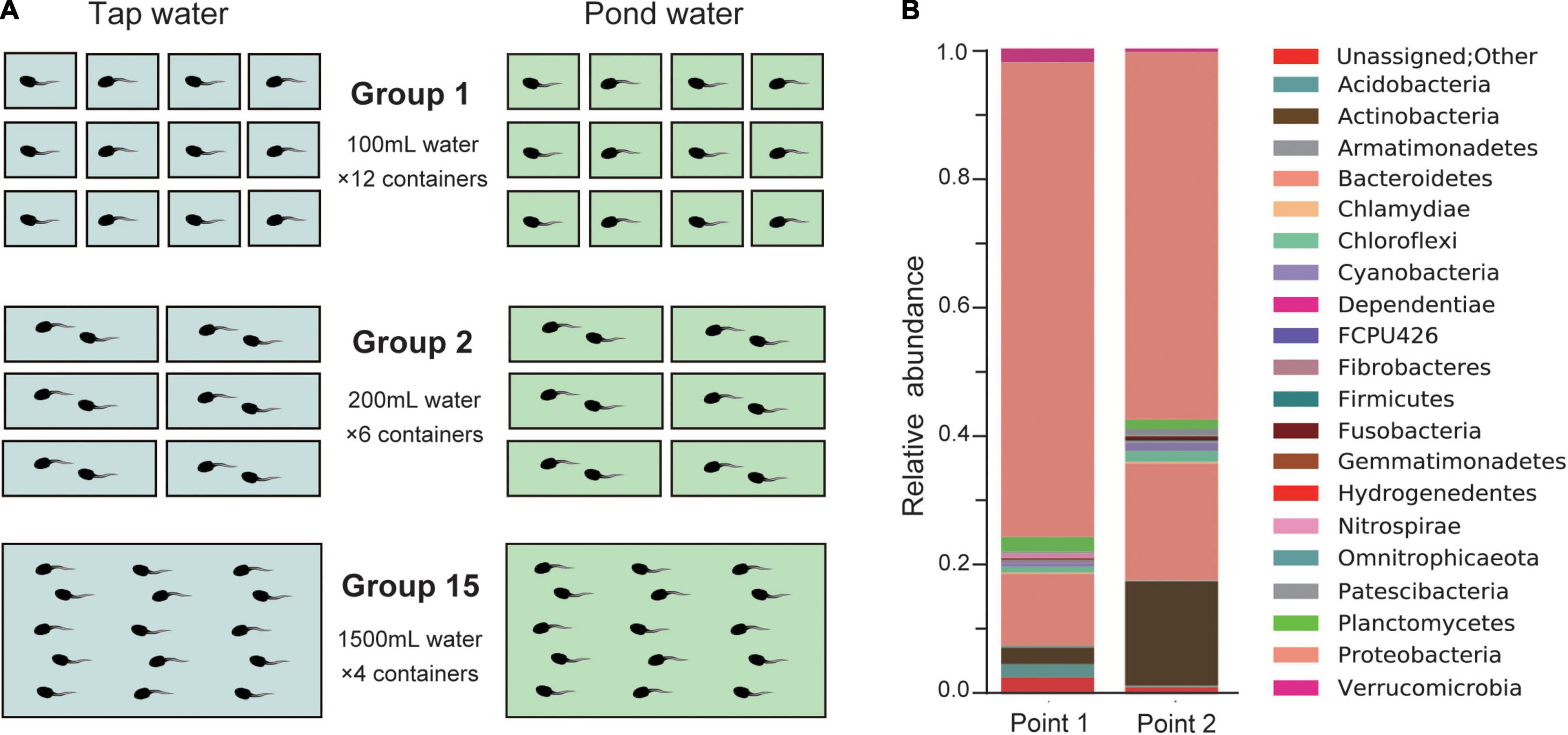
Figure 1. (A) Diagram of the rearing experiment. Bufo japonicus larvae reared in three group sizes, 1, 2, and 15 were prepared. The length, width, and height (mm) of the plastic containers were 80 × 80 × 53, 80 × 156 × 53, and 156 × 235 × 83, respectively. Before use, tap water was dechlorinated, and pond water was filtered through a polyethylene net (100 mesh). The amount of water per larva was kept the same at 100 mL, and the water was changed every 2 days in all containers simultaneously. When the larvae began to swim [stage 25 (Gosner, 1960)], I started feeding them with food pellets (PLECO; Kyorin, Inc., Ltd., Hyogo, Japan). After the forelimbs grew out, the amount of water was reduced by half, and the container was slanted to make space for the toadlets to land. (B) Relative abundances of bacterial taxonomy at phylum level of Ichiniro pond water. Points 1 and 2 correspond to the rearing water’s sampling location at Ichiniro-ike (Supplementary Figure 1).
Assessment for Water Characteristics
The chemical composition of the tap and pond water was evaluated using a water quality test (Supplementary Table 3). The microflora of pond water was analyzed by 16S-rRNA gene-targeted amplicon sequencing. I have prepared two samples of 2 L of environmental water collected from the Ichiniro-ike (Supplementary Figure 1) and filtered through membranes (0.22 μm, MF-Millipore™). The water samples underwent Next generation sequencing of 16S ribosomal RNA gene PCR amplicons (16S-rRNA gene-targeted amplicon sequencing) at FASMAC Co., Ltd. (Kanagawa, Japan). Bacterial taxonomy (phylum/division) was analyzed using QIIME [ver. 1.9.0, (Caporaso et al., 2010)] based on the representative sequence of operational taxonomic units formed by 97% homology. The result of bacteria taxonomy analysis at the phylum level is shown in Figure 1B.
DNA Extract and Genotyping
For dead individuals of the rearing experiment, total DNA content was extracted from the tail-tips (2–3 mm) using DNeasy Blood & Tissue Kits (Qiagen, Inc., Valencia, CA, United States) following the product protocol. For the alive toadlets, toe tips (approximately 1 mm) were left to digest overnight in ethylenediaminetetraacetic acid–sodium dodecyl sulfate (EDTA-SDS) solution (0.3% SDS, 400 mmol/L NaCl, 5 mmol/L EDTA, 20 mmol/L Tris–HCl, pH 8.0) containing 200 μg/mL proteinase K at 55°C.
To evaluate whether the genetic background of immunity links to the survivorship, MHC class II antigen (partial exon) polymorphism of the reared larvae was ascertained. MHC allele sequences were amplified by PCR using locus-specific two primers from previous study (May et al., 2011; Bataille et al., 2015), 2F347 (5′-GTGACCCTCTGCTCTCCATT-3′) and 2R307b (5′-ATAATTCAGTATATACAGGGTCTCACC-3′), and a newly developed primer for Bufo japonicus, R_BjMHCII (CCATAGTTG TRTTTACAGWATSTCTCC). PCR was performed according to the product protocol using KAPA2G Fast HotStart Ready Mix (Kapa Biosystems, Inc., Wilmington, MA, United States) to obtain amplified fragments. Sequencing was performed with a newly developed primer, F_BjMHCII (CCTCTGCTCTCCATTACAGATGC), located inside of the fragment. For neutral genetic markers, I selected four microsatellite loci regarding previous studies: Bbufu23, Bbufu39, Bbufu62 (Brede et al., 2001), and Bjap14 (Hase et al., 2013). Following a method described previously (Hase et al., 2013), the loci were amplified with two multiplex reactions using a KAPA2G Fast Multiplex PCR Kits (NIPPON Genetics Co., Ltd.) according to the manufacturer’s protocol. The sequencing and fragment size analysis of the PCR products were conducted by 3130xl Genetic Analyzer. In addition, I tried to check for infection with Batrachochytrium dendrobatidis of the larvae, referring to the previous study (Goka et al., 2009), but no chytrid presence was detected. All genotype data for 72 individuals are available in Supplementary Table 4, and sequences of detected unique MHC alleles are deposited in GenBank (accession number: OM994394–OM994396). Results of the phylogenetic relationship for MHC alleles and genetic clustering for the genotype of microsatellite loci are shown in Supplementary Figure 2.
Data Analysis
To evaluate survival in each group, I created Kaplan–Meier survival curves between tap and pond water using log-rank tests. The effect on development was investigated by applying a general linear mixed model (GLMM) with a binomial error distribution. Survival days, days to hindlimb emergence, forelimb emergence, and metamorphosis (toadlet) were used as response variables. Group size was used as a random variable, while water type was a fixed explanatory variable. SVL and body mass were evaluated using a GLMM with Gaussian distribution and were used as response variables. The statistical analysis was performed using R 4.1.2 (R Core Team, 2021). The population genetic analyses between dead and alive individuals were carried out based on the MHC class II exon and four microsatellite loci by the program GenALEx v. 6 (Peakall and Smouse, 2006).
Results
Table 1 shows the results of the rearing experiment. Water quality was significantly related to differences in larval survival: B. japonicus larvae were alive better when raised in tap water than in pond water (z = 19.37, p < 0.001). However, Kaplan-Meier estimator, the group size effect was evident only for group 15: the results of the log-rank test for group sizes 1, 2, and 15 were p = 0.8, p = 0.8, and p < 0.001, respectively (Figures 2A–C). The development rate was significantly lower in pond water (days to hindlimb, z = −2.38, p = 0.017; forelimb, z = −3.83, p < 0.001; toadlet, z = −5.07, p < 0.001; Figure 2D). Neither the SVL nor the body mass of the toadlets differed according to water type (SVL, df = 107.17, t = −1.20, p = 0.23; mass, df = 103.42, t = −1.903, p = 0.06; Figures 2E,F).
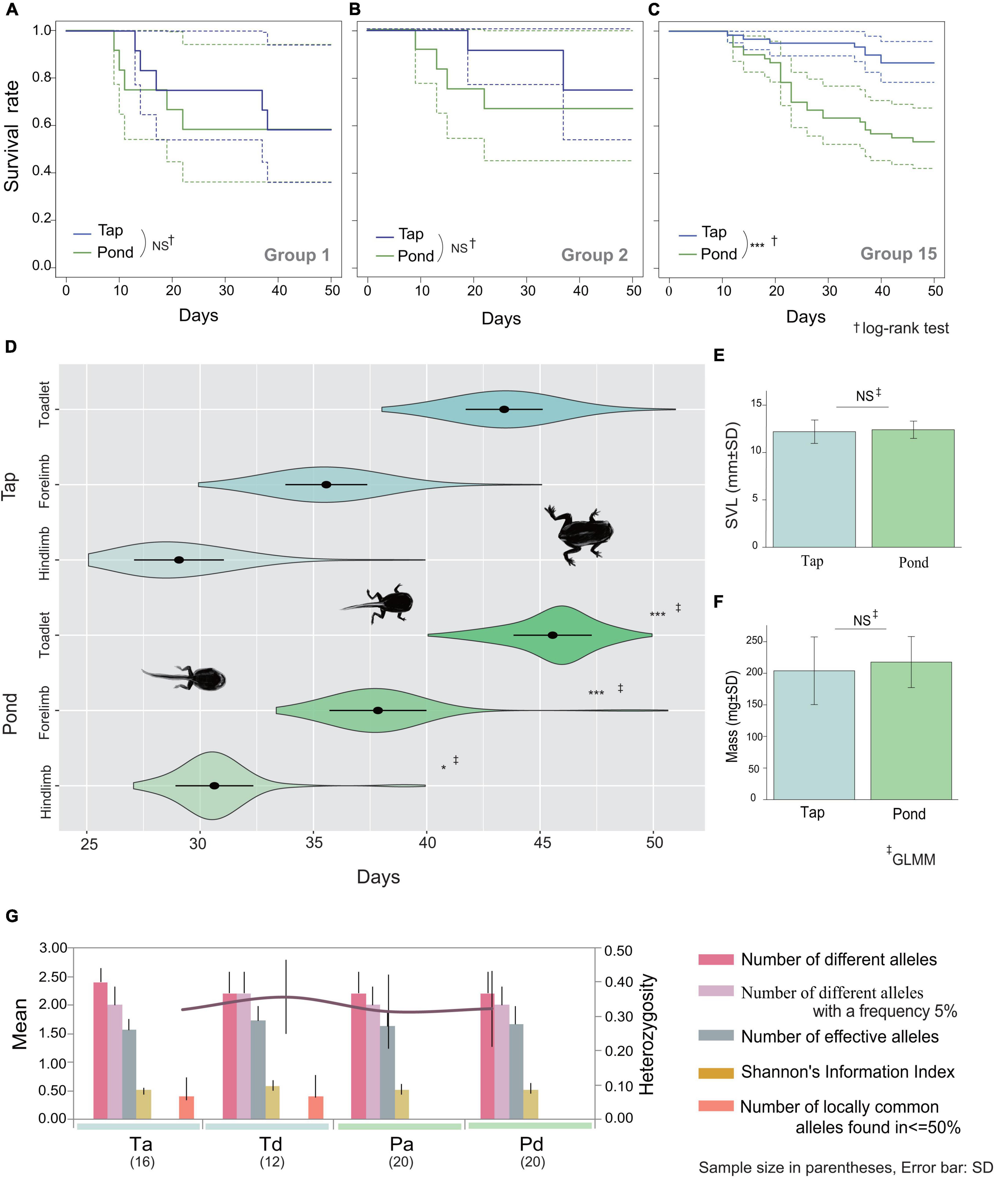
Figure 2. Kaplan-Meier Curves of rearing tadpoles over 50 days for group sizes 1 (A), 2 (B), and 15 (C). Dashed lines represent 95% confidence intervals. (D) Violin plot with mean and standard deviation for the days taken to develop between tap (N = 72) and pond (N = 47) waters, from left to right, hindlimbs emergence, forelimbs emergence, and completion of metamorphosis (toadlets). SVL (E) and mass (F) of toadlets. (G) Allelic patterns by category: Ta, tap water and alive; Td, tap water and dead; Pa, pond water and alive; Pd, pond water and dead. NS, not significant; *p < 0.05; ***p < 0.001. Detailed information on allele frequency is presented in Supplementary Table 5.
There was no association between genotype and the results of the rearing experiment (Figure 2G). The unique MHC alleles were not explicitly relevant to larval survival and the microsatellite genotype did not explain the differences according to viability and water type (Figure 2G, Supplementary Figure 2, and Supplementary Table 5).
Discussion
Bufo japonicus larvae reared in pond water were more likely to die and developed more slowly than their siblings in tap water (Table 1). Large groups of tadpoles in pond water were at greater risk of death (Figures 2A–C). Changes in larval growth depended only on the water conditions (Figure 2D); no distinctive genotypes were detected in the individuals that died (Figure 2G). Two factors likely contributed to these results.
First, the pond water did not inherently favor the development of the larvae. Ichiniro pond water contains abundant microflora, particularly Proteobacteria and Bacteroidetes (Figure 1B). Some deaths may be attributable to the same disease because deaths in the group 15 often occurred in succession, suggesting them to be infection-related (Figure 2C and Supplementary Table 1). Twenty-one bacterial taxa were detected (Figure 1B); even if bacteria are linked to the cause of death, the bacteria responsible are likely to vary among groups, containers, and individuals. A wide variety of causes of death would also make it even more difficult to assess the association with genetic background, especially in the immune system associated locus, i.e., MHC genes. In addition, microflora can alter the chemical composition of the water (Garcia-Ochoa and Gomez, 2009; Garcia-Ochoa et al., 2010). The water quality testing showed that the biological oxygen demand of pond water was three times higher than that of tap water (Supplementary Table 3), which indicates the possibility of microbial modification. Deterioration of the quality of the pond water would delay the development of larvae.
Second, a local population decline could debilitate larvae. The number of B. japonicus in Ichiniro pond is decreasing rapidly, and at the time of sampling for this study, only one egg string was found. Therefore, the genetic diversity of toads at this site has decreased. In a previous rearing experiment using tap water conducted at the same site, the larvae had a higher survival rate [99% (Hase et al., 2022)] than in the present study (81%; Table 1). Even in the absence of pathogenic microorganisms, larval viability has decreased. Genetic deterioration is likely occurring in B. japonicus in this area due to the rapid population decline (Willi et al., 2006).
More research is needed to reveal the link between environmental microflora and animal health. Under optimal conditions, animals grown with symbiotic bacteria are protected from pathogens (Sommer and Bäckhed, 2013). Even in animal taxa that are not highly hierarchical, disease management efforts should consider behavioral traits such as grouping.
Data Availability Statement
The datasets presented in this study can be found in online repositories. The names of the repository/repositories and accession number(s) can be found below: NCBI (accession: OM994394–OM994396).
Ethics Statement
All tadpoles were treated carefully to minimise physical stress, in compliance with the ethical guidelines of the institute. After genotyping, all toadlets were released into their field near the home pond.
Author Contributions
KH designed the research, conducted the experiments, ran the analysis, and prepared the manuscript.
Funding
This project was supported by a Grant-in-Aid from the Japan Society for the Promotion of Science (JSPS) Research Fellowship (No. 19K16226).
Conflict of Interest
The author declares that the research was conducted in the absence of any commercial or financial relationships that could be construed as a potential conflict of interest.
Publisher’s Note
All claims expressed in this article are solely those of the authors and do not necessarily represent those of their affiliated organizations, or those of the publisher, the editors and the reviewers. Any product that may be evaluated in this article, or claim that may be made by its manufacturer, is not guaranteed or endorsed by the publisher.
Acknowledgments
The author appreciate Dr. B. Waldman of Oklahoma State University for helpful suggestions for the draft manuscript. The author sincerely thank Dr. A. Matsushita and T. Kawai of The Graduate University for Advanced Studies (SOKENDAI) for undertaking the water quality test. The author also appreciate Dr. Y. Terai of SOKENDAI for advising on the experiment and Dr. S. Kubota of FASMAC Co., Ltd., for proceeding with 16S-rRNA gene-targeted amplicon sequencing.
Supplementary Material
The Supplementary Material for this article can be found online at: https://www.frontiersin.org/articles/10.3389/fevo.2022.917067/full#supplementary-material
References
Bataille, A., Cashins, S. D., Grogan, L., Skerratt, L. F., Hunter, D., McFadden, M., et al. (2015). Susceptibility of amphibians to chytridiomycosis is associated with MHC class II conformation. Proc. Biol. Sci. 282:20143127. doi: 10.1098/RSPB.2014.3127
Bataille, A., Lee-Cruz, L., Tripathi, B., Kim, H., and Waldman, B. (2016). Microbiome variation across amphibian skin regions: implications for chytridiomycosis mitigation efforts. Microb. Ecol. 71, 221–232. doi: 10.1007/s00248-015-0653-0
Bates, K. A., Clare, F. C., O’Hanlon, S., Bosch, J., Brookes, L., Hopkins, K., et al. (2018). Amphibian chytridiomycosis outbreak dynamics are linked with host skin bacterial community structure. Nat. Commun. 9:693. doi: 10.1038/s41467-018-02967-w
Becker, M. H., Walke, J. B., Cikanek, S., Savage, A. E., Mattheus, N., Santiago, C. N., et al. (2015). Composition of symbiotic bacteria predicts survival in Panamanian golden frogs infected with a lethal fungus. Proc. Biol. Sci. 282:20142881. doi: 10.1098/rspb.2014.2881
Bernardo-Cravo, A. P., Schmeller, D. S., Chatzinotas, A., Vredenburg, V. T., and Loyau, A. (2020). Environmental factors and host microbiomes shape host–pathogen dynamics. Trends Parasitol. 36, 616–633. doi: 10.1016/J.PT.2020.04.010
Beyer, S. E., Phillips, C. A., and Schooley, R. L. (2015). Canopy cover and drought influence the landscape epidemiology of an amphibian chytrid fungus. Ecosphere 6:art78. doi: 10.1890/ES14-00263.1
Bletz, M. C., Perl, R. G. B., Bobowski, B. T. C., Japke, L. M., Tebbe, C. C., Dohrmann, A. B., et al. (2017). Amphibian skin microbiota exhibits temporal variation in community structure but stability of predicted Bd-inhibitory function. ISME J. 11, 1521–1534. doi: 10.1038/ismej.2017.41
Brede, E. G., Rowe, G., Trojanowski, J., and Beebee, T. J. C. (2001). Polymerase chain reaction primers for microsatellite loci in the common toad Bufo bufo. Mol. Ecol. Notes 1, 308–310. doi: 10.1046/J.1471-8278.2001.00120.X
Caporaso, J. G., Kuczynski, J., Stombaugh, J., Bittinger, K., Bushman, F. D., Costello, E. K., et al. (2010). QIIME allows analysis of high-throughput community sequencing data. Nat. Methods 7:335. doi: 10.1038/NMETH.F.303
R Core Team (2021). R: A Language and Environment for Statistical Computing. Vienna: R Foundation for Statistical Computing.
Daszak, P., Berger, L., Cunningham, A. A., Hyatt, A. D., Earl Green, D., and Speare, R. (1999). Emerging infectious diseases and amphibian population declines. Emerg. Infect. Dis. 5:735. doi: 10.3201/eid0506.990601
Fisher, M. C., Henk, D. A., Briggs, C. J., Brownstein, J. S., Madoff, L. C., McCraw, S. L., et al. (2012). Emerging fungal threats to animal, plant and ecosystem health. Nature 484, 186–194. doi: 10.1038/nature10947
Garcia-Ochoa, F., and Gomez, E. (2009). Bioreactor scale-up and oxygen transfer rate in microbial processes: an overview. Biotechnol. Adv. 27, 153–176. doi: 10.1016/J.BIOTECHADV.2008.10.006
Garcia-Ochoa, F., Gomez, E., Santos, V. E., and Merchuk, J. C. (2010). Oxygen uptake rate in microbial processes: an overview. Biochem. Eng. J. 49, 289–307. doi: 10.1016/J.BEJ.2010.01.011
Goka, K., Yokoyama, J., Une, Y., Kuroki, T., Suzuki, K., Nakahara, M., et al. (2009). Amphibian chytridiomycosis in Japan: distribution, haplotypes and possible route of entry into Japan. Mol. Ecol. 18, 4757–4774. doi: 10.1111/J.1365-294X.2009.04384.X
Gosner, K. L. (1960). A simplified table for staging anuran embryos and larvae with notes on identification. Herpetologica 16, 183–190.
Harris, R. N., Brucker, R. M., Walke, J. B., Becker, M. H., Schwantes, C. R., Flaherty, D. C., et al. (2009). Skin microbes on frogs prevent morbidity and mortality caused by a lethal skin fungus subject category: microbe-microbe and microbe-host interactions. ISME J. 3, 818–824. doi: 10.1038/ismej.2009.27
Hase, K., and Shimada, M. (2014). Female polyandry and size-assortative mating in isolated local populations of the Japanese common toad Bufo japonicus. Biol. J. Linn. Soc. 113, 236–242.
Hase, K., Nikoh, N., and Shimada, M. (2013). Population admixture and high larval viability among urban toads. Ecol. Evol. 3, 1677–1691. doi: 10.1002/ece3.578
Hase, K., Nikoh, N., and Shimada, M. (2022). Data from: population admixture and high larval viability among urban toads. Dryad Dataset doi: 10.5061/dryad.67r45
Hernández-Gómez, O., Hoverman, J. T., and Williams, R. N. (2017). Cutaneous microbial community variation across populations of eastern hellbenders (Cryptobranchus alleganiensis alleganiensis). Front. Microbiol. 8:1379. doi: 10.3389/fmicb.2017.01379
Hill, M. J., Biggs, J., Thornhill, I., Briers, R. A., Gledhill, D. G., White, J. C., et al. (2017). Urban ponds as an aquatic biodiversity resource in modified landscapes. Glob. Chang Biol. 23, 986–999. doi: 10.1111/gcb.13401
Jani, A. J., Bushell, J., Arisdakessian, C. G., Belcaid, M., Boiano, D. M., Brown, C., et al. (2021). The amphibian microbiome exhibits poor resilience following pathogen-induced disturbance. ISME J. 15, 1628–1640. doi: 10.1038/s41396-020-00875-w
Jiménez, R. R., and Sommer, S. (2017). The amphibian microbiome: natural range of variation, pathogenic dysbiosis, and role in conservation. Biodivers. Conserv. 26, 763–786. doi: 10.1007/s10531-016-1272-x
Kappeler, P. M., Cremer, S., and Nunn, C. L. (2015). Sociality and health: impacts of sociality on disease susceptibility and transmission in animal and human societies. Philos. Trans. R. Soc. B 370:20140116. doi: 10.1098/RSTB.2014.0116
Krynak, K. L., Burke, D. J., and Benard, M. F. (2016). Landscape and water characteristics correlate with immune defense traits across Blanchard’s cricket frog (Acris blanchardi) populations. Biol. Conserv. 193, 153–167. doi: 10.1016/j.biocon.2015.11.019
Kueneman, J. G., Parfrey, L. W., Woodhams, D. C., Archer, H. M., Knight, R., and McKenzie, V. J. (2014). The amphibian skin-associated microbiome across species, space and life history stages. Mol. Ecol. 23, 1238–1250. doi: 10.1111/mec.12510
Lambert, M. R., and Donihue, C. M. (2020). Urban biodiversity management using evolutionary tools. Nat. Ecol. Evol. 4, 903–910. doi: 10.1038/s41559-020-1193-7
May, S., Zeisset, I., and Beebee, T. J. C. (2011). Larval fitness and immunogenetic diversity in chytrid-infected and uninfected natterjack toad (Bufo calamita) populations. Conserv. Genet. 12, 805–811. doi: 10.1007/s10592-011-0187-z
Mcfall-Ngai, M., Hadfield, M. G., Bosch, T. C. G., Carey, H. V., Domazet-Lo, T., Douglas, A. E., et al. (2013). Animals in a bacterial world, a new imperative for the life sciences. PNAS 110, 3229–3236. doi: 10.1073/pnas.1218525110/-/DCSupplemental
Menetrey, N. L., Sager, L., Oertli, B., and Lachavanne, J.-B. (2005). Looking for metrics to assess the trophic state of ponds. Macroinvertebrates and amphibians. Aquat. Conserv. 15, 653–664. doi: 10.1002/aqc.746
Mills, J. G., Brookes, J. D., Gellie, N. J. C., Liddicoat, C., Lowe, A. J., Sydnor, H. R., et al. (2019). Relating urban biodiversity to human health with the “Holobiont” concept. Front. Microbiol. 10:550. doi: 10.3389/FMICB.2019.00550/BIBTEX
Ode Sang, Å, Thorpert, P., and Fransson, A.-M. (2022). Planning, design and managing green roofs and green walls for public health – an ecosystem service approach. Front. Ecol. Evol. 10:336. doi: 10.3389/FEVO.2022.804500
Peakall, R., and Smouse, P. (2006). GenALEX 6: genetic analysis in excel. Population genetic software for teaching and research. Mol. Ecol. Notes 6, 288–295. doi: 10.1111/j.1471-8286.2005.01155.x
Pinter-Wollman, N., Jelic, A., and Wells, N. M. (2018). The impact of the built environment on health behaviours and disease transmission in social systems. Philos. Trans. R. Soc. Lond. B Biol. Sci. 373:20170245. doi: 10.1098/RSTB.2017.0245
Rabinowitz, P., and Conti, L. (2013). Links among human health, animal health, and ecosystem health. Annu. Rev. Public Health 34, 189–204. doi: 10.1146/annurev-publhealth-031912-114426
Rebollar, E. A., Hughey, M. C., Medina, D., Harris, R. N., Ibáñez, R., and Belden, L. K. (2016). Skin bacterial diversity of Panamanian frogs is associated with host susceptibility and presence of Batrachochytrium dendrobatidis. ISME J. 10, 1682–1695. doi: 10.1038/ismej.2015.234
Sah, P., Mann, J., and Bansal, S. (2018). Disease implications of animal social network structure: a synthesis across social systems. J. Anim. Ecol. 87, 546–558. doi: 10.1111/1365-2656.12786
Sandel, A. A., Rushmore, J., Negrey, J. D., Mitani, J. C., Lyons, D. M., and Caillaud, D. (2020). Social network predicts exposure to respiratory infection in a wild chimpanzee group. Ecohealth 17, 437–448. doi: 10.1007/S10393-020-01507-7/FIGURES/4
Skerratt, L. F., Berger, L., Speare, R., Cashins, S., McDonald, K. R., Phillott, A. D., et al. (2007). Spread of chytridiomycosis has caused the rapid global decline and extinction of frogs. Ecohealth 4, 125–134. doi: 10.1007/s10393-007-0093-5
Sommer, F., and Bäckhed, F. (2013). The gut microbiota-masters of host development and physiology. Nat. Rev. Microbiol. 11, 227–238. doi: 10.1038/nrmicro2974
Stockmaier, S., Stroeymeyt, N., Shattuck, E. C., Hawley, D. M., Meyers, L. A., and Bolnick, D. I. (2021). Infectious diseases and social distancing in nature. Science 371:eabc8881. doi: 10.1126/science.abc8881
Walke, J. B., and Belden, L. K. (2016). Harnessing the microbiome to prevent fungal infections: lessons from amphibians. PLoS Pathog. 12:e1005796. doi: 10.1371/JOURNAL.PPAT.1005796
Walke, J. B., Becker, M. H., Loftus, S. C., House, L. L., Cormier, G., Jensen, R. V., et al. (2014). Amphibian skin may select for rare environmental microbes. ISME J. 8, 2207–2217. doi: 10.1038/ismej.2014.77
Watt, P. J., Nottingham, S. F., and Young, S. (1997). Toad tadpole aggregation behaviour: evidence for a predator avoidance function. Anim. Behav. 54, 865–872. doi: 10.1006/ANBE.1996.0512
Wells, K. D. (2010). The Ecology and Behavior of Amphibians. Chicago, IL: University of Chicago press, 557–598.
Willi, Y., van Buskirk, J., and Hoffmann, A. A. (2006). Limits to the adaptive potential of small populations. Annu. Rev. Ecol. Evol. Syst. 37, 433–458. doi: 10.1146/ANNUREV.ECOLSYS.37.091305.110145
Keywords: amphibian larvae, infection disease, population decline, major histocompatibility complex (MHC), urban pond, wild animal health, Bufo japonicus, group size
Citation: Hase K (2022) Microflora Influence: The Aquatic Environment Changes Grouping Risk and Development Speed of Toad Tadpoles. Front. Ecol. Evol. 10:917067. doi: 10.3389/fevo.2022.917067
Received: 10 April 2022; Accepted: 30 May 2022;
Published: 22 June 2022.
Edited by:
Steven Petersen, Brigham Young University, United StatesReviewed by:
Jessica Wooten, Piedmont University, United StatesPaul Székely, Universidad Técnica Particular de Loja, Ecuador
Copyright © 2022 Hase. This is an open-access article distributed under the terms of the Creative Commons Attribution License (CC BY). The use, distribution or reproduction in other forums is permitted, provided the original author(s) and the copyright owner(s) are credited and that the original publication in this journal is cited, in accordance with accepted academic practice. No use, distribution or reproduction is permitted which does not comply with these terms.
*Correspondence: Kazuko Hase, aGFzZWthenVrb0BnbWFpbC5jb20=