- 1Anhui Key Laboratory of Resource and Plant Biology, School of Life Science, Huaibei Normal University, Huaibei, China
- 2East China Institute of Forest Inventory and Planning, State Forestry Administration, Hangzhou, China
- 3Anhui Province Key Laboratory of Wetland Ecosystem Protection and Restoration, Anhui University, Hefei, China
Ecological shifts (e.g., eutrophication) can affect the genetic differentiation of zooplankton populations in lakes. However, the role of environmental change in a lasting high-phosphorus lake driving the genetic differentiation of zooplankton population structure over time is poorly understood. In this paper, the changes of the genetic diversity and differentiation of Daphnia sinensis population were studied by using the mitochondrial COI gene and microsatellite markers on modern groups (from January to June 2016) and historic groups (obtained from resting eggs in the sediments) in Lake Chaohu. Based on the microsatellite markers, six modern groups were clustered into two clusters (the WG cluster and SG cluster) during the seasonal dynamics, whereas the genetic differentiation of the five historic groups showed a wave-like pattern and had evolved into four clusters. Moreover, the haplotype network showed that six modern groups had one origin center whereas five historic groups had two origin centers based on the mitochondrial COI gene marker. Fu’s Fs neutral test and Tajima’s test indicated that the five historic groups deviated from neutral evolution and showed a bottleneck effect in the history process. Water temperature and total dissolved phosphorus were obviously associated with the seasonal genetic differentiation of D. sinensis, whereas nitrogen content of the sediments was significantly related to the long-term microevolution of D. sinensis in the high-phosphorus environment. Therefore, the changing pattern of D. sinensis population genetic structure was one of the environmental selections probably combined with co-evolutionary, where rapid-increasing nitrogen level had a large impact on D. sinensis population genetic structure in lasting high phosphorus environment in Lake Chaohu.
Introduction
Understanding evolutionary responses in aquatic organisms to long-term environment change is a challenge in contemporary biology (Frisch et al., 2014). The main reason for the challenges is the lack of objective conditions and techniques for continuous and lasting sampling for a single population. Revealing long-term variation of population genetic structure and clarifying the mechanism on population genetic isolation at a specific time scale are difficult due to the seasonal succession of zooplankton in lakes. Fortunately, some investigations have been reported to reconstruct Daphnia population genetic structure based on genetic data of resting eggs from the sediments (Hairston et al., 1999; Decaestecker et al., 2007; Brede et al., 2009; Orsini et al., 2013a; Möst et al., 2015; Monchamp et al., 2017). In lake ecosystems, the resting eggs of Daphnia are buried in the sediments in chronological order and can remain alive for decades, centuries, or even longer (Brede et al., 2009; Frisch et al., 2014; Monchamp et al., 2017). Similarly, succession processes of lake environments (e.g., nutrient levels) can be also replicated by analyzing the information preserved in the sediments (Yao and Shen, 2003; Li et al., 2005). Direct observation of population genetic variations of the organisms over time, complemented by long-term environmental data, will allow us to validate our current mechanisms understanding on evolutionary responses of natural populations to environmental changes (Brede et al., 2009; Orsini et al., 2013a; Frisch et al., 2014).
Environmental changes (e.g., eutrophication) enhance the evolution of zooplankton populations (Straile and Geller, 1998; Løvik and Kjelliberg, 2003; Stige et al., 2009; Hsieh et al., 2011; Frisch et al., 2017). Excessive phosphorus loading promote significantly the increase of phytoplankton biomass in lakes, and then alter the nutrition of zooplankton, such as the basic aquatic herbivores, Daphnia (Sterner and Elser, 2002). Daphnia are the phosphorus-rich consumers, which are therefore highly sensitive to changes in phosphorus loading (Elser et al., 1988). Jeyasingh and Weider (2007) found that the genetic variations of Daphnia populations were related to phosphorus availability. In Lake Constance, the biomass and community structure of crustacean zooplankton were affected by eutrophication (Straile and Geller, 1998). Frisch et al. (2014) observed that the population differentiation of Daphnia pulicaria was associated with phosphorus accumulation in Lake Minnesota (North America), and ancient population (preserved in the sediments for more than 700 years) had a higher growth rate under low-phosphorus condition and a lower growth rate under high-phosphorus condition whereas modern population exhibited the opposite trend. Similarly, in two European lakes, the changes of total phosphorus were clearly associated with a shift in Daphnia species composition and population structure of evolutionary lineages (Brede et al., 2009). Therefore, in order to adapt habitat environments, the genetic variations of Daphnia could be induced and preserved through resting eggs in lake sediments.
Daphnia species often inhabit freshwater lakes, ponds, and reservoirs, which are affected by environmental factors such as temperature, nutrient availability, competition and predation (Roff et al., 1981; Wellborn et al., 1996; Cottenie et al., 2001). Daphnia species perform cyclic parthenogenesis under good conditions but are concerted into sexual reproduction and produce resting eggs enclosed in the ephippium when the environment conditions become worse, such as lower temperature, larger predation pressure or higher population density. The resting eggs of Daphnia are preserved in lake sediments, thus forming an egg bank. When these resting eggs are suspended in water because of wind wave or other reasons, they could hatch under suitable conditions and form new populations (Hairston and Cáceres, 1996). At present, the phylogeography of Daphnia species is mainly focused on large-scale spatial pattern of modern populations (De Gelas and De Meester, 2005; So et al., 2015; Ma et al., 2016, 2020; Kotov et al., 2021). However, fewer studies are focused on the long-term genetic differentiation of Daphnia species based on the information of resting eggs located in different sediment layers (Brede et al., 2009; Möst et al., 2015; Monchamp et al., 2017).
Lake Chaohu (Anhui province, China) is a large subtropical eutrophic lake located in the middle reaches of the Yangtze River, with a total surface area of 755 km2. Phosphate rocks are abundant in the north shore of the lake, and the exploitation of phosphate rock has led to the long-term phosphorus-rich state in the water since the 1960s (Xie, 2009). It has been reported that the lake water has suffered from eutrophication since the 1970s (Yao and Shen, 2003; Zhang and Kong, 2015). The average total phosphorus and total nitrogen concentrations reached the peak (0.417 and 4.62 mg/L, respectively) in 1995 (Xie, 2009). Although total phosphorus (TP) and total nitrogen (TN) concentrations dropped from 1995 to 2007 because of the control of waste water and sewage draining, the water quality of the lake still maintained a high nutrient level after 2008 (Zhang and Kong, 2015). In 1980–1981, only a few individuals of Daphnia sp. were occasionally observed in Lake Chaohu (Ye, unpublished data). In 2002–2003, 23 species of crustacean zooplankton belonging to 20 genera were recorded in Lake Chaohu, and three Daphnia species (D. sinensis, D. pulex, and D. galeata) dominated in spring, and then disappeared in mid-summer (Deng et al., 2008). In recent years, Daphnia had also dominated in spring and early summer (Li et al., 2015). These long-term changes of Daphnia density were consistent with the ephippia or resting eggs of Daphnia observed in the lake sediments over time (Zhang et al., 2020). Limited information was available on the study of zooplankton community structure in the lake, especially on the genetic diversity and phylogenetics of Daphnia species in a lasting high-phosphorus environment.
In this study, population genetic structure and differentiation of D. sinensis were studied using mitochondrial COI gene and microsatellite markers in Lake Chaohu. We used five historical groups that were hatched from resting eggs preserved in 15-cm sediment layers (long-term) and six modern groups that were collected during January and June of 2016 (short-term). The effects of environmental factors (particularly nutrients and water temperature) on the genetic structure and phylogenetics of D. sinensis population in Lake Chaohu at short-term and long-term scales were discussed. We hypothesized environmental shifts to coincide with the shifts in genetic structure of D. sinensis population in Lake Chaohu, potentially indicating that rapid-increasing nitrogen level could lead to an evolutionary response of D. sinensis in lasting high-phosphorus environment. Additionally, we also proposed that under a lasting high-phosphorus environment, population genetic differentiation should be consistent with the law of neutral mutations and genetic drift.
Materials and methods
Collection and culture of Daphnia sinensis
In 2016, D. sinensis were collected monthly from 12 sampling sites using a 13# plankton net (112 μm) for short-term samples (Figure 1). Additionally, 20 L of mixed water samples were filtered to determine the density of D. sinensis using a 25# plankton net (64 μm) at a 0.5 m or 1 m interval. According to previous literatures (Deng et al., 2008; Li et al., 2015), no D. sinensis individuals were found from July to December in Lake Chaohu. In this study, the individuals collected in each month were defined as a group, with a total of six modern groups. The species was identified under a microscope (Olympus CX31, Japan) according to Jiang and Du (1979) and Benzie (2005). Two hundred and eighty-six individuals were employed to estimate the genetic structure and differentiation of D. sinensis population during January and June (Table 1). Each individual employed in the experiment was cultured monoclonally at (25 ± 1) °C with 12 h light:12 h dark photoperiod (Zhang et al., 2015). Tap water that had been filtered and aerated for 48 h was utilized as the culture medium. Tetradesmus obliquus was used as the food.
For long-term samples, four sediment cores of 24–30 cm in length were collected from the center of Lake Chaohu (6#) using a columnar gravity (Nanjing Institute of Geography and Limnology, Chinese Academy of Sciences) with PVC tubes of 84 mm in diameter in August 2014 (Zhang et al., 2020). The sedimentary core was sectioned through a 1-cm cutting ring and stored in a sealed bag, then taken back to the laboratory and preserved at 4°C.
All 1 cm-thick sections from three sediment cores were used to examine the resting eggs enclosed in the ephippium of D. sinensis. Each 1 cm-thick section was rinsed with aerated tap water and then filtered through a 300 mesh sieve (48 μm). The remaining part was put into a 50 mL beaker. All remains were observed under a microscope (Olympus CX31, Japan), and the ephippia containing resting eggs of D. sinensis were gathered with a glue head dropper and transferred to a 1 mL EB tube at 4°C for preservation. Few of these ephippia containing resting eggs were found in the sedimentary layers of below 15 cm (Zhang et al., 2020). Therefore, the ephippia containing resting eggs in the 0–15 cm sedimentary layers were selected in this study. These resting eggs were, respectively, placed in a 50 mL beaker and hatched in an intelligent incubator (Ningbo Saifu, Ningbo, China) at 25 ± 1°C with illumination period of L:D = 12 h:12 h. Each hatched individual was cultured monoclonally in a 150 mL beaker containing 100 mL culture medium (aerated tap water over 48 h). Tetradesmus obliquus was used as the food. In order to confirm the activity and genetic integrity of resting eggs, hatched individuals rather than resting eggs were used to evaluate the genetic structure and microevolution of D. sinensis. All hatched individuals of D. sinensis at 3 cm interval along the 15 cm sediment layers according to the changes of nutrient contents were regarded as a group (Zhang et al., 2020). In total, five historic groups (namely respectively, Pop1, 1–3 cm; Pop2, 4–6 cm; Pop3, 7–9 cm; Pop4, 10–12 cm; and Pop5, 13–15 cm) were obtained.
All 1 cm-thick sections of one sediment core were dried in the laboratory to measure dating and nutrient contents (Zhang et al., 2020). The dry samples were ground and filtered using a 100-mesh sieve (150 μm). 137Cs and 210Pb were measured by a high purity germanium gamma spectrometer (AMETEK-AMT ORTEC) at State Key Laboratory of Lake Science and Environment, Nanjing Institute of Geography and Limnology, Chinese Academy of Sciences. According to the attenuation law of radioactive isotope, the radioactive dates were calculated using the constant rate of supply (CRS) 210Pb dating model (Chen et al., 2014).
Determination of physical and chemical parameters
For short-term samples, water samples were collected using a 2.5 L modified Patalas’ sampler at 0.5 or 1 m interval from each sampling site. Water temperature (WT) and dissolved oxygen were measured in situ using a portable dissolved oxygen/temperature analyzer (HANNA, Italy). pH was measured with a portable pH/ORP analyzer (HACA, United States), and transparency was measured using a Secchi disk. One liter of water sample mixture was used to determine the nutrient concentrations. In the laboratory, the mixed lake water was filtered with Whatman GF/C filter prior to measuring dissolved total phosphorus (DTP) concentrations. TP and DTP concentrations were measured by colorimetry after the water samples were digested with K2S2O8 to orthophosphate (Ebina et al., 1983). By contrast, TN were analyzed by the UV spectrophotometric method (Huang, 1999) after the water samples were digested with K2S2O8 + NaOH.
Fifty milligram of each 1 cm-thick dried sample was used to measure the TN and TP contents in the sediment using K2S2O8 digestion method (Qian et al., 1990).
DNA extraction and PCR amplification
After starving for 24 h, adult D. sinensis individuals were rinsed with double-distilled water and crushed with a sterile 10 μL tip before extraction. Total DNA of D. sinensis was extracted using the TNAMP Micro DNA Kit (Tiangen Biotech Co., Ltd., Beijing, China).
Fourteen SSR primers were used in this study, including 11 pairs primers developed in the laboratory (NCBI accession number: KY440958, KY440961, KY440963, KY440966, KY440964, KY440968, KY440960, KY440965, KY440959, KY440962, and KY440967; Wu et al., 2019b) and three pairs primers downloaded from NCBI (accession number: AF233360, AF233362, and AY057865). Mitochondrial COI gene was amplified with the COI F (5′-AYCAATCATAAGGACYATTGGRAC-3′) and the COI R (5′-KGTGATWCCNACHGCTCAKAC-3′). The PCR reaction system (25 μL) of COI gene and the PCR reaction system (25 μL) of the SSR were referred to Wu et al. (2019a).
Electrophoresis and sequencing
The PCR amplification products of mitochondrial COI genes were analyzed by gel electrophoresis, purified by Biological Engineering Company (Shanghai, China), and sequenced with forward and reverse primers (GenScript, Nanjing, China). Sequence alignment was conducted using SeqMan software in DNAStar (version 7.1, DNAStar, Madison, United States). According to the peak in SeqMan, the bidirectional sequencing of nucleotide sequence was proofread by DNAstar to delete the unreliable bases at both ends. PCR products were detected by agarose gel electrophoresis and capillary electrophoresis, and then was analyzed by ABI 3730 sequencer (Shanghai Generay Biotech Co., Ltd).
Data analysis
Genetic distances among groups were calculated using the Kimura two-parameter (K-2P) model in MEGA version 4.0 (Tamura et al., 2007). Pairwise FST values among groups were analyzed by DNAsp version 5.0 software (Librado and Rozas, 2009). The haplotype network was drawn using Network 2.3.1, and the differences of nucleic acid sequence among different D. sinensis groups were systematically analyzed (Excoffier and Lischer, 2010). Fu’s Fs test, Tajima’s neutral test, and mismatch distribution were conducted by using Arlequin 3.5 software (Tajima, 1989; Fu, 1997; Excoffier and Lischer, 2010) to detect the historical change of the genetic structure of D. sinensis population in Lake Chaohu.
GeneMarker 2.25 software was used to assess the quality of microsatellite markers by peak diagram. The observed number of alleles, effective number of alleles, Nei’s genetic diversity, Shannon’s diversity index, and percentage of polymorphic loci were calculated based on the transformed peak diagram data using Popgene version 1.31 software (Yeh et al., 1999). Based on the microsatellite markers, the pairwise Fst values were performed by Arlequin 3.5 software to analyze the differences in the genetic diversity of D. sinensis among modern and historic groups, respectively. The population genetic structure of D. sinensis was reconstructed using the 14 polymorphic microsatellite loci in STRUCTURE HARVESTER v0.6094 (http://taylor0.biology.Ucla.edu/structureHarvester/). Posterior likelihood values were calculated from K = 1 to K = 12 (Evanno et al., 2005). The assumed K value of 1–12 was repeated 10 times to confirm the best K value (Supplementary Figure 1). The genetic structure of D. sinensis was analyzed by Structure 2.3.3 software (Pritchard et al., 2000).
The non-metric multidimensional scaling (NMDS) ordination method was used to analyze the discrepancies of genetic diversity indexes based on both mitochondrial COI gene and microsatellite markers among 11 D. sinensis groups according to Euclidean distances in Past 3.0 software (http://folk.Uio.no/ohammer/past/). Principal component analysis (PCA) with CANOCO 5.0 was used to analyze the relationships between environmental factors (e.g., WT, TN, DTP amd TP) and genetic diversity indexes (including Na, Ne, h, I, P, Fis, Hd, π, and D) of D. sinensis in both water and the sediments. The data was transformed into log10 (x + 1) before running the program. SPSS 19.0 software was used to analyze the differences on the genetic diversity between modern and historical groups of D. sinensis by independent sample t-tests.
Results
Environment parameters and population dynamics of Daphnia sinensis in Lake Chaohu
During the sampling period, water temperature showed an increasing trend, with the minimum value (3.1°C) in January and the maximum value (27.6°C) in June. The lake water was alkaline, with a pH range of 7.83 in April to 9.15 in January. The average water depth was 3.17 ± 0.47 m, with the maximum depth (3.96 m) in June and the lowest depth (2.74 m) in February. The concentrations of Chl a ranged from 12.37 μg/L (March) to 70.41 μg/L (June). The average TP and DTP concentration were 0.35 ± 0.29 mg/L and 0.15 ± 0.12 mg/L. The maximum values of both TP and DTP concentrations were 0.82 mg/L and 0.31 mg/L in May. The average TN concentration was 2.28 ± 0.31 mg/L, and it gradually decreased from January (2.22 mg/L) to March (1.77 mg/L) and then raised to its maximum in June (2.68 mg/L; Supplementary Table 1). The values of TN and TP contents in the sediments were from previously published data (Zhang et al., 2020).
The density of D. sinensis in the water showed an increasing trend and then decreasing trend (Figure 2C). The densities of D. sinensis increased from 0.57 ± 0.53 ind./L in January to 2.63 ± 3.29 ind./L in February, and reached the peak (25.51 ± 21.62 ind./L) in March. Then, it remained at higher levels (16.5–20.0 ind./L) from April to June, and disappeared in July, which was consistent with the classification of two clusters based on the microsatellites markers (Figure 2A).
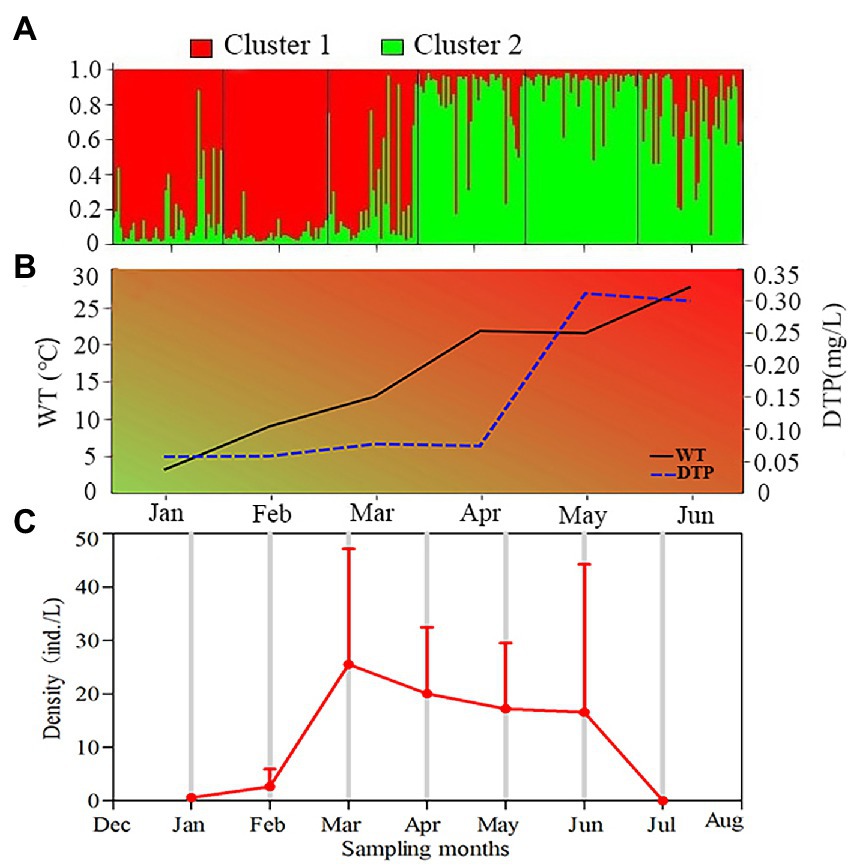
Figure 2. Variations of water temperature, DTP concentration and genetic structure of Daphnia sinensis based on the microsatellite markers during 6 months in Lake Chaohu. (A) The genetic structure of six modern D. sinensis groups. Each individual is represented by a vertical line, and two colors reflect the Bayesian cluster (assignment probability for K = 2). (B) The black line represents water temperature (WT), and the blue dotted line represents dissolved total phosphorus concentration (DTP). The Abbreviations are used for the months in the plot. (C): The temporal distribution of D. sinensis density from January to July 2016.
Genetic diversity and genetic differentiation of the Daphnia sinensis population in Lake Chaohu
Based on the microsatellite markers, the six modern D. sinensis groups were clustered into two groups (Figure 2A). The specimens collected in January, February and March were clustered into the winter and spring group (WG, Cluster 1), whereas the specimens collected in April, May and June were clustered into the spring and summer group (SG, Cluster 2; Figure 2A).
The five historic D. sinensis groups were grouped into four clusters (Figure 3). These historic D. sinensis groups were mainly composed of two dominant clusters (Clusters 1 and 2). Cluster 3 appeared intermittently in different sedimentary depths, indicating that time isolation occurred in this group. Cluster 4 was observed in each sedimentary depth, but it only dominated in the Pop 2 group.
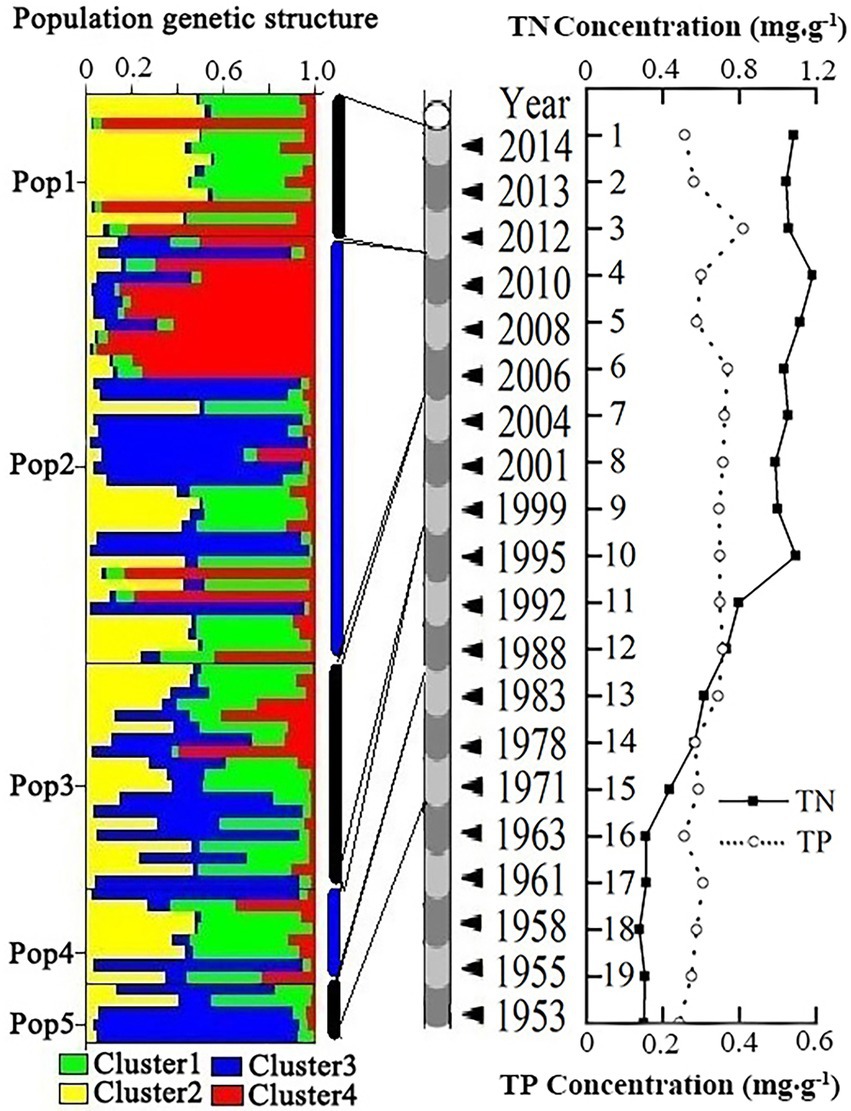
Figure 3. Variations of nutrient contents and genetic structure of five historic D. sinensis groups in the sediments of Lake Chaohu based on the microsatellite markers. Left panel stands for the genetic structure of five historic D. sinensis groups. Each individual is represented by a horizontal line, and four colors reflect the Bayesian cluster (assignment probability for K = 4). Center panel stands for the dated sediment core. Right panel stands for TN and TP contents in the sediments of Lake Chaohu. The age of sediments, and TN and TP contents in the sediments cited from Zhang et al. (2020).
Based on the microsatellite markers, both Na and Ne in lake water were higher than those in the sediment, and there were significant differences (Na: F = 2.387, p < 0.01; Ne: F = 4.787, p < 0.01). The Nei’s genetic diversity and Shannon’s diversity index of six modern D. sinensis groups ranged from 0.129 to 0.141 and from 0.208 to 0.226, respectively, whereas those of the five historic D. sinensis groups ranged from 0.101 to 0.124 and from 0.152 to 0.197, respectively. The genetic diversity indexes (except Fis) of the Pop1 and Pop2 groups located in the surface sedimentary layers were higher than those of the Pop3, Pop4, and Pop5 groups located in the lower sedimentary layers. The peaks of all these indexes appeared in Pop2, except for Fis (Table 1).
Based on the mitochondrial COI gene sequence, the haplotype diversity (Hd) of the six modern D. sinensis was between 0.808 and 0.936, whereas that of the five historic D. sinensis was between 0.667 and 0.973. The nucleotide diversity (π) of these 11 D. sinensis was between 0.002 and 0.009 (Table 1).
Based on all genetic diversity indexes in Table 1, the temporal trends in genetic differentiation among D. sinensis groups of Lake Chaohu were showed in Figure 4. The stress values for all NMDS configurations were lower than 0.08 based on Euclidean distances. For NMDS analysis, D. sinensis groups exhibited significant temporal shifts based on genetic diversity indexes (Global r = 0.595, p = 0.006; Figure 4). There was the highest similarity of genetic diversity indexes in modern D. sinensis groups between May and other months, whereas the genetic diversity indexes of Pop3 and Pop5 groups were significantly different from that of the other three groups. Moreover, the distance of modern D. sinensis group in February was closer with both Pop2 and Pop1 groups in the sediments, indicating that they had higher similarity (Figure 4).
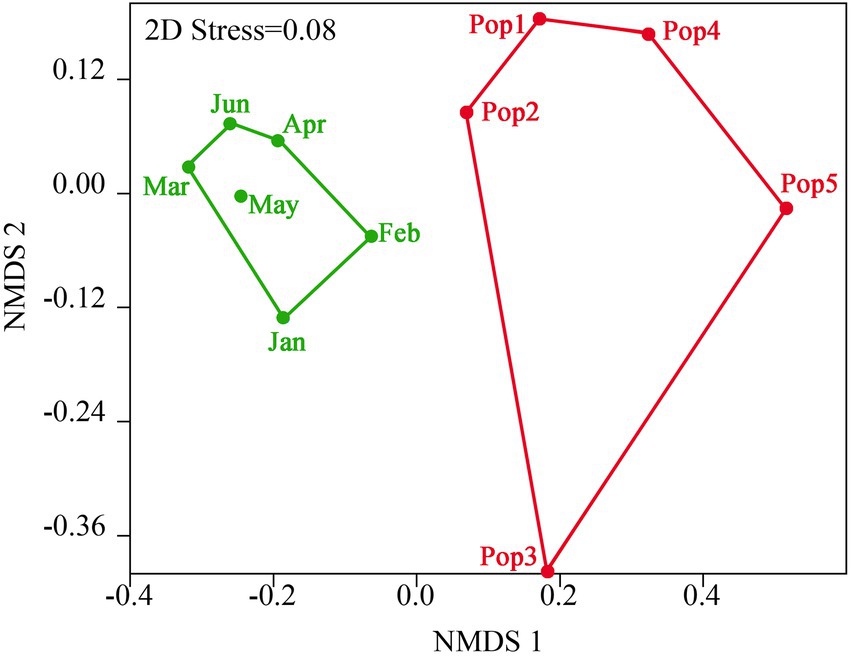
Figure 4. Norrmetric multidimensional scaling (NMDS) represents based on genetic diversity indexes of D. sinensis population in Lake Chaohu. Blue group represents six modern groups during January and June. Red group represents five historic groups in the 1–15 cm sedimentary layers.
Based on the microsatellite markers, the pairwise FST values among the six modern D. sinensis groups ranged from 0.048 to 0.113 (Supplementary Table 2). The minimum Fst value was found between March and April groups (0.048), whereas the maximum (0.113) occurred between February and May groups. The pairwise Fst values of D. sinensis population increased gradually with the season, indicating that the seasonal genetic differentiation of D. sinensis increased with the increase of water temperature (Figure 2B).
Pairwise Fst values among the five historic D. sinensis groups ranged from 0.015 to 0.096 (Supplementary Table 3). Moreover, the pairwise Fst value between Pop3 and Pop4 groups was the smallest (0.015), whereas the maximum (0.096) occurred between Pop1 and Pop5 groups.
Evolution of Daphnia sinensis haplotypes in Lake Chaohu
Twenty-four haplotypes, including 15 specific and nine shared haplotypes, were obtained from the six modern D. sinensis groups. The haplotype network showed one origin (H5) (Figure 5A).
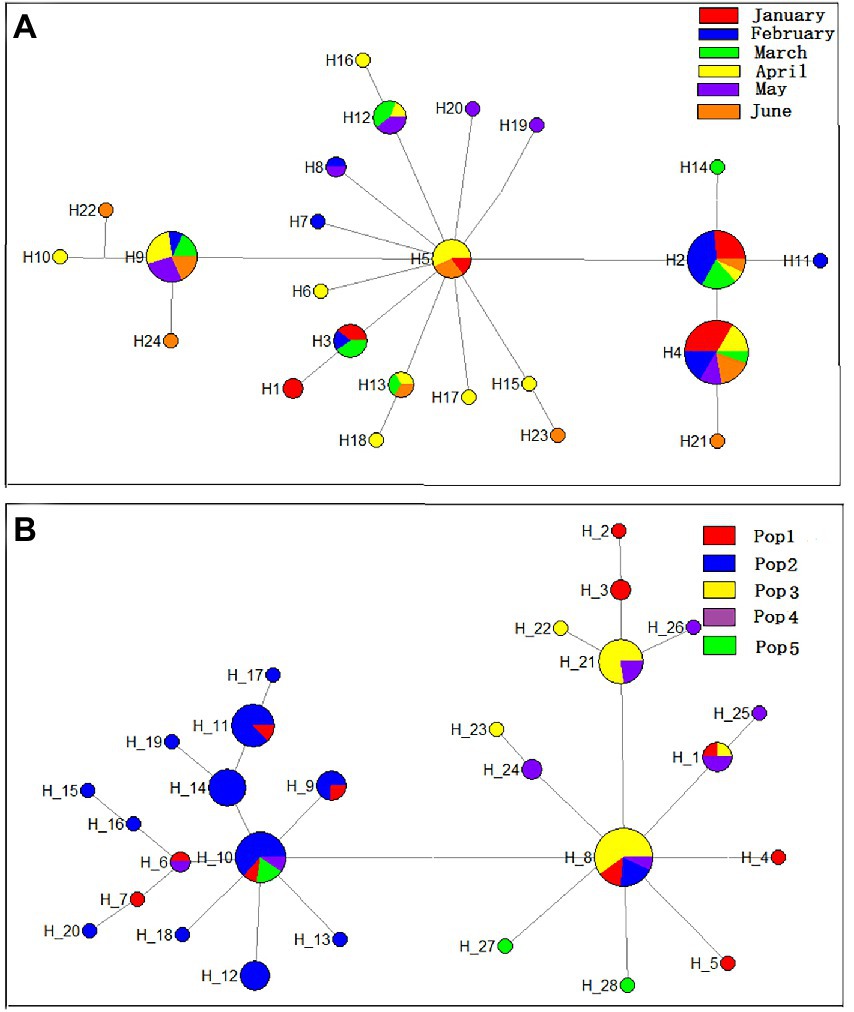
Figure 5. Haplotype networks of six modern (A) and five historic (B) groups of D. sinensis in the sediments of Lake Chaohu based on the mitochondrial COI gene sequence.
Twenty-eight haplotypes, including 19 specific and nine shared haplotypes, were obtained from the five historic D. sinensis groups in Lake Chaohu. The haplotype network showed two origins (Figure 5B). As one of the origin centers, the haplotype H-10 appeared in the most groups. The haplotypes H-14, H-12, and H-6 were mainly distributed in the Pop1 and Pop2 groups. As the other origin center, the haplotype H-8 was mainly distributed in the Pop1, Pop3, Pop4, and Pop5 groups. Therefore, the genetic differentiation of D. sinensis population could be divided into two stages in the last 50 years. The Pop3, Pop4, and Pop5 groups were regarded as one branch, in which the evolutionary direction of the haplotypes was simpler. The Pop1 and Pop2 groups were regarded as the other branch, in which the evolutionary direction of the haplotypes was dispersed, and the two sub-centers of H-14 and H-6 were also observed.
Based on the mitochondrial COI gene sequences of the five historic D. sinensis groups, Fu’s FS neutral test (D = −16.744, p = 0.000) and Tajima’s test (D = −1.798, p < 0.05) indicated that the five historic D. sinensis groups deviated from neutral evolution, and bottleneck effect and population expansion had occurred in the evolution history of D. sinensis in Lake Chaohu.
Relationships between the genetic diversity of Daphnia sinensis and environmental factors in Lake Chaohu
Principal Component Analysis (PCA) showed distinct patterns in the evolution of the genetic diversity of 11 D. sinensis groups in Lake Chaohu, which could be well separated with time scale in the ordination space (Figure 6). For Figure 6A, the first axis (PCA1) was mainly described by the inbreeding coefficient (Fis) in May and June groups. Total phosphorus (TP) and dissolve total phosphorus (DTP) had significant effects on Fis index in May and June groups, as well as total nitrogen and water temperature. For Figure 6B, the second axis (PCA2) is mainly related to Fis of Pop3 (1999–2006) group in the sediments. Total phosphorus contents of the sediments had significant effects on Fis index in Pop3 (1999–2006) and Pop4 (1988–1999). However, total nitrogen contents were positively correlated with other genetic diversity indexes (I, h, Ne) in Pop2 (2006–2011) group in the sediment but negatively correlated with I, h and Ne of modern D. sinensis groups in lake water.
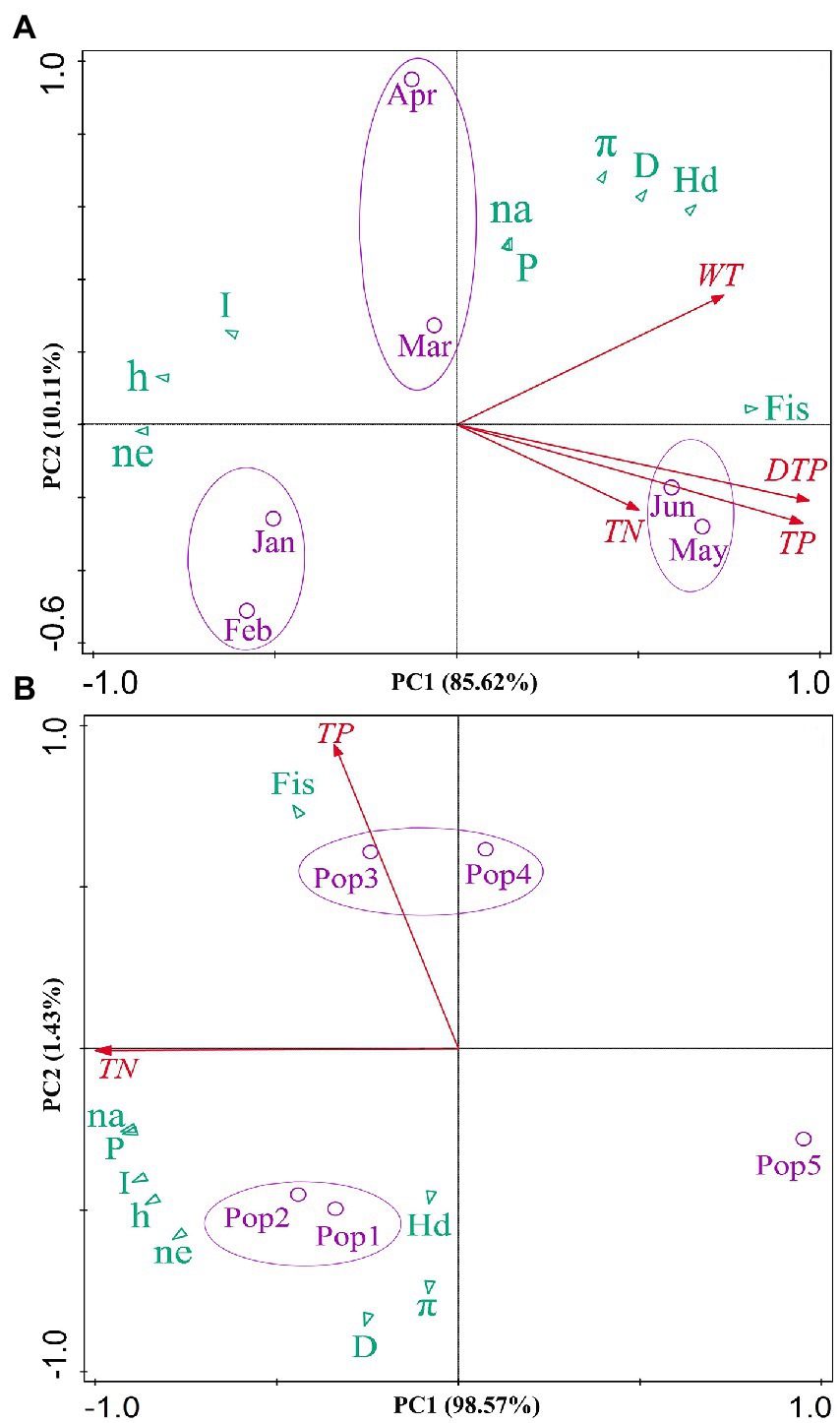
Figure 6. Principal Component Analysis (PCA) of six modern (A) and five historic (B) groups of D. sinensis in Lake Chaohu, showing the relationships between different genetic diversity indexes and environmental factors. Na stands for the observed number of alleles, Ne-the effective number of alleles, h-Nei’s gene diversity, I-Shannon’s diversity index, P-percentage of polymorphic loci, Fis-inbreeding coefficient, Hd-haplotype diversity, π-Nucleotide diversity, D-the genetic distance within groups.
Discussion
The phylogenetic evolution of Daphnia sinensis in Lake Chaohu
In this study, the population genetic variation of an aquatic organism (D. sinensis) from resting eggs deposited in the lake sediments were reconstructed. The resting egg bank typically could provide an accurate ecological and evolutionary representation of past populations (Monchamp et al., 2017). In Lake Chaohu, the density of Daphnia species was low in 1980–1981 (unpublished data), but it remained at higher levels in 2002–2003 (Deng et al., 2008) and in 2013 (Li et al., 2015). According to Zhang et al. (2020), the variations of the occurrence and densities of Daphnia resting eggs in the sediments were consistent with the densities in Lake Chaohu that were observed in the literatures (Ye, unpublished data; Deng et al., 2008; Li et al., 2015). Therefore, it was reliable and appropriate by employing resting eggs deposited in the sediments to study population genetic variation of Daphnia in Lake Chaohu.
Haplotype diversity (Hd) and nucleotide diversity (π) indexes could reveal different historical events, such as the founder effect, bottleneck effect and neutral evolution of organisms (Tajima, 1989; Grant and Bowen, 1998; Crandall et al., 2011). In natural population, higher Hd and lower π values indicated that the species could expand after lower population density (Crandall et al., 2011). Founder effect could lead to new population with the source population genetic differentiation and make new population genetic diversity is low. Bottlenecks effect occurred after the population decline, population genetic information were from a few survived completely. In Lake Chaohu, the density of Daphnia species was low in Pop5. The replenishment of aquatic population mainly came from the hatch of dormant eggs of sediments in January and February, with the increase of temperature, Daphnia population began to carry out parthenogenesis and rapid growth. In this study, higher Hd and lower π values implied that D. sinensis population experienced a bottleneck effect in the history process of Lake Chaohu. In Lake Chaohu, the density of Daphnia resting eggs was low in Pop5 (the lower layer of the sediment), and then increased obviously in the upper layers of the sediments. Moreover, the replenishment of Daphnia population mainly came from the hatching of resting eggs in the sediments of the lake in January and February. As the temperature rose, the Daphnia population began to expand rapidly by parthenogenesis. For the natural population, the genetic differentiation (Fst) value ranged between 0.05 and 0.15, indicating a medium differentiation level, and the Fst value were between 0.15 and 0.25, indicating a high differentiation level (Weight, 1978). In this study, the Fst among the six modern D. sinensis groups ranged from 0.048 to 0.113. The minimum Fst value (0.048) appeared between March and April groups, whereas the maximum (0.113) occurred between February and May groups. This seemed to be related to the increase of population density in D. sinensis. During the sampling period, the density of D. sinensis remained at lower levels in January and February, and the replenishment of population mainly depended on the hatching of resting eggs from the surface sediments. Under suitable conditions (e.g., higher water temperature), D. sinensis showed rapid population increase by cyclical parthenogenesis after March. Usually, some dormant eggs could be carried from the bottom layer to the surface layer by wind or benthic animals. This phenomenon might contribute to the population genetic evolution of D. sinensis in Lake Chaohu. However, the genetic differentiation of the five historic D. sinensis groups showed a wave-like pattern over time, yet their Fst were all <0.1, suggesting the adaptation of different D. sinensis groups to different environmental conditions occurred during population evolution in Lake Chaohu. Moreover, based on the COI gene sequences, the FST values of D. sinensis population ranged from 0.015 to 0.257 between Lake Chaohu and other lakes which were located in the middle and lower reaches of the Yangtze River (Wu et al., 2019b), indicating that D. sinensis had higher genetic differentiation in different lakes.
The primitive haplotypes were often distributed in the center of the evolutionary network and exhibited a broad expansion advantage, whereas new haplotypes were located at the end of the evolutionary network and had a narrow distribution (Palumbi, 2003). According to the mitochondrial COI gene sequences from the five historical D. sinensis groups, D. sinensis population originated from two distinct ancestral haplotypes over the history of Lake Chaohu.
Moreover, 13 haplotypes were found in the 4–6 cm sedimentary layer among which nine were specific (Pop2) (2006–2010). Based on the microsatellite markers, cluster 4 was mainly distributed in the 4–6 cm sedimentary layer (Pop2). These findings indicated that the genetic variation and microevolution of D. sinensis group (Pop2) were active and complicated between 2006 and 2010. It might result from a consequence of severe eutrophication in the 4–6 cm sedimentary layer (Pop2) in Lake Chaohu.
Effects of environmental changes on the genetic diversity and differentiation of Daphnia sinensis population in Lake Chaohu
Some mechanisms (e.g., phylogeographical structure, geographical isolation model, and climate change) have been used to explain how population structures of vertebrates evolve in an environment without any geographical boundaries (Gysels et al., 2004; Adams et al., 2006; Wilson, 2006). For some aquatic organisms in different environments, population genetic structure determined their ability to adapt to environmental pressure (Ma et al., 2016). Some investigations had shown that water temperature and nutrients could affect zooplankton community structure and life history strategy (DeMott et al., 2004; Sarma et al., 2005; Hsieh et al., 2011). Related literatures reported that the nutrient levels (especially nitrogen) had increased rapidly since the 1970s (Yao and Shen, 2003; Zhang and Kong, 2015), and reached the peak in Lake Chaohu in 1995 (Xie, 2009), which was highly consistent with the changing pattern of nitrogen contents in the sediments. Water eutrophication enhanced the change in phytoplankton density, biomass and structure (such as dominant cyanobacteria) of the lake (Deng et al., 2007). Moreover, long-term eutrophication and increasing edible phytoplankton biomass affected the density and structure of crustacean zooplankton in Lake Chaohu (Deng et al., 2008). Correspondingly, in Lake Chaohu, Daphnia density was few and found occasionally in 1980–1981 (Ye et al., unpublished data), and then obviously increased (31.8 ind./L and 29.7 ind./L in April and May, respectively) in 2002–2003 (Deng et al., 2008) and remained at a higher level (the maximum value of 172.9 ind./L at a sampling site in April) in 2013 (Li et al., 2015). In this study, the density of Daphnia species reached also116.5 ind./L at a sampling site in June. In addition, Daphnia dominated in spring, had higher density in early summer, and then disappeared in mid-summer in Lake Chaohu (Deng et al., 2008; Li et al., 2015). Therefore, environmental changes (including water temperature, phytoplankton biomass and structure and fish predation) determined Daphnia population size, and then might accelerate Daphnia gene exchange and affect Daphnia population genetic structure. Hairston et al. (1999, 2001) also confirmed that Daphnia could evolve to tolerate toxic cyanobacteria in the diet in response to nutrient enrichment in freshwater lakes. Therefore, understanding the drivers and consequences of Daphnia traits, such as co-evolutionary dynamics with cyanobacteria, was very important for future studies of eutrophic lakes (Ger et al., 2016).
During the seasonal sampling period, our results showed that DTP concentration was negatively correlated with Nei’s gene diversity (h) and effective number of alleles (Ne), and had significant effects on Fis index in May and June groups. Water temperature had negative correlations with Nei’s gene diversity (h) and observed number of alleles (Na) whereas it was positively related to haplotype diversity (Hd). Therefore, water temperature and P level played important roles in the short-term microevolution of D. sinensis population in Lake Chaohu. DeMott et al. (2004) found that the differences of P-content in natural lakes affected the seasonal distribution of Daphnia species. Frisch et al. (2017) also observed that the increasing phosphorus contents of the sediments strongly affected the population genetic structure of Daphnia pulicaria under the anthropogenic environmental change in South Center Lake of North America, and thought that ancient clones with limited phosphorus supplied more adaptive to low phosphorus diet whereas the phenotype plasticity of modern clones to phosphorus change enhanced (such as higher growth efficiency under high phosphorus condition and lower growth efficiency under low phosphorus condition). Through conducting an in-lake mesocosm experiment, Chislock et al. (2019) also confirmed that D. pulicaria could rapidly evolve to adapt to eutrophication and grazing-resistant cyanobacteria stresses. In a Europen lake, Lake Constance, the changes in P-levels seemed to facilitate the coexistence of Daphnia species and interspecific hybridization, causing lasting changes in the genotype architecture of Daphnia (Brede et al., 2009).
However, both N and P nutrients were related to the short-term and long-term changes of genetic diversity and microevolution of D. sinensis in Lake Chaohu. This phenomenon might result from the unique cause of eutrophication and the geological structure of the lake. Phosphate rocks were abundant in the north shore of Lake Chaohu, and the exploitation of phosphate rock had led to a long-term phosphorus-rich state in the lake since the 1960s (Xie, 2009). In this study, the fluctuation of TP contents in the sediments of Lake Chaohu was relatively small (0.26–0.41 g/kg), but the TN contents have gradually increased since the 1970s (0.28–1.18 g/kg). The genetic diversity indexes (h, Na, Ne, P and I) of the five historic D. sinensis groups in the sediments had significantly correlated with TN contents but not with TP contents. Therefore, phosphorus played an important role in the short-term (seasonal) genetic differentiation of D. sinensis population in Lake Chaohu, whereas nitrogen could promote the long-term microevolution of D. sinensis population in the stable high-phosphorus environment (about 50 years), which was inconsistent with the results in other lakes that phosphorus concentration increased rapidly during the lake eutrophication (Brede et al., 2009; Frisch et al., 2014). Rellstab et al. (2011) also verified that the change of Daphnia population structure in the pre-alpine lakes of Central Europe was the result of anthropocentric eutrophication, it depended not only on the general nutritional level of the lake but also the range of nutritional increase. Usually, higher nitrogen level could promote the growth and development of small-sized edible phytoplankton (such as Tetradesmus) and larger-sized phytoplankton (especially cyanobacteria such as Microcystis), and then favor the growth and reproduction of crustacean zooplankton (Sommer et al., 1986; Deng et al., 2008). Daphnia which were phosphorus-limited could rapidly increase population size in higher phosphorus environment and then accelerate their microevolution. Therefore, the changing pattern of D. sinensis population genetic structure in Lake Chaohu was one of the environmental selections probably combined with co-evolutionary, where the rapid-increasing nitrogen levels had a large impact on D. sinensis genetic structure in a lasting high phosphorus environment. Previous investigation indicated also that environmental selection directly impacted genetic variation at loci of Daphnia magna from nineteen shallow ponds in Belgium (Orsini et al., 2013b).
Conclusion
The genetic diversity of the D. sinensis population in Lake Chaohu varied over time. During seasonal environmental variations and lake eutrophication, the population genetic structure of D. sinensis was experienced adaptive microevolution. The genetic structure of the six modern D. sinensis groups had two dominant clusters (the WG cluster and SG cluster) during the seasonal dynamics. Moreover, the five historic D. sinensis groups had two different evolutionary centers of haplotypes in the sediments of Lake Chaohu, which seemed to be related to a rapid increase of total nitrogen contents. Based on the microsatellite markers, the genetic structure network showed that D. sinensis population had evolved the four dominant clusters, and the population differentiation experienced a time-dependent genetic isolation in the sediments of Lake Chaohu.
The phylogenetics of D. sinensis population was related to the accumulation of N and P nutrients in Lake Chaohu, but lake eutrophication exhibited a hysteresis effect on the genetic differentiation of D. sinensis population. Water temperature and dissolved total phosphorus were associated with the short-term genetic differentiation of D. sinensis population in Lake Chaohu, whereas total nitrogen content was significantly related to the microevolution of D. sinensis population in the long-term high phosphorus environment. Therefore, the changing pattern of D. sinensis population genetic structure in Lake Chaohu was one of the environmental selections probably combined with co-evolutionary, where the rapid-increasing nitrogen level had a large impact on D. sinensis population genetic structure in a lasting high phosphorus environment.
Data availability statement
The original contributions presented in the study are included in the article/Supplementary material, further inquiries can be directed to the corresponding author.
Author contributions
KZ and JW: formal analysis, investigation, data curation, and writing original draft. DD: resources, methodology, software, writing review and editing, project administration, supervision, and funding. HZ, QL, and SP: data collection. YZ: software and validation. ZZ: review and editing. All authors contributed critically to the drafts and gave final approval for publication.
Funding
This study was financially supported by the National Natural Science Foundation of China (nos. 31870451, 32001155, and 31370470) and by the Open Project of Anhui Province Key Laboratory of Wetland Ecosystem Protection and Restoration, Anhui University, no. AKLWEPR-K-2020-01.
Acknowledgments
We thank Wenping Wang, Xiaoxue Xu and Ping He for their assistance in our work. We thank the reviewers provided constructive recommendations for improving our manuscript.
Conflict of interest
The authors declare that the research was conducted in the absence of any commercial or financial relationships that could be construed as a potential conflict of interest.
Publisher’s note
All claims expressed in this article are solely those of the authors and do not necessarily represent those of their affiliated organizations, or those of the publisher, the editors and the reviewers. Any product that may be evaluated in this article, or claim that may be made by its manufacturer, is not guaranteed or endorsed by the publisher.
Supplementary material
The Supplementary material for this article can be found online at: https://www.frontiersin.org/articles/10.3389/fevo.2022.913738/full#supplementary-material
References
Adams, S. M., Lindmeier, J. B., and Duvernell, D. D. (2006). Microsatellite analysis of the phylogeography, Pleistocene history and secondary contact hypotheses for the killifish, Fundulus heteroclitus. Mol. Ecol. 15, 1109–1123. doi: 10.1111/j.1365-294X.2006.02859.x
Benzie, J. A. H. (2005). The Genus daphnia (including Daphniopsis) (Anomopoda: Daphniidae). Leiden (Netherlands) and Ghent (Belgium): Backhuys Publishers and Kenobi Productions.
Brede, N., Sandrock, C., Straile, D., Spaak, P., Jankowski, T., Streit, B., et al. (2009). The impact of human-made ecological changes on the genetic architecture of daphnia species. Proc. Natl. Acad. Sci. U. S. A. 106, 4758–4763. doi: 10.1073/pnas.0807187106
Chen, C. H., Zhao, L. Y., Zhu, C., Wang, J. Z., Jiang, J. H., and Yang, S. (2014). Response of diatom community in Lugu Lake (Yunnan-Guizhou Plateau, China) to climate change over the past century. J. Paleolimnol. 51, 357–373. doi: 10.1007/s10933-013-9760-4
Chislock, M. F., Kaul, R. B., Durham, K. A., Sarnelle, O., and Wilson, A. E. (2019). Eutrophication mediates rapid clonal evolution in Daphnia pulicaria. Freshw. Biol. 64, 1275–1283. doi: 10.1111/fwb.13303
Cottenie, K., Nuytten, N., Michels, E., and Meester, L. D. (2001). Zooplankton community structure and environmental conditions in a set of interconnected ponds. Hydrobiologia 443, 339–350. doi: 10.1023/A:1017505619088
Crandall, E. D., Sbrocco, E. J., Deboer, T. S., Barber, P. H., and Carpenter, K. E. (2011). Expansion dating: calibrating molecular clocks in marine species from expansions onto the Sunda shelf following the last glacial maximum. Mol. Biol. Evol. 29, 707–719. doi: 10.1093/molbev/msr227
De Gelas, K., and De Meester, L. (2005). Phylogeography of Daphnia magna in Europe. Mol. Ecol. 14, 753–764. doi: 10.1111/j.1365-294X.2004.02434.x
Decaestecker, E., Gaba, S., Raeymaekers, J., Stoks, R., Van Kerckhoven, L., and Ebert, D. (2007). Host-parasite red queen dynamics archived in pond sediment. Nature 450, 870–873. doi: 10.1038/nature06291
DeMott, W. R., Pape, B. J., and Tessier, A. J. (2004). Patterns and sources of variation in daphnia phosphorus content in nature. Aquat. Ecol. 38, 433–440. doi: 10.1023/B:AECO.0000035183.53389.66
Deng, D. G., Xie, P., Zhou, Q., Yang, H., and Guo, L. G. (2007). Studies on temporal and spatial variations of phytoplankton in Lake Chaohu. J. Integr. Plant Biol. 49, 409–418. doi: 10.1111/j.1672-9072.2006.00390.x
Deng, D. G., Xie, P., Zhou, Q., Yang, H., Guo, L. G., and Geng, H. (2008). Field and experimental studies on the combined impacts of cyanobacterial blooms and small algae on crustacean zooplankton in a large, eutrophic, subtropical, Chinese lake. Limnology 9, 1–11. doi: 10.1007/s10201-007-0229-x
Ebina, J., Tsutsui, T., and Shirai, T. (1983). Simultaneous determination of total nitrogen and total phosphorus in water using peroxodisulfate oxidation. Water Resour. 17, 1721–1726. doi: 10.1016/0043-1354(83)90192-6
Elser, J. J., Elser, M. M., MacKay, N. A., and Carpenter, S. R. (1988). Zooplankton-mediated transitions between N- and P-limited algal growth. Limnol. Oceanogr. 33, 1–14. doi: 10.4319/lo.1988.33.1.0001
Evanno, G. S., Regnaut, S. J., and Goudet, J. (2005). Detecting the number of clusters of individuals using the software STRUCTURE: a simulation study. Mol. Ecol. 14, 2611–2620. doi: 10.1111/j.1365-294X.2005.02553.x
Excoffier, L., and Lischer, H. E. L. (2010). Arlequin suite ver 3.5: a new series of programs to perform population genetics analyses under Linux and windows. Mol. Ecol. Resour. 10, 564–567. doi: 10.1111/j.1755-0998.2010.02847.x
Frisch, D., Morton, P. K., Chowdhury, P. R., Culver, B. W., Colbourne, J. K., Weider, L. J., et al. (2014). A millennial-scale chronicle of evolutionary responses to cultural eutrophication in daphnia. Ecol. Lett. 17, 360–368. doi: 10.1111/ele.12237
Frisch, D., Morton, P. K., Culver, B. W., Edlund, M. B., Jeyasingh, P. D., and Weider, L. J. (2017). Paleogenetic records of Daphnia pulicaria in two north American lakes reveal the impact of cultural eutrophication. Glob. Chang. Biol. 23, 708–718. doi: 10.1111/gcb.13445
Fu, Y. X. (1997). Statistical tests of neutrality of mutations against population growth, hitchhiking and background selection. Genetics 147, 915–925. doi: 10.1093/genetics/147.2.915
Ger, K. A., Urrutia-Cordero, P., Frost, P. C., Hansson, L. A., Sarnelle, O., Wilson, A. E., et al. (2016). The interaction between cyanobacteria and zooplankton in a more eutrophic world. Harmful Algae 54, 128–144. doi: 10.1016/j.hal.2015.12.005
Grant, W., and Bowen, B. (1998). Shallow population histories in deep evolutionary lineages of marine fishes: insights from sardines and anchovies and lessons for conservation. J. Hered. 89, 415–426. doi: 10.1093/jhered/89.5.415
Gysels, E. S., Hellemans, B., Pampoulie, C., and Volckaert, F. A. M. (2004). Phylogeography of the common goby, Pomatoschistus microps, with particular emphasis on the colonization of the Mediterranean and the North Sea. Mol. Ecol. 13, 403–417. doi: 10.1046/j.1365-294X.2003.02087.x
Hairston, N. G., and Cáceres, C. E. (1996). Distribution of crustacean diapause: micro- and macroevolutionary pattern and process. Hydrobiologia 320, 27–44. doi: 10.1007/BF00016802
Hairston, N. G., Holtmeier, C. L., Lampert, W., Weider, L. J., Post, D. M., Fischer, J. M., et al. (2001). Natural selection for grazer resistance to toxic cyanobacteria: evolution of phenotypic plasticity? Evolution 55, 2203–2214. doi: 10.1111/j.0014-3820.2001.tb00736.x
Hairston, N. G., Lampert, W., Cáceres, C. E., Holtmeier, C. L., Weider, L. J., Gaedke, U., et al. (1999). Lake ecosystems – rapid evolution revealed by dormant eggs. Nature 401:446. doi: 10.1038/46731
Hsieh, C. H., Sakai, Y., Ban, S., and Ishikawa, K. (2011). Eutrophication and warming effects on long-term variation of zooplankton in Lake Biwa. Biogeosciences 8, 1383–1399. doi: 10.5194/bg-8-1383-2011
Huang, X. F. (1999). Survey, Observation and Analysis of Lake Ecology. Beijing: Science Press. (in Chinese).
Jeyasingh, P. D., and Weider, L. J. (2007). Fundamental links between genes and elements: evolutionary implications of ecological stoichiometry. Mol. Ecol. 16, 4649–4661. doi: 10.1111/j.1365-294X.2007.03558.x
Jiang, X. Z., and Du, N. S. (1979). Fauna Sinica: Crustacean, Freshwater Cladocera. Beijing: Science Press. (in Chinese).
Kotov, A. A., Garibian, P. G., Bekker, E. I., Taylor, D. J., and Karabanov, D. P. (2021). A new species group from the Daphnia curvirostris species complex (Cladocera: Anomopoda) from the eastern palaearctic: taxonomy, phylogeny and phylogeography. Zool. J. Linnean Soc. 191, 772–822. doi: 10.1093/zoolinnean/zlaa046
Li, J., Cui, K., Lu, W. X., Cheng, Y. S., and Jiang, Y. Y. (2015). Community dynamics of spring-summer plankton in Lake Chaohu. Acta Hydrobio. Sin. 39, 185–192. doi: 10.7541/2015.23 (in Chinese)
Li, J., Liu, C. Q., Wang, S. L., Zhou, Z. H., Zhu, Z. Z., and Xiao, H. Y. (2005). Seasonal variations in composition and distribution of dissolved nutrients in the water column of Taihu Lake. China. Geol. Geochem. 47, 1459–1469. doi: 10.1111/j.1744-7909.2005.00184.x (in Chinese)
Librado, P., and Rozas, J. (2009). DnaSP v5: a software for comprehensive analysis of DNA polymorphism data. Bioinformatics 25, 1451–1452. doi: 10.1093/bioinformatics/btp187
Løvik, J. E., and Kjelliberg, G. (2003). Long-term changes of the crustacean zooplankton community in Lake Mjøsa, the largest lake in Norway. J. Limnol. 62, 143–150. doi: 10.4081/jlimnol.2003.143
Ma, K. Y., Craig, M. T., Choat, J. H., and Herwerden, L. V. (2016). The historical biogeography of groupers: clade diversification patterns and processes. Mol. Phylogenet. Evol. 100, 21–30. doi: 10.1016/j.ympev.2016.02.012
Ma, X., Ni, Y. J., Wang, X. Y., Hu, W., and Yin, M. B. (2020). Lineage diversity, morphological and genetic divergence in Daphnia magna (Crustacea) among Chinese lakes at different altitudes. Contrib. Zool. 89, 450–470. doi: 10.1163/18759866-bja10011
Monchamp, M. E., Enache, I., Turko, P., Pomati, F., Rîşnoveanu, G., and Spaak, P. (2017). Sedimentary and egg-bank DNA from 3 European lakes reveal concurrent changes in the composition and diversity of cyanobacterial and daphnia communities. Hydrobiologia 800, 155–172. doi: 10.1007/s10750-017-3247-7
Möst, M., Oexle, S., Markova, S., Aidukaite, D., Baumgartner, L., Stich, H. B., et al. (2015). Population genetic dynamics of an invasion reconstructed from the sediment egg bank. Mol. Ecol. 24, 4074–4093. doi: 10.1111/mec.13298
Orsini, L., Mergeay, J., Vanoverbeke, J., and De Meester, L. (2013b). The role of selection in driving landscape genomic structure of the waterflea Daphnia magna. Mol. Ecol. 22, 583–601. doi: 10.1111/mec.12117
Orsini, L., Schwenk, K., De Meester, L., Colbourne, J. K., Pfrender, M. E., and Weider, L. J. (2013a). The evolutionary time machine: using dormant propagules to forecast how populations can adapt to changing environments. Trends Ecol. Evol. 28, 274–282. doi: 10.1016/j.tree.2013.01.009
Palumbi, S. R. (2003). Population genetics, demographic connectivity, and the design of marine reserves. Ecol. Appl. 13, 146–158. doi: 10.1890/1051-0761(2003)013[0146,PGDCAT]2.0.CO;2
Pritchard, J. K., Stephens, M., and Donnelly, P. (2000). Inference of population structure using multilocus genotype data. Genetics 155, 945–959. doi: 10.1093/genetics/155.2.945
Qian, J. L., Zhang, L. D., and Le, M. L. (1990). Determination of total nitrogen and total phosphorus in soil by persulfate digestion. Soil 22, 258–262. (in Chinese)
Rellstab, C., Keller, B., Girardclos, S., Anselmetti, F. S., and Spaak, P. (2011). Anthropogenic eutrophication shapes the past and present taxonomic composition of hybridizing daphnia in unproductive lakes. Limnol. Oceanogr. 56, 292–302. doi: 10.4319/lo.2011.56.1.0292
Roff, J. C., Sprules, W. G., Carter, J. C. H., and Dadswell, M. J. (1981). The structure of crustacean zooplankton communities in glaciated eastern North America. Can. J. Fish. Aquat. Sci. 38, 1428–1437. doi: 10.1139/f81-189
Sarma, S. S. S., Nandini, S., and Gulati, R. D. (2005). Life history strategies of cladocerans: comparisons of tropical and temperate taxa. Hydrobiologia 542, 315–333. doi: 10.1007/s10750-004-3247-2
So, M., Ohtsuki, H., Makino, W., Ishida, S., Kumagai, H., Yamaki, K. G., et al. (2015). Invasion and molecular evolution of Daphnia pulex in Japan. Limnol. Oceanogr. 60, 1129–1138. doi: 10.1002/lno.10087
Sommer, U., Gliwicz, Z. M., Lampert, W., and Duncan, A. (1986). The PEG-model of seasonal succession of planktonic events in fresh waters. Arch. Hydrobiol. 106, 433–471.
Sterner, R. W., and Elser, J. J., (2002). Ecological Stoichiometry: The Biology of Elements From Molecules to the Biosphere. Princeton University Press. Princeton, NJ.
Stige, L. C., Lajus, D. L., Chan, K. S., Dalpadado, P., Basedow, S. L., Berchenko, I., et al. (2009). Climatic forcing of zooplankton dynamics is stronger during low densities of planktivorous fish. Limnol. Oceanogr. 54, 1025–1036. doi: 10.4319/lo.2009.54.4.1025
Straile, D., and Geller, W. (1998). Crustacean zooplankton in Lake Constance from 1920 to 1995: response to eutrophication and re-oligotrophication. Advanc. Limnol. 53, 255–274. Available at: http://nbn-resolving.de/urn:nbn:de:bsz:352-opus-39858
Tajima, F. (1989). Statistical method for testing the neutral mutation hypothesis by DNA polymorphism. Genetics 123, 585–595. doi: 10.1093/genetics/123.3.585
Tamura, K., Dudley, J., Nei, M., and Kumar, S. (2007). MEGA4: molecular evolutionary genetics analysis (MEGA) software version 4.0. Mol. Biol. Evol. 24, 1596–1599. doi: 10.1093/molbev/msm092
Weight, S. (1978). Evolution and the Genetics of Population Variability within and Among Natural Population. Chicago: University of Chicago Press.
Wellborn, B. A., Skelly, D. K., and Werner, E. E. (1996). Mechanisms creating community structure across a fresh water habitat gradient. Annu. Rev. Ecol. Syst. 27, 337–363. doi: 10.1146/annurev.ecolsys.27.1.337
Wilson, A. B. (2006). Genetic signature of recent glaciation on populations of a near-shore marine fish species (Syngnathus leptorhynchus). Mol. Ecol. 15, 1857–1871. doi: 10.1111/j.1365-294X.2006.02911.x
Wu, J. X., Wang, W. P., Deng, D. G., Zhang, K., Peng, S. X., Xu, X. X., et al. (2019b). Genetic diversity and phylogeography of Daphnia similoides sinensis located in the middle and lower reaches of the Yangtze River. Ecol. Evol. 9, 4362–4372. doi: 10.1002/ece3.4880
Wu, J. X., Zhang, Y. N., Deng, D. G., Xu, X. X., and Zhou, Z. Z. (2019a). Analysis on microsatellite loci of Daphnia similoides sinensis and rapid development of primer based on transcriptome sequencing. Russ. J. Genet. 55, 711–719. doi: 10.1134/S1022795419060188
Xie, P., (2009). Reading About the Histories of Cyanobacteria, Eutrophication and Geological Evolution in Lake Chaohu. Beijing: Science China Press.
Yao, S. C., and Shen, J. (2003). A preliminary study of N-alkanes in a sedimentary core from Lake Chaohu. J. Lake Sci. 15, 200–204. doi: 10.18307/2003.0302 (in Chinese).
Yeh, F. C., Yang, R. C., and Boyle, T., (1999). Popgene version 1.31. Microsoft Window-Based Freeware for Population Genetic Analysis. University of Alberta and the Centre for International Forestry Research. Available at: www.ualberta.ca/wfyeh/
Zhang, K., Deng, D. G., Wang, W. P., Peng, S. X., Liu, F., and Ji, L. (2020). Long-term eutrophication affects vertical changes of Daphnia ephippia in the sediments of a subtropical Chinese lake. Environ. Sci. Pollut. R. 27, 4737–4745. doi: 10.1007/s11356-019-07157-9
Zhang, X. L., Deng, D. G., Zhang, K., Wang, W. P., and Ji, L. (2015). Combined effects of colonial size and concentration of Microcystis aeruginosa on the life history traits of Daphnia similoides. Acta Biol. Hung. 66, 282–292. doi: 10.1556/018.66.2015.3.4
Keywords: Daphnia sinensis, eutrophication, genetic differentiation, resting eggs, sediment
Citation: Zhang K, Wu J, Deng D, Zhao H, Liu Q, Peng S, Zhang Y and Zhou Z (2023) Population genetic differentiation of Daphnia sinensis in a lasting high-phosphorus Chinese lake, Lake Chaohu. Front. Ecol. Evol. 10:913738. doi: 10.3389/fevo.2022.913738
Edited by:
Mohd Hafiz Mohd, Universiti Sains Malaysia (USM), MalaysiaReviewed by:
Dmitry Yurievich Sherbakov, Limnological Institute (RAS), RussiaTuty Arisuryanti, Gadjah Mada University, Indonesia
Copyright © 2023 Zhang, Wu, Deng, Zhao, Liu, Peng, Zhang and Zhou. This is an open-access article distributed under the terms of the Creative Commons Attribution License (CC BY). The use, distribution or reproduction in other forums is permitted, provided the original author(s) and the copyright owner(s) are credited and that the original publication in this journal is cited, in accordance with accepted academic practice. No use, distribution or reproduction is permitted which does not comply with these terms.
*Correspondence: Daogui Deng, dengdg@263.net
†These authors have contributed equally to this work